- 1The Fish Molecular Genetics and Biotechnology Laboratory, Aquatic Genomics Unit, School of Fisheries, Aquaculture and Aquatic Sciences, Auburn University, Auburn, AL, United States
- 2Department of Biology, College of Art and Sciences, Syracuse University, Syracuse, NY, United States
Catfish is one of the major aquaculture species in the United States. However, the catfish industry is threatened by several bacterial diseases such as enteric septicemia of catfish (ESC), columnaris disease and Aeromonas disease, as well as by abiotic stresses such as high temperature and low oxygen. Research has been conducted for several decades to understand the host responses to these diseases and abiotic stresses. With the development of sequencing technologies, and the application of genome-wide association studies in aquaculture species, significant progress has been made. This review article summarizes recent progress in understanding the molecular responses of catfish after bacterial infection and stress challenges, and in understanding of genomic and genetic basis for disease resistance and stress tolerance.
Introduction
Diseases cause significant amount of economic losses in aquaculture (Meyer, 1991). The disease problems in aquaculture systems are increased following exposure to abiotic stresses. Thus, diseases and abiotic stresses are related, and they together post greater challenges for aquaculture industry. Great efforts have been made to elucidate the molecular mechanisms for disease resistance and stress tolerance. A good example is the identification of quantitative trait locus (QTL) and determination of the causal gene for the infectious pancreatic necrosis virus (IPNV) resistance in Atlantic salmon Salmo salar (Houston et al., 2008, 2010; Moen et al., 2015), and such information is now applied in the aquaculture industry to control IPVN. As summarized in a recent white paper (Abdelrahman et al., 2017), increasingly more QTL studies are being conducted to identify genetic variants and genomic regions associated with disease resistance and stress tolerance in various aquaculture species, including channel catfish (Ictalurus punctatus), Atlantic salmon, rainbow trout (Oncorhynchus mykiss), Asian seabass (Lates calcarifer), and Japanese flounder (Paralichthys olivaceus). Moreover, transcriptomic analyses after disease infection and stress challenges were conducted in aquaculture species (Qian et al., 2014; Eissa and Wang, 2016), providing insights into differentially expressed genes, and their involved pathways, all providing information for the development of genome-based technologies for disease control for a sustainable aquaculture industry.
Catfish is a diverse group with approximately 4,000 species. Several catfish species are important for aquaculture across the world including African catfish (Clarias gariepinus), walking catfish (Clarias batrachus), shark catfish (Pangasius bocourti), Thai catfish (Clarias macrobrachium), channel catfish and blue catfish (I. furcatus). In North America, channel catfish and hybrid catfish produced by mating female channel catfish with male blue catfish are the most important. Catfish production is the largest of U.S. finfish aquaculture, valued at approximately $423 million in 2012, and the economic impact of catfish industry is $2.5 billion (Dunham and Elaswad, 2018). However, the catfish production declined in recent years due to various reasons including fierce international competition, increased costs of fish feed, and disease problems. Bacterial diseases cause major problems for the U.S. catfish industry. In particular, three bacterial diseases including enteric septicemia of catfish (ESC), columnaris disease, and motile Aeromonas septicemia (MAS) are the primary disease concerns (Wagner et al., 2002; Plumb and Hanson, 2011). In addition to disease problems, abiotic stresses such as high water temperature and low levels of dissolved oxygen also lead to significant economic losses (Burggren and Cameron, 1980; Ficke, 2005; Welker et al., 2007).
Huge efforts have been devoted to reducing the economic losses including development of vaccines, and generation of disease resistant and stress tolerant catfish strains and families. For the later, great genetic variations among catfish species and strains in response to diseases and stresses provide biological sources for the analysis of genetic and epigenetic regulation of disease resistance. After the publication of the channel catfish reference genome sequence (Liu et al., 2016), rapid progress has been made for the determination of QTL associated with disease resistance (Geng et al., 2015; Zhou et al., 2017b; Shi et al., 2018; Tan et al., 2018) and stress tolerance (Jin et al., 2017; Wang et al., 2017b; Zhong et al., 2017).
In this paper, we review progress made in understanding pathogenesis, host responses, and genomic basis for disease resistance of ESC, columnaris, and Aeromonas diseases, as well as for abiotic stress such as heat stress, and hypoxia.
Part I: Enteric Septicemia of Catfish (ESC)
Impact of ESC Disease to the Catfish Industry
Enteric septicemia of catfish disease is caused by pathogen Edwardsiella ictaluri. It is one of the most serious diseases of catfish. The disease was first reported in 1976 (Hawke et al., 1981). Direct economic loss to the U.S. catfish industry due to ESC disease was estimated to be approximately $60 million annually. The disease affects all size classes of catfish including market size fish, and causes high mortality. About 19.3% of fingerling catfish and 36.6% of adult catfish had ESC disease problem as revealed by a survey in 2010 (APHIS, USDA).
Enteric septicemia of catfish outbreaks occur when water temperature is between 20 and 30°C. The symptoms of ESC include red and white ulcers; red spots under their heads or belly regions; and red pimples at the cranial foramen between the eyes that can from a “hole-in-head” condition (Hawke et al., 1998).
Vaccine for ESC Disease Control
Vaccines for ESC have been researched for over three decades (Saeed and Plumb, 1986; Newman, 1993; Thune et al., 1994). The modified live E. ictaluri isolate RE-33 was the most successful vaccine. This isolate is not pathogenic but stimulate protective immunity (Klesius and Shoemaker, 1999; Shoemaker et al., 1999). The vaccine was commercialized by Intervet, Inc. The vaccine can be delivered through immersion, and the product is marketed under AQUAVAC-ESC (Intervet, Inc., Millsboro, DE, United States). The immersion studies reported successful protection in channel catfish fingerlings (Wise et al., 2000), and eggs (Shoemaker et al., 2002, 2007).
Additional studies for new vaccines for ESC disease are still being carried out. The E. ictaluri purA mutant (Lawrence et al., 1997), E. ictaluri ghost generated by PhiX174 lysis gene E (Wang et al., 2016), E. ictaluri outer membrane proteins (Yang Q. et al., 2016), ESC-NDKL1 generated by modification of FRDA gene in a gcvP-sdh mutant (Nho et al., 2017) and E. ictaluri HemR mutants (Abdelhamed et al., 2018) were constructed, and demonstrated to be potential vaccines against ESC in channel catfish. The immersion-based vaccines are typically used for mass delivery with very young fish whose immune system is not fully developed. An oral vaccine for channel catfish fingerlings was developed by attenuation of E. ictaluri isolate (S97-773). This oral vaccine increased antibody production by 18-fold, improved survival, and increased feed conversion efficiency and total harvest in pound trails (Wise et al., 2015). Additional evaluation confirmed that this vaccine could improve survival rate of channel catfish after exposed to E. ictaluri (Peterson et al., 2016).
The application and effectiveness of vaccine for ESC was also assessed by catfish producers. During 1989–1990, no obvious effects were observed after ESC vaccination. With the development of vaccine-oil emulsion coating method in 1990–1991, the overall survival rate in vaccinated fish was significantly improved (Thune et al., 1994). A survey indicated that 12.3% of the industry catfish fry was vaccinated against ESC, and 6.7% vaccinated catfish was used by the food-sized catfish producers during 2009 (Bebak and Wagner, 2012). About 41.9% of the producers that stocked ESC-vaccinated catfish believe that survival rate was improved in vaccinated fish. However, 37.5% of producers did not know whether vaccination changes survival rates (Bebak and Wagner, 2012). Apparently, ESC vaccines were quite effective in laboratories but were not consistently effective under the commercial pond environment (Dunham and Elaswad, 2018). Therefore, future studies are required to demonstrate the effectiveness of vaccines in commercial settings.
Pathogenesis of E. ictaluri
The first step for E. ictaluri pathogenesis is interacting with potential susceptible cells, crossing mucosal barriers, and entering the host. The E. ictaluri infects channel catfish by utilizing host cellular transport systems to traverse through the epithelial-lined tissues, such as intestine and olfactory mucosa (Skirpstunas and Baldwin, 2002). The intestinal epithelial cells uptake E. ictaluri with the involvement of actin polymerization and endocytosis mediated by receptors (Skirpstunas and Baldwin, 2002). An RNA-Seq analysis of channel catfish intestinal immune response after E. ictaluri infection provided additional evidence for the involvement of the actin cytoskeletal polymerization remodeling in E. ictaluri infection (Li et al., 2012). Pathogenic members of the Enterobacteriaceae are commonly using receptor mediated endocytosis for entering host cells (Finlay and Falkow, 1989; Finlay et al., 1989). As an important member of Enterobacteriaceae, the infection mechanisms utilized by E. ictalrui are speculated to be similar: Cytochalasin D binds to actin resulting in microfilament depolymerization, which lead to the cell morphology alteration and bacterial adherence and internalization (Ewanowich and Peppler, 1990; Skirpstunas and Baldwin, 2002). The oligo-polysaccharide (OP-S) subunits of the bacterium E. ictaluri play a major role during pathogenesis (Santander et al., 2014). Recently, it was reported that NCK1 may play a key role in the E. ictaluri pathogenesis by initiating actin pedestal formation, and linking the surface located ligand and host cell receptors (Zhou et al., 2017b).
Once passing the epithelial barrier, the bacterium was believed to be taken up by propria macrophages (Miyazaki and Plumb, 1985). Normally, macrophages have a crucial role in resisting bacterial infections by migrating to the infection site, engulfing and killing pathogens (Kordon et al., 2018). However, numerous intracellular E. ictaluri were observed within clear vacuoles in the infected macrophage cultures of catfish (Booth et al., 2006), indicating that E. ictaluri could survive in channel catfish macrophages. The E. ictaluri in macrophages is then spread systemically through the bloodstream.
Variation of ESC Disease Resistance of Catfish
Enteric septicemia of catfish disease resistance of catfish is highly variable among species and strains from different geographic locations (Dunham and Elaswad, 2018). In general, blue catfish is more tolerant to ESC than channel catfish, although significant difference in channel catfish ESC resistance was found among various strains and families (Wolters and Johnson, 1994; Wolters et al., 1996; Tucker and Hargreaves, 2004). Red river strain channel catfish showed highest ESC resistance, followed by Mississippi-select and Mississippi-normal fish (Wolters and Johnson, 1994). Hybrids of Norris female channel catfish and male blue catfish had intermediate resistance compared to blue catfish or channel catfish (Wolters et al., 1996; Hawke et al., 1998), while hybrids of NWAC103 channel catfish and blue catfish showed lower mortality than either parental species when exposed to natural diseases of ESC (Dunham et al., 2008), suggesting that combination of strains and families of the parent species influence disease resistance of the hybrids. Hybrid catfish have become common in the catfish production industry in the United States, now make up 50–70% of all catfish production (Dunham and Elaswad, 2018). Therefore, analysis of resistance among various hybrid catfish becomes important. In addition to production importance, the interspecific hybrid catfish offers an ideal system for the study of disease resistance by take advantage of the phenotypic contrast of channel catfish and blue catfish (Geng et al., 2015).
Transcriptome Responses of Catfish After E. ictaluri Infection
Microarrays were initially used for the analysis of the transcriptome variance in channel catfish liver after the E. ictaluri infection. A set of genes were found to be differentially expressed after infection, particularly for the genes related to iron homeostasis (Peatman et al., 2007). Efforts were made to understand the molecular basis for disease resistance of blue catfish. Analysis of gene expression differences in liver of blue catfish after E. ictaluri infection by using microarrays indicated significant induction of inflammatory immune response pathways (Peatman et al., 2008). In particular, several MHC class I pathway members were differentially induced in blue catfish after E. ictaluri infection.
RNA-Seq was conducted to determine the involvement of the intestinal epithelium during E. ictaluri infection (Li et al., 2012). The results reveled that actin cytoskeletal polymerization, remodeling, and junctional regulation were important processes for E. ictaluri entry. A total of 1,633 genes were identified to be differentially expressed between E. ictaluri infected and control samples of channel catfish. After that, bulked segregant RNA-Seq (BSR-Seq) for the liver of hybrid catfish after E. ictaluri challenge identified a set of 1,255 differentially expressed genes between the resistant and susceptible catfish, and 8 linkage groups (LG) that contain QTL for ESC disease resistance (Wang et al., 2013). Many of the up-regulated genes in the susceptible hybrid catfish involve in the acute phase responses, as reported in the microarray analysis (Peatman et al., 2007, 2008). Similarly, RNA-Seq analysis of yellow catfish (Pelteobagrus fulvidraco) after E. ictaluri infection revealed a total of 5,527 differentially expressed genes, and the subsequent enrichment analysis revealed the involvement of innate and adaptive immune pathways (Zhu et al., 2017).
QTL Analysis of ESC Disease Resistance
It was technically much easier to determine the genomic basis of ESC disease resistance after the publication of channel catfish reference genome sequence (Liu et al., 2016). Initially, a genome-wide association study (GWAS) was conducted with the fourth generation of backcross catfish progenies by using the 250K SNP array; a significant QTL on LG 1, and two suggestive QTL on LG 12 and 16 were identified to be associated with ESC disease resistance of catfish (Zhou et al., 2017b) (Table 1). A set of 16 genes with immune related functions were identified within the significant QTL on LG 1. Among them, the NCK1 gene was significantly upregulated in the intestine of channel catfish after E. ictaluri infection (Zhou et al., 2017a), and was considered as a possible candidate gene that confers ESC susceptibility, presumably by serving as an adaptor facilitating the pathogen entry into the host cells, similar to the situation of enteropathogenic Escherichia coli (EPEC) in humans (Zhou et al., 2017b).
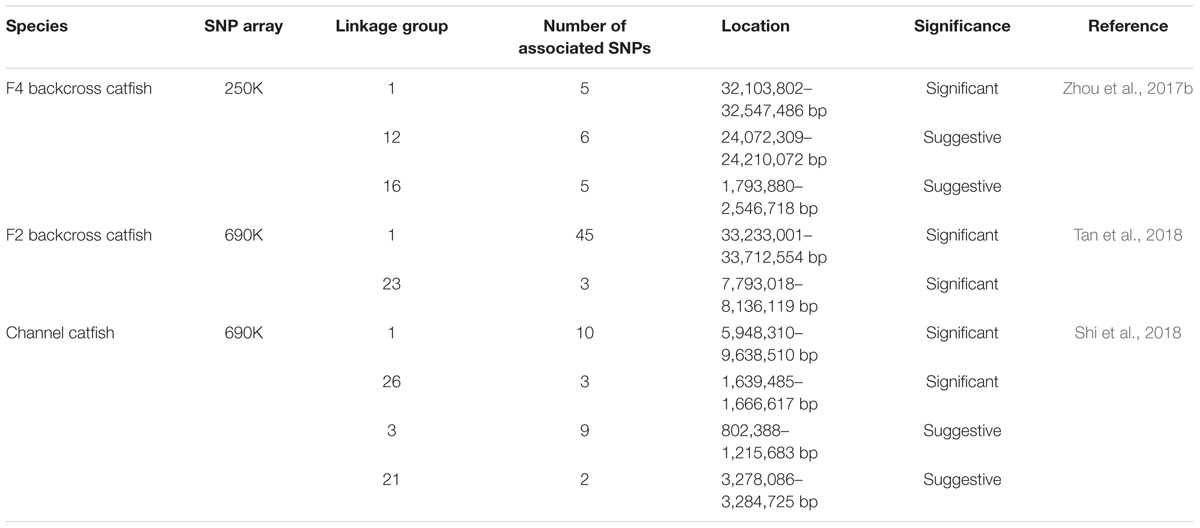
TABLE 1. Summary of the QTL associated with ESC disease resistance of catfish as revealed by genome wide association studies.
Following that initial work, GWAS analysis was conducted in second generation of backcross catfish progenies by using the 690K SNP arrays (Zeng et al., 2017; Tan et al., 2018). Two QTL on LG 1 and LG 23 were identified to be significantly associated with ESC resistance (Table 1). Notably, the same genomic region on LG 1 was identified in both of the second and the fourth generation of backcross catfish progenies.
Most recently, GWAS analysis was conducted in channel catfish by using the 690K SNP array (Shi et al., 2018). Two QTL on LG 1 and one QTL on LG 26 were significantly associated with ESC resistance. Besides, three QTL located on LG 1, 3 and 21 respectively, were suggestively associated with ESC resistance (Table 1). Again, this study validated the QTL on LG 1 previously identified using the second and the fourth-generation backcross progeny populations. Taken together, it suggests that there are only a few major QTL associated with ESC disease resistance, making marker-assisted selection a possibility for genetic enhancement of ESC resistance.
Gene Families Involved in ESC Disease Responses
RNA-Seq data were utilized to further investigate various gene families in channel catfish during E. ictaluri infection. Many gene families were induced after E. ictaluri infection, including heat shock proteins (Song et al., 2014, 2016; Xie et al., 2015), interlekin 17 (Wang X. et al., 2014), l-rhamnose-binding lectins (Thongda et al., 2014), nitric oxide synthase genes (Yao et al., 2014), claudins (Sun et al., 2015), peptidoglycan recognition proteins (Sun et al., 2014), Rab GTPases (Wang R. et al., 2014), Rho GTPase (Tan et al., 2017), suppressors of cytokine signaling genes (Yao et al., 2015), cytochrome P450 genes (Zhang et al., 2014), serpins (Li et al., 2015), bcl-2 genes (Yuan et al., 2016), cathepsins (Geng et al., 2015; Wang et al., 2015; Dong et al., 2016), tumor suppressor genes (Mu et al., 2015), complement regulatory protein genes (Jiang et al., 2015), septins (Fu et al., 2016), chemokines (Fu et al., 2017a,b,c), galectins (Zhou et al., 2016), apolipoproteins (Yang et al., 2017), receptor tyrosine kinases (Tian et al., 2015), NF-kB related genes (Wang et al., 2017c), phosphoinositide-3-kinases (Li et al., 2016), NCK and ABI adaptor genes (Zhou et al., 2017a), JAK and STAT genes (Jin et al., 2018). These studies indicated that most of the immune related genes were involved in the E. ictaluri disease responses. However, the regulatory network of immune system especially for those induced genes families is still unknown.
Future Research of ESC Disease
Although research need to be done in multiple dimensions to understand and control ESC disease, the most promising ways are vaccine development and testing, and genetic enhancement of disease resistance. All studies of vaccines in the laboratory have shown their effectiveness and efficacy, but field studies, especially the timing, dose, and methods of vaccine application need to be determined for field conditions. Genetic studies of disease resistance indicated that only a limited number of QTL are involved in disease resistance against ESC disease. If validated, marker-assisted selection can be a quite effective approach for the enhancement of disease resistance. However, industry involvement is essential. With the known QTL information, it is now possible to determine the level of disease resistance of various lines used in the catfish industry. Introgression of the superior disease resistance QTL from blue catfish is a realistic approach to develop disease resistant catfish breeds.
Part Ii: Columnaris Disease
Impact of Columnaris Disease on Catfish Aquaculture
The columnaris was reported in 1922 from freshwater fish in Mississippi River (Davis, 1922), the threat of columnaris disease was one of the most significant risk to the aquaculture, particularly in the warm summer temperatures (Pacha, 1970; Becker and Fujihara, 1978). Although the pathogen Flavobacterium columnare is ubiquitous in the freshwater environment and can cause catastrophic mortalities in both wild as well as domesticated species (Schachte, 1983; Decostere et al., 1998; Morley and Lewis, 2010), channel catfish and blue catfish are especially vulnerable to F. columnare infections. The disease is often initiated as an external infection on the body surface, fins or gills, and subsequently developed into yellow-orange lesions along the dorsal midline, known as “saddleback” (Bullock et al., 1986; Plumb, 1999). In extreme conditions, the mortality for channel catfish fries after columnaris infection can reach up to 90%. The mortality rate for pond raised channel catfish can also reach as high as 50–60% (Decostere et al., 1998; Morley and Lewis, 2010; Plumb and Hanson, 2011), causing annual economic loss of approximately $30 million for the U.S. catfish industry (Shoemaker et al., 2011).
Pathology and Host Specificity of Columnaris in Catfish
Flavobacterium columnare was originally named Bacillus columnaris and was renamed as F. columnare in Bernardet et al. (1996). It belongs to Flavobacteriaceae family; it is Gram negative, with smooth and yellowish colonies (Anacker and Ordal, 1955; Kunttu et al., 2009; Noga, 2011; Roberts, 2012). The F. columnare bacteria is rod shaped with 4–10 μm in length and 0.3–0.5 μm in width. F. columnare is featured with its rhizoid pattern on the low agar medium and its ability to grow on neomycin and polymixin B containing media (Griffin, 1992; Plumb, 1999). F. columnare can secret various of extracellular enzymes and is endowed with the ability to degrade polysaccharide, destructing gills, muscle and skin (Bernardet and Bowman, 2006). Based on restriction fragment length polymorphism (RFLP) analysis of the 16S rDNA, the F. columnare in North America can be assigned into three major categories (Wakabayashi, 1999). Among them, the genomovar I is more virulent in cold water species (Evenhuis and LaFrentz, 2016); genomovar II isolates are more lethal to the warm water species, such as catfish or bluegill (Triyanto, 1999; Arias et al., 2004; Darwish and Ismaiel, 2005; Olivares-Fuster et al., 2007; Shoemaker et al., 2008; Bullard et al., 2013; Mohammed and Arias, 2014). The pathogenesis of columnaris still remains largely unknown (Declercq et al., 2013). It is known that the adhesion ability, especially to the gills, is of vital importance to infection. The adhesion ability of F. columnare is affected by various factors such as the concentration of nitrite, organic matter or temperature (Decostere et al., 1999; Decostere et al., 2002). Thus, the outbreaks of columnaris disease is heavily dependent on the environmental factors such as the temperature, pH and hardness of water. The optimal temperature for the bacteria growth is 20–25°C (Bullock et al., 1986). In aquaculture productions, the risk for the columnaris is associated with the environmental stress; the risk increases with higher temperature, higher feeding rates, more organic loads as well as the increasing stocking densities (Olivares-Fuster, 2010). However, the decreasing of water hardness as well as the pH can suppress the spreading of F. columnare in the water (Fijan, 1968).
High levels of genetic variations exist among catfish with regard to their resistance against columnaris disease. For example, some channel catfish stocks are highly susceptible to columnaris infections such as the Rio Grande and Rio Grande S. stock (Dunham and Smitherman, 1984). Although channel catfish and blue catfish are both susceptible to F. columnare (Dunham et al., 1994), blue catfish is more susceptible to the disease in general. Hybrid catfish exhibit significant heterosis in terms of columnaris resistance (Birchler et al., 2003).
Columnaris Vaccines for Catfish
Large efforts have been devoted to developing columnaris vaccines. Immersion with formalin-killed bacteria of channel catfish can provide a low level of protection from columnaris (Moore et al., 1990). Fryvacc 1 and Fryvacc 2 vaccines were developed and licensed for the control of F. columnaris (Bondad-Reantaso et al., 2012). The AQUAVAC-COL vaccine, developed from genomovar I and launched in 2005 (Arias et al., 2004), was demonstrated to be safe and effective to protect catfish from the infection of columnaris (Shoemaker et al., 2005, 2006, 2007, 2011). In addition, the AQUAVAC-COL can also protect other species such as largemouth bass from columnaris (Bebak et al., 2009). Gene expression analysis indicated that treatment with AQUAVAC-COL vaccine triggered expression of genes with the functions in cell maintenance of the host, immune responses, signal transduction and transcriptional regulation (Pridgeon and Klesius, 2010). However, AQUAVAC-COL is ineffective against genomovar II of columnaris. Most recently, a new vaccine (17-23), derived from attenuated genomovar II, was developed (Olivares-Fuster and Arias, 2011; Mohammed et al., 2013). RNA-seq analysis indicated that this vaccine can enhance the mucus system of fish and stimulate eosinophilic granular cells and the expression of neuroendocrine cells mediators in the fish gill (Zhang et al., 2017).
Molecular Mechanisms of Columnaris Resistance
RNA-Seq analysis after F. columnare infection was conducted in catfish to identify transcriptome level of responses after infection. Since the gill is the primary organ for F. columnare adhesion and entry into the host (Decostere et al., 1999; Olivares-Fuster et al., 2011), RNA-Seq analysis of gene expression profiles was mostly conducted in gill tissues. Among the induced genes, rhamnose-binding lectin was significantly induced, along with down regulation of NF-κB signaling pathway (Sun et al., 2012). Previous researches had indicated that rhamnose-binding lectin has important role in the innate immune response (Watanabe et al., 2009). It functions as germline-encoded pattern recognition proteins by recognizing the lipopolysaccharides and lipoteichoic acid on the surface of Gram-negative and Gram-positive bacteria, respectively (Shiina et al., 2002; Tateno et al., 2002). The regulation of rhamnose-binding lectin during F. columnare infection suggested its potential role in bacterial aggregation and attachment (Beck et al., 2012). In addition, the comparisons between susceptible and resistance channel catfish transcriptome allowed the identification of the potential candidate genes associated with disease resistance. Some of the differentially expressed genes included inducible nitric oxide synthase 2b, lysozyme C, interleukin-8, and tumor necrosis factor-alpha, all of which were higher expressed in tolerant catfish than that in intolerant catfish. On the contrary, rhamnose-binding lectin was found to be induced in susceptible catfish than in resistant catfish (Peatman et al., 2013), suggesting association of high expression of rhamnose-binding lectin with susceptibility.
Genome-wide association study was conducted using backcross hybrid catfish (Geng et al., 2015). A 620-Kb region on LG 7 was observed to be significantly associated with columnaris resistance. Moreover, three additional QTL were identified on LG 7, 12 and 14. Interestingly, analysis of genes within the above identified QTL regions involve genes related to phosphoinositide 3-kinase pathway such as PIK3R3b, CYLD-like, ADCYAP1R1, ADCYAP1R1-like, and MAST2. Previous research indicated that phosphoinositide 3-kinase pathway are actively involved in the immune response, and 8 of 14 phosphoinositide 3-kinase genes were regulated after F. columnare infections in channel catfish (Li et al., 2016), suggesting the importance of phosphoinositide 3-kinase pathway for disease resistance of catfish against columnaris infections.
Part Iii: Aeromonas Disease
An Emerging Disease but Serious Threats to the Catfish Industry
Motile aeromonad septicemia (MAS), caused by the Gram-negative bacterium Aeromonas hydrophila, is an emerging disease threatening the catfish industry (Pridgeon and Klesius, 2011; Hossain et al., 2014; Pang et al., 2015). A. hydrophila is a mesophilic, rod-shaped, Gram-negative bacterium. The pathogen is able to infect a large number of hosts including amphibians, reptiles, avians, and mammals (Krieg and Holt, 1984), especially fish species (Ventura and Grizzle, 1987). Various fish species can be infected by A. hydrophila, including eel (Anguilla anguilla) (Esteve et al., 1994), Nile tilapia (Oreochromis niloticus) (Abd-El-Rhman, 2009), goldfish (Carassius auratus) (Harikrishnan et al., 2009), common carp (Cyprinus carpio) (Harikrishnan et al., 2003; Jeney et al., 2009; Robinson et al., 2012), and channel catfish (Hemstreet, 2010; Pridgeon and Klesius, 2011). MAS has been reported to cause huge economic losses in aquaculture. For instance, the cyprinid fish industry in central China was seriously impacted by MAS since 1989 (Zhang et al., 2014). The disease was first reported in the southern United States in the catfish industry during the summer of 2009, causing the loss of millions of pounds of food-size channel catfish (Hemstreet, 2010). The MAS outbreak was etiologically determined to be caused by a new virulent strain of A. hydrophila (Tekedar et al., 2013). Since the initial outbreak in 2009, the virulent A. hydrophila has spread to other states, including Mississippi and Arkansas (Pridgeon and Klesius, 2011), leading to an estimated loss of at least 12 million U.S. dollars (Hossain et al., 2014).
Pathogenesis of A. hydrophila
The symptoms of A. hydrophila infection include sores on the skin, dropsy, necrosis, ulceration, and hemorrhagic septicemia (Pridgeon and Klesius, 2011). The pathogenicity of A. hydrophila is multifactorial (Pang et al., 2015). Many virulence factors have been identified, including secretion systems, motility and adhesins, toxins, enzymes, quorum systems, iron acquisition and antibiotic resistance (Tomás, 2012). The genomic sequence of A. hydrophila provided an insight into the pathogenicity (Pang et al., 2015). Pang et al. (2015) sequenced genomes of A. hydrophila strains and performed comparative genomic analyses. They found that utilization pathways for myo-inositol, L-fucose and sialic acid were associated with the virulent nature of epidemic A. hydrophila.
Resistance of Catfish Against Aeromonas Disease
Similar to the performance in resistance to ESC and columnaris diseases, catfish exhibit a high level of variations in resistance against the MAS disease. Such variations are both interspecific and intraspecific between and within channel catfish and blue catfish. Blue catfish is observed to be more resistant to virulent A. hydrophila than either channel catfish or channel catfish × blue catfish hybrid catfish (Li et al., 2013). Moreover, the hybrid catfish was observed to be more resistant than channel catfish to A. hydrophila (Dunham and Masser, 2012). However, detailed analysis of variations among various channel catfish strains and blue catfish strains have not yet been conducted.
Molecular Mechanisms Under Aeromonas Disease Resistance
Transcriptomic analyses following infection with A. hydrophila have been conducted in channel catfish and blue catfish. In one experiment, 60K Agilent microarrays were utilized to determine gene expression profiles in the skin of channel catfish and blue catfish after infection with virulent A. hydrophila (Li et al., 2013). In channel catfish, a total of 2,168 genes were significantly regulated after the bacterial challenge. These differentially expressed genes were involved in various pathways including immune, antioxidant, cytoskeletal, and nervous system pathways. Similarly, a set of 1,155 genes were differentially expressed in blue catfish after A. hydrophila infection. Dysregulation of genes was reported for genes involved in immunity, antioxidant responses, apoptosis, and cytoskeletal rearrangement. In addition to analysis in catfish, analysis of differently expressed genes in grass carp (Ctenopharyngodon idellus) infected with A. hydrophila revealed that they were implicated in complement system, cell adhesion molecules, apoptosis, and antioxidant responses. The similar functional pathways in different fish species after the same bacterial infection suggest evolutionary conservation of the molecular mechanisms of host response, and perhaps disease resistance (Yang Y. et al., 2016).
MicroRNAs (miRNAs) play significant roles in gene regulation by binding to complementary regions of mRNA to induce degradation or repress translation (Bartel, 2004). The identification of conserved and novel miRNAs was reported in catfish (Barozai, 2012; Xu et al., 2013), while the differential expression analysis of miRNAs after A. hydrophila infection has not been performed. Such analyses have been performed in other teleost fish species, contributing to a better understanding of the functional role of miRNAs in immune responses. For instance, 21 and nine miRNAs were found to be differentially expressed between A. hydrophila-susceptible and A. hydrophila-resistant grass carp in spleen and kidney, respectively (Xu et al., 2014, 2015). Results showed that miRNA targets were enriched in various physiological processes including immune system process.
QTL for Aeromonas Disease Resistance
Identification of QTL and markers associated with disease resistance is of great significance because such information can be used for genetic improvement of broodstocks through breeding. In rohu (Labeo rohita), 21 SNPs on 10 LG were reported to be associated with the timing of death after A. hydrophila infection (Robinson et al., 2014). Genes of interest were identified that may be related to the observed QTL, including heat shock proteins, mucin, lectin, major histocompatibility loci, complement protein components, perforin, ubiquitin, T-cell antigen receptor and lymphocyte specific protein tyrosine kinase. To select for improved resistance to A. hydrophila, immune parameters were measured in resistant and susceptible lines of rohu to identify associated immune markers. The expression levels of ceruloplasmin and serum ceruloplasmin were found to be significantly higher in the resistant line over the susceptible line, suggesting the possibility of using immune parameters as indirect selection markers for disease resistance. In our laboratory, research for the identification of Aeromonas disease resistance QTL in catfish is underway, but unfortunately, grant funds are lacking to continue this important project.
Part Iv: Heat Stress
The Effects of Heat Stress on Catfish Industry
Stress caused by sudden changes in temperature or chronic heat stimuli above optimum conditions can interrupt cellular homeostasis and result in serious deficiency in development and growth, and even death. The global climate change is believed to affect all organisms in the ecosystems (Pörtner and Peck, 2010). In the freshwater systems, climate change is likely to increase water temperature, decrease dissolved oxygen and increase toxicity of pollutant (Ficke et al., 2007). Therefore, for the fish living in natural or artificial systems, global climate change will become a more significant stressor as it worsens over time. As a major factor of seasonal environment, water temperature experiences daily fluctuations and erratic changes. Physiologically, aquatic ectotherms has evolved to adapt to a limited scope of environmental variation, and living over that scope can be stressful or fatal (Roessig et al., 2004). The magnitude and direction of the temperature fluctuation, frequency of temperature change can significantly affect their life history (Todd et al., 2008). A variety of physiological functions such as growth, metabolism, reproduction success, food consumption, and the capacity to maintain internal homeostasis capacity of aquatic species will be affected in response to temperature fluctuation (Pörtner and Peck, 2010).
Catfish species have a wide range of natural habitats, and harbor great plasticity when they encounter temperature variations. Water temperatures in aquaculture ponds currently approach upper thermal tolerance levels for channel catfish, particularly in June-August, which routinely see daily maximum values of 29°C and higher (Arnold et al., 2013). Production rate in catfish ponds could be decreased due to reduced dissolved oxygen levels and an increased virulence of pathogens caused by high temperature. A drop in dissolved oxygen levels can lower the management capacity of water from uneaten feed, fecal matter, and fish metabolism, which could lower the reproductive capacity of catfish ponds (Ficke, 2005).
Heat stress also adversely affect growth, reproduction, and survival of channel catfish (McCauley and Beitinger, 1992; Arnold et al., 2013; Stewart et al., 2015). Three cycling upper-range temperature regimes (23–27°C, 27–31°C, and 31–35°C) of aquaculture environments affected the growth, and feeding performance of juvenile channel catfish (Arnold et al., 2013). The survival rate of catfish was significantly decreased for individuals under the highest temperature regime. Also, the catfish growth rate was decreased. Therefore, increased water temperature may present major challenges to the catfish industry (Arnold et al., 2013).
Heat Tolerance Between Channel Catfish, Blue Catfish and Hybrid Catfish
In United States, the majority of catfish production are in the southeast (92%), where some of the warmest temperatures are found (Stewart et al., 2015). Channel catfish has a natural distribution from southern Canada to northern Mexico, which represents a temperature range from 5 to 35°C (Bennett et al., 1998; Tavares-Dias and Moraes, 2007; Stewart et al., 2015). Blue Catfish are distributed further south, spanning from the Mississippi River basin and Gulf Coast through Mexico and into Guatemala and Belize (Graham, 1999). In general, blue catfish has a higher capability of heat tolerance than channel catfish. The hybrid catfish also have a higher level of heat tolerance than channel catfish (Stewart et al., 2015). It was reported that the optimum water temperature for channel catfish best growth performance ranging from 27 to 32°C (Stewart et al., 2015). However, the Southeastern U.S. ponds reach daily maximum as high as of 34–36°C with daily fluctuations averaging 4°C in May–August (Arnold et al., 2013), indicating that aquacultured catfish is under constant heat stress.
Knowledge about the heat tolerance between different channel catfish strains, blue catfish strains and their hybrid catfish is very limited. It was first found that little to no geographic variation in incipient upper lethal temperature (IULT) of Channel Catfish from Florida and Ohio (Hart, 1952). However, the sample size for this study was small. Thereafter, critical thermal maximum (CTmax) was used to examine the thermal sensitivity of catfish to acute temperature fluctuations (Bennett et al., 1998). It was observed that the CTmax ranged from 38.6°C to 40.3°C for two geographically distinct strains of channel catfish. Catfish with a southern distribution (Delta Select strain, from the Mississippi Delta, MS, United States) showed a higher CTmax than catfish with a northern distribution (Red River strain, from the Red River, ND, United States) (Stewart and Allen, 2014). In hybrid catfish, geographic differences in thermal tolerance were observed as well, providing an evidence of genetic component for thermal tolerance in catfish.
The Molecular Mechanisms Underlying Heat Stress in Catfish
Research using several model species revealed that a series of evolutionarily conserved stress-associated genes display distinct expression for heat stress, including genes involved protein folding and repair, protein degradation and biosynthesis, energy metabolism, cell cycle and signaling, cytoskeletal reorganization and apoptosis (Kültz, 2005; Logan and Somero, 2011; Liu et al., 2013). Increasing the levels and magnitudes of stress sequentially can lead to different components of the stress response (Feder and Hofmann, 1999; Kültz, 2005; Logan and Somero, 2011; Logan and Buckley, 2015). For instance, under mild heat stress, chaperone proteins could be highly expressed to refold proteins to maintain protein homeostasis (Feder and Hofmann, 1999). Under moderate levels of heat stress, many abnormal folded proteins will be degraded by proteolysis processes. Additionally, under sever heat stress conditions, basal cell activities may be induced because of the cellular damage. Such as the cell proliferation and cytoskeletal reorganization may be up-regulated, which has attendant effects on cellular structure and function. Furthermore, when suffering severe heat stress, significant cell damage will lead to induction of apoptotic pathways (Buckley et al., 2006; Logan and Somero, 2011).
The first described proteins in relation to heat stimuli are heat shock proteins (HSPs), which are a class of proteins that are produced by cells in response to environmental stresses (Zhu et al., 2016). Several heat shock proteins are play function as intra-cellular chaperons to fold and unfold other proteins under heat stress, oxidative and other cellular stimuli (Buckley and Hofmann, 2004). In catfish, some of heat shock genes were first characterized in response to heat. The heat-shock protein (stress-70 family) was isolated from liver of channel catfish in Abukhalaf et al. (1994). Stress-70s from tissues of several fish species contain common antigenic determinants of the protein (Abukhalaf et al., 1994). It has been suggested that expression levels or accumulation of this protein could be a useful predictor of a stressful environment. Increased expression of stress-70s were detected in stressed fish (Welch, 1990). Straight after, the cDNA sequence of a member of heat shock protein 70 family in channel catfish was identified in 1996. The CF Hsp70 mRNA expression in three leukocyte cell lines was examined as well (Luft et al., 1996). At optimal culture temperature (27°C), the CF Hsp70 mRNA was constitutively expressed at high levels. However, at heat shock condition (37°C), only a modest up-regulation of CF Hsp70 expression was detected (Luft et al., 1996).
In order to obtain a broad understanding of gene expression profiles under heat stress in catfish, RNA-Seq was conducted in hybrid catfish (Liu et al., 2013). In this study, samples of gill and liver from heat sensitive, tolerant and control catfish groups were used for conducting RNA-Seq. In total, 2,260 differentially expressed genes were identified between control fish and heat sensitive and/or heat resistant fish following heat stress. The differentially expressed genes were classified into six functional groups, including protein folding, protein degradation, protein biosynthesis, energy metabolism, molecule and ion transport, and cytoskeleton reorganization (Liu et al., 2013). Especially, the dramatically up-regulated genes are those participated in protein folding and degradation, oxygen transport, and metabolic process. Whereas, the lethal heat stress (37°C) in this study caused significant reduction of expression levels of genes encoding for general protein synthesis. Gene encoding for molecular chaperones were most strongly up-regulated. The chaperones are critical in stabilizing protein homeostasis under heat stress in order to avoid aggregation and degradation of proteins (Parsell and Lindquist, 1993). For example, members of the HSP40 family (DNAJA1, DNAJA4, and DNAJB1B), HSP70 proteins (HSPA5, HSPA1A, and HSC73L), and the HSP90 family (HSP90AA1, HSP90AA2, HSP90AB1, and HSP90B1) were significantly upregulated after heat challenge. In spite of the protein folding and rescue process participated by chaperone proteins and related factors, some damaged proteins that cannot be rescued by chaperones are degraded by either autophagy-lysosomal pathway or ubiquitin proteasome pathway (UPP). Numerous proteases were significantly induced in this study, such as cathepsins and legumain. In comparison, heat stress caused reduced expression levels for several genes involved in UPP proteolysis process, demonstrating the downregulation of the ATP-dependent proteolysis way (Liu et al., 2013). Genes with functions in transporting lipids, proteins, iron and glucose were identified after heat stress in catfish (Liu et al., 2013). As expected, the expression of genes involved in regulating metabolism and repair system showed up regulation in response to heat, because these processes are energy-costing. However, several genes in relation to respiratory chain were down-regulated, such as genes coding for mt-ND1, mt-ND2, mt-ND6, and COX2. It’s well recognized that heat stress preferentially leads to upregulation of specific stress-related genes while downregulation of general genes involved in protein synthesis (Buckley and Hofmann, 2002; Buckley et al., 2006). Similarly, our study observed significantly reduction of ribosomal protein genes in gills after heat stress stimuli as well. In addition to affecting the internal cellular activities, heat stress can induce the expression of several cytoskeleton-associated proteins, including G3BP2, TPM4, MMP9, MMP13, MMP18, COLLA1A, and COLLA1B.
QTL Associated With Heat Stress in Catfish
Genome-wide association study analysis has been conducted to detect QTL for heat stress tolerance. With a well-assembled reference genome sequence, potential candidate genes within QTLs can be identified simultaneously, which is a useful way to understand the molecular mechanisms of a trait by identification of genes in proximity to QTL (Dikmen et al., 2013; Geng et al., 2015). As described above, GWAS was conducted to identify QTL associated with several important traits, including disease resistance for columnaris (Geng et al., 2015) and ESC (Zhou et al., 2017b; Shi et al., 2018; Tan et al., 2018), growth rate (Li et al., 2017), head size (Geng et al., 2016), body conformation (Geng et al., 2017), and low oxygen tolerance (Wang et al., 2017b; Zhong et al., 2017). As the global climate change worsens over time, it is important to develop heat-tolerant lines. GWAS was conducted to identify heat stress-associated QTL, by using interspecific backcross progenies and the 250K catfish SNP array (Jin et al., 2017).
At the genome-wide significance level, three SNP markers were found to be associated with heat stress. One SNP was identified from LG 14, two additional SNPs were found from LG 16 (Jin et al., 2017). Around the three heat-related SNP markers, 14 heat stress associated genes were found. Among them, five genes (TRAF2, FBXW5, ANAPC2, UBR1 and KLHL29) are involved in the proteolysis process via the way of ubiquitination (Jin et al., 2017). This observation indicated that such lethal heat treatment resulted in irregular folding of, and irreversible disruption to, proteins, therefore, increased proteolytic degradation were required to remove the damaged proteins. However, observations from RNA-Seq analysis indicated that molecular mechanisms during heat stress is very complex; the levels and magnitudes of stress can lead to different components of the stress responses (Logan and Buckley, 2015). In the GWAS study, genes involved in protein biosynthesis, protein folding, molecule and iron transport, cytoskeletal reorganization and energy metabolism processes were also identified in the genome-wide significantly associated regions (Jin et al., 2017). The results further supported the notion that except for the involvement of intensive proteolysis, heat stress induces various molecular mechanisms to maintain cellular hemostasis (Liu et al., 2013; Jin et al., 2017).
Comparison of Molecular Responses of Catfish to Diseases and Heat Stress
The heat-shock response (HSR) is one of the highly conserved molecular responses to disruptions of protein homeostasis. HSPs are induced to protect the proteome from elevated temperatures. As mentioned above, HSPs are significantly induced under acute and mild heat stresses. Likewise, in response to acute stress from pathogen infection and hypoxia, HSPs are also induced. Because of the constitutive expression in non-stressed cells, HSPs are always been used as housekeeping proteins (Song et al., 2014). However, recent studies have revealed that several HSPs play essential roles in innate and adaptive immunity. For instance, HSPs play roles in regulate humoral immune responses; in extracellular environment, HSPs are considered to be a danger signal to trigger innate immune cells such as macrophages and dendritic cells (Srivastava, 2002). In catfish, families of HSP40, HSP60, HSP70, HSP90 and sHSP were characterized, and their expression profiles following E. ictaluri and F. columnare bacterial infection were determined. Pathogen-specific, tissue-specific and time-specific patterns were found of these heat shock genes after infection with the two diseases (Song et al., 2014, 2016; Xie et al., 2015). Most of the HSP genes were induced at the earliest stages of disease infections, while more and more HSP genes became down-regulated with the progression of the diseases.
Similarly, the up-regulation of claudin and cathepsin genes were detected after bacterial infection and heat stimuli by RNA-Seq studies. As a group of transmembrane proteins, claudins have significant functions in cytoskeletal reorganization (Sun et al., 2015). Claudin-1, a, e, i, were significantly induced after heat stress in catfish (Liu et al., 2013). Using existing RNA-Seq datasets, Sun et al. (2015) reported dramatic down-regulation of claudins at the earliest phase (3 h) of infection, suggesting that pathogens could interrupt the mucosal barrier via repressing activities of claudins (Sun et al., 2015). Cathepsins are a large group of proteases that serve as enzymes degrading damaged proteins to avoid forming cytotoxic aggregates. After heat stress, the expression of cathepsin Z, B, D, L were dramatically induced (Liu et al., 2013). After infection of E. ictaluri and F. columnare, expression of catfish cathepsin D, H, L, S genes were significantly induced. However, these genes displayed pathogen-specific or tissue specific patterns following infections, indicating their disparate roles or distinct tissue-specific functions in immunity (Yeh and Klesius, 2009; Feng et al., 2011; Wang et al., 2015; Dong et al., 2016). However, further functional studies are required to fully understand the roles of these genes after biotic and abiotic stresses.
Part V: Hypoxia
Impact of Hypoxia on the Catfish Industry
Dissolved oxygen is important for the survival and life of aquatic organisms. Under aquaculture conditions, hypoxia can be caused by natural phenomena (e.g., weather, temperature, or water flow rate), water pollution and eutrophication, high stocking density, and improper use of aeration (Wu, 2002; Zhang et al., 2010; Green et al., 2016). In spite of the high tolerance of catfish to low levels of oxygen, hypoxia can still lead to huge economic losses to the catfish industry. Exposure to low oxygen stress can affect growth rate (Mallya, 2007), feed conversion rate (Buentello et al., 2000), and increase the susceptibility to infectious diseases of catfish (Geng et al., 2014). Further, hypoxia can also negatively affects several additional aspects of biological behaviors such as increased vulnerability to predation (Domenici et al., 2007), reduced reproductive capacity (Landry et al., 2007), delayed embryonic development (Shang and Wu, 2004), and reduced metabolic rate (Richards, 2009).
Variation of Hypoxia Tolerance Among Catfish Species and Strains
In general, channel catfish has been believed to be more tolerant to low oxygen than blue catfish (Graham, 1999). In one experiment, the tolerance to low dissolved oxygen of six strains in channel catfish, including 103KS strain, Kansas strain, KMix strain, Marion strain, Marion S strain, and Thompson strain, was evaluated (Wang et al., 2017a). Compared with other channel catfish strains, two strains (103KS strain and Marion S strain) exhibited higher tolerance to low oxygen stress, whereas Marion strain of channel catfish showed the lowest tolerance to hypoxia stress (Wang et al., 2017a). Compared with their maternal parent (channel catfish) and paternal parent (blue catfish), interspecific hybrid catfish exhibited stronger tolerance to low dissolved oxygen levels (Dunham et al., 1983). The strains of their parents have an influence on the tolerance of hybrids to low dissolved oxygen (Dunham et al., 2014). For instance, at low temperatures (9 and 17°C), hybrid catfish sired by Alabama strains showed the highest tolerance to hypoxia stress than hybrid catfish sired by other strains of blue catfish (Dunham et al., 2014).
Molecular Mechanism on Hypoxia Tolerance in Catfish
Fish live in aquatic environments with low dissolved oxygen levels. They have evolved with various strategies to adapt to such environment, including their enzymatic activities, metabolic rate, and increased surface (gill lamellae) for oxygen exchange (Burggren and Cameron, 1980; Jensen et al., 1993). HIF-1 and HIF-2 are two important regulators for hypoxia responses and oxygen homeostasis (Semenza, 2000; Giannetto et al., 2015). After hypoxia treatment, the HIF genes were differentially expressed in catfish at the transcriptional levels as well, rather than just at the post-translational levels as believed previously (Geng et al., 2014). Several gene families were involved in the hypoxia response including receptor tyrosine kinases (RTKs) (Tian et al., 2015), bcl-2 genes (Yuan et al., 2016), CC and CXC chemokine families (including ligands and receptors) (Fu et al., 2017a,b,c), and claudin genes (Sun et al., 2015).
Multiple molecular pathways are involved in hypoxia responses. These molecular pathways included HIF signaling pathway (Bruick, 2003), PI3K-Akt signaling pathway (Mazure et al., 1997), MAPK signaling pathway (Koong et al., 1994), mTOR signaling pathway (Lu et al., 2006), VEGF signaling pathway (Lee et al., 2007), AMPK signaling pathway (Nagata et al., 2003) and FoxO signaling pathway (Eijkelenboom and Burgering, 2013). In a recent study with catfish, Yang et al. (2018) used RNA-Seq analysis to provide systematic knowledge about gene regulation under hypoxia conditions. Through enrichment analysis of differentially expressed genes, they confirmed the involvement of HIF, MAPK, PI3K/Akt/mTOR and Ras signaling pathway under hypoxia.
GWAS Studies on Low Oxygen Tolerance in Catfish
Two GWAS studies were recently conducted on hypoxia tolerance, using channel catfish (Wang et al., 2017b) and hybrid catfish (Zhong et al., 2017). In one experiment with six strains of channel catfish, QTL were identified in all six strains, but they rarely overlaped, suggesting the complexity of the genetic architecture of hypoxia tolerance in different channel catfish strains (Wang et al., 2017b). Genes annotated within QTL were involved in not only MAPK signaling pathway but also PI3K/AKT/mTOR signaling pathways (Wang et al., 2017b). It is apparent that mutations in any of the several gene pathways can lead to QTL associated with tolerance to low oxygen.
In another experiment, Zhong et al. (2017) used the catfish 250K SNP arrays to determine QTL with the hybrid catfish. A total of four QTL (on LG 2, 4, 23 and 29) involved in hypoxia tolerance were identified in hybrid catfish. In pathway analysis, annotated genes within QTL associated with hypoxia tolerance belonged to several pathways, for instance, PI3K/Akt/mTOR signaling pathway (Zhong et al., 2017). The QTL identified from the interspecific hybrid catfish, for the most part, had rare overlaps with the QTL within the six strains from channel catfish (Wang et al., 2017b), further supporting the notion that the genetic architecture of low oxygen tolerance in catfish is extremely complex (Zhong et al., 2017).
Potential Interactions Between the Responses to Hypoxia Stress and Bacterial Infection in Catfish
The interdependence of hypoxic responses and innate immune responses has been observed in mammals (Nizet and Johnson, 2009). For instance, the key regulator of hypoxia responses, HIF-1α, has been demonstrated to function as an essential regulator of inflammation and innate immunity (Zinkernagel et al., 2007). Similarly, the potential linkage between immune responses and the responses to hypoxia were also observed in catfish. After infection with high challenge dose of E. ictaluri, channel catfish in the hypoxic groups had higher mortality (∼36%) than the catfish in control groups (∼12%) (Welker et al., 2007). Furthermore, PI3K signaling pathway was found to be involved in the molecular responses to both hypoxia stress and bacterial infections, as supported by GWAS analysis on tolerance to low dissolved oxygen of both channel catfish (Wang et al., 2017b) and hybrid catfish (Zhong et al., 2017), and the resistance to columnaris disease in hybrid catfish (Geng et al., 2015). In spite of being preliminary, these studies suggested similarities of responses to bacterial infections and those to hypoxia, and potential interactions of the host responses to biotic and abiotic stresses.
Author Contributions
TZ, ZY, ST, YJ, YY, HS, WW, DN, LG, and WJ wrote the draft manuscript. DG and ZL reviewed, revised, and finalized the manuscript.
Funding
Research in our laboratory has been supported by USDA NIFA AFRI programs including Animal Genomics, Genetics and Breeding Program, Animal Health and Disease Program, and Aquaculture Research Program (Grants: 2015-67015-22975, 2015-67015-22907, and 2017-67015-26295).
Conflict of Interest Statement
The authors declare that the research was conducted in the absence of any commercial or financial relationships that could be construed as a potential conflict of interest.
References
Abdelhamed, H., Ibrahim, I., Baumgartner, W., Lawrence, M. L., and Karsi, A. (2018). The virulence and immune protection of Edwardsiella ictaluri HemR mutants in catfish. Fish Shellfish Immunol. 72, 153–160. doi: 10.1016/j.fsi.2017.10.041
Abdelrahman, H., ElHady, M., Alcivar-Warren, A., Allen, S., Al-Tobasei, R., Bao, L., et al. (2017). Aquaculture genomics, genetics and breeding in the United States: current status, challenges, and priorities for future research. BMC Genomics 18:191. doi: 10.1186/s12864-017-3557-1
Abd-El-Rhman, A. M. (2009). Antagonism of Aeromonas hydrophila by propolis and its effect on the performance of Nile tilapia, Oreochromis niloticus. Fish Shellfish Immunol. 27, 454–459. doi: 10.1016/j.fsi.2009.06.015
Abukhalaf, I. K., Zimmerman, E. G., Dickson, K. L., Masaracchia, R. A., Donahue, M. J., and Covington, S. (1994). Purification of the 70-kDa heat-shock protein from catfish liver: immunological comparison of the protein in different fish species and its potential use as a stress indicator. Environ. Toxicol. Chem. 13, 1251–1257. doi: 10.1002/etc.5620130806
Anacker, R. L., and Ordal, E. J. (1955). Study of a bacteriophage infecting the myxobacterium Chondrococcus columnaris. J. Bacteriol. 70, 738–741.
Arias, C., Welker, T., Shoemaker, C., Abernathy, J., and Klesius, P. (2004). Genetic fingerprinting of Flavobacterium columnare isolates from cultured fish. J. Appl. Microbiol. 97, 421–428. doi: 10.1111/j.1365-2672.2004.02314.x
Arnold, M. B., Torrans, E. L., and Allen, P. J. (2013). Influences of cyclic, high temperatures on juvenile channel catfish growth and feeding. N. Am. J. Aquac. 75, 77–84. doi: 10.1080/15222055.2012.732674
Barozai, M. Y. K. (2012). The MicroRNAs and their targets in the channel catfish (Ictalurus punctatus). Mol. Biol. Rep. 39, 8867–8872. doi: 10.1007/s11033-012-1753-2
Bartel, D. P. (2004). MicroRNAs: genomics, biogenesis, mechanism, and function. Cell 116, 281–297. doi: 10.1016/S0092-8674(04)00045-5
Bebak, J., Matthews, M., and Shoemaker, C. (2009). Survival of vaccinated, feed-trained largemouth bass fry (Micropterus salmoides floridanus) during natural exposure to Flavobacterium columnare. Vaccine 27, 4297–4301. doi: 10.1016/j.vaccine.2009.05.026
Bebak, J., and Wagner, B. (2012). Use of vaccination against enteric septicemia of catfish and columnaris disease by the US catfish industry. J. Aquat. Anim. Health 24, 30–36. doi: 10.1080/08997659.2012.667048
Beck, B. H., Farmer, B. D., Straus, D. L., Li, C., and Peatman, E. (2012). Putative roles for a rhamnose binding lectin in Flavobacterium columnare pathogenesis in channel catfish Ictalurus punctatus. Fish Shellfish Immunol. 33, 1008–1015. doi: 10.1016/j.fsi.2012.08.018
Becker, C. D., and Fujihara, M. (1978). The Bacterial Pathogen Flexibacter columnaris and its Epizootiology among Columbia River fish: A Review and Synthesis. Washington, DC: American Fisheries Society.
Bennett, W. A., McCauley, R. W., and Beitinger, T. L. (1998). Rates of gain and loss of heat tolerance in channel catfish. Trans. Am. Fish. Soc. 127, 1051–1058. doi: 10.1577/1548-8659(1998)127<1051:ROGALO>2.0.CO;2
Bernardet, J.-F., and Bowman, J. P. (2006). ”The genus Flavobacterium,” in The Prokaryotes: A Handbook on the Biology of Bacteria, 3rd Edn, Vol. 7, eds M. Dworkin, S. Falkow, E. Rosenberg, K. H.Schleifer, and E. Stackebrandt (New York, NY: Springer), 481–531.
Bernardet, J.-F., Segers, P., Vancanneyt, M., Berthe, F., Kersters, K., and Vandamme, P. (1996). Cutting a Gordian knot: emended classification and description of the genus Flavobacterium, emended description of the family Flavobacteriaceae, and proposal of Flavobacterium hydatis nom. nov.(Basonym, Cytophaga aquatilis Strohl and Tait 1978). Int. J. Syst. Evol. Microbiol. 46, 128–148. doi: 10.1099/00207713-46-1-128
Birchler, J. A., Auger, D. L., and Riddle, N. C. (2003). In search of the molecular basis of heterosis. Plant Cell 15, 2236–2239. doi: 10.1105/tpc.151030
Bondad-Reantaso, M. G., Arthur, J. R., Subasinghe, R. P., Bravo, S., Koonse, B., Alday-Sanz, V., et al. (2012). Improving Biosecurity through Prudent and Responsible use of Veterinary Medicines in Aquatic Food Production. Roma: FAO.
Booth, N. J., Elkamel, A., and Thune, R. L. (2006). Intracellular replication of Edwardsiella ictaluri in channel catfish macrophages. J. Aquat. Anim. Health 18, 101–108. doi: 10.1577/H05-025.1
Bruick, R. K. (2003). Oxygen sensing in the hypoxic response pathway: regulation of the hypoxia-inducible transcription factor. Genes Dev. 17, 2614–2623. doi: 10.1101/gad.1145503
Buckley, B. A., Gracey, A. Y., and Somero, G. N. (2006). The cellular response to heat stress in the goby Gillichthys mirabilis: a cDNA microarray and protein-level analysis. J. Exp. Biol. 209, 2660–2677. doi: 10.1242/jeb.02292
Buckley, B. A., and Hofmann, G. E. (2002). Thermal acclimation changes DNA-binding activity of heat shock factor 1 (HSF1) in the goby Gillichthys mirabilis: implications for plasticity in the heat-shock response in natural populations. J. Exp. Biol. 205, 3231–3240.
Buckley, B. A., and Hofmann, G. E. (2004). Magnitude and duration of thermal stress determine kinetics of hsp gene regulation in the goby Gillichthys mirabilis. Physiol. Biochem. Zool. 77, 570–581. doi: 10.1086/420944
Buentello, J. A., Gatlin, D. M. III, and Neill, W. H. (2000). Effects of water temperature and dissolved oxygen on daily feed consumption, feed utilization and growth of channel catfish (Ictalurus punctatus). Aquaculture 182, 339–352. doi: 10.1016/S0044-8486(99)00274-4
Bullard, S., Mohammed, H., and Arias, C. (2013). First record of the fish pathogen Flavobacterium columnare genomovar II from bluegill, Lepomis macrochirus (Rafinesque), with observations on associated lesions. J. Fish Dis. 36, 447–451. doi: 10.1111/jfd.12005
Bullock, G., Hsu, T.-C., and Shotts, E. Jr. (1986). Columnaris Disease of Fishes. Washington, DC: U.S. Fish and Wildlife Service.
Burggren, W. W., and Cameron, J. N. (1980). Anaerobic metabolism, gas exchange, and acid-base balance during hypoxic exposure in the channel catfish, Ictalurus punctatus. J. Exp. Zool. Part A 213, 405–416. doi: 10.1002/jez.1402130312
Darwish, A. M., and Ismaiel, A. A. (2005). Genetic diversity of Flavobacterium columnare examined by restriction fragment length polymorphism and sequencing of the 16S ribosomal RNA gene and the 16S–23S rDNA spacer. Mol. Cell. Probes 19, 267–274. doi: 10.1016/j.mcp.2005.04.003
Davis, H. S. (1922). A New Bacterial Disease of Fresh-Water Fishes: By HS Davis. Washington, BC: Government Printing Office. doi: 10.5962/bhl.title.49773
Declercq, A. M., Haesebrouck, F., Van den Broeck, W., Bossier, P., and Decostere, A. (2013). Columnaris disease in fish: a review with emphasis on bacterium-host interactions. BMC Vet. Res. 44:27. doi: 10.1186/1297-9716-44-27
Decostere, A., Haesebrouck, F., and Devriese, L. (1998). Characterization of four Flavobacterium columnare (Flexibacter columnaris) strains isolated from tropical fish. Vet. Microbiol. 62, 35–45. doi: 10.1016/S0378-1135(98)00196-5
Decostere, A., Haesebrouck, F., Van Driessche, E., Charlier, G., and Ducatelle, R. (1999). Characterization of the adhesion of Flavobacterium columnare (Flexibacter columnaris) to gill tissue. J. Fish Dis. 22, 465–474. doi: 10.1046/j.1365-2761.1999.00198.x
Decostere, A., Henckaerts, K., Ducatelle, R., and Haesebrouck, F. (2002). An alternative model to study the association of rainbow trout (Oncorhynchus mykiss L.) pathogens with the gill tissue. Lab. Anim. 36, 396–402. doi: 10.1258/002367702320389053
Dikmen, S., Cole, J. B., Null, D. J., and Hansen, P. J. (2013). Genome-wide association mapping for identification of quantitative trait loci for rectal temperature during heat stress in Holstein cattle. PLoS One 8:e69202. doi: 10.1371/journal.pone.0069202
Domenici, P., Lefrancois, C., and Shingles, A. (2007). Hypoxia and the antipredator behaviours of fishes. Philos. Trans. R. Soc. Lond. B Biol. Sci. 362, 2105–2121. doi: 10.1098/rstb.2007.2103
Dong, X., Ye, Z., Song, L., Su, B., Zhao, H., Peatman, E., et al. (2016). Expression profile analysis of two cathepsin S in channel catfish (Ictalurus punctatus) mucosal tissues following bacterial challenge. Fish Shellfish Immunol. 48, 112–118. doi: 10.1016/j.fsi.2015.11.030
Dunham, R., and Elaswad, A. (2018). Catfish biology and farming. Annu. Rev. Anim. Biosci. 6, 305–325. doi: 10.1146/annurev-animal-030117-014646
Dunham, R. A., Hyde, C., Masser, M., Plumb, J. A., Smitherman, R. O., Perez, R., et al. (1994). Comparison of culture traits of channel catfish, Ictalurus punctatus, and blue catfish I. furcatus. J. Appl. Aquac. 3, 257–268. doi: 10.1300/J028v03n03_04
Dunham, R. A., and Masser, M. P. (2012). Production of Hybrid Catfish. SRAC Publication No. 190. Stoneville, MS: Southeastern Regional Aquaculture Center.
Dunham, R. A., Ramboux, A. C., and Perera, D. A. (2014). Effect of strain on tolerance of low dissolved oxygen of channel X blue catfish hybrids. Aquaculture 420, S25–S28. doi: 10.1016/j.aquaculture.2013.09.039
Dunham, R. A., and Smitherman, R. O. (1984). Ancestry and Breeding of Catfish in the United States. Available at: http://hdl.handle.net/11200/2059
Dunham, R. A., Smitherman, R. O., and Webber, C. (1983). Relative tolerance of channel x blue hybrid and channel catfish to low oxygen concentrations. Prog. Fish Cult. 45, 55–57. doi: 10.1577/1548-8659(1983)45[55:RTOCXB]2.0.CO;2
Dunham, R. A., Umali, G. M., Beam, R., Kristanto, A. H., and Trask, M. (2008). Comparison of production traits of NWAC103 channel catfish, NWAC103 channel catfish × blue catfish hybrids, Kansas Select 21 channel catfish, and blue catfish grown at commercial densities and exposed to natural bacterial epizootics. N. Am. J. Aquac. 70, 98–106. doi: 10.1577/A07-006.1
Eijkelenboom, A., and Burgering, B. M. (2013). FOXOs: signalling integrators for homeostasis maintenance. Nat. Rev. Mol. Cell Biol. 14, 83–97. doi: 10.1038/nrm3507
Eissa, N., and Wang, H. P. (2016). Transcriptional stress responses to environmental and husbandry stressors in aquaculture species. Rev. Aquac. 8, 61–88. doi: 10.1016/j.ygcen.2014.04.016
Esteve, C., Amaro, C., and Toranzo, A. E. (1994). O-serogrouping and surface components of Aeromonas hydrophila and Aeromonas jandaei pathogenic for eels. FEMS Microbiol. Lett. 117, 85–90. doi: 10.1111/j.1574-6968.1994.tb06746.x
Evenhuis, J. P., and LaFrentz, B. R. (2016). Virulence of Flavobacterium columnare genomovars in rainbow trout Oncorhynchus mykiss. Dis. Aquat. Organ. 120, 217–224. doi: 10.3354/dao03027
Ewanowich, C., and Peppler, M. (1990). Phorbol myristate acetate inhibits HeLa 229 invasion by Bordetella pertussis and other invasive bacterial pathogens. Infect. Immun. 58, 3187–3193.
Feder, M. E., and Hofmann, G. E. (1999). Heat-shock proteins, molecular chaperones, and the stress response: evolutionary and ecological physiology. Annu. Rev. Physiol. 61, 243–282. doi: 10.1146/annurev.physiol.61.1.243
Feng, T., Zhang, H., Liu, H., Zhou, Z., Niu, D., Wong, L., et al. (2011). Molecular characterization and expression analysis of the channel catfish cathepsin D genes. Fish Shellfish Immunol. 31, 164–169. doi: 10.1016/j.fsi.2011.04.006
Ficke, A. A. (2005). The Effects of Global Climate Change on the Fishes of the Southeastern United States. Gland: World Wildlife Fund for Nature.
Ficke, A. D., Myrick, C. A., and Hansen, L. J. (2007). Potential impacts of global climate change on freshwater fisheries. Rev. Fish Biol. Fish. 17, 581–613. doi: 10.1007/s11160-007-9059-5
Fijan, N. (1968). The survival of Chondrococcus columnaris in waters of different quality. Bull. Off. Int. Épizoot. 69, 1159–1166.
Finlay, B. B., and Falkow, S. (1989). Common themes in microbial pathogenicity. Microbiol. Rev. 53, 210–230.
Finlay, B. B., Heffron, F., and Falkow, S. (1989). Epithelial cell surfaces induce Salmonella proteins required for bacterial adherence and invasion. Science 243, 940–943. doi: 10.1126/science.2919285
Fu, Q., Li, Y., Yang, Y., Li, C., Yao, J., Zeng, Q., et al. (2016). Septin genes in channel catfish (Ictalurus punctatus) and their involvement in disease defense responses. Fish Shellfish Immunol. 49, 110–121. doi: 10.1016/j.fsi.2015.12.022
Fu, Q., Yang, Y., Li, C., Zeng, Q., Zhou, T., Li, N., et al. (2017a). The chemokinome superfamily: II. The 64 CC chemokines in channel catfish and their involvement in disease and hypoxia responses. Dev. Comp. Immunol. 73, 97–108. doi: 10.1016/j.dci.2017.03.012
Fu, Q., Yang, Y., Li, C., Zeng, Q., Zhou, T., Li, N., et al. (2017b). The CC and CXC chemokine receptors in channel catfish (Ictalurus punctatus) and their involvement in disease and hypoxia responses. Dev. Comp. Immunol. 77, 241–251. doi: 10.1016/j.dci.2017.08.012
Fu, Q., Zeng, Q., Li, Y., Yang, Y., Li, C., Liu, S., et al. (2017c). The chemokinome superfamily in channel catfish: I. CXC subfamily and their involvement in disease defense and hypoxia responses. Fish Shellfish Immunol. 60, 380–390. doi: 10.1016/j.fsi.2016.12.004
Geng, X., Feng, J., Liu, S., Wang, Y., Arias, C., and Liu, Z. (2014). Transcriptional regulation of hypoxia inducible factors alpha (HIF-α) and their inhibiting factor (FIH-1) of channel catfish (Ictalurus punctatus) under hypoxia. Comp. Biochem. Physiol. B Biochem. Mol. Biol. 169, 38–50. doi: 10.1016/j.cbpb.2013.12.007
Geng, X., Liu, S., Yao, J., Bao, L., Zhang, J., Li, C., et al. (2016). A genome-wide association study identifies multiple regions associated with head size in catfish. G3 6, 3389–3398. doi: 10.1534/g3.116.032201
Geng, X., Liu, S., Yuan, Z., Jiang, Y., Zhi, D., and Liu, Z. (2017). A genome-wide association study reveals that genes with functions for bone development are associated with body conformation in catfish. Mar. Biotechnol. 19, 570–578. doi: 10.1007/s10126-017-9775-3
Geng, X., Sha, J., Liu, S., Bao, L., Zhang, J., Wang, R., et al. (2015). A genome-wide association study in catfish reveals the presence of functional hubs of related genes within QTLs for columnaris disease resistance. BMC Genomics 16:196. doi: 10.1186/s12864-015-1409-4
Giannetto, A., Maisano, M., Cappello, T., Oliva, S., Parrino, V., Natalotto, A., et al. (2015). Hypoxia-inducible factor α and Hif-prolyl hydroxylase characterization and gene expression in short-time air-exposed Mytilus galloprovincialis. Mar. Biotechnol. 17, 768–781. doi: 10.1007/s10126-015-9655-7
Graham, K. (1999). “A review of the biology and management of blue catfish,” in Proceedings of the International Ictalurid Symposium, (Symposium: American Fisheries Society), 37–49.
Green, B., Rawles, S., Fuller, S., Beck, B., and McEntire, M. (2016). Hypoxia affects performance traits and body composition of juvenile hybrid striped bass (Morone chrysops × M. saxatilis). Aquac. Res. 47, 2266–2275. doi: 10.1111/are.12678
Griffin, B. (1992). A simple procedure for identification of Cytophaga columnaris. J. Aquat. Anim. Health 4, 63–66. doi: 10.1577/1548-8667(1992)004<0063:ASPFIO>2.3.CO;2
Harikrishnan, R., Balasundaram, C., and Heo, M.-S. (2009). Effect of chemotherapy, vaccines and immunostimulants on innate immunity of goldfish infected with Aeromonas hydrophila. Dis. Aquat. Organ. 88, 45–54. doi: 10.3354/dao02143
Harikrishnan, R., Rani, M. N., and Balasundaram, C. (2003). Hematological and biochemical parameters in common carp, Cyprinus carpio, following herbal treatment for Aeromonas hydrophila infection. Aquaculture 221, 41–50. doi: 10.1016/S0044-8486(03)00023-1
Hart, J. (1952). Geographic Variations of some Physiological and Morphological Characters in Certain Freshwater Fish. Toronto: University of Toronto press.
Hawke, J. P., Durborow, R., Thune, R., and Camus, A. (1998). ESC: Enteric Septicemia of Catfish. Stoneville, MS: Southern Regional Aquaculture Center.
Hawke, J. P., McWhorter, A. C., Steigerwalt, A. G., and Brenner, D. J. (1981). Edwardsiella ictaluri sp. nov., the causative agent of enteric septicemia of catfish. Int. J. Syst. Evol. Microbiol. 31, 396–400. doi: 10.1099/00207713-31-4-396
Hemstreet, B. (2010). An update on Aeromonas hydrophila from a fish health specialist for summer 2010. Catfish J. 24:4.
Hossain, M. J., Sun, D., McGarey, D. J., Wrenn, S., Alexander, L. M., Martino, M. E., et al. (2014). An Asian origin of virulent Aeromonas hydrophila responsible for disease epidemics in United States-farmed catfish. mBio 5:e848-14. doi: 10.1128/mBio.00848-14
Houston, R. D., Haley, C. S., Hamilton, A., Guy, D. R., Mota-Velasco, J. C., Gheyas, A. A., et al. (2010). The susceptibility of Atlantic salmon fry to freshwater infectious pancreatic necrosis is largely explained by a major QTL. Heredity 105, 318–327. doi: 10.1038/hdy.2009.171
Houston, R. D., Haley, C. S., Hamilton, A., Guy, D. R., Tinch, A. E., Taggart, J. B., et al. (2008). Major quantitative trait loci affect resistance to infectious pancreatic necrosis in Atlantic salmon (Salmo salar). Genetics 178, 1109–1115. doi: 10.1534/genetics.107.082974
Jeney, Z., Rácz, T., Thompson, K. D., Poobalane, S., Ardó, L., Adams, A., et al. (2009). Differences in the antibody response and survival of genetically different varieties of common carp (Cyprinus carpio L.) vaccinated with a commercial Aeromonas salmonicida/A. hydrophila vaccine and challenged with A. hydrophila. Fish Physiol. Biochem. 35, 677–682. doi: 10.1007/s10695-009-9329-3
Jensen, F. B., Nikinmaa, M., and Weber, R. E. (1993). “Environmental perturbations of oxygen transport in teleost fishes: causes, consequences and compensations,” in Fish Ecophysiology. Chapman & Hall Fish and Fisheries Series, Vol. 9, eds J. C. Rankin and F. B. Jensen (Dordrecht: Springer), 161–179. doi: 10.1007/978-94-011-2304-4_6
Jiang, C., Zhang, J., Yao, J., Liu, S., Li, Y., Song, L., et al. (2015). Complement regulatory protein genes in channel catfish and their involvement in disease defense response. Dev. Comp. Immunol. 53, 33–41. doi: 10.1016/j.dci.2015.06.002
Jin, Y., Zhou, T., Geng, X., Liu, S., Chen, A., Yao, J., et al. (2017). A genome-wide association study of heat stress-associated SNPs in catfish. Anim. Genet. 48, 233–236. doi: 10.1111/age.12482
Jin, Y., Zhou, T., Li, N., Liu, S., Xu, X., Pan, Y., et al. (2018). JAK and STAT members in channel catfish: identification, phylogenetic analysis and expression profiling after Edwardsiella ictaluri infection. Dev. Comp. Immunol. 81, 334–341. doi: 10.1016/j.dci.2017.12.019
Klesius, P. H., and Shoemaker, C. A. (1999). Development and use of modified live Edwardsiella ictaluri vaccine against enteric septicemia of catfish. Adv. Vet. Med. 41, 523–537. doi: 10.1016/S0065-3519(99)80039-1
Koong, A. C., Chen, E. Y., Mivechi, N. F., Denko, N. C., Stambrook, P., and Giaccia, A. J. (1994). Hypoxic activation of nuclear factor-κB is mediated by a RAS and RAF signaling pathway and does not involve MAP kinase (ERK1 or ERK2). Cancer Res. 54, 5273–5279.
Kordon, A. O., Abdelhamed, H., Ahmed, H., Park, J. Y., Karsi, A., and Pinchuk, L. M. (2018). Phagocytic and bactericidal properties of channel catfish peritoneal macrophages exposed to Edwardsiella ictaluri live attenuated vaccine and wild-type strains. Front. Microbiol. 8:2638. doi: 10.3389/fmicb.2017.02638
Krieg, N. R., and Holt, J. G. (1984). Bergey’s Manual of Systematic Bacteriology, Vol. 1. Baltimore, MD: Williams & Wilkins Co, 161–172.
Kültz, D. (2005). Molecular and evolutionary basis of the cellular stress response. Annu. Rev. Physiol. 67, 225–257. doi: 10.1146/annurev.physiol.67.040403.103635
Kunttu, H. M., Suomalainen, L.-R., Jokinen, E. I., and Valtonen, E. T. (2009). Flavobacterium columnare colony types: connection to adhesion and virulence? Microb. Pathog. 46, 21–27. doi: 10.1016/j.micpath.2008.10.001
Landry, C. A., Steele, S. L., Manning, S., and Cheek, A. O. (2007). Long term hypoxia suppresses reproductive capacity in the estuarine fish, Fundulus grandis. Comp. Biochem. Physiol. A Mol. Integr. Physiol. 148, 317–323. doi: 10.1016/j.cbpa.2007.04.023
Lawrence, M. L., Cooper, R. K., and Thune, R. L. (1997). Attenuation, persistence, and vaccine potential of an Edwardsiella ictaluri purA mutant. Infect. Immun. 65, 4642–4651.
Lee, S., Chen, T. T., Barber, C. L., Jordan, M. C., Murdock, J., Desai, S., et al. (2007). Autocrine VEGF signaling is required for vascular homeostasis. Cell 130, 691–703. doi: 10.1016/j.cell.2007.06.054
Li, C., Beck, B., Su, B., Terhune, J., and Peatman, E. (2013). Early mucosal responses in blue catfish (Ictalurus furcatus) skin to Aeromonas hydrophila infection. Fish Shellfish Immunol. 34, 920–928. doi: 10.1016/j.fsi.2013.01.002
Li, C., Zhang, Y., Wang, R., Lu, J., Nandi, S., Mohanty, S., et al. (2012). RNA-seq analysis of mucosal immune responses reveals signatures of intestinal barrier disruption and pathogen entry following Edwardsiella ictaluri infection in channel catfish, Ictalurus punctatus. Fish Shellfish Immunol. 32, 816–827. doi: 10.1016/j.fsi.2012.02.004
Li, N., Zhou, T., Geng, X., Jin, Y., Wang, X., Liu, S., et al. (2017). Identification of novel genes significantly affecting growth in catfish through GWAS analysis. Mol. Genet. Genomics 293, 587–599. doi: 10.1007/s00438-017-1406-1
Li, Y., Liu, S., Qin, Z., Yao, J., Jiang, C., Song, L., et al. (2015). The serpin superfamily in channel catfish: identification, phylogenetic analysis and expression profiling in mucosal tissues after bacterial infections. Dev. Comp. Immunol. 49, 267–277. doi: 10.1016/j.dci.2014.12.006
Li, Z., Yao, J., Xie, Y., Geng, X., and Liu, Z. (2016). Phosphoinositide 3-kinase family in channel catfish and their regulated expression after bacterial infection. Fish Shellfish Immunol. 49, 364–373. doi: 10.1016/j.fsi.2016.01.002
Liu, S., Wang, X., Sun, F., Zhang, J., Feng, J., Liu, H., et al. (2013). RNA-Seq reveals expression signatures of genes involved in oxygen transport, protein synthesis, folding, and degradation in response to heat stress in catfish. Physiol. Genomics 45, 462–476. doi: 10.1152/physiolgenomics.00026.2013
Liu, Z., Liu, S., Yao, J., Bao, L., Zhang, J., Li, Y., et al. (2016). The channel catfish genome sequence provides insights into the evolution of scale formation in teleosts. Nat. Commun. 7:11757. doi: 10.1038/ncomms11757
Logan, C. A., and Buckley, B. A. (2015). Transcriptomic responses to environmental temperature in eurythermal and stenothermal fishes. J. Exp. Biol. 218, 1915–1924. doi: 10.1242/jeb.114397
Logan, C. A., and Somero, G. N. (2011). Effects of thermal acclimation on transcriptional responses to acute heat stress in the eurythermal fish Gillichthys mirabilis (Cooper). Am. J. Physiol. Regul. Integr. Comp. Physiol. 300, R1373–R1383. doi: 10.1152/ajpregu.00689.2010
Lu, D.-Y., Liou, H.-C., Tang, C.-H., and Fu, W.-M. (2006). Hypoxia-induced iNOS expression in microglia is regulated by the PI3-kinase/Akt/mTOR signaling pathway and activation of hypoxia inducible factor-1α. Biochem. Pharmacol. 72, 992–1000. doi: 10.1016/j.bcp.2006.06.038
Luft, J. C., Wilson, M. R., Bly, J. E., Miller, N. W., and Clem, L. W. (1996). Identification and characterization of a heat shock protein 70 family member in channel catfish (Ictalurus punctatus). Comp. Biochem. Physiol. B Biochem. Mol. Biol. 113, 169–174. doi: 10.1016/0305-0491(95)02022-5
Mallya, Y. J. (2007). The Effects of Dissolved Oxygen on Fish growth in Aquaculture. Reykjavik: The United Nations University fisheries training programmer.
Mazure, N. M., Chen, E. Y., Laderoute, K. R., and Giaccia, A. J. (1997). Induction of vascular endothelial growth factor by hypoxia is modulated by a phosphatidylinositol 3-kinase/Akt signaling pathway in Ha-RAS-transformed cells through a hypoxia inducible factor-1 transcriptional element. Blood 90, 3322–3331.
McCauley, R., and Beitinger, T. (1992). Predicted effects of climate warming on the commercial culture of the channel catfish, Ictalurus punctatus. GeoJournal 28, 61–66. doi: 10.1007/BF00216407
Meyer, F. (1991). Aquaculture disease and health management. J. Anim. Sci. 69, 4201–4208. doi: 10.2527/1991.69104201x
Miyazaki, T., and Plumb, J. (1985). Histopathology of Edwardsiella ictaluri in channel catfish, Ictalurus punctatus (Rafinesque). J. Fish Dis. 8, 389–392. doi: 10.1111/j.1365-2761.1985.tb00961.x
Moen, T., Torgersen, J., Santi, N., Davidson, W. S., Baranski, M., Ødegård, J., et al. (2015). Epithelial cadherin determines resistance to infectious pancreatic necrosis virus in Atlantic salmon. Genetics 200, 1313–1326. doi: 10.1534/genetics.115.175406
Mohammed, H., Olivares-Fuster, O., LaFrentz, S., and Arias, C. R. (2013). New attenuated vaccine against columnaris disease in fish: choosing the right parental strain is critical for vaccine efficacy. Vaccine 31, 5276–5280. doi: 10.1016/j.vaccine.2013.08.052
Mohammed, H. H., and Arias, C. R. (2014). Epidemiology of columnaris disease affecting fishes within the same watershed. Dis. Aquat. Organ. 109, 201–211. doi: 10.3354/dao02739
Moore, A. A., Eimers, M. E., and Cardella, M. A. (1990). Attempts to control Flexibacter columnaris epizootics in pond-reared channel catfish by vaccination. J. Aquat. Anim. Health 2, 109–111. doi: 10.1577/1548-8667(1990)002<0109:ATCCEI>2.3.CO;2
Morley, N., and Lewis, J. (2010). Consequences of an outbreak of columnaris disease (Flavobacterium columnare) to the helminth fauna of perch (Perca fluviatilis) in the Queen Mary reservoir, south-east England. J. Helminthol. 84, 186–192. doi: 10.1017/S0022149X09990459
Mu, W., Yao, J., Zhang, J., Liu, S., Wen, H., Feng, J., et al. (2015). Expression of tumor suppressor genes in channel catfish after bacterial infections. Dev. Comp. Immunol. 48, 171–177. doi: 10.1016/j.dci.2014.10.004
Nagata, D., Mogi, M., and Walsh, K. (2003). AMP-activated Protein Kinase (AMPK) signaling in endothelial cells is essential for angiogenesis in response to hypoxic stress. J. Biol. Chem. 278, 31000–31006. doi: 10.1074/jbc.M300643200
Newman, S. G. (1993). Bacterial vaccines for fish. Annu. Rev. Fish Dis. 3, 145–185. doi: 10.1016/0959-8030(93)90033-8
Nho, S. W., Abdelhamed, H., Karsi, A., and Lawrence, M. L. (2017). Improving safety of a live attenuated Edwardsiella ictaluri vaccine against enteric septicemia of catfish and evaluation of efficacy. Vet. Microbiol. 210, 83–90. doi: 10.1016/j.vetmic.2017.09.004
Nizet, V., and Johnson, R. S. (2009). Interdependence of hypoxic and innate immune responses. Nat. Rev. Immunol. 9, 609–617. doi: 10.1038/nri2607
Olivares-Fuster, O., and Arias, C. (2011). Development and characterization of rifampicin-resistant mutants from high virulent strains of Flavobacterium columnare. J. Fish Dis. 34, 385–394. doi: 10.1111/j.1365-2761.2011.01253.x
Olivares-Fuster, O., Baker, J. L., Terhune, J. S., Shoemaker, C. A., Klesius, P. H., and Arias, C. R. (2007). Host-specific association between Flavobacterium columnare genomovars and fish species. Syst. Appl. Microbiol. 30, 624–633. doi: 10.1016/j.syapm.2007.07.003
Olivares-Fuster, O., Bullard, S. A., McElwain, A., Llosa, M. J., and Arias, C. R. (2011). Adhesion dynamics of Flavobacterium columnare to channel catfish Ictalurus punctatus and zebrafish Danio rerio after immersion challenge. Dis. Aquat. Organ. 96, 221–227. doi: 10.3354/dao02371
Olivares-Fuster, Ó (2010). Development, Characterization and Early Evaluation of New Modified Live Vaccines Against Columnaris Disease. Master thesis, Auburn, AL, Auburn University.
Pang, M., Jiang, J., Xie, X., Wu, Y., Dong, Y., Kwok, A. H., et al. (2015). Novel insights into the pathogenicity of epidemic Aeromonas hydrophila ST251 clones from comparative genomics. Sci. Rep. 5:9833. doi: 10.1038/srep09833
Parsell, D., and Lindquist, S. (1993). The function of heat-shock proteins in stress tolerance: degradation and reactivation of damaged proteins. Annu. Rev. Genet. 27, 437–496. doi: 10.1146/annurev.ge.27.120193.002253
Peatman, E., Baoprasertkul, P., Terhune, J., Xu, P., Nandi, S., Kucuktas, H., et al. (2007). Expression analysis of the acute phase response in channel catfish (Ictalurus punctatus) after infection with a Gram-negative bacterium. Dev. Comp. Immunol. 31, 1183–1196. doi: 10.1016/j.dci.2007.03.003
Peatman, E., Li, C., Peterson, B. C., Straus, D. L., Farmer, B. D., and Beck, B. H. (2013). Basal polarization of the mucosal compartment in Flavobacterium columnare susceptible and resistant channel catfish (Ictalurus punctatus). Mol. Immunol. 56, 317–327. doi: 10.1016/j.molimm.2013.04.014
Peatman, E., Terhune, J., Baoprasertkul, P., Xu, P., Nandi, S., Wang, S., et al. (2008). Microarray analysis of gene expression in the blue catfish liver reveals early activation of the MHC class I pathway after infection with Edwardsiella ictaluri. Mol. Immunol. 45, 553–566. doi: 10.1016/j.molimm.2007.05.012
Peterson, B. C., Flora, C., Wood, M., Bosworth, B. G., Quiniou, S. M., Greenway, T. E., et al. (2016). Vaccination of full-sib channel catfish families against enteric septicemia of catfish with an oral live attenuated Edwardsiella ictaluri vaccine. J. World Aquac. Soc. 47, 207–211. doi: 10.1111/jwas.12264
Plumb, J. (1999). Health Maintenance and Principal Microbial Diseases of Cultured Fishes. Ames, IA: Iowa State University Press.
Plumb, J. A., and Hanson, L. A. (2011). Health Maintenance and Principal Microbial Diseases of Cultured Fishes. Hoboken, NJ: John Wiley & Sons.
Pörtner, H.-O., and Peck, M. (2010). Climate change effects on fishes and fisheries: towards a cause-and-effect understanding. J. Fish Biol. 77, 1745–1779. doi: 10.1111/j.1095-8649.2010.02783.x
Pridgeon, J. W., and Klesius, P. H. (2010). Identification and expression profile of multiple genes in channel catfish fry 10 min after modified live Flavobacterium columnare vaccination. Vet. Immunol. Immunopathol. 138, 25–33. doi: 10.1016/j.vetimm.2010.06.011
Pridgeon, J. W., and Klesius, P. H. (2011). Molecular identification and virulence of three Aeromonas hydrophila isolates cultured from infected channel catfish during a disease outbreak in west Alabama (USA) in 2009. Dis. Aquat. Organ. 94, 249–253. doi: 10.3354/dao02332
Qian, X., Ba, Y., Zhuang, Q., and Zhong, G. (2014). RNA-Seq technology and its application in fish transcriptomics. OMICS 18, 98–110. doi: 10.1089/omi.2013.0110
Richards, J. G. (2009). “Metabolic and molecular responses of fish to hypoxia,” in Fish Physiology, Vol. 27, eds J. G. Richards, A. P. Farrell, and C. J. Brauner (San Diego, CA: Elsevier), 443–485.
Robinson, N., Baranski, M., Mahapatra, K. D., Saha, J. N., Das, S., Mishra, J., et al. (2014). A linkage map of transcribed single nucleotide polymorphisms in rohu (Labeo rohita) and QTL associated with resistance to Aeromonas hydrophila. BMC Genomics 15:541. doi: 10.1186/1471-2164-15-541
Robinson, N., Sahoo, P. K., Baranski, M., Mahapatra, K. D., Saha, J. N., Das, S., et al. (2012). Expressed sequences and polymorphisms in rohu carp (Labeo rohita, Hamilton) revealed by mRNA-seq. Mar. Biotechnol. 14, 620–633. doi: 10.1007/s10126-012-9433-8
Roessig, J. M., Woodley, C. M., Cech, J. J., and Hansen, L. J. (2004). Effects of global climate change on marine and estuarine fishes and fisheries. Rev. Fish Biol. Fish. 14, 251–275. doi: 10.1007/s11160-004-6749-0
Saeed, M., and Plumb, J. (1986). Immune response of channel catfish to lipopolysaccharide and whole cell Edwardsiella ictaluri vaccines. Dis. Aquat. Organ. 2, 21–25. doi: 10.3354/dao002021
Santander, J., Kilbourne, J., Park, J.-Y., Martin, T., Loh, A., Diaz, I., et al. (2014). Inflammatory effects of Edwardsiella ictaluri lipopolysaccharide modifications in catfish gut. Infect. Immun. 82, 3394–3404. doi: 10.1128/IAI.01697-14
Schachte, J. H. (1983). Columnaris disease. A guide to integrated fish health management in the Great Lakes basin. Great Lakes Fish. Comm. Spec. Publ. 8, 199–203.
Semenza, G. L. (2000). HIF-1: mediator of physiological and pathophysiological responses to hypoxia. J. Appl. Physiol. 88, 1474–1480. doi: 10.1152/jappl.2000.88.4.1474
Shang, E. H., and Wu, R. S. (2004). Aquatic hypoxia is a teratogen and affects fish embryonic development. Environ. Sci. Technol. 38, 4763–4767. doi: 10.1021/es0496423
Shi, H., Zhou, T., Wang, X., Yang, Y., Wu, C., Liu, S., et al. (2018). Genome-wide association analysis of intra-specific QTL associated with the resistance for enteric septicemia of catfish. Mol. Genet. Genomics doi: 10.1007/s00438-018-1463-0 [Epub ahead of print].
Shiina, N., Tateno, H., Ogawa, T., Muramoto, K., Saneyoshi, M., and Kamiya, H. (2002). Isolation and characterization of L-rhamnose-binding lectins from chum salmon (Oncorhynchus keta) eggs. Fish. Sci. 68, 1352–1366. doi: 10.1046/j.1444-2906.2002.00575.x
Shoemaker, C., Olivares-Fuster, O., Arias, C., and Klesius, P. (2008). Flavobacterium columnare genomovar influences mortality in channel catfish (Ictalurus punctatus). Vet. Microbiol. 127, 353–359. doi: 10.1016/j.vetmic.2007.09.003
Shoemaker, C. A., Klesius, P. H., and Bricker, J. M. (1999). Efficacy of a modified live Edwardsiella ictaluri vaccine in channel catfish as young as seven days post hatch. Aquaculture 176, 189–193. doi: 10.1016/S0044-8486(99)00116-7
Shoemaker, C. A., Klesius, P. H., Drennan, J. D., and Evans, J. J. (2011). Efficacy of a modified live Flavobacterium columnare vaccine in fish. Fish Shellfish Immunol. 30, 304–308. doi: 10.1016/j.fsi.2010.11.001
Shoemaker, C. A., Klesius, P. H., and Evans, J. J. (2002). In ovo methods for utilizing the modified live Edwardsiella ictaluri vaccine against enteric septicemia in channel catfish. Aquaculture 203, 221–227. doi: 10.1016/S0044-8486(01)00631-7
Shoemaker, C. A., Klesius, P. H., and Evans, J. J. (2005). Modified live Flavobacterium columnare against columnaris disease in fish. U.S. Patent No US6881412B1. Washington, DC: US Department of Agriculture.
Shoemaker, C. A., Klesius, P. H., and Evans, J. J. (2006). Method of protecting fish against columnaris disease with modified live Flavobacterium columnare. U.S. Patent No US6991793B2. Washington, DC: US Department of Agriculture.
Shoemaker, C. A., Klesius, P. H., and Evans, J. J. (2007). Immunization of eyed channel catfish, Ictalurus punctatus, eggs with monovalent Flavobacterium columnare vaccine and bivalent F. columnare and Edwardsiella ictaluri vaccine. Vaccine 25, 1126–1131. doi: 10.1016/j.vaccine.2006.09.055
Skirpstunas, R. T., and Baldwin, T. J. (2002). Edwardsiella ictaluri invasion of IEC-6, Henle 407, fathead minnow and channel catfish enteric epithelial cells. Dis. Aquat. Organ. 51, 161–167. doi: 10.3354/dao051161
Song, L., Li, C., Xie, Y., Liu, S., Zhang, J., Yao, J., et al. (2016). Genome-wide identification of Hsp70 genes in channel catfish and their regulated expression after bacterial infection. Fish Shellfish Immunol. 49, 154–162. doi: 10.1016/j.fsi.2015.12.009
Song, L., Zhang, J., Li, C., Yao, J., Jiang, C., Li, Y., et al. (2014). Genome-wide identification of Hsp40 genes in channel catfish and their regulated expression after bacterial infection. PLoS One 9:e115752. doi: 10.1371/journal.pone.0115752
Srivastava, P. (2002). Roles of heat-shock proteins in innate and adaptive immunity. Nat. Rev. Immunol. 2, 185–194. doi: 10.1038/nri749
Stewart, H. A., and Allen, P. J. (2014). Critical thermal maxima of two geographic strains of channel and hybrid catfish. N. Am. J. Aquac. 76, 104–111. doi: 10.1080/15222055.2013.856827
Stewart, H. A., Bosworth, B., Petrie-Hanson, L., Martin, J. A., and Allen, P. J. (2015). Effects of chronic upper temperature regimes on growth of two geographical strains of channel and hybrid catfish. Aquac. Res. 46, 2407–2417. doi: 10.1111/are.12399
Sun, F., Peatman, E., Li, C., Liu, S., Jiang, Y., Zhou, Z., et al. (2012). Transcriptomic signatures of attachment, NF-κB suppression and IFN stimulation in the catfish gill following columnaris bacterial infection. Dev. Comp. Immunol. 38, 169–180. doi: 10.1016/j.dci.2012.05.006
Sun, L., Liu, S., Bao, L., Li, Y., Feng, J., and Liu, Z. (2015). Claudin multigene family in channel catfish and their expression profiles in response to bacterial infection and hypoxia as revealed by meta-analysis of RNA-Seq datasets. Comp. Biochem. Physiol. Part D Genomics Proteomics 13, 60–69. doi: 10.1016/j.cbd.2015.01.002
Sun, L., Liu, S., Wang, R., Li, C., Zhang, J., and Liu, Z. (2014). Pathogen recognition receptors in channel catfish: IV. Identification, phylogeny and expression analysis of peptidoglycan recognition proteins. Dev. Comp. Immunol. 46, 291–299. doi: 10.1016/j.dci.2014.04.018
Tan, S., Yao, J., Zhou, T., Liu, S., Yuan, Z., Tian, C., et al. (2017). Identification, annotation and expression analysis of 29 Rho GTPase genes from channel catfish (Ictalurus punctatus) after bacterial infections. Dev. Comp. Immunol. 67, 445–451. doi: 10.1016/j.dci.2016.10.005
Tan, S., Zhou, T., Wang, W., Jin, Y., Wang, X., Geng, X., et al. (2018). GWAS analysis using interspecific backcross progenies reveals superior blue catfish alleles responsible for strong resistance against enteric septicemia of catfish. Mol. Genet. Genomics doi: 10.1007/s00438-018-1443-4 [Epub ahead of print].
Tateno, H., Ogawa, T., Muramoto, K., Kamiya, H., and Saneyoshi, M. (2002). Rhamnose-binding lectins from steelhead trout (Oncorhynchus mykiss) eggs recognize bacterial lipopolysaccharides and lipoteichoic acid. Biosci. Biotechnol. Biochem. 66, 604–612. doi: 10.1271/bbb.66.604
Tavares-Dias, M., and Moraes, F. (2007). Haematological and biochemical reference intervals for farmed channel catfish. J. Fish Biol. 71, 383–388. doi: 10.1111/j.1095-8649.2007.01494.x
Tekedar, H. C., Waldbieser, G. C., Karsi, A., Liles, M. R., Griffin, M. J., Vamenta, S., et al. (2013). Complete genome sequence of a channel catfish epidemic isolate, Aeromonas hydrophila strain ML09-119. Genome Announc. 1, e755-13. doi: 10.1128/genomeA.00755-13
Thongda, W., Li, C., Luo, Y., Beck, B. H., and Peatman, E. (2014). L-rhamnose-binding lectins (RBLs) in channel catfish, Ictalurus punctatus: characterization and expression profiling in mucosal tissues. Dev. Comp. Immunol. 44, 320–331. doi: 10.1016/j.dci.2014.01.018
Thune, R. L., Hawke, J. P., and Johnson, M. C. (1994). Studies on vaccination of channel catfish, Ictalurus punctatus, against Edwardsiella ictaluri. J. Appl. Aquac. 3, 11–24. doi: 10.1300/J028v03n01_02
Tian, Y., Yao, J., Liu, S., Jiang, C., Zhang, J., Li, Y., et al. (2015). Identification and expression analysis of 26 oncogenes of the receptor tyrosine kinase family in channel catfish after bacterial infection and hypoxic stress. Comp. Biochem. Physiol. Part D Genomics Proteomics 14, 16–25. doi: 10.1016/j.cbd.2015.02.001
Todd, A. S., Coleman, M. A., Konowal, A. M., May, M. K., Johnson, S., Vieira, N. K., et al. (2008). Development of new water temperature criteria to protect Colorado’s fisheries. Fisheries 33, 433–443. doi: 10.1577/1548-8446-33.9.433
Tomás, J. (2012). The main Aeromonas pathogenic factors. ISRN Microbiol. 2012:256261. doi: 10.5402/2012/256261
Triyanto, W. (1999). Genotypic diversity of strains of Flavobacterium columnare from diseased fishes. Fish Pathol. 34, 65–71. doi: 10.3147/jsfp.34.65
Tucker, C. S., and Hargreaves, J. A. (2004). Biology and Culture of Channel Catfish. New York, NY: Elsevier.
Ventura, M., and Grizzle, J. M. (1987). Evaluation of portals of entry of Aeromonas hydrophila in channel catfish. Aquaculture 65, 205–214. doi: 10.1016/0044-8486(87)90232-8
Wagner, B. A., Wise, D. J., Khoo, L. H., and Terhune, J. S. (2002). The epidemiology of bacterial diseases in food-size channel catfish. J. Aquat. Anim. Health 14, 263–272. doi: 10.1577/1548-86672002014
Wakabayashi, H. (1999). Genotypic diversity of strains of Flavobacterium columnare from diseased fishes. Fish Pathol. 34, 65–71. doi: 10.3147/jsfp.34.65
Wang, R., Song, L., Su, B., Zhao, H., Zhang, D., Peatman, E., et al. (2015). Mucosal expression signatures of two Cathepsin L in channel catfish (Ictalurus punctatus) following bacterial challenge. Fish Shellfish Immunol. 47, 582–589. doi: 10.1016/j.fsi.2015.09.047
Wang, R., Sun, L., Bao, L., Zhang, J., Jiang, Y., Yao, J., et al. (2013). Bulk segregant RNA-seq reveals expression and positional candidate genes and allele-specific expression for disease resistance against enteric septicemia of catfish. BMC Genomics 14:929. doi: 10.1186/1471-2164-14-929
Wang, R., Zhang, Y., Liu, S., Li, C., Sun, L., Bao, L., et al. (2014). Analysis of 52 Rab GTPases from channel catfish and their involvement in immune responses after bacterial infections. Dev. Comp. Immunol. 45, 21–34. doi: 10.1016/j.dci.2014.01.026
Wang, X., Li, C., Thongda, W., Luo, Y., Beck, B., and Peatman, E. (2014). Characterization and mucosal responses of interleukin 17 family ligand and receptor genes in channel catfish Ictalurus punctatus. Fish Shellfish Immunol. 38, 47–55. doi: 10.1016/j.fsi.2014.02.020
Wang, R. H., Xiao, T. Y., Zeng, L. B., Liu, X. Y., Zhou, Y., and Ma, J. (2016). Generation and use of Edwardsiella ictaluri ghosts as a vaccine against enteric septicemia of catfish (ESC). Aquaculture 456, 9–15. doi: 10.1016/j.aquaculture.2016.01.017
Wang, X., Liu, S., Dunham, R., and Liu, Z. (2017a). Effects of strain and body weight on low-oxygen tolerance of channel catfish (Ictalurus punctatus). Aquac. Int. 25, 1645–1652. doi: 10.1007/s10499-017-0125-2
Wang, X., Liu, S., Jiang, C., Geng, X., Zhou, T., Li, N., et al. (2017b). Multiple across-strain and within-strain QTLs suggest highly complex genetic architecture for hypoxia tolerance in channel catfish. Mol. Genet. Genomics 292, 63–76. doi: 10.1007/s00438-016-1256-2
Wang, X., Liu, S., Yang, Y., Fu, Q., Abebe, A., and Liu, Z. (2017c). Identification of NF-κB related genes in channel catfish and their expression profiles in mucosal tissues after columnaris bacterial infection. Dev. Comp. Immunol. 70, 27–38. doi: 10.1016/j.dci.2017.01.003
Watanabe, Y., Tateno, H., Nakamura-Tsuruta, S., Kominami, J., Hirabayashi, J., Nakamura, O., et al. (2009). The function of rhamnose-binding lectin in innate immunity by restricted binding to Gb3. Dev. Comp. Immunol. 33, 187–197. doi: 10.1016/j.dci.2008.08.008
Welch, W. (1990). “The mammalian stress response: cell physiology and biochemistry of stress proteins,” in Stress Proteins in Biology and Medicine, eds R. I. Morimoto, A. Tissières, and C. Georgopoulos (New York, NY: Cold Spring Harbor Laboratory Press), 223–278.
Welker, T. L., Mcnulty, S. T., and Klesius, P. H. (2007). Effect of sublethal hypoxia on the immune response and susceptibility of channel catfish, Ictalurus punctatus, to enteric septicemia. J. World Aquac. Soc. 38, 12–23. doi: 10.1111/j.1749-7345.2006.00069.x
Wise, D. J., Greenway, T. E., Byars, T. S., Griffin, M. J., and Khoo, L. H. (2015). Oral vaccination of channel catfish against enteric septicemia of catfish using a live attenuated Edwardsiella ictaluri Isolate. J. Aquat. Anim. Health 27, 135–143. doi: 10.1080/08997659.2015.1032440
Wise, D. J., Klesius, P. H., Shoemaker, C. A., and Wolters, W. R. (2000). Vaccination of mixed and full-sib families of channel catfish Ictalurus punctatus against enteric septicemia of catfish with a live attenuated Edwardsiella ictaluri Isolate (RE-33). J. World Aquac. Soc. 31, 206–212. doi: 10.1111/j.1749-7345.2000.tb00354.x
Wolters, W. R., and Johnson, M. R. (1994). Enteric septicemia resistance in blue catfish and three channel catfish strains. J. Aquat. Anim. Health 6, 329–334. doi: 10.1577/1548-8667(1994)006<0329:ESRIBC>2.3.CO;2
Wolters, W. R., Wise, D. J., and Klesius, P. H. (1996). Survival and antibody response of channel catfish, blue catfish, and channel catfish female × blue catfish male hybrids after exposure to Edwardsiella ictaluri. J. Aquat. Anim. Health 8, 249–254. doi: 10.1577/1548-8667(1996)008<0249:SAAROC>2.3.CO;2
Wu, R. S. (2002). Hypoxia: from molecular responses to ecosystem responses. Mar. Pollut. Bull. 45, 35–45. doi: 10.1016/S0025-326X(02)00061-9
Xie, Y., Song, L., Weng, Z., Liu, S., and Liu, Z. (2015). Hsp90, Hsp60 and sHsp families of heat shock protein genes in channel catfish and their expression after bacterial infections. Fish Shellfish Immunol. 44, 642–651. doi: 10.1016/j.fsi.2015.03.027
Xu, X., Shen, Y., Fu, J., Lu, L., and Li, J. (2014). De novo assembly of the grass carp Ctenopharyngodon idella transcriptome to identify miRNA targets associated with motile aeromonad septicemia. PLoS One 9:e112722. doi: 10.1371/journal.pone.0112722
Xu, X., Shen, Y., Fu, J., Lu, L., and Li, J. (2015). Next-generation sequencing identified microRNAs that associate with motile aeromonad septicemia in grass carp. Fish Shellfish Immunol. 45, 94–103. doi: 10.1016/j.fsi.2015.02.008
Xu, Z., Chen, J., Li, X., Ge, J., Pan, J., and Xu, X. (2013). Identification and characterization of microRNAs in channel catfish (Ictalurus punctatus) by using Solexa sequencing technology. PLoS One 8:e54174. doi: 10.1371/journal.pone.0054174
Yang, Q., Pan, Y.-L., Wang, K.-Y., Wang, J., He, Y., Wang, E.-L., et al. (2016). OmpN, outer membrane proteins of Edwardsiella ictaluri are potential vaccine candidates for channel catfish (Ictalurus punctatus). Mol. Immunol. 78, 1–8. doi: 10.1016/j.molimm.2016.08.011
Yang, Y., Yu, H., Li, H., and Wang, A. (2016). Transcriptome profiling of grass carp (Ctenopharyngodon idellus) infected with Aeromonas hydrophila. Fish Shellfish Immunol. 51, 329–336. doi: 10.1016/j.fsi.2016.02.035
Yang, Y., Fu, Q., Wang, X., Liu, Y., Zeng, Q., Li, Y., et al. (2018). Comparative transcriptome analysis of the swimbladder reveals expression signatures in response to low oxygen stress in channel catfish, Ictalurus punctatus. Physiol. Genomics doi: 10.1152/physiolgenomics.00125.2017 [Epub ahead of print].
Yang, Y., Fu, Q., Zhou, T., Li, Y., Liu, S., Zeng, Q., et al. (2017). Analysis of apolipoprotein genes and their involvement in disease response of channel catfish after bacterial infection. Dev. Comp. Immunol. 67, 464–470. doi: 10.1016/j.dci.2016.09.007
Yao, J., Li, C., Zhang, J., Liu, S., Feng, J., Wang, R., et al. (2014). Expression of nitric oxide synthase (NOS) genes in channel catfish is highly regulated and time dependent after bacterial challenges. Dev. Comp. Immunol. 45, 74–86. doi: 10.1016/j.dci.2014.02.005
Yao, J., Mu, W., Liu, S., Zhang, J., Wen, H., and Liu, Z. (2015). Identification, phylogeny and expression analysis of suppressors of cytokine signaling in channel catfish. Mol. Immunol. 64, 276–284. doi: 10.1016/j.molimm.2014.12.003
Yeh, H.-Y., and Klesius, P. H. (2009). Channel catfish, Ictalurus punctatus, cysteine proteinases: cloning, characterisation and expression of cathepsin H and L. Fish Shellfish Immunol. 26, 332–338. doi: 10.1016/j.fsi.2008.11.010
Yuan, Z., Liu, S., Yao, J., Zeng, Q., Tan, S., and Liu, Z. (2016). Expression of Bcl-2 genes in channel catfish after bacterial infection and hypoxia stress. Dev. Comp. Immunol. 65, 79–90. doi: 10.1016/j.dci.2016.06.018
Zeng, Q., Fu, Q., Li, Y., Waldbieser, G., Bosworth, B., Liu, S., et al. (2017). Development of a 690K SNP array in catfish and its application for genetic mapping and validation of the reference genome sequence. Sci. Rep. 7:40347. doi: 10.1038/srep40347
Zhang, D., Thongda, W., Li, C., Zhao, H., Beck, B. H., Mohammed, H., et al. (2017). More than just antibodies: protective mechanisms of a mucosal vaccine against fish pathogen Flavobacterium columnare. Fish Shellfish Immunol. 71, 160–170. doi: 10.1016/j.fsi.2017.10.001
Zhang, J., Gilbert, D., Gooday, A., Levin, L., Naqvi, S., Middelburg, J., et al. (2010). Natural and human-induced hypoxia and consequences for coastal areas: synthesis and future development. Biogeosciences 7, 1443–1467. doi: 10.5194/bg-7-1443-2010
Zhang, J., Yao, J., Wang, R., Zhang, Y., Liu, S., Sun, L., et al. (2014). The cytochrome P450 genes of channel catfish: their involvement in disease defense responses as revealed by meta-analysis of RNA-Seq data sets. Biochim. Biophys. Acta 1840, 2813–2828. doi: 10.1016/j.bbagen.2014.04.016
Zhong, X., Wang, X., Zhou, T., Jin, Y., Tan, S., Jiang, C., et al. (2017). Genome-wide association study reveals multiple novel QTL associated with low oxygen tolerance in hybrid catfish. Mar. Biotechnol. 19, 379–390. doi: 10.1007/s10126-017-9757-5
Zhou, S., Zhao, H., Thongda, W., Zhang, D., Su, B., Yu, D., et al. (2016). Galectins in channel catfish, Ictalurus punctatus: characterization and expression profiling in mucosal tissues. Fish Shellfish Immunol. 49, 324–335. doi: 10.1016/j.fsi.2016.01.005
Zhou, T., Li, N., Liu, S., Jin, Y., Fu, Q., Gao, S., et al. (2017a). The NCK and ABI adaptor genes in catfish and their involvement in ESC disease response. Dev. Comp. Immunol. 73, 119–123. doi: 10.1016/j.dci.2017.03.016
Zhou, T., Liu, S., Geng, X., Jin, Y., Jiang, C., Bao, L., et al. (2017b). GWAS analysis of QTL for enteric septicemia of catfish and their involved genes suggest evolutionary conservation of a molecular mechanism of disease resistance. Mol. Genet. Genomics 292, 231–242. doi: 10.1007/s00438-016-1269-x
Zhu, Q., Zhang, L., Li, L., Que, H., and Zhang, G. (2016). Expression characterization of stress genes under high and low temperature stresses in the Pacific oyster, Crassostrea gigas. Mar. Biotechnol. 18, 176–188. doi: 10.1007/s10126-015-9678-0
Zhu, R., Liu, X.-X., Lv, X., Li, S.-Y., Li, Y.-D., Yu, X.-J., et al. (2017). Deciphering transcriptome profile of the yellow catfish (Pelteobagrus fulvidraco) in response to Edwardsiella ictaluri. Fish Shellfish Immunol. 70, 593–608. doi: 10.1016/j.fsi.2017.08.040
Keywords: enteric septicemia of catfish, columnaris, Aeromonas, heat, hypoxia, disease, stress, quantitative trait locus
Citation: Zhou T, Yuan Z, Tan S, Jin Y, Yang Y, Shi H, Wang W, Niu D, Gao L, Jiang W, Gao D and Liu Z (2018) A Review of Molecular Responses of Catfish to Bacterial Diseases and Abiotic Stresses. Front. Physiol. 9:1113. doi: 10.3389/fphys.2018.01113
Received: 29 April 2018; Accepted: 25 July 2018;
Published: 23 August 2018.
Edited by:
Nour Eissa, University of Manitoba, CanadaReviewed by:
Mamdouh Yousif Elgendy, National Research Centre, EgyptAlexssandro Geferson Becker, Universidade Federal do Paraná, Brazil
Copyright © 2018 Zhou, Yuan, Tan, Jin, Yang, Shi, Wang, Niu, Gao, Jiang, Gao and Liu. This is an open-access article distributed under the terms of the Creative Commons Attribution License (CC BY). The use, distribution or reproduction in other forums is permitted, provided the original author(s) and the copyright owner(s) are credited and that the original publication in this journal is cited, in accordance with accepted academic practice. No use, distribution or reproduction is permitted which does not comply with these terms.
*Correspondence: Zhanjiang Liu, am9obmxpdUBzeXIuZWR1
†These authors have contributed equally to this work