- 1Helsinki Institute of Life Science, University of Helsinki, Helsinki, Finland
- 2Medicum, University of Helsinki, Helsinki, Finland
- 3Developmental Biology Division, Cincinnati Children’s Hospital, Cincinnati, OH, United States
- 4GM-Unit, Laboratory Animal Centre, Helsinki Institute of Life Science, University of Helsinki, Helsinki, Finland
Classically, trophic factors are considered as proteins which support neurons in their growth, survival, and differentiation. However, most neurotrophic factors also have important functions outside of the nervous system. Especially essential renal growth and differentiation regulators are glial cell line-derived neurotrophic factor (GDNF), bone morphogenetic proteins (BMPs), and fibroblast growth factors (FGFs). Here we discuss how trophic factor-induced signaling contributes to the control of ureteric bud (UB) branching morphogenesis and to maintenance and differentiation of nephrogenic mesenchyme in embryonic kidney. The review includes recent advances in trophic factor functions during the guidance of branching morphogenesis and self-renewal versus differentiation decisions, both of which dictate the control of kidney size and nephron number. Creative utilization of current information may help better recapitulate renal differentiation in vitro, but it is obvious that significantly more basic knowledge is needed for development of regeneration-based renal therapies.
Introduction
Trophic factors, also known as neurotrophic factors, have important functions outside of the nervous system (Sariola, 2001; Vega et al., 2003; Prakash et al., 2010), especially transforming growth factor beta (TGFβ) superfamily members glial cell line-derived neurotrophic factor (GDNF), bone morphogenetic proteins (BMPs), and fibroblast growth factors (FGFs), which all together with their receptors and modulators are essential for normal renal development. Other trophic factors, such as vascular endothelial growth factor (VEGF), leukemia inhibitory factor (LIF), and ephrins are less studied but also show important functions in developing functional kidneys (Weiss and Kispert, 2016).
Nephron endowment is established during the embryonic period of an individual and critically dictates renal health and function in adults; hence, a detailed understanding of the signals and events regulating renal differentiation is a necessity. Our current understanding of mammalian kidney development derives from experiments in mice, and to a lesser extent in rats and zebrafish (Davidson, 2008; Cheng et al., 2015; Drummond and Davidson, 2016). The great advances made in characterizing renal differentiation in humans during the past few years (Saraga-Babic et al., 2012; O’Brien et al., 2016; Lindstrom et al., 2018a,b,c,d; Menon et al., 2018; Wang et al., 2018) allows us to begin to ponder previous knowledge in the light of these new findings. Importantly, though these studies identified differences in rodent and human kidney development, the molecular and cellular regulation of kidney organogenesis in these species remains relatively conserved. In this review, we discuss in detail how trophic factors regulate the two major morphogenetic processes of kidney development: ureteric bud (UB) branching and nephron differentiation (Figure 1). The third important component, the stroma, is included to a significantly lesser extent. We largely focus on rodent experiments, and embryonic staging is defined for mice, if not stated otherwise. The corresponding events and timing in human fetuses were reviewed recently (Cullen-McEwen et al., 2016).
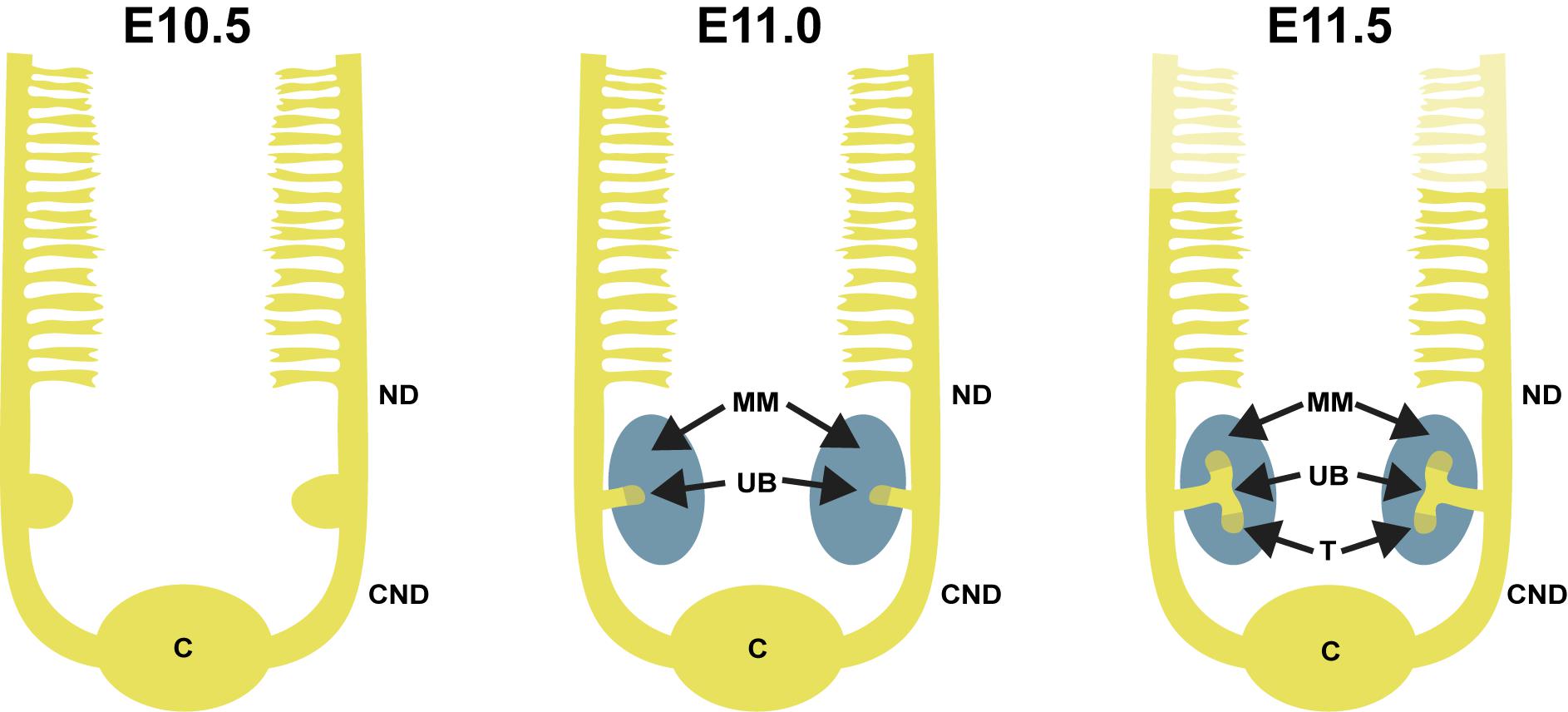
FIGURE 1. Overview of early kidney development in mouse. At embryonic day 11.0, the ureteric bud (UB) grows out from the nephric duct (ND) and enters the metanephric mesenchyme (MM). By E11.5, the UB bifurcates into two distinct UB tips (T). The cloaca is indicated as (C), and the common nephric duct is indicated as (CND).
Mouse Kidney Development – an Overview
Kidneys, like lungs, mammary and salivary glands, prostate, and seminal vesicles develop through branching morphogenesis. The characteristic growth and shape of these organs is achieved via novel branch generation that is highly specific for each organ. Thus, the pattern of epithelial branching during the differentiation of lungs is very different from that in kidneys and is largely dictated by the signals derived from the surrounding cells in the nascent mesenchymal compartment (Saxen, 1987; Lin et al., 2001).
Renal development in mammals is a multistage process during which three spatially and temporally distinct kidneys, the pronephros, mesonephros, and metanephros are formed (Figure 1). These all derive from intermediate mesoderm by spatially and temporally distinct processes (Taguchi et al., 2014; Takasato and Little, 2015). Paired nephric ducts emerge at embryonic day 8.75 (E8.75) in mice and day 28 in humans, and grow posteriorly until they reach the cloaca while inducing lateral mesonephric tubular structures (Costantini and Kopan, 2010; Little and McMahon, 2012; Combes et al., 2015). Pro- and mesonephos are transient structures that disappear by mid-gestation except in males, where the most caudal tubules of mesonephros and distal nephric duct differentiate into ductuli efferents, epididymis, vas deferens, and seminal vesicles (Jacob et al., 2012).
Ureteric Bud Branching
The definitive kidney, the metanephros, begins forming when metanephric mesenchyme (MM) cells induce outgrowth of the UB from the nephric duct at E10.5 in mice (weeks 4–5 of gestation in humans). Subsequent renal morphogenesis is guided by reciprocal inductive interactions between the MM and the UB. After the initial UB outgrowth, it secretes signals that induce the MM to condense around the growing UB tip, forming the cap mesenchyme (CM). This is the beginning of CM-guided UB branching morphogenesis that happens rapidly until mid-gestation and slows down after E15.5 (Cebrian et al., 2004; Short et al., 2014).
UB branching is reiterated for multiple rounds to form the entirety of the collecting duct system (Cebrian et al., 2004; Short et al., 2014). Throughout kidney development, the UB is compartmentalized into two regions, the tip and the stalk, which contain cells with distinct characteristics (Figure 2). Tip cells are the immature, proliferating cells that interact with the MM, while stalk cells, which are derivatives of tips, differentiate to form the collecting duct system (Michael and Davies, 2004; Shakya et al., 2005). The progenitor pool for the entire collecting duct system thus lies in the UB tip, and the stalk is formed by left behind tip cells. It remains unclear how specific signaling pathways control UB tip properties, but RTK signaling has been shown to keep UB cells in the tip niche (Chi et al., 2009; Kuure et al., 2010b).
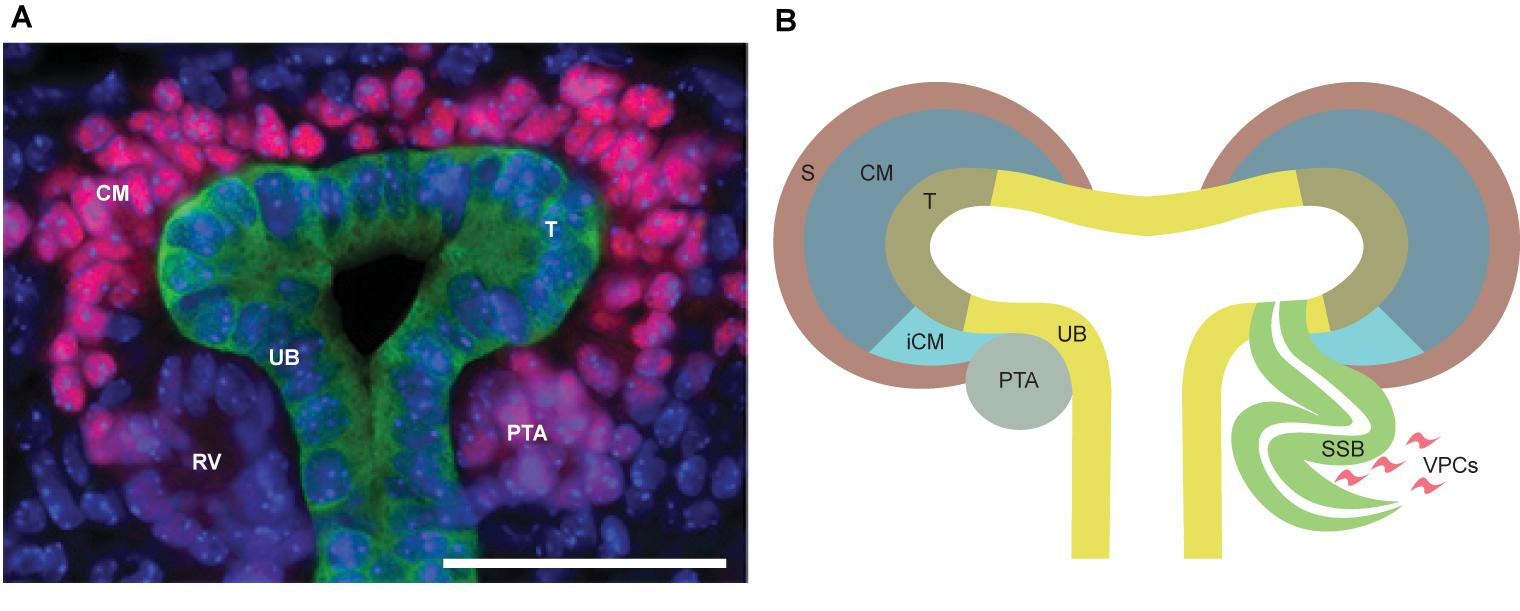
FIGURE 2. (A) In vivo representation of developing kidney at embryonic day 15.5. SIX2 staining (pink) strongly localizes to the nephron progenitors in the cap mesenchyme (CM), while being less intensive in pretubular aggregate (PTA) and almost non-existent in renal vesicle (RV). Calbindin staining (green) highlights ureteric bud (UB) epithelium. Scale bar is 50 μm. (B) Schematic depiction of nephrogenesis. The UB is segmented into molecularly-distinct tip (T, olive green) and stalk (UB, yellow) regions. UB tips are surrounded by CM (dark blue), including the nephron progenitors and the surrounding stroma (S, brown). A subset of the nephron progenitors in CM are induced (iCM, turquoise) to differentiate. The first differentiating nephron precursor structure is a pre-tubular aggregate (PTA, gray), which through epithelialization, turns into an S-shaped body (SSB, green) that will eventually become the functional nephron. Vascular endothelial progenitor cells (VPCs, pink) enter the cleft of the S-shaped body to begin formation of the glomerular capillary bed.
Nephron Differentiation
Nephrons are derived from a multipotent, self-renewing progenitor population of CM cells wrapping around the UB tip (Sariola, 2002; Boyle et al., 2008; Kobayashi et al., 2008). The size of the progenitor population determines the final nephron number, as indicated by progenitor depletion studies (Cebrian et al., 2014). The nephron progenitor (NP) cells rely on contacts with each other as well as with the UB cells and the interstitial progenitors to establish the progenitor niche (Figure 1), although the exact mechanisms behind niche arrangement are only beginning to be elucidated (Ihermann-Hella et al., 2018). During the active branching process, the NP population is maintained, but a subset of NP cells are also induced to differentiate and cluster to form a pretubular aggregate beneath the UB tip. The pretubular aggregate begins to epithelialize and forms the renal vesicle, which grows and is subsequently patterned into a comma-shaped and S-shaped body. The distal epithelium of the S-shaped body differentiates into the distal and connecting tubule that plumb into the collecting duct system allowing fluid to flow from the kidney. The proximal and medial segments of the S-shaped body give rise to the glomerulus and proximal tubule/Loop of Henle, respectively (Saxen and Sariola, 1987; Georgas et al., 2009). Glomerular capillary loop differentiation begins in the cleft of S-shaped body (Figure 2) simultaneously to specification of podocytes in the proximal epithelium.
After cessation of branching morphogenesis, nephrogenesis continues to produce the final number of nephrons in an individual (Cebrian et al., 2004; Short et al., 2014). In humans, nephron induction ceases in utero (by week 36), while in mice it lasts until post-natal day three (P3) (Cebrian et al., 2004; Hartman et al., 2007; Rumballe et al., 2011; Short et al., 2014). The mechanisms driving cessation of nephrogenesis involve loss of self-renewal in the progenitor cells that instead undergo differentiation. The molecular mechanisms driving this process are poorly understood, but recent studies identified Hamartin (encoded by Tsc1) as a possible regulator of progenitor aging (Volovelsky et al., 2018).
Vascularization and Innervation
Development of the renal vasculature is not well understood, despite its critical role in renal function and overall health. Vascularization of the mouse kidney likely occurs via more than one developmental route (Abrahamson et al., 1998). Tracing of vascular development in vivo and studies of organ explants suggest that the main arterial network in the kidney develops independently of the glomerular vasculature. Initially, OSR1-positive precursors give rise to glomerular vascular progenitors, while at later stages in development, FLK1-positive vascular progenitors are present in the UB tip niche (Hyink et al., 1996; Mugford et al., 2008, 2009). It was also demonstrated that FLK1-positive progenitors can give rise to primitive vascular networks in cultured kidney rudiments (Tufro-McReddie et al., 1997). However, conclusive evidence of distinct developmental pathways has yet to be found.
The renal arterial tree begins forming at E12.5 in mice when several branches from the aorta enter the kidney rudiment. This network is remodeled into the single renal artery with 3–4 main lateral branches by E13.5. At E17.5, the main arterial branches extend through the medulla and terminate in the cortex where they are extensively branched to bring blood to the glomeruli (Herzlinger and Hurtado, 2014). It is not yet known which signaling factors play roles in this branching, but one possible mediator is the renin-angiotensin system which was shown to mediate vascular branching in post-natal rat kidneys (Tufro-McReddie et al., 1995).
In addition to vascularization, the functional kidney requires a neuronal network. The pattern of sympathetic innervation is closely linked to the arterial pattern in the kidney, which could indicate that the development of both networks is coordinated by the same or similar cues. Renal stroma exhibits neuronal-like cells (Sainio et al., 1994), and kidney organogenesis requires functional nerve growth factor receptor (Sariola et al., 1991). Although the regulation of the sympathetic nervous system in adults is well studied, understanding of the innervation process during renal organogenesis is largely lacking (Sariola et al., 1988).
The Trophic Factor Signaling Pathways Essential for Renal Differentiation
GDNF-RET Signaling
GDNF was first isolated from cultures of rat glial cell line B49 and identified as a distant member of the TGFβ superfamily with potent neurotrophic activity (Lin et al., 1993). The ability of GDNF to promote survival and differentiation of dopaminergic neurons immediately prompted further research into its use for the treatment of Parkinson’s disease. However, the blood-brain barrier prevents the use of systemic GDNF and intracranial infusion often fails to deliver sufficient GDNF to the target areas of the brain, stalling its use as a therapeutic agent for the disease (reviewed in Patel and Gill, 2007; Grondin et al., 2018). Modifications in Neurturin (NRTN), another family member expressed in developing kidney, show better spreading inside the brain (Runeberg-Roos et al., 2016).
Ret proto-oncogene was characterized first in humans (Takahashi et al., 1988) and later in mice (Iwamoto et al., 1993; Pachnis et al., 1993) as an orphan tyrosine kinase receptor after which it was shortly identified as the receptor for GDNF (Durbec et al., 1996; Trupp et al., 1996). GDNF requires binding to GFRα1, a GPI-anchored cell surface co-receptor, to acquire high affinity for RET. Binding of GDNF/GFRα1 double homodimers to RET induces RET dimerization and autophosphorylation of multiple tyrosine residues. As a result, downstream signaling cascades including SRC, PLCγ, RAS-MAPK, PI3K-AKT, NFkB and JNK are activated (Figure 3; Hayashi et al., 2000; Asai et al., 2006; Davis et al., 2014). Additional complexity of intracellular signaling induction comes from alternative splicing of Ret resulting in three different isoforms in humans (RET9, 43, and 51) (Arighi et al., 2005).
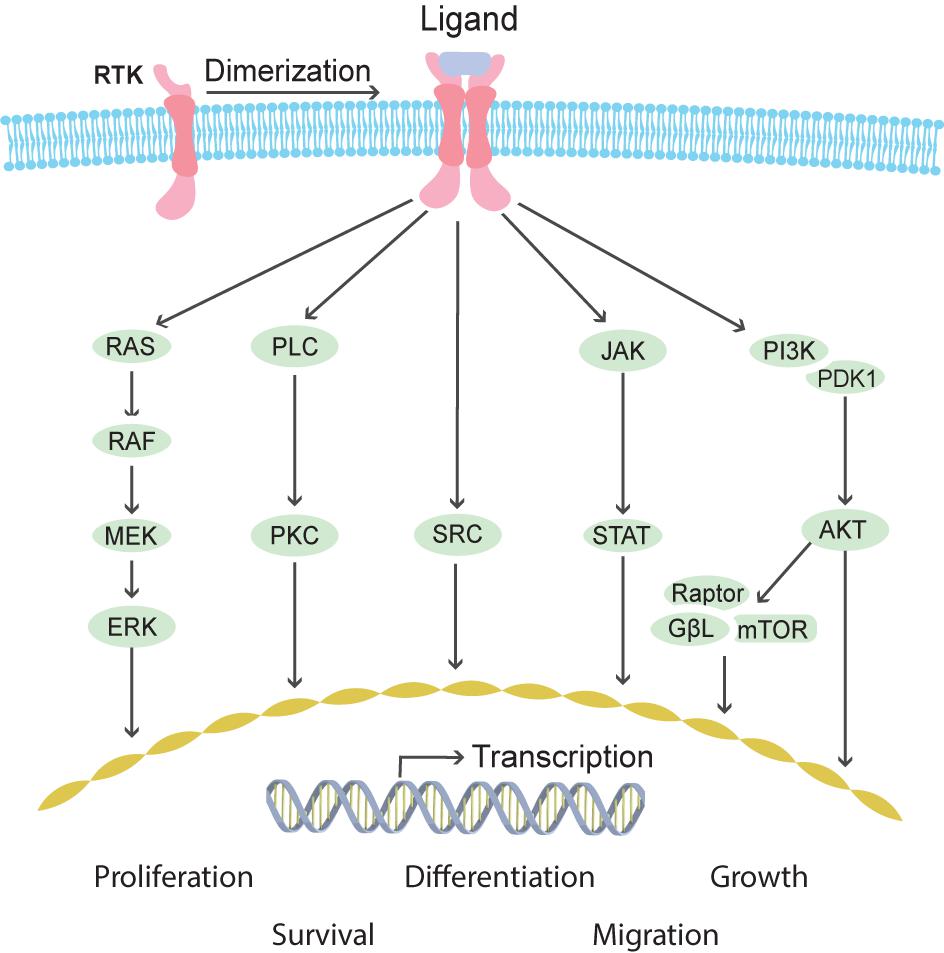
FIGURE 3. Schematic overview of pathways activated downstream of receptor tyrosine kinase (RTK) signaling. RTK receptors mediate outside signals to the interior of the cell through the cell membrane penetrating domains. Extracellular ligand binding induces receptor dimerization, which activates several intracellular cascades including MAPK, PLC/PKC, SRC, JAK/STAT, and PI3K/AKT. These signaling pathways induce transcriptional responses in the nucleus and changes of protein activities in the cytosol leading to cellular reactions such as proliferation, differentiation, growth, survival, and migration.
In the shadow of its neurotrophic role, GDNF is also expressed in other organs including the developing kidney, testes, stomach, and intestine (Hellmich et al., 1996; Golden et al., 1999). The developmental role for GDNF in these organs became evident upon the generation of mice with a null mutation in GDNF; these mice showed kidney agenesis or severely hypoplastic kidneys as well as defective enteric innervation (Moore et al., 1996; Pichel et al., 1996; Sanchez et al., 1996). Indeed, mouse models with null mutations in Ret or Gfrα1 demonstrated that signaling through GDNF-RET-GFRα1 is critical for kidney development, as these mice also present renal agenesis or severe renal hypoplasia (Schuchardt et al., 1994; Cacalano et al., 1998).
FGF Signaling
The family of FGFs signal through receptor tyrosine kinase (RTK) receptors (Figure 2) to regulate organogenesis, tissue homeostasis, and metabolism via its effects on proliferation, survival, migration, and differentiation (Eswarakumar et al., 2005; Turner and Grose, 2010; Hu et al., 2013). The manifold functions in most, if not all, mammalian cell types reflect the multitude of ligands identified in mammals and alternative splicing of their receptors. A total of 22 FGF ligands, a single non-signaling receptor, and four signaling receptors have been identified to date. Of the ligands, 18 signal through FGF RTKs, of which FGFR1-3 are generally present in two major isoforms (IIIb or IIIc) (Miki et al., 1992; Chellaiah et al., 1994; Zhang et al., 2006).
Tight regulation of FGF signaling, or RTK in general, takes place at many levels, and occurs both extra- and intracellularly. It involves heparin/heparin sulfate proteoglygans (HSPG), non-TK FGFR (FGFL1), FRS2α, and Sprouty proteins (Bullock et al., 1998; Walker et al., 2016). Heparin and HSPG not only bind secreted ligands to restrict their diffusion in the extracellular matrix but also act as cofactors to provide more specificity and affinity (Rapraeger et al., 1991; Yayon et al., 1991; Matsuo and Kimura-Yoshida, 2013). Intracellular FRS2α and Sprouty largely modulate the timing, strength, and location of RAS-MAPK and PI3K-AKT cascades activation (Gotoh, 2008; Yu et al., 2011).
A multitude of FGF ligands and receptors are expressed in the developing kidney (Cancilla et al., 1999; Dudley et al., 1999). Typically, epithelial tissues, including the UB, express Fgfr1 and -2 IIIb splice variants while their ligands are usually expressed by the mesenchyme (Bellusci et al., 1997; Beyer et al., 2003). Conversely, Fgfr1-2 IIIc variants localize to mesenchymal tissues and bind ligands present in the epithelium (MacArthur et al., 1995; Sun et al., 2002). Deletion of either Frfr3 or -4 does not interfere with renal development, supporting the major signaling function for the two other receptors (Colvin et al., 1996; Weinstein et al., 1998). The function of FGFs in renal differentiation was recently reviewed (Walker et al., 2016).
Eph/Ephrin Signaling
A bidirectional signaling module composed of 16 erythropoietin-producing hepatocellular (Eph) RTKs and nine Eph-receptor-interacting proteins (ephrins) comprises the largest RTK family in mammals (Eph-Nomenclature-Committee, 1997; Kullander and Klein, 2002). Based on sequence and function, both Ephs and ephrins are divided into A and B subfamilies with promiscuous receptor-ligand pairing within the same subfamily. A glimpse of further complexity in Eph signaling comes from the fact that both Ephs and ephrins can act as signal transducing receptors.
Activation of Eph/ephrin signaling cascades occurs exclusively upon direct contact of juxtaposed cells. Forward signaling in Eph-expressing cells triggers a large number of signal-mediating, kinase-dependent or -independent pathways, while reverse signaling in the ephrin-expressing cell causes focal adhesion kinase activation, cytoskeletal changes, and/or transcriptional responses (Binns et al., 2000; Palmer et al., 2002). The cellular responses to Eph/ephrin signaling diverge from those with RTK signaling and include cell adhesion, repulsion initiation, migration, and mitogenesis. Functionally, Eph/ephrin signaling is best recognized by several aspects of neuronal connectivity (Davy and Soriano, 2005). However, it is becoming evident that angiogenesis, craniofacial development, intestinal homeostasis, cancer, and skeletal development/homeostasis also require Eph/ephrin signaling (Bush and Soriano, 2012). Eph/ephrin signaling–mediated cell and tissue interactions in the developing kidney and urogenital system are discussed in the Regulation of vasculature development section.
TGFβ and BMP Signaling
The TGFβ superfamily of secreted proteins contains at least 30 members and includes Activins, Nodals, BMPs, and Growth and Differentiation Factors (GDFs). Due to its large presentation in living organisms, it also has major functions in many diverse contexts, including developmental, homeostatic, and disease processes where members of this signaling family control basically all cell biology aspects (Weiss and Attisano, 2013). For the focus of this review, the most relevant TGFβ family members are GDNF (described earlier), BMPs, and TGFβ2.
TGFβ2, similarly, to its family members, is synthesized as a precursor and forms homodimers to activate a heteromeric receptor complex composed of type I or II transmembrane serine/threonine kinase receptors (Feng and Derynck, 2005). Regardless of the ligand-receptor complex formation mechanism, signaling activation involves constitutive type II receptor, which phosphorylates the glycine-serine domain in type I receptor to initiate RTK activity (Weiss and Attisano, 2013). Extracellular proteins, such as Noggin and Gremlin, restrict the ligand availability and thus antagonize pathway activation (Brazil et al., 2015).
The intracellular mediators, the SMAD proteins, are shared in TGFβ and BMP pathways and include both receptor-regulated SMADs (R-SMADs; SMAD2 and -3) and common mediator SMADs (co-SMAD; SMAD4). The function of SMAD2/3 is negatively regulated by inhibitory SMADs (SMAD6 and -7), which block their interaction with the receptors. Also SMAD-independent signaling, mainly through small guanosine triphosphatases (GTPase, also known as G-protein), MAPK, and PI3K pathways, may mediate TGFβ response in context-dependent situations (Lamouille and Derynck, 2011; Mu et al., 2012).
Function of Trophic Factor Signaling in Developing Kidney
Ureteric Bud Morphogenesis
GDNF signaling via Ret and GFRα1 is critical for kidney development and underscores the relevance of reciprocal signaling between the UB and the MM in coordinating the balance of branching and nephrogenesis (Figure 4). GDNF is expressed by the MM, and lineage tracing with a reporter line demonstrates the identity of GDNF-expressing cells as self-renewing NPs (Cebrian et al., 2014). Ret, on the other hand, is expressed at the tips (but not the trunk) of the UB, and Ret-expressing cells give rise to the entire collecting duct system (Riccio et al., 2016). Gfrα1 mRNA is expressed both by the UB and the MM (Golden et al., 1999), however, renal organogenesis proceeds normally in a mouse model where Gfrα1 expression is ablated in non-Ret-expressing cells (Enomoto et al., 2004), and excision of Gfrα1 from the UB progenitors fully recapitulates the renal phenotype of the null mice (Keefe Davis et al., 2013), indicating that MM expression of GFRα1 is dispensable for kidney development.
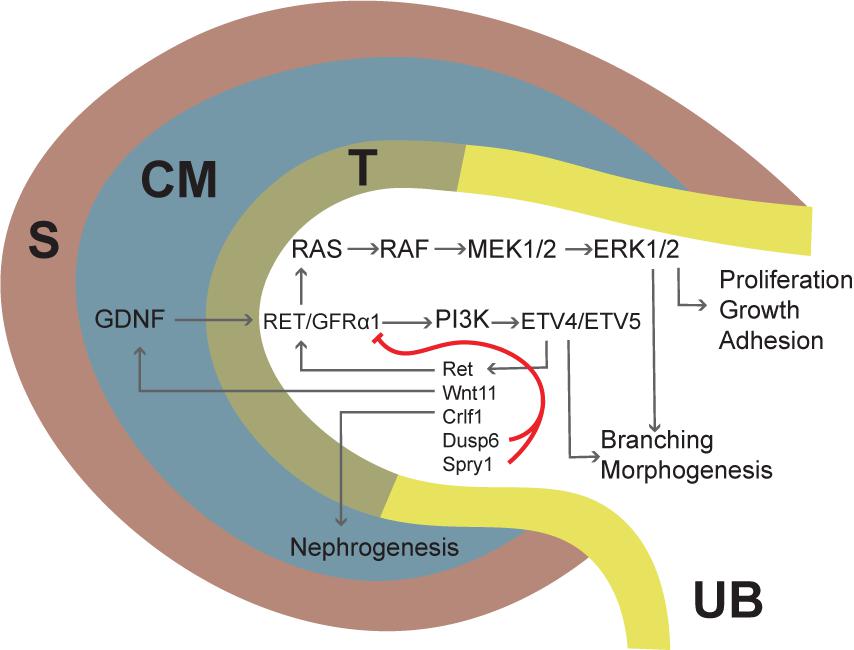
FIGURE 4. Schematic depiction of GDNF/RET/GFRα1 signaling in the ureteric bud (UB) tip during kidney development. Gdnf is first expressed in the CM (dark blue) and later also in the surrounding stroma (S, brown). GDNF binds to RET/GRFα1 complex in the UB tip (T, olive green), initiating downstream cascades such as the MAPK and PI3K pathways. Several factors are transcriptionally activated downstream of RET signaling and include Ret itself; Wnt11, which upregulates GDNF; Crlf1, which induces nephrogenesis; and Dusp6 and Spry1, which inhibit RET/GFRα1. ETV4 and -5 are transcription factors mediating the GDNF/RET response to UB epithelium and their expression depends on PI3K signaling. Signaling via the MAPK pathway is important for proliferation, growth, and adhesion, and plays a major role in branching morphogenesis (Moore et al., 1996).
The role of GDNF/Ret/GFRα1 signaling pathway in kidney development has been studied since the mid-nineties, but identification of its cellular functions and downstream targets remained elusive. Early in vitro studies suggested a mitogenic function for GDNF in UB outgrowth and branching while later experiments challenge this view by demonstrating cell organization and movement related functions (Sainio et al., 1997; Michael and Davies, 2004; Shakya et al., 2005; Chi et al., 2009; Riccio et al., 2016). Moreover, studies with a potential GDNF target Cofilin1 (Cfl1) and another actin depolymerizing factor Destrin (Dstn) demonstrated that organization of epithelial cell cytoskeleton is important for new branch formation (Kuure et al., 2010a). A few genes regulated by GDNF during kidney development were identified (Pepicelli et al., 1997; Majumdar et al., 2003; Basson et al., 2005), but it wasn’t until 2009 that transcription factors Etv4 and Etv5 were reported as downstream targets of the pathway (Lu et al., 2009). ETV4 and ETV5 are two members of the PEA3 family of ETS transcription factors, they are expressed at the tips of the UB as well as in the MM and developing nephrons, and they functionally overlap in promoting UB morphogenesis downstream of GDNF (Lu et al., 2009). While mutations in either gene had no discernible effect on kidney development, double mutant mice presented renal agenesis and/or severe renal hypoplasia; hence Etv4 and Etv5 account for the profound effects of GDNF signaling during kidney development. There is also evidence that other transcription factors such as hepatocyte nuclear factor-1 beta (HNF1b) may contribute to signal transduction of GDNF/RET/GFRα1 and that HNF1b can directly bind to regulatory regions of Etv5 and Gfra1 to modulate transcriptional activation (Desgrange et al., 2017).
Identification of Etv4 and Etv5 as downstream targets of the GDNF/RET/GFRα1 signaling pathway opened new avenues to study the mechanisms by which GDNF exerts its trophic role on branching morphogenesis. The analysis of embryonic chimeras and Mosaic Analysis with Double Markers (MADM) mouse models with Ret and Etv4/Etv5 mutant cells demonstrated that GDNF signaling at the UB tip is required for tip cells to remain at the tip. Therefore, loss of Ret or Etv4 and Etv5 cell-autonomously compromises the ability of the tip cell to remain in the tip (Chi et al., 2009; Kuure et al., 2010b; Riccio et al., 2016). These data point toward a role of GDNF signaling in promoting cell movement and/or retention and less as a promoter of proliferation, as no differences in proliferation were detected between mutant and wild type cells (Riccio et al., 2016). These studies suggest that GDNF/RET/GFRα1 signaling may positively regulate self-renewal of UB tip-residing collecting duct progenitors. Advances in RNA sequencing techniques revealed previously unrecognized expression sites for Gdnf, including stromal cells (Magella et al., 2018). It remains to be seen whether future studies will identify novel functions for GDNF in processes not primarily linked to UB morphogenesis.
FGF signaling The earliest events in ureteric bud formation and outgrowth are well-known to depend on GDNF signaling (Costantini, 2012). However, exogenous application of FGF protein together with inhibition of TGFβ family member activin A induces supernumerary ureter budding in cultured kidneys even in the absence of GDNF/RET signaling (Maeshima et al., 2007). Thus, the FGF-induced, GDNF-independent budding may serve as a back-up mechanism that functions to ensure UB formation in the absence of GDNF/RET signaling (Basson et al., 2005; Rozen et al., 2009; Michos et al., 2010).
Fgf10 is expressed early on in the developing kidney, and an elegant series of compound gene inactivation studies demonstrated that it is essential for UB formation in the simultaneous absence of Gdnf and Spry1 (Michos et al., 2010). On their own, FGF7 and -10, secreted from the CM, regulate the extent of UB branching as shown by smaller kidneys with reduced nephron numbers in the corresponding knockout mice (Qiao et al., 1999; Ohuchi et al., 2000). A significantly more severe phenotype in UB-specific loss of Fgfr2 than in any of the single ligand knockouts suggests that several FGF ligands converge their function on UB morphogenesis through this receptor (Zhao et al., 2004). This is further supported by the finding that UB-specific deletion of Fgfr1, alone or in combination with Frs2α, does not impact renal differentiation, while FRS2α in the UB is required for the normal branching (Sims-Lucas et al., 2009, 2011b). It appears that FGFR2 and FRS2α function both distinctly and additively in the UB lineage, challenging the view of FRS2α being the major docking protein for FGF signal transduction (Sims-Lucas et al., 2011b).
In addition to the above described cell autonomous functions, FGF signaling also critically impacts UB development via cell non-autonomous effects in the mesenchyme. Disruption of FGFR1 signaling by Pax3Cre-mediated deletion has no overt effect on renal differentiation, while a similar strategy with FGFR2 results in multiple budding, misshaped kidneys due to UB duplication, and obstructed ureters (Poladia et al., 2006; Hains et al., 2008, 2010). Simultaneous loss of both receptors allows UB outgrowth but fails to support its elongation and further differentiation, leading to renal aplasia (Poladia et al., 2006; Sims-Lucas et al., 2011a). Finally, mice lacking mesenchymal FGFR1 and concurrently deficient for FRS2α-binding in FGFR2 show remarkably milder UB defects that are also distinct from those reported for mesenchymal loss of both receptors (Poladia et al., 2006; Sims-Lucas et al., 2012). Interestingly, UB tips in the kidneys lacking mesenchymal FGFR1; FGFR2/FRS2α signaling are expanded and hyperproliferative, resembling tips seen in GDNF hypermorphic kidneys (Kumar et al., 2015). This may suggest that FGFR signaling in the MM is required to restrict biophysical and/or molecular properties of the nephron niche that reciprocally limit tip size in normal kidneys.
Nephron Differentiation
The role of FGF signaling in the promotion of nephrogenesis became evident more than 20 years ago. Isolated MM cultures demonstrated that FGF2 can mediate the condensation and survival of nephrogenic mesenchyme while additional factors, including LIF and TGFβ2, were needed for mesenchyme-to-epithelium transformation (MET), hallmarking the major event in nephron differentiation in rats (Perantoni et al., 1995; Barasch et al., 1997, 1999; Plisov et al., 2001). The species-specific differences in MET were revealed by showing that transient activation of WNT pathway induces nephrogenesis both in mice and rats (Davies and Garrod, 1995; Kuure et al., 2007). More recent in vitro studies show that FGF signaling is involved in maintenance and expansion of isolated NPs (Brown et al., 2011, 2015).
FGF signaling has become evidently one of the major regulatory pathway in NP maintenance and differentiation. Pax3Cre-mediated simultaneous loss of Fgfr1 and -2 results in the failure to establish proper MM and thus, supports FGFs essential function in the formation of the initial NP pool (Poladia et al., 2006). A similar deletion strategy in the SIX2-positive population results in a remarkably less dramatic phenotype and depletion of NPs only at significantly later stages (Di Giovanni et al., 2015). The difference in phenotype severity may derive from the earlier recombination with Pax3Cre, which again suggest that FGF signaling establishes the earliest NP population or is involved in its maintenance. The essential functions of FGF signaling in creating MM and NP pool are further supported by the requirement of FGF ligand in all protocols used for stem cell-derived kidney organoid differentiation (Takasato et al., 2014, 2015; Morizane et al., 2015; Morizane and Bonventre, 2017).
Of the ligands, Fgf7, -8, -9, -10, and -20 are expressed in the developing kidney and have been demonstrate to play roles in nephron differentiation (Walker et al., 2016). Genetic studies with FGF9 and -20, of which FGF9 is mainly expressed by UB epithelium and FGF20 by NPs, show that deletion of Fgf9 alone is compatible with normal renal differentiation, while deletion of Fgf20 causes mildly reduced kidney size and nephron number (Barak et al., 2012). Loss of all four Fgf9;20 alleles results in renal agenesis resembling the severe phenotype seen also in MM-specific loss of both receptors (Fgfr1/2) and thus highlighting the importance of FGF signaling in establishing nephrogenic potential. On the other hand, Fgf9 +/-; Fgf20-/- compound mutants show premature NP differentiation and greatly diminished total nephron number suggesting that FGF signaling activated by these ligands is needed to maintain undifferentiated status in progenitors. Also, studies with some of the FGF signaling regulators support its role in maintaining the NP population while additionally reveal control of cellular processes such survival and proliferation (Ahn et al., 2013; Motamedi et al., 2014). Interestingly, a decrease in FGF signaling coincides with cessation of nephrogenesis, suggesting a role for FGFs in increased NP cell cycle exit rates (Chen et al., 2015).
Although the majority of single ligand deletions either show normal renal organogenesis or cause embryonic lethality, deletion of Fgf7 or Fgf10 results in smaller kidneys with less nephrons, which likely derives from branching morphogenesis defects (Qiao et al., 1999; Ohuchi et al., 2000). However, studies with FGF8, expressed by the progenitors and differentiating renal vesicles, demonstrated that FGF8 signaling maintains NP cells but also advances nephrogenesis beyond comma-shaped body (Grieshammer et al., 2005; Perantoni et al., 2005). Molecularly, FGF8-induced signaling is required for Wnt4 and Lim1 expression in differentiating nephrons, which are severely truncated in mutant mice. The unconventional receptor FGFR-like 1 (FGFRL1), lacking the intracellular domains that provoke typical TK pathways, is also needed for Wnt4 and Lim1 expression and appears to regulate both UB branching and mesenchymal condensation prior to renal vesicle formation (Gerber et al., 2009). FGFRL1 binds ligands and heparin with high affinity but does not exert the mitogenic function typical for FGFR signaling (Yang et al., 2016). Rather, it promotes cellular adhesion, and some evidence suggests that it might be shed from cell membranes by a cleavage by a yet unidentified protease (Steinberg et al., 2010). The finding that intracellular domains are dispensable for its normal function supports the hypothesis that FGFRL1 could function as a secreted decoy receptor for FGF ligands, but further studies are needed to reveal its mechanistic functions in the developing kidney (Bluteau et al., 2014). Table 1 summarizes the general outcomes of genetic studies with trophic factors discussed in this review.
Vasculature Development
Similarly to the development of vasculature elsewhere in the body, the molecular regulation in the developing kidney is dictated by VEGF signaling. During the formation of the S-shaped body, vascular cells accumulate in the glomerular cleft and begin organizing into the glomerular vasculature. The beginning of the organization of the glomerular vasculature occurs concurrently with the production of VEGF from podocyte precursors, which is likely a driving factor of the glomerular vascular development as VEGF signaling from mature podocytes continues to maintain the glomerular vasculature (Eremina et al., 2003). In addition to the glomerular vasculature, VEGF derived from the renal tubule has been shown to play a role in the maintenance of peritubular vasculature (Dimke et al., 2015). Renal tubules also express TGF-β (Pelton et al., 1991; Bridgewater et al., 2011), which is required for vessel formation from endothelial progenitors (Dickson et al., 1995). It is thus likely that local cues within the cortex control arterial branching.
Vascular mural cells (VMCs) may have an important role in the arrangement of the renal vasculature. It has been shown that peritubular capillary development is mediated by angiopoietin-2, which antagonizes Ang1-dependent Tie2 signaling by the endothelia to promote VMC differentiation (Pitera et al., 2004). Vascular development is also regulated by CXCR and Eph RTK signaling (Cheng et al., 2002; Miao and Wang, 2009). Cxcl12 secreted by stromal cells and podocytes acts through its receptor Cxcr4, and mice lacking either Cxcl12 or Cxcr4 show normal kidney morphogenesis, except for a vascular patterning defect (Takabatake et al., 2009). Eph/ephrin signaling has been implicated in regulation of urorectal septation, insertion of the nephric duct into the cloaca, and glomerulogenesis (Weiss et al., 2014). EphA2 expressed in the UB epithelium shows in vitro negative effect on branching morphogenesis as seen by collapsing branch structures, and defects in chemotactic migration (Miao et al., 2003). It is possible that EphB4 and EphrinB2 have functions in glomerulogenesis since they are involved with angiogenesis in general (Wang et al., 1998; Weiss and Kispert, 2016), and their expression patterns suggest that they could have a role in development of non-vascular cells of glomerulus and Bowman’s capsule (Takahashi et al., 2001; Weiss and Kispert, 2016).
Cascades Active Downstream of Trophic Factor Receptors
Several intracellular pathways are activated downstream of trophic factor-induced RTK signaling. Combinations of elegant in vivo and traditional in vitro experiments have demonstrated that RAS/MAPK, PI3K/AKT, and PLCγ cascades are essential for UB morphogenesis (De Graaff et al., 2001; Fisher et al., 2001; Tang et al., 2002; Jijiwa et al., 2004; Watanabe and Costantini, 2004; Jain et al., 2006; Willecke et al., 2011; Ihermann-Hella et al., 2014).
Important advances were made in studies aiming to maintain NPs in culture, induce isolated MM to differentiate, and generate stem cell-derived renal organoids. These efforts established the necessity of Smad, PI3K, MAPK/ERK, GSK3β, and ROCK pathways for nephrogenesis (Oxburgh and Robertson, 2002; Kuure et al., 2007; Unbekandt and Davies, 2010; Brown et al., 2013, 2015; Lindstrom et al., 2015a; Li et al., 2016; Ihermann-Hella et al., 2018), but surprisingly little efforts have been made to genetically test the requirement of individual intracellular cascades in the developing kidney (Table 1), leaving the distinct and/or synergistic functions of different pathways unknown.
Ureteric Bud Morphogenesis
RAS/MAPK pathway consists of four separate cascades, extracellular signal-regulated kinases (Figure 5) (ERK1/2), Jun amino-terminal kinases (JNK1/2/3), ERK5, and p38-MAPK. Each cascade consists of three or more components including a MAPK kinase kinase (MAP3K), MAPK kinase (MAP2K), and a MAPK. Once activated, these MAP kinases activate various substrate proteins including transcription factors and protein kinases, among others (Roskoski, 2012a). MAPK signaling is controlled by positive and negative feedback loops as well crosstalk between the different pathways, and these feedback mechanisms have been reviewed elsewhere (Lake et al., 2016). Very briefly, ERK1/2 directly phosphorylates upstream components and also induces transcription of pathway inhibitors, notably here Sprouty and Dusps, which are expressed in the developing kidney (Basson et al., 2005; Lu et al., 2009).
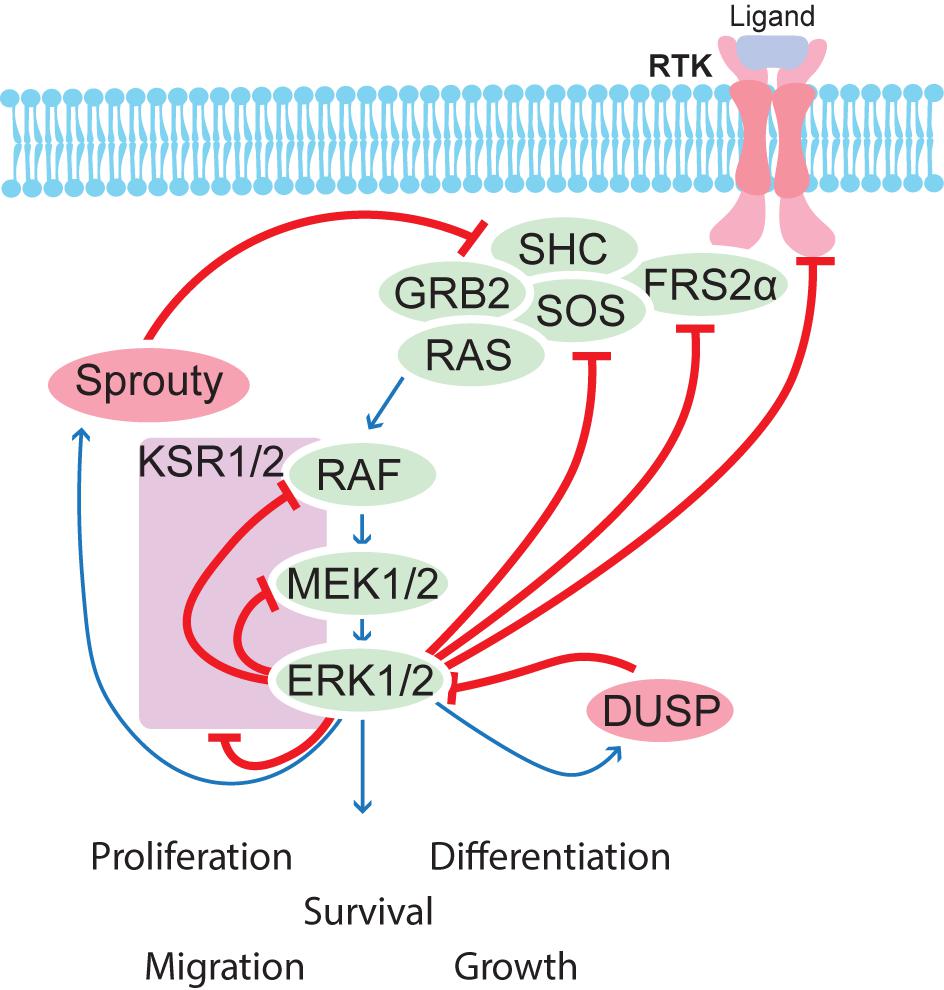
FIGURE 5. Schematic overview of feedback regulation of the MAPK pathway. Several feedback mechanisms are in place to control the MAPK pathway. ERK1/2 phosphorylates many upstream components and induces transcription of regulators such as Sproutys and DUSPs, which help to balance correct signaling strength in a given cell.
RAS-GDP is activated by various mitogens or growth factors to become RAS-GTP which has many downstream pathways, including RAF-MEK-ERK (Pylayeva-Gupta et al., 2011; Wortzel and Seger, 2011). RAS-GTP activates RAF kinase family members (Roskoski, 2010), which in turn catalyze the activation of MEK1 and MEK2 through their phosphorylation. Activated MEK1/2 then mediate phosphorylation and activation of ERK1 and ERK2 (Roskoski, 2012b). JNK, p38, and ERK5 pathways are activated by pro-inflammatory cytokines or cellular stress (Plotnikov et al., 2011). The MEK-ERK signal transduction cascade (also known as the MAPK/ERK cascade) regulates processes such as differentiation, proliferation, transcription, metabolism, cell cycle progression, migration, survival, and adhesion while the JNK family controls apoptosis and immune cell development (Weston and Davis, 2007). While the other MAPK cascades have been extensively studied, not much is known about the MEK5-ERK5 cascade (Drew et al., 2012).
The MAPK pathway is activated in several cell types of the developing kidney (Fisher et al., 2001; Hida et al., 2002; Ihermann-Hella et al., 2014, 2018). Initially, the involvement of the MAPK pathway in UB morphogenesis was revealed by in vitro kidney cultures, which showed that inhibition of MEK proteins results in decreased tip cell proliferation and abnormal branching morphogenesis (Fisher et al., 2001; Watanabe and Costantini, 2004). At the UB outgrowth stage, MAPK/ERK activity is polarized on the side of the Wolffian duct, which gives rise to the bud, and is completely lost in Ret knockout ducts (Chi et al., 2009). Disruption of the docking site involved in MAPK and PI3K activation downstream of RET (Y1062) supports the requirement for these pathways in UB branching (De Graaff et al., 2001; Wong et al., 2005; Jain et al., 2006). However, later studies revealed that the situation is more complicated as specific mutations in distinct isoforms also have different outcomes for renal differentiation (Jijiwa et al., 2004; Jain et al., 2010). Genetic disruption of the MAPK pathway by tissue-specific deletion of Mek1 in a Mek2 knockout background demonstrated normal UB outgrowth but requirement for UB branch formation. Removal of MAPK activity results in elongation-only phenotype where UB fails to branch due to cell cycle progression defect and accumulation of E-cadherin on basolateral cell sides (Ihermann-Hella et al., 2014). Additional studies are needed to clarify how MAPK pathway exactly regulates E-cadherin and what are the consequences on adhesive forces in UB cells with different levels of MAPK/ERK activation.
The importance of precise regulation on MAPK activation strength and duration in developing kidneys was initially revealed by ectopic Sprouty2 expression in the UB, which suggested changes in GDNF and FGF signaling as well as UB morphogenesis (Chi et al., 2004). Deletion of Sprouty1 confirmed the fundamental role of negative regulation in GDNF/RET-mediated UB outgrowth (Basson et al., 2005, 2006). Moreover, the expression of not only Sprouty1 but also two other negative regulators of the MAPK/ERK pathway, Spred2 and Dusp6 is induced by GDNF (Lu et al., 2009; Ola et al., 2011). Elegant genetic experiments demonstrated that synergistic GDNF/RET/Sprouty1 signaling critically balances UB outgrowth and branching (Basson et al., 2005; Rozen et al., 2009; Michos et al., 2010) while the exact roles of Spred2 and Dusp6 remain to be studied.
PI3K/AKT pathway The PI3Ks form a family of lipid kinases, which utilize membrane-bound phospholipids (PIP3) as secondary messengers. The class I PI3Ks mediate trophic factor-induced RTK and G protein-coupled receptor signaling, and through PIP3 recruit and activate, e.g., PI3K-dependent kinase-1 (PDK1), AKT, and small GTPases (Fruman et al., 2017). AKT is fully activated by PDK1 phosphorylation, and phosphatase and tensin homolog (PTEN) together with mammalian target of rapamycin (mTOR) complex 2 provide additional negative/positive regulation for signal transduction length, substrate selectivity, stability, and possibly subcellular localization (Manning and Toker, 2017). Activation of mTOR complex1 results in cell survival and increased protein synthesis through phosphorylation of e.g., ribosomal S6 kinase (Lamouille and Derynck, 2011). Elevated PI3K/AKT/mTOR activation is associated with many cancers, and its physiological function in normal development and homeostasis is only poorly studied. The use of pluripotent stem cells has shed new light on its function in embryonic development, self-renewal of stem cells, and maintenance of pluripotency (Yu and Cui, 2016).
Based on chemical inhibition and in vivo mutagenesis of Ret Y1062 docking site, PI3K is also required for normal UB branching (De Graaff et al., 2001; Tang et al., 2002; Jijiwa et al., 2004; Wong et al., 2005; Jain et al., 2006). The essential function of PI3K is supported by the finding that the expression of GDNF target transcription factors, Etv4 and -5, requires normal PI3K activation but does not, at least at the mRNA level, depend on MAPK/ERK activity (Lu et al., 2009). FGF-stimulated, GDNF-independent UB outgrowth appears to utilize AKT activation without involvement of PI3K, and suggests differences in the use of intracellular mediators (Tee et al., 2013). Though genetic experiments specifically targeting AKT/PI3K pathway members are scarce, UB-specific deletion of Pten, an antagonist of PI3K activity, suggests that the UB branching pattern is shaped by PI3K activation (Kim and Dressler, 2007).
Of the other intracellular cascades activated downstream of RTK signaling, PLCγ and SRC pathways appear important for UB morphogenesis. Isoform specific mutations in tyrosine 1015 activating PLCγ causes renal abnormalities related to UB branching, but only in the context of the RET51 isoform (Jain et al., 2006). Chemical inhibition of SRC activity, on the other hand, blocks UB morphogenesis by inhibiting both new bud formation and trunk elongation while allowing abundant nephrogenesis to take place (Kuure et al., 2007). Some indications of p38 MAPK functions in collecting duct differentiation were suggested by the studies with integrin-linked kinase in the UB (Smeeton et al., 2010), but its fundamental role remains to be studied. In conclusion, combined approaches of biochemistry, cell biology, and genetics will be needed to better understand the function of individual intracellular cascades and even more important, to interpret how they cooperate in a context-dependent manner to mediate extracellular stimuli of trophic factors.
Nephrogenesis
SMAD pathway Without activated TGFβ superfamily signaling, the SMAD proteins, the intracellular mediators of this pathway, are shuttling constantly between the cytoplasm and nucleus (Xu et al., 2002). Upon the tertiary ligand-receptor complex formation, the receptor-regulated SMAD proteins specific to TGFβ, R-SMAD2/3, and those activated downstream of BMP, R-SMAD1/5/8, are recruited to the complex. This triggers their phosphorylation by type I receptor and again frees them into the cytosol, where they are able to form heterodimeric complexes with common SMAD4. The trimeric complex of two R-SMADs together with SMAD4 then accumulates in the cytosol from where it is imported into the nucleus (Hill, 2009). Additional input from the other upstream pathways is important in determining the duration, strength, and response of the signal (Massague, 2012).
Several SMAD proteins localize to NPs while being downregulated in the induced nephron precursors (Oxburgh and Robertson, 2002). This suggests that BMP/TGFβ-induced SMAD signaling exhibits essential functions in NP biology and the importance of BMP7 signaling was demonstrated in classical knockout studies (Dudley et al., 1995; Luo et al., 1995). More recent experiments reveal that BMP7-induced signaling plays an important role in progenitor proliferation, survival, and preventing premature differentiation (Blank et al., 2009; Tomita et al., 2013). Mitogenic response appears to be mediated via Jun (also known as c-jun) N-terminal kinases (JNKs) while signaling through the SMAD pathway promotes progenitor differentiation via commitment to transient amplifying cells (Brown et al., 2013). Accordingly, SMAD inhibition maintains progenitors in a more stem-like state, as shown by the expansion of the CITED1/SIX2-positive compartment (Brown et al., 2015), which is considered as the least differentiated NP population in the developing kidney (Costantini and Kopan, 2010). Long-term in vitro culture of NPs supports the requirement for BMP and Rho kinase pathways as the addition of BMP7 and ROCK inhibitor together with WNT activation and FGF supply enables their maintenance (Unbekandt and Davies, 2010; Li et al., 2016).
RTK activated intracellular pathways WNT/β-catenin signaling is the major driver that pushes NPs to differentiate (Stark et al., 1994; Kuure et al., 2007; Park et al., 2007). Additionally, the JNK pathway appears to be activated downstream of WNT-induced nephron differentiation, at least in cultured colony-forming progenitors (Osafune et al., 2006). The complexity in control of the self-renewal versus differentiation decision was demonstrated by a series of time-lapse imaging of nephrogenesis in in vitro cultured kidneys (Lindstrom et al., 2015a,b). It showed that balancing signaling strengths, as shown for PI3K activation, determines whether to maintain a stem-like character or converge the differentiation program. Similarly, our recent study showed that MAPK/ERK activation, as revealed by live-imaging of embryonic kidneys isolated from Förster resonance energy transfer (FRET) biosensor of ERK, is heterogeneous among the NP population and very strong in RVs (Ihermann-Hella et al., 2018). We showed by NP-specific inactivation that MAPK/ERK activity controls niche organization and communication with extracellular matrix and is essential for normal NP differentiation. In the absence of MAPK activity, nephrogenesis proceeded quite normally up to RV stage but then halted almost exclusively. Interestingly, although strong ERK activation and pERK1/2 signal is detected in the connecting piece of nephron to collecting duct, no obvious defects were seen in this process. It remains to be studied how activation strength in trophic factor-induced intracellular pathways contributes to the biophysical properties of progenitor niche and nephron differentiation, e.g., in specifying nephron segments.
Future Aspects
Since the identification of the first trophic factors in the 1970s, remarkable progress has been made toward identifying additional members of this large group of signaling molecules and understanding the varied mechanisms by which they exert their trophic roles. These mechanisms often present redundancy and/or synergism in a tissue- and time-dependent manner. Two somewhat overlapping lines of research are now providing a stepping stone to better understand the role of these trophic factors in kidney development. On the one hand, there has been a significant effort from the McMahon lab and others to characterize human kidney development not only at an anatomical level but also at the molecular level using single-cell sequencing (Lindstrom et al., 2018a,b,c,d; Menon et al., 2018; Wang et al., 2018). Complementary to these studies, and somehow preceding them, researchers can now induce differentiation of human embryonic stem cells into kidney organoids that, among many other applications, can be used to finely characterize signaling pathways that drive human kidney organogenesis (Lam et al., 2014; Taguchi et al., 2014; Takasato et al., 2014; Morizane et al., 2015). As is often the case with basic research, answering some questions will also open many new lines of inquiry, and there is still much to learn about how these growth factors drive and modulate the delicate process of kidney organogenesis.
Author Contributions
All authors contributed equally to the writing of this review manuscript.
Funding
This work was supported by grants to SK from the Academy of Finland (294243), Jane and Aatos Erkko, Sigrid Juselius, and Maud Kuistila Foundations.
Conflict of Interest Statement
The authors declare that the research was conducted in the absence of any commercial or financial relationships that could be construed as a potential conflict of interest.
Acknowledgments
We thank Santiago Cortés for help in preparation of the figures and illustrations and Anneliis Ihermann-Hella for providing the in vivo image of immunostained kidney.
References
Abrahamson, D. R., Robert, B., Hyink, D. P., St John, P. L., and Daniel, T. O. (1998). Origins and formation of microvasculature in the developing kidney. Kidney Int. Suppl. 67, S7–S11. doi: 10.1046/j.1523-1755.1998.06702.x
Ahn, S. Y., Kim, Y., Kim, S. T., Swat, W., and Miner, J. H. (2013). Scaffolding proteins DLG1 and CASK cooperate to maintain the nephron progenitor population during kidney development. J. Am. Soc. Nephrol. 24, 1127–1138. doi: 10.1681/ASN.2012111074
Arighi, E., Borrello, M. G., and Sariola, H. (2005). RET tyrosine kinase signaling in development and cancer. Cytokine Growth Factor Rev. 16, 441–467. doi: 10.1016/j.cytogfr.2005.05.010
Asai, N., Fukuda, T., Wu, Z., Enomoto, A., Pachnis, V., Takahashi, M., et al. (2006). Targeted mutation of serine 697 in the Ret tyrosine kinase causes migration defect of enteric neural crest cells. Development 133, 4507–4516. doi: 10.1242/dev.02616
Barak, H., Huh, S. H., Chen, S., Jeanpierre, C., Martinovic, J., Parisot, M., et al. (2012). FGF9 and FGF20 maintain the stemness of nephron progenitors in mice and man. Dev. Cell 22, 1191–1207. doi: 10.1016/j.devcel.2012.04.018
Barasch, J., Qiao, J., Mcwilliams, G., Chen, D., Oliver, J. A., and Herzlinger, D. (1997). Ureteric bud cells secrete multiple factors, including bFGF, which rescue renal progenitors from apoptosis. Am. J. Physiol. 273, F757–F767. doi: 10.1152/ajprenal.1997.273.5.F757
Barasch, J., Yang, J., Ware, C. B., Taga, T., Yoshida, K., Erdjument-Bromage, H., et al. (1999). Mesenchymal to epithelial conversion in rat metanephros is induced by LIF. Cell 99, 377–386. doi: 10.1016/S0092-8674(00)81524-X
Basson, M. A., Akbulut, S., Watson-Johnson, J., Simon, R., Carroll, T. J., Shakya, R., et al. (2005). Sprouty1 is a critical regulator of GDNF/RET-mediated kidney induction. Dev. Cell 8, 229–239. doi: 10.1016/j.devcel.2004.12.004
Basson, M. A., Watson-Johnson, J., Shakya, R., Akbulut, S., Hyink, D., Costantini, F. D., et al. (2006). Branching morphogenesis of the ureteric epithelium during kidney development is coordinated by the opposing functions of GDNF and Sprouty1. Dev. Biol. 299, 466–477. doi: 10.1016/j.ydbio.2006.08.051
Bellusci, S., Grindley, J., Emoto, H., Itoh, N., and Hogan, B. L. (1997). Fibroblast growth factor 10 (FGF10) and branching morphogenesis in the embryonic mouse lung. Development 124, 4867–4878.
Beyer, T. A., Werner, S., Dickson, C., and Grose, R. (2003). Fibroblast growth factor 22 and its potential role during skin development and repair. Exp. Cell Res. 287, 228–236. doi: 10.1016/S0014-4827(03)00139-3
Binns, K. L., Taylor, P. P., Sicheri, F., Pawson, T., and Holland, S. J. (2000). Phosphorylation of tyrosine residues in the kinase domain and juxtamembrane region regulates the biological and catalytic activities of Eph receptors. Mol. Cell. Biol. 20, 4791–4805. doi: 10.1128/MCB.20.13.4791-4805.2000
Blank, U., Brown, A., Adams, D. C., Karolak, M. J., and Oxburgh, L. (2009). BMP7 promotes proliferation of nephron progenitor cells via a JNK-dependent mechanism. Development 136, 3557–3566. doi: 10.1242/dev.036335
Bluteau, G., Zhuang, L., Amann, R., and Trueb, B. (2014). Targeted disruption of the intracellular domain of receptor FgfrL1 in mice. PLoS One 9:e105210. doi: 10.1371/journal.pone.0105210
Boyle, S., Misfeldt, A., Chandler, K. J., Deal, K. K., Southard-Smith, E. M., Mortlock, D. P., et al. (2008). Fate mapping using Cited1-CreERT2 mice demonstrates that the cap mesenchyme contains self-renewing progenitor cells and gives rise exclusively to nephronic epithelia. Dev. Biol. 313, 234–245. doi: 10.1016/j.ydbio.2007.10.014
Brazil, D. P., Church, R. H., Surae, S., Godson, C., and Martin, F. (2015). BMP signalling: agony and antagony in the family. Trends Cell Biol. 25, 249–264. doi: 10.1016/j.tcb.2014.12.004
Bridgewater, D., Di Giovanni, V., Cain, J. E., Cox, B., Jakobson, M., Sainio, K., et al. (2011). Beta-catenin causes renal dysplasia via upregulation of Tgfbeta2 and Dkk1. J. Am. Soc. Nephrol. 22, 718–731. doi: 10.1681/ASN.2010050562
Brown, A. C., Adams, D., De Caestecker, M., Yang, X., Friesel, R., and Oxburgh, L. (2011). FGF/EGF signaling regulates the renewal of early nephron progenitors during embryonic development. Development 138, 5099–5112. doi: 10.1242/dev.065995
Brown, A. C., Muthukrishnan, S. D., Guay, J. A., Adams, D. C., Schafer, D. A., Fetting, J. L., et al. (2013). Role for compartmentalization in nephron progenitor differentiation. Proc. Natl. Acad. Sci. U.S.A. 110, 4640–4645. doi: 10.1073/pnas.1213971110
Brown, A. C., Muthukrishnan, S. D., and Oxburgh, L. (2015). A synthetic niche for nephron progenitor cells. Dev. Cell 34, 229–241. doi: 10.1016/j.devcel.2015.06.021
Bullock, S. L., Fletcher, J. M., Beddington, R. S. P., and Wilson, V. A. (1998). Renal agenesis in mice homozygous for a gene trap mutation in the gene encoding heparan sulfate 2-sulfotransferase. Genes Dev. 12, 1894–1906. doi: 10.1101/gad.12.12.1894
Bush, J. O., and Soriano, P. (2012). Eph/ephrin signaling: genetic, phosphoproteomic, and transcriptomic approaches. Semin. Cell Dev. Biol. 23, 26–34. doi: 10.1016/j.semcdb.2011.10.018
Cacalano, G., Farinas, I., Wang, L. C., Hagler, K., Forgie, A., Moore, M., et al. (1998). GFRalpha1 is an essential receptor component for GDNF in the developing nervous system and kidney. Neuron 21, 53–62. doi: 10.1016/S0896-6273(00)80514-0
Cancilla, B., Ford-Perriss, M. D., and Bertram, J. F. (1999). Expression and localization of fibroblast growth factors and fibroblast growth factor receptors in the developing rat kidney. Kidney Int. 56, 2025–2039. doi: 10.1046/j.1523-1755.1999.00781.x
Cebrian, C., Asai, N., D’agati, V., and Costantini, F. (2014). The number of fetal nephron progenitor cells limits ureteric branching and adult nephron endowment. Cell Rep. 7, 127–137. doi: 10.1016/j.celrep.2014.02.033
Cebrian, C., Borodo, K., Charles, N., and Herzlinger, D. A. (2004). Morphometric index of the developing murine kidney. Dev. Dyn. 231, 601–608. doi: 10.1002/dvdy.20143
Chellaiah, A. T., Mcewen, D. G., Werner, S., Xu, J., and Ornitz, D. M. (1994). Fibroblast growth factor receptor (FGFR) 3. Alternative splicing in immunoglobulin-like domain III creates a receptor highly specific for acidic FGF/FGF-1. J. Biol. Chem. 269, 11620–11627.
Chen, S., Brunskill, E. W., Potter, S. S., Dexheimer, P. J., Salomonis, N., Aronow, B. J., et al. (2015). Intrinsic age-dependent changes and cell-cell contacts regulate nephron progenitor lifespan. Dev. Cell 35, 49–62. doi: 10.1016/j.devcel.2015.09.009
Cheng, C. N., Verdun, V. A., and Wingert, R. A. (2015). Recent advances in elucidating the genetic mechanisms of nephrogenesis using zebrafish. Cells 4, 218–233. doi: 10.3390/cells4020218
Cheng, N., Brantley, D. M., and Chen, J. (2002). The ephrins and Eph receptors in angiogenesis. Cytokine Growth Factor Rev. 13, 75–85. doi: 10.1016/S1359-6101(01)00031-4
Chi, L., Zhang, S., Lin, Y., Prunskaite-Hyyrylainen, R., Vuolteenaho, R., Itaranta, P., et al. (2004). Sprouty proteins regulate ureteric branching by coordinating reciprocal epithelial Wnt11, mesenchymal Gdnf and stromal Fgf7 signalling during kidney development. Development 131, 3345–3356. doi: 10.1242/dev.01200
Chi, X., Michos, O., Shakya, R., Riccio, P., Enomoto, H., Licht, J. D., et al. (2009). Ret-dependent cell rearrangements in the Wolffian duct epithelium initiate ureteric bud morphogenesis. Dev. Cell 17, 199–209. doi: 10.1016/j.devcel.2009.07.013
Colvin, J. S., Bohne, B. A., Harding, G. W., Mcewen, D. G., and Ornitz, D. M. (1996). Skeletal overgrowth and deafness in mice lacking fibroblast growth factor receptor 3. Nat. Genet. 12, 390–397. doi: 10.1038/ng0496-390
Combes, A. N., Davies, J. A., and Little, M. H. (2015). Cell-cell interactions driving kidney morphogenesis. Curr. Top. Dev. Biol. 112, 467–508. doi: 10.1016/bs.ctdb.2014.12.002
Costantini, F. (2012). Genetic controls and cellular behaviors in branching morphogenesis of the renal collecting system. Wiley Interdiscip. Rev. Dev. Biol. 1, 693–713. doi: 10.1002/wdev.52
Costantini, F., and Kopan, R. (2010). Patterning a complex organ: branching morphogenesis and nephron segmentation in kidney development. Dev. Cell 18, 698–712. doi: 10.1016/j.devcel.2010.04.008
Cullen-McEwen, L. A., Drago, J., and Bertram, J. F. (2001). Nephron endowment in glial cell line-derived neurotrophic factor (GDNF) heterozygous mice. Kidney Int. 60, 31–36. doi: 10.1046/j.1523-1755.2001.00767.x
Cullen-McEwen, L. A., Sutherland, M. R., and Black, M. J. (2016). “The human kidney: parallels in structure, spatial development, and timing of nephrogenesis,” in Kidney Development, Disease, Repair, and Regeneration, eds M. Little (Cambridge, MA: Academic Press), 27–40. doi: 10.1016/B978-0-12-800102-8.00003-5
Davies, J. A., and Garrod, D. R. (1995). Induction of early stages of kidney tubule differentiation by lithium ions. Dev. Biol. 167, 50–60. doi: 10.1006/dbio.1995.1006
Davis, T. K., Hoshi, M., and Jain, S. (2014). To bud or not to bud: the RET perspective in CAKUT. Pediatr. Nephrol. 29, 597–608. doi: 10.1007/s00467-013-2606-5
Davy, A., and Soriano, P. (2005). Ephrin signaling in vivo: look both ways. Dev. Dyn. 232, 1–10. doi: 10.1002/dvdy.20200
De Graaff, E., Srinivas, S., Kilkenny, C., D’agati, V., Mankoo, B. S., Costantini, F., et al. (2001). Differential activities of the RET tyrosine kinase receptor isoforms during mammalian embryogenesis. Genes Dev. 15, 2433–2444. doi: 10.1101/gad.205001
Desgrange, A., Heliot, C., Skovorodkin, I., Akram, S. U., Heikkila, J., Ronkainen, V. P., et al. (2017). HNF1B controls epithelial organization and cell polarity during ureteric bud branching and collecting duct morphogenesis. Development 144, 4704–4719. doi: 10.1242/dev.154336
Di Giovanni, V., Walker, K. A., Bushnell, D., Schaefer, C., Sims-Lucas, S., Puri, P., et al. (2015). Fibroblast growth factor receptor-Frs2alpha signaling is critical for nephron progenitors. Dev. Biol. 400, 82–93. doi: 10.1016/j.ydbio.2015.01.018
Dickson, M. C., Martin, J. S., Cousins, F. M., Kulkarni, A. B., Karlsson, S., and Akhurst, R. J. (1995). Defective haematopoiesis and vasculogenesis in transforming growth factor β1 knock out mice. Development 121, 1845–1854.
Dimke, H., Sparks, M. A., Thomson, B. R., Frische, S., Coffman, T. M., and Quaggin, S. E. (2015). Tubulovascular cross-talk by vascular endothelial growth factor a maintains peritubular microvasculature in kidney. J. Am. Soc. Nephrol. 26, 1027–1038. doi: 10.1681/ASN.2014010060
Drew, B. A., Burow, M. E., and Beckman, B. S. (2012). MEK5/ERK5 pathway: the first fifteen years. Biochim. Biophys. Acta 1825, 37–48. doi: 10.1016/j.bbcan.2011.10.002
Drummond, I. A., and Davidson, A. J. (2016). Zebrafish kidney development. Methods Cell Biol. 134, 391–429. doi: 10.1016/bs.mcb.2016.03.041
Dudley, A. T., Godin, R. E., and Robertson, E. J. (1999). Interaction between FGF and BMP signaling pathways regulates development of metanephric mesenchyme. Genes Dev. 13, 1601–1613. doi: 10.1101/gad.13.12.1601
Dudley, A. T., Lyons, K. M., and Robertson, E. J. (1995). A requirement for bone morphogenetic protein-7 during development of the mammalian kidney and eye. Genes Dev. 9, 2795–2807. doi: 10.1101/gad.9.22.2795
Durbec, P., Marcos-Gutierrez, C. V., Kilkenny, C., Grigoriou, M., Wartiowaara, K., Suvanto, P., et al. (1996). GDNF signalling through the ret receptor tyrosine kinase. Nature 381, 789–793. doi: 10.1038/381789a0
Enomoto, H., Hughes, I., Golden, J., Baloh, R. H., Yonemura, S., Heuckeroth, R. O., et al. (2004). GFRalpha1 expression in cells lacking RET is dispensable for organogenesis and nerve regeneration. Neuron 44, 623–636. doi: 10.1016/j.neuron.2004.10.032
Eph-Nomenclature-Committee (1997). Unified nomenclature for Eph family receptors and their ligands, the ephrins. Cell 90, 403–404. doi: 10.1016/S0092-8674(00)80500-0
Eremina, V., Sood, M., Haigh, J., Nagy, A., Lajoie, G., Ferrara, N., et al. (2003). Glomerular-specific alterations of VEGF-A expression lead to distinct congenital and acquired renal diseases. J. Clin. Invest. 111, 707–716. doi: 10.1172/JCI17423
Eswarakumar, V. P., Lax, I., and Schlessinger, J. (2005). Cellular signaling by fibroblast growth factor receptors. Cytokine Growth Factor. Rev. 16, 139–149. doi: 10.1016/j.cytogfr.2005.01.001
Feng, X. H., and Derynck, R. (2005). Specificity and versatility in tgf-beta signaling through Smads. Annu. Rev. Cell Dev. Biol. 21, 659–693. doi: 10.1146/annurev.cellbio.21.022404.142018
Fisher, C. E., Michael, L., Barnett, M. W., and Davies, J. A. (2001). Erk MAP kinase regulates branching morphogenesis in the developing mouse kidney. Development 128, 4329–4338.
Fruman, D. A., Chiu, H., Hopkins, B. D., Bagrodia, S., Cantley, L. C., and Abraham, R. T. (2017). The PI3K pathway in human disease. Cell 170, 605–635. doi: 10.1016/j.cell.2017.07.029
Georgas, K., Rumballe, B., Valerius, M. T., Chiu, H. S., Thiagarajan, R. D., Lesieur, E., et al. (2009). Analysis of early nephron patterning reveals a role for distal RV proliferation in fusion to the ureteric tip via a cap mesenchyme-derived connecting segment. Dev. Biol. 332, 273–286. doi: 10.1016/j.ydbio.2009.05.578
Gerber, S. D., Steinberg, F., Beyeler, M., Villiger, P. M., and Trueb, B. (2009). The murine Fgfrl1 receptor is essential for the development of the metanephric kidney. Dev. Biol. 335, 106–119. doi: 10.1016/j.ydbio.2009.08.019
Golden, J. P., Demaro, J. A., Osborne, P. A., Milbrandt, J., and Johnson, E. M., Jr. (1999). Expression of neurturin, GDNF, and GDNF family-receptor mRNA in the developing and mature mouse. Exp. Neurol. 158, 504–528. doi: 10.1006/exnr.1999.7127
Gotoh, N. (2008). Regulation of growth factor signaling by FRS2 family docking/scaffold adaptor proteins. Cancer Sci. 99, 1319–1325. doi: 10.1111/j.1349-7006.2008.00840.x
Grieshammer, U., Cebrian, C., Ilagan, R., Meyers, E., Herzlinger, D., and Martin, G. R. (2005). FGF8 is required for cell survival at distinct stages of nephrogenesis and for regulation of gene expression in nascent nephrons. Development 132, 3847–3857. doi: 10.1242/dev.01944
Grondin, R., Littrell, O. M., Zhang, Z., Ai, Y., Huettl, P., Pomerleau, F., et al. (2018). GDNF revisited: a novel mammalian cell-derived variant form of GDNF increases dopamine turnover and improves brain biodistribution. Neuropharmacology doi: 10.1016/j.neuropharm.2018.05.014[Epub ahead of print].
Hains, D., Sims-Lucas, S., Kish, K., Saha, M., Mchugh, K., and Bates, C. M. (2008). Role of fibroblast growth factor receptor 2 in kidney mesenchyme. Pediatr. Res. 64, 592–598. doi: 10.1203/PDR.0b013e318187cc12
Hains, D. S., Sims-Lucas, S., Carpenter, A., Saha, M., Murawski, I., Kish, K., et al. (2010). High incidence of vesicoureteral reflux in mice with Fgfr2 deletion in kidney mesenchyma. J. Urol. 183, 2077–2084. doi: 10.1016/j.juro.2009.12.095
Hartman, H. A., Lai, H. L., and Patterson, L. T. (2007). Cessation of renal morphogenesis in mice. Dev. Biol. 310, 379–387. doi: 10.1016/j.ydbio.2007.08.021
Hayashi, H., Ichihara, M., Iwashita, T., Murakami, H., Shimono, Y., Kawai, K., et al. (2000). Characterization of intracellular signals via tyrosine 1062 in RET activated by glial cell line-derived neurotrophic factor. Oncogene 19, 4469–4475. doi: 10.1038/sj.onc.1203799
Hellmich, H. L., Kos, L., Cho, E. S., Mahon, K. A., and Zimmer, A. (1996). Embryonic expression of glial cell-line derived neurotrophic factor (GDNF) suggests multiple developmental roles in neural differentiation and epithelial-mesenchymal interactions. Mech. Dev. 54, 95–105. doi: 10.1016/0925-4773(95)00464-5
Herzlinger, D., and Hurtado, R. (2014). Patterning the renal vascular bed. Semin. Cell Dev. Biol. 36, 50–56. doi: 10.1016/j.semcdb.2014.08.002
Hida, M., Omori, S., and Awazu, M. (2002). ERK and p38 MAP kinase are required for rat renal development. Kidney Int. 61, 1252–1262. doi: 10.1046/j.1523-1755.2002.00273.x
Hill, C. S. (2009). Nucleocytoplasmic shuttling of smad proteins. Cell Res. 19, 36–46. doi: 10.1038/cr.2008.325
Hu, M. C., Shiizaki, K., Kuro-O, M., and Moe, O. W. (2013). Fibroblast growth factor 23 and Klotho: physiology and pathophysiology of an endocrine network of mineral metabolism. Annu. Rev. Physiol. 75, 503–533. doi: 10.1146/annurev-physiol-030212-183727
Hyink, D. P., Tucker, D. C., St John, P. L., Leardkamolkarn, V., Accavitti, M. A., Abrass, C. K., et al. (1996). Endogenous origin of glomerular endothelial and mesangial cells in grafts of embryonic kidneys. Am. J. Physiol. 270, F886–F899. doi: 10.1152/ajprenal.1996.270.5.F886
Ihermann-Hella, A., Hirashima, T., Kupari, J., Kurtzeborn, K., Li, H., Kwon, H. N., et al. (2018). Dynamic MAPK/ERK activity sustains nephron progenitors through niche regulation and primes precursors for differentiation. Stem Cell Rep. 11, 912–928. doi: 10.1016/j.stemcr.2018.08.012
Ihermann-Hella, A., Lume, M., Miinalainen, I. J., Pirttiniemi, A., Gui, Y., Peranen, J., et al. (2014). Mitogen-activated protein kinase (MAPK) pathway regulates branching by remodeling epithelial cell adhesion. PLoS Genet 10:e1004193. doi: 10.1371/journal.pgen.1004193
Iwamoto, T., Taniguchi, M., Asai, N., Ohkusu, K., Nakashima, I., and Takahashi, M. (1993). cDNA cloning of mouse ret proto-oncogene and its sequence similarity to the cadherin superfamily. Oncogene 8, 1087–1091.
Jacob, M., Yusuf, F., and Jacob, H. J. (2012). “Development, differentiation and derivatives of the Wolffian and Müllerian ducts,” in The Human Embryo, ed. S. Yamada (Rijeka: Intech).
Jain, S., Encinas, M., Johnson, E. M. Jr., and Milbrandt, J. (2006). Critical and distinct roles for key RET tyrosine docking sites in renal development. Genes Dev. 20, 321–333. doi: 10.1101/gad.1387206
Jain, S., Knoten, A., Hoshi, M., Wang, H., Vohra, B., Heuckeroth, R. O., et al. (2010). Organotypic specificity of key RET adaptor-docking sites in the pathogenesis of neurocristopathies and renal malformations in mice. J. Clin. Invest. 120, 778–790. doi: 10.1172/JCI41619
Jijiwa, M., Fukuda, T., Kawai, K., Nakamura, A., Kurokawa, K., Murakumo, Y., et al. (2004). A targeting mutation of tyrosine 1062 in Ret causes a marked decrease of enteric neurons and renal hypoplasia. Mol. Cell. Biol. 24, 8026–8036. doi: 10.1128/MCB.24.18.8026-8036.2004
Keefe Davis, T., Hoshi, M., and Jain, S. (2013). Stage specific requirement of Gfralpha1 in the ureteric epithelium during kidney development. Mech. Dev. 130, 506–518. doi: 10.1016/j.mod.2013.03.001
Kim, D., and Dressler, G. R. (2007). PTEN modulates GDNF/RET mediated chemotaxis and branching morphogenesis in the developing kidney. Dev. Biol. 307, 290–299. doi: 10.1016/j.ydbio.2007.04.051
Kobayashi, A., Valerius, M. T., Mugford, J. W., Carroll, T. J., Self, M., Oliver, G., et al. (2008). Six2 defines and regulates a multipotent self-renewing nephron progenitor population throughout mammalian kidney development. Cell Stem Cell 3, 169–181. doi: 10.1016/j.stem.2008.05.020
Kullander, K., and Klein, R. (2002). Mechanisms and functions of Eph and ephrin signalling. Nat. Rev. Mol. Cell Biol. 3, 475–486. doi: 10.1038/nrm856
Kumar, A., Kopra, J., Varendi, K., Porokuokka, L. L., Panhelainen, A., Kuure, S., et al. (2015). GDNF overexpression from the native locus reveals its role in the nigrostriatal dopaminergic system function. PLoS Genet. 11:e1005710. doi: 10.1371/journal.pgen.1005808
Kuure, S., Cebrian, C., Machingo, Q., Lu, B. C., Chi, X., Hyink, D., et al. (2010a). Actin depolymerizing factors cofilin1 and destrin are required for ureteric bud branching morphogenesis. PLoS Genet. 6:e1001176. doi: 10.1371/journal.pgen.1001176
Kuure, S., Chi, X., Lu, B., and Costantini, F. (2010b). The transcription factors Etv4 and Etv5 mediate formation of the ureteric bud tip domain during kidney development. Development 137, 1975–1979. doi: 10.1242/dev.051656
Kuure, S., Popsueva, A., Jakobson, M., Sainio, K., and Sariola, H. (2007). Glycogen synthase kinase-3 inactivation and stabilization of beta-catenin induce nephron differentiation in isolated mouse and rat kidney mesenchymes. J. Am. Soc. Nephrol. 18, 1130–1139. doi: 10.1681/ASN.2006111206
Lake, D., Correa, S. A., and Muller, J. (2016). Negative feedback regulation of the ERK1/2 MAPK pathway. Cell Mol. Life Sci. 73, 4397–4413. doi: 10.1007/s00018-016-2297-8
Lam, A. Q., Freedman, B. S., Morizane, R., Lerou, P. H., Valerius, M. T., and Bonventre, J. V. (2014). Rapid and efficient differentiation of human pluripotent stem cells into intermediate mesoderm that forms tubules expressing kidney proximal tubular markers. J. Am. Soc. Nephrol. 25, 1211–1225. doi: 10.1681/ASN.2013080831
Lamouille, S., and Derynck, R. (2011). Emergence of the phosphoinositide 3-kinase-Akt-mammalian target of rapamycin axis in transforming growth factor-beta-induced epithelial-mesenchymal transition. Cells Tissues Organs 193, 8–22. doi: 10.1159/000320172
Li, Z., Araoka, T., Wu, J., Liao, H. K., Li, M., Lazo, M., et al. (2016). 3D culture supports long-term expansion of mouse and human nephrogenic progenitors. Cell Stem Cell 19, 516–529. doi: 10.1016/j.stem.2016.07.016
Lin, L. F., Doherty, D. H., Lile, J. D., Bektesh, S., and Collins, F. (1993). GDNF: a glial cell line-derived neurotrophic factor for midbrain dopaminergic neurons. Science 260, 1130–1132. doi: 10.1126/science.8493557
Lin, Y., Zhang, S., Rehn, M., Itaranta, P., Tuukkanen, J., Heljasvaara, R., et al. (2001). Induced repatterning of type XVIII collagen expression in ureter bud from kidney to lung type: association with sonic hedgehog and ectopic surfactant protein C. Development 128, 1573–1585.
Lindstrom, N. O., Carragher, N. O., and Hohenstein, P. (2015a). The PI3K pathway balances self-renewal and differentiation of nephron progenitor cells through beta-catenin signaling. Stem Cell Rep. 4, 551–560. doi: 10.1016/j.stemcr.2015.01.021
Lindstrom, N. O., Lawrence, M. L., Burn, S. F., Johansson, J. A., Bakker, E. R., Ridgway, R. A., et al. (2015b). Integrated beta-catenin, BMP, PTEN, and Notch signalling patterns the nephron. Elife 3:e04000. doi: 10.7554/eLife.04000
Lindstrom, N. O., De Sena Brandine, G., Tran, T., Ransick, A., Suh, G., Guo, J., et al. (2018a). Progressive recruitment of mesenchymal progenitors reveals a time-dependent process of cell fate acquisition in mouse and human nephrogenesis. Dev. Cell 45:e654. doi: 10.1016/j.devcel.2018.05.010
Lindstrom, N. O., Guo, J., Kim, A. D., Tran, T., Guo, Q., De Sena Brandine, G., et al. (2018b). Conserved and divergent features of mesenchymal progenitor cell types within the cortical nephrogenic niche of the human and mouse kidney. J. Am. Soc. Nephrol. 29, 806–824. doi: 10.1681/ASN.2017080890
Lindstrom, N. O., Mcmahon, J. A., Guo, J., Tran, T., Guo, Q., Rutledge, E., et al. (2018c). Conserved and divergent features of human and mouse kidney organogenesis. J. Am. Soc. Nephrol. 29, 785–805. doi: 10.1681/ASN.2017080887
Lindstrom, N. O., Tran, T., Guo, J., Rutledge, E., Parvez, R. K., Thornton, M. E., et al. (2018d). Conserved and divergent molecular and anatomic features of human and mouse nephron patterning. J. Am. Soc. Nephrol. 29, 825–840. doi: 10.1681/ASN.2017091036
Little, M. H., and McMahon, A. P. (2012). Mammalian kidney development: principles, progress, and projections. Cold Spring Harb. Perspect. Biol. 4:a008300. doi: 10.1101/cshperspect.a008300
Lu, B. C., Cebrian, C., Chi, X., Kuure, S., Kuo, R., Bates, C. M., et al. (2009). Etv4 and Etv5 are required downstream of GDNF and ret for kidney branching morphogenesis. Nat. Genet. 41, 1295–1302. doi: 10.1038/ng.476
Luo, G., Hofmann, C., Bronckers, A. L., Sohocki, M., Bradley, A., and Karsenty, G. (1995). BMP-7 is an inducer of nephrogenesis, and is also required for eye development and skeletal patterning. Genes Dev. 9, 2808–2820. doi: 10.1101/gad.9.22.2808
MacArthur, C. A., Lawshe, A., Xu, J., Santos-Ocampo, S., Heikinheimo, M., Chellaiah, A. T., et al. (1995). FGF-8 isoforms activate receptor splice forms that are expressed in mesenchymal regions of mouse development. Development 121, 3603–3613.
Maeshima, A., Sakurai, H., Choi, Y., Kitamura, S., Vaughn, D. A., Tee, J. B., et al. (2007). Glial cell-derived neurotrophic factor independent ureteric bud outgrowth from the Wolffian duct. J. Am. Soc. Nephrol. 18, 3147–3155. doi: 10.1681/ASN.2007060642
Magella, B., Adam, M., Potter, A. S., Venkatasubramanian, M., Chetal, K., Hay, S. B., et al. (2018). Cross-platform single cell analysis of kidney development shows stromal cells express Gdnf. Dev. Biol. 434, 36–47. doi: 10.1016/j.ydbio.2017.11.006
Majumdar, A., Vainio, S., Kispert, A., Mcmahon, J., and Mcmahon, A. P. (2003). Wnt11 and Ret/Gdnf pathways cooperate in regulating ureteric branching during metanephric kidney development. Development 130, 3175–3185. doi: 10.1242/dev.00520
Manning, B. D., and Toker, A. (2017). AKT/PKB signaling: navigating the network. Cell 169, 381–405. doi: 10.1016/j.cell.2017.04.001
Massague, J. (2012). TGFbeta signalling in context. Nat. Rev. Mol. Cell Biol. 13, 616–630. doi: 10.1038/nrm3434
Matsuo, I., and Kimura-Yoshida, C. (2013). Extracellular modulation of fibroblast growth factor signaling through heparan sulfate proteoglycans in mammalian development. Curr. Opin. Genet. Dev. 23, 399–407. doi: 10.1016/j.gde.2013.02.004
Menon, R., Otto, E. A., Kokoruda, A., Zhou, J., Zhang, Z., Yoon, E., et al. (2018). Single-cell analysis of progenitor cell dynamics and lineage specification in the human fetal kidney. Development 145:dev164038. doi: 10.1242/dev.164038
Miao, H., Nickel, C. H., Cantley, L. G., Bruggeman, L. A., Bennardo, L. N., and Wang, B. (2003). EphA kinase activation regulates HGF-induced epithelial branching morphogenesis. J. Cell Biol. 162, 1281–1292. doi: 10.1083/jcb.200304018
Miao, H., and Wang, B. (2009). Eph/ephrin signaling in epithelial development and homeostasis. Int. J. Biochem. Cell Biol. 41, 762–770. doi: 10.1016/j.biocel.2008.07.019
Michael, L., and Davies, J. A. (2004). Pattern and regulation of cell proliferation during murine ureteric bud development. J. Anat. 204, 241–255. doi: 10.1111/j.0021-8782.2004.00285.x
Michos, O., Cebrian, C., Hyink, D., Grieshammer, U., Williams, L., D’agati, V., et al. (2010). Kidney development in the absence of Gdnf and Spry1 requires Fgf10. PLoS Genet 6:e1000809. doi: 10.1371/journal.pgen.1000809
Miki, T., Bottaro, D. P., Fleming, T. P., Smith, C. L., Burgess, W. H., Chan, A. M., et al. (1992). Determination of ligand-binding specificity by alternative splicing: two distinct growth factor receptors encoded by a single gene. Proc. Natl. Acad. Sci. U.S.A. 89, 246–250. doi: 10.1073/pnas.89.1.246
Moore, M. W., Klein, R. D., Farinas, I., Sauer, H., Armanini, M., Phillips, H., et al. (1996). Renal and neuronal abnormalities in mice lacking GDNF. Nature 382, 76–79. doi: 10.1038/382076a0
Morizane, R., and Bonventre, J. V. (2017). Generation of nephron progenitor cells and kidney organoids from human pluripotent stem cells. Nat. Protoc. 12, 195–207. doi: 10.1038/nprot.2016.170
Morizane, R., Lam, A. Q., Freedman, B. S., Kishi, S., Valerius, M. T., and Bonventre, J. V. (2015). Nephron organoids derived from human pluripotent stem cells model kidney development and injury. Nat. Biotechnol. 33, 1193–1200. doi: 10.1038/nbt.3392
Motamedi, F. J., Badro, D. A., Clarkson, M., Lecca, M. R., Bradford, S. T., Buske, F. A., et al. (2014). WT1 controls antagonistic FGF and BMP-pSMAD pathways in early renal progenitors. Nat. Commun. 5:4444. doi: 10.1038/ncomms5444
Mu, Y., Gudey, S. K., and Landstrom, M. (2012). Non-Smad signaling pathways. Cell Tissue Res. 347, 11–20. doi: 10.1007/s00441-011-1201-y
Mugford, J. W., Sipila, P., Kobayashi, A., Behringer, R. R., and Mcmahon, A. P. (2008). Hoxd11 specifies a program of metanephric kidney development within the intermediate mesoderm of the mouse embryo. Dev. Biol. 319, 396–405. doi: 10.1016/j.ydbio.2008.03.044
Mugford, J. W., Yu, J., Kobayashi, A., and Mcmahon, A. P. (2009). High-resolution gene expression analysis of the developing mouse kidney defines novel cellular compartments within the nephron progenitor population. Dev. Biol. 333, 312–323. doi: 10.1016/j.ydbio.2009.06.043
O’Brien, L. L., Guo, Q., Lee, Y., Tran, T., Benazet, J. D., Whitney, P. H., et al. (2016). Differential regulation of mouse and human nephron progenitors by the Six family of transcriptional regulators. Development 143, 595–608. doi: 10.1242/dev.127175
Ohuchi, H., Hori, Y., Yamasaki, M., Harada, H., Sekine, K., Kato, S., et al. (2000). FGF10 acts as a major ligand for FGF receptor 2 IIIb in mouse multi-organ development. Biochem. Biophys. Res. Commun. 277, 643–649. doi: 10.1006/bbrc.2000.3721
Ola, R., Jakobson, M., Kvist, J., Perala, N., Kuure, S., Braunewell, K. H., et al. (2011). The GDNF target Vsnl1 marks the ureteric tip. J. Am. Soc. Nephrol. 22, 274–284. doi: 10.1681/ASN.2010030316
Osafune, K., Takasato, M., Kispert, A., Asashima, M., and Nishinakamura, R. (2006). Identification of multipotent progenitors in the embryonic mouse kidney by a novel colony-forming assay. Development 133, 151–161. doi: 10.1242/dev.02174
Oxburgh, L., and Robertson, E. J. (2002). Dynamic regulation of smad expression during mesenchyme to epithelium transition in the metanephric kidney. Mech. Dev. 112, 207–211. doi: 10.1016/S0925-4773(01)00648-7
Pachnis, V., Mankoo, B., and Costantini, F. (1993). Expression of the c-ret proto-oncogene during mouse embryogenesis. Development 119, 1005–1017.
Palmer, A., Zimmer, M., Erdmann, K. S., Eulenburg, V., Porthin, A., Heumann, R., et al. (2002). EphrinB phosphorylation and reverse signaling: regulation by Src kinases and PTP-BL phosphatase. Mol. Cell. 9, 725–737. doi: 10.1016/S1097-2765(02)00488-4
Park, J. S., Valerius, M. T., and Mcmahon, A. P. (2007). Wnt/beta-catenin signaling regulates nephron induction during mouse kidney development. Development 134, 2533–2539. doi: 10.1242/dev.006155
Patel, N. K., and Gill, S. S. (2007). GDNF delivery for Parkinson’s disease. Acta Neurochir. Suppl. 97, 135–154. doi: 10.1007/978-3-211-33081-4_16
Pelton, R. W., Saxena, B., Jones, M., Moses, H. L., and Gold, L. I. (1991). Immunohistochemical localization of TGF beta 1, TGF beta 2, and TGF beta 3 in the mouse embryo: expression patterns suggest multiple roles during embryonic development. J. Cell Biol. 115, 1091–1105. doi: 10.1083/jcb.115.4.1091
Pepicelli, C. V., Kispert, A., Rowitch, D. H., and Mcmahon, A. P. (1997). GDNF induces branching and increased cell proliferation in the ureter of the mouse. Dev. Biol. 192, 193–198. doi: 10.1006/dbio.1997.8745
Perantoni, A. O., Dove, L. F., and Karavanova, I. (1995). Basic fibroblast growth factor can mediate the early inductive events in renal development. Proc. Natl. Acad. Sci. U.S.A. 92, 4696–4700. doi: 10.1073/pnas.92.10.4696
Perantoni, A. O., Timofeeva, O., Naillat, F., Richman, C., Pajni-Underwood, S., Wilson, C., et al. (2005). Inactivation of FGF8 in early mesoderm reveals an essential role in kidney development. Development 132, 3859–3871. doi: 10.1242/dev.01945
Pichel, J. G., Shen, L., Sheng, H. Z., Granholm, A. C., Drago, J., Grinberg, A., et al. (1996). Defects in enteric innervation and kidney development in mice lacking GDNF. Nature 382, 73–76. doi: 10.1038/382073a0
Pitera, J. E., Woolf, A. S., Gale, N. W., Yancopoulos, G. D., and Yuan, H. T. (2004). Dysmorphogenesis of kidney cortical peritubular capillaries in angiopoietin-2-deficient mice. Am. J. Pathol. 165, 1895–1906. doi: 10.1016/S0002-9440(10)63242-7
Plisov, S. Y., Yoshino, K., Dove, L. F., Higinbotham, K. G., Rubin, J. S., and Perantoni, A. O. (2001). TGF beta 2, LIF and FGF2 cooperate to induce nephrogenesis. Development 128, 1045–1057.
Plotnikov, A., Zehorai, E., Procaccia, S., and Seger, R. (2011). The MAPK cascades: signaling components, nuclear roles and mechanisms of nuclear translocation. Biochim. Biophys. Acta 1813, 1619–1633. doi: 10.1016/j.bbamcr.2010.12.012
Poladia, D. P., Kish, K., Kutay, B., Hains, D., Kegg, H., Zhao, H., et al. (2006). Role of fibroblast growth factor receptors 1 and 2 in the metanephric mesenchyme. Dev. Biol. 291, 325–339. doi: 10.1016/j.ydbio.2005.12.034
Prakash, Y., Thompson, M. A., Meuchel, L., Pabelick, C. M., Mantilla, C. B., Zaidi, S., et al. (2010). Neurotrophins in lung health and disease. Expert Rev. Respir. Med. 4, 395–411. doi: 10.1586/ers.10.29
Puri, P., Bushnell, D., Schaefer, C. M., and Bates, C. M. (2016). Six2creFrs2alpha knockout mice are a novel model of renal cystogenesis. Sci. Rep. 6:36736. doi: 10.1038/srep36736
Pylayeva-Gupta, Y., Grabocka, E., and Bar-Sagi, D. (2011). RAS oncogenes: weaving a tumorigenic web. Nat. Rev. Cancer 11, 761–774. doi: 10.1038/nrc3106
Qiao, J., Uzzo, R., Obara-Ishihara, T., Degenstein, L., Fuchs, E., and Herzlinger, D. (1999). FGF-7 modulates ureteric bud growth and nephron number in the developing kidney. Development 126, 547–554.
Rapraeger, A. C., Krufka, A., and Olwin, B. B. (1991). Requirement of heparan sulfate for bFGF-mediated fibroblast growth and myoblast differentiation. Science 252, 1705–1708. doi: 10.1126/science.1646484
Riccio, P., Cebrian, C., Zong, H., Hippenmeyer, S., and Costantini, F. (2016). Ret and Etv4 promote directed movements of progenitor cells during renal branching morphogenesis. PLoS Biol. 14:e1002382. doi: 10.1371/journal.pbio.1002488
Roskoski, R. Jr. (2012a). ERK1/2 MAP kinases: structure, function, and regulation. Pharmacol. Res. 66, 105–143. doi: 10.1016/j.phrs.2012.04.005
Roskoski, R. Jr. (2012b). MEK1/2 dual-specificity protein kinases: structure and regulation. Biochem. Biophys. Res. Commun. 417, 5–10. doi: 10.1016/j.bbrc.2011.11.145
Roskoski, R. Jr. (2010). RAF protein-serine/threonine kinases: structure and regulation. Biochem. Biophys. Res. Commun. 399, 313–317. doi: 10.1016/j.bbrc.2010.07.092
Rozen, E. J., Schmidt, H., Dolcet, X., Basson, M. A., Jain, S., and Encinas, M. (2009). Loss of Sprouty1 rescues renal agenesis caused by ret mutation. J. Am. Soc. Nephrol. 20, 255–259. doi: 10.1681/ASN.2008030267
Rumballe, B. A., Georgas, K. M., Combes, A. N., Ju, A. L., Gilbert, T., and Little, M. H. (2011). Nephron formation adopts a novel spatial topology at cessation of nephrogenesis. Dev. Biol. 360, 110–122. doi: 10.1016/j.ydbio.2011.09.011
Runeberg-Roos, P., Piccinini, E., Penttinen, A. M., Matlik, K., Heikkinen, H., Kuure, S., et al. (2016). Developing therapeutically more efficient Neurturin variants for treatment of Parkinson’s disease. Neurobiol. Dis. 96, 335–345. doi: 10.1016/j.nbd.2016.07.008
Sainio, K., Nonclercq, D., Saarma, M., Palgi, J., Saxen, L., and Sariola, H. (1994). Neuronal characteristics in embryonic renal stroma. Int. J. Dev. Biol. 38, 77–84.
Sainio, K., Suvanto, P., Davies, J., Wartiovaara, J., Wartiovaara, K., Saarma, M., et al. (1997). Glial-cell-line-derived neurotrophic factor is required for bud initiation from ureteric epithelium. Development 124, 4077–4087.
Sanchez, M. P., Silos-Santiago, I., Frisen, J., He, B., Lira, S. A., and Barbacid, M. (1996). Renal agenesis and the absence of enteric neurons in mice lacking GDNF. Nature 382, 70–73. doi: 10.1038/382070a0
Saraga-Babic, M., Vukojevic, K., Bocina, I., Drnasin, K., and Saraga, M. (2012). Ciliogenesis in normal human kidney development and post-natal life. Pediatr. Nephrol. 27, 55–63. doi: 10.1007/s00467-011-1941-7
Sariola, H. (2001). The neurotrophic factors in non-neuronal tissues. Cell Mol. Life Sci. 58, 1061–1066. doi: 10.1007/PL00000921
Sariola, H. (2002). Nephron induction revisited: from caps to condensates. Curr. Opin. Nephrol. Hypertens 11, 17–21. doi: 10.1097/00041552-200201000-00003
Sariola, H., Holm, K., and Henke, F. S. (1988). Early innervation of the metanephric kidney. Development 104, 589–599.
Sariola, H., Saarma, M., Sainio, K., Arumae, U., Palgi, J., Vaahtokari, A., et al. (1991). Dependence of kidney morphogenesis on the expression of nerve growth factor receptor. Science 254, 571–573. doi: 10.1126/science.1658930
Saxen, L. (1987). Organogenesis of the Kidney. Cambridge: Cambridge University Press. doi: 10.1017/CBO9780511565083
Saxen, L., and Sariola, H. (1987). Early organogenesis of the kidney. Pediatr. Nephrol. 1, 385–392. doi: 10.1007/BF00849241
Schuchardt, A., D’agati, V., Larsson-Blomberg, L., Costantini, F., and Pachnis, V. (1994). Defects in the kidney and enteric nervous system of mice lacking the tyrosine kinase receptor Ret. Nature 367, 380–383. doi: 10.1038/367380a0
Shakya, R., Watanabe, T., and Costantini, F. (2005). The role of GDNF/Ret signaling in ureteric bud cell fate and branching morphogenesis. Dev. Cell 8, 65–74. doi: 10.1016/j.devcel.2004.11.008
Short, K. M., Combes, A. N., Lefevre, J., Ju, A. L., Georgas, K. M., Lamberton, T., et al. (2014). Global quantification of tissue dynamics in the developing mouse kidney. Dev. Cell 29, 188–202. doi: 10.1016/j.devcel.2014.02.017
Sims-Lucas, S., Cullen-Mcewen, L., Eswarakumar, V. P., Hains, D., Kish, K., Becknell, B., et al. (2009). Deletion of Frs2alpha from the ureteric epithelium causes renal hypoplasia. Am. J. Physiol. Renal Physiol. 297, F1208–F1219. doi: 10.1152/ajprenal.00262.2009
Sims-Lucas, S., Cusack, B., Baust, J., Eswarakumar, V. P., Masatoshi, H., Takeuchi, A., et al. (2011a). Fgfr1 and the IIIc isoform of Fgfr2 play critical roles in the metanephric mesenchyme mediating early inductive events in kidney development. Dev. Dyn. 240, 240–249. doi: 10.1002/dvdy.22501
Sims-Lucas, S., Cusack, B., Eswarakumar, V. P., Zhang, J., Wang, F., and Bates, C. M. (2011b). Independent roles of Fgfr2 and Frs2alpha in ureteric epithelium. Development 138, 1275–1280. doi: 10.1242/dev.062158
Sims-Lucas, S., Di Giovanni, V., Schaefer, C., Cusack, B., Eswarakumar, V. P., and Bates, C. M. (2012). Ureteric morphogenesis requires Fgfr1 and Fgfr2/Frs2alpha signaling in the metanephric mesenchyme. J. Am. Soc. Nephrol. 23, 607–617. doi: 10.1681/ASN.2011020165
Smeeton, J., Zhang, X., Bulus, N., Mernaugh, G., Lange, A., Karner, C. M., et al. (2010). Integrin-linked kinase regulates p38 MAPK-dependent cell cycle arrest in ureteric bud development. Development 137, 3233–3243. doi: 10.1242/dev.052845
Stark, K., Vainio, S., Vassileva, G., and Mcmahon, A. P. (1994). Epithelial transformation of metanephric mesenchyme in the developing kidney regulated by Wnt-4. Nature 372, 679–683. doi: 10.1038/372679a0
Steinberg, F., Zhuang, L., Beyeler, M., Kalin, R. E., Mullis, P. E., Brandli, A. W., et al. (2010). The FGFRL1 receptor is shed from cell membranes, binds fibroblast growth factors (FGFs), and antagonizes FGF signaling in Xenopus embryos. J. Biol. Chem. 285, 2193–2202. doi: 10.1074/jbc.M109.058248
Sun, X., Mariani, F. V., and Martin, G. R. (2002). Functions of FGF signalling from the apical ectodermal ridge in limb development. Nature 418, 501–508. doi: 10.1038/nature00902
Taguchi, A., Kaku, Y., Ohmori, T., Sharmin, S., Ogawa, M., Sasaki, H., et al. (2014). Redefining the in vivo origin of metanephric nephron progenitors enables generation of complex kidney structures from pluripotent stem cells. Cell Stem Cell 14, 53–67. doi: 10.1016/j.stem.2013.11.010
Takabatake, Y., Sugiyama, T., Kohara, H., Matsusaka, T., Kurihara, H., Koni, P. A., et al. (2009). The CXCL12 (SDF-1)/CXCR4 axis is essential for the development of renal vasculature. J. Am. Soc. Nephrol. 20, 1714–1723. doi: 10.1681/ASN.2008060640
Takahashi, M., Buma, Y., Iwamoto, T., Inaguma, Y., Ikeda, H., and Hiai, H. (1988). Cloning and expression of the ret proto-oncogene encoding a tyrosine kinase with two potential transmembrane domains. Oncogene 3, 571–578.
Takahashi, T., Takahashi, K., Gerety, S., Wang, H., Anderson, D. J., and Daniel, T. O. (2001). Temporally compartmentalized expression of ephrin-B2 during renal glomerular development. J. Am. Soc. Nephrol. 12, 2673–2682.
Takasato, M., Er, P. X., Becroft, M., Vanslambrouck, J. M., Stanley, E. G., Elefanty, A. G., et al. (2014). Directing human embryonic stem cell differentiation towards a renal lineage generates a self-organizing kidney. Nat. Cell Biol. 16, 118–126. doi: 10.1038/ncb2894
Takasato, M., Er, P. X., Chiu, H. S., Maier, B., Baillie, G. J., Ferguson, C., et al. (2015). Kidney organoids from human iPS cells contain multiple lineages and model human nephrogenesis. Nature 526, 564–568. doi: 10.1038/nature15695
Takasato, M., and Little, M. H. (2015). The origin of the mammalian kidney: implications for recreating the kidney in vitro. Development 142, 1937–1947. doi: 10.1242/dev.104802
Tang, M. J., Cai, Y., Tsai, S. J., Wang, Y. K., and Dressler, G. R. (2002). Ureteric bud outgrowth in response to RET activation is mediated by phosphatidylinositol 3-kinase. Dev. Biol. 243, 128–136. doi: 10.1006/dbio.2001.0557
Tee, J. B., Choi, Y., Dnyanmote, A., Decambre, M., Ito, C., Bush, K. T., et al. (2013). GDNF-independent ureteric budding: role of PI3K-independent activation of AKT and FOSB/JUN/AP-1 signaling. Biol. Open 2, 952–959. doi: 10.1242/bio.20135595
Tomita, M., Asada, M., Asada, N., Nakamura, J., Oguchi, A., Higashi, A. Y., et al. (2013). Bmp7 maintains undifferentiated kidney progenitor population and determines nephron numbers at birth. PLoS One 8:e73554. doi: 10.1371/journal.pone.0073554
Trupp, M., Arenas, E., Fainzilber, M., Nilsson, A. S., Sieber, B. A., Grigoriou, M., et al. (1996). Functional receptor for GDNF encoded by the c-ret proto-oncogene. Nature 381, 785–788. doi: 10.1038/381785a0
Tufro-McReddie, A., Norwood, V. F., Aylor, K. W., Botkin, S. J., Carey, R. M., and Gomez, R. A. (1997). Oxygen regulates vascular endothelial growth factor-mediated vasculogenesis and tubulogenesis. Dev. Biol. 183, 139–149. doi: 10.1006/dbio.1997.8513
Tufro-McReddie, A., Romano, L. M., Harris, J. M., Ferder, L., and Gomez, R. A. (1995). Angiotensin II regulates nephrogenesis and renal vascular development. Am. J. Physiol. 269, F110–F115. doi: 10.1152/ajprenal.1995.269.1.F110
Turner, N., and Grose, R. (2010). Fibroblast growth factor signalling: from development to cancer. Nat. Rev. Cancer 10, 116–129. doi: 10.1038/nrc2780
Unbekandt, M., and Davies, J. A. (2010). Dissociation of embryonic kidneys followed by reaggregation allows the formation of renal tissues. Kidney Int. 77, 407–416. doi: 10.1038/ki.2009.482
Vega, J. A., Garcia-Suarez, O., Hannestad, J., Perez-Perez, M., and Germana, A. (2003). Neurotrophins and the immune system. J. Anat. 203, 1–19. doi: 10.1046/j.1469-7580.2003.00203.x
Volovelsky, O., Nguyen, T., Jarmas, A. E., Combes, A. N., Wilson, S. B., Little, M. H., et al. (2018). Hamartin regulates cessation of mouse nephrogenesis independently of Mtor. Proc. Natl. Acad. Sci. U.S.A. 115, 5998–6003. doi: 10.1073/pnas.1712955115
Walker, K. A., Sims-Lucas, S., and Bates, C. M. (2016). Fibroblast growth factor receptor signaling in kidney and lower urinary tract development. Pediatr. Nephrol. 31, 885–895. doi: 10.1007/s00467-015-3151-1
Wang, H. U., Chen, Z. F., and Anderson, D. J. (1998). Molecular distinction and angiogenic interaction between embryonic arteries and veins revealed by ephrin-B2 and its receptor Eph-B4. Cell 93, 741–753. doi: 10.1016/S0092-8674(00)81436-1
Wang, P., Chen, Y., Yong, J., Cui, Y., Wang, R., Wen, L., et al. (2018). Dissecting the global dynamic molecular profiles of human fetal kidney development by single-cell RNA sequencing. Cell Rep. 24:e3553. doi: 10.1016/j.celrep.2018.08.056
Watanabe, T., and Costantini, F. (2004). Real-time analysis of ureteric bud branching morphogenesis in vitro. Dev. Biol. 271, 98–108. doi: 10.1016/j.ydbio.2004.03.025
Weinstein, M., Xu, X., Ohyama, K., and Deng, C. X. (1998). FGFR-3 and FGFR-4 function cooperatively to direct alveogenesis in the murine lung. Development 125, 3615–3623.
Weiss, A., and Attisano, L. (2013). The TGFbeta superfamily signaling pathway. Wiley Interdiscip. Rev. Dev. Biol. 2, 47–63. doi: 10.1002/wdev.86
Weiss, A. C., Airik, R., Bohnenpoll, T., Greulich, F., Foik, A., Trowe, M. O., et al. (2014). Nephric duct insertion requires EphA4/EphA7 signaling from the pericloacal mesenchyme. Development 141, 3420–3430. doi: 10.1242/dev.113928
Weiss, A. C., and Kispert, A. (2016). Eph/ephrin signaling in the kidney and lower urinary tract. Pediatr. Nephrol. 31, 359–371. doi: 10.1007/s00467-015-3112-8
Weston, C. R., and Davis, R. J. (2007). The JNK signal transduction pathway. Curr. Opin. Cell Biol. 19, 142–149. doi: 10.1016/j.ceb.2007.02.001
Willecke, R., Heuberger, J., Grossmann, K., Michos, O., Schmidt-Ott, K., Walentin, K., et al. (2011). The tyrosine phosphatase Shp2 acts downstream of GDNF/Ret in branching morphogenesis of the developing mouse kidney. Dev. Biol. 360, 310–317. doi: 10.1016/j.ydbio.2011.09.029
Wong, A., Bogni, S., Kotka, P., De Graaff, E., D’agati, V., Costantini, F., et al. (2005). Phosphotyrosine 1062 is critical for the in vivo activity of the Ret9 receptor tyrosine kinase isoform. Mol. Cell. Biol. 25, 9661–9673. doi: 10.1128/MCB.25.21.9661-9673.2005
Wortzel, I., and Seger, R. (2011). The ERK cascade: distinct functions within various subcellular organelles. Genes Cancer 2, 195–209. doi: 10.1177/1947601911407328
Xu, L., Kang, Y., Col, S., and Massague, J. (2002). Smad2 nucleocytoplasmic shuttling by nucleoporins CAN/Nup214 and Nup153 feeds TGFbeta signaling complexes in the cytoplasm and nucleus. Mol. Cell 10, 271–282. doi: 10.1016/S1097-2765(02)00586-5
Yang, X., Steinberg, F., Zhuang, L., Bessey, R., and Trueb, B. (2016). Receptor FGFRL1 does not promote cell proliferation but induces cell adhesion. Int. J. Mol. Med. 38, 30–38. doi: 10.3892/ijmm.2016.2601
Yayon, A., Klagsbrun, M., Esko, J. D., Leder, P., and Ornitz, D. M. (1991). Cell surface, heparin-like molecules are required for binding of basic fibroblast growth factor to its high affinity receptor. Cell 64, 841–848. doi: 10.1016/0092-8674(91)90512-W
Yu, J. S., and Cui, W. (2016). Proliferation, survival and metabolism: the role of PI3K/AKT/mTOR signalling in pluripotency and cell fate determination. Development 143, 3050–3060. doi: 10.1242/dev.137075
Yu, T., Yaguchi, Y., Echevarria, D., Martinez, S., and Basson, M. A. (2011). Sprouty genes prevent excessive FGF signalling in multiple cell types throughout development of the cerebellum. Development 138, 2957–2968. doi: 10.1242/dev.063784
Zhang, X., Ibrahimi, O. A., Olsen, S. K., Umemori, H., Mohammadi, M., and Ornitz, D. M. (2006). Receptor specificity of the fibroblast growth factor family, the complete mammalian FGF family. J. Biol. Chem. 281, 15694–15700. doi: 10.1074/jbc.M601252200
Keywords: receptor tyrosin kinase, development, kidney, intracelluar signaling, renal differentiation, morphogenesis, kidney morphogenesis
Citation: Kurtzeborn K, Cebrian C and Kuure S (2018) Regulation of Renal Differentiation by Trophic Factors. Front. Physiol. 9:1588. doi: 10.3389/fphys.2018.01588
Received: 27 August 2018; Accepted: 23 October 2018;
Published: 12 November 2018.
Edited by:
George Grant, University of Aberdeen, United KingdomReviewed by:
Anna Julie Peired, Università degli Studi di Firenze, ItalyZubaida R. Saifudeen, Tulane University, United States
Gianandrea Pasquinelli, Università degli Studi di Bologna, Italy
Copyright © 2018 Kurtzeborn, Cebrian and Kuure. This is an open-access article distributed under the terms of the Creative Commons Attribution License (CC BY). The use, distribution or reproduction in other forums is permitted, provided the original author(s) and the copyright owner(s) are credited and that the original publication in this journal is cited, in accordance with accepted academic practice. No use, distribution or reproduction is permitted which does not comply with these terms.
*Correspondence: Satu Kuure, c2F0dS5rdXVyZUBoZWxzaW5raS5maQ==