- Department of Medical Sciences, Uppsala University, Uppsala, Sweden
Ever since Claude Bernards discovery in the mid 19th-century that a lesion in the floor of the third ventricle in dogs led to altered systemic glucose levels, a role of the CNS in whole-body glucose regulation has been acknowledged. However, this finding was later overshadowed by the isolation of pancreatic hormones in the 20th century. Since then, the understanding of glucose homeostasis and pathology has primarily evolved around peripheral mechanism. Due to scientific advances over these last few decades, however, increasing attention has been given to the possibility of the brain as a key player in glucose regulation and the pathogenesis of metabolic disorders such as type 2 diabetes. Studies of animals have enabled detailed neuroanatomical mapping of CNS structures involved in glucose regulation and key neuronal circuits and intracellular pathways have been identified. Furthermore, the development of neuroimaging techniques has provided methods to measure changes of activity in specific CNS regions upon diverse metabolic challenges in humans. In this narrative review, we discuss the available evidence on the topic. We conclude that there is much evidence in favor of active CNS involvement in glucose homeostasis but the relative importance of central vs. peripheral mechanisms remains to be elucidated. An increased understanding of this field may lead to new CNS-focusing pharmacologic strategies in the treatment of type 2 diabetes.
Introduction
The global prevalence of diabetes in adults – approximately 90% consisting of type 2 diabetes – was estimated to 6.4% in 2010 and is predicted to increase to 7.7% in 2030 (Nolan et al., 2011). The macro- and microvascular complications that are associated with diabetes lead to increased morbidity and mortality and the economic burden posed by management of diabetes and its complications is substantial (Ng et al., 2014; Norhammar et al., 2016). Type 2 diabetes typically evolves gradually. An initial phase of insulin resistance with maintained normoglycemia is followed by a transitional phase of impaired fasting glucose and/or impaired glucose tolerance until manifest diabetes is established. While the pancreatic beta cells can compensate for the insulin resistance by increasing insulin secretion at first, they eventually fail to do so as the disease progresses, frequently necessitating exogenously administered insulin in advanced stages. Since the discovery of the pancreatic hormones insulin and glucagon, the prevailing understanding of type 2 diabetes development has circled around processes in the periphery, particularly in the pancreas. Likewise, pharmacological targets in the treatment of type 2 diabetes have been largely limited to the peripheral domain. However, this “islet-centric” model has these last decades been challenged by mounting evidence in favor of a “brain-centric” model, according to which the brain is actively involved in systemic glucose regulation. Further advances in this area may change the way we look at metabolic disorders and may specifically result in new CNS-targeted strategies for the pharmacological management of type 2 diabetes. In this narrative review, we aim to present the current knowledge of the field. In the first section, we will provide a brief summary of findings from animal studies that have been extensively reviewed by other authors (Marty et al., 2007; Carey et al., 2013; Grayson et al., 2013; Mergenthaler et al., 2013; Roh et al., 2016; Tups et al., 2017; López-Gambero et al., 2019). This will be followed up by a more in-depth presentation of evidence from human studies where the implementation and advances of neuroimaging techniques has offered new and interesting insights.
Evidence From Animal Studies
In 1854, Claude Bernard reported that a lesion in the floor of the fourth ventricle in dogs altered glucose levels, thereby presenting the first evidence of the brain’s role in glucose regulation (Bernard, 1855). In the 1960s two sets of neurons were identified in the CNS that responded to high and low values of glucose, respectively (Anand et al., 1964; Oomura et al., 1964, 1969, 1974). These neurons were subsequently termed glucose-excitatory (GE, responding to high levels of glucose) and glucose-inhibitory (GI, responding to low levels of glucose) (Routh et al., 2014). While present in the entire CNS, these neurons are especially numerous in several nuclei of the hypothalamus and the brainstem (López-Gambero et al., 2019).
The hypothalamus is located below the thalamus and above the pituitary gland and brain stem. It constitutes the floor of the third ventricle which contains cerebrospinal fluid (CSF). This anatomical position allows for access to nutrients and hormones. It consists of a network of interconnected nuclei among which the arcuate nucleus (ARC), ventromedial hypothalamus (VMH), dorsomedial nucleus (DMN), paraventricular nucleus (PVN), and the lateral hypothalamus (LH) are implicated in the regulation of glucose homeostasis. In the brainstem the nucleus of the solitary tract (NTS), area postrema (AP), dorsal motor nucleus of the vagus (DMNX) and the rostral ventrolateral medulla (RVLM) are key regions (López-Gambero et al., 2019). Glucose-excited neurons are primarily prevalent in the ARC, VMH, and PVN, whereas glucose-inhibited neurons are concentrated in the LH, AN, and PVN. In the brainstem, both of these types of neurons are represented in the areas mentioned above (NTS, AP, and DMNX) (Roh et al., 2016) (Figure 1).
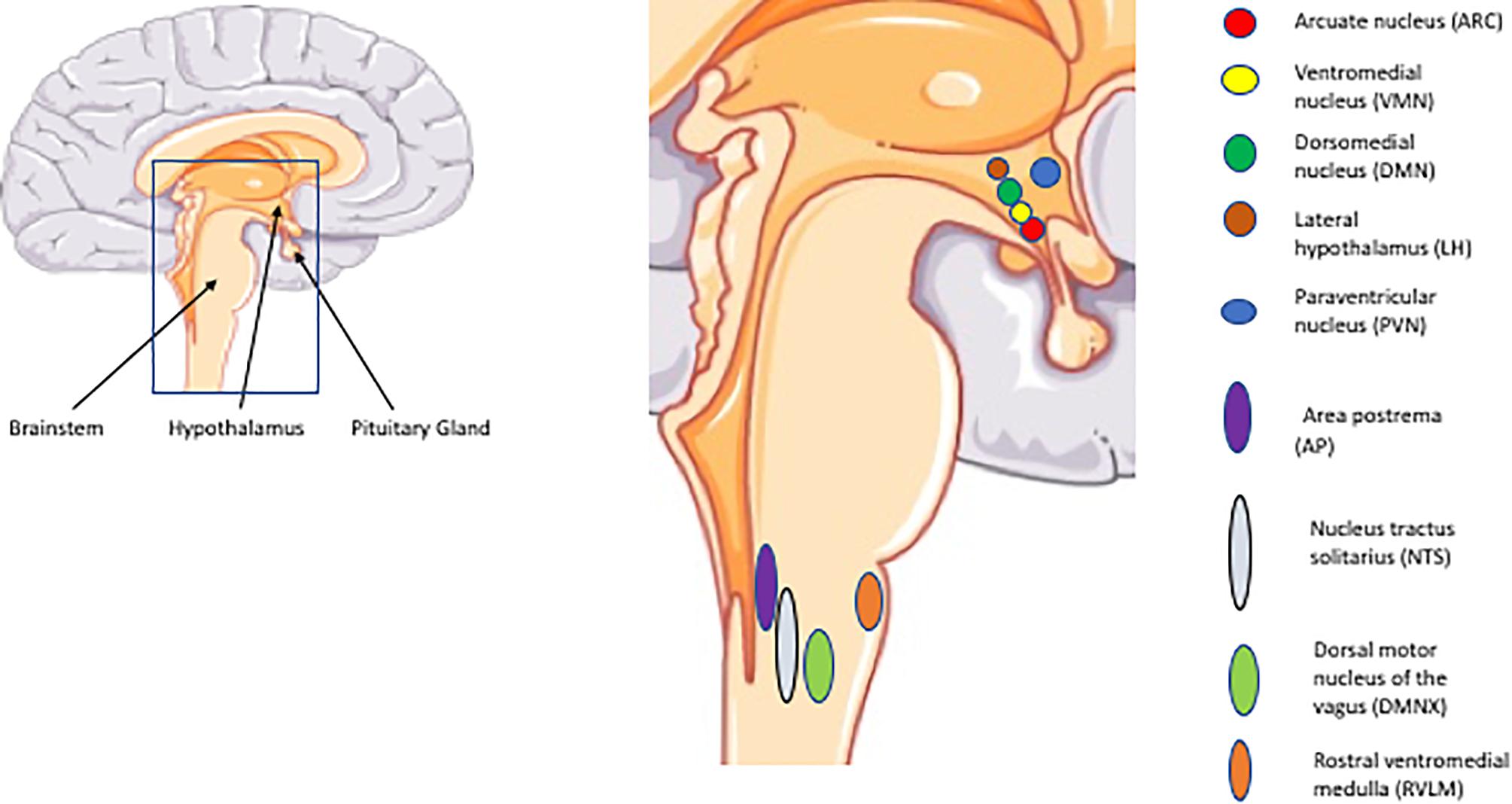
Figure 1. Important CNS regions and nuclei implicated in glucose regulation. The illustrations of anatomical structures were retrieved from http://smart.servier.com and have thereafter been assembled and processed.
Glucose injected into the third ventricle of rodents leads to a reduction of systemic glucose levels, principally by suppression of hepatic glucose production (HGP) (Lam et al., 2005a). The same has been demonstrated for long-chain fatty acids (Obici et al., 2002b; Lam et al., 2005b) and amino acids such as leucine (Su et al., 2012), proline (Arrieta-Cruz et al., 2013) and histidine (Kimura et al., 2013). Apart from being able to sense levels of circulating glucose, the brain also receives afferent nervous input via the brainstem related to glucose availability from taste buds in the oral cavity (Ahrén, 2000), from the intestinal mucosa (Ashley Blackshaw and Young, 2011) and from hepatoportal glucoreceptors (Delaere et al., 2010). This allows for rapid responses to ingested and circulating glucose such as observed in the cephalic insulin phase (Marty et al., 2007). Likewise, ingestion of lipids leads to release of CCK whereas amino acids stimulate release of CCK, GLP-1, and PYY. These gut hormones in turn activate vagal afferents projecting to the brainstem (Duca and Yue, 2014; Liu et al., 2016).
Not only neurons but also surrounding glial cells are believed to have an active role in the sensing of nutrients. It has been hypothesized that astrocytes may metabolize glucose and proline into lactate for subsequent translocation in the so-called astrocyte-neuron-lactate-shuttle to neurons to signal glucose depletion (Arrieta-Cruz et al., 2013). Likewise, oxidation of fatty acids may occur to a larger extent in astrocytes, generating ketones which after translocation to neurons may override the influence of glucose and fatty acid sensing (Bruce et al., 2017).
Similarly, central administration of hormones or targeting of their intracellular pathways in neurons have demonstrated that insulin (Obici et al., 2002a,c; Pocai et al., 2005), leptin (Schwartz et al., 1996; Kievit et al., 2006; Koch et al., 2010), glucagon (Mighiu et al., 2013; LaPierre et al., 2015), GLP-1 (Sandoval et al., 2008) and CCK (Zhu et al., 2012; Montgomery et al., 2013) reduces systemic glucose levels by CNS actions, whereas the effect of ghrelin seems to be the opposite (Scott et al., 2012; Wang et al., 2013; Stark et al., 2015; Lee et al., 2016). Perhaps most interesting is the potent CNS-mediated glucose lowering properties of the fibroblast growth factors FGF-1 (Scarlett et al., 2016) and FGF-19 (Fu et al., 2004; Morton et al., 2013; Marcelin et al., 2014). A single low dose of FGF-1 injected into the third ventricle induced sustained diabetic remission in mice with diet-induced obesity. The effect was rapid and sustained. Thus, fasting blood glucose concentration was reduced by 25% within 6 h, normalized after 7 days and remained in the normal range for the full 17 weeks studied without any observed hypoglycemia (Scarlett et al., 2016).
The transport of glucose across the blood–brain barrier (BBB) is primarily mediated by insulin-independent GLUT-1 and, particularly in conditions of CNS ischemia and glucose deprivation, by SGLT-1 (Patching, 2017). Interestingly though, insulin-mediated increase in CNS glucose uptake via upregulation of GLUT-1 in astrocytes has been described (García-Cáceres et al., 2016). Additionally, insulin-dependent GLUT4 is expressed in cells of the BBB, albeit at low levels (Patching, 2017). The existence of a more direct route, bypassing the BBB by fenestrated capillaries or via the CSF has been proposed and is supported by some work (Langlet et al., 2013; Routh et al., 2014). Fatty acids and amino acids have been demonstrated to cross the BBB (Smith, 2000; Rapoport et al., 2001; Smith and Nagura, 2001) by mechanisms that are not fully understood but may involve fatty acid transport proteins (FATPs) (Mitchell et al., 2011) and similar systems for amino acids (Smith, 2000). The levels of the large hormones insulin and leptin are considerably lower in the CFS than in the systemic circulation, suggesting the existence of saturable transporter proteins rather than simple diffusion (Banks et al., 1996; Gray and Barrett, 2018). While the exact mechanisms of CNS transport for these hormones remain elusive, hormone receptors seem to be involved (Banks et al., 1997; Bjørbaek et al., 1998; Boado et al., 1998; Hileman et al., 2002; Meijer et al., 2016). GLP-1 is synthesized within the CNS where it acts as transmitter (Katsurada et al., 2014; Katsurada and Yada, 2016), whereas the hypothesized intracerebral insulin synthesis is still a matter of some controversy (Gray and Barrett, 2018).
The intracellular mechanisms of nutrient and hormonal sensing in the CNS have been intensively investigated in animal studies. Glucose uptake into neurons occurs mainly via insulin-independent glucose transporter channels (GLUT1, 2, and 3) and this activates signaling pathways that differ for GE and GI neurons, involving activation of KATP-channels in the former and the signaling substance AMPK in the latter. In GE neurons, metabolism-independent sensing mechanisms involving SGLT1 and 3 and the sweet receptor T1R2/3 are also implicated (López-Gambero et al., 2019). The receptors of insulin (Chen et al., 2017), leptin (Mercer et al., 1996), glucagon (Abraham and Lam, 2016), GLP-1 (Katsurada and Yada, 2016), CCK (Pathak et al., 2018), and ghrelin (Cowley et al., 2003; Sun et al., 2007) have been identified in various regions of the CNS. Insulin signaling in neurons is dependent on activation of KATP-channels (Pocai et al., 2005) and is facilitated by activation of the IRS-PI3K- pathway (Niswender et al., 2003). Leptin also acts on this pathway by phosphorylation of IRS1 (Koch et al., 2010) in addition to the JAK2-STAT3 and WNT signaling pathways (Tups et al., 2017). Thus, leptin may sensitize neurons in the hypothalamus to the effects of insulin but may also exert central effects independent of insulin, as is supported by the fact that leptin can reverse hyperglycemia in mice with streptozotocin-induced type 1 diabetes (Yu et al., 2008).
There is much evidence that the central effects of insulin and leptin differ in obese compared to lean animals. Impairments in CNS transport, receptor expression and the downstream signaling cascades have been observed for both hormones in models of obesity (Van Heek et al., 1997; El-Haschimi et al., 2000; Kaiyala et al., 2000; Spanswick et al., 2000; Carvalheira et al., 2003; Asilmaz et al., 2004; Wilsey and Scarpace, 2004; Urayama and Banks, 2008). Hypothalamic inflammation has been proposed as a causative factor. Increased expression of inflammatory mediators such as TNF-alpha and increased endoplasmatic reticulum (ER) stress is a well-established feature of obesity (Hotamisligil et al., 1993; Ozcan et al., 2004). Cytokines and ER stress lead to activation of the intracellular JNK1 and IKKβ/NFkB pathways. This has been demonstrated to induce insulin resistance in peripheral tissues through serine phosphorylation of IRS-1. Obesity and over-nutrition is associated with hypothalamic inflammation, which may in turn result in insulin and leptin resistance via the mechanisms described above (Hosoi et al., 2008; Zhang et al., 2008; Milanski et al., 2009; Ozcan et al., 2009; Posey et al., 2009). Indeed, central inhibition of IKKβ has been observed to reduce food intake and increase insulin sensitivity (Zhang et al., 2008; Ozcan et al., 2009; Posey et al., 2009). Moreover, structural changes to the hypothalamus and evidence of increased inflammation have been observed well before the onset of obesity (Zhang et al., 2008; Thaler et al., 2012). Free fatty acids may also activate these pathways as well as activate macrophages by binding to toll-like receptors (TLRs) on their cell surface (Hotamisligil, 2006). High fat feeding, selective intake of saturated fatty acids and ICV infusion of palmitate have been associated with hypothalamic inflammation independently of excess caloric intake (Milanski et al., 2009; Posey et al., 2009). Finally, switching the diet to more unsaturated fats was observed to reduce this inflammation as well as hypothalamic and systemic insulin resistance (Cintra et al., 2012).
The role of inflammation in obesity is still disputed and may be multi-faceted, as is demonstrated by the positive effects of the cytokines IL-6 and IL-10 on energy and glucose metabolism, which seem to be partly mediated by central mechanisms (Wallenius et al., 2002; Benrick et al., 2009; Gotoh et al., 2012; Timper et al., 2017). The expression and circulating levels of these cytokines are increased in obese humans (Kern et al., 2001; Vozarova et al., 2001; Esposito et al., 2003). IL-6 administered to mice intracerebroventricularly was recently shown to induce reduced food intake and improved glucose metabolism, the latter mediated by enhanced hepatic insulin action. Moreover, this was more marked in obese, leptin-resistant mice than in lean ones (Timper et al., 2017). Thus, the increased levels of these cytokines may actually counteract rather than add to the other detrimental metabolic effects of obesity. Of further interest, IL-6 secretion by myocytes increases considerably after physical exercise and leads to subsequent increase in circulating levels of IL-10, secreted by immune cells (Steensberg et al., 2003; Pedersen and Febbraio, 2008). IL-6 and IL-10 have been shown to mediate positive metabolic effects of exercise through inhibition of IKKβ and ER stress in the hypothalamus (Ropelle et al., 2010).
In order to exert its proposed influence on peripheral glucose metabolism, the CNS input from nutrients, hormones and other neurons has to result in an efferent response that is conveyed to peripheral target organs. The known routes for this response is the autonomic nervous system (ANS) – further divided into the sympathetic nervous system (SNS) and the parasympathetic nervous system (PASY) – and the hypothalamic-pituitary axis (Figure 2).
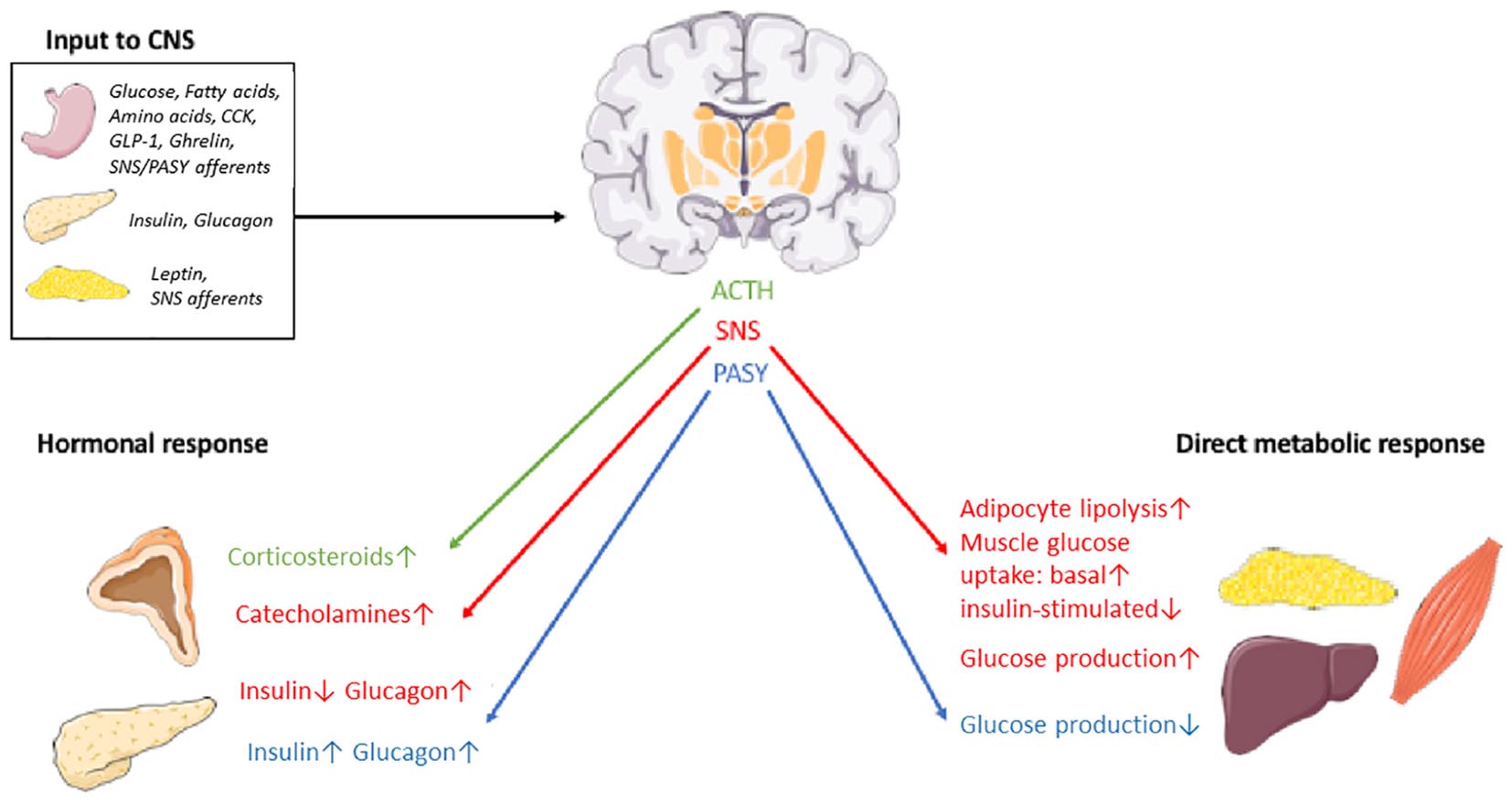
Figure 2. Schematic overview of some organs, nutrients, hormones and effector pathways involved in the postulated CNS-coordinated glucose regulation. SNS, sympathetic nervous system. PASY, parasympathetic nervous system. The illustrations of anatomical structures were retrieved from http://smart.servier.com and have thereafter been assembled and processed.
The DMNX of the brainstem contains the soma of preganglionic parasympathetic nerve fibers and can thus transmit PASY signals to target organs like the liver (Yi et al., 2010) (reducing hepatic glucose production and increasing glycogen synthesis) and the pancreas (Thorens, 2014) (increasing insulin secretion and glucagon secretion). Neurons in the RVLM connect with the SNS to increase hepatic glucose production (HGP) (Yi et al., 2010), decrease insulin secretion and increase glucagon secretion (Thorens, 2014), promote glucose uptake in skeletal muscles (Seoane-Collazo et al., 2015) and lipolysis in adipocytes (Bartness et al., 2005). Neurons in the PVN engage in the hypothalamic-pituitary-adrenal axis by secreting corticotrophin releasing hormone (CRH) in the bloodstream for downstream release of ACTH in the pituitary and ultimately secretion of glucocorticoids from the adrenal cortex. TRH, somatostatin, vasopressin and oxytocin is also synthesized in the PVN and may also contribute to long-term regulation of energy and glucose metabolism (Roh et al., 2016; López-Gambero et al., 2019). Finally, growth hormone (GH) release from the pituitary is stimulated by growth hormone-releasing hormone (GHRH) and inhibited by somatostatin, both released from the hypothalamus. The increase in GH levels observed during hypoglycemia seem to be dependent on alpha-adrenergic dependent somatostatin inhibition and GHRH stimulation (Lim and Khoo, 2000). GH raises glucose levels in the periphery by essentially counteracting insulin. This effect is, however, opposed by the insulin-like action of IGF-1, release of which from the liver is stimulated by GH (Clemmons, 2012). Nevertheless, type 2 diabetes is a distinct clinical consequence of GH excess in acromegaly (Hannon et al., 2017), attesting to a net hyperglycemic effect of the growth hormone axis. A subset of neurons in the ARC express GHRH and seem to be involved in the regulation of GH secretion in a complex interplay with POMC-, NPY- and somatostatin-expressing neurons (Steyn et al., 2016). Indeed, POMC and NPY/AgRP neurons are implicated in CNS-mediated glucose and energy regulation, acting in opposite directions (Tups et al., 2017). Thus, the hypothalamus-pituitary-growth hormone axis may provide an additional effector pathway from the brain to the periphery that influences systemic glucose homeostasis. By binding to the receptor GHSR on GHRH neurons, ghrelin may interfere with this pathway along with a direct effect on NPY/AgRP neurons (Steyn et al., 2016).
Evidence of CNS involvement in glucose homeostasis also comes from a study in which mice that moved from a warm to a cold environment had a coordinated reduction in both insulin secretion and insulin sensitivity, despite the absence of hypoglycemia. This was reversed within 4 h of returning to room temperature and within 30 min of administration of the alpha-adrenergic blocker phentolamine. According to the authors, the results imply a key role of the brain in rapidly coordinating insulin secretion and insulin sensitivity via the SNS (Morton et al., 2017). The control of body temperature is coordinated in the hypothalamus (Morrison, 2016) and crosstalk between this neuronal circuitry and those that are supposedly involved in glucose regulation would not be surprising.
Evidence From Studies in Humans
Clinical Observations in CNS and Metabolic Disorders
There is an intriguing epidemiological correlation between features of the metabolic syndrome and various diseases affecting the CNS of humans that gives indirect support to the brain’s involvement in peripheral glucose regulation. Depression is twice as common in patients with type 2 diabetes (Moulton et al., 2015). A correlation between depression and insulin resistance that was attenuated when adjusting for bodyweight and other confounders was reported in a meta-analysis from 2013 (Kan et al., 2013). In a longitudinal study, depression in middle-aged women was associated with increased insulin resistance and increased incidence of diabetes type 2, both of which were explained by increased central adiposity (Everson-Rose et al., 2004). Other longitudinal studies have highlighted an increased risk in patients with depression to develop the metabolic syndrome and/or its components (Marazziti et al., 2014).
An increased prevalence of the metabolic syndrome has also been reported repeatedly in studies of patients with psychotic illness including schizophrenia (Newcomer, 2007). While this may be partly due to the well-known side effects of antipsychotics, a meta-analysis from 2016 reported that first-episode psychosis was associated with increased insulin resistance and impaired glucose tolerance (Perry et al., 2016). Similar associations have been observed for anxiety and bipolar disorder (Czepielewski et al., 2013; Smith et al., 2013) while studies addressing the connection between work-related stress and development of diabetes type 2 have been inconsistent (Cosgrove et al., 2012; Krajnak, 2014). Patients with type 2 diabetes also have an increased risk of developing dementia, particularly vascular dementia and Alzheimer’s disease and it has been hypothesized that insulin resistance in the brain contributes to the development of Alzheimer’s disease (Li et al., 2015; Chatterjee and Mudher, 2018).
Naturally, epidemiological observations such as these do not prove causation, still leaving us with the question: does CNS diseases cause metabolic disorders or vice versa? In some cases, there is probably a bidirectional causality, e.g., for Alzheimer’s disease and type 2 diabetes (Shinohara and Sato, 2017). However, one plausible causal mechanism is activation of the stress system (primarily the ANS and the hypothalamic-pituitary-adrenal axis) in psychiatric and neurodegenerative diseases leading to metabolic consequences down the line. As outlined previously, these two systems constitute efferent pathways by which the brain may exert its putative influence on whole-body glucose homeostasis. Over-activation of either or both of these systems have been postulated to contribute to the development of metabolic disorders.
Disturbances of the Autonomic Nervous System in CNS and Metabolic Disorders
As previously described, the ANS consists of the SNS and the PASY. It has a range of biologic effects including influencing glucose homeostasis in the periphery. Activation of the SNS raises circulating glucose levels by inhibiting insulin release from beta cells, stimulating release of glucagon from alpha cells, increasing release of epinephrine from the adrenal glands (augmenting and prolonging the effect of nervous activity) and increasing hepatic glucose production. In contrast, PASY activation lowers glucose levels by stimulating the secretion of insulin from beta cells and suppressing hepatic glucose production, while similarly increasing glucagon levels (Roh et al., 2016). While intravenous use of adrenalin more or less replicates the effects of SNS activity, blocking the cholinergic receptors of the PASY with atropin was reported to induce an unexpected rise in insulin sensitivity in one study (Svensson et al., 2011). This is possibly explained by differential effects of nicotinic and muscarinic receptors on peripheral insulin sensitivity. By and large though, chronic over-activation of SNS and/or under-activation of PASY would in theory raise blood glucose levels and thereby possibly contribute to the development of type 2 diabetes and other metabolic disorders.
Studies employing biochemical measurements of catecholamines as markers of SNS activity in subjects with insulin resistance, obesity and the metabolic syndrome have been inconsistent (Kjeldsen et al., 1983; Troisi et al., 1991; Tuck et al., 1992; Young et al., 1992; Tataranni et al., 1997; Lee et al., 2001; De Pergola et al., 2008; Surwit et al., 2010; Dai et al., 2012; Reimann et al., 2017). Those using measurement of sympathetic reactivity as reflected by levels of catecholamines in serum or urine after provocation by mental stress, cold stress or food intake have found some correlation to obesity (Schwartz et al., 1987), future development of obesity (Flaa et al., 2008b) and insulin resistance (Flaa et al., 2008a).
By using microneurography, muscle sympathetic nerve activity (MSNA) can be measured as pulse synchronous nerve bursts in resting muscles and constitute a surrogate measure for sympathetic activity in the whole body (Parati and Esler, 2012). Some studies using this method have demonstrated increased SNS in subjects with the metabolic syndrome compared to those without (Huggett et al., 2004; Grassi et al., 2009).
Heart rate variability can be computed by analyzing long-term or short-term ECG monitoring and used as a marker of sympathetic and parasympathetic activity and to reflect the balance of the two. The PASY and the SNS activity influence the heart rate variability in partially differential frequency bands. PASY activity is affected by the respiratory cycle and is detectable up to 1 Hz while SNS activity is largely dependent on input from baroreceptors, mediating a slower response up to 0.15 Hz. Therefore, the high frequency (HF) band of 0,15–0,40 Hz corresponds well to PASY activity while the low frequency (LF) parameter of 0.04–0.15 Hz is influenced by both SNS and PASY activity (Malik et al., 1996). There are several other indices of HRV (Kleiger et al., 2005). The ratio of LF/HF has been used to represent balance between SNS and PASY activity, although this has been criticized as being somewhat simplistic (Perini and Veicsteinas, 2003). Moreover, it has been pointed out that increased heart rate as such contributes to unfavorable HRV profiles, and that this must be taken into consideration when interpreting results from HRV studies (Monfredi et al., 2014). Henceforth, the term “low HRV,” although somewhat imprecise, will be used to describe an HRV profile indicative of relative over-activation of the SNS.
Several studies have shown a lower HRV in subjects with T2DM but whether HRV is a consequence of glycemic dysregulation or a causative mechanism behind the development of diabetes type 2 is not clear (Benichou et al., 2018). One study reported that reduced variability in the LF range was associated with increased risk of developing type 2 diabetes (Carnethon et al., 2003) while another study only found a correlation between baseline heart rate (and no other HRV indices) and the incidence of type 2 diabetes (Carnethon et al., 2006).
It is generally accepted that increasing insulin resistance precedes the development of overt type 2 diabetes. First degree relatives to patients with type 2 diabetes have a markedly increased risk of developing type 2 diabetes and are more insulin resistant than subjects with no family history of type 2 diabetes (Eriksson et al., 1989; Harrison et al., 2003). One study aimed at studying the association between insulin resistance and HRV in first-degree relatives of patients with diabetes type 2 compared with controls. While discovering no differences in insulin resistance in the groups, BMI and the LF/HF ratio were significantly and independently associated with insulin resistance (Svensson et al., 2016). Several other studies have found a correlation between measures of insulin resistance, glycemic status and HRV (Reims et al., 2004; Perciaccante et al., 2006; Stein et al., 2007; Thiyagarajan et al., 2012; Charles et al., 2013; Jarczok et al., 2013; Li et al., 2013; Cherkas et al., 2015; Indumathy et al., 2015; Meyer et al., 2016). In other studies, no correlation has been observed (Rannelli et al., 2017) or a differential correlation between men and women has been reported (Flanagan et al., 1999; Rannelli et al., 2017).
Multiple studies have also found an association between obesity and impaired HRV. BMI in itself has been significantly related to HRV in some studies (Molfino et al., 2009; Alrefaie, 2014; Hillebrand et al., 2015; Indumathy et al., 2015; Johncy et al., 2015) but not in others (Antelmi et al., 2004). Some studies suggest a stronger correlation between HRV and other anthropometric indices such as visceral adipose tissue, waist-hip ratio, waist circumference and body fat percentage: arguably markers that better reflect metabolically detrimental adiposity (Lindmark et al., 2005; Kimura et al., 2006; Sztajzel et al., 2009; Poliakova et al., 2012; Windham et al., 2012; Yi et al., 2013; Chintala et al., 2015; Indumathy et al., 2015; Yoo et al., 2016; Rastović et al., 2017; Yadav et al., 2017). Several studies have shown an improvement of HRV following weight-loss after surgery or by caloric restriction (Karason et al., 1999; Akehi et al., 2001; Ito et al., 2001; Nault et al., 2007; de Jonge et al., 2010; Sjoberg et al., 2011; Lips et al., 2013; Maser et al., 2013; Pontiroli et al., 2013; Casellini et al., 2016).
In a systematic review from 2014 on the correlation between HRV and the metabolic syndrome, the authors concluded that heart rate variability was generally reduced in females with the metabolic syndrome compared to those without while results in men were inconsistent (Stuckey et al., 2014). Since then, some studies have demonstrated a correlation between the metabolic syndrome and indices of low HRV (Ma et al., 2017; Carvalho et al., 2018) and one study has supported the differential correlations with regards to sex mentioned above (Stuckey et al., 2015). However, some studies suggest that the link between the metabolic syndrome and autonomic dysregulation as measured by HRV is fully explained by insulin resistance (Balcioğlu et al., 2016; Saito et al., 2017) and while low HRV was reported to predict the development of the metabolic syndrome in one study (Wulsin et al., 2016), another study demonstrated that this correlation was only significant for development of hyperglycemia and high blood pressure, whereas the risk of developing obesity and dyslipidemia were not significantly correlated to low HRV (Wulsin et al., 2015).
There is some support of ANS disturbances in various CNS disorders. Low employment status and high psychosocial stress have been associated with low HRV (Hemingway et al., 2005; Brosschot et al., 2007). An impaired HRV has also been reported in anxiety disorder (Chalmers et al., 2014), bipolar disorder (Faurholt-Jepsen et al., 2017), depression (Kemp et al., 2010), schizophrenia (Montaquila et al., 2015), and dementia (da Silva et al., 2018) although it has been suggested that this correlation may be confounded by the effects of medication (Sgoifo et al., 2015). Evidence of ANS and HPA dysregulation in CNS and metabolic disorders are summarized in Table 1.
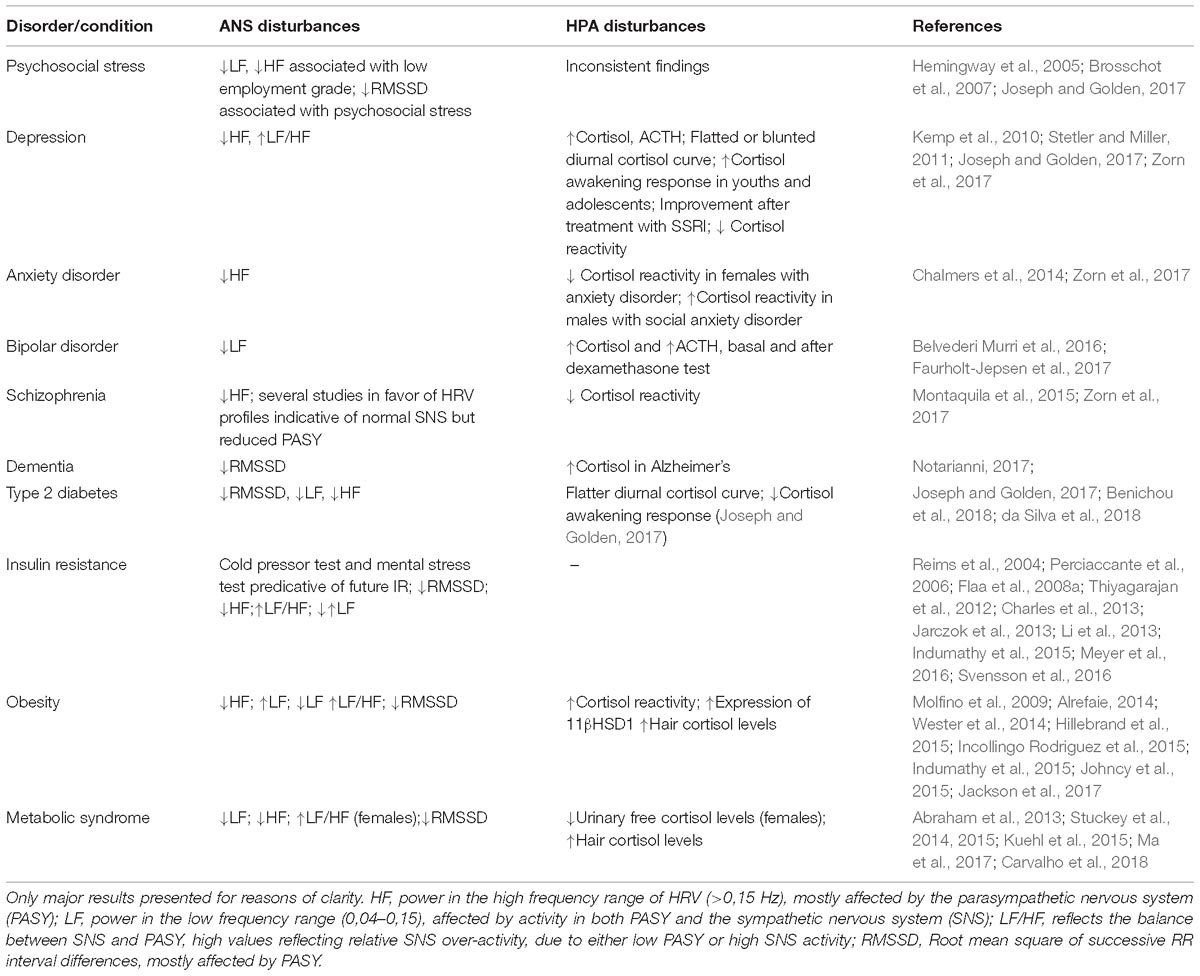
Table 1. Summary of documented disturbances of the autonomic nervous system and the hypothalamus-pituitary-adrenal axis associated with CNS and metabolic disorders.
The well-documented propensity of beta-blockers to cause insulin resistance and impair glycemic control in patients with diabetes (Lithell, 1991) and the fact that physical exercise improves insulin sensitivity in spite of increased SNS activity (Motahari-Tabari et al., 2014; Fisher et al., 2015) attest to the complicated relation between ANS disturbances and metabolic disorders. A troublesome aspect of studies utilizing measurement of HRV as methods is the plethora of indices available and the inconsistent manner in which these are used in different studies. This complicates comparison and somewhat limits the validity of the combined results.
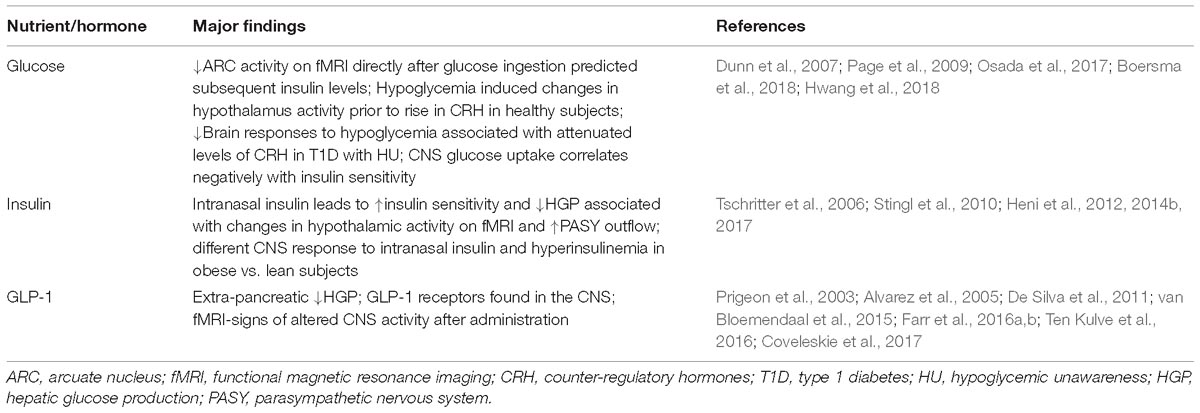
Table 2. Summary of some important studies on CNS effects of nutrients and hormones on systemic glucose metabolism in humans.
Disturbances of the Hypothalamus-Pituitary-Adrenal Axis in CNS and Metabolic Disorders
An excess of glucocorticoids, such as seen in Cushing’s disease or pharmacological glucocorticoid treatment, typically leads to insulin resistance and obesity as well as other features of the metabolic syndrome (Liu et al., 2014; Pivonello et al., 2016). This raises the question if elevated levels of glucocorticoids are also a feature in the “normal” pathophysiology of metabolic diseases. Glucocorticoids are produced and secreted by the adrenal cortex. This is regulated by the hypothalamus-pituitary-adrenal (HPA) axis in which hypothalamic release of CRH stimulates pituitary release of ACTH. This in turn stimulates glucocorticoid production and secretion. Feedback mechanisms occur at several levels. In the periphery, particularly in the liver and in adipose tissue, 11βHSD1 converts inactive cortisone to active cortisol whereas 11βHSD2 inactivates cortisol in a reverse manner, collectively providing an additional regulatory mechanism of glucocorticoid activity.
Circulating glucocorticoids act in several ways to disrupt normal glucose homeostasis. Glucocorticoids may cause beta cell dysfunction by influencing the uptake and metabolism of glucose in beta cells and may also attenuate the effects of GLP-1. In the periphery, glucocorticoids counteract insulin action by inducing insulin resistance through reduction of insulin downstream signaling substrates as well as indirectly by increasing lipolysis and proteolysis. Glucocorticoids also increase hepatic glucose production by activating enzymes involved in gluconeogenesis. Moreover, glucocorticoids also cause obesity and consequently insulin resistance by promoting differentiation and proliferation of adipocytes. Since glucocorticoid receptors are more abundant on visceral adipocytes, this favors visceral obesity (Di Dalmazi et al., 2012). Finally, excess glucocorticoids may lead to general weight gain (Berthon et al., 2014), probably at least partially by stimulating appetite through actions in the CNS, as is supported by a recent study (Serfling et al., 2019).
There is a multitude of ways to assess activity in the HPA-axis. These include plasma levels of cortisol, salivary levels of cortisol, 24 h urinary excretion of cortisol (UFC) and hair cortisol concentration (HCC). Activity in the HPA axis is highly variable and influenced by a range of factors including circadian rhythmicity, exercise, stress, and food-intake. Therefore, not only absolute concentrations of but also the diurnal curve of levels and dynamic changes in cortisol levels, as measured by cortisol reactivity to stress and dexamethasone suppression test, are interesting markers.
A few reviews on the topic have been published (Abraham et al., 2013; Incollingo Rodriguez et al., 2015) and will not be covered in detail here. From these and from original studies we can conclude that connections between obesity or the metabolic syndrome and basal levels of cortisol as measured in plasma, saliva, or urine are either inconsistent or disparate with regards to sex (Abraham et al., 2013; Incollingo Rodriguez et al., 2015). HCC is arguably a more reliable indicator of long-term levels of cortisol (Russell et al., 2015), in analogy with HbA1c, and studies using this outcome are more consistently in favor of such connections (Wester et al., 2014; Kuehl et al., 2015; Jackson et al., 2017). Moreover, consistently elevated cortisol reactivity tests in obese subjects as well as tendencies toward a flatter cortisol curve during day-time in obese subjects and toward impaired dexamethasone suppression tests in subjects with abdominal obesity was reported in one systematic review (Incollingo Rodriguez et al., 2015). Studies of HPA dysregulation in CNS disorder show variable results that are summarized in Table 1 (Stetler and Miller, 2011; Belvederi Murri et al., 2016; Joseph and Golden, 2017; Notarianni, 2017; Zorn et al., 2017).
In summary, long-term elevations, disturbances in the diurnal pattern, abnormal reactivity to stress and impaired feedback regulation of cortisol may have a small but limited impact on the development of obesity and metabolic disorder. Finally, there is substantial evidence that the expression of 11βHSD1 is upregulated in the adipose tissue of obese humans and animals. Thus, local dysregulation of glucocorticoids just as HPA dysregulation may contribute to the development of obesity and the metabolic syndrome (Incollingo Rodriguez et al., 2015).
BOX 1 | Neuroimaging techniques.
Magnetoencephalography (MEG)
This technique measures magnetic fields produced by electrical currents in the brain just as EEG measures electric fields. It is limited by the fact that cortical but not subcortical regions (including the hypothalamus) can be evaluated and has been largely replaced by other techniques to study the impact of metabolic input on brain activity.
Functional Magnetic Resonance Imaging (fMRI)
Blood oxygen level dependent (BOLD) signal is based on the fact that deoxygenated hemoglobin is paramagnetic whereas oxygenated hemoglobin is not, resulting in a higher signal in T2 weighted MRIs in situations with increased blood flow. Cerebral blood flow can also be measured by arterial spin labeling (ASL) where the water of incoming blood in for instance carotid arteries are labeled magnetically which changes the T1 signaling of water in the region that is examined.
The resolution in time and space is better than PET. Neuronal activity is not measured directly but via cerebral blood flow as a surrogate marker. By measuring spontaneous fluctuations in resting state brain activity, functional connectivity can be assessed and utilized to divide the brain into small functional units.
Positron Emission Tomography (PET)
This method measures gamma-rays that are emitted by unstable isotopes. Molecules that are labeled with positron-emitting isotopes are injected into the circulation. Fludeoxyglucose (18F) or FDG is widely used as a measure of glucose-uptake into tissues including the brain. To allow for neuroanatomic correlation of the signals, the method is combined with either CT or MRI and thus the space resolution does not differ from fMRI. However, the half-life of FDG is 110 min and it takes considerable time to generate a strong enough signal for the analysis. Hence, the time resolution is markedly limited compared to other methods.
Single-Photon Emission Computed Tomography (SPECT)
Similar to PET, this technique utilizes radioactive tracer material, but these emit gamma rays directly instead of positrons, limiting the spatial resolution as compared to PET.
Central Effects of Glucose and Insulin on Systemic Glucose Metabolism: What Have We Learned From Neuroimaging Studies of Humans?
The methods employed in animal research previously discussed in this review are for obvious reasons not directly transferable to studies involving human subjects. The development and refinement of neuroimaging techniques has, however, offered an indirect way to explore changes in brain activity induced by and associated with metabolic changes. The neuroimaging techniques at hand (described in Box 1) have been extensively used to study CNS control of feeding and appetite which has been reviewed comprehensively elsewhere (De Silva et al., 2012; Zanchi et al., 2017). While PET is still used to a limited degree, fMRI has largely replaced other techniques as the method of choice for investigative purposes. Studies may use imaging of the whole brain or, more commonly, specific regions of interest (ROI) such as the hypothalamus. The small size and the location of the hypothalamus makes it difficult to image. In early studies, the hypothalamus was divided by a mid-sagittal slice resulting in the upper anterior, upper posterior, lower anterior, and lower posterior hypothalamus (Matsuda et al., 1999; Smeets et al., 2005). This division is too rough to allow for differential analysis of individual nuclei. However, a recent study utilized areal parcellation of the hypothalamus with a resolution of 1.25 mm × 1.25 mm and resting-state functional connectivity to generate 10 focii corresponding to different nuclei based on a previous histological study, demonstrating that such analysis of individual nuclei is indeed feasible (Osada et al., 2017).
It is not within the scope of this review to fully discuss the findings from the numerous studies aimed at exploring functional changes on brain activity as related to appetite or feeding control. However, a brief summary is warranted given the intimate relationship between energy intake and glucose regulation. A decrease in neural activity following glucose and meal intake has been reported in the hypothalamus and elsewhere (Matsuda et al., 1999; Tataranni et al., 1999; Gautier et al., 2000; Liu et al., 2000; Smeets et al., 2005, 2007; Flanagan et al., 2012; Page et al., 2013) that differs in obesity and type 2 diabetes according to some studies (Matsuda et al., 1999; Gautier et al., 2000; Vidarsdottir et al., 2007). Moreover, studies employing task-based fMRI protocols have demonstrated activation of several CNS regions (in particular the orbitofrontal cortex, amygdala, hippocampus and insula) upon presenting the subject with visual food cues (LaBar et al., 2001; Killgore et al., 2003; St-Onge et al., 2005; Porubská et al., 2006; Führer et al., 2008) which was attenuated in the fed state in some studies (Baldo and Kelley, 2007; De Silva et al., 2011) and different in obesity or insulin resistance in others (Killgore and Yurgelun-Todd, 2005; Rothemund et al., 2007; Stoeckel et al., 2008; Martin et al., 2010; Wallner-Liebmann et al., 2010; Dimitropoulos et al., 2012; Heni et al., 2014a; Alsaadi and Van Vugt, 2015). Caloric restriction normalized the hypothalamic response to glucose challenge in patients with type 2 diabetes in one study (Teeuwisse et al., 2012). Finally, appetite and feeding patterns in the CNS have been examined with respect to pharmacological administration and circulating levels of implicated hormones (Zanchi et al., 2017).
Collectively it seems that there are relatively well-characterized brain responses that are triggered by ingested and circulating levels of nutrients and hormones. Whether these brain responses have an actual effect on peripheral glucose metabolism has not, however, been investigated to a similar extent. The existence of the cephalic phase of insulin secretion as well as the observation that visual food-cues leads to lower postprandial glucose levels without affecting food-intake, insulin levels or other neuroendocrine parameters (Brede et al., 2017) is suggestive of such a response, the exact effector mechanisms of which remains elusive.
In the previously discussed study by Osada et al. (2017), 12 healthy subjects, both male and female, underwent fMRIs on two separates days following ingestion of 75 g of glucose solution or an equal volume of water. The hypothalamus was the region of interest and the imaging was analyzed by area parcellation, enabling analysis of individual nuclei. Thereby, the authors could report that the decrease in ARC activity between 0 and 10 min after the glucose ingestion significantly predicted insulin levels at 10 min. Thus, a causative link between the two events was plausible, according to the authors.
Hypoglycemia, on the other hand, was shown to induce changes in hypothalamic activity prior to the rise in counter-regulatory hormones (glucagon, cortisol, adrenaline, or noradrenaline) in healthy subjects (Page et al., 2009). The effect of hypoglycemia on CNS activation has been intensively studied in the setting of type 1 diabetes with unawareness. These patients have been shown to have a blunted rise in cerebral blood flow in hypoglycemia (Dunn et al., 2007; Mangia et al., 2012; Hwang et al., 2018) associated with a corresponding attenuation of counter-regulatory hormonal response (Mangia et al., 2012; Hwang et al., 2018).
While the intracerebroventricular administration of nutrients and hormones performed in animal studies is not an option in studies of humans, intranasal treatment of insulin preferentially targets the CNS, and thus provides an alternative for studying CNS effects isolated from systemic effects (Born et al., 2002). Intriguingly, intranasal insulin treatment has been demonstrated to lead to weight loss (Hallschmid et al., 2004), suppressed lipolysis (Iwen et al., 2014), positive cognitive results (Benedict et al., 2004; Hallschmid et al., 2008) and rapid reduction of glucose levels without any impact on C-peptide or insulin levels (Hallschmid et al., 2012). Heni et al. (2012, 2014b, 2017) have investigated the mechanisms behind this in a series of experiments where they showed an improvement of insulin sensitivity that was correlated with changes in hypothalamic blood flow on fMRI. An increase of HRV in the HF band was seen after intranasal insulin in one study, suggestive of augmented PASY outflow activity as a possible mediator between the CNS and the periphery (Heni et al., 2014b). In conflict with these studies, Ott et al. (2015) found no central effect of intranasal insulin aspart that could be discerned from its spillover effect in the periphery. Heni et al. (2018) recently reported yet unpublished results showing increased C-peptide levels 10 min after intranasal insulin administration during a hyperglycemic clamp in subjects with hypothalamic insulin sensitivity (as evidenced by a decrease of hypothalamic blood flow following administration) compared to those without. No reduction of C-peptide levels occurred in either group after administration, speaking against a substantial spill-over effect of intranasal insulin in this experiment (Heni et al., 2018).
Dash et al. (2015a) reported an inhibition of HPG 180 min after treatment with intranasal insulin, much later than what was seen in the studies by Heni et al. (2012, 2014b, 2017, 2018) According to the authors, this delayed response could be explained by a CNS-mediated effect on gene expression of proteins involved in hepatic gluconeogenesis rather than a more direct effect (Dash et al., 2015b). Similar kinetics of central insulin effects have been reported in dogs (Ramnanan et al., 2011) and rodents (Obici et al., 2002c). Moreover, the potent KATP channel opener diazoxide induced a 30% reduction of HGP after 6 h in humans in the settings of a pancreatic clamp, excluding the influence of pancreatic hormones and suggesting extra-pancreatic effects (Kishore et al., 2011). As previously discussed, intracellular insulin signaling in the hypothalamus is dependent on functional KATP-channels and the brain may therefore be the extra-pancreatic site where diazoxide exerts its antidiabetic effects. In rats, ICV administration of diazoxide did indeed cause a reduction of HGP explained by downregulation of genes involved in gluconeogenesis (Kishore et al., 2011). Finally, human subjects with type 2 diabetes compared to controls did not exhibit suppression of HGP following diazoxide treatment in another study (Esterson et al., 2016), perhaps because of resistance to its extra-pancreatic effects.
There are several other findings suggesting the existence of CNS insulin resistance. A different response to intranasal insulin in obese vs. lean subjects has been reported in many studies (Hallschmid et al., 2004; Tschritter et al., 2006; Stingl et al., 2010; Heni et al., 2014b, 2017). In a recent study by our research team, whole-body 18FDG-PET was performed on patients with varying degrees of insulin sensitivity during a hyperinsulinemic-euglycemic clamp. Interestingly the M-value, an index of insulin sensitivity, correlated positively with uptake in muscles, adipose tissue and the liver and correlated negatively with glucose uptake in the brain (Boersma et al., 2018). This is corroborated by yet unpublished results by Rebelos et al. (2018). In their study of 18FDG-PET examinations in 151 subjects, the glucose uptake in the brain was correlated positively with BMI and negatively with insulin sensitivity. Of further interest, glucose uptake in the brain correlated positively with MRI signs of inflammation in the hypothalamus and amygdala (Rebelos et al., 2018), which is in consistency with another study (Thaler et al., 2012). Thus, hypothalamic inflammation may exist in obese humans in accordance with previously discussed findings in animal studies and may cause hypothalamic insulin resistance by the same proposed cellular mechanisms. Finally, Hirvonen et al. (2011) reported that brain glucose metabolism as assessed by 18FDG-PET increased in subjects with impaired glucose tolerance during a hyperinsulinemic-euglycemic clamp but not in healthy subjects, in whom the effect of insulin on brain glucose metabolism appeared to be saturated at fasting levels of insulin. Although this may suggest that insulin resistant subjects need more insulin to have a maximal effect on brain glucose metabolism, the fact that there was no difference in brain glucose metabolism at baseline between the two groups argues against the concept of such an insulin resistance in the CNS as a feature of systemic insulin resistance (Hirvonen et al., 2011).
Aside from the potential role of hypothalamic inflammation, the observation that the CSF:plasma ratio of insulin is inversely related to BMI (Kern et al., 2006) supports deficient transport of insulin as a cause for the putative hypothalamic insulin resistance. Table 2 summarizes some important studies of CNS effects of nutrients and hormones on systemic glucose metabolism in humans.
Central Effects of Other Hormones, Bariatric Surgery and Pharmacological Agents on Systemic Glucose Metabolism
Leptin seems to regulate energy homeostasis in humans by central mechanisms (Rosenbaum et al., 2008; Grosshans et al., 2012) and to have an impact on whole-body glucose regulation in humans, as witnessed by its antidiabetic effects on patients with congenital leptin deficiency, congenital lipodystrophy, HIV-associated lipodystrophy and uncontrolled diabetes type 1 (Moon et al., 2013; Ramos-Lobo and Donato, 2017). However, it is not clear to which extent glucose regulation is carried out by peripheral vs. central mechanisms and no large effects have been demonstrated on the ANS (Eikelis et al., 2003; Machleidt et al., 2013) or the HPA-axis (Licinio et al., 1997; Sienkiewicz et al., 2011; Khan et al., 2012) that would grant indirect support to a contribution of central mechanisms. Oxytocin has demonstrated positive effects on various indices of glucose metabolism in several studies when given as a nasal spray (Ott et al., 2013; Lawson et al., 2015; Thienel et al., 2016), whereas the evidence from intravenous use is inconsistent (Lawson, 2017). Whether this is due to different central vs. peripheral effects on glucose metabolism and the preferential targeting of CNS by intranasal administration has not been illuminated.
The dopaminergic system has also been suggested to influence glucose homeostasis. The dopamine agonist bromocriptine-QR has been associated with an absolute reduction of HbA1c of 0,69% and is approved by the FDA for treatment of type 2 diabetes in adults (Garber et al., 2013). There are several possible mechanisms, probably working in concert. Firstly, dopamine receptors (D2R) are expressed in beta cells of the pancreas and activation of these have been shown to inhibit insulin secretion and cause an anti-proliferative effect (Rubi et al., 2005; Ustione et al., 2013). How this would lead to an improvement of glycemic indices is, however, challenging to comprehend. Secondly, activation of D2R in the pituitary gland reduces secretion of prolactin (excess of which is associated with hyperphagia and lipid accumulation in adipose tissue) and growth hormone (Woodside, 2007; Ben-Jonathan and Hugo, 2015). Thirdly, D2R-receptors are ubiquitous in the CNS where dopamine agonists have been shown to suppress levels of noradrenalin and serotonin in the hypothalamus (Luo et al., 1998), possibly resulting in reduced outflowing activity of the SNS from the brainstem to the periphery down the line. The dopaminergic system and D2R is also implicated in reward and appetite regulation (Baik, 2013). Interestingly, impairment of the dopaminergic system is a feature of Parkinson’s disease as well as an effect of antipsychotic medication and may partly explain the association of metabolic disorders with these conditions (Lopez Vicchi et al., 2016).
The propensity of bariatric surgery to improve glycemic control in patients is well-recognized and may offer insights regarding the development and pathogenesis of type 2 diabetes. Superiority of surgery to pharmacological approaches has been consistently documented in a vast number of randomized controlled trials (Koliaki et al., 2017). According to guidelines endorsed by several international diabetes organizations, so-labeled metabolic surgery in the treatment of patients with type 2 diabetes and BMI > 30 is advocated when lifestyle and pharmacological approaches has proved insufficient (Rubino et al., 2017). While still a matter of some controversy, there is substantial evidence indicating that the improvement of glucose indices seen after surgery is not fully explained by weight loss alone. The existence of weight independent mechanisms is suggested by the observed rapid improvement of glucose metabolism, occurring only days after surgery (Pories et al., 1995). Moreover, rates of diabetes remission differ depending on surgical technique, those bypassing the foregut, such as Roux-en-Y gastric bypass and biliopancreatic diversion being more efficacious in this regard than those who simply restricts the volume of the ventricle, such as gastric banding and gastric sleeve gastrectomy (Kodama et al., 2018).
A number of neuroimaging studies have demonstrated a profound impact of bariatric surgery on structural and functional features of the CNS. Obesity is associated with radiological signs of brain volume reduction and two studies have demonstrated normalization of these signs after bariatric surgery (Tuulari et al., 2016; Zhang et al., 2016). The enhanced response in reward regions to visual food-cues before surgery (and consistently demonstrated in obesity) have been reported to resolve after surgery (Scholtz et al., 2014; Frank et al., 2016). Two studies have shown an increase in these responses post-surgery following administration of octreotide (Goldstone et al., 2016) (an inhibitor of pancreatic hormones) and a GLP-1 receptor antagonist (Ten Kulve et al., 2017), respectively, suggesting altered secretion of gut hormones as a mediator. The role of GLP-1 in mediating satiety via the CNS is further supported by other neuroimaging studies (De Silva et al., 2011; van Bloemendaal et al., 2015; Farr et al., 2016a,b; Ten Kulve et al., 2016; Coveleskie et al., 2017). GLP-1 receptors are expressed in the brainstem and hypothalamus of humans (Alvarez et al., 2005) and some animal studies suggest that the effects of GLP-1 on reduction of food intake are partly if not primarily mediated by CNS actions (Kanoski et al., 2011; Secher et al., 2014). An effect on glucose metabolism independent of food intake has also been observed in animals (Sandoval et al., 2008) and in humans where a suppression of HGP without any measurable impact on pancreatic hormone levels has been reported (Prigeon et al., 2003).
The postprandial secretion of GLP-1 is augmented after bariatric surgery (Hutch and Sandoval, 2017). This is also true for oxyntomodulin (Laferrère et al., 2010), another gut hormone that has demonstrably induced weight loss in humans and rodents by reducing food intake and increasing energy expenditure (Dakin et al., 2001; Cohen et al., 2003; Baggio et al., 2004; Wynne et al., 2005, 2006). It appears to have a dual mechanism, acting on both GLP-1 and glucagon receptors (GCR) (Pocai, 2012). In rodents, a superior effect on weight loss was observed compared to GLP-1RA treatment alone at similar antihyperglycemic effects (Kosinski et al., 2012) making combined targeting of GLP1R and GCR attractive targets for future treatment of obesity as well as type 2 diabetes. Indeed, the combined agonist SAR425899 was recently evaluated in a phase 2 trial, reporting a significant weight loss and HbA1c reduction compared to placebo (Tillner et al., 2019). The concept of targeting glucagon receptors in the treatment of type 2 diabetes might be challenging to accept at first. Hyperglycemia or attenuation of the hypoglycemic effects of GLP-1R action would be a warranted concern. However, the improvement of glycemic indices reported in the study implies that the GLP-1R mediated effects on glucose homeostasis overrides those mediated by GCR. Intriguingly, glucagon injected into the CNS of rodents unexpectedly lowers systemic glucose levels by inhibition of HGP (Mighiu et al., 2013; LaPierre et al., 2015). Furthermore, intravenous infusion of glucagon leads to a transient hyperglycemia in rats, and this transiency is abolished when simultaneously blocking hypothalamic glucagon signaling (Mighiu et al., 2013). Thus, glucagon may actually have delayed CNS-mediated hypoglycemic effects opposing the acute hyperglycemic peripheral effects. The net effect of this could furthermore contribute to rather than impede the observed glycemic improvement of SAR425899.
The dual GIP/GLP-1 receptor agonists LY3298176 have been developed and recently demonstrated superiority to single GLP-1 RA treatment in terms of HbA1c reduction and weight loss (Frias et al., 2018). While considerably less is known about the CNS effects of GIP compared to GLP-1 and glucagon, dual GIP/GLP-1 RA treatment has shown positive effects on animal models of Alzheimer’s disease and Parkinson’s disease (Hölscher, 2018).
Circulating bile acids also increases after bariatric surgery (Jahansouz et al., 2016) and appear to have positive metabolic effects on their own accord (Sinclair et al., 2018). Bile acids have been demonstrated to cross the blood–brain-barrier where the expression of one of its receptors GPBAR1 has been reported (Keitel et al., 2010). Binding to FXR, another receptor, leads to positive metabolic effects in several organs and increases expression of FGF 15/19 from the foregut (Pournaras et al., 2012). As described previously, members of the FGF family have been shown to exert long-lasting and pronounced antidiabetic effects that appears to be mediated largely by central mechanisms (Fu et al., 2004; Morton et al., 2013; Marcelin et al., 2014; Scarlett et al., 2016). The targeting of bile acid signaling and in particular FGF signaling thus constitute promising future strategies in the treatment of type 2 diabetes.
There are a handful of drugs approved for treatment of obesity that primarily target the CNS. Among these, both lorcaserin and topiramate-phentermine have shown positive effects on glycemic indices. Lorcaserin, a 5HT-2c agonist was found non-inferior to several glucose-lowering drugs in terms of HbA1c reduction (0.55% for lorcaserin) and proportion of patients reaching target HbA1c in a recent meta-analysis (Neff et al., 2017). The authors conclude that lorcaserin should be considered as a second line add-on treatment in patients with type 2 diabetes and BMI > 27. A recently published RCT reported a more moderate reduction of HbA1c of 0.33% for patients with type 2 diabetes compared to placebo (Bohula et al., 2018). The combination of the amphetamine-like drug phentermine and the antiepileptic drug topiramate approved for treatment of obesity has likewise demonstrated a reduction in HbA1c of 4.4 mmol/mol compared to placebo in one study (Garvey et al., 2014a) and reduced progression to diabetes in patients with prediabetes and the metabolic syndrome was reported in another study (Garvey et al., 2014b). Although the antidiabetic effects of these CNS acting antiobesity drugs are relatively small-scale and probably a consequence of weight loss, an independent effect on glucose metabolism cannot be excluded at this stage.
Recently, the growth differentiating factor 15 (GDF15) has emerged as a satiety signal and potential target for treating obesity and metabolic disorders. It is secreted by adipocytes and many other cell types in response to cell injury and inflammation (Tsai et al., 2016). GDF15 has been demonstrated to induce weight loss and reduce insulin resistance in mice and primates by binding to its receptor GFRAL, the expression of which has not been demonstrated anywhere but the AP and the NTS in the brainstem (Mullican et al., 2017). Peripheral administration of GDF15 in mice induced neuronal activity in the AP and NTS and the anorexic effects were reversed by ablation of these regions (Tsai et al., 2014). Thus, existing evidence from animal studies indicate the brainstem as the target organ for GDF15. In humans, increased circulating levels of GDF15 has been observed as soon as 1 week after bariatric surgery and levels correlate linearly with insulin sensitivity both before and after surgery (Kleinert et al., 2019). Additionally, mitochondrial stress in mouse skeletal muscle cells increases the expression of GDF15 (Chung et al., 2017) and increased circulating levels in humans after physical exercise has been reported (Kleinert et al., 2018). As previously discussed, IL-6 and IL-10 also increase after exercise in humans and have been shown to mediate positive metabolic effects via the CNS in animals (Pedersen and Febbraio, 2008; Ropelle et al., 2010).
Physical exercise has been associated with altered brain fMRI responses to food-cues, favoring low-calorie foods over high calorie-foods (Killgore et al., 2013; Crabtree et al., 2014). While other studies demonstrating changes in brain fMRI responses to exercise are abundant, these have primarily focused on brain regions and processes involved in memory and learning, that are implicated in the pathogenesis of neurodegenerative disorders and dementia. To our knowledge, there are no neuroimaging studies that address the possible link between functional brain alterations and the well-recognized positive metabolic effects associated with physical exercise.
Conclusion
Taken together, evidence from animal and human studies demonstrates that the brain detects levels of circulating nutrients and hormones and consequently organizes an outward response that contributes to the regulation of whole-body glucose homeostasis. However, there are major knowledge gaps about the exact nature of this response and its relative importance compared to peripheral processes. As we have seen, animal studies have provided an anatomical map of CNS glucose regulation and have identified important neurons and neural circuits involved. Additionally, the CNS sensing of key nutrients and hormones has been characterized in detail and the intracellular signaling pathways have been outlined for most of them.
Studies of humans entail inherent methodological challenges compared to animal studies that may explain the inconsistent findings in some areas. There are some intriguing epidemiological relationships between CNS and metabolic disorders and dysregulation of the ANS or HPA axis have both been proposed as the mediators of this connection. Attempts to demonstrate this have yielded somewhat discordant findings, however.
The extra-pancreatic effects of diazoxide and GLP-1 may very well be extra-cerebral as well and the favorable effect of intranasal oxytocin on glucose metabolism is interesting but not necessarily secondary to actions in the CNS. The use of somatostatin and octreotide in some studies to inhibit pancreatic hormone secretion may possibly induce CNS effects on their own accord and may thereby affect the results. The use of intranasal preparations of insulin arguably has a preferential CNS effect but dose-dependent spillover into the systemic circulation has been demonstrated in some studies that would make interpretation of results precarious.
Neuroimaging techniques have a large potential for further exploration of the interplay between CNS and systemic glucose regulation and have been used more extensively for investigation of appetite and feeding control with special regards to obesity. In these studies, resting-state or task-based neuroimaging is usually performed before and after some sort of metabolic intervention (i.e., OGTT or intranasal insulin) and the imaging is then compared for differences before vs. after the intervention and in between different subjects (i.e., lean vs. obese). Arguably, studies aimed at investigating acute CNS effects on glucose regulation must adopt a more sophisticated study design. Ideally, neuroimaging would be performed before vs. after some sort of metabolic intervention with concomitant measurements of hormonal activity and nutrients. If a detectable change in the activity of a brain region were to occur after the metabolic intervention but before a change in hormonal activity and nutrient levels, a causative link between the two events could be argued, in congruency with the results from Page et al. (2009) and Osada et al. (2017). A prerequisite for such a study design is that the utilized neuroimaging modality has a small enough time resolution to allow for such delicate temporal analysis, a description that fits fMRI but not PET. A matter of additional complexity is that the temporal relation between the CNS response to metabolic interventions and the putative peripheral effects is largely unknown and may differ between different stimuli. For instance, the response to hypoglycemia seems to be fast, occurring within 60 min in one study (Page et al., 2009), whereas the CNS-mediated insulin effects are not evident until after 180 min according to some studies (Obici et al., 2002c; Ramnanan et al., 2011; Dash et al., 2015a). Furthermore, the CNS response to shifts in nutrient availability and hormones may be conveyed to target organs by routes other than the ANS and the HPA-axis and alternative hormonal or neuronal pathways should at least be considered and evaluated in the future.
Other than in the work by Osada et al. (2017), the spatial resolution of fMRI protocols that were utilized in the reviewed studies have not permitted image analysis of separate nuclei of the hypothalamus but have either measured blood flow in the hypothalamus as a whole or in more crudely defined subdivisions of it. Since there is evidence that the relative proportion of GE and GI neurons varies in different nuclei (Mergenthaler et al., 2013), accurate subdivision of the hypothalamus may be imperative to produce reliable and relevant results. The mild inconsistencies between different neuroimaging studies is quite possibly a consequence of suboptimal resolution. This is best illustrated by the work of Heni et al. (2014b, 2017), in which one study examining the effect of intranasal insulin showed an increase of hypothalamic CBF whereas a decrease after administration was seen in another study. It is also important to underscore that the use of the surrogate markers CBF in fMRI and 18FDG uptake in PET may not correctly reflect changes in neuronal activity. The glucoCEST technique in MRI uses measurement of the proportion of phosphorylated and unphosphorylated 2-deoxy-D-glucose (2DG) and could thereby provide a better assessment of glucose metabolism than 18FDG-PET, while also embodying all the advantages with the fMRI modality. Likewise, MR spectroscopy provides a non-invasive method of measuring metabolite concentration in tissues and has for example been used to study hypothalamic glucose levels after hypoglycemic preconditioning (Seaquist et al., 2017). Nevertheless, neurons may exert either stimulatory or inhibitory downstream effects which are not discernable by methods limited to measurement of general neuronal activity, be that by proxy of cerebral blood flow or glucose metabolism.
In one study by Page et al. (2009), small reductions in systemic glucose levels induced increases in hypothalamic CBF before counter-regulatory hormones had risen. This raises the question: does this pattern differ in patients with glycemic dysregulation compared to normal subjects? The “brain-centric” model of development of type 2 diabetes stipulates that the hypothalamus regulates systemic glucose levels around a set-point that is skewed upwards as the disease progresses (Deem et al., 2017). Accordingly, glucose levels below this set-point would lead to processes aiming at raising glucose levels. Most likely, these processes would consist of the same counter-regulatory response that is mounted upon hypoglycemia in healthy individuals. It follows that the hypothalamic and counter-regulatory hormonal response would be activated at higher glucose levels in subjects with prediabetes compared to normal subjects. If this could be demonstrated in a hypoglycemic clamp during concomitant functional neuroimaging it would indeed lend strong support to the concepts of the “brain-centric” model. To our knowledge, this has not been demonstrated to date. Conversely, obese subjects exhibit different fMRI-responses to visual food cues during hyperglycemia than normal weight subjects according to one study (Belfort-DeAguiar et al., 2018). Whether the hypothalamic response to hyperglycemia differs in subjects with prediabetes compared to normal subjects and if such a possible difference corresponds to differences in hormonal activity remains to be investigated.
Since the brain is more or less dependent on glucose as a fuel substrate, its postulated ability to influence systemic glucose values or at least to correct hypoglycemia makes perfect sense from a teleological standpoint. As a consequence of the counter-regulatory response to hypoglycemia, systemic glucose levels are maintained at the expense of glucose uptake and utilization in insulin-sensitive organs such as muscles, adipose tissue and liver. Thus, the insulin-independent brain essentially prioritizes its own need for fuel over that of the rest of the body by mobilizing this counter-regulatory response. Expanding on this and on the “brain-centric” model, type 2 diabetes may be considered a condition in which the brain is “spoiled” by ever-increasing glucose levels and maintains those in a “selfish” fashion at the expense of the rest of the body.
In an elegant study published recently by Rodriguez-Diaz et al. (2018), pancreatic islets from three different species (humans, rhesus monkeys, and C57BL/6 mice) were transplanted to mice with streptozotocin-induced type 1 diabetes after which the glucose levels of the transplanted mice adapted to the normal ranges of the donors (varying considerably in between species). Thus, pancreatic islets were sufficient to regulate glucose levels on their own accord and this could not be overpowered by possible extra-pancreatic glucose regulation mechanisms. These findings indeed challenge the “brain-centric” model, but the transferability of these results from rodent models with experimental type 1 diabetes to humans with type 2 diabetes is a matter of reasonable doubt. Additionally, there may be brain-islet communication pathways that are species-specific and lost upon xenotransplantation.
Overall, there is robust evidence in favor of brain involvement in the regulation of glucose metabolism. Further investigations are needed to characterize the neurocircuits involved and the cross-talk with peripheral tissues. The use of refined neuroimaging methods is a promising way to test hypotheses derived from animal studies on human subjects. Such research will deepen our understanding of the pathogenesis of type 2 diabetes and potentially open up avenues for novel pharmacological approaches.
Author Contributions
ML did most of the literature search. ML and JE conceived and wrote the manuscript. All authors contributed to literature search, reviewed and approved the final version.
Funding
This review was funded by grants from the Swedish Diabetes Foundation, Ernfors foundation, ALF (Swedish Government Research Fund), and the European Union’s Horizon 2020 Research and Innovation Programme under the Marie Skłodowska-Curie grant agreement 721236-TREATMENT.
Conflict of Interest Statement
The authors declare that the research was conducted in the absence of any commercial or financial relationships that could be construed as a potential conflict of interest.
Acknowledgments
We are particularly grateful of the valuable scientific advice given by Professor Emeritus Anders Karlsson, Professor emeritus Elna-Marie Larsson, and Professor Johan Wickström at Uppsala University.
References
Abraham, M. A., and Lam, T. K. T. (2016). Glucagon action in the brain. Diabetologia 59, 1367–1371. doi: 10.1007/s00125-016-3950-3
Abraham, S. B., Rubino, D., Sinaii, N., Ramsey, S., and Nieman, L. K. (2013). Cortisol, obesity, and the metabolic syndrome: a cross-sectional study of obese subjects and review of the literature. Obesity 21, E105–E117. doi: 10.1002/oby.20083
Ahrén, B. (2000). Autonomic regulation of islet hormone secretion - Implications for health and disease. Diabetologia 43, 393–410. doi: 10.1007/s001250051322
Akehi, Y., Yoshimatsu, H., Kurokawa, M., Sakata, T., Eto, H., Ito, S., et al. (2001). VLCD-induced weight loss improves heart rate variability in moderately obese Japanese. Exp. Biol. Med. 226, 440–445. doi: 10.1177/153537020122600508
Alrefaie, Z. (2014). Brief assessment of supine heart rate variability in normal weight, overweight, and obese females. Ann. Noninvasive Electrocardiol. 19, 241–246. doi: 10.1111/anec.12120
Alsaadi, H. M., and Van Vugt, D. A. (2015). Insulin sensitivity affects corticolimbic brain responses to visual food cues in polycystic ovary syndrome patients. Horm Mol. Biol. Clin. Investig. 24, 101–115. doi: 10.1515/hmbci-2015-0048
Alvarez, E., Martínez, M. D., Roncero, I., Chowen, J. A., García-Cuartero, B., Gispert, J. D., et al. (2005). The expression of GLP-1 receptor mRNA and protein allows the effect of GLP-1 on glucose metabolism in the human hypothalamus and brainstem. J. Neurochem. 92, 798–806. doi: 10.1111/j.1471-4159.2004.02914.x
Anand, B. K., Chhina, G. S., Sharma, K. N., Dua, S., and Singh, B. (1964). Activity of single neurons in the hypothalamic feeding centers: effect of glucose. Am. J. Physiol. 207, 1146–1154. doi: 10.1152/ajplegacy.1964.207.5.1146
Antelmi, I., de Paula, R. S., Shinzato, A. R., Peres, C. A., Mansur, A. J., and Grupi, C. J. (2004). Influence of age, gender, body mass index, and functional capacity on heart rate variability in a cohort of subjects without heart disease. Am. J. Cardiol. 93, 381–385. doi: 10.1016/j.amjcard.2003.09.065
Arrieta-Cruz, I., Su, Y., Knight, C. M., Lam, T. K. T., and Gutiérrez-Juárez, R. (2013). Evidence for a role of proline and hypothalamic astrocytes in the regulation of glucose metabolism in rats. Diabetes 62, 1152–1158. doi: 10.2337/db12-0228
Ashley Blackshaw, L., and Young, R. L. (2011). Detection and signaling of glucose in the intestinal mucosa - Vagal pathway. Neurogastroenterol. Motil. 23, 591–594. doi: 10.1111/j.1365-2982.2011.01719.x
Asilmaz, E., Cohen, P., Miyazaki, M., Dobrzyn, P., Ueki, K., Fayzikhodjaeva, G., et al. (2004). Site and mechanism of leptin action in a rodent form of congenital lipodystrophy. J. Clin. Invest. 113, 414–424. doi: 10.1172/JCI200419511
Baggio, L. L., Huang, Q., Brown, T. J., and Drucker, D. J. (2004). Oxyntomodulin and glucagon-like peptide-1 differentially regulate murine food intake and energy expenditure. Gastroenterology 127, 546–558. doi: 10.1053/j.gastro.2004.04.063
Baik, J. H. (2013). Dopamine signaling in food addiction: role of dopamine D2 receptors. BMB Rep. 46, 519–526. doi: 10.5483/BMBRep.2013.46.11.207
Balcioğlu, A. S., Akinci, S., Çiçek, D., Eldem, H. O., Çoner, A., Bal, U. A., et al. (2016). Which is responsible for cardiac autonomic dysfunction in non-diabetic patients with metabolic syndrome: prediabetes or the syndrome itself? Diabetes Metab. Syndr. Clin. Res. Rev. 10 (1 Suppl. 1), S13–S20. doi: 10.1016/j.dsx.2015.09.001
Baldo, B. A., and Kelley, A. E. (2007). Discrete neurochemical coding of distinguishable motivational processes: insights from nucleus accumbens control of feeding. Psychopharmacology 191, 439–459. doi: 10.1007/s00213-007-0741-z
Banks, W. A., Jaspan, J. B., Huang, W., and Kastin, A. J. (1997). Transport of insulin across the blood-brain barrier: saturability at euglycemic doses of insulin. Peptides 18, 1423–1429. doi: 10.1016/S0196-9781(97)00231-3
Banks, W. A., Kastin, A. J., Huang, W., Jaspan, J. B., and Maness, L. M. (1996). Leptin enters the brain by a saturable system independent of insulin. Peptides 17, 305–311. doi: 10.1016/0196-9781(96)00025-3
Bartness, T. J., Song, C. K., Shi, H., Bowers, R. R., and Foster, M. T. (2005). Brain–adipose tissue cross talk. Proc. Nutr. Soc. 64, 53–64. doi: 10.1079/PNS2004409
Belfort-DeAguiar, R., Seo, D., Lacadie, C., Naik, S., Schmidt, C., Lam, W., et al. (2018). Humans with obesity have disordered brain responses to food images during physiological hyperglycemia. Am. J. Physiol. Metab. 314, E522–E529. doi: 10.1152/ajpendo.00335.2017
Belvederi Murri, M., Prestia, D., Mondelli, V., Pariante, C., Patti, S., Olivieri, B., et al. (2016). The HPA axis in bipolar disorder: systematic review and meta-analysis. Psychoneuroendocrinology 63, 327–342. doi: 10.1016/j.psyneuen.2015.10.014
Benedict, C., Hallschmid, M., Hatke, A., Schultes, B., Fehm, H. L., Born, J., et al. (2004). Intranasal insulin improves memory in humans. Psychoneuroendocrinology 29, 1326–1334. doi: 10.1016/j.psyneuen.2004.04.003
Benichou, T., Pereira, B., Mermillod, M., Tauveron, I., Pfabigan, D., Maqdasy, S., et al. (2018). Heart rate variability in type 2 diabetes mellitus: a systematic review and meta-analysis. PLoS One 13:e0195166. doi: 10.1371/journal.pone.0195166
Ben-Jonathan, N., and Hugo, E. (2015). Prolactin (prl) in adipose tissue: regulation and functions. Adv. Exp. Med. Biol. 846, 1–35. doi: 10.1007/978-3-319-12114-7_1
Benrick, A., Schéle, E., Pinnock, S. B., Wernstedt-Asterholm, I., Dickson, S. L., Karlsson-Lindahl, L., et al. (2009). Interleukin-6 gene knockout influences energy balance regulating peptides in the hypothalamic paraventricular and supraoptic nuclei. J. Neuroendocrinol. 21, 620–628. doi: 10.1111/j.1365-2826.2009.01879.x
Bernard, C. (1855). Leçons de Physiologie Experimentale Applique a la Medicine Faites au College de France. Paris: Baillère et fils, 296–313.
Berthon, B. S., MacDonald-Wicks, L. K., and Wood, L. G. (2014). A systematic review of the effect of oral glucocorticoids on energy intake, appetite, and body weight in humans. Nutr. Res. 34, 179–190. doi: 10.1016/j.nutres.2013.12.006
Bjørbaek, C., Elmquist, J. K., Michl, P., Ahima, R. S., van Bueren, A., McCall, A. L., et al. (1998). Expression of leptin receptor isoforms in rat brain microvessels. Endocrinology 139, 3485–3491. doi: 10.1210/endo.139.8.6154
Boado, R. J., Golden, P. L., Levin, N., and Pardridge, W. M. (1998). Up-regulation of blood-brain barrier short-form leptin receptor gene products in rats fed a high fat diet. J. Neurochem. 71, 1761–1764. doi: 10.1046/j.1471-4159.1998.71041761.x
Boersma, G. J., Johansson, E., Pereira, M. J., Heurling, K., Skrtic, S., Lau, J., et al. (2018). Altered glucose uptake in muscle, visceral adipose tissue, and brain predict whole-body insulin resistance and may contribute to the development of type 2 diabetes: a combined PET/MR study. Horm Metab. Res. 50, 627–639. doi: 10.1055/a-0643-4739
Bohula, E. A., Scirica, B. M., Inzucchi, S. E., McGuire, D. K., Keech, A. C., Smith, S. R., et al. (2018). Effect of lorcaserin on prevention and remission of type 2 diabetes in overweight and obese patients (CAMELLIA-TIMI 61): a randomised, placebo-controlled trial. Lancet 392, 2269–2279. doi: 10.1016/S0140-6736(18)32328-6
Born, J., Lange, T., Kern, W., McGregor, G. P., Bickel, U., and Fehm, H. L. (2002). Sniffing neuropeptides: a transnasal approach to the human brain. Nat. Neurosci. 5, 514–516. doi: 10.1038/nn0602-849
Brede, S., Sputh, A., Hartmann, A. C., Hallschmid, M., Lehnert, H., and Klement, J. (2017). Visual food cues decrease postprandial glucose concentrations in lean and obese men without affecting food intake and related endocrine parameters. Appetite 117, 255–262. doi: 10.1016/j.appet.2017.07.001
Brosschot, J. F., Van Dijk, E., and Thayer, J. F. (2007). Daily worry is related to low heart rate variability during waking and the subsequent nocturnal sleep period. Int. J. Psychophysiol. 63, 39–47. doi: 10.1016/j.ijpsycho.2006.07.016
Bruce, K. D., Zsombok, A., and Eckel, R. H. (2017). Lipid processing in the brain: a key regulator of systemic metabolism. Front. Endocrinol. 8:60. doi: 10.3389/fendo.2017.00060
Carey, M., Kehlenbrink, S., and Hawkins, M. (2013). Evidence for central regulation of glucose metabolism. J. Biol. Chem. 288, 34981–34988. doi: 10.1074/jbc.R113.506782
Carnethon, M. R., Golden, S. H., Folsom, A. R., Haskell, W., and Liao, D. (2003). Prospective investigation of autonomic nervous system function and the development of type 2 diabetes: the atherosclerosis risk in communities study, 1987-1998. Circulation 107, 2190–2195. doi: 10.1161/01.CIR.0000066324.74807.95
Carnethon, M. R., Prineas, R. J., Temprosa, M., Zhang, Z. M., Uwaifo, G., and Molitch, M. E. (2006). The association among autonomic nervous system function, incident diabetes, and intervention arm in the diabetes prevention program. Diabetes Care 29, 914–919. doi: 10.2337/diacare.29.04.06.dc05-1729
Carvalheira, J. B. C., Ribeiro, E. B., Araújo, E. P., Guimarães, R. B., Telles, M. M., Torsoni, M., et al. (2003). Selective impairment of insulin signalling in the hypothalamus of obese Zucker rats. Diabetologia 46, 1629–1640. doi: 10.1007/s00125-003-1246-x
Carvalho, L. P., Di Thommazo-Luporini, L., Mendes, R. G., Cabiddu, R., Ricci, P. A., Basso-Vanelli, R. P., et al. (2018). Metabolic syndrome impact on cardiac autonomic modulation and exercise capacity in obese adults. Auton Neurosci. Basic Clin. 213, 43–50. doi: 10.1016/j.autneu.2018.05.008
Casellini, C. M., Parson, H. K., Hodges, K., Edwards, J. F., Lieb, D. C., Wohlgemuth, S. D., et al. (2016). Bariatric surgery restores cardiac and sudomotor autonomic C-fiber dysfunction towards normal in obese subjects with type 2 diabetes. PLoS One 11:e0154211. doi: 10.1371/journal.pone.0154211
Chalmers, J. A., Quintana, D. S., Abbott, M. J. A., and Kemp, A. H. (2014). Anxiety disorders are associated with reduced heart rate variability: a meta-analysis. Front. Psychiatry 5:80. doi: 10.3389/fpsyt.2014.00080
Charles, L. E., Andrew, M. E., Sarkisian, K., Shengqiao, L., Mnatsakanova, A., Violanti, J. M., et al. (2013). Associations between insulin and heart rate variability in police officers. Am. J. Hum. Biol. 26, 56–63. doi: 10.1002/ajhb.22475
Chatterjee, S., and Mudher, A. (2018). Alzheimer’s disease and type 2 diabetes: a critical assessment of the shared pathological traits. Front. Neurosci. 12:383. doi: 10.3389/fnins.2018.00383
Chen, W., Balland, E., and Cowley, M. A. (2017). Hypothalamic insulin resistance in obesity: effects on glucose homeostasis. Neuroendocrinology 104, 364–381. doi: 10.1159/000455865
Cherkas, A., Abrahamovych, O., Golota, S., Nersesyan, A., Pichler, C., Serhiyenko, V., et al. (2015). The correlations of glycated hemoglobin and carbohydrate metabolism parameters with heart rate variability in apparently healthy sedentary young male subjects. Redox Biol. 5, 301–307. doi: 10.1016/j.redox.2015.05.007
Chintala, K. K., Krishna, B. H., and Mr, N. (2015). Heart rate variability in overweight health care students: correlation with visceral fat. J. Clin. Diagn. Res. 9, CC06–CC08. doi: 10.7860/JCDR/2015/12145.5434
Chung, H. K., Ryu, D., Kim, K. S., Chang, J. Y., Kim, Y. K., Yi, H. S., et al. (2017). Growth differentiation factor 15 is a myomitokine governing systemic energy homeostasis. J. Cell Biol. 216, 149–165. doi: 10.1083/jcb.201607110
Cintra, D. E., Ropelle, E. R., Moraes, J. C., Pauli, J. R., Morari, J., de Souza, C. T., et al. (2012). Unsaturated fatty acids revert diet-induced hypothalamic inflammation in obesity. PLoS One 7:e30571. doi: 10.1371/journal.pone.0030571
Clemmons, D. R. (2012). Metabolic actions of insulin-like growth factor-I in normal physiology and diabetes. Endocrinol. Metab. Clin. N. Am. 41, 425–443. doi: 10.1016/j.ecl.2012.04.017
Cohen, M. A., Ellis, S. M., Le Roux, C. W., Batterham, R. L., Park, A., Patterson, M., et al. (2003). Oxyntomodulin suppresses appetite and reduces food intake in humans. J. Clin. Endocrinol. Metab. 88, 4696–4701. doi: 10.1210/jc.2003-030421
Cosgrove, M. P., Sargeant, L. A., Caleyachetty, R., and Griffin, S. J. (2012). Work-related stress and Type 2 diabetes: systematic review and meta-analysis. Occup. Med. 62, 167–173. doi: 10.1093/occmed/kqs002
Coveleskie, K., Kilpatrick, L. A., Gupta, A., Stains, J., Connolly, L., Labus, J. S., et al. (2017). The effect of the GLP-1 analogue exenatide on functional connectivity within an NTS-based network in women with and without obesity. Obes. Sci. Pract. 3, 434–445. doi: 10.1002/osp4.124
Cowley, M. A., Smith, R. G., Diano, S., Tschöp, M., Pronchuk, N., Grove, K. L., et al. (2003). The distribution and mechanism of action of ghrelin in the CNS demonstrates a novel hypothalamic circuit regulating energy homeostasis. Neuron 37, 649–661. doi: 10.1016/S0896-6273(03)00063-1
Crabtree, D. R., Chambers, E. S., Hardwick, R. M., and Blannin, A. K. (2014). The effects of high-intensity exercise on neural responses to images of food1-3. Am. J. Clin. Nutr. 99, 258–267. doi: 10.3945/ajcn.113.071381
Czepielewski, L., Filho, L. D., Brietzke, E., and Grassi-Oliveira, R. (2013). Bipolar disorder and metabolic syndrome: a systematic review. Rev. Bras. Psiquiatr. 35, 88–93. doi: 10.1016/j.rbp.2012.07.004
da Silva, V. P., Ramalho Oliveira, B. R., Tavares Mello, R. G., Moraes, H., Deslandes, A. C., and Laks, J. (2018). Heart rate variability indexes in dementia: a systematic review with a quantitative analysis. Curr. Alzheimer Res. 15, 80–88. doi: 10.2174/1567205014666170531082352
Dai, X.-P., Liu, Z.-Q., Xu, L.-Y., Gong, Z.-C., Huang, Q., Dong, M., et al. (2012). Association of plasma epinephrine level with insulin sensitivity in metabolically healthy but obese individuals. Auton Neurosci. 167, 66–69. doi: 10.1016/j.autneu.2011.10.006
Dakin, C. L., Gunn, I., Small, C. J., Edwards, C. M. B., Hay, D. L., Smith, D. M., et al. (2001). Oxyntomodulin inhibits food intake in the rat. Endocrinology 142, 4244–4250. doi: 10.1210/endo.142.10.8430
Dash, S., Xiao, C., Morgantini, C., Koulajian, K., and Lewis, G. F. (2015a). Intranasal insulin suppresses endogenous glucose production in humans compared with placebo in the presence of similar venous insulin concentrations. Diabetes 64, 766–774. doi: 10.2337/db14-0685
Dash, S., Xiao, C., Morgantini, C., Koulajian, K., and Lewis, G. F. (2015b). Is insulin action in the brain relevant in regulating blood glucose in humans. J. Clin. Endocrinol. Metab. 100, 2525–2531.
de Jonge, L., Moreira, E. A. M., Martin, C. K., and Ravussin, E. (2010). Impact of 6-month caloric restriction on autonomic nervous system activity in healthy, overweight, individuals. Obesity 18, 414–416. doi: 10.1038/oby.2009.408
De Pergola, G., Giorgino, F., Benigno, R., Guida, P., and Giorgino, R. (2008). Independent influence of insulin, catecholamines, and thyroid hormones on metabolic syndrome. Obesity 16, 2405–2411. doi: 10.1038/oby.2008.382
De Silva, A., Salem, V., Long, C. J., Makwana, A., Newbould, R. D., Rabiner, E. A., et al. (2011). The gut hormones PYY 3-36 and GLP-1 7-36 amide reduce food intake and modulate brain activity in appetite centers in humans. Cell Metab. 14, 700–706. doi: 10.1016/j.cmet.2011.09.010
De Silva, A., Salem, V., Matthews, P. M., and Dhillo, W. S. (2012). The use of functional MRI to study appetite control in the CNS. Exp. Diabetes Res. 2012, 1–13. doi: 10.1155/2012/764017
Deem, J. D., Muta, K., Scarlett, J. M., Morton, G. J., and Schwartz, M. W. (2017). How should we think about the role of the brain in glucose homeostasis and diabetes? Diabetes 66, 1758–1765. doi: 10.2337/dbi16-0067
Delaere, F., Magnan, C., and Mithieux, G. (2010). Hypothalamic integration of portal glucose signals and control of food intake and insulin sensitivity. Diabetes Metab. 36, 257–262. doi: 10.1016/j.diabet.2010.05.001
Di Dalmazi, G., Pagotto, U., Pasquali, R., and Vicennati, V. (2012). Glucocorticoids and type 2 diabetes: from physiology to pathology. J. Nutr. Metab. 2012:525093. doi: 10.1155/2012/525093
Dimitropoulos, A., Tkach, J., Ho, A., and Kennedy, J. (2012). Greater corticolimbic activation to high-calorie food cues after eating in obese vs. normal-weight adults. Appetite 58, 303–312. doi: 10.1016/j.appet.2011.10.014
Duca, F. A., and Yue, J. T. Y. (2014). Fatty acid sensing in the gut and the hypothalamus: in vivo and in vitro perspectives. Mol. Cell. Endocrinol. 397, 23–33. doi: 10.1016/j.mce.2014.09.022
Dunn, J. T., Cranston, I., Marsden, P. K., Amiel, S. A., and Reed, L. J. (2007). Attenuation of amydgala and frontal cortical responses to low blood glucose concentration in asymptomatic hypoglycemia in type 1 diabetes. Diabetes 56, 2766–2773. doi: 10.2337/db07-0666
Eikelis, N., Schlaich, M., Aggarwal, A., Kaye, D., and Esler, M. (2003). Interactions between leptin and the human sympathetic nervous system. Hypertension 41, 1072–1079. doi: 10.1161/01.HYP.0000066289.17754.49
El-Haschimi, K., Pierroz, D. D., Hileman, S. M., Bjørbæk, C., and Flier, J. S. (2000). Two defects contribute to hypothalamic leptin resistance in mice with diet-induced obesity. J. Clin. Invest. 105, 1827–1832. doi: 10.1172/JCI9842
Eriksson, J., Franssila-Kallunki, A., Ekstrand, A., Saloranta, C., Widén, E., Schalin, C., et al. (1989). Early metabolic defects in persons at increased risk for non-insulin-dependent diabetes mellitus. N. Engl. J. Med. 321, 337–343. doi: 10.1056/NEJM198908103210601
Esposito, K., Pontillo, A., Giugliano, F., Giugliano, G., Marfella, R., Nicoletti, G., et al. (2003). Association of low interleukin-10 levels with the metabolic syndrome in obese women 15. J. Clin. Endocrinol. Metab. 88, 1055–1058. doi: 10.1210/jc.2002-021437
Esterson, Y. B., Carey, M., Boucai, L., Goyal, A., Raghavan, P., Zhang, K., et al. (2016). Central regulation of glucose production may be impaired in type 2 diabetes. Diabetes 65, 2569–2579. doi: 10.2337/db15-1465
Everson-Rose, S. A., Meyer, P. M., Powell, L. H., Pandey, D., Torréns, J. I., Kravitz, H. M., et al. (2004). Depressive symptoms, insulin resistance, and risk of diabetes in women at midlife. Diabetes Care 27, 2856–2862. doi: 10.2337/diacare.27.12.2856
Farr, O. M., Sofopoulos, M., Tsoukas, M. A., Dincer, F., Thakkar, B., Sahin-Efe, A., et al. (2016a). GLP-1 receptors exist in the parietal cortex, hypothalamus and medulla of human brains and the GLP-1 analogue liraglutide alters brain activity related to highly desirable food cues in individuals with diabetes: a crossover, randomised, placebo-controlled. Diabetologia 59, 954–965. doi: 10.1007/s00125-016-3874-y
Farr, O. M., Tsoukas, M. A., Triantafyllou, G., Dincer, F., Filippaios, A., Ko, B. J., et al. (2016b). Short-term administration of the GLP-1 analog liraglutide decreases circulating leptin and increases GIP levels and these changes are associated with alterations in CNS responses to food cues: a randomized, placebo-controlled, crossover study. Metabolism 65, 945–953. doi: 10.1016/j.metabol.2016.03.009
Faurholt-Jepsen, M., Kessing, L. V., and Munkholm, K. (2017). Heart rate variability in bipolar disorder: a systematic review and meta-analysis. Neurosci. Biobehav. Rev. 73, 68–80. doi: 10.1016/j.neubiorev.2016.12.007
Fisher, J. P., Young, C. N., and Fadel, P. J. (2015). Autonomic adjustments to exercise in humans. Compr. Physiol. 5, 475–512. doi: 10.1002/cphy.c140022
Flaa, A., Aksnes, T. A., Kjeldsen, S. E., Eide, I., and Rostrup, M. (2008a). Increased sympathetic reactivity may predict insulin resistance: an 18-year follow-up study. Metabolism 57, 1422–1427. doi: 10.1016/j.metabol.2008.05.012
Flaa, A., Sandvik, L., Kjeldsen, S. E., Eide, I. K., and Rostrup, M. (2008b). Does sympathoadrenal activity predict changes in body fat? An 18-y follow-up study. Am. J. Clin. Nutr. 87, 1596–1601.
Flanagan, D. E., Fulford, J., Krishnan, B., Benattayallah, A., Watt, A., and Summers, I. R. (2012). Functional MRI of the hypothalamic response to an oral glucose load. Diabetologia 55, 2080–2082. doi: 10.1007/s00125-012-2559-4
Flanagan, D. E., Vaile, J. C., Petley, G. W., Moore, V. M., Godsland, I. F., Cockington, R. A., et al. (1999). The autonomic control of heart rate and insulin resistance in young adults. J. Clin. Endocrinol. Metab. 84, 1263–1267. doi: 10.1210/jc.84.4.1263
Frank, S., Heinze, J. M., Fritsche, A., Linder, K., Von Feilitzsch, M., Königsrainer, A., et al. (2016). Neuronal food reward activity in patients with type 2 diabetes with improved glycemic control after bariatric surgery. Diabetes Care 39, 1311–1317. doi: 10.2337/dc16-0094
Frias, J. P., Nauck, M. A., Van, J., Kutner, M. E., Cui, X., Benson, C., et al. (2018). Efficacy and safety of LY3298176, a novel dual GIP and GLP-1 receptor agonist, in patients with type 2 diabetes: a randomised, placebo-controlled and active comparator-controlled phase 2 trial. Lancet 392, 2180–2193. doi: 10.1016/S0140-6736(18)32260-8
Fu, L., John, L. M., Adams, S. H., Yu, X. X., Tomlinson, E., Renz, M., et al. (2004). Fibroblast growth factor 19 increases metabolic rate and reverses dietary and leptin-deficient diabetes. Endocrinology 145, 2594–2603. doi: 10.1210/en.2003-1671
Führer, D., Zysset, S., and Stumvoll, M. (2008). Brain activity in hunger and satiety: an exploratory visually stimulated fMRI study. Obesity 16, 945–950. doi: 10.1038/oby.2008.33
Garber, A., Blonde, L., Bloomgarden, Z., Handelsman, Y., and Dagogo-Jack, S. (2013). The role of bromocriptine-QR in the management of type 2 diabetes expert panel recommendations. Endocr. Pract. 19, 100–106. doi: 10.4158/EP12325.OR
García-Cáceres, C., Quarta, C., Varela, L., Gao, Y., Gruber, T., Legutko, B., et al. (2016). Astrocytic insulin signaling couples brain glucose uptake with nutrient availability. Cell 166, 867–880. doi: 10.1016/j.cell.2016.07.028
Garvey, W. T., Ryan, D. H., Bohannon, N. J. V., Kushner, R. F., Rueger, M., Dvorak, R. V., et al. (2014a). Weight-loss therapy in type 2 diabetes: effects of phentermine and topiramate extended release. Diabetes Care 37, 3309–3316. doi: 10.2337/dc14-0930
Garvey, W. T., Ryan, D. H., Henry, R., Bohannon, N. J. V., Toplak, H., Schwiers, M., et al. (2014b). Prevention of type 2 diabetes in subjects with prediabetes and metabolic syndrome treated with phentermine and topiramate extended release. Diabetes Care 37, 912–921. doi: 10.2337/dc13-1518
Gautier, J. F., Chen, K., Salbe, A. D., Bandy, D., Pratley, R. E., Heiman, M., et al. (2000). Differential brain responses to satiation in obese and lean men. Diabetes 49, 838–846. doi: 10.2337/diabetes.49.5.838
Goldstone, A. P., Miras, A. D., Scholtz, S., Jackson, S., Neff, K. J., Pénicaud, L., et al. (2016). Link between increased satiety gut hormones and reduced food reward after gastric bypass surgery for obesity. J. Clin. Endocrinol. Metab. 101, 599–609. doi: 10.1210/jc.2015-2665
Gotoh, K., Inoue, M., Masaki, T., Chiba, S., Shimasaki, T., Ando, H., et al. (2012). A novel anti-inflammatory role for spleen-derived interleukin-10 in obesity-induced hypothalamic inflammation. J. Neurochem. 120, 752–764. doi: 10.1111/j.1471-4159.2011.07617.x
Grassi, G., Quarti-Trevano, F., Seravalle, G., Dell’Oro, R., Dubini, A., and Mancia, G. (2009). Differential sympathetic activation in muscle and skin neural districts in the metabolic syndrome. Metabolism 58, 1446–1451. doi: 10.1016/j.metabol.2009.04.028
Gray, S. M., and Barrett, E. J. (2018). Insulin transport into the brain. Am. J. Physiol. Physiol. 315, C125–C136. doi: 10.1152/ajpcell.00240.2017
Grayson, B. E., Seeley, R. J., and Sandoval, D. A. (2013). Wired on sugar: the role of the CNS in the regulation of glucose homeostasis. Nat. Rev. Neurosci. 14, 24–37. doi: 10.1038/nrn3409
Grosshans, M., Vollmert, C., Vollstädt-Klein, S., Tost, H., Leber, S., Bach, P., et al. (2012). Association of leptin with food cue-induced activation in human reward pathways. Arch. Gen. Psychiatry 69, 529–537. doi: 10.1001/archgenpsychiatry.2011.1586
Hallschmid, M., Benedict, C., Schultes, B., Born, J., and Kern, W. (2008). Obese men respond to cognitive but not to catabolic brain insulin signaling. Int. J. Obes. 32, 275–282. doi: 10.1038/sj.ijo.0803722
Hallschmid, M., Benedict, C., Schultes, B., Fehm, H. L., Born, J., and Kern, W. (2004). Intranasal insulin reduces body fat in men but not in women. Diabetes 53, 3024–3029. doi: 10.2337/diabetes.53.11.3024
Hallschmid, M., Higgs, S., Thienel, M., Ott, V., and Lehnert, H. (2012). Postprandial administration of intranasal insulin intensifies satiety and reduces intake of palatable snacks in women. Diabetes 61, 782–789. doi: 10.2337/db11-1390
Hannon, A. M., Thompson, C. J., and Sherlock, M. (2017). Diabetes in patients with acromegaly. Curr. Diab. Rep. 17:8. doi: 10.1007/s11892-017-0838-7
Harrison, T. A., Hindorff, L. A., Kim, H., Wines, R. C. M., Bowen, D. J., McGrath, B. B., et al. (2003). Family history of diabetes as a potential public health tool. Am. J. Preven. Med. 24, 152–159. doi: 10.1016/S0749-3797(02)00588-3
Hemingway, H., Shipley, M., Brunner, E., Britton, A., Malik, M., and Marmot, M. (2005). Does autonomic function link social position to coronary risk? The whitehall II study. Circulation 111, 3071–3077. doi: 10.1161/CIRCULATIONAHA.104.497347
Heni, M., Kullmann, S., Ketterer, C., Guthoff, M., Bayer, M., Staiger, H., et al. (2014a). Differential effect of glucose ingestion on the neural processing of food stimuli in lean and overweight adults. Hum. Brain Mapp. 35, 918–928. doi: 10.1002/hbm.22223
Heni, M., Wagner, R., Kullmann, S., Veit, R., Mat Husin, H., Linder, K., et al. (2014b). Central insulin administration improves whole-body insulin sensitivity via hypothalamus and parasympathetic outputs in men. Diabetes 63, 4083–4088. doi: 10.2337/db14-0477
Heni, M., Kullmann, S., Ketterer, C., Guthoff, M., Linder, K., Wagner, R., et al. (2012). Nasal insulin changes peripheral insulin sensitivity simultaneously with altered activity in homeostatic and reward-related human brain regions. Diabetologia 55, 1773–1782. doi: 10.1007/s00125-012-2528-y
Heni, M., Wagner, R., Kullmann, S., Gancheva, S., Roden, M., Peter, A., et al. (2017). Hypothalamic and striatal insulin action suppresses endogenous glucose production and may stimulate glucose uptake during hyperinsulinemia in lean but not in overweight men. Diabetes 66, 1797–1806. doi: 10.2337/db16-1380
Heni, M., Willmann, C., Wagner, R., Vosseler, A., Preissl, H., Peter, A., et al. (2018). “Brain insulin action stimulates pancreatic insulin secretion: results from hyperglycaemic clamps,” in Proceedings of the 54th annual meeting of the European Association for the Study of Diabetes, Berlin.
Hileman, S. M., Pierroz, D. D., Masuzaki, H., Bjørbaek, C., El-Haschimi, K., Banks, W. A., et al. (2002). Characterizaton of short isoforms of the leptin receptor in rat cerebral microvessels and of brain uptake of leptin in mouse models of obesity. Endocrinology 143, 775–783. doi: 10.1210/endo.143.3.8669
Hillebrand, S., Swenne, C. A., Gast, K. B., Maan, A. C., le Cessie, S., Jukema, J. W., et al. (2015). The role of insulin resistance in the association between body fat and autonomic function. Nutr. Metab. Cardiovasc. Dis. 25, 93–99. doi: 10.1016/j.numecd.2014.07.009
Hirvonen, J., Virtanen, K. A., Nummenmaa, L., Hannukainen, J. C., Honka, M. J., Bucci, M., et al. (2011). Effects of insulin on brain glucose metabolism in impaired glucose tolerance. Diabetes 60, 443–447. doi: 10.2337/db10-0940
Hölscher, C. (2018). Novel dual GLP-1/GIP receptor agonists show neuroprotective effects in Alzheimer’s and Parkinson’s disease models. Neuropharmacology 136(Pt B), 251–259. doi: 10.1016/j.neuropharm.2018.01.040
Hosoi, T., Sasaki, M., Miyahara, T., Hashimoto, C., Matsuo, S., Yoshii, M., et al. (2008). Endoplasmic reticulum stress induces leptin resistance. Mol. Pharmacol. 74, 1610–1619. doi: 10.1124/mol.108.050070
Hotamisligil, G. S. (2006). Inflammation and metabolic disorders. Nature 444, 860–867. doi: 10.1038/nature05485
Hotamisligil, G. S., Shargill, N. S., and Spiegelman, B. M. (1993). Adipose expression of tumor necrosis factor-alpha: direct role in obesity-linked insulin resistance. Science 259, 87–91. doi: 10.1126/science.7678183
Huggett, R. J., Burns, J., Mackintosh, A. F., and Mary, D. A. (2004). Sympathetic neural activation in nondiabetic metabolic syndrome and its further augmentation by hypertension. Hypertension 44, 847–852. doi: 10.1161/01.HYP.0000147893.08533.d8
Hutch, C. R., and Sandoval, D. (2017). The role of GLP-1 in the metabolic success of bariatric surgery. Endocrinology 158, 4139–4151. doi: 10.1210/en.2017-00564
Hwang, J. J., Parikh, L., Lacadie, C., Seo, D., Lam, W., Hamza, M., et al. (2018). Hypoglycemia unawareness in type 1 diabetes suppresses brain responses to hypoglycemia. J. Clin. Invest. 128, 1485–1495. doi: 10.1172/JCI97696
Incollingo Rodriguez, A. C., Epel, E. S., White, M. L., Standen, E. C., Seckl, J. R., and Tomiyama, A. J. (2015). Hypothalamic-pituitary-adrenal axis dysregulation and cortisol activity in obesity: a systematic review. Psychoneuroendocrinology 62, 301–318. doi: 10.1016/j.psyneuen.2015.08.014
Indumathy, J., Pal, G. K., Pal, P., Ananthanarayanan, P. H., Parija, S. C., Balachander, J., et al. (2015). Association of sympathovagal imbalance with obesity indices, and abnormal metabolic biomarkers and cardiovascular parameters. Obes. Res. Clin. Pract. 9, 55–66. doi: 10.1016/j.orcp.2014.01.007
Ito, H., Ohshima, A., Tsuzuki, M., Ohto, N., Yanagawa, M., Maruyama, T., et al. (2001). Effects of increased physical activity and mild calorie restriction on heart rate variability in obese women. Jpn. Heart J. 42, 459–469. doi: 10.1536/jhj.42.459
Iwen, K. A., Scherer, T., Heni, M., Sayk, F., Wellnitz, T., Machleidt, F., et al. (2014). Intranasal insulin suppresses systemic but not subcutaneous lipolysis in healthy humans. J. Clin. Endocrinol. Metab. 99, E246–E251. doi: 10.1210/jc.2013-3169
Jackson, S. E., Kirschbaum, C., and Steptoe, A. (2017). Hair cortisol and adiposity in a population-based sample of 2,527 men and women aged 54 to 87 years. Obesity 25, 539–544. doi: 10.1002/oby.21733
Jahansouz, C., Xu, H., Hertzel, A. V., Serrot, F. J., Kvalheim, N., Cole, A., et al. (2016). Bile acids increase independently from hypocaloric restriction after bariatric surgery. Ann. Surg. 264, 1022–1028. doi: 10.1097/SLA.0000000000001552
Jarczok, M. N., Li, J., Mauss, D., Fischer, J. E., and Thayer, J. F. (2013). Heart rate variability is associated with glycemic status after controlling for components of the metabolic syndrome. Int. J. Cardiol. 167, 855–861. doi: 10.1016/j.ijcard.2012.02.002
Johncy, S. S., Karthik, C. S., Bondade, S. Y., and Jayalakshmi, M. K. (2015). Altered cardiovascular autonomic function in young normotensive offspring of hypertensive parents - Is obesity an additional risk factor? J. Basic Clin. Physiol. Pharmacol. 26, 531–537. doi: 10.1515/jbcpp-2014-0068
Joseph, J. J., and Golden, S. H. (2017). Cortisol dysregulation: the bidirectional link between stress, depression, and type 2 diabetes mellitus. Ann. N. Y. Acad. Sci. 1391, 20–34. doi: 10.1111/nyas.13217
Kaiyala, K. J., Prigeon, R. L., Kahn, S. E., Woods, S. C., and Schwartz, M. W. (2000). Obesity induced by a high-fat diet is associated with reduced brain insulin transport in dogs. Diabetes 49, 1525–1533. doi: 10.2337/diabetes.49.9.1525
Kan, C., Silva, N., Golden, S. H., Rajala, U., Timonen, M., Stahl, D., et al. (2013). A systematic review and meta-analysis of the association between depression and insulin resistance. Diabetes Care 36, 480–489. doi: 10.2337/dc12-1442
Kanoski, S. E., Fortin, S. M., Arnold, M., Grill, H. J., and Hayes, M. R. (2011). Peripheral and central GLP-1 receptor populations mediate the anorectic effects of peripherally administered GLP-1 receptor agonists, liraglutide and exendin-4. Endocrinology 152, 3103–3112. doi: 10.1210/en.2011-0174
Karason, K., Mølgaard, H., Wikstrand, J., and Sjöström, L. (1999). Heart rate variability in obesity and the effect of weight loss. Am. J. Cardiol. 83, 1242–1247. doi: 10.1016/S0002-9149(99)00066-1
Katsurada, K., Maejima, Y., Nakata, M., Kodaira, M., Suyama, S., Iwasaki, Y., et al. (2014). Endogenous GLP-1 acts on paraventricular nucleus to suppress feeding: projection from nucleus tractus solitarius and activation of corticotropin-releasing hormone, nesfatin-1 and oxytocin neurons. Biochem. Biophys. Res. Commun. 451, 276–281. doi: 10.1016/j.bbrc.2014.07.116
Katsurada, K., and Yada, T. (2016). Neural effects of gut- and brain-derived glucagon-like peptide-1 and its receptor agonist. J. Diabetes Investig. 7(Suppl. 1), 64–69. doi: 10.1111/jdi.12464
Keitel, V., Görg, B., Bidmon, H. J., Zemtsova, I., Spomer, L., Zilles, K., et al. (2010). The bile acid receptor TGR5 (Gpbar-1) acts as a neurosteroid receptor in brain. Glia 58, 1794–1805. doi: 10.1002/glia.21049
Kemp, A. H., Quintana, D. S., Gray, M. A., Felmingham, K. L., Brown, K., and Gatt, J. M. (2010). Impact of depression and antidepressant treatment on heart rate variability: a review and meta-analysis. Biol. Psychiatry 67, 1067–1074. doi: 10.1016/j.biopsych.2009.12.012
Kern, P. A., Ranganathan, S., Li, C., Wood, L., and Ranganathan, G. (2001). Adipose tissue tumor necrosis factor and interleukin-6 expression in human obesity and insulin resistance. Am. J. Physiol. Metab. 280, E745–E751. doi: 10.1152/ajpendo.2001.280.5.E745
Kern, W., Benedict, C., Schultes, B., Plohr, F., Moser, A., Born, J., et al. (2006). Low cerebrospinal fluid insulin levels in obese humans. Diabetologia 49, 2790–2792. doi: 10.1007/s00125-006-0409-y
Khan, S. M., Hamnvik, O. P. R., Brinkoetter, M., and Mantzoros, C. S. (2012). Leptin as a modulator of neuroendocrine function in humans. Yonsei Med. J. 53, 671–679. doi: 10.3349/ymj.2012.53.4.671
Kievit, P., Howard, J. K., Badman, M. K., Balthasar, N., Coppari, R., Mori, H., et al. (2006). Enhanced leptin sensitivity and improved glucose homeostasis in mice lacking suppressor of cytokine signaling-3 in POMC-expressing cells. Cell Metab. 4, 123–132. doi: 10.1016/j.cmet.2006.06.010
Killgore, W. D. S., Kipman, M., Schwab, Z. J., Tkachenko, O., Preer, L., Gogel, H., et al. (2013). Physical exercise and brain responses to images of high-calorie food. Neuroreport 24, 962–967. doi: 10.1097/WNR.0000000000000029
Killgore, W. D. S., Young, A. D., Femia, L. A., Bogorodzki, P., Rogowska, J., and Yurgelun-Todd, D. A. (2003). Cortical and limbic activation during viewing of high- versus low-calorie foods. Neuroimage 19, 1381–1394. doi: 10.1016/S1053-8119(03)00191-5
Killgore, W. D. S., and Yurgelun-Todd, D. A. (2005). Body mass predicts orbitofrontal activity during visual presentations of high-calorie foods. Neuroreport 16, 859–863. doi: 10.1097/00001756-200505310-00016
Kimura, K., Nakamura, Y., Inaba, Y., Matsumoto, M., Kido, Y., Asahara, S. I., et al. (2013). Histidine augments the suppression of hepatic glucose production by central insulin action. Diabetes 62, 2266–2277. doi: 10.2337/db12-1701
Kimura, T., Matsumoto, T., Akiyoshi, M., Owa, Y., Miyasaka, N., Aso, T., et al. (2006). Body fat and blood lipids in postmenopausal women are related to resting autonomic nervous system activity. Eur. J. Appl. Physiol. 97, 542–547. doi: 10.1007/s00421-006-0207-8
Kishore, P., Boucai, L., Zhang, K., Li, W., Koppaka, S., Kehlenbrink, S., et al. (2011). Activation of KATP channels suppresses glucose production in humans. J. Clin. Invest. 121, 4916–4920. doi: 10.1172/JCI58035
Kjeldsen, S. E., Eide, I., Aakesson, I., and Leren, P. (1983). Influence of body weight on plasma catecholamine patterns in middle-aged, normotensive men. Scand. J. Clin. Lab. Invest. 43, 339–342. doi: 10.3109/00365518309168268
Kleiger, R. E., Stein, P. K., and Bigger, J. T. (2005). Heart rate variability: measurement and clinical utility. Ann. Noninvasive Electrocardiol. 10, 88–101. doi: 10.1111/j.1542-474X.2005.10101.x
Kleinert, M., Bojsen-Møller, K., Jørgensen, N., Svane, M., Martinussen, C., Kiens, B., et al. (2019). Effect of bariatric surgery on plasma GDF15 in humans. Am. J. Physiol. Endocrinol. Metab. 316, E615–E621. doi: 10.1152/ajpendo.00010.2019
Kleinert, M., Clemmensen, C., Sjøberg, K. A., Carl, C. S., Jeppesen, J. F., Wojtaszewski, J. F. P., et al. (2018). Exercise increases circulating GDF15 in humans. Mol. Metab. 9, 187–191. doi: 10.1016/j.molmet.2017.12.016
Koch, C., Augustine, R. A., Steger, J., Ganjam, G. K., Benzler, J., Pracht, C., et al. (2010). Leptin rapidly improves glucose homeostasis in obese mice by increasing hypothalamic insulin sensitivity. J. Neurosci. 30, 16180–16187. doi: 10.1523/JNEUROSCI.3202-10.2010
Kodama, S., Fujihara, K., Horikawa, C., Harada, M., Ishiguro, H., Kaneko, M., et al. (2018). Network meta-analysis of the relative efficacy of bariatric surgeries for diabetes remission. Obes. Rev. 19, 1621–1629. doi: 10.1111/obr.12751
Koliaki, C., Liatis, S., le Roux, C. W., and Kokkinos, A. (2017). The role of bariatric surgery to treat diabetes: current challenges and perspectives. BMC Endocr. Disord. 17:50. doi: 10.1186/s12902-017-0202-6
Kosinski, J. R., Hubert, J., Carrington, P. E., Chicchi, G. G., Mu, J., Miller, C., et al. (2012). The glucagon receptor is involved in mediating the body weight-lowering effects of oxyntomodulin. Obesity 20, 1566–1571. doi: 10.1038/oby.2012.67
Krajnak, K. M. (2014). Potential contribution of work-related psychosocial stress to the development of cardiovascular disease and type II diabetes: a brief review. Environ. Health Insights 8(Suppl. 1), 41–45. doi: 10.4137/EHI.S15263
Kuehl, L. K., Hinkelmann, K., Muhtz, C., Dettenborn, L., Wingenfeld, K., Spitzer, C., et al. (2015). Hair cortisol and cortisol awakening response are associated with criteria of the metabolic syndrome in opposite directions. Psychoneuroendocrinology 51, 365–370. doi: 10.1016/j.psyneuen.2014.09.012
LaBar, K. S., Gitelman, D. R., Parrish, T. B., Kim, Y. H., Nobre, A. C., and Mesulam, M. M. (2001). Hunger selectively modulates corticolimbic activation to food stimuli in humans. Behav. Neurosci. 115, 493–500. doi: 10.1037/0735-7044.115.2.493
Laferrère, B., Swerdlow, N., Bawa, B., Arias, S., Bose, M., Oliván, B., et al. (2010). Rise of oxyntomodulin in response to oral glucose after gastric bypass surgery in patients with type 2 diabetes. J. Clin. Endocrinol. Metab. 95, 4072–4076. doi: 10.1210/jc.2009-2767
Lam, T. K. T., Gutierrez-Juarez, R., Pocai, A., and Rossetti, L. (2005a). Regulation of blood glucose by hypothalamic pyruvate metabolism. Science 309, 943–947.
Lam, T. K. T., Pocai, A., Gutierrez-Juarez, R., Obici, S., Bryan, J., Aguilar-Bryan, L., et al. (2005b). Hypothalamic sensing of circulating fatty acids is required for glucose homeostasis. Nat. Med. 11, 320–327.
Langlet, F., Levin, B. E., Luquet, S., Mazzone, M., Messina, A., Dunn-Meynell, A. A., et al. (2013). Tanycytic VEGF-A boosts blood-hypothalamus barrier plasticity and access of metabolic signals to the arcuate nucleus in response to fasting. Cell Metab. 17, 607–617. doi: 10.1016/j.cmet.2013.03.004
LaPierre, M. P., Abraham, M. A., Yue, J. T., Filippi, B. M., and Lam, T. K. (2015). Glucagon signalling in the dorsal vagal complex is sufficient and necessary for high-protein feeding to regulate glucose homeostasis in vivo. EMBO Rep. 16, 1299–1307. doi: 10.15252/embr.201540492
Lawson, E. A. (2017). The effects of oxytocin on eating behaviour and metabolism in humans. Nat. Rev. Endocrinol. 13, 700–709. doi: 10.1038/nrendo.2017.115
Lawson, E. A., Marengi, D. A., Desanti, R. L., Holmes, T. M., Schoenfeld, D. A., and Tolley, C. J. (2015). Oxytocin reduces caloric intake in men. Obesity 23, 950–956. doi: 10.1002/oby.21069
Lee, J. H., Lin, L., Xu, P., Saito, K., Wei, Q., Meadows, A. G., et al. (2016). Neuronal deletion of ghrelin receptor almost completely prevents diet-induced obesity. Diabetes 65, 2169–2178. doi: 10.2337/db15-1587
Lee, Z. S. K., Critchley, J. A., Tomlinson, B., Young, R. P., Thomas, G. N., Cockram, C. S., et al. (2001). Urinary epinephrine and norepinephrine interrelations with obesity, insulin, and the metabolic syndrome in Hong Kong Chinese. Metabolism 50, 135–143. doi: 10.1053/meta.2001.19502
Li, X., Song, D., and Leng, S. X. (2015). Link between type 2 diabetes and Alzheimer’s disease: from epidemiology to mechanism and treatment. Clin. Interv. Aging 10, 549–560. doi: 10.2147/CIA.S74042
Li, Z., Tang, Z. H., Zeng, F., and Zhou, L. (2013). Associations between the severity of metabolic syndrome and cardiovascular autonomic function in a Chinese population. J. Endocrinol. Invest. 36, 993–999. doi: 10.3275/9005
Licinio, J., Mantzoros, C., Negrão, A. B., Cizza, G., Wong, M. L., Bongiorno, P. B., et al. (1997). Human leptin levels are pulsatile and inversely related to pituitary-adrenal function. Nat. Med. 3, 575–579. doi: 10.1038/nm0597-575
Lim, C. T., and Khoo, B. (2000). Normal Physiology of ACTH and GH Release in the Hypothalamus and Anterior Pituitary in Man. Dartmouth, MA: MDText.com, Inc.
Lindmark, S., Lönn, L., Wiklund, U., Tufvesson, M., Olsson, T., and Eriksson, J. W. (2005). Dysregulation of the autonomic nervous system can be a link between visceral adiposity and insulin resistance. Obes. Res. 13, 717–728. doi: 10.1038/oby.2005.81
Lips, M. A., De Groot, G. H., De Kam, M., Berends, F. J., Wiezer, R., Van Wagensveld, B. A., et al. (2013). Autonomic nervous system activity in diabetic and healthy obese female subjects and the effect of distinct weight loss strategies. Eur. J. Endocrinol. 169, 383–390. doi: 10.1530/EJE-13-0506
Lithell, H. O. (1991). Effect of antihypertensive drugs on insulin, glucose, and lipid metabolism. Diabetes Care 14, 203–209. doi: 10.2337/diacare.14.3.203
Liu, J., Yu, K., and Zhu, W. (2016). Amino acid sensing in the gut and its mediation in gut-brain signal transduction. Anim. Nutr. 2, 69–73. doi: 10.1016/j.aninu.2016.03.007
Liu, X. X., Zhu, X. M., Miao, Q., Ye, H. Y., Zhang, Z. Y., and Li, Y. M. (2014). Hyperglycemia induced by glucocorticoids in nondiabetic patients: a meta-analysis. Ann. Nutr. Metab. 65, 324–332. doi: 10.1159/000365892
Liu, Y., Gao, J. H., Liu, H. L., and Fox, P. T. (2000). The temporal response of the brain after eating revealed by functional MRI. Nature 405, 1058–1062. doi: 10.1038/35016590
Lopez Vicchi, F., Luque, G. M., Brie, B., Nogueira, J. P., Garcia Tornadu, I., and Becu-Villalobos, D. (2016). Dopaminergic drugs in type 2 diabetes and glucose homeostasis. Pharmacol. Res. 109, 74–80. doi: 10.1016/j.phrs.2015.12.029
López-Gambero, A. J., Martínez, F., Salazar, K., Cifuentes, M., and Nualart, F. (2019). Brain glucose-sensing mechanism and energy homeostasis. Mol. Neurobiol. 56, 769–796. doi: 10.1007/s12035-018-1099-4
Luo, S., Meier, A. H., and Cincotta, A. H. (1998). Bromocriptine reduces obesity, glucose intolerance and extracellular monoamine metabolite levels in the ventromedial hypothalamus of Syrian hamsters. Neuroendocrinology 68, 1–10. doi: 10.1159/000054344
Ma, Y., Tseng, P. H., Ahn, A., Wu, M. S., Ho, Y. L., Chen, M. F., et al. (2017). Cardiac autonomic alteration and metabolic syndrome: an ambulatory ECG-based study in a general population. Sci. Rep. 7:44363. doi: 10.1038/srep44363
Machleidt, F., Simon, P., Krapalis, A. F., Hallschmid, M., Lehnert, H., and Sayk, F. (2013). Experimental hyperleptinemia acutely increases vasoconstrictory sympathetic nerve activity in healthy humans. J. Clin. Endocrinol. Metab. 98, E491–E496. doi: 10.1210/jc.2012-3009
Malik, M., Camm, A. J., Bigger, J. T., Breithardt, G., Cerutti, S., Cohen, R. J., et al. (1996). Heart rate variability. Standards of measurement, physiological interpretation, and clinical use. Eur. Heart J. 17, 354–381. doi: 10.1093/oxfordjournals.eurheartj.a014868
Mangia, S., Tesfaye, N., De Martino, F., Kumar, A. F., Kollasch, P., Moheet, A. A., et al. (2012). Hypoglycemia-induced increases in thalamic cerebral blood flow are blunted in subjects with type 1 diabetes and hypoglycemia unawareness. J. Cereb. Blood Flow Metab. 32, 2084–2090. doi: 10.1038/jcbfm.2012.117
Marazziti, D., Rutigliano, G., Baroni, S., Landi, P., and Dell’Osso, L. (2014). Metabolic syndrome and major depression. CNS Spectrums 19, 293–304. doi: 10.1017/S1092852913000667
Marcelin, G., Jo, Y. H., Li, X., Schwartz, G. J., Zhang, Y., Dun, N. J., et al. (2014). Central action of FGF19 reduces hypothalamic AGRP/NPY neuron activity and improves glucose metabolism. Mol. Metab. 3, 19–28. doi: 10.1016/j.molmet.2013.10.002
Martin, L. E., Holsen, L. M., Chambers, R. J., Bruce, A. S., Brooks, W. M., Zarcone, J. R., et al. (2010). Neural mechanisms associated with food motivation in obese and healthy weight adults. Obesity 18, 254–260. doi: 10.1038/oby.2009.220
Marty, N., Dallaporta, M., and Thorens, B. (2007). Brain glucose sensing, counterregulation, and energy homeostasis. Physiology 22, 241–251. doi: 10.1152/physiol.00010.2007
Maser, R. E., Lenhard, M. J., Peters, M. B., Irgau, I., and Wynn, G. M. (2013). Effects of surgically induced weight loss by Roux-en-Y gastric bypass on cardiovascular autonomic nerve function. Surg. Obes. Relat. Dis. 9, 221–226. doi: 10.1016/j.soard.2011.11.014
Matsuda, M., Liu, Y., Mahankali, S., Pu, Y., Mahankali, A., Wang, J., et al. (1999). Altered hypothalamic function in response to glucose ingestion in obese humans. Diabetes 48, 1801–1806. doi: 10.2337/diabetes.48.9.1801
Meijer, R. I., Gray, S. M., Aylor, K. W., and Barrett, E. J. (2016). Pathways for insulin access to the brain: the role of the microvascular endothelial cell. Am. J. Physiol. Hear Circ. Physiol. 311, H1132–H1138. doi: 10.1152/ajpheart.00081.2016
Mercer, J. G., Hoggard, N., Williams, L. M., Lawrence, C. B., Hannah, L. T., and Trayhurn, P. (1996). Localization of leptin receptor mRNA and the long form splice variant (Ob-Rb) in mouse hypothalamus and adjacent brain regions by in situ hybridization. FEBS Lett. 387, 113–116. doi: 10.1016/0014-5793(96)00473-5
Mergenthaler, P., Lindauer, U., Dienel, G. A., and Meisel, A. (2013). Sugar for the brain: the role of glucose in physiological and pathological brain function. Trends Neurosci. 36, 587–597. doi: 10.1016/j.tins.2013.07.001
Meyer, M. L., Gotman, N. M., Soliman, E. Z., Whitsel, E. A., Arens, R., Cai, J., et al. (2016). Association of glucose homeostasis measures with heart rate variability among Hispanic/Latino adults without diabetes: the hispanic community health study/study of latinos (HCHS/SOL). Cardiovasc. Diabetol. 15:45. doi: 10.1186/s12933-016-0364-y
Mighiu, P. I., Yue, J. T. Y., Filippi, B. M., Abraham, M. A., Chari, M., Lam, C. K. L., et al. (2013). Hypothalamic glucagon signaling inhibits hepatic glucose production. Nat. Med. 19, 766–772. doi: 10.1038/nm.3115
Milanski, M., Degasperi, G., Coope, A., Morari, J., Denis, R., Cintra, D. E., et al. (2009). Saturated fatty acids produce an inflammatory response predominantly through the activation of TLR4 signaling in hypothalamus: implications for the pathogenesis of obesity. J. Neurosci. 29, 359–370. doi: 10.1523/JNEUROSCI.2760-08.2009
Mitchell, R. W., On, N. H., Del Bigio, M. R., Miller, D. W., and Hatch, G. M. (2011). Fatty acid transport protein expression in human brain and potential role in fatty acid transport across human brain microvessel endothelial cells. J. Neurochem. 117, 735–746. doi: 10.1111/j.1471-4159.2011.07245.x
Molfino, A., Fiorentini, A., Tubani, L., Martuscelli, M., Fanelli, F. R., and Laviano, A. (2009). Body mass index is related to autonomic nervous system activity as measured by heart rate variability. Eur. J. Clin. Nutr. 63, 1263–1265. doi: 10.1038/ejcn.2009.35
Monfredi, O., Lyashkov, A. E., Johnsen, A. B., Inada, S., Schneider, H., Wang, R., et al. (2014). Biophysical characterization of the underappreciated and important relationship between heart rate variability and heart rate. Hypertension 64, 1334–1343. doi: 10.1161/HYPERTENSIONAHA.114.03782
Montaquila, J. M., Trachik, B. J., and Bedwell, J. S. (2015). Heart rate variability and vagal tone in schizophrenia: a review. J. Psychiatr. Res. 69, 57–66. doi: 10.1016/j.jpsychires.2015.07.025
Montgomery, I. A., Irwin, N., and Flatt, P. R. (2013). Beneficial effects of (pGlu-Gln)-CCK-8 on energy intake and metabolism in high fat fed mice are associated with alterations of hypothalamic gene expression. Horm Metab. Res. 45, 471–473. doi: 10.1055/s-0032-1331767
Moon, H. S., Dalamaga, M., Kim, S. Y., Polyzos, S. A., Hamnvik, O. P., Magkos, F., et al. (2013). Leptin’s role in lipodystrophic and nonlipodystrophic insulin-resistant and diabetic individuals. Endocr. Rev. 34, 377–412. doi: 10.1210/er.2012-1053
Morrison, S. F. (2016). Central control of body temperature. F1000Res. 5:F1000FacultyRev–880. doi: 10.12688/f1000research.7958.1
Morton, G. J., Matsen, M. E., Bracy, D. P., Meek, T. H., Nguyen, H. T., Stefanovski, D., et al. (2013). FGF19 action in the brain induces insulin-independent glucose lowering. J. Clin. Invest. 123, 4799–4808. doi: 10.1172/JCI70710
Morton, G. J., Muta, K., Kaiyala, K. J., Rojas, J. M., Scarlett, J. M., Matsen, M. E., et al. (2017). Evidence that the sympathetic nervous system elicits rapid, coordinated, and reciprocal adjustments of insulin secretion and insulin sensitivity during cold exposure. Diabetes 66, 823–834. doi: 10.2337/db16-1351
Motahari-Tabari, N., Ahmad Shirvani, M., Shirzad-e-Ahoodashty, M., Yousefi-Abdolmaleki, E., and Teimourzadeh, M. (2014). The effect of 8 weeks aerobic exercise on insulin resistance in type 2 diabetes: a randomized clinical trial. Glob. J. Health Sci. 7, 115–121. doi: 10.5539/gjhs.v7n1p115
Moulton, C. D., Pickup, J. C., and Ismail, K. (2015). The link between depression and diabetes: the search for shared mechanisms. Lancet Diabetes Endocrinol. 3, 461–471. doi: 10.1016/S2213-8587(15)00134-5
Mullican, S. E., Lin-Schmidt, X., Chin, C. N., Chavez, J. A., Furman, J. L., Armstrong, A. A., et al. (2017). GFRAL is the receptor for GDF15 and the ligand promotes weight loss in mice and nonhuman primates. Nat. Med. 23, 1150–1157. doi: 10.1038/nm.4392
Nault, I., Nadreau, E., Paquet, C., Brassard, P., Marceau, P., Marceau, S., et al. (2007). Impact of bariatric surgery-induced weight loss on heart rate variability. Metabolism 56, 1425–1430. doi: 10.1016/j.metabol.2007.06.006
Neff, L. M., Broder, M. S., Beenhouwer, D., Chang, E., Papoyan, E., and Wang, Z. W. (2017). Network meta-analysis of lorcaserin and oral hypoglycaemics for patients with type 2 diabetes mellitus and obesity. Clin. Obes. 7, 337–346. doi: 10.1111/cob.12213
Newcomer, J. W. (2007). Metabolic syndrome and mental illness. Am. J. Manag. Care 13(7 Suppl.), S170–S177.
Ng, C. S., Lee, J. Y. C., Toh, M. P., and Ko, Y. (2014). Cost-of-illness studies of diabetes mellitus: a systematic review. Diabetes Res. Clin. Pract. 105, 151–163. doi: 10.1016/j.diabres.2014.03.020
Niswender, K. D., Morrison, C. D., Clegg, D. J., Olson, R., Baskin, D. G., Myers, M. G., et al. (2003). Insulin activation of phosphatidylinositol 3-kinase in the hypothalamic arcuate nucleus: a key mediator of insulin-induced anorexia. Diabetes 52, 227–231. doi: 10.2337/diabetes.52.2.227
Nolan, C. J., Damm, P., and Prentki, M. (2011). Type 2 diabetes across generations: from pathophysiology to prevention and management. Lancet 378, 169–181. doi: 10.1016/S0140-6736(11)60614-4
Norhammar, A., Bodegård, J., Nyström, T., Thuresson, M., Eriksson, J. W., and Nathanson, D. (2016). Incidence, prevalence and mortality of type 2 diabetes requiring glucose-lowering treatment, and associated risks of cardiovascular complications: a nationwide study in Sweden, 2006–2013. Diabetologia 59, 1692–1701. doi: 10.1007/s00125-016-3971-y
Notarianni, E. (2017). Cortisol: mediator of association between Alzheimer’s disease and diabetes mellitus? Psychoneuroendocrinology 81, 129–137. doi: 10.1016/j.psyneuen.2017.04.008
Obici, S., Feng, Z., Karkanias, G., Baskin, D. G., and Rossetti, L. (2002a). Decreasing hypothalamic insulin receptors causes hyperphagia and insulin resistance in rats. Nat. Neurosci. 5, 566–572.
Obici, S., Feng, Z., Morgan, K., Stein, D., Karkanias, G., and Rossetti, L. (2002b). Central administration of oleic acid inhibits glucose production and food intake. Diabetes 51, 271–275. doi: 10.2337/diabetes.51.2.271
Obici, S., Zhang, B. B., Karkanias, G., and Rossetti, L. (2002c). Hypothalamic insulin signaling is required for inhibition of glucose production. Nat. Med. 8, 1376–1382.
Oomura, Y., Kimura, K., Ooyama, H., Maeno, T., Iki, M., and Kuniyoshi, M. (1964). Reciprocal activities of the ventromedial and lateral hypothalamic areas of cats. Science 143, 484–485. doi: 10.1126/science.143.3605.484
Oomura, Y., Ono, T., Ooyama, H., and Wayner, M. J. (1969). Glucose and osmosensitive neurones of the rat hypothalamus. Nature 222, 282–284. doi: 10.1038/222282a0
Oomura, Y., Ooyama, H., Sugimori, M., Nakamura, T., and Yamada, Y. (1974). Glucose inhibition of the glucose-sensitive neurone in the rat lateral hypothalamus. Nature 247, 284–286. doi: 10.1038/247284a0
Osada, T., Suzuki, R., Ogawa, A., Tanaka, M., Hori, M., Aoki, S., et al. (2017). Functional subdivisions of the hypothalamus using areal parcellation and their signal changes related to glucose metabolism. Neuroimage 162, 1–12. doi: 10.1016/j.neuroimage.2017.08.056
Ott, V., Finlayson, G., Lehnert, H., Heitmann, B., Heinrichs, M., Born, J., et al. (2013). Oxytocin reduces reward-driven food intake in humans. Diabetes 62, 3418–3425. doi: 10.2337/db13-0663
Ott, V., Lehnert, H., Staub, J., Wönne, K., Born, J., and Hallschmid, M. (2015). Central nervous insulin administration does not potentiate the acute glucoregulatory impact of concurrent mild hyperinsulinemia. Diabetes 64, 760–765. doi: 10.2337/db14-0931
Ozcan, L., Ergin, A. S., Lu, A., Chung, J., Sarkar, S., Nie, D., et al. (2009). Endoplasmic reticulum stress plays a central role in development of leptin resistance. Cell Metab. 9, 35–51. doi: 10.1016/j.cmet.2008.12.004
Ozcan, U., Cao, Q., Yilmaz, E., Lee, A.-H., Iwakoshi, N. N., Ozdelen, E., et al. (2004). Endoplasmic reticulum stress links obesity, insulin action, and type 2 diabetes. Science 306, 457–461. doi: 10.1126/science.1103160
Page, K. A., Arora, J., Qiu, M., Relwani, R., Constable, R. T., and Sherwin, R. S. (2009). Small decrements in systemic glucose provoke increases in hypothalamic blood flow prior to the release of counterregulatory hormones. Diabetes 58, 448–452. doi: 10.2337/db08-1224
Page, K. A., Chan, O., Arora, J., Belfort-DeAguiar, R., Dzuira, J., Roehmholdt, B., et al. (2013). Effects of fructose vs glucose on regional cerebral blood flow in brain regions involved with appetite and reward pathways. JAMA 309, 63–70. doi: 10.1001/jama.2012.116975
Parati, G., and Esler, M. (2012). The human sympathetic nervous system: its relevance in hypertension and heart failure. Eur. Heart J. 33, 1058–1066. doi: 10.1093/eurheartj/ehs041
Patching, S. G. (2017). Glucose transporters at the blood-brain barrier: function, regulation and gateways for drug delivery. Mol. Neurobiol. 54, 1046–1077. doi: 10.1007/s12035-015-9672-6
Pathak, V., Flatt, P. R., and Irwin, N. (2018). Cholecystokinin (CCK) and related adjunct peptide therapies for the treatment of obesity and type 2 diabetes. Peptides 100, 229–235. doi: 10.1016/j.peptides.2017.09.007
Pedersen, B. K., and Febbraio, M. A. (2008). Muscle as an endocrine organ: focus on muscle-derived interleukin-6. Physiol. Rev. 88, 1379–1406. doi: 10.1152/physrev.90100.2007
Perciaccante, A., Fiorentini, A., Paris, A., Serra, P., and Tubani, L. (2006). Circadian rhythm of the autonomic nervous system in insulin resistant subjects with normoglycemia, impaired fasting glycemia, impaired glucose tolerance, type 2 diabetes mellitus. BMC Cardiovasc. Disord. 6:19. doi: 10.1186/1471-2261-6-19
Perini, R., and Veicsteinas, A. (2003). Heart rate variability and autonomic activity at rest and during exercise in various physiological conditions. Eur. J. Appl. Physiol. 90, 317–325. doi: 10.1007/s00421-003-0953-9
Perry, B. I., McIntosh, G., Weich, S., Singh, S., and Rees, K. (2016). The association between first-episode psychosis and abnormal glycaemic control: systematic review and meta-analysis. Lancet Psychiatry 3, 1049–1058. doi: 10.1016/S2215-0366(16)30262-0
Pivonello, R., Isidori, A. M., De Martino, M. C., Newell-Price, J., Biller, B. M. K., and Colao, A. (2016). Complications of cushing’s syndrome: state of the art. Lancet Diabetes Endocrinol. 4, 611–629. doi: 10.1016/S2213-8587(16)00086-3
Pocai, A. (2012). Unraveling oxyntomodulin, GLP1’s enigmatic brother. J. Endocrinol. 215, 335–346. doi: 10.1530/JOE-12-0368
Pocai, A., Lam, T. K. T., Gutierrez-Juarez, R., Obici, S., Schwartz, G. J., Bryan, J., et al. (2005). Hypothalamic KATP channels control hepatic glucose production. Nature 434, 1026–1031. doi: 10.1038/nature03439
Poliakova, N., Després, J. P., Bergeron, J., Alméras, N., Tremblay, A., and Poirier, P. (2012). Influence of obesity indices, metabolic parameters and age on cardiac autonomic function in abdominally obese men. Metabolism 61, 1270–1279. doi: 10.1016/j.metabol.2012.02.006
Pontiroli, A. E., Merlotti, C., Veronelli, A., and Lombardi, F. (2013). Effect of weight loss on sympatho-vagal balance in subjects with grade-3 obesity: restrictive surgery versus hypocaloric diet. Acta Diabetol. 50, 843–850. doi: 10.1007/s00592-013-0454-1
Pories, W. J., Swanson, M. S., MacDonald, K. G., Long, S. B., Morris, P. G., and Brown, B. M. (1995). Who would have thought it? An operation proves to be the most effective therapy for adult-onset diabetes mellitus. Ann. Surg. 222, 339–350. doi: 10.1097/00000658-199509000-00011
Porubská, K., Veit, R., Preissl, H., Fritsche, A., and Birbaumer, N. (2006). Subjective feeling of appetite modulates brain activity. An fMRI study. Neuroimage 32, 1273–1280. doi: 10.1016/j.neuroimage.2006.04.216
Posey, K. A., Clegg, D. J., Printz, R. L., Byun, J., Morton, G. J., Vivekanandan-Giri, A., et al. (2009). Hypothalamic proinflammatory lipid accumulation, inflammation, and insulin resistance in rats fed a high-fat diet. Am. J. Physiol. Endocrinol. Metab. 296, E1003–E1012. doi: 10.1152/ajpendo.90377.2008
Pournaras, D. J., Glicksman, C., Vincent, R. P., Kuganolipava, S., Alaghband-Zadeh, J., Mahon, D., et al. (2012). The role of bile after Roux-en-Y gastric bypass in promoting weight loss and improving glycaemic control. Endocrinology 153, 3613–3619. doi: 10.1210/en.2011-2145
Prigeon, R. L., Quddusi, S., Paty, B., and D’Alessio, D. A. (2003). Suppression of glucose production by GLP-1 independent of islet hormones: a novel extrapancreatic effect. Am. J. Physiol. Endocrinol. Metab. 285, E701–E707. doi: 10.1152/ajpendo.00024.2003
Ramnanan, C. J., Saraswathi, V., Smith, M. S., Donahue, E. P., Farmer, B., Farmer, T. D., et al. (2011). Brain insulin action augments hepatic glycogen synthesis without suppressing glucose production or gluconeogenesis in dogs. J. Clin. Invest. 121, 3713–3723. doi: 10.1172/JCI45472
Ramos-Lobo, A. M., and Donato, J. (2017). The role of leptin in health and disease. Temperature 4, 258–291. doi: 10.1080/23328940.2017.1327003
Rannelli, L. A., MacRae, J. M., Mann, M. C., Ramesh, S., Hemmelgarn, B. R., Rabi, D., et al. (2017). Sex differences in associations between insulin resistance, heart rate variability, and arterial stiffness in healthy women and men: a physiology study. Can. J. Physiol. Pharmacol. 95, 349–355. doi: 10.1139/cjpp-2016-0122
Rapoport, S. I., Chang, M. C. J., and Spector, A. A. (2001). Delivery and turnover of plasma-derived essential PUFAs in mammalian brain. J. Lipid Res. 42, 678–685.
Rastović, M., Srdić-Galić, B., Barak, O., and Stokić, E. (2017). Association between anthropometric measures of regional fat mass and heart rate variability in obese women. Nutr. Diet 74, 51–60. doi: 10.1111/1747-0080.12280
Rebelos, E., Virtanen, K., Nuutila, P., Nummenmaa, L., Mari, A., Hyypia, A., et al. (2018). “The influence of brain metabolism in insulin secretion and action assessed with FDG-PET in humans,” in Proceedings of the 54th Annual Meeting of the European Association for the Study of Diabetes, Berlin.
Reimann, M., Qin, N., Gruber, M., Bornstein, S. R., Kirschbaum, C., Ziemssen, T., et al. (2017). Adrenal medullary dysfunction as a feature of obesity. Int. J. Obes. 41, 714–721. doi: 10.1038/ijo.2017.36
Reims, H. M., Sevre, K., Fossum, E., Høieggen, A., Mellem, H., and Kjeldsen, S. E. (2004). Relations between insulin sensitivity, fitness and autonomic cardiac regulation in healthy, young men. J. Hypertens 22, 2007–2015. doi: 10.1097/00004872-200410000-00025
Rodriguez-Diaz, R., Molano, R. D., Weitz, J. R., Abdulreda, M. H., Berman, D. M., Leibiger, B., et al. (2018). Paracrine interactions within the pancreatic islet determine the glycemic set point. Cell Metab. 27, 549–558.e4. doi: 10.1016/j.cmet.2018.01.015
Roh, E., Song, D. K., and Kim, M.-S. (2016). Emerging role of the brain in the homeostatic regulation of energy and glucose metabolism. Exp. Mol. Med. 48:e216. doi: 10.1038/emm.2016.4
Ropelle, E. R., Flores, M. B., Cintra, D. E., Rocha, G. Z., Pauli, J. R., Morari, J., et al. (2010). IL-6 and IL-10 anti-inflammatory activity links exercise to hypothalamic insulin and leptin sensitivity through IKKβ and ER stress inhibition. PLoS Biol. 8:e1000465. doi: 10.1371/journal.pbio.1000465
Rosenbaum, M., Sy, M., Pavlovich, K., Leibel, R. L., and Hirsch, J. (2008). Leptin reverses weight loss-induced changes in regional neural activity responses to visual food stimuli. J. Clin. Invest. 118, 2583–2591. doi: 10.1172/JCI35055
Rothemund, Y., Preuschhof, C., Bohner, G., Bauknecht, H. C., Klingebiel, R., Flor, H., et al. (2007). Differential activation of the dorsal striatum by high-calorie visual food stimuli in obese individuals. Neuroimage 37, 410–421. doi: 10.1016/j.neuroimage.2007.05.008
Routh, V. H., Hao, L., Santiago, A. M., Sheng, Z., and Zhou, C. (2014). Hypothalamic glucose sensing: making ends meet. Front. Syst. Neurosci. 8:236. doi: 10.3389/fnsys.2014.00236
Rubi, B., Ljubicic, S., Pournourmohammadi, S., Carobbio, S., Armanet, M., Bartley, C., et al. (2005). Dopamine D2-like receptors are expressed in pancreatic beta cells and mediate inhibition of insulin secretion. J. Biol. Chem. 280, 36824–36832. doi: 10.1074/jbc.M505560200
Rubino, F., Nathan, D. M., Eckel, R. H., Schauer, P. R., Alberti, K. G., Zimmet, P. Z., et al. (2017). Metabolic surgery in the treatment algorithm for type 2 diabetes: a joint statement by international diabetes organizations. Obes. Surg. 27, 2–21. doi: 10.1007/s11695-016-2457-9
Russell, E., Kirschbaum, C., Laudenslager, M. L., Stalder, T., De Rijke, Y., Van Rossum, E. F. C., et al. (2015). Toward standardization of hair cortisol measurement: results of the first international interlaboratory round robin. Ther. Drug Monit. 37, 71–75. doi: 10.1097/FTD.0000000000000148
Saito, I., Maruyama, K., Eguchi, E., Kato, T., Kawamura, R., Takata, Y., et al. (2017). Low heart rate variability and sympathetic dominance modifies the association between insulin resistance and metabolic syndrome – the toon health study –. Circ. J. 81, 1447–1453. doi: 10.1253/circj.CJ-17-0192
Sandoval, D. A., Bagnol, D., Woods, S. C., D’Alessio, D. A., and Seeley, R. J. (2008). Arcuate glucagon-like peptide 1 receptors regulate glucose homeostasis but not food intake. J. Diabetes 57, 2046–2054. doi: 10.2337/db07-1824
Scarlett, J. M., Rojas, J. M., Matsen, M. E., Kaiyala, K. J., Stefanovski, D., Bergman, R. N., et al. (2016). Central injection of fibroblast growth factor 1 induces sustained remission of diabetic hyperglycemia in rodents. Nat. Med. 22, 800–806. doi: 10.1038/nm.4101
Scholtz, S., Miras, A. D., Chhina, N., Prechtl, C. G., Sleeth, M. L., Daud, N. M., et al. (2014). Obese patients after gastric bypass surgery have lower brain-hedonic responses to food than after gastric banding. Gut 63, 891–902. doi: 10.1136/gutjnl-2013-305008
Schwartz, M. W., Baskin, D. G., Bukowski, T. R., Kuijper, J. L., Foster, D., Lasser, G., et al. (1996). Specificity of leptin action on elevated blood glucose levels and hypothalamic neuropeptide Y gene expression in ob/ob mice. Diabetes 45, 531–535.
Schwartz, R. S., Jaeger, L. F., and Veith, R. C. (1987). The importance of body composition to the increase in plasma norepinephrine appearance rate in elderly men. J. Gerontol. 42, 546–551. doi: 10.1093/geronj/42.5.546
Scott, M. M., Perello, M., Chuang, J. C., Sakata, I., Gautron, L., Lee, C. E., et al. (2012). Hindbrain ghrelin receptor signaling is sufficient to maintain fasting glucose. PLoS One 7:e44089. doi: 10.1371/journal.pone.0044089
Seaquist, E. R., Moheet, A., Kumar, A., Deelchand, D. K., Terpstra, M., Kubisiak, K., et al. (2017). Hypothalamic glucose transport in humans during experimentally induced hypoglycemia-associated autonomic failure. J. Clin. Endocrinol. Metab. 102, 3571–3580. doi: 10.1210/jc.2017-00477
Secher, A., Jelsing, J., Baquero, A. F., Hecksher-Sørensen, J., Cowley, M. A., Dalbøge, L. S., et al. (2014). The arcuate nucleus mediates GLP-1 receptor agonist liraglutide-dependent weight loss. J. Clin. Invest. 124, 4473–4488. doi: 10.1172/JCI75276
Seoane-Collazo, P., Fernø, J., Gonzalez, F., Diéguez, C., Leis, R., Nogueiras, R., et al. (2015). Hypothalamic-autonomic control of energy homeostasis. Endocrine 50, 276–291. doi: 10.1007/s12020-015-0658-y
Serfling, G., Buades-Rotger, M., Harbeck, B., Krämer, U. M., and Brabant, G. (2019). The corticosteroid prednisolone increases amygdala and insula reactivity to food approach signals in healthy young men. Psychoneuroendocrinology 99, 154–165. doi: 10.1016/j.psyneuen.2018.09.007
Sgoifo, A., Carnevali, L., Alfonso Mde, L. A., and Amore, M. (2015). Autonomic dysfunction and heart rate variability in depression. Stress 18, 343–352. doi: 10.3109/10253890.2015.1045868
Shinohara, M., and Sato, N. (2017). Bidirectional interactions between diabetes and Alzheimer’s disease. Neurochem. Int. 108, 296–302. doi: 10.1016/j.neuint.2017.04.020
Sienkiewicz, E., Magkos, F., Aronis, K. N., Brinkoetter, M., Chamberland, J. P., Chou, S., et al. (2011). Long-term metreleptin treatment increases bone mineral density and content at the lumbar spine of lean hypoleptinemic women. Metabolism 60, 1211–1221. doi: 10.1016/j.metabol.2011.05.016
Sinclair, P., Brennan, D. J., and le Roux, C. W. (2018). Gut adaptation after metabolic surgery and its influences on the brain, liver and cancer. Nat. Rev. Gastroenterol. Hepatol. 15, 606–624. doi: 10.1038/s41575-018-0057-y
Sjoberg, N., Brinkworth, G. D., Wycherley, T. P., Noakes, M., and Saint, D. A. (2011). Moderate weight loss improves heart rate variability in overweight and obese adults with type 2 diabetes. J. Appl. Physiol. 110, 1060–1064. doi: 10.1152/japplphysiol.01329.2010
Smeets, P. A., De Graaf, C., Stafleu, A., Van Osch, M. J., and Van Der Grond, J. (2005). Functional MRI of human hypothalamic responses following glucose ingestion. Neuroimage 24, 363–368. doi: 10.1016/j.neuroimage.2004.07.073
Smeets, P. A. M., Vidarsdottir, S., de Graaf, C., Stafleu, A., van Osch, M. J. P., Viergever, M. A., et al. (2007). Oral glucose intake inhibits hypothalamic neuronal activity more effectively than glucose infusion. AJP Endocrinol. Metab. 293, E754–E758. doi: 10.1152/ajpendo.00231.2007
Smith, K. J., Béland, M., Clyde, M., Gariépy, G., Pagé, V., Badawi, G., et al. (2013). Association of diabetes with anxiety: a systematic review and meta-analysis. J. Psychosom. Res. 74, 89–99. doi: 10.1016/j.jpsychores.2012.11.013
Smith, Q. R. (2000). Transport of glutamate and other amino acids at the blood-brain barrier. J. Nutr. 130(4S Suppl.), 1016S–1022S. doi: 10.1093/jn/130.4.1016S
Smith, Q. R., and Nagura, H. (2001). Fatty acid uptake and incorporation in brain: studies with the perfusion model. J. Mol. Neurosci. 16, 167–172. doi: 10.1385/JMN:16:2-3:167
Spanswick, D., Smith, M. A., Mirshamsi, S., Routh, V. H., and Ashford, M. L. (2000). Insulin activates ATP-sensitive K+ channels in hypothalamic neurons of lean, but not obese rats. Nat. Neurosci. 3, 757–758. doi: 10.1038/77660
Stark, R., Reichenbach, A., Lockie, S. H., Pracht, C., Wu, Q., Tups, A., et al. (2015). Acyl ghrelin acts in the brain to control liver function and peripheral glucose homeostasis in male mice. Endocrinology 156, 858–868. doi: 10.1210/en.2014-1733
Steensberg, A., Fischer, C. P., Keller, C., Møller, K., and Pedersen, B. K. (2003). IL-6 enhances plasma IL-1ra, IL-10, and cortisol in humans. Am. J. Physiol. Endocrinol. Metab. 285, E433–E437. doi: 10.1152/ajpendo.00074.2003
Stein, P. K., Barzilay, J. I., Domitrovich, P. P., Chaves, P. M., Gottdiener, J. S., Heckbert, S. R., et al. (2007). The relationship of heart rate and heart rate variability to non-diabetic fasting glucose levels and the metabolic syndrome: the cardiovascular health study. Diabet Med. 24, 855–863. doi: 10.1111/j.1464-5491.2007.02163.x
Stetler, C., and Miller, G. E. (2011). Depression and hypothalamic-pituitary-adrenal activation: a quantitative summary of four decades of research. Psychosom. Med. 73, 114–126. doi: 10.1097/PSY.0b013e31820ad12b
Steyn, F. J., Tolle, V., Chen, C., and Epelbaum, J. (2016). Neuroendocrine regulation of growth hormone secretion. Compr. Physiol. 6, 687–735. doi: 10.1002/cphy.c150002
Stingl, K. T., Kullmann, S., Guthoff, M., Heni, M., Fritsche, A., and Preissl, H. (2010). Insulin modulation of magnetoencephalographic resting state dynamics in lean and obese subjects. Front. Syst. Neurosci. 4:157. doi: 10.3389/fnsys.2010.00157
Stoeckel, L. E., Weller, R. E., Cook, E. W., Twieg, D. B., Knowlton, R. C., and Cox, J. E. (2008). Widespread reward-system activation in obese women in response to pictures of high-calorie foods. Neuroimage 41, 636–647. doi: 10.1016/j.neuroimage.2008.02.031
St-Onge, M.-P., Sy, M., Heymsfield, S. B., and Hirsch, J. (2005). Human cortical specialization for food: a functional magnetic resonance imaging investigation. J. Nutr. 135, 1014–1018. doi: 10.1093/jn/135.5.1014
Stuckey, M. I., Kiviniemi, A., Gill, D. P., Shoemaker, J. K., and Petrella, R. J. (2015). Associations between heart rate variability, metabolic syndrome risk factors, and insulin resistance. Appl. Physiol. Nutr. Metab. 40, 734–740. doi: 10.1139/apnm-2014-0528
Stuckey, M. I., Tulppo, M. P., Kiviniemi, A. M., and Petrella, R. J. (2014). Heart rate variability and the metabolic syndrome: a systematic review of the literature. Diabetes Metab. Res. Rev. 30, 784–793. doi: 10.1002/dmrr.2555
Su, Y., Lam, T. K. T., He, W., Pocai, A., Bryan, J., Aguilar-Bryan, L., et al. (2012). Hypothalamic leucine metabolism regulates liver glucose production. Diabetes 61, 85–93. doi: 10.2337/db11-0857
Sun, Y., Asnicar, M., and Smith, R. G. (2007). Central and peripheral roles of ghrelin on glucose homeostasis. Neuroendocrinology 86, 215–228. doi: 10.1159/000109094
Surwit, R. S., Williams, R. B., Lane, J. D., Feinglos, M. N., Kuhn, C. M., and Georgiades, A. (2010). Plasma epinephrine predicts fasting glucose in centrally obese African-American women. Obesity 18, 1683–1687. doi: 10.1038/oby.2010.43
Svensson, M., Jansson, P. A., Persson, A. L., Sjöstrand, M., and Eriksson, J. W. (2011). Atropine improves insulin sensitivity in both lean and abdominally obese subjects. J. Clin. Endocrinol. Metab. 96, E1843–E1847. doi: 10.1210/jc.2011-0669
Svensson, M. K., Lindmark, S., Wiklund, U., Rask, P., Karlsson, M., Myrin, J., et al. (2016). Alterations in heart rate variability during everyday life are linked to insulin resistance. A role of dominating sympathetic over parasympathetic nerve activity? Cardiovasc. Diabetol. 15:91. doi: 10.1186/s12933-016-0411-8
Sztajzel, J., Golay, A., Makoundou, V., Lehmann, T. N. O., Barthassat, V., Sievert, K., et al. (2009). Impact of body fat mass extent on cardiac autonomic alterations in women. Eur. J. Clin. Invest. 39, 649–656. doi: 10.1111/j.1365-2362.2009.02158.x
Tataranni, P. A., Gautier, J. F., Chen, K., Uecker, A., Bandy, D., Salbe, A. D., et al. (1999). Neuroanatomical correlates of hunger and satiation in humans using positron emission tomography. Proc. Natl. Acad. Sci. U.S.A. 96, 4569–4574. doi: 10.1073/pnas.96.8.4569
Tataranni, P. A., Young, J. B., Bogardus, C., and Ravussin, E. (1997). A low sympathoadrenal activity is associated with body weight gain and development of central adiposity in Pima Indian men. Obes. Res. 5, 341–347. doi: 10.1002/j.1550-8528.1997.tb00562.x
Teeuwisse, W. M., Widya, R. L., Paulides, M., Lamb, H. J., Smit, J. W. A., De Roos, A., et al. (2012). Short-term caloric restriction normalizes hypothalamic neuronal responsiveness to glucose ingestion in patients with type 2 diabetes. Diabetes 61, 3255–3259. doi: 10.2337/db11-1835
Ten Kulve, J. S., Veltman, D. J., Gerdes, V. E. A., Van Bloemendaal, L., Barkhof, F., Deacon, C. F., et al. (2017). Elevated postoperative endogenous GLP-1 levels mediate effects of roux-en-Y gastric bypass on neural responsivity to food cues. Diabetes Care 40, 1522–1529. doi: 10.2337/dc16-2113
Ten Kulve, J. S., Veltman, D. J., Van Bloemendaal, L., Groot, P. F. C., Ruhé, H. G., Barkhof, F., et al. (2016). Endogenous GLP1 and GLP1 analogue alter CNS responses to palatable food consumption. J. Endocrinol. 229, 1–12. doi: 10.1530/JOE-15-0461
Thaler, J. P., Yi, C. X., Schur, E. A., Guyenet, S. J., Hwang, B. H., Dietrich, M. O., et al. (2012). Obesity is associated with hypothalamic injury in rodents and humans. J. Clin. Invest. 122, 153–162. doi: 10.1172/JCI59660
Thienel, M., Fritsche, A., Heinrichs, M., Peter, A., Ewers, M., Lehnert, H., et al. (2016). Oxytocin’s inhibitory effect on food intake is stronger in obese than normal-weight men. Int. J. Obes. 40, 1707–1714. doi: 10.1038/ijo.2016.149
Thiyagarajan, R., Subramanian, S. K., Sampath, N., Trakroo, M., Pal, P., Bobby, Z., et al. (2012). Association between cardiac autonomic function, oxidative stress and inflammatory response in impaired fasting glucose subjects: cross-sectional study. PLoS One 7:e41889. doi: 10.1371/journal.pone.0041889
Thorens, B. (2014). Neural regulation of pancreatic islet cell mass and function. Diabetes Obes. Metab. 16(Suppl. 1), 87–95. doi: 10.1111/dom.12346
Tillner, J., Posch, M. G., Wagner, F., Teichert, L., Hijazi, Y., Einig, C., et al. (2019). A novel dual glucagon-like peptide and glucagon receptor agonist SAR425899: results of randomized, placebo-controlled first-in-human and first-in-patient trials. Diabetes Obes. Metab. 21, 120–128. doi: 10.1111/dom.13494
Timper, K., Denson, J. L., Steculorum, S. M., Heilinger, C., Engström-Ruud, L., Wunderlich, C. M., et al. (2017). IL-6 improves energy and glucose homeostasis in obesity via enhanced central IL-6 trans-signaling. Cell Rep. 19, 267–280. doi: 10.1016/j.celrep.2017.03.043
Troisi, R. J., Weiss, S. T., Parker, D. R., Sparrow, D., Young, J. B., and Landsberg, L. (1991). Relation of obesity and diet to sympathetic nervous system activity. Hypertension 17, 669–677. doi: 10.1161/01.HYP.17.5.669
Tsai, V. W. W., Lin, S., Brown, D. A., Salis, A., and Breit, S. N. (2016). Anorexia-cachexia and obesity treatment may be two sides of the same coin: role of the TGF-b superfamily cytokine MIC-1/GDF15. Int. J. Obes. 40, 193–197. doi: 10.1038/ijo.2015.242
Tsai, V. W. W., Manandhar, R., Jørgensen, S. B., Lee-Ng, K. K. M., Zhang, H. P., Marquis, C. P., et al. (2014). The anorectic actions of the TGFβ cytokine MIC-1/GDF15 require an intact brainstem area postrema and nucleus of the solitary tract. PLoS One 9:e100370. doi: 10.1371/journal.pone.0100370
Tschritter, O., Preissl, H., Hennige, A. M., Stumvoll, M., Porubska, K., Frost, R., et al. (2006). The cerebrocortical response to hyperinsulinemia is reduced in overweight humans: a magnetoencephalographic study. Proc. Natl. Acad. Sci. U.S.A. 103, 12103–12108. doi: 10.1073/pnas.0604404103
Tuck, M. L., Stec, D. E., Willis, M. A., Silva, A. A., and da Hall, J. E. (1992). Obesity, the sympathetic nervous system, and essential hypertension. Hypertens 19(1 Suppl.), I67–I77. doi: 10.1161/01.HYP.19.1_Suppl.I67
Tups, A., Benzler, J., Sergi, D., Ladyman, S. R., and Williams, L. M. (2017). Central regulation of glucose homeostasis. Compr. Physiol. 7, 741–764. doi: 10.1002/cphy.c160015
Tuulari, J. J., Karlsson, H. K., Antikainen, O., Hirvonen, J., Pham, T., Salminen, P., et al. (2016). Bariatric surgery induces white and grey matter density recovery in the morbidly obese: a voxel-based morphometric study. Hum. Brain Mapp. 37, 3745–3756. doi: 10.1002/hbm.23272
Urayama, A., and Banks, W. A. (2008). Starvation and triglycerides reverse the obesity-induced impairment of insulin transport at the blood-brain barrier. Endocrinology 149, 3592–3597. doi: 10.1210/en.2008-0008
Ustione, A., Piston, D. W., and Harris, P. E. (2013). Minireview: dopaminergic regulation of insulin secretion from the pancreatic islet. Mol. Endocrinol. 27, 1198–1207. doi: 10.1210/me.2013-1083
van Bloemendaal, L., Veltman, D. J., Ten Kulve, J. S., Groot, P. F. C., Ruhé, H. G., Barkhof, F., et al. (2015). Brain reward-system activation in response to anticipation and consumption of palatable food is altered by glucagon-like peptide-1 receptor activation in humans. Diabetes Obes. Metab. 17, 878–886. doi: 10.1111/dom.12506
Van Heek, M., Compton, D. S., France, C. F., Tedesco, R. P., Fawzi, A. B., Graziano, M. P., et al. (1997). Diet-induced obese mice develop peripheral, but not central, resistance to leptin. J. Clin. Invest. 99, 385–390. doi: 10.1172/JCI119171
Vidarsdottir, S., Smeets, P. A. M., Eichelsheim, D. L., Van Osch, M. J. P., Viergever, M. A., Romijn, J. A., et al. (2007). Glucose ingestion fails to inhibit hypothalamic neuronal activity in patients with type 2 diabetes. Diabetes 56, 2547–2550. doi: 10.2337/db07-0193
Vozarova, B., Weyer, C., Hanson, K., Tataranni, P. A., Bogardus, C., and Pratley, R. E. (2001). Circulating interleukin-6 in relation to adiposity, insulin action, and insulin secretion. Obes. Res. 9, 414–417. doi: 10.1038/oby.2001.54
Wallenius, K., Wallenius, V., Sunter, D., Dickson, S. L., and Jansson, J. O. (2002). Intracerebroventricular interleukin-6 treatment decreases body fat in rats. Biochem. Biophys. Res. Commun. 293, 560–565. doi: 10.1016/S0006-291X(02)00230-9
Wallner-Liebmann, S., Koschutnig, K., Reishofer, G., Sorantin, E., Blaschitz, B., Kruschitz, R., et al. (2010). Insulin and hippocampus activation in response to images of high-calorie food in normal weight and obese adolescents. Obesity 18, 1552–1557. doi: 10.1038/oby.2010.26
Wang, Q., Liu, C., Uchida, A., Chuang, J. C., Walker, A., Liu, T., et al. (2013). Arcuate AgRP neurons mediate orexigenic and glucoregulatory actions of ghrelin. Mol. Metab. 3, 64–72. doi: 10.1016/j.molmet.2013.10.001
Wester, V. L., Staufenbiel, S. M., Veldhorst, M. A., Visser, J. A., Manenschijn, L., Koper, J. W., et al. (2014). Long-term cortisol levels measured in scalp hair of obese patients. Obesity 22, 1956–1958. doi: 10.1002/oby.20795
Wilsey, J., and Scarpace, P. J. (2004). Caloric restriction reverses the deficits in leptin receptor protein and leptin signaling capacity associated with diet-induced obesity: role of leptin in the regulation of hypothalamic long-form leptin receptor expression. J. Endocrinol. 181, 297–306. doi: 10.1677/joe.0.1810297
Windham, B. G., Fumagalli, S., Ble, A., Sollers, J. J., Thayer, J. F., Najjar, S. S., et al. (2012). The relationship between heart rate variability and adiposity differs for central and overall adiposity. J. Obes. 2012:149516. doi: 10.1155/2012/149516
Woodside, B. (2007). Prolactin and the hyperphagia of lactation. Physiol. Behav. 91, 375–382. doi: 10.1016/j.physbeh.2007.04.015
Wulsin, L. R., Horn, P. S., Perry, J. L., Massaro, J. M., and D’Agostino, R. B. (2015). Autonomic imbalance as a predictor of metabolic risks, cardiovascular disease, diabetes, and mortality. J. Clin. Endocrinol. Metab. 100, 2443–2448. doi: 10.1210/jc.2015-1748
Wulsin, L. R., Horn, P. S., Perry, J. L., Massaro, J. M., and D’Agostino, R. B. (2016). The contribution of autonomic imbalance to the development of metabolic syndrome. Psychosom. Med. 78, 474–480. doi: 10.1097/PSY.0000000000000290
Wynne, K., Park, A. J., Small, C. J., Meeran, K., Ghatei, M. A., Frost, G. S., et al. (2006). Oxyntomodulin increases energy expenditure in addition to decreasing energy intake in overweight and obese humans: a randomised controlled trial. Int. J. Obes. 30, 1729–1736. doi: 10.1038/sj.ijo.0803344
Wynne, K., Park, A. J., Small, C. J., Patterson, M., Ellis, S. M., Murphy, K. G., et al. (2005). Subcutaneous oxyntomodulin reduces body weight in overweight and obese subjects: a double-blind, randomized, controlled trial. Diabetes 54, 2390–2395. doi: 10.2337/diabetes.54.8.2390
Yadav, R. L., Yadav, P. K., Yadav, L. K., Agrawal, K., Sah, S. K., and Islam, M. N. (2017). Association between obesity and heart rate variability indices: an intuition toward cardiac autonomic alteration – a risk of CVD. Diabetes Metab. Syndr. Obes. Targets Ther. 10, 57–64. doi: 10.2147/DMSO.S123935
Yi, C. X., la Fleur, S. E., Fliers, E., and Kalsbeek, A. (2010). The role of the autonomic nervous liver innervation in the control of energy metabolism. Biochim. Biophys. Acta 1802, 416–431. doi: 10.1016/j.bbadis.2010.01.006
Yi, S. H., Lee, K., Shin, D. G., Kim, J. S., and Kim, H. C. (2013). Differential association of adiposity measures with heart rate variability measures in Koreans. Yonsei Med. J. 54, 55–61. doi: 10.3349/ymj.2013.54.1.55
Yoo, H. J., Hwang, S. Y., Choi, K. M., Baik, S. H., Lee, E. M., Kim, E. J., et al. (2016). Clinical implication of body size phenotype on heart rate variability. Metabolism 65, 1589–1596. doi: 10.1016/j.metabol.2016.08.002
Young, J. B., Troisi, R. J., Weiss, S. T., Parker, D. R., Sparrow, D., and Landsberg, L. (1992). Relationship of catecholamine excretion to body size, obesity, and nutrient intake in middle-aged and elderly men. Am. J. Clin. Nutr. 56, 827–834. doi: 10.1093/ajcn/56.5.827
Yu, X., Park, B.-H., Wang, M.-Y., Wang, Z. V., and Unger, R. H. (2008). Making insulin-deficient type 1 diabetic rodents thrive without insulin. Proc. Natl. Acad. Sci. U.S.A. 105, 14070–14075. doi: 10.1073/pnas.0806993105
Zanchi, D., Depoorter, A., Egloff, L., Haller, S., Mählmann, L., Lang, U. E., et al. (2017). The impact of gut hormones on the neural circuit of appetite and satiety: a systematic review. Neurosci. Biobehav. Rev. 80, 457–475. doi: 10.1016/j.neubiorev.2017.06.013
Zhang, X., Zhang, G., Zhang, H., Karin, M., Bai, H., and Cai, D. (2008). Hypothalamic IKKβ/NF-κB and ER stress link overnutrition to energy imbalance and obesity. Cell 135, 61–73. doi: 10.1016/j.cell.2008.07.043
Zhang, Y., Ji, G., Xu, M., Cai, W., Zhu, Q., Qian, L., et al. (2016). Recovery of brain structural abnormalities in morbidly obese patients after bariatric surgery. Int. J. Obes. 40, 1558–1565. doi: 10.1038/ijo.2016.98
Zhu, G., Yan, J., Smith, W. W., Moran, T. H., and Bi, S. (2012). Roles of dorsomedial hypothalamic cholecystokinin signaling in the controls of meal patterns and glucose homeostasis. Physiol. Behav. 105, 234–241. doi: 10.1016/j.physbeh.2011.08.007
Keywords: CNS, hypothalamus, glucose, regulation, fMRI, neuroimaging, neuroendocrine, autonomic nervous system
Citation: Lundqvist MH, Almby K, Abrahamsson N and Eriksson JW (2019) Is the Brain a Key Player in Glucose Regulation and Development of Type 2 Diabetes? Front. Physiol. 10:457. doi: 10.3389/fphys.2019.00457
Received: 04 December 2018; Accepted: 01 April 2019;
Published: 26 April 2019.
Edited by:
Chris Rayner, The University of Adelaide, AustraliaReviewed by:
Cristina M. Sena, University of Coimbra, PortugalIlkka H. A. Heinonen, University of Turku, Finland
Copyright © 2019 Lundqvist, Almby, Abrahamsson and Eriksson. This is an open-access article distributed under the terms of the Creative Commons Attribution License (CC BY). The use, distribution or reproduction in other forums is permitted, provided the original author(s) and the copyright owner(s) are credited and that the original publication in this journal is cited, in accordance with accepted academic practice. No use, distribution or reproduction is permitted which does not comply with these terms.
*Correspondence: Martin H. Lundqvist, bWFydGluLmx1bmRxdmlzdEBtZWRzY2kudXUuc2U= Jan W. Eriksson, amFuLmVyaWtzc29uQG1lZHNjaS51dS5zZQ==