- 1Agriculture-Environment-Biology Group, College of Animal Sciences, Zhejiang University, Hangzhou, China
- 2Zhejiang Mariculture Research Institute, Wenzhou, China
An enormous amount of anthropogenic carbon dioxide (CO2) has been dissolved into the ocean, leading to a lower pH and changes in the chemical properties of seawater, which has been termed ocean acidification (OA). The impacts of pCO2-driven acidification on immunity have been revealed recently in various marine organisms. However, the mechanism causing the reduction in phagocytosis still remains unclear. Therefore, the impacts of pCO2-driven OA at present and near-future levels (pH values of 8.1, 7.8, and 7.4) on the rate of phagocytosis, the abundance of cytoskeleton components, the levels of nitric oxide (NO), and the concentration and activity of lysozymes (LZM) of hemocytes were investigated in a commercial bivalve species, the blood clam (Tegillarca granosa). In addition, the effects of OA on the expression of genes regulating actin skeleton and nitric oxide synthesis 2 (NOS2) were also analyzed. The results obtained showed that the phagocytic rate, cytoskeleton component abundance, concentration and activity of LZM of hemocytes were all significantly reduced after a 2-week exposure to the future OA scenario of a pH of 7.4. On the contrary, a remarkable increase in the concentration of NO compared to that of the control was detected in clams exposed to OA. Furthermore, the expression of genes regulating the actin cytoskeleton and NOS were significantly up-regulated after OA exposure. Though the mechanism causing phagocytosis seemed to be complicated based on the results obtained in the present study and those reported previously, our results suggested that OA may reduce the phagocytosis of hemocytes by (1) decreasing the abundance of cytoskeleton components and therefore hampering the cytoskeleton-mediated process of engulfment, (2) reducing the concentration and activity of LZM and therefore constraining the degradation of the engulfed pathogen through an oxygen-independent pathway, and (3) inducing the production of NO, which may negatively regulate immune responses.
Introduction
Ocean acidification (OA) has become a global environmental issue under the spotlight for the last decade (Kelly et al., 2011). Tremendous anthropogenic CO2 has been emitted since the Industrial Revolution, which has resulted in the rise of atmospheric CO2 levels from 280 to 390 μatm (Orr et al., 2005). Approximately 1/4 to 1/3 of emitted CO2 was absorbed by the ocean, which increases the concentration of H+ and alters the equilibrium of the oceanic carbonate system in surface seawater (Caldeira and Wickett, 2003; Sabine et al., 2004; Raven et al., 2005). The pH of surface seawater has decreased by 0.1 units compared to the pre-industrial level, and according to the prediction of the Intergovernmental Panel on Climate Change (IPCC), it may decrease to 7.8 and 7.4 by the end of the 21st century and the year 2300, respectively (Caldeira and Wickett, 2003). OA may therefore exert significant impacts on various aspects of organisms inhabiting the sea, such as growth (Wu et al., 2014), metabolism (Lannig et al., 2010), immune response (Liu et al., 2016), and sensory ability (Ashur et al., 2017), which on a large scale may pose a great threat to the entire marine ecosystem (Zhang et al., 2012).
The phylum Mollusca is one of the largest and most important groups in the animal kingdom, with approximately 130,000 living species (Parkhaev, 2017). As a category of invertebrates, mollusks mainly rely on non-specific immunity against pathogen challenges (Song et al., 2010). Nevertheless, recent studies have shown that OA could hamper the immune responses of various marine bivalve species, such as Crassostrea gigas (Wang et al., 2016), Mercenaria mercenaria (Ivanina et al., 2016), and Mytilus edulis (Mackenzie et al., 2014). Among all the parameters evaluating immune responses, the phagocytosis of hemocytes, one of the most important defense measures in non-specific immunity (Wang et al., 2012), including the engulfment and degradation of pathogen particles, has been widely used in previous studies (Liu et al., 2016; Shi et al., 2017; Su et al., 2017). Though an OA-induced reduction in phagocytosis has been reported in bivalve species, the mechanism manifesting this impact still remains unclear (Canesi et al., 2002; Sokolnikova et al., 2015).
Phagocytosis includes a series of sequential processes, such as the attachment of hemocytes to the targeted particles, the recognition of pathogen particles by pattern recognition receptors (PRRs), the engulfment facilitated by the cytoskeleton, and the degradation of foreign particles through oxygen-dependent and oxygen-independent pathways (Song et al., 2010). It has been suggested that OA could hamper the recognition of pathogen particles by hemocytes of marine invertebrates by affecting PRRs (Liu et al., 2016; Castillo et al., 2017). In addition, previous studies have shown that OA may lead to an increase in the production of reactive oxygen species (ROS), which is crucial for the oxygen-dependent degradation of engulfed pathogen particles (Duprécrochet et al., 2013; Wang et al., 2016). All of these previous studies contribute significantly to a better understanding of the potential mechanism underlying the OA-induced reduction in phagocytosis. However, the present knowledge of the impacts of OA on the processes of cytoskeleton-mediated engulfment and lysozyme-facilitated oxygen-independent degradation still remains scarce.
The engulfment of pathogen particles is facilitated by the ROS-sensitive cytoskeleton, mainly via the actin–myosin contractile system (Allen and Aderem, 1995). Therefore, theoretically, an increase in OA-induced ROS may exert a significant impact on the engulfment process by affecting the cytoskeleton of hemocytes (Tomanek et al., 2011; Goncalves et al., 2017). After engulfment, the phagosomes and lysosomes fuse together, and the engulfed target is destroyed within phagosomes mainly by lysozymes (the oxygen-independent degradation) or ROS (the oxygen-dependent degradation) (Buggé et al., 2007). However, since opposite results were reported in different species, the impact of OA on the lysozyme concentration still remains controversial (Munari, 2014; Bresolin et al., 2016). In addition, nitric oxide (NO) plays versatile roles in immune responses such as phagocytosis in which NO not only functions as an active antimicrobial molecule in vivo but also has been recognized as one of the most important immune regulators (Bogdan, 2001). Nevertheless, little is known about the effects of OA on the production of NO in hemocytes of marine bivalves to date.
The blood clam, Tegillarca granosa, is a traditional commercial marine bivalve species that is naturally distributed from the coasts of South Africa to the Asia Pacific (Shao et al., 2009; Peng et al., 2015). The blood clam occupies an important ecological niche in the intertidal zone and is therefore crucial for the material and energy flows of intertidal ecosystems (Liu et al., 2014). In addition, inhabiting in the intertidal zone where surface runoff converges into the sea, the blood clam is often challenged by various pollutants and deemed as an ideal marine bivalve species with which to investigate the impacts of environmental stresses on immune responses (Zhu et al., 2011; Liu et al., 2016; Shi et al., 2017; Su et al., 2017).
Therefore, in order to obtain a better understanding of the impact and mechanism of the effect of OA on the process of phagocytosis, the rate of phagocytosis, the abundance of the cytoskeleton component, the levels of NO, and the concentration and activity of lysozymes (LZM) of hemocytes under present and future OA scenarios were investigated in the blood clam, T. granosa. In addition, the impacts of OA on the expression of key genes regulating the actin skeleton and nitric oxide synthesis 2 (NOS2) were also analyzed in the present study.
Materials and Methods
Experimental Animals and Acclimation
Adult individuals of T. granosa with a shell length of 28.53 ± 0.52 mm were collected from Qingjiang, Wenzhou, and Zhejiang Provinces of China (28°28′ N and 121°11′ E) in July 2017. Before the commencement of the experiment, individuals were acclimated for a week in a 1000-L tank filled with 800 L of sand-filtered 24-h-aerated seawater (temperature at 22.18 ± 1.30°C, pH at 8.11 ± 0.02, and salinity at 20.67 ± 0.09). The seawater in the tank was replaced once daily 2 h after the individuals were fed with the microalgae Platymonas subcordiformis (Han et al., 2016).
Ocean Acidification Stimulation and Seawater Chemistry Analysis
According to the near-future OA scenarios predicted by the IPCC, pH levels of 8.1, 7.8, and 7.4 were employed to simulate the pH levels at present and in the years 2100 and 2300, respectively. According to the method of Zhao et al. (2017a), the stimulation of the acidified scenario was achieved by bubbling dry air or a mixture of carbon dioxide and dry air with different but constant percentages.
After 1 week of acclimation, blood clams with similar sizes were selected out from the acclimating tank and allocated into separated experimental tanks filled with 30 L of seawater at desired experimental pH. Thirty individuals were randomly allocated to each of the three pH levels, and three replicates were performed for each pH tested. During the 2-week exposure, blood clams were fed with the microalgae P. subcordiformis 2 h before the replacement of seawater everyday. The seawater of each trial was replaced with freshly sand-filtered seawater or pre-acidified seawater once daily.
To ensure consistent seawater conditions throughout the experimental progression in each trial, pHNBS, salinity and temperature were monitored daily, and the total alkalinity (TA) was monitored once a week (Table 1). The pHNBS of each level was measured by a pH meter (PB-10, Sartorius) calibrated with NBS standard buffers. Salinity was determined using a conductivity meter (Multi 3410 WTW, Germany). The water temperature was measured with a mercury thermometer. TA was obtained by a potentio-metric titration (Anderson and Robinson, 1946). The carbonate system parameters were calculated from the measured pHNBS, salinity, temperature, and TA values using the open-source program CO2SYS (Pierrot et al., 2006), with the constants supplied by Mehrbach et al. (1973) and refitted by Dickson and Millero (1987), along with the K2SO4 dissociation constant from Dixson et al. (2010).
Phagocytosis Assays
Following the methods described by Evelyne et al. (1991) and Shi et al. (2017), after a 2-week experimental treatment, three individuals were randomly picked from each trial for the phagocytosis assays. A yeast (Instant dry yeast, AngelYeast) suspension containing (1.45 ± 0.05) × 108 yeast cells per ml was prepared by dissolving 7 mg of yeast powder in 1000 μl of Alsever’s solution (ALS, Noble Ryder) and supersonic vibration was used for a sufficient homogenized mixing. A volume of about 150 μl of hemolymph was extracted from each individual followed by a quick determination of hemocyte concentration with 20 μl hemolymph using a Neubauer’s hemocytometer (XB-K-25, Anxin Optical Instrument) under Nikon eclipse E600 microscopy at the magnification of 400×. Meantime, 100 μl of the hemolymph extracted was transferred into a 1.5-ml centrifuge tube, which was pre-filled with 100 μl of Alsever’s solution. After spinning at 1000 rpm for 15 s, 100 μl of supernatant was removed and a calculated volume of yeast suspension was added at the yeast-hemocyte rate of 10:1 (Cima et al., 2000). The yeast-hemocyte mixture was incubated at room temperature (25°C) for 30 min and then fixed with 100 μl of 2.5% glutaraldehyde. Blood smears were subsequently prepared and stained with Wright-Giemsa stain (G1020, Solarbio). The phagocytic rate for each individual was estimated using the blood smear under a Nikon eclipse E600 microscope at the magnification of 400×.
Fluorescence Staining of the Cytoskeleton of Hemocytes
After a 2-week exposure to acidified seawater, three individuals from each pH level were selected for fluorescence staining of F-actin using Rhodamine Phalloidin (amanita phalloides, Cytoskeleton, Inc., #PHDR1) following the provided protocol and the method described by Kryukova et al. (2015). Briefly, the hemocytes were obtained as described above and then washed with PBS, followed by fixation with glutaraldehyde for 10 min at room temperature. After another 30-s PBS wash, the hemocytes were incubated in 0.1% Triton X-100 for 5 min. The blood cells were then washed again for 30 s with PBS and stained with 100 nM Rhodamine Phalloidin for 30 min in darkness. After three PBS washes, the hemocytes were collected and used to make slides for fluorescent analysis. Following the method described by Catapane et al. (2016) and Guo et al. (2017), the fluorescence intensity was determined under a Nikon Eclipse E600 microscope (at excitation and emission wavelengths of 535 and 585 nm, respectively) and then was used as an indicator for the abundance of cytoskeleton components. In brief, the fluorescence intensities of images captured were calculated and analyzed in Image Pro-Plus (IPP), using the equation: F = IOD/area, where F is the fluorescence intensity of the F-actin stained, integrated optical density (IOD) represents the total optical density value of the stained hemocyte area, and ‘area’ is the stained area of the hemocyte.
Concentrations of NO in Hemocytes
Following the method described by Wang et al. (2008) and Shi et al. (2012), after a 2-week exposure to acidified seawater, three individuals from each pH level were selected for the concentration of NO in hemocytes. A volume of about 150 μl of hemolymph was extracted from each individual followed by a quick determination of hemocyte concentration with 20 μl hemolymph using a Neubauer’s hemocytometer (XB-K-25, Anxin Optical Instrument) under Nikon eclipse E600 microscopy at the magnification of 400×. The concentration of NO in hemocytes was determined by the nitrate reductase method using the NO assay kit A012 (Nanjing Jiancheng Bioengineering Institute). The NO content was analyzed after converting all in the testing sample into using nitrate reductase according to the manufacturer’s instructions. In brief, hemocyte sample of 100 μl was mixed with reagents 1 and 2 and then incubated at 37°C for 1 h. After adding reagents 3 and 4, the mixture was incubated for another 10 min, followed by spinning at 4000 rpm for 10 min. The chromogenic reagent was subsequently added to the collected supernatant. After 10 min of incubation, the absorbance values were determined at the wavelength of 550 nm using a microplate reader (Multiskan GO, Thermo). The concentration of NO were subsequently determined by putting the obtained absorption values into the standard curves and divided by the total hemocytes counts of the testing sample.
Concentration and Activity of LZM in Hemocytes
The enzymatic activity and concentration of lysozymes of hemocytes were determined with ELISA kits FK-97441 and FK-97442 (FKBIO, Shanghai), respectively. As described above, after extraction and a quick determination of hemocyte concentration, 10 μl of the testing sample was mixed with 40 μl of diluent in a microwell plate and then incubated at 37°C for 30 min following the protocol provided. After the microwells were washed five times with a wash buffer, 50 μl of the conjugate reagent was added and then incubated at 37°C for another 30 min. The microwell was washed with the wash buffer again before adding 50 μl of each chromogenic reagent A and B. After 15 min of mixing in the dark, the stop buffer was used to terminate the chromogenic reaction. The values of absorbance were then determined at the wavelength of 450 nm using a microplate reader (Multiskan GO, Thermo). The concentration and activity of LZM of each sample were subsequently determined by referring to corresponding standard curves and calibrated with the hemocytes count.
Gene Expression Related to Cytoskeleton Regulation and NOS2
After exposure to the corresponding experimental seawater, three individuals were randomly picked from each pH level for the gene expression analysis. Total RNA was extracted from 100 μl hemolymph using an EASYspin Plus tissue/cell rapid RNA exaction kit (Aidlab, RN2802) following the method described by Peng et al. (2016). The concentration and quality of the RNA were verified with a NanoDrop 1000 UV/visible spectrophotometer (Thermo Scientific) and gel electrophoresis, respectively. One microgram of high-quality RNA was reversely transcribed into first strand cDNA using the PrimeScriptTM RT reagent kit (TaKaRa, RR037Q).
Genes of actin-related protein 2 (ARPC2), actin-related protein 3 (ARPC3), Rho-associated protein kinase (ROCK), p21-activated kinase 2 (PAK2), Ras homolog gene family member A (Rho), GTPase Kras (KRAS), GTPase Mras (MRAS), and Ras-related C3 botulinum toxin substrate 1 (Rac1) out of the actin cytoskeleton regulation pathway and NOS2 were analyzed in the present study. Primers and accession numbers for these tested genes and the reference 18S rRNA are listed in Table 2. All primers were synthesized by Sangon Biotech (Shanghai, China).
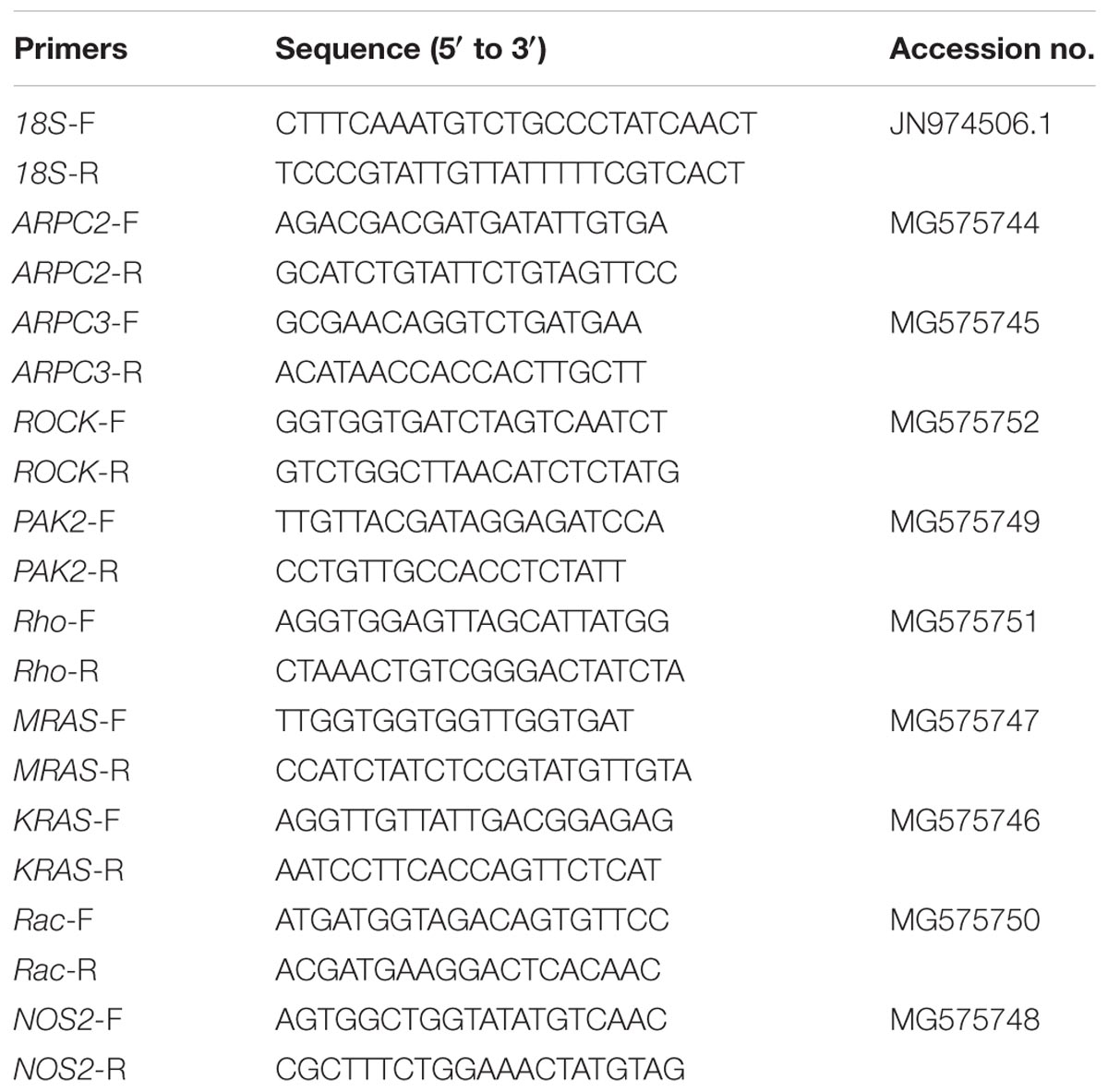
TABLE 2. Primers sequences for the genes investigated and the internal reference 18S rRNA (F and R after the dash line in the primer name indicate forward and reverse primer, respectively).
Statistical Analysis
One-way ANOVAs followed by Tukey’s post hoc tests were conducted to detect differences in the rates of phagocytosis, the abundances of the cytoskeleton components, the levels of NO, and the concentrations and activities of lysozymes (LZM) of the hemocytes among treatments. For all analyses, the assumptions of normality and homogeneity of variances were assessed using Shapiro–Wilk’s and Levene’s tests, respectively. For cases where these assumptions were not satisfied by the raw data, such as the percentage data for the phagocytosis assays, the data were arcsine square root transformed prior to the analysis, following the method described by Su et al. (2017). Expression levels of each tested gene were compared with the control by a t-test. All of the statistical analyses were conducted using OriginPro 8.0, and a p-value of less than 0.05 was accepted as a statistically significant difference.
Results
The Impact of OA Exposure on Phagocytosis
As shown in Figure 1, OA exposure exerted significant impacts on the rate of phagocytosis of hemocytes. Though no significant difference was detected between the pH 7.8 group and the control, the phagocytic rate significantly (p < 0.05) decreased to only approximately 69.24% of that of the control when the clams were exposed to seawater acidified at a pH of 7.4.
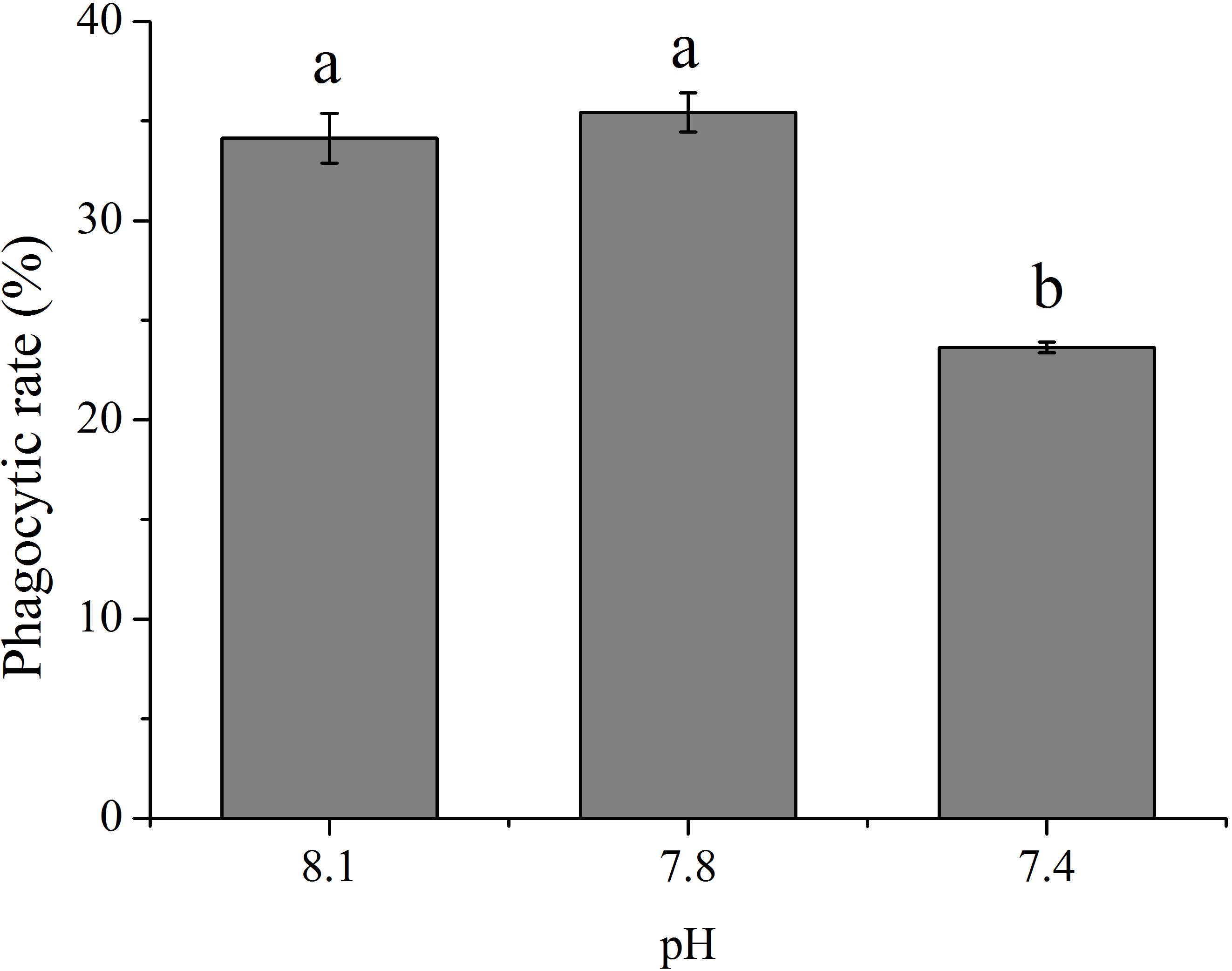
FIGURE 1. The rate of phagocytosis of T. granosa after 2-week exposure to present (pH 8.1) and predicted pCO2-driven ocean acidification (OA) scenarios (pH 7.8 and pH 7.4). All data was presented as means ± SE (n = 3) and different superscripts indicated a significant difference at p < 0.05.
The Impact of OA on the Abundance of Cytoskeleton Components
As shown in Figure 2, the abundances of cytoskeletons of hemocytes in terms of the fluorescence intensity of microfilament were significantly (p < 0.05) reduced when the clams were exposed to acidified seawater and were approximately 99.35 and 96.90% of that of the control for the pH 7.8 and pH 7.4 experimental groups, respectively.
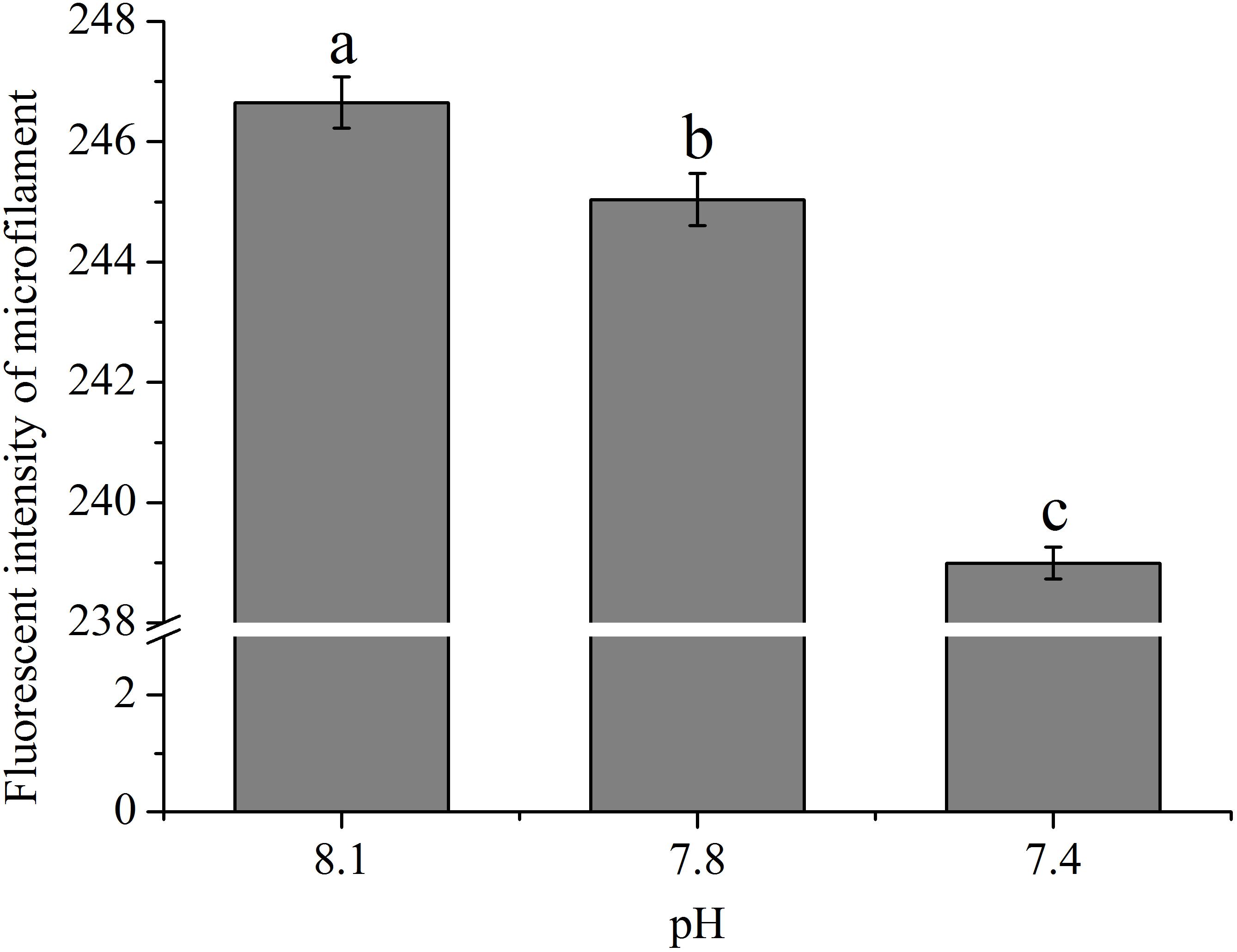
FIGURE 2. The fluorescence intensity of cytoskeleton (F-actin) of hemocytes of T. granosa after 2-week exposure to present (pH 8.1) and predicted pCO2-driven OA scenarios (pH 7.8 and pH 7.4). All data was presented as means ± SE (n = 4) and different superscripts indicated a significant difference at p < 0.05.
The Impact of OA on the Concentration of NO
With an increase in the pCO2 level of the exposure seawater, an evident increase (p < 0.05) in the concentration of NO was detected in hemocytes of clams (Figure 3). After exposure to future OA scenarios for 2 weeks, the NO concentration in hemocytes increased to approximately 1.86 and 6.13 times that of the control for the pH 7.8 and pH 7.4 groups, respectively.
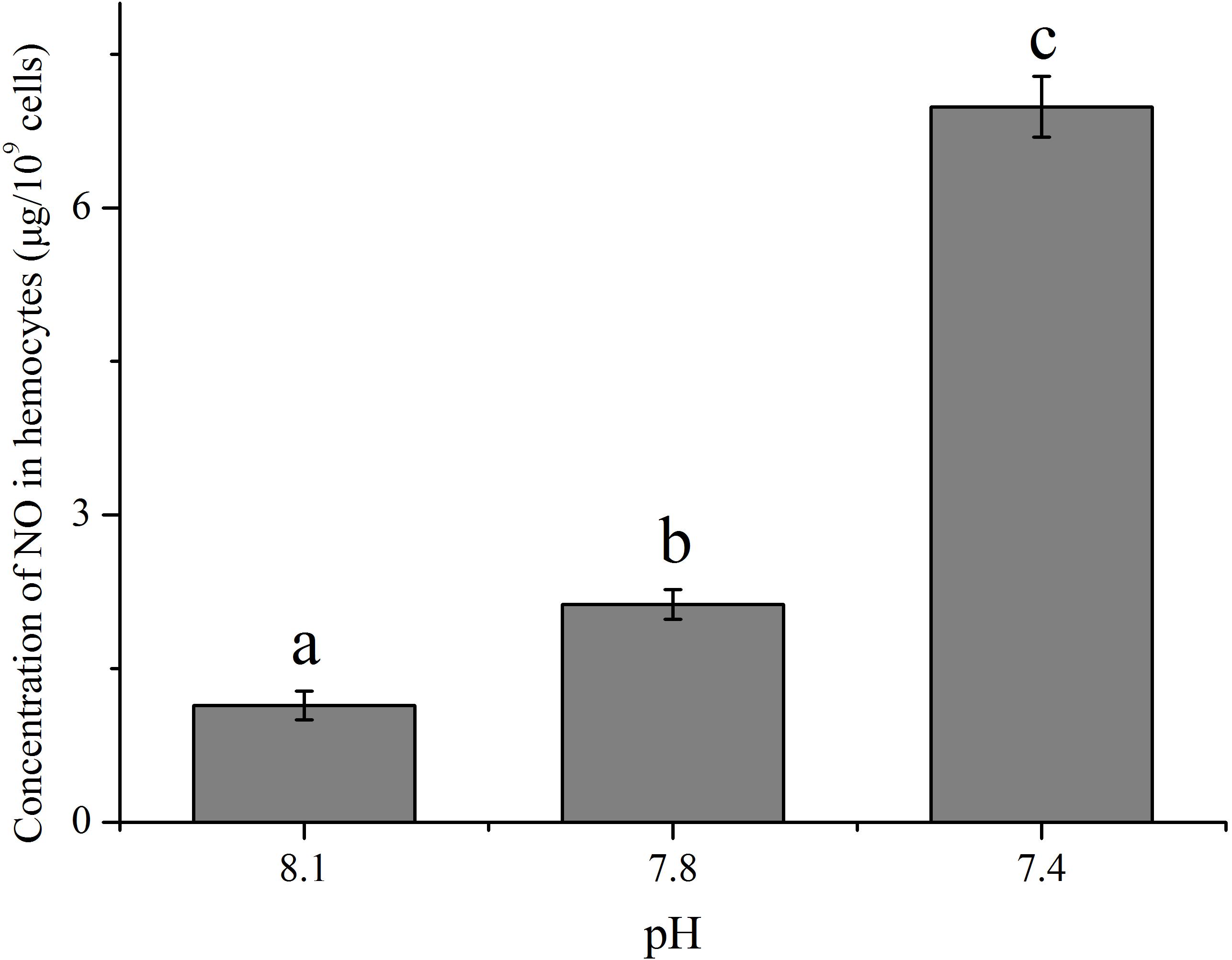
FIGURE 3. The concentration of nitric oxide (NO) in hemocytes of T. granosa after 2-week exposure to present (pH 8.1) and predicted pCO2-driven OA scenarios (pH 7.8 and pH 7.4). All data was presented as means ± SE (n = 3) and different superscripts indicated a significant difference at p < 0.05.
The Impact of OA on the Concentration and Enzymatic Activity of LZM
The concentration and enzymatic activity of LZM in hemocytes under the present and near-future OA scenarios are shown in Figures 4A,B, respectively. OA exposure showed significant impacts on the concentration of LZM of hemocytes. Though no significant difference was detected between the pH 7.8 group and the control, the concentration of LZM significantly (p < 0.05) decreased to only approximately 56.60% of that of the control when the clams were exposed to acidified seawater at a pH of 7.4 (Figure 4A). As illustrated in Figure 4B, the enzymatic activities of LZM in hemocytes were significantly (p < 0.05) reduced by about 10% when the clams were exposed to acidified seawater.
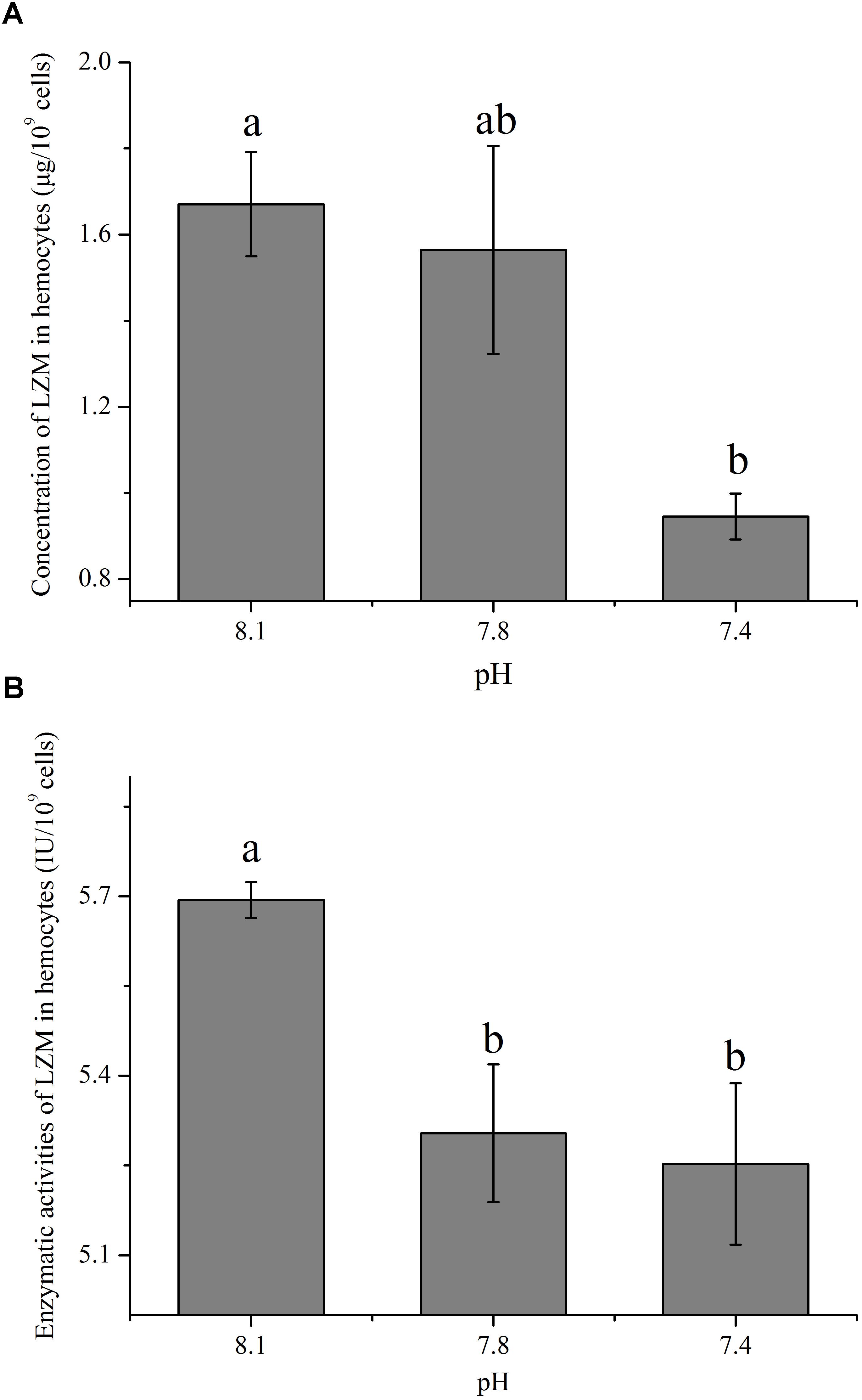
FIGURE 4. The concentration (A) and activity (B) of lysozyme (LZM) in hemocytes of T. granosa after 2-week exposure to present (pH 8.1) and predicted pCO2-driven OA scenarios (pH 7.8 and pH 7.4). All data was presented as means ± SE (n = 3) and different superscripts indicated a significant difference at p < 0.05.
The Effect of OA Exposure on the Expression of Tested Genes
As shown in Figure 5, except Rho, the expression of all the other tested genes (ARPC2, ARPC3, Rac, KRAS, MRAS, ROCK, and PAK2) from the actin cytoskeleton regulation pathway was significantly up-regulated (p < 0.05) after exposure of clams to acidified seawater. Similarly, the expression of the NOS2 gene was significantly (p < 0.05) up-regulated after treatment of clams with acidified seawater, which were approximately 2.71 and 4.47 times that of the control for the pH 7.8 and pH 7.4 experimental groups, respectively (Figure 6).
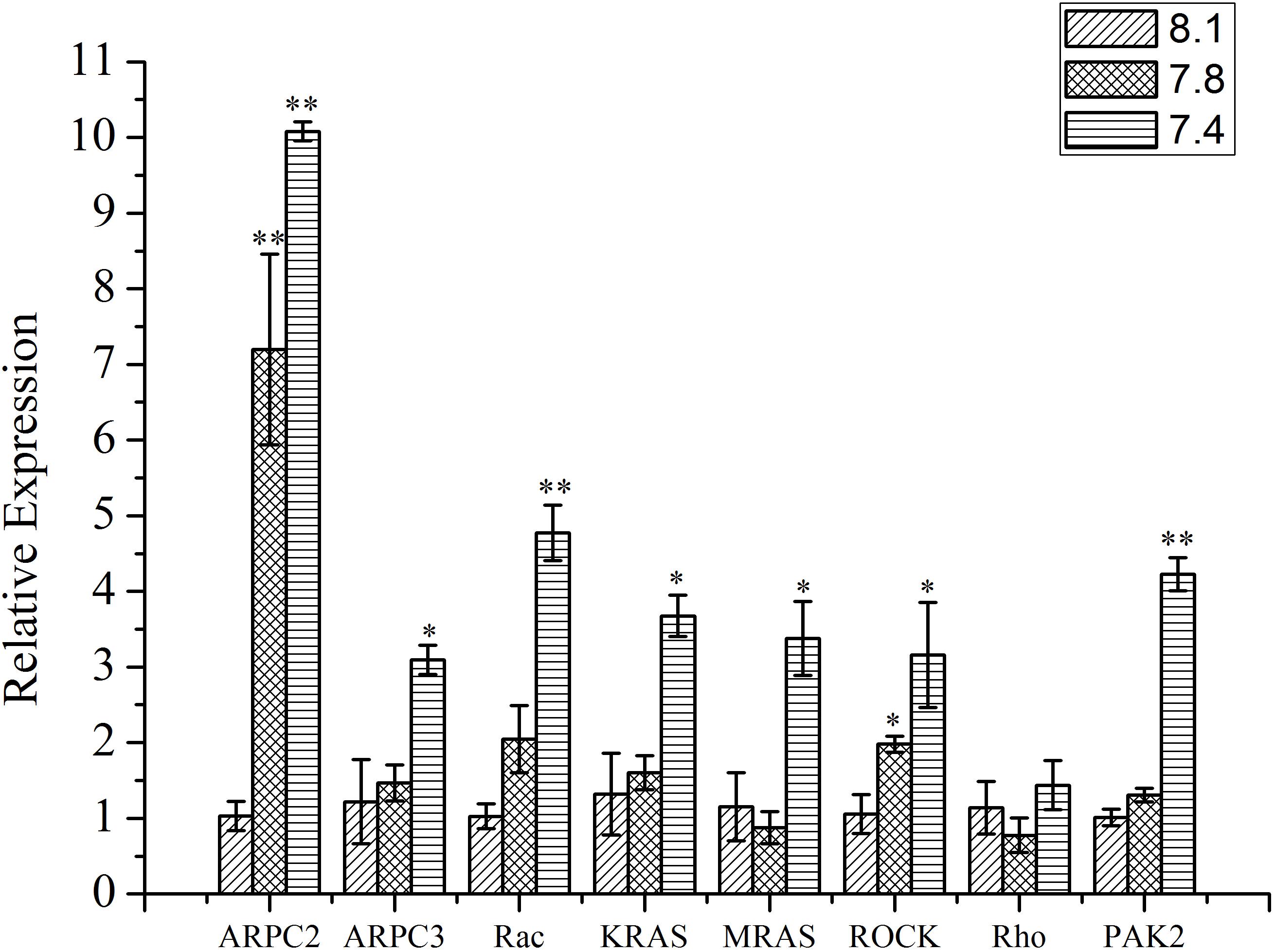
FIGURE 5. The expressions of key genes in actin cytoskeleton regulation pathway of T. granosa after 2-week exposure to present (pH 8.1) and predicted pCO2-driven OA scenarios (pH 7.8 and pH 7.4). All data was presented as means ± SE (n = 3). ∗p < 0.05 and ∗∗p < 0.01.
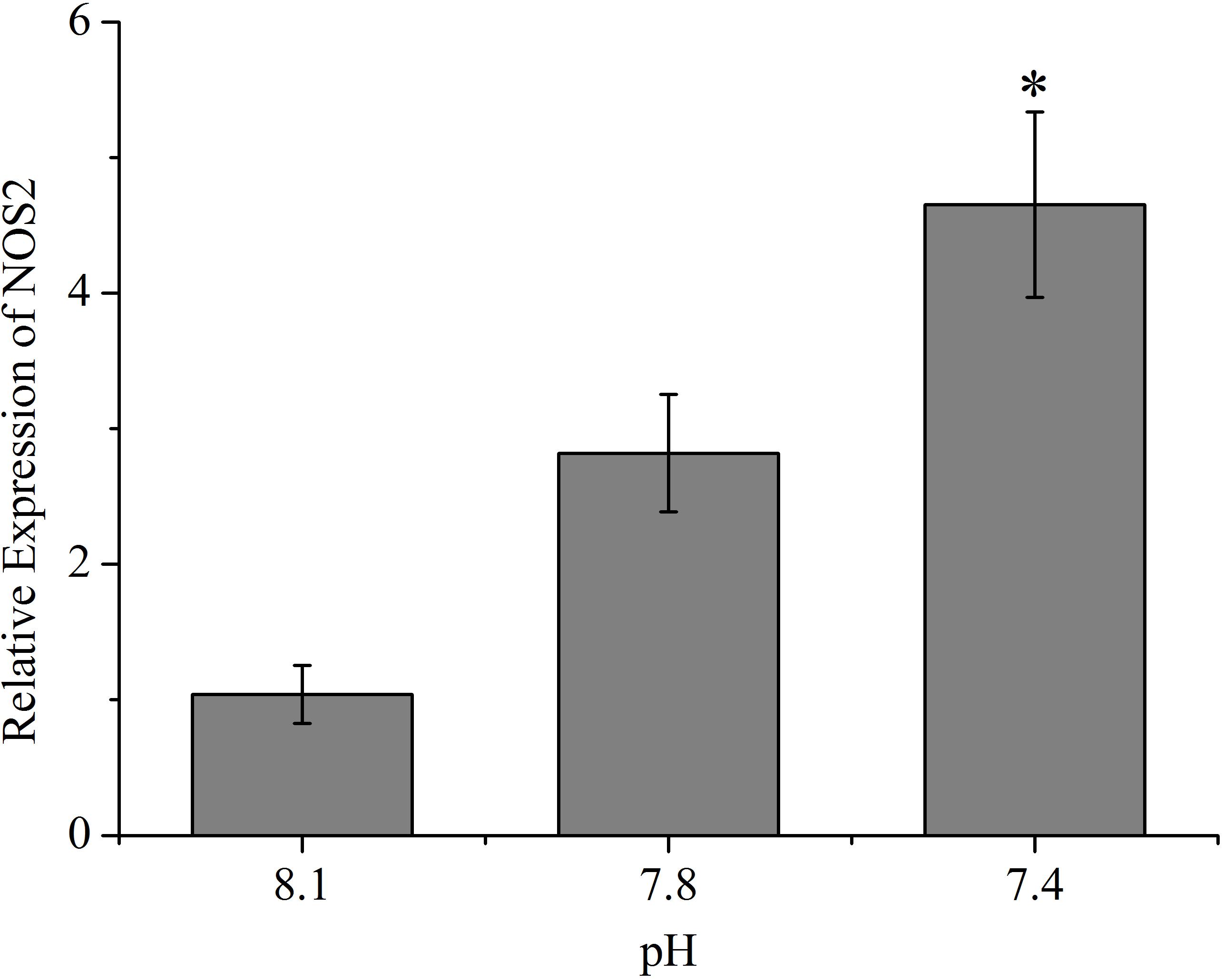
FIGURE 6. The expressions of nitric oxide synthesis 2 (NOS2) of T. granosa after 2-week exposure to present (pH 8.1) and predicted pCO2-driven OA scenarios (pH 7.8 and pH 7.4). All data was presented as means ± SE (n = 3). ∗p < 0.05.
Discussion
When putting the results obtained in the present study and those reported previously together, as illustrated in Figure 7, the mechanism affecting the underlying OA-induced reduction in phagocytosis of hemocytes can be summarized as (1) OA hampers PRRs, which constraints the ability of hemocytes to recognizing foreign particles; (2) OA induces ROS, which increases apoptosis of hemocytes, hampers cytoskeleton mediated engulfment, and alters oxygen-dependent degradation; (3) OA induces the production of NO, which may directly enhance the oxygen-dependent degradation but negatively regulate immune responses; (4) OA reduces the concentration and activity of lysozyme, which hampers the oxygen-independent degradation of engulfed particles.
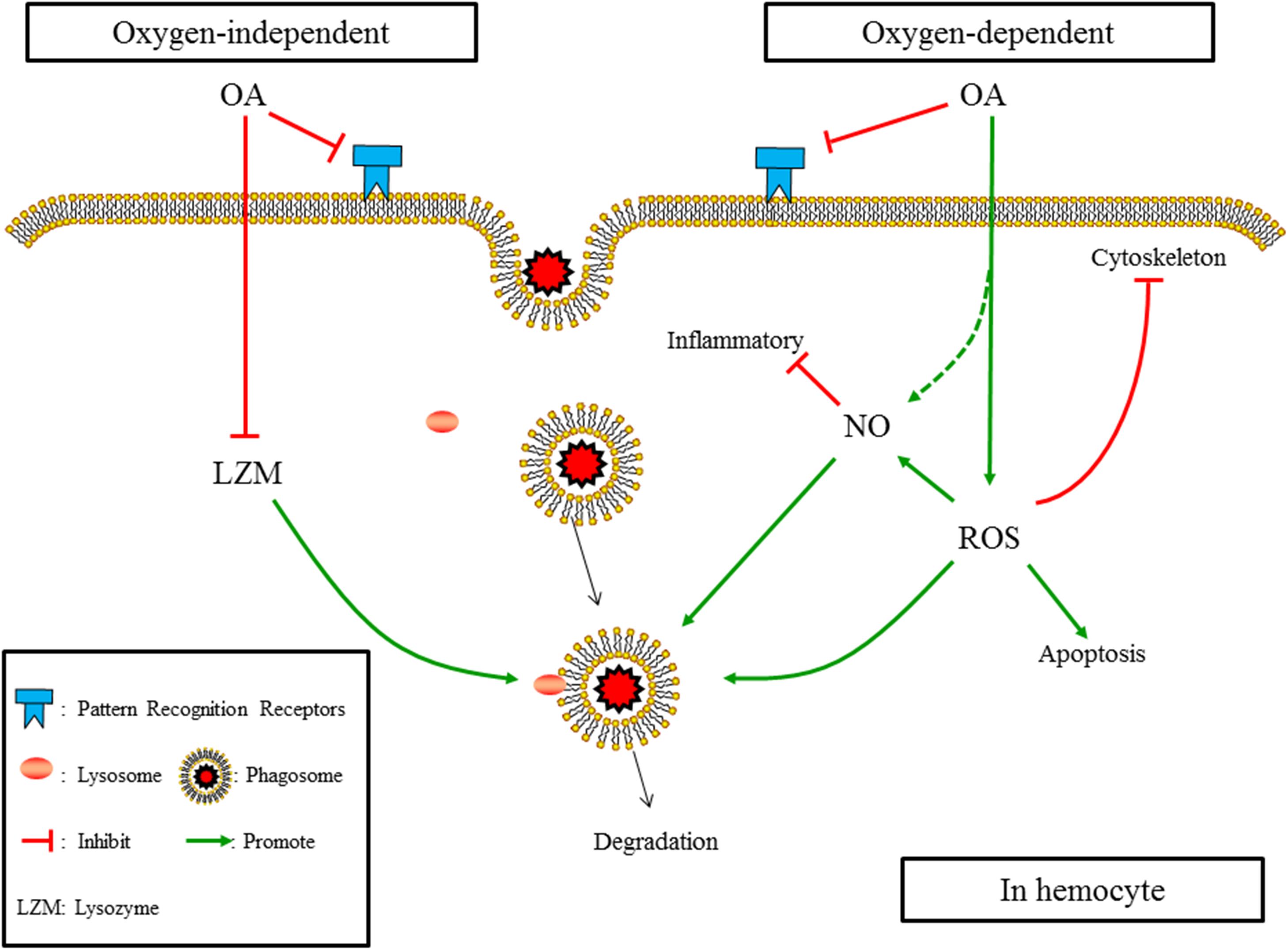
FIGURE 7. The summarized affecting mechanism of OA induced reduction in phagocytosis according to results obtained in the present study and those published previously. (1) OA hampers PRRs; (2) OA induces ROS, which increases apotosis of hemocytes, hampers cytoskeleton mediated engulfment, and alters oxygen-dependent degradation; (3) OA induces the production of NO, which may directly enhance the oxygen-dependent degradation but negatively regulates immune responses; (4) OA reduces the concentration and activity of lysozyme, which hampers the oxygen-independent degradation of engulfed particles.
Previous studies have consistently shown that OA could induce the production of ROS in bivalve species, such as C. gigas (Wang et al., 2016), M. coruscus (Huang et al., 2016; Wu et al., 2016), and C. virginica (Tomanek et al., 2011). Though induced ROS suggests an enhanced oxygen-dependent degradation potency of hemoytes, it may cause apotosis of hemocytes and constrain the cytoskeleton-mediated process of engulfment. Three major cell types of hemocytes were identified in T. granosa (Zhu et al., 2011), among which the red granulocyte was predicted to have the highest ability of phagocytosis. According to previous results reported by Liu et al. (2016) and Su et al. (2017), OA could reduce the proportion and number of red granulocytes in blood clams probably due to OA-induced production of ROS. Therefore, the reduction of phagocytosis detected could be partially explained by the OA-induced production of ROS and subsequent apoptosis of hemocytes, especially those of red granulocytes.
F-actin, one of the essential components of microfilaments, which plays an essential role in the process of phagocytosis, is subject to the increase in ROS (Pollard and Cooper, 2009; Tomanek et al., 2011). Therefore, the reduction in the abundance of the cytoskeleton components in terms of the F-actin-specific fluorescence intensity detected in the present study could be attributed to the OA-induced production of ROS as well. Since the cytoskeleton-mediated process of engulfment is crucial for the phagocytic activity of hemocytes (Pollard and Cooper, 2009), OA could therefore hamper the engulfment of pathogen particles by reducing the abundance of the cytoskeleton component. Unlike the abundance of the cytoskeleton component, the key genes of the actin cytoskeleton regulation pathway were found to be up-regulated after OA exposure. Since ARPC2 and ARPC3 function as a complex to assemble the monomers into polymers in the process of producing F-actin from G-actin (Insall et al., 2001), the up-regulation of these two genes indicated an increased ability to produce the cytoskeleton component, which was also supported by the up-regulation of their upstream genes (MRAS, KRAS, and Rac). In addition, since PAK2 stabilizes actin (Bokoch, 2003), the up-regulation of PAK2 indicated an increased stabilization potency of elementary actin. Similar to the results reported previously (Tomanek et al., 2011), since the cytoskeleton is essential for the normal function of cells, the up-regulation of these genes could be a feedback to compensate for the reduction in cytoskeleton components caused by OA exposure.
It has been widely accepted that lysozymes play a crucial role in the oxygen-independent degradation of engulfed pathogen particles. However, only a few studies have investigated the impacts of OA on LZM of marine organisms, and controversial results have been reported in different organisms (Munari, 2014; Bresolin et al., 2016). For instance, a previous study conducted in M. galloprovincialis and V. philippinarum showed that OA exerted different impacts on the activities of LZM of these two bivalve species (Munari, 2014). The reason leading to the differences among investigations might be due to the fact that different types of lysozymes exist in different species (Takeshita et al., 2004; Xue et al., 2004; Zhao et al., 2007). For example, i-type and g-type LZMs, which may respond to OA differently, have been identified in C. virginica (Xue et al., 2004) and C. farreri (Zhao et al., 2007), respectively. In the present study, it has been shown that OA exposure led to a significant reduction in both the concentration and activity of LZM of hemocytes in the blood clam. These reductions indicated a hampered degradation of engulfed particles through the oxygen-independent pathway, which may also account for the reduction in phagocytosis under OA scenarios.
The increase in the production of in vivo NO induced by OA detected in the present study may be attributed to the following reasons. First, previous studies have suggested that reduced pH could promote the generation of NO in mammals or plants (Rõszer, 2012). Since it has been shown that OA exposure decreased the pH level of hemolymphs in blood clams (Zhao et al., 2017b), the acidosis caused by OA could be one of the reasons for the increased production of in vivo NO. Second, OA exposure could cause oxidative stress and lead to an increase in ROS, which may subsequently hamper the homeostasis of mitochondria and induce the generation of antioxidant enzymes, such as various reductases (Hu et al., 2015). On the one hand, since reductive NO can be produced from by reductases, the increase in reductases may promote the production of reductive NO (Rõszer, 2012). On the other hand, the generation of NO may be induced in response to ROS to stabilize mitochondria as well (Mao et al., 2013). Third, since in vivo oxidative NO is generated mainly through nitric oxide synthase (NOS), the detected up-regulation of the NOS2 gene may also increase the production of oxidative NO under OA scenarios. Since the transcription of NOS2 can be triggered by specific stimuli such as inflammation, the up-regulation of NOS2 may be due to increased inflammatory reactions induced by OA stress (Ganster et al., 2001). Also, since the in vivo NO plays versatile roles in innate immunity, various consequences in terms of phagocytosis may be caused by the increased production of NO. On the one hand, as an active antimicrobial molecule, the increase in in vivo NO after OA exposure seems to indicate an enhanced ability to degrade engulfed particles. On the other hand, as one of the most important immune regulators, the induced production of in vivo NO could suppress immune responses such as phagocytosis (Mao et al., 2013).
In addition, according to previous studies, the hampered recognition of pathogen particles by hemocytes through PRRs (Liu et al., 2016; Castillo et al., 2017) and the re-allocation of energy among various life processes (Kurihara, 2008; Pan et al., 2015) could account for the reduction in phagocytosis detected as well. As been suggested in previous studies (Lannig et al., 2010; Pan et al., 2015; Liu et al., 2016), under the stress of OA, marine organisms may allocate more energy to critical life processes such as basal metabolism and internal ion regulation and therefore put a constraint on the energy available needed for the process of phagocytosis.
Conclusion
The results obtained in the present study suggested that OA suppresses the process of phagocytosis through hampering the recognition of pathogen particles, inhibiting the process of engulfment by reducing the abundant of cytoskeleton components, as well as altering the oxygen-dependent and -independent degradation by enhancing the production of NO whereas reducing the concentration and activity of lysozyme.
Author Contributions
WS and GL: conceived and designed the experimental plan; analyzed the data and drafted the manuscript. WS, JR, SZ, MY, and JF: performed the experiments.
Funding
This work was funded by National Natural Science Foundation of China (Grant No. 31672634), Open Fund of Key Laboratory for Marine Ecosystem and Biogeochemistry, SOA (Grant No. LMEB201708), and Open Fund of Key Laboratory of Eco-Environment & Disaster Preservation of Shandong, North China Sea Branch of SOA (Grant No. 201704).
Conflict of Interest Statement
The authors declare that the research was conducted in the absence of any commercial or financial relationships that could be construed as a potential conflict of interest.
References
Allen, L. H., and Aderem, A. (1995). A role for MARCKS, the alpha isozyme of protein kinase C and myosin I in zymosan phagocytosis by macrophages. J. Exp. Med. 182, 182–829. doi: 10.1084/jem.182.3.829
Anderson, D. H., and Robinson, R. J. (1946). Rapid electrometric determination of alkalinity of sea water using glass electrode. Ind. Eng. Chem. 18, 767–769. doi: 10.1021/i560160a011
Ashur, M. M., Johnston, N. K., and Dixson, D. L. (2017). Impacts of ocean acidification on sensory function in marine organisms. Integr. Comp. Biol. 57, 63–80. doi: 10.1093/icb/icx010
Bogdan, C. (2001). Nitric oxide and the immune response. Nat. Immunol. 2, 907–916. doi: 10.1038/ni1001-907
Bokoch, G. M. (2003). Biology of the p21-activated kinases. Annu. Rev. Biochem. 72, 743–781. doi: 10.1146/annurev.biochem.72.121801.161742
Bresolin, D. S. K., Asker, N., Jönsson, E., Förlin, L., and Sturve, J. (2016). Increased activity of lysozyme and complement system in Atlantic halibut exposed to elevated CO2 at six different temperatures. Mar. Environ. Res. 122, 143–147. doi: 10.1016/j.marenvres.2016.09.005
Buggé, D. M., Hégaret, H., Wikfors, G. H., and Allam, B. (2007). Oxidative burst in hard clam (Mercenaria mercenaria) hemocytes. Fish Shellfish Immunol. 23, 188–196. doi: 10.1016/j.fsi.2006.10.006
Caldeira, K., and Wickett, M. E. (2003). Oceanography: anthropogenic carbon and ocean pH. Nature 425:365. doi: 10.1038/425365a
Canesi, L., Gallo, G., Gavioli, M., and Pruzzo, C. (2002). Bacteria-hemocyte interactions and phagocytosis in marine bivalves. Microsc. Res. Techniq. 57, 469–476. doi: 10.1002/jemt.10100
Castillo, N., Saavedra, L. M., Vargas, C. A., Gallardoescárate, C., and Détrée, C. (2017). Ocean acidification and pathogen exposure modulate the immune response of the edible mussel Mytilus chilensis. Fish Shellfish Immunol. 70, 149–155. doi: 10.1016/j.fsi.2017.08.047
Catapane, E. J., Nelson, M., Adams, T., and Carroll, A. M. (2016). Innervation of gill lateral cells in the bivalve mollusc Crassostrea virginica affects cellular membrane potential and cilia activity. J. Pharmacol. Rep. 1, 36–50.
Cima, F., Matozzo, V., Marin, M. G., and Ballarin, L. (2000). Haemocytes of the clam Tapes philippinarum (Adams & Reeve, 1850): morphofunctional characterisation. Fish Shellfish Immunol. 10, 677–693. doi: 10.1006/fsim.2000.0282
Dickson, A. G., and Millero, F. J. (1987). A comparison of the equilibrium constants for the dissociation of carbonic acid in seawater media. Deep Sea Res. Part I 34, 1733–1743. doi: 10.1016/0198-0149(87)90021-5
Dixson, D. L., Munday, P. L., and Jones, G. P. (2010). Ocean acidification disrupts the innate ability of fish to detect predator olfactory cues. Ecol. Lett. 13, 68–75. doi: 10.1111/j.1461-0248.2009.01400.x
Duprécrochet, S., Erard, M., and Nüβe, O. (2013). ROS production in phagocytes: why, when, and where. J. Leukoc. Biol. 94, 657–670. doi: 10.1189/jlb.1012544
Evelyne, B., Dominique, H., and Eric, M. (1991). Luminol-dependent chemiluminescence by hemocytes of two marine bivalves, Ostrea edulis and Crassostrea gigas. Dis. Aquat. Organ. 11, 173–180. doi: 10.1292/jvms.55.287
Ganster, R. W., Taylor, B. S., Shao, L., and Geller, D. A. (2001). Complex regulation of human inducible nitric oxide synthase gene transcription by Stat 1 and NF-kappa B. Proc. Natl. Acad. Sci. U.S.A. 98, 8638–8643. doi: 10.1073/pnas.151239498
Goncalves, P., Jones, D. B., Thompson, E. L., Parker, L. M., Ross, P. M., and Raftos, D. A. (2017). Transcriptomic profiling of adaptive responses to ocean acidification. Mol. Ecol. 26, 5974–5988. doi: 10.1111/mec.14333
Guo, C., Han, Y., Shi, W., Zhao, X., Teng, S., Xiao, G., et al. (2017). Ca2+-channel and calmodulin play crucial roles in the fast electrical polyspermy blocking of Tegillarca granosa (Bivalvia: Arcidae). J. Mollus. Stud. 83, 289–294. doi: 10.1093/mollus/eyx016
Han, Y., Shi, W., Guo, C., Zhao, X., Liu, S., Wang, Y., et al. (2016). Characteristics of chitin synthase (CHS) gene and its function in polyspermy blocking in the blood clam Tegillarca granosa. J. Mollus. Stud. 82, 550–557. doi: 10.1093/mollus/eyw018
Hu, M., Li, L., Sui, Y., Li, J., Wang, Y., Lu, W., et al. (2015). Effect of pH and temperature on antioxidant responses of the thick shell mussel Mytilus coruscus. Fish Shellfish Immunol. 46, 573–583. doi: 10.1016/j.fsi.2015.07.025
Huang, X., Lin, D., Ning, K., Sui, Y., Hu, M., Lu, W., et al. (2016). Hemocyte responses of the thick shell mussel Mytilus coruscus exposed to nano-TiO2 and seawater acidification. Aquat. Toxicol. 180, 1–10. doi: 10.1016/j.aquatox.2016.09.008
Insall, R., Müller-Taubenberger, A., Machesky, L., Köhler, J., Simmeth, E., Atkinson, S. J., et al. (2001). Dynamics of the dictyostelium Arp2/3 complex in endocytosis, cytokinesis, and chemotaxis. Cytoskeleton 50, 115–128. doi: 10.1002/cm.10005
Ivanina, A. V., Hawkins, C., and Sokolova, I. M. (2016). Interactive effects of copper exposure and environmental hypercapnia on immune functions of marine bivalves Crassostrea virginica and Mercenaria mercenaria. Fish Shellfish Immunol. 49, 54–65. doi: 10.1016/j.fsi.2015.12.011
Kelly, R. P., Foley, M. M., Fisher, W. S., Feely, R. A., Halpern, B. S., Waldbusser, G. G., et al. (2011). Mitigating local causes of ocean acidification with existing laws. Science 332, 1036–1037. doi: 10.1126/science.1203815
Kryukova, N. A., Chertkova, E. A., Semenova, A. D., Glazachev, Y. I., Slepneva, I. A., and Glupov, V. V. (2015). Venom from the ectoparasitic wasp Habrobracon hebetor activates calcium- dependent degradation of Galleria mellonella larval hemocytes. Arch. Insect Biochem. 90, 117–130. doi: 10.1002/arch.21247
Kurihara, H. (2008). Effects of CO2-driven ocean acidification on the early developmental stages of invertebrates. Mar. Ecol. Prog. Ser. 373, 275–284. doi: 10.3354/meps07802
Lannig, G., Eilers, S., Pörtner, H. O., Sokolova, I. M., and Bock, C. (2010). Impact of ocean acidification on energy metabolism of oyster, Crassostrea gigas - changes in metabolic pathways and thermal response. Mar. Drugs 8, 2318–2339. doi: 10.3390/md8082318
Liu, G., Shu, M., Chai, X., Shao, Y., Wu, H., Sun, C., et al. (2014). Effect of chronic sublethal exposure of major heavy metals on filtration rate, sex ratio, and gonad development of a bivalve species. Bull. Environ. Contam. Tox. 92, 71–74. doi: 10.1007/s00128-013-1138-9
Liu, S., Shi, W., Guo, C., Zhao, X., Han, Y., Peng, C., et al. (2016). Ocean acidification weakens the immune response of blood clam through hampering the NF-kappa β and toll-like receptor pathways. Fish Shellfish Immunol. 54, 322–327. doi: 10.1016/j.fsi.2016.04.030
Mackenzie, C. L., Lynch, S. A., Culloty, S. C., and Malham, S. K. (2014). Future oceanic warming and acidification alter immune response and disease status in a commercial shellfish species, Mytilus edulis L. PLoS One 9:e99712. doi: 10.1371/journal.pone.0099712
Mao, K., Chen, S., Chen, M., Ma, Y., Wang, Y., Huang, B., et al. (2013). Nitric oxide suppresses NLRP3 inflammasome activation and protects against LPS-induced septic shock. Cell Res. 23, 201–212. doi: 10.1038/cr.2013.6
Mehrbach, C., Culberson, C. H., Hawley, J. E., and Pytkowicx, R. M. (1973). Measurement of the apparent dissociation constants of carbonic acid in seawater at atmospheric pressure. Limnol. Oceanogr. 18, 897–907. doi: 10.4319/lo.1973.18.6.0897
Munari, M. (2014). Combined Effects of Seawater Acidification and Emerging Contaminants on Marine Bivalves. Università degli Studi di Padova. Available at: http://paduaresearch.cab.unipd.it/6759/
Orr, J. C., Fabry, V. J., Aumont, O., Bopp, L., Doney, S. C., Feely, R. A., et al. (2005). Anthropogenic ocean acidification over the twenty-first century and its impact on calcifying organisms. Nature 437, 681–686. doi: 10.1038/nature04095
Pan, T. C., Applebaum, S. L., and Manahan, D. T. (2015). Experimental ocean acidification alters the allocation of metabolic energy. Proc. Natl. Acad. Sci. U.S.A. 112, 4696–4701. doi: 10.1073/pnas.1416967112
Parkhaev, P. Y. (2017). Origin and the early evolution of the phylum mollusca. Paleontol. J. 51, 663–686. doi: 10.1134/S003103011706003X
Peng, C., Zhao, X., Liu, S., Shi, W., Han, Y., Guo, C., et al. (2016). Effects of anthropogenic sound on digging behavior, metabolism, Ca2+/Mg2+ ATPase activity, and metabolism-related gene expression of the bivalve Sinonovacula constricta. Sci. Rep. 6:24266. doi: 10.1038/srep24266
Peng, C., Zhao, X., Shi, W., Liu, S., Liu, G., et al. (2015). Toxic effects of chronic sub-lethal Cu2+, Pb2+ and Cd2+ on antioxidant enzyme activities in various tissues of the blood cockle, Anadara granosa. J. Residuals Sci. Tech. 12, 125–131. doi: 10.12783/issn.1544-8053/12/3/2
Pierrot, D., Lewis, E., Wallace, R., Wallace, D., Wallace, W., and Wallace, D. W. R. (2006). MS Excel Program Developed for CO2 System Calculations. Oak Ridge, TN: Carbon Dioxide Information Analysis Center.
Pollard, T. D., and Cooper, J. A. (2009). Actin, a central player in cell shape and movement. Science 326, 1208–1212. doi: 10.1126/science.1175862
Raven, J., Caldeira, K., Elderfield, H., Hoegh-Guldberg, O., Liss, P., Riebesell, U., et al. (2005). Ocean acidification due to increasing atmospheric carbon dioxide. Science 215, 1–60.
Rõszer, T. (2012). The Biology of Subcellular Nitric Oxide. Dordrecht: Springer. doi: 10.1007/978-94-007-2819-6
Sabine, C. L., Feely, R. A., Gruber, N., Key, R. M., Lee, K., Bullister, J. L., et al. (2004). The oceanic sink for anthropogenic CO2. Science 305, 367–371. doi: 10.1126/science.1097403
Shao, Y., Chai, X., Xiao, G., Zhang, J., Lin, Z., and Liu, G. (2009). Population genetic structure of the blood clam, Tegillarca granosa, along the Pacific coast of Asia: isolation by distance in the sea. Malacologia 59, 303–312. doi: 10.4002/040.059.0208
Shi, W., Han, Y., Guo, C., Zhao, X., Liu, S., Su, W., et al. (2017). Immunotoxicity of nanoparticle nTiO2 to a commercial marine bivalve species, Tegillarca granosa. Fish Shellfish Immunol. 66, 300–306. doi: 10.1016/j.fsi.2017.05.036
Shi, X., Wang, L., Zhou, Z., Yang, C., Gao, Y., Wang, L., et al. (2012). The arginine kinase in Zhikong scallop Chlamys farreri is involved in immunomodulation. Dev. Comp. Immunol. 37, 270–278. doi: 10.1016/j.dci.2012.03.008
Sokolnikova, Y. N., Trubetskaya, E. V., Beleneva, I. A., Grinchenko, A. V., and Kumeiko, V. V. (2015). Fluorescent in vitro phagocytosis assay differentiates hemocyte activity of the bivalve molluscs Modiolus kurilensis (Bernard, 1983) inhabiting impacted and non-impacted water areas. Russ. J. Mar. Biol. 41, 118–126. doi: 10.1134/S106307401502011X
Song, L., Wang, L., Qiu, L., and Zhang, H. (2010). Bivalve immunity. Adv. Exp. Med. Biol. 708, 44–65. doi: 10.1007/978-1-4419-8059-5_3
Su, W., Zha, S., Wang, Y., Shi, W., Xiao, G., Chai, X., et al. (2017). Benzo[a]pyrene exposure under future ocean acidification scenarios weakens the immune responses of blood clam, Tegillarca granosa. Fish Shellfish Immunol. 63, 465–470. doi: 10.1016/j.fsi.2017.02.046
Takeshita, K., Hashimoto, Y., Thujihata, Y., So, T., Ueda, T., and Iomoto, T. (2004). Determination of the complete cDNA sequence, construction of expression systems, and elucidation of fibrinolytic activity for Tapes japonica lysozyme. Protein Expres. Purif. 36, 254–262. doi: 10.1016/j.pep.2004.05.001
Tomanek, L., Zuzow, M. J., Ivanina, A. V., Beniash, E., and Sokolova, I. M. (2011). Proteomic response to elevated pCO2 level in eastern oysters, Crassostrea virginica: evidence for oxidative stress. J. Exp. Biol. 214, 1836–1844. doi: 10.1242/jeb.055475
Wang, M. L., Suo, X., Gu, J. H., Zhang, W. W., Fang, Q., and Wang, X. (2008). Influence of grape seed proanthocyanidin extract in broiler chickens: effect on chicken coccidiosis and antioxidant status. Poult. Sci. 87, 2273–2280. doi: 10.3382/ps.2008-00077
Wang, Q., Cao, R., Ning, X., You, L., Mu, C., Wang, C., et al. (2016). Effects of ocean acidification on immune responses of the Pacific oyster Crassostrea gigas. Fish Shellfish Immunol. 49, 24–33. doi: 10.1016/j.fsi.2015.12.025
Wang, X., Ding, L., Yan, M., Chai, X., Lu, R., Wang, Q., et al. (2012). Polysaccharides, saponins, and water decoction of Astragalus membranaceus significantly enhance the non-specific immune response of spotted maigre (Nibea albiflora). Isr. J. Aquac. 64, 557–560.
Wu, F., Lu, W., Shang, Y., Kong, H., Li, L., Sui, Y., et al. (2016). Combined effects of seawater acidification and high temperature on hemocyte parameters in the thick shell mussel Mytilus coruscus. Fish Shellfish Immunol. 56, 554–562. doi: 10.1016/j.fsi.2016.08.012
Wu, Y., Campbell, D. A., Irwin, A. J., Suggett, D. J., and Finkel, Z. V. (2014). Ocean acidification enhances the growth rate of larger diatoms. Limnol. Oceanogr. 59, 1027–1034. doi: 10.4319/lo.2014.59.3.1027
Xue, Q. G., Peyre, J. F. L., and Schey, K. L. (2004). Purification and characterization of lysozyme from plasma of the eastern oyster (Crassostrea virginica). Comp. Biochem. Phys. B 139, 11–25. doi: 10.1016/j.cbpc.2004.05.011
Zhang, J., Zhang, Z., and Zhang, Y. (2012). Ocean acidification and its impacts on marine ecosystem. Mar. Geol. Front. 28, 1–9.
Zhao, J., Song, L., Li, C., Zou, H., Ni, D., Wang, W., et al. (2007). Molecular cloning of an invertebrate goose-type lysozyme gene from Chlamys farreri, and lytic activity of the recombinant protein. Mol. Immunol. 44, 1198–1208. doi: 10.1016/j.molimm.2006.06.008
Zhao, X., Guo, C., Han, Y., Che, Z., Wang, Y., Wang, X., et al. (2017a). Ocean acidification decreases mussel byssal attachment strength and induces molecular byssal responses. Mar. Ecol. Prog. Ser. 565, 67–77. doi: 10.3354/meps11992
Zhao, X., Shi, W., Han, Y., Liu, S., Guo, C., Fu, W., et al. (2017b). Ocean acidification adversely influences metabolism, extracellular pH and calcification of an economically important marine bivalve, Tegillarca granosa. Mar. Environ. Res. 125, 82–89. doi: 10.1016/j.marenvres.2017.01.007
Keywords: ocean acidification, phagocytosis, cytoskeleton, NO, lysozyme
Citation: Su W, Rong J, Zha S, Yan M, Fang J and Liu G (2018) Ocean Acidification Affects the Cytoskeleton, Lysozymes, and Nitric Oxide of Hemocytes: A Possible Explanation for the Hampered Phagocytosis in Blood Clams, Tegillarca granosa. Front. Physiol. 9:619. doi: 10.3389/fphys.2018.00619
Received: 14 March 2018; Accepted: 07 May 2018;
Published: 23 May 2018.
Edited by:
Youji Wang, Shanghai Ocean University, ChinaReviewed by:
Anna Ivanina, University of North Carolina at Charlotte, United StatesJianmin Zhao, Yantai Institute of Coastal Zone Research (CAS), China
Rongqing Zhang, Tsinghua University, China
Copyright © 2018 Su, Rong, Zha, Yan, Fang and Liu. This is an open-access article distributed under the terms of the Creative Commons Attribution License (CC BY). The use, distribution or reproduction in other forums is permitted, provided the original author(s) and the copyright owner are credited and that the original publication in this journal is cited, in accordance with accepted academic practice. No use, distribution or reproduction is permitted which does not comply with these terms.
*Correspondence: Guangxu Liu, Z3Vhbmd4dV9saXVAemp1LmVkdS5jbg==