- Department of Stomatology, Union Hospital, Tongji Medical College, Huazhong University of Science and Technology, Wuhan, China
Circadian rhythms (CR) are a series of endogenous autonomous oscillators generated by the molecular circadian clock which acting on coordinating internal time with the external environment in a 24-h daily cycle. The circadian clock system is a major regulatory factor for nearly all physiological activities and its disorder has severe consequences on human health. CR disruption is a common issue in modern society, and researches about people with jet lag or shift works have revealed that CR disruption can cause cognitive impairment, psychiatric illness, metabolic syndrome, dysplasia, and cancer. In this review, we summarized the synchronizers and the synchronization methods used in experimental research, and introduced CR monitoring and detection methods. Moreover, we evaluated conventional CR databases, and analyzed experiments that characterized the underlying causes of CR disorder. Finally, we further discussed the latest developments in understanding of CR disruption, and how it may be relevant to health and disease. Briefly, this review aimed to synthesize previous studies to aid in future studies of CR and CR-related diseases.
Introduction
Circadian rhythms (CR) are endogenous autonomous oscillators of physiological activities resulting 24-h day/night cycles, which allow organisms to adapt to a fluctuating environment (Reppert and Weaver, 2002; Dibner et al., 2010). The core pacemaker of CR lies in the SCN, which plays crucial roles in maintenance of systemic CR and regulates peripheral tissue clocks through secretion of endogenous regulatory factors (Dibner et al., 2010). The molecular clock of the CR system, which is present in all cells, is made up of oscillating clock-related proteins that compose TTFLs (Gekakis et al., 1998). The core TTFL is composed of the transcriptional activator proteins CLOCK and BMAL1, and the repressor proteins Period-1 (PER1), PER2, PER3, Cryptochrome-1 (CRY1) and CRY2 (Gekakis et al., 1998). Other loops are coupled to the core TTFL to maintain oscillation. The first sub-loop is composed of RORs and nuclear REV-ERB receptors. The second sub-loop comprises D-box-related genes, which include DBP, TEF, and HLF (Figure 1; Preitner et al., 2002; Sato et al., 2004). Moreover, recent studies have suggested that circadian regulation is heavily involved in gene expression. A considerable portion (approximately 10%) of genes expressed in cells or tissues have been found to display circadian oscillations, resulting in identification of these genes as “CCGs” (Duffield, 2003; Zhang et al., 2014).
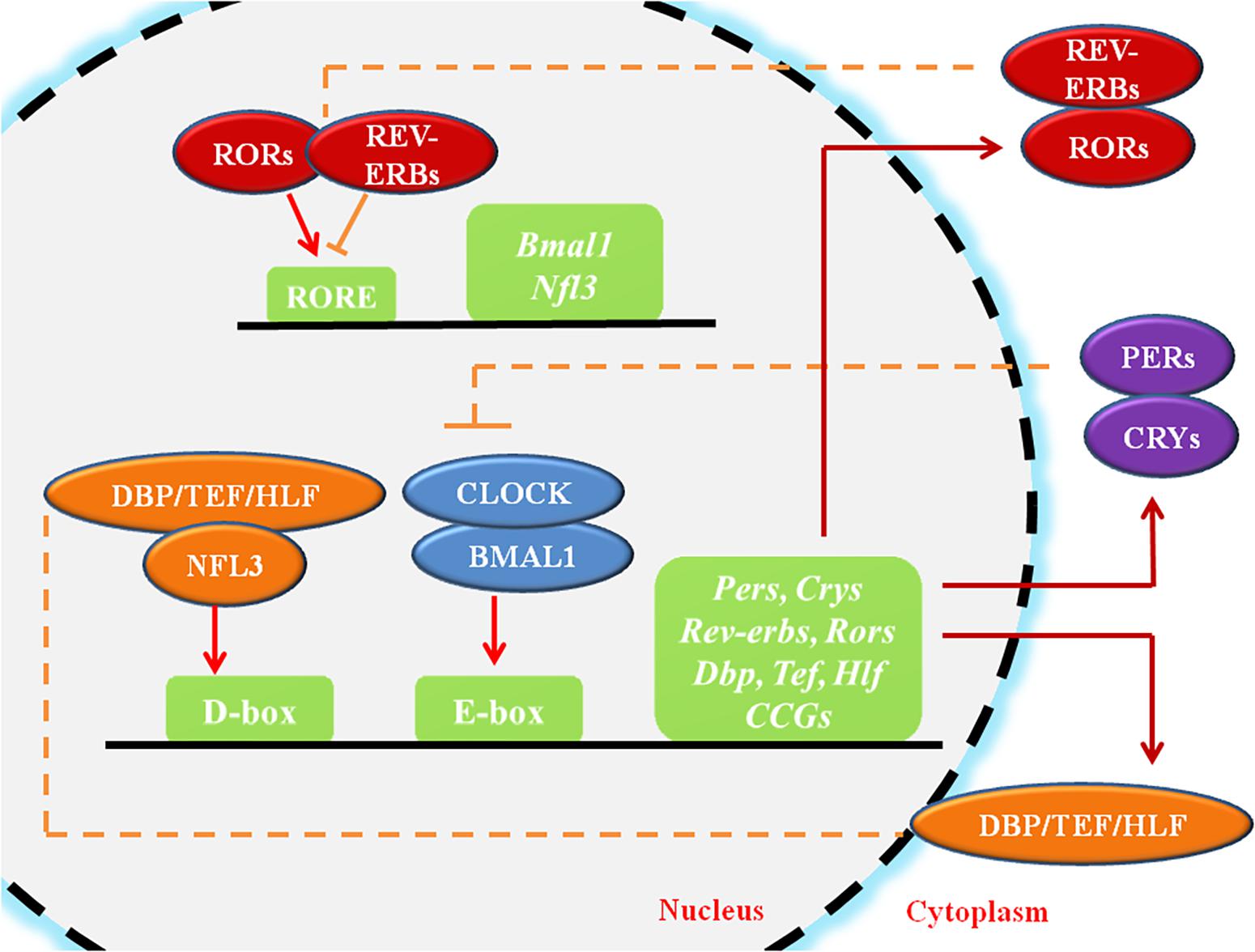
Figure 1. The molecular mechanism of circadian rhythms. CLOCK and BMAL1 activate the cis-acting element E-box to initiate the transcription of downstream genes such as Pers and Crys, while the accumulated PER and CRY proteins, in turn, bind to CLOCK/BMAL1 and switch them from an activated state to an inhibited state, suppressing the transcriptional activation of downstream genes. ROR/REV-ERB and DBP (TEF, HLF)/E4BP4, acting on other cis-acting elements such as RORE and D-box, participating in the regulation of the core feedback loop. CCGs refer to the clock-controlled genes. The circles represent proteins, the squares represent genes or clock-related elements, the red arrows represent transcriptional activation, and the orange lines with horizontal bars represent transcriptional inhibition.
Mammalian tissues and cells have an autonomous circadian oscillator with a period of roughly 24 h. External stimuli are essential for maintaining the appropriate circadian oscillations (Bass and Takahashi, 2010; Roenneberg and Merrow, 2016; Tahara et al., 2017). In vivo, CR is mainly entrained by environmental signals such as light, food, and arousal stimuli. In the SCN, the circadian clock mainly responds to the LD cycle. In peripheral tissues, CR can be synchronized by food or temperature (Damiola et al., 2000; Hara et al., 2001; Buhr et al., 2010). Moreover, internal signals such as circulating hormones, cytokines, metabolites, sympathetic nervous activation, and body temperature are significant timing cues that regulate peripheral clocks (Albrecht, 2012; Menaker et al., 2013). In vitro, CR are difficult to observe due to lack of SCN signals. As such, external stimuli should be applied to induce CR in cultured cells or explants (Figure 2).
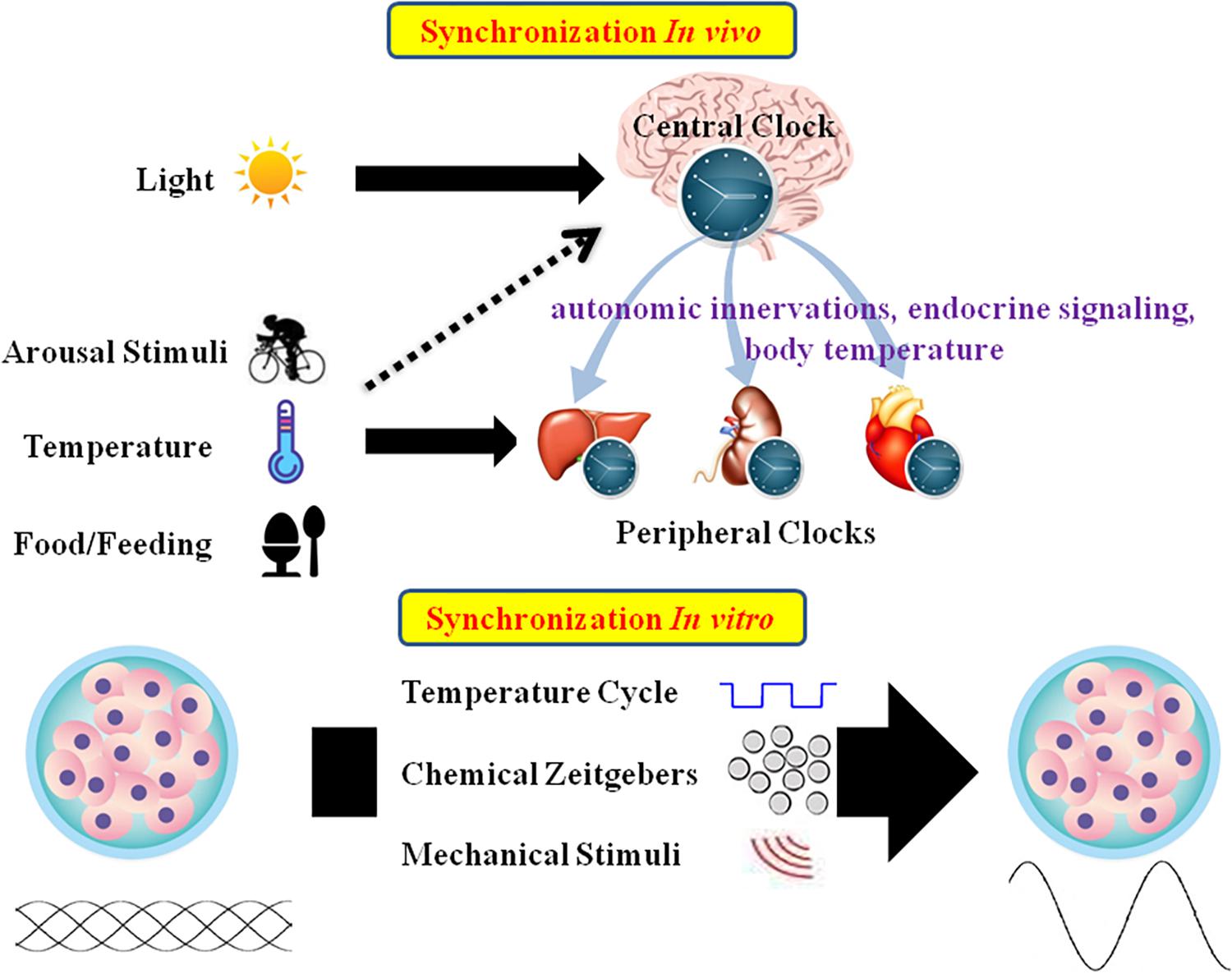
Figure 2. Schematic summary of in vivo and in vitro circadian synchronization. In vivo, the photic zeitgeber mainly entrains the central clock, which regulates the peripheral clocks through the internal timing cues including autonomic innervations, endocrine signaling and body temperature; the non-photic zeitgebers including arousal stimuli, temperature and food mainly entrain the peripheral clocks. In vitro, the circadian oscillations of cells or explants can be synchronized by temperature cycles, chemical factors (such as Dex, Fsk, or horse serum) and mechanical stimuli.
The field of chronobiology requires detection of features of CR in tissues or cells. Through chronological collection or luminescence monitoring, the basic data resulting from circadian fluctuations in vivo or in vitro can be accurately recorded. Approaches such as FFT (Moore et al., 2014), JTK-Cycle (Hughes et al., 2010), or autocorrelation (Levine et al., 2002) can be used to analyze the rhythmic features of CR. Subsequently, we introduced several circadian databases established in recent years.
Finally, we summarized in vivo and in vitro factors that can alter CR. In vivo factors include entrainments such as light, food, and temperature, while the in vitro factors include cell density (Noguchi et al., 2013), osmotic pressure, PH, mechanical stimulus, temperature, oxygen concentration, and microorganisms (Haspel et al., 2014). These factors can lead to circadian disruptions, and result in various diseases such as cancer, dysplasia, and metabolic and behavioral disorders (Takahashi et al., 2008).
The Mammalian Circadian Clock System
The circadian clock system is the totality of all oscillators in organisms coupled to various physiological processes. This system generally consists of three parts in mammals including the input pathway, the core circadian clock, and the output pathway. The input pathway senses external timing signals, for example, light/dark, and sends information to the core circadian clock. The core circadian clock forms endogenous CR according to external time cues to allow for adaptation to the environment. Based on changes in the core circadian clock, the output pathway adjusts the physiological activities in various tissues and organs through neurohumoral regulation.
In mammals, the core pacemaker of the CR system exists in the SCN, which exhibits endogenous rhythmic oscillations both at the tissue and cell levels, plays a vital role in maintenance and alterations of CR, and provides outputs to peripheral tissues after synchronization by external time cues (Dibner et al., 2010). The SCN can be distinguished into two main areas: the VL core area and the DM shell area (Welsh et al., 2010). The core area mainly expresses the neuropeptide VIP, while the shell area expresses AVP. The VL-SCN, which controls essential physiological activities such as exercise, body temperature, heart rate, and hormone synthesis, serves to couple the circadian system. VIP is released periodically from the VL core region, binding to VPAC2 on the neuronal surface, resulting in cell depolarization and induction of PER1 and PER2 (Stepanyuk et al., 2014). VIP deficiency impairs synchronization of the cells, leading to weakening of whole-body rhythms (Loh et al., 2011). AVP-deficient rats exhibit weakened rhythms, but do not experience changes in circadian pacemaking. As such, the AVP-SCN is considered to function as the output (Mieda, 2019). In addition to VIP and AVP, other neurotransmitters such as glutamate and GABA, which are present in the SCN, conduce to regulate areas upstream and downstream of the circadian clock system (Morin et al., 2006). The SCN has historically been considered to be the only endogenous circadian clock with autonomous oscillations, and peripheral clocks were not believed to spontaneously oscillate, but could oscillate under pacing by the SCN. However, studies have demonstrated that the peripheral clocks also have the ability to oscillate autonomously and keep their internal rhythms (Balsalobre et al., 1998; Yoo et al., 2004). The predominant theory is that the SCN, as the master pacemaker, can drive peripheral clock rhythms (Dibner et al., 2010). Peripheral clocks are under the regulation of endogenous regulatory factors from the SCN. In an organized CR system, the connection between the SCN and peripheral tissues is a complex network, and the gene expression patterns of peripheral tissues are under the control of various complex factors including autonomic innervations, endocrine signaling, temperature, and local signals (Figure 2; Mohawk et al., 2012).
Within cells, the CR system regulates cell biological behaviors either directly through the TTFLs or indirectly (Figure 1). The core TTFL contains two transcription activators, CLOCK and BMAL1, which generate a heterodimer, bind to the cis-acting element E-box (5′-CACGTG-3′), and activates transcription of the PER and CRY genes at the beginning of the cycle (Gekakis et al., 1998). After several hours, PER and CRY proteins accumulate, dimerize, and generate a complex which translocates to the nucleus and inhibits the CLOCK/BMAL1 heterodimer (Xu et al., 2015). Other loops are coupled to the core TTFL to complete the oscillation. The first sub-loop comprises RORs and REV-ERB, which directly targets at CLOCK/BMAL1 or ROREs (Preitner et al., 2002; Sato et al., 2004). The second CLOCK/BMAL1-driven sub-loop contains the PAR-bZip factors DBP, TEF, and HLF. The repressor NFIL3 (or E4BP4), driven by the REV-ERB/ROR loop, interacts with these proteins at sites containing D-boxes (Mitsui et al., 2001; Gachon et al., 2004; Takahashi, 2017). These three interlocking TTFLs form 24-h cycles of transcription with diverse expression phases, resting with the combination of cis-elements (E-box, RORE, D-box) in the promoters and enhancers of specific CCGs.
Circadian Rhythm Synchronizers
Nearly every eukaryote has a cell-autonomous circadian clock which exhibits 24-h physiological oscillations and can be influenced by external timing cues. These external timing cues, also called “synchronizers,” “zeitgeber,” or ”entraining agents” can reset the body’s circadian clock and place all cells at the same phase of circadian oscillation, a process called circadian rhythm synchronization. The word “ZT” is used to describe external cues that synchronize CR such as LD cycles or temperature cycles, and the word “CT” is utilized to describe timing without external signals (Li et al., 2017). In the field of chronobiology research, whether in vivo or in vitro, researchers use these entraining agents to synchronize CR of the experimental animals or cells. A variety of factors which act as synchronizers are summarized in Figure 2.
Light
Most organisms acquire the time information through changing light intensity throughout the day to reset their own clock, referred to as “photic entrainment” (Roenneberg and Foster, 1997). In chronobiology experiments, light is often served as a stimulus to induce responses from the circadian clock. The light signaling cascades that entrain the circadian clock are fairly complex. In vivo, pRGCs receive light information, which is transmitted to the SCN directly through the RHT (Hattar et al., 2002; Ishida et al., 2005; Kalsbeek et al., 2006; van Diepen et al., 2015; Pilorz et al., 2016; Mouland et al., 2017; Astiz et al., 2019), resulting in regulation of the peripheral clocks by the SCN via secreting neurohumoral factors (Mohawk et al., 2012). The light-sensitive photopigment melanopsin (Provencio et al., 2000), is expressed in the pRGCs which mediate a series of responses to light (Hankins et al., 2008; Do and Yau, 2010; Schmidt et al., 2011). Stimulation of melanopsin causes activation of G protein coupled signaling cascades and the PLC pathway, leading to opening of TRPCs such as TRPC6 and TRPC7, Ca2+ influx, and cellular depolarization (Poletini et al., 2015). The input pathway to the SCN mainly depends on the monosynaptic RHT. The primary neurotransmitters in the RHT are glutamate, PACAP, SP, and aspartate (Ebling, 1996; Chen et al., 1999; Hannibal, 2002; Golombek et al., 2003; Hannibal and Fahrenkrug, 2004; Fahrenkrug, 2006). Glutamate, which binds to glutamatergic receptors such as NMDA or AMPA receptors, is the main signal for photic entrainment (Mikkelsen et al., 1993; Golombek and Ralph, 1996; Chambille, 1999; Peytevin et al., 2000; Michel and Colwell, 2001; Paul et al., 2005), and induces increased intracellular Ca2+ concentrations (Kim et al., 2005). PACAP, which activates PAC1 receptor, is also responsible for mediating synchronization to light (Hannibal et al., 1998, 2008; Shen et al., 2000; Colwell et al., 2004; Hashimoto et al., 2006). Ca2+ influx activates a range of kinases including PKA, MAPK, CaMK, PKCA, and PKG (Meijer and Schwartz, 2003). These kinases are involved in phosphorylation of CREB, which binds to cAMP response elements in promoters, resulting in transcription of clock genes such as PER1 and PER2 (Meijer and Schwartz, 2003). Moreover, transcription factors such as c-FOS (Travnickova-Bendova et al., 2002) and EGR1 (Riedel et al., 2018) also participate in regulation of the SCN clock through optical signals.
Based on RHT innervation and the neurochemical natures of the cells, the SCN is distinguished into two main subdivisions: the VL area (core area) and the DM area (shell area) (Moore et al., 2002). The VL-SCN, which contains VIP and gastrin-releasing peptide, is located above the optic chiasm, and receives most of its photic input from RHT innervation. In contrast, the DM-SCN region, where neurons contain AVP and calretinin, receives mostly neural signals from the hypothalamus, limbic areas, and the VL-SCN (Golombek and Rosenstein, 2010). VL-SCN neurons respond to photic stimuli during the subjective night and communicate with DM-SCN neurons through several neurotransmitters such as VIP, GRP, and SP (Best et al., 1999; Berson et al., 2002), resulting in synchronization of the DM-SCN to the expression of these proteins. Therefore, DM-SCN neurons depend on neuropeptide release from the VL-SCN, although they show stronger autonomous oscillations (Moga and Moore, 1997; Meijer and Schwartz, 2003; Antle and Silver, 2005).
Arousal Stimuli
Other stimuli can synchronize CR, resulting in “non-photic entrainment.” Arousal stimuli are non-photic entrainments which include social interactions, exercise, restraint stress, and caffeine-induced arousal (Mistlberger and Skene, 2004). Different species respond differently to arousal stimuli. For example, during the daytime, wheel running or gentle handling cause a robust phase advance in locomotor activity rhythms of Syrian hamsters, whereas these stimuli do not arouse a similar phase advance in the behavioral rhythms of mice or rats (Antle and Mistlberger, 2000; Mistlberger et al., 2002). However, phase advance in peripheral clocks such as liver and kidney can be induced by restraint stress or running wheel exercise during the intermediate of the light phase in mice (Tahara et al., 2015; Sasaki et al., 2016). These studies suggested that under LD cycles, peripheral tissues directly respond to behavioral entrainment without influencing the SCN. However, in constant darkness during projected daytime, behavioral rhythms of mice can also be observed under several-hour daily wheel stimulation (Mistlberger, 1991). These results imply that the master clock in mice is primarily impacted by light rather than arousal stimuli.
A review by Golombek and Rosenstein (2010) showed that arousal stimuli transmit to the SCN through two major pathways. The GHT from the thalamic IGL, employs NPY, GABA, and endorphins as neurotransmitters, and plays a role in activation of the Y2 receptor/PKC pathway. The other is the serotonergic median raphe nucleus projection to the SCN, where serotonergic effects are mediated by 5-HT1A/7 receptors and PKA activation (Golombek and Rosenstein, 2010). The mechanisms responsible for behavioral entrainment of peripheral clocks include physiological factors such as glucocorticoids, sympathetic nerves, oxidative stress, hypoxia, PH, cytokines, and temperature (Tahara and Shibata, 2018).
Food/Feeding
The CR of mammals can be synchronized by light, and thus the central clock for light response, the SCN, is considered a light-entrainable oscillator. Food is a non-photic stimulus that can reset the circadian rhythm. Many studies have confirmed the existence of a FEO, but the anatomical location(s) and molecular timekeeping mechanisms of the FEO have not been determined. In the case of mammalian research, food is provided and consumed within a few hours. This condition is called time-restricted feeding, or temporal food restriction, and is frequently used to study FAA (Challet et al., 1998). FAA refers to the output of the FEO, which appears under time-restricted feeding, but disappears under ad libitum access, and reoccurs during the following fasting (Pendergast and Yamazaki, 2018). Mice allowed access to a standard diet ad libitum typically ingest 60–80% of their daily food intake at night.
Feeding-fasting signals reset the circadian clocks in the peripheral tissues by causing periodic availability of many circulating macronutrients (Woods, 2005). For example, ingestion causes release of insulin into the blood and induces clock gene expression in insulin-sensitive tissues (such as liver, adipose, and muscle) (Oike et al., 2014). Feeding also enhances blood glucose levels, and high glucose concentrations can down-regulate the expression of PER1 and PER2 in fibroblasts (Hirota et al., 2010), and indirectly regulate AMPK, which controls the stability of CRYs (Lamia et al., 2009). Recent evidence showed that the liver may be a communication center of the FAA synchronization signal. Under time-restricted feeding, the liver increased βOHB production through regulation of Cpt1a and Hmgcs2 by Per2, which was then used as a signal to cause animals to anticipate feeding time (Chavan et al., 2016). In addition, food restriction may alter the anabolic/catabolic cycles of tissues, which may affect the cellular redox state (Rutter et al., 2002) and further influence the circadian feedback loop (Mendoza, 2007). For instance, NADH and NADPH, classical cofactors for intracellular redox reactions, can promote binding of CLOCK/BMAL1 and NPAS2/BMAL1 dimers to DNA, while their oxidized forms, NAD(P)+, inhibit this binding (Rutter et al., 2001, 2002; Schibler and Naef, 2005). Furthermore, restricted feeding may influence body temperature cycles, which have been confirmed to entrain peripheral clocks (Brown et al., 2002).
Although the central oscillators are not affected by temporal food restriction during the light phase of the day (Damiola et al., 2000; Stokkan et al., 2001), SCN-derived physiological rhythms such as locomotor activity and body temperature can be entrained by caloric restriction (also called hypocaloric diet, characterized by caloric intake reduction to 60% of the animal’s normal daily food intake) under LD cycles (Challet et al., 1997) or time-restricted feeding in constant darkness (Holmes and Mistlberger, 2000). Although the mechanisms by which caloric restriction affects the SCN clock has not been characterized, previous studies suggested that receptors of metabolism-related hormones such as insulin, leptin, and ghrelin, which exist in SCN cells, may involve in synchronizing the SCN (Guan et al., 1997; Zigman et al., 2006). Moreover, feeding regulates brain structures that project straightly to the SCN such as the DM hypothalamus and the arcuate nucleus through orexin and ghrelin (Akiyama et al., 2004; Mieda et al., 2004; LeSauter et al., 2009; Moriya et al., 2009; Acosta-Galvan et al., 2011). Moreover, glucose influences the neural activity phase in vitro SCN slices (Hall et al., 1997). Further researches are required to evaluate the involvement of these factors and their probable impacts on the SCN.
Temperature
Temperature is a non-photic synchronizer with a weaker synchronization effect than that of light. Roberto Refinetti found that only 60–80% mice can be synchronized by ambient temperature cycles, and stable entrainment takes longer in response to temperature cycles than LD cycles (Refinetti, 2010). Though multiple physiological processes rely on ambient temperature, the CR system has a significant feature called temperature compensation, in which circadian oscillations remain resistant to temperature changes, resulting in the period length still maintaining approximately 24 h despite ambient temperature changes (Isojima et al., 2009; Narasimamurthy and Virshup, 2017). In addition, the SCN clock does not respond to temperature stimuli, whereas cells and tissues outside of the SCN can be synchronized by temperature fluctuations (Brown et al., 2002; Buhr et al., 2010; Ohnishi et al., 2014). The reason why the SCN is resistant to temperature changes could be that the circadian clocks in the SCN cells have robust couplings. After uncoupling of SCN cells using tetrodotoxin or nimodipine, temperature sensitivity was detected (Buhr et al., 2010). Because temperature is a weak synchronizer, it is not typically used as a zeitgeber in animal experiments.
The phase shift of the clock in response to simulated body temperature fluctuations has been demonstrated in vitro (Brown et al., 2002; Buhr et al., 2010; Saini et al., 2012; Dudek et al., 2017), and the amplitude of circadian gene expression was enhanced by temperature cycles (Dibner et al., 2009; Sporl et al., 2011; Dudek et al., 2017). Mammalian cells sense temperature fluctuations through a series of temperature-stimulated TRP channel subfamily members called thermo-TRPs, each of which is activated in a narrow thermal range (Poletini et al., 2015). The intrinsic mechanism of temperature effects on CR may be mediated by HSF1 and CIRBPs (Ki et al., 2015). HSF1 is a circadian transcription factor which binds the heat shock element sequence, causing circadian activation of downstream promoters such as Per2 (Kornmann et al., 2007; Reinke et al., 2008; Tamaru et al., 2011). CIRBP-mediated post-transcriptional regulation allows high-amplitude clock genes express, including that of the core clock gene CLOCK (Ki et al., 2015).
Chemical Factors
In vitro experiments lack the humoral and neuronal factors that can act as entrainments in vivo. As such, cultured cells or explants are desynchronized and circadian oscillations are absent. Thus, to observe endogenous oscillations of cells or explants in vitro, chemical factors with similar effects to in vivo entrainments are required.
Many chemical factors can function as synchronizers. It has been reported that glucocorticoid, a kind of anti-inflammatory hormones released by the adrenal cortex, serves as a vigorous synchronizer of peripheral tissues (Balsalobre et al., 2000a, b; Yamamoto et al., 2005; Segall et al., 2006; So et al., 2009; Cheon et al., 2013). Dex, an artificial glucocorticoid, exposure for 1 h can be used to restart the oscillations of circadian clock genes (Balsalobre et al., 2000b; Wu et al., 2008). Glucocorticoids activate GRs, which bind to the GREs on the promoters of core clock genes such as Per1, Per2, and E4bp4, thereby directly activating the core clock cycle, or by activating the transcription factor HNF4alpha, which targets downstream rhythmic genes without GRE elements (Reddy et al., 2007; Cheon et al., 2013). Dex cannot be used for SCN synchronization, because the SCN does not express GRs.
A study performed in 1998 indicated that serum shock could induce the rhythmic expression of Perl, Per2, Reverb-α, Dbp, and Tef in cultured rat fibroblasts (Balsalobre et al., 1998). Thereafter, serum shock was used to synchronize the circadian oscillations of various tissues, immortalized cells, and fibroblast cells. This study suggested that various factors in the blood could stimulate rhythmic oscillations. Previous reports showed that various factors such as such as EGF (Izumo et al., 2006), calcium (Balsalobre et al., 2000b), glucose (Hirota et al., 2002), PGE2 (Tsuchiya et al., 2005), 1α,25-dihydroxyvitamin D3 (Gutierrez-Monreal et al., 2014), and atomoxetine (O’Keeffe et al., 2012) can synchronize clock genes. Moreover, serum shock can induce Ser/Thr phosphorylation of CLOCK through the Ca2+-dependent PKC pathway (Shim et al., 2007), or activate the p42/44 MAPK pathway in a manner similar to that observed in response to light pulses (Ginty et al., 1993; Yagita and Okamura, 2000), which may cause resetting of the clock. In addition, a previous report showed that blood-borne signals activate GTPase RhoA, which promotes G-actin polymerization into F-actin, resulting in release of MRTFs into the nucleus, thus regulating the transcription of clock genes (Esnault et al., 2014).
Another common chemical synchronizing agent, Fsk, has a similar mechanism to that of serum. Fsk directly activates AC, which activates CREB through phosphorylation by promoting the synthesis of cAMP and activating PKA indirectly (Yagita and Okamura, 2000).
Mechanical Stimuli and Oxidative/ Hypoxia Stress
A recent study showed that intermittent uniaxial stretching of bone marrow-derived mesenchymal stem cells, DPSCs, and adipose tissue-derived mesenchymal stem cells can reset their CR, resulting in a synchronization effect similar to that observed in response to Dex treatment (Rogers et al., 2017). Changing media also could reset cellular CR (Yeom et al., 2010; Guenthner et al., 2014). Mechanical stimuli provide researchers with alternate mechanisms to reset the circadian clocks of cells, such as DPSCs, which cannot be synchronized by other methods. However, the mechanisms by which mechanical stimuli induced synchronization are still unclear. It might be involved the RhoA pathway, by which short-duration fluid shear force can induce changes expression levels of clock genes in osteoblasts (Hamamura et al., 2012).
Oxidative or hypoxic stimuli may lead to circadian clock entrainment. In vitro, oxidative stimulation via hydrogen peroxide synchronizes cellular circadian oscillations in dose- and time-dependent manners (Tamaru et al., 2013). In vivo, phase shifts in peripheral clocks such as those in the kidney and liver are observed after hydrogen peroxide injection (Geerdink et al., 2016). However, whether the master clock responds to oxidative stress is unknown. Oxygen cycles (12-h 5%:12-h 8%) synchronize cellular clocks via a key transcription factor in cultured fibroblasts, HIF1α, which has similar a similar PAS domain to CLOCK and BMAL1 (Adamovich et al., 2017). Furthermore, at the onset of a 6-h-shifted dark period, hypoxic stimulation (14% O2) for 2 h advanced phases of locomotor activities in response to a new LD cycle (Adamovich et al., 2017). These results suggested that both central and peripheral oscillations can be reset by hypoxic stimulation. Taken together, oxygen signals may induce circadian synchronization in vitro.
Common Synchronization Methods Used in Lab
Synchronization in vivo
Light Stimuli
Most researchers choose light to synchronize CR in animals. Most studies utilize a rectangular LD cycle, which includes sudden transitions from light/dark to dark/light. Under laboratory condition, the 24-h LD cycle can be represented in various ways, such as a short photoperiod (shorter than 12-h light per day, e.g., 6L:18D), an equinox photoperiod (12-h light per day, i.e., 12L:12D), and a long photoperiod (longer than 12-h light per day, e.g., 18L: 6D). In nature, however, the transition between light and dark is not abrupt, and is characterized by gradual changes in light intensity and spectral composition. Mimicking natural conditions as closely as possible requires a light transition time (usually 0–2 h) in which the log of light intensity increases (or decreased) over time from 0 to 100 lux, and these changes can be implemented at any point during the transition from light to dark or dark to light. Different photoperiods lead to different waveforms of the SCN population rhythm. In SCN, short photoperiods result in waveforms that are slim and high-amplitude, while long photoperiods lead to the wide and low-amplitude waveforms (Inagaki et al., 2007; VanderLeest et al., 2007). Increasing the photoperiod compresses the activity phase of mice, but this effect is weakened by a long twilight duration (2-h) (Comas and Hut, 2009). In addition, light intensities impact the phase angle of entrainment and the velocity of re-synchronization to a new LD cycle. Specifically, low light intensity results in a longer phase angle (Wright et al., 2005), decreasing the rate of re-entrainment. If light intensity is insufficient, entrainment may fail (Leloup and Goldbeter, 2013).
An equinox photoperiod is used to study normal CR in animals. However, sometimes the 16L:8D cycle is used for mice because the locomotor period of mice is usually longer than 12 h (Albrecht and Foster, 2002). Furthermore, to observe the autonomous rhythms of animals, constant darkness (i.e., dark-dark, or DD condition) after a stable entrainment of 7–10 days in the LD cycle is required to avoid the effects of light (Albrecht and Foster, 2002). However, genetically engineered mice with no functional clock such as Per1/Per2 and the Cry1/Cry2 double-mutant mice (van der Horst et al., 1999; Zheng et al., 2001) seem to lose circadian rhythmicity in DD conditions, but show obvious rhythmic activity under LD conditions. The condition in which rhythmicity is induced by the LD cycle but not by the endogenous clock is called masking (Mrosovsky et al., 2001).
Longer light durations are used to affect the frequency of rhythms, whereas short light pulses are used to induce phasic entrainment of the clock (Daan, 1977; Roenneberg et al., 2003). The durations of short light pulse experiments are usually 15–30 min. Light pulses during the activity period of the mouse (subjective night) induce phase shift (advance or delay) of the clock, while light stimulus during the resting period (subjective day) alters the phase of the circadian clock (Johnson, 1999; Johnson et al., 2003). Use of this phenomenon allows for production of a phase response curve. In fact, using one or two light pulses to determine the frontiers of the subjective day and night of animals (also called “skeleton photoperiod”) can reduce negative masking (Lewy et al., 2001; Kennaway, 2004).
To study biological behaviors resulting from disrupted CR, jet lag protocols that advance the LD cycle (delaying may mask the onset of activity) can be used (Cao et al., 2013; Jagannath et al., 2013; Yamaguchi et al., 2013). In addition, constant light conditions can prolong the clock period compared to constant dark conditions, leading to a daily phase delay of activity in a light intensity-dependent manner. The sensitivity of these responses can be expressed as an IRC (Daan and Pittendrigh, 1976). Different wavelengths of light have different effects on phase, resulting in multiple IRCs that enable formation of an action spectrum (Peirson et al., 2005; Foster et al., 2007).
Time-Restricted Feeding
In addition to LD cycles, time-restricted feeding is used to examine the function of food intake timing on the circadian clock, and to synchronize peripheral oscillators, particularly in studies of metabolism-related tissues such as the liver, adipose, pancreas, and kidney. In these experiments, food access timing is restricted to between 2 and 12 h during the day or night. Within a few days, the rodents begin to predict the timing of food arrival and eat their meals during the period of food supply. If the period of food supply is less than 6 h, the animals cannot eat an equivalent amount of food compared to those allowed food ad libitum. When the period of food supply is over 8 h, the animals ingest nearly an equal amount of calories as those allowed food ad libitum. Thus, time-restricted feeding that allows greater than 8 h of access to food is an effective method to examine the role of food timing entrainment in response to changes in nutrition quality or quantity (Manoogian and Panda, 2017).
Because the synchronization effects in response to temperature and arousal stimuli are comparatively weaker than those in response to light, these factors are not commonly used in vivo experiments (unless the purpose is to study the synchronization effects of these two factors), but researchers should work to control for these factors to prevent interference with the experimental results.
Synchronization in vitro
In cultured cells or explants, chemical synchronization reagents such as Dex, Fsk, or 50% horse serum are commonly used to reset circadian oscillations. As previously discussed, Dex is unable to synchronize SCN cell lines or explants due to lack of GRs. Different synchronization reagents have different abilities to induce clock gene oscillations. Mariko Izumo et al. compared the synchronization effects of ten different compounds in Rat-1 fibroblasts, and found that horse serum, Dex, Fsk, and EGF induced the largest oscillatory amplitudes, among which Fsk and Dex induced the highest-amplitude rhythms (Izumo et al., 2006). In addition, Dex-induced rhythms reached the strongest amplitude 30 min after administration, faster than Fsk, and the amplitude of Dex-treated cells was consistently higher than that of Fsk (Izumo et al., 2006; Takarada et al., 2017). These discrepancies may have been by reason of differences in synchronization mechanisms (Izumo et al., 2006). In addition to these chemical synchronization approaches, other synchronization approaches should be considered under some circumstances. For instance, when synchronization reagents are not readily permeable (e.g., some dense explants or cells are grown in 3D materials), temperature cycles could be a good alternative. An example protocol is as follows. Cells or explants are cultured for 2–3 days with a square-wave temperature cycle of 12-h low temperature (35.5–36.5°C) and 12-h high temperature (38.5°C). After 2–3 days, when cells return to a constant temperature condition of 37°C (CT0), the rhythm of synchronized cells or explants can be monitored (Dibner et al., 2009; Sporl et al., 2011; Dudek et al., 2017; Yang et al., 2017; Williams et al., 2018). Similar to Dex, temperature cycles cannot be used to synchronize SCN oscillations because of robust couplings in the SCN. Newly discovered mechanical stimulation synchronization may also be useful, but specific instances where this is the case have not been identified.
Some other “synchronization” methods such as centrifugal washing and serum starvation (Banfalvi, 2017) are used to synchronize the cell cycle so that all cells are in the same phase of the cell cycle. The cell cycle is defined as the whole process from the end of last round of mitosis to the beginning of next round of mitosis, which contains four distinct phases: G1 phase (RNA and ribosomes synthesis), S phase (chromosome replication), G2 phase, and M phase (mitosis). G0 phase refers to the quiescent state in which cells have temporarily stopped dividing. Similar to the CR system, the cell cycle appears to be an oscillator, where the periodic expression of cyclins controls cell cycle progression in a sequential and unidirectional manner. Both the cell cycle and the CR system exhibit sequential phases in the transcription/translation of genes and modification/degradation of proteins. As two basic periodic processes that occur within a day, these two systems are interrelated and interact. Control of the cell cycle progression by the circadian clock is known as circadian gating of the cell cycle. Previous reports have indicated that the CR system regulates cell cycle progress both at the G1/S (Geyfman et al., 2012; Kowalska et al., 2013) and at G2/M (Matsuo et al., 2003; Tulina et al., 2014) transitions. In contrast, the cell cycle can regulate the circadian clock as well. Nagoshi et al. (2004) indicated that cell division could influence the circadian clock by impacting the concentrations of PER/CRY protein complex. Bieler et al. (2014) showed that the cell cycle oscillator has a significant effect on the clock oscillator, resulting in a robust synchronization of both cycles in NIH3T3 cells.
Studies have indicated that the circadian oscillator and the cell cycle oscillator are bidirectionally coupled. For example, the cell-cycle duration is usually within the range of the circadian period in many mammalian cell lines (Glass, 2001). When cells are arrested to the same stage of the cell cycle by methods such as serum starvation (retaining cells at the G0/G1 phase), we observed some synchronization of CR (Nagy et al., 2016). Moreover, circadian synchronization induced by Dex leads to clusters of dividing cells, suggesting that the cell cycle is synchronized through the circadian clock (Feillet et al., 2015). Coupling (also called phase locking or mode locking) is defined by the coupling ratio p:q, in which p cell cycles finish during q circadian cycles (Glass, 2001). In the loss of external timing signals, the circadian clock and cell cycle display phase locking with a ratio of 1:1 (Bieler et al., 2014). However, other studies have shown that CR are not exactly synchronized with the cell cycle synchronization, and while there may be coupling between CR and the cell cycle, the coupling ratio is not always 1:1, and can be altered with increases in serum concentration or cell differentiation (Feillet et al., 2014; Matsu-Ura et al., 2016). In conclusion, researchers can choose different synchronization methods based on the advantages and disadvantages of various synchronizers to address different experimental questions (summarized in Table 1).
Circadian Rhythm Monitoring and Detecting Methods
Rhythm Monitoring Methods
Since the discovery of CR, researchers have used various methods to monitor this phenomenon. In animal studies, a wheel-running system with passive infrared sensing elements is utilized to monitor the rhythm of locomotor activity (Albrecht and Foster, 2002). RNA or proteins are extracted from cells or tissues by successive sampling to determine the oscillatory patters of clock genes in vitro or in vivo. This chronological collection approach is severely limited by many practical issues (Gaspar and Brown, 2015). After sampling chronologically, detection of gene fluctuations at the transcriptional or translational levels requires complex steps including extraction, RNA reverse transcription/protein denaturation and PCR/immunoblotting, which may increase the error of the results. In addition, since detection is not in real-time, time intervals are between sample collections at different time points may result in maximum or minimum values not being captured. Moreover, chronological collection is relatively labor-intensive, making it difficult to decreasing errors by enhancing temporal resolution (Sellix et al., 2010). Furthermore, it is difficult to distinguish the defects whether come from intercellular synchrony or cell-autonomous clock function because the individual cellular clocks cannot be monitored (Sellix et al., 2010).
In recent years, there has been rapid progress in bioluminescence reporter technologies and specialized dynamic imaging techniques, allowing for real-time quantitative monitoring of rhythmic oscillations, resulting in advances in the field of chronobiology (Ballesta et al., 2017). Since the early 1990s, luciferases have been utilized as indicators of circadian clock genes (Welsh and Kay, 2005). By fusing the promoter of a target gene to the firefly luciferase reporter gene at the cellular or organismal level, stable reporter cell populations or stable reporter transgenic mice can be generated (Yamazaki et al., 2000; Izumo et al., 2003). In vitro, after synchronizing the reporter cells or tissue explants, the samples are placed in medium containing luciferin (the substrate for luciferase). Apparatuses with PMTs are used to detect oxidation of luciferin by luciferase (Yoo et al., 2004), allowing for real-time monitoring of gene expression. By inserting an optical fiber into the SCN of a PER1-luciferase transgenic mouse, the in vivo central clock oscillations can be recorded in real-time (Yamaguchi et al., 2001). In addition, by subcutaneously injecting luciferin into Per2::luc mice (Tahara et al., 2012), or by injecting Adv-Bmal1-luc viral vectors targeting hepatocytes via tail vein injection in combination with intraperitoneal injection of a fluorescein solution (Saini et al., 2013), oscillations of peripheral clocks such as the liver, kidney, and submandibular glands can be surveilled in vivo. Apparatuses such as fluorescent imaging systems, cooled CCD cameras, or RT-Biolumicorders (designed by Saini et al., 2013) are used to monitor circadian oscillations. The CCD camera, which can detect a single photon, allows for single-cell fluorescence rhythm monitoring in vitro or in vivo (Welsh and Kay, 2005; Sellix et al., 2010; Lande-Diner et al., 2015).
The luciferase reporter system can be employed to supervise circadian oscillations at the transcriptional level in real time. In these experiments, the generated luminescence is very weak and the background luminescence is nearly zero, which has the advantage of preventing phototoxicity related to long-term illumination (Sellix et al., 2010). However, the luciferase reporter system is not suitable for measuring the fluctuations of clock proteins and their sub-cellular localization, and their requirement for luciferin as substrate can result in luciferase chemistry-dependent artifacts (Smyllie et al., 2016). Smyllie et al. (2016) generated a PER2::VENUS mouse, which expresses the fluorescent fusion protein PER2::VENUS, allowing for dynamic acquisition of information regarding PER2 in individual mammalian cells. The PER2::VENUS half-life is similar to that of PER2::LUC, but it directly measures the circadian protein PER2 instead of indirectly measuring enzymatic luciferase activity. As such this strategy may provide a more accurate estimate of PER2 stability and rhythm (Smyllie et al., 2016).
Taken together, a number of methods for monitoring CR are available and can be chosen according to experimental conditions and the limitations and benefits of each of the above introduced monitoring methods (summary in Table 2).
Rhythmicity Detecting Methods
One of the basic problems in CR research is detection and analysis of the rhythmicity of measured data. Following collection of physiological data from a cell over many days, we are able to evaluate periodicity. Rhythmic data has several basic characteristics. Period is defined as how often the cycle is repeated. If the period is 24 h, the data pattern of oscillation reoccurs every 24 h. Phase refers to the time point of peak or trough expression, which reflects the timing in the individual tissues or cells. Phase angle of entrainment means the appropriate phase relationship between the endogenous CT and external environmental time. Amplitude is the magnitude of oscillation between peak and trough. If the data is rhythmic, we must evaluate more parameters than whether the data vary over time. We should also estimate whether the waveform meets the characteristics of a periodic function, such as a cosine function, as determined by its amplitude, period, and phase (Herzog et al., 2015). FFT is the most common method for detection of periodicity, and generally assumes that the fundamental rhythm is in the form of several cosine waves. This method fits the data to cosine functions with different periods, amplitudes, and phases (Moore et al., 2014). The periodicity in the data is fitted to the highest-amplitude cosine function (Herzog et al., 2015). Another method is JTK-Cycle, which is a non-parametric statistical algorithm for identifying and characterizing rhythmic expression in large datasets. It can reliably detect the transcripts in datasets with oscillating abundance because of its statistical power, specificity, accuracy, and precision (Hughes et al., 2010). Other approaches such as cross-over analysis, autocorrelation (Levine et al., 2002), and curve-fitting (Straume, 2004) could also be used for detection and evaluation of periodicity.
Circadian Rhythm-Related Databases
Previous studies have reported that various tissues and cells exhibit CR in vivo or in vitro. As a growing number of tissues and cells have been found to display periodic gene expression, and the number of identified CCGs has increased. Based on accumulation of new data, researchers urgently need platforms to collect and obtain this information. Several databases have been constructed, including CircadiOmics1, CircaDB2, Bioclock3, SCNseq4, CGDB5, and CirGRDB6, which have different characteristics and functions. These databases allow investigators to search for genes of interest that exhibited rhythmic expression patterns in different species.
CircadiOmics contains circadian–related data sets from the livers of wild-type and Clock mutant mice, and is integrated with genomic, transcriptomic, proteomic, and metabolomic data sets (Patel et al., 2012). The CircaDB database contains circadian transcriptional profiles of over 3000 potential circadian genes in mice and humans (Pizarro et al., 2013). Bioclock is a s resource library which contains diel and circadian microarray expression data from Aedes aegypti (1674 potential circadian genes) and Anopheles gambiae (∼1000 potential circadian genes) (Leming et al., 2014). SCNseq is an important chronobiological resource that contains data from mouse SCN, and contains 4569 rhythmic genes and 3187 intergenic non-coding RNAs (Pembroke et al., 2015).
Among the above databases, Bioclock is focused on insects (mosquitoes), and CircadiOmics, SCNseq, and CircaDB are focused on mammals (mouse and human). However, these databases are still not considered comprehensive despite having been established for several years. CGDB, a new database of cycling genes in eukaryotes established in recent years, contains ∼73,000 oscillating genes across phyla (68 animals, 39 plants, and 41 fungi) from published small-scale or high-throughput data obtained from various species (Li et al., 2017). This database can be browsed “by species” or “by external conditions,” and genes can be reported in peaks or troughs at specific time points (Li et al., 2017). In addition, another new database, CirGRDB, has integrated over than 4936 genome-wide assays, and allows users to retrieve three groups of transcriptome profiles including normal conditions (oscillatory patterns in normal tissues or cell lines), different conditions (oscillatory patterns under different conditions), and other conditions (expression pattern of specific genes under knock-out/down or over-expression) (Li et al., 2018). This database also provides information about regulatory mechanisms including transcriptional factors, histone modification, chromatin accessibility, enhancer RNAs, microRNAs, RNA binding proteins, RNA editing, and RNA methylation, and can be used to construct a regulatory network of rhythmic genes at multiple regulatory layers (Li et al., 2018). The CirGRDB database adds information about post-translational modifications, transcriptional factors, and epigenetic modifications that were not available in previous databases. These databases that integrate circadian information have been established successively in recent years, and each have different characteristics and functions. These databases allow for convenient evaluation of past results, which may provide scientific direction for future studies.
Experimental Causes of Circadian Rhythm Disorders
After synchronizing the CR by appropriate means and monitoring the circadian oscillations of subjects, any inconsistency in our observations compared with previous results requires careful evaluation of the experimental conditions, since there are lots of factors that can influence the circadian clock.
Light, food, temperature, arousal stimulation, and oxygen concentration in the environment can affect CR in mammals. As previously described, exposure light pulses, different photoperiods, constant light conditions, differing light intensity, and different wavelengths of light can alter the phase, period, or amplitude of CR. In addition to entrainment, negative masking is another response of animals to light. Thus, in CR experiments researchers must control all appropriate parameters and avoid negative masking.
As the previous description, time-restricted feeding entrains the clock, and shifts the phase of peripheral clocks from that observed with ad libitum feeding (Damiola et al., 2000; Stokkan et al., 2001) and calorie restriction (Mendoza et al., 2008; Patel et al., 2016). In addition, different ingredients in food can cause inconsistencies in circadian rhythm expression patterns. For example, high fat diet impacts the central and peripheral clocks, and significantly decreases the amplitude of circadian oscillations in the liver (Kohsaka et al., 2007). This may be due to elevated blood glucose levels, insulin resistance, or other metabolic changes (Bass and Takahashi, 2010). Moreover, high fat diet signals could promote release of gastrointestinal tract-derived peptides or bile, resulting in dysregulation of PPAR-α signaling in the liver and other tissues, which may explain high fat diet-induced rhythm disruption (Asher et al., 2010; Zarrinpar et al., 2016). Timed restricted feeding resets the circadian oscillations of animals on a high fat diet and prevents high-fat diet-caused obesity through improving CREB, mTOR, and AMPK pathway function (Hatori et al., 2012; Sherman et al., 2012). High salt diet also influences clock oscillations. Two weeks of 4% NaCl administration resulted in phase advances in mouse peripheral clocks (Oike et al., 2010). Therefore, it is necessary to control the ingredients and caloric value of the diet, and the feeding approach (time-restricted feeding or ad libitum feeding), to control the peripheral oscillators. Although other non-photic entrainment factors such as arousal stimuli, ambient temperature and oxygen concentration have weaker effects on the animal CR system (previously described), it is still necessary to control all the above factors to maintain consistent and reproducible experimental conditions.
Causes of circadian rhythm disturbance in cultured cells or tissues include cell density, osmotic pressure, media PH, mechano-environment, temperature, oxygen concentration, and microorganisms. The circadian amplitude depends on cell density. The rhythmicity of low-density SCN neurons and fibroblasts was greatly reduced, and was significantly enhanced in high-density cultures (Liu et al., 2007). Coupling between SCN neurons could enhance the rhythmicity of a cell population (Liu et al., 2007), and the normal rhythmic expression of fibroblasts need paracrine signals from adjacent cells (Noguchi et al., 2013). Moreover, a stiff extracellular matrix increases the amplitudes of circadian oscillations in mammary epithelial cells, and decreases in amplitudes in mammary fibroblasts occur through mechanotransduction pathways mediated by integrin adhesion and Rho signaling (Yang et al., 2017; Williams et al., 2018). Furthermore, the circadian period can be regulated by osmotic stress. A previous report showed that the circadian period of mouse embryonic fibroblasts can be lengthened using hypertonic media and shortened using hypotonic media through ASK -dependent phosphorylation of proteins (Imamura et al., 2018). Furthermore, an intense alter in media pH (±0.4) induces extensive phase shifts (>8 h) of clock genes in rat fibroblasts, likely through the TGF-β signaling pathway (Kon et al., 2008). As previously described, circadian oscillations can be entrained by changes in ambient temperature. In addition, temperature pulses for 1 or 6 h also cause phase shifts in ex vivo pituitary or lung cultures (Kon et al., 2008). Furthermore, oxygen levels affect CR. These results imply that it is crucial to control cell density, the mechano-environment, osmotic pressure, pH, and oxygen concentration when evaluating circadian rhythms. Cells contaminated microorganisms can exhibit circadian genes expression disruption. The presence of bacteria can reduce the amplitudes circadian rhythms in mouse enterocytes (Mukherji et al., 2013; Wang et al., 2017) and lung epithelial cells (Haspel et al., 2014) by directly invading or secreting endotoxins that activate the TLR signaling pathway. Moreover, multiple viruses such as Hepatitis B and C viruses, human immunodeficiency virus, Coxsackie virus A16, human T-lymphocyte virus type 1, and influenza can also disrupt the expression of clock genes (Zhuang et al., 2017). Therefore, it is necessary to prevent microbial contamination when evaluating CR.
In addition to the above environmental factors, the age of animals or cells should also be considered when studying CR. Circadian rhythm oscillations in humans and rodents gradually dampen with increasing age, although whether this age-related change is owing to dysfunction of inherent core clock or insufficient environmental entrainment is unclear (Chang and Guarente, 2013; Wang et al., 2015). In general, CR can be affected by many environmental factors. Any imbalance of these factors can lead to circadian disorders, which can contribute to several diseases through a series of signal pathways and molecular mechanisms.
Circadian Rhythm Disorder and Diseases
In mammals, many physiological functions under the regulation of the circadian clock are affected by external cues such as sleep and wakefulness, alertness and motor ability, body temperature fluctuations, the urinary system, hormone secretion, immune regulation, cytokine release, and cell cycle progression (Panda et al., 2002; Bass and Takahashi, 2010). When circadian systems are disrupted by various environmental or genetic defects, dysfunction of various physiological processes can occur (Hastings et al., 2003).
A common reason of human circadian disorder is misalignment between the environmental rhythm (e.g., light–night cycle) and the endogenous circadian oscillators. Previous reports have shown that human subjects who were maintained in a controlled circadian misalignment condition experienced severe imbalances in glucose homeostasis, insulin action, and appetite control (Buxton et al., 2012; McHill et al., 2014). In animal studies, when the intrinsic clocks of animals are desynchronized from external timing cues, disorders in many organs or tissues can occur, including diet-induced obesity (Kohsaka et al., 2007; Arble et al., 2009), a light-induced pro-inflammatory state (Lucassen et al., 2016), cardiac fibrosis, and systolic dysfunction (Penev et al., 1998; Martino et al., 2008). Genetically, since the molecular clock is highly conserved, diseases caused by mutations of clock genes (which are often used in animal models to study clock gene-related dysfunction) are rare in humans. An examples of these kinds of mutations is familial advanced sleep phase syndrome, which is induced by a missense mutation (S662G) of the core clock gene PER2 (Jones et al., 1999; Reid et al., 2001). In addition, several clock gene single nucleotide polymorphisms related to metabolic syndrome, hypertension, and diabetes mellitus also have been identified in genome-wide association studies (Saxena et al., 2007; Woon et al., 2007; Zeggini et al., 2007; Scott et al., 2008).
A large quantity of studies have suggested that circadian disruptions are closely associated with the formation and development of various diseases including cancer (Momma et al., 2017; Polo et al., 2017; Xiong et al., 2018), dysplasia (Vilches et al., 2014; Voiculescu et al., 2016; Zhao et al., 2018; Zhou et al., 2018), cardiovascular disease (Angelousi et al., 2018), obesity (Antunes et al., 2010), diabetes (Pan et al., 2011), and sleep disorder (Logan and McClung, 2019). As previously described, the cell cycle and the circadian clock are two basic periodic processes of a day, and they are interrelated. Clock genes contribute to the occurrence and development of tumors by regulating and interfering with cell cycle-related genes such as c-Myc, P53, and P21 (Angelousi et al., 2018). In addition, our previous studies also suggested that circadian disruption led to an abnormal increase in the precancerous gene PFKFB3, thereby promoting differentiation from normal cells to tumor cells (Chen et al., 2016). Further, deletion of the core clock protein BMAL1 attenuated inhibition of TERT transcription, and TERT upregulation is closely related to tumorigenesis (Tang et al., 2017).
Disorders of the circadian clock also affect the development of organs such as the brain and bone, leading to dysplasia. CR are essential for brain development (Novakova et al., 2010). Studies have indicated that rhythms of clock genes and genes encoding NMDA receptor subunits are inhibited in the hippocampus of rat adult progeny subjected to persistent illumination during pregnancy, resulting in impaired spatial memory in these animals (Vilches et al., 2014; Voiculescu et al., 2016). Further, recent human and animal studies suggested that sleep and circadian disruptions during pubescence can impact brain development and may lead to susceptibility to mood and substance use disorders (Logan and McClung, 2019). Moreover, circadian clocks participate in regulation of neurogenesis. Studies have displayed that the clock component REV-ERBα directly inhibited the promoter of the FABP7 gene, a marker for neuronal progenitor cells, resulting in alterations in neuronal differentiation (Young et al., 2013; Giachino et al., 2014). Loss of REV-ERBα resulted in increased FABP7 expression and hippocampal neurogenesis, which was in connection with changes in mood-related behaviors (Schnell et al., 2014). Furthermore, our previous studies showed the importance of the circadian clock on bone development. Circadian disruption resulted in Bmal1 down-regulation, leading to direct inhibition of Opg transcription (Zhou et al., 2018) and an indirect increase in Mmp3 expression through P65 phosphorylation (Zhao et al., 2018). These changes promoted osteoclasis and suppressed osteogenesis, resulting in bone loss and abnormal mandibular development.
Circadian-related metabolic diseases may be associated with defective glucose tolerance and insulin resistance, and abnormal glucose metabolism (Stenvers et al., 2018). Circadian disruption may result in aberrant glucocorticoid and melatonin levels, which could affect insulin secretion (glucocorticoids decrease insulin secretion, and melatonin increases insulin secretion) (Stenvers et al., 2018). In addition, a study exhibited that the circadian clock may maintain long time energy balance through regulating the leptin endocrine feedback loop between adipose tissue and the brain, as demonstrated by circadian clock defects or long-time jet lag resulting in leptin-resistant animals (Kettner et al., 2015). Moreover, other studies indicated that the circadian clock regulates levels of neurohormones involved in cardiovascular function such as angiotensin II, renin, aldosterone, growth hormone, and atrial natriuretic peptide (Bhatnagar, 2017). These neurohormones may be responsible for increased blood pressure and inflammation in response to circadian misalignment (Scheer et al., 2009; Morris et al., 2015).
Higher incidences of brain diseases such as sleep disorders and depression were observed in a population with interrupted CR (Logan and McClung, 2019). Moreover, circadian dysregulation can induce emotional and psychotic attacks in individuals already suffering from psychiatric disorders (Logan and McClung, 2019). Several processes such as oxidative stress, inflammation, dopamine synthesis, and cellular metabolism are under the control of the circadian clock, and may contribute to neurodegeneration (Logan and McClung, 2019). Although most studies have suggested that circadian disorder is a symptom of neurodegenerative diseases including Alzheimer’s disease and Parkinson’s disease, some evidence indicates that circadian dysregulation induced by night illumination can cause enhanced incidence of tau deposition and neurodegeneration (Kim et al., 2018). Furthermore, increased risk of Alzheimer’s disease and Parkinson’s disease has been associated with the incidence of single-nucleotide polymorphisms in CLOCK and BMAL1, and in BMAL1 and PER1, respectively (Logan and McClung, 2019). Therefore, circadian rhythm disorders may act on accelerating the progression of diseases in vulnerable individuals.
Taken together, many studies have highlighted that increased risk of multiple diseases including carcinoma, dysplasia, metabolic disorders, and neurodegenerative diseases are associated with circadian disorders induced by changes in external environmental cues. The mechanisms responsible for these associations may be physiological processes including cell proliferation, differentiation, oxidative stress, inflammation, synthesis, and cellular metabolism, which are regulated by the circadian clock.
Conclusion and Future Prospects
Circadian clock system plays vital roles in the regulation of physiological processes, including cell cycle progression, cytokine release, hormone secretion, sleep and wakefulness, immune regulation, etc. Multiple systemic diseases are proven to be closely associated with circadian rhythm disorders, so chronobiology research is becoming a focal point in biological and medical fields at present. With the development of chronobiology in these years, external cues that can be used as synchronizers to reset the circadian oscillators of animals or cells have been gradually discovered. From time-serial collection to real-time monitoring through luciferase reporter genes and fluorescent proteins, the methods to observe biological rhythms are rapidly advancing and becoming more diverse. In addition, researchers have also established a number of circadian clock-related databases to facilitate access to previous research results. Through the combination of various in vivo and in vitro experiments, the mechanisms underlying circadian oscillations are constantly being elucidated, and the complicated connections between circadian rhythm disorders and various diseases are also being identified. Illuminating the crosstalk between circadian rhythm and human diseases can help us better clarify the pathogenesis of circadian-related diseases, which provides new strategies and ideas for disease prevention and treatment.
Author Contributions
YX and QT contributed equally to this review. LC, QT, and YX conceived and wrote the manuscript. GC, MX, and SY contributed to data acquisition, analysis, and interpretation. LC and JZ critically revised the manuscript.
Funding
This work was supported by the National Natural Science Foundation of China for Distinguished Young Scholars (31725011 to LC).
Conflict of Interest Statement
The authors declare that the research was conducted in the absence of any commercial or financial relationships that could be construed as a potential conflict of interest.
Abbreviations
βOHB, beta-hydroxybutyrate; AMPA, α-amino -3-hydroxy-5-methyl-4-isoxazolepropionic acid; AMPK, adenosine monophosphate-activated protein kinase; ASK, apoptosis signal-regulating kinase; AVP, arginine vasopressin; BMAL1, brain and muscle Arnt-like protein 1; CaMK, calcium-calmodulin kinase; cAMP, cyclic adenosine monophosphate; CCD, charge-coupled device; CCGs, clock-controlled genes; CIRBPs, cold-inducible RNA binding proteins; CLOCK, circadian locomotor output cycles kaput; CR, circadian rhythms; CRE, cAMP response elements; CREB, cAMP response element-binding protein; Cry, cryptochrome; CT, circadian time; DBP, D-box binding protein; DD, dark-dark; Dex, dexamethasone; DM, dorsomedial; DPSC, dental pulp-derived stem cells; E4BP4, E4 Promoter-Binding Protein 4; EGF, epidermal growth factor; EGR1, Early growth response protein 1; FAA, food anticipatory activity; FABP7, fatty acid binding protein 7; FEO, food-entrainable oscillator; FFT, fast fourier transform; Fsk, forskolin; GABA, gamma-aminobutyric acid; GHT, geniculohypothalamic tract; GR, glucocorticoid receptor; GRE, glucocorticoid response element; HLF, hepatic leukemia factor; HSF1, heat shock factor 1; IGL, intergeniculate leaflet; IRC, irradiance response curve; LD, light-dark; MAPK pathway, mitogen-activated protein kinase pathway; Mmp3, matrix metalloproteinase 3; MRTFs, myocardin-related transcription factors; mTOR, mammalian target of rapamycin; NADH, nicotinamide adenine dinucleotide; NADPH, nicotinamide adenine dinucleotide phosphate; NFIL3, nuclear factor interleukin-3-regulated protein; NMDA, N-methyl D-aspartate; NPAS2, neuronal PAS domain protein 2; NPY, neuropeptide Y; Opg, osteoprotegerin; PAC1, proteasome assembly chaperone 1; PACAP, pituitary adenylyl cyclase activating peptide; PAR-bZip, proline and acidic amino acid-rich basic leucine zipper; PCR, polymerase chain reaction; Per, Period; PGE2, prostaglandin E2; PKA, protein kinase A; PKCA, protein kinase Cα; PKG, protein kinase G; PMTs, photomultiplier tubes; PPAR, peroxisome proliferator-activated receptors; pRGC, photosensitive retinal ganglion cell; RHT, retinohypothalamic tract; ROREs, ROR binding elements; RORs, retinoic acid receptor-related orphan receptors; SCN, suprachiasmatic nucleus; SP, substance P; TEF, thyrotroph embryonic factor; TERT, telomerase reverse transcriptase; TGF-β, transforming growth factor beta; TLR, toll-like receptor; TRP channel, transient-receptor potential channel; TRPCs, transient-receptor potential channels; TTFLs, transcription and translation feedback loops; VIP, vasoactive intestinal polypeptide; VL, ventrolateral; VPAC2, vasoactive intestinal peptide receptor 2; ZT, zeitgeber time.
Footnotes
- ^ http://circadiomics.igb.uci.edu/
- ^ http://circadb.hogeneschlab.org/
- ^ https://www3.nd.edu/~bioclock/
- ^ www.wgpembroke.com/shiny/SCNseq/
- ^ http://cgdb.biocuckoo.org/
- ^ http://cirgrdb.biols.ac.cn/
References
Acosta-Galvan, G., Yi, C. X., van der Vliet, J., Jhamandas, J. H., Panula, P., Angeles-Castellanos, M., et al. (2011). Interaction between hypothalamic dorsomedial nucleus and the suprachiasmatic nucleus determines intensity of food anticipatory behavior. Proc. Natl. Acad. Sci. U.S.A. 108, 5813–5818. doi: 10.1073/pnas.1015551108
Adamovich, Y., Ladeuix, B., Golik, M., Koeners, M. P., and Asher, G. (2017). Rhythmic oxygen levels reset circadian clocks through HIF1alpha. Cell Metab. 25, 93–101. doi: 10.1016/j.cmet.2016.09.014
Akiyama, M., Yuasa, T., Hayasaka, N., Horikawa, K., Sakurai, T., and Shibata, S. (2004). Reduced food anticipatory activity in genetically orexin (hypocretin) neuron-ablated mice. Eur. J. Neurosci. 20, 3054–3062. doi: 10.1111/j.1460-9568.2004.03749.x
Albrecht, U. (2012). Timing to perfection: the biology of central and peripheral circadian clocks. Neuron 74, 246–260. doi: 10.1016/j.neuron.2012.04.006
Albrecht, U., and Foster, R. G. (2002). Placing ocular mutants into a functional context: a chronobiological approach. Methods 28, 465–477. doi: 10.1016/s1046-2023(02)00266-9
Angelousi, A., Kassi, E., Nasiri-Ansari, N., Weickert, M. O., Randeva, H., and Kaltsas, G. (2018). Clock genes alterations and endocrine disorders. Eur. J. Clin. Invest. 48:e12927. doi: 10.1111/eci.12927
Antle, M. C., and Mistlberger, R. E. (2000). Circadian clock resetting by sleep deprivation without exercise in the Syrian hamster. J. Neurosci. 20, 9326–9332. doi: 10.1523/jneurosci.20-24-09326.2000
Antle, M. C., and Silver, R. (2005). Orchestrating time: arrangements of the brain circadian clock. Trends Neurosci. 28, 145–151. doi: 10.1016/j.tins.2005.01.003
Antunes, L. C., Levandovski, R., Dantas, G., Caumo, W., and Hidalgo, M. P. (2010). Obesity and shift work: chronobiological aspects. Nutr. Res. Rev. 23, 155–168. doi: 10.1017/s0954422410000016
Arble, D. M., Bass, J., Laposky, A. D., Vitaterna, M. H., and Turek, F. W. (2009). Circadian timing of food intake contributes to weight gain. Obesity 17, 2100–2102. doi: 10.1038/oby.2009.264
Asher, G., Reinke, H., Altmeyer, M., Gutierrez-Arcelus, M., Hottiger, M. O., and Schibler, U. (2010). Poly(ADP-ribose) polymerase 1 participates in the phase entrainment of circadian clocks to feeding. Cell 142, 943–953. doi: 10.1016/j.cell.2010.08.016
Astiz, M., Heyde, I., and Oster, H. (2019). Mechanisms of communication in the mammalian circadian timing system. Int. J. Mol. Sci. 20:E343. doi: 10.3390/ijms20020343
Ballesta, A., Innominato, P. F., Dallmann, R., Rand, D. A., and Levi, F. A. (2017). Systems chronotherapeutics. Pharmacol. Rev. 69, 161–199. doi: 10.1124/pr.116.013441
Balsalobre, A., Brown, S. A., Marcacci, L., Tronche, F., Kellendonk, C., Reichardt, H. M., et al. (2000a). Resetting of circadian time in peripheral tissues by glucocorticoid signaling. Science 289, 2344–2347. doi: 10.1126/science.289.5488.2344
Balsalobre, A., Marcacci, L., and Schibler, U. (2000b). Multiple signaling pathways elicit circadian gene expression in cultured Rat-1 fibroblasts. Curr. Biol. 10, 1291–1294. doi: 10.1016/s0960-9822(00)00758-2
Balsalobre, A., Damiola, F., and Schibler, U. (1998). A serum shock induces circadian gene expression in mammalian tissue culture cells. Cell 93, 929–937. doi: 10.1016/s0092-8674(00)81199-x
Banfalvi, G. (2017). Overview of cell synchronization. Methods Mol. Biol. 1524, 3–27. doi: 10.1007/978-1-4939-6603-5_1
Bass, J., and Takahashi, J. S. (2010). Circadian integration of metabolism and energetics. Science 330, 1349–1354. doi: 10.1126/science.1195027
Berson, D. M., Dunn, F. A., and Takao, M. (2002). Phototransduction by retinal ganglion cells that set the circadian clock. Science 295, 1070–1073. doi: 10.1126/science.1067262
Best, J. D., Maywood, E. S., Smith, K. L., and Hastings, M. H. (1999). Rapid resetting of the mammalian circadian clock. J. Neurosci. 19, 828–835. doi: 10.1523/jneurosci.19-02-00828.1999
Bhatnagar, A. (2017). Environmental determinants of cardiovascular disease. Circ. Res. 121, 162–180. doi: 10.1161/circresaha.117.306458
Bieler, J., Cannavo, R., Gustafson, K., Gobet, C., Gatfield, D., and Naef, F. (2014). Robust synchronization of coupled circadian and cell cycle oscillators in single mammalian cells. Mol. Syst. Biol. 10:739. doi: 10.15252/msb.20145218
Brown, S. A., Zumbrunn, G., Fleury-Olela, F., Preitner, N., and Schibler, U. (2002). Rhythms of mammalian body temperature can sustain peripheral circadian clocks. Curr. Biol. 12, 1574–1583. doi: 10.1016/s0960-9822(02)01145-4
Buhr, E. D., Yoo, S.-H., and Takahashi, J. S. (2010). Temperature as a universal resetting cue for mammalian circadian oscillators. Science 330, 379–385. doi: 10.1126/science.1195262
Buxton, O. M., Cain, S. W., O’Connor, S. P., Porter, J. H., Duffy, J. F., Wang, W., et al. (2012). Adverse metabolic consequences in humans of prolonged sleep restriction combined with circadian disruption. Sci. Transl. Med. 4:129ra143. doi: 10.1126/scitranslmed.3003200
Cao, R., Robinson, B., Xu, H., Gkogkas, C., Khoutorsky, A., Alain, T., et al. (2013). Translational control of entrainment and synchrony of the suprachiasmatic circadian clock by mTOR/4E-BP1 signaling. Neuron 79, 712–724. doi: 10.1016/j.neuron.2013.06.026
Challet, E., Pevet, P., Vivien-Roels, B., and Malan, A. (1997). Phase-advanced daily rhythms of melatonin, body temperature, and locomotor activity in food-restricted rats fed during daytime. J. Biol. Rhythms 12, 65–79. doi: 10.1177/074873049701200108
Challet, E., Solberg, L. C., and Turek, F. W. (1998). Entrainment in calorie-restricted mice: conflicting zeitgebers and free-running conditions. Am. J. Physiol. 274(6 Pt 2), R1751–R1761. doi: 10.1152/ajpregu.1998.274.6.R1751
Chambille, I. (1999). Circadian rhythm of AMPA receptor GluR2/3 subunit-immunoreactivity in the suprachiasmatic nuclei of Syrian hamster and effect of a light-dark cycle. Brain Res. 833, 27–38. doi: 10.1016/s0006-8993(99)01460-2
Chang, H. C., and Guarente, L. (2013). SIRT1 mediates central circadian control in the SCN by a mechanism that decays with aging. Cell 153, 1448–1460. doi: 10.1016/j.cell.2013.05.027
Chavan, R., Feillet, C., Costa, S. S., Delorme, J. E., Okabe, T., Ripperger, J. A., et al. (2016). Liver-derived ketone bodies are necessary for food anticipation. Nat. Commun. 7:10580. doi: 10.1038/ncomms10580
Chen, D., Buchanan, G. F., Ding, J. M., Hannibal, J., and Gillette, M. U. (1999). Pituitary adenylyl cyclase-activating peptide: a pivotal modulator of glutamatergic regulation of the suprachiasmatic circadian clock. Proc. Natl. Acad. Sci. U.S.A. 96, 13468–13473. doi: 10.1073/pnas.96.23.13468
Chen, L., Zhao, J., Tang, Q., Li, H., Zhang, C., Yu, R., et al. (2016). PFKFB3 control of cancer growth by responding to circadian clock outputs. Sci. Rep. 6:24324. doi: 10.1038/srep24324
Cheon, S., Park, N., Cho, S., and Kim, K. (2013). Glucocorticoid-mediated Period2 induction delays the phase of circadian rhythm. Nucleic Acids Res. 41, 6161–6174. doi: 10.1093/nar/gkt307
Colwell, C. S., Michel, S., Itri, J., Rodriguez, W., Tam, J., Lelievre, V., et al. (2004). Selective deficits in the circadian light response in mice lacking PACAP. Am. J. Physiol. Regul. Integr. Comp. Physiol. 287, R1194–R1201. doi: 10.1152/ajpregu.00268.2004
Comas, M., and Hut, R. A. (2009). Twilight and photoperiod affect behavioral entrainment in the house mouse (Mus musculus). J. Biol. Rhythms 24, 403–412. doi: 10.1177/0748730409343873
Daan, S. (1977). Tonic and phasic effects of light in the entrainment of circadian rhythms. Ann. N. Y. Acad. Sci. 290, 51–59. doi: 10.1111/j.1749-6632.1977.tb39716.x
Daan, S., and Pittendrigh, C. S. (1976). A functional analysis of circadian pacemakers in nocturnal rodents. J. Comp. Physiol. 106, 267–290. doi: 10.1007/bf01417858
Damiola, F., Le Minh, N., Preitner, N., Kornmann, B., Fleury-Olela, F., and Schibler, U. (2000). Restricted feeding uncouples circadian oscillators in peripheral tissues from the central pacemaker in the suprachiasmatic nucleus. Genes Dev. 14, 2950–2961. doi: 10.1101/gad.183500
Dibner, C., Sage, D., Unser, M., Bauer, C., d’Eysmond, T., Naef, F., et al. (2009). Circadian gene expression is resilient to large fluctuations in overall transcription rates. EMBO J. 28, 123–134. doi: 10.1038/emboj.2008.262
Dibner, C., Schibler, U., and Albrecht, U. (2010). The mammalian circadian timing system: organization and coordination of central and peripheral clocks. Annu. Rev. Physiol. 72, 517–549. doi: 10.1146/annurev-physiol-021909-135821
Do, M. T., and Yau, K. W. (2010). Intrinsically photosensitive retinal ganglion cells. Physiol. Rev. 90, 1547–1581. doi: 10.1152/physrev.00013.2010
Dudek, M., Yang, N., Ruckshanthi, J. P., Williams, J., Borysiewicz, E., Wang, P., et al. (2017). The intervertebral disc contains intrinsic circadian clocks that are regulated by age and cytokines and linked to degeneration. Ann. Rheum. Dis. 76, 576–584. doi: 10.1136/annrheumdis-2016-209428
Duffield, G. E. (2003). DNA microarray analyses of circadian timing: the genomic basis of biological time. J. Neuroendocrinol. 15, 991–1002. doi: 10.1046/j.1365-2826.2003.01082.x
Ebling, F. J. (1996). The role of glutamate in the photic regulation of the suprachiasmatic nucleus. Prog. Neurobiol. 50, 109–132. doi: 10.1016/s0301-0082(96)00032-9
Esnault, C., Stewart, A., Gualdrini, F., East, P., Horswell, S., Matthews, N., et al. (2014). Rho-actin signaling to the MRTF coactivators dominates the immediate transcriptional response to serum in fibroblasts. Genes Dev. 28, 943–958. doi: 10.1101/gad.239327.114
Fahrenkrug, J. (2006). PACAP–a multifacetted neuropeptide. Chronobiol. Int. 23, 53–61. doi: 10.1080/07420520500464569
Feillet, C., Krusche, P., Tamanini, F., Janssens, R. C., Downey, M. J., Martin, P., et al. (2014). Phase locking and multiple oscillating attractors for the coupled mammalian clock and cell cycle. Proc. Natl. Acad. Sci. U.S.A. 111, 9828–9833. doi: 10.1073/pnas.1320474111
Feillet, C., van der Horst, G. T., Levi, F., Rand, D. A., and Delaunay, F. (2015). Coupling between the circadian clock and cell cycle oscillators: implication for healthy cells and malignant growth. Front. Neurol. 6:96. doi: 10.3389/fneur.2015.00096
Foster, R. G., Hankins, M. W., and Peirson, S. N. (2007). Light, photoreceptors, and circadian clocks. Methods Mol. Biol. 362, 3–28. doi: 10.1007/978-1-59745-257-1_1
Gachon, F., Fonjallaz, P., Damiola, F., Gos, P., Kodama, T., Zakany, J., et al. (2004). The loss of circadian PAR bZip transcription factors results in epilepsy. Genes Dev. 18, 1397–1412. doi: 10.1101/gad.301404
Gaspar, L., and Brown, S. A. (2015). Measuring circadian clock function in human cells. Methods Enzymol. 552, 231–256. doi: 10.1016/bs.mie.2014.10.023
Geerdink, M., Walbeek, T. J., Beersma, D. G., Hommes, V., and Gordijn, M. C. (2016). Short blue light pulses (30 Min) in the morning support a sleep-advancing protocol in a home setting. J. Biol. Rhythms 31, 483–497. doi: 10.1177/0748730416657462
Gekakis, N., Staknis, D., Nguyen, H. B., Davis, F. C., Wilsbacher, L. D., King, D. P., et al. (1998). Role of the CLOCK protein in the mammalian circadian mechanism. Science 280, 1564–1569. doi: 10.1126/science.280.5369.1564
Geyfman, M., Kumar, V., Liu, Q., Ruiz, R., Gordon, W., Espitia, F., et al. (2012). Brain and muscle Arnt-like protein-1 (BMAL1) controls circadian cell proliferation and susceptibility to UVB-induced DNA damage in the epidermis. Proc. Natl. Acad. Sci. U.S.A. 109, 11758–11763. doi: 10.1073/pnas.1209592109
Giachino, C., Basak, O., Lugert, S., Knuckles, P., Obernier, K., Fiorelli, R., et al. (2014). Molecular diversity subdivides the adult forebrain neural stem cell population. Stem Cells 32, 70–84. doi: 10.1002/stem.1520
Ginty, D. D., Kornhauser, J. M., Thompson, M. A., Bading, H., Mayo, K. E., Takahashi, J. S., et al. (1993). Regulation of CREB phosphorylation in the suprachiasmatic nucleus by light and a circadian clock. Science 260, 238–241. doi: 10.1126/science.8097062
Glass, L. (2001). Synchronization and rhythmic processes in physiology. Nature 410, 277–284. doi: 10.1038/35065745
Golombek, D. A., Ferreyra, G. A., Agostino, P. V., Murad, A. D., Rubio, M. F., Pizzio, G. A., et al. (2003). From light to genes: moving the hands of the circadian clock. Front. Biosci. 8, s285–s293.
Golombek, D. A., and Ralph, M. R. (1996). Let there be light: signal transduction in a mammalian circadian system. Braz. J. Med. Biol. Res. 29, 131–140.
Golombek, D. A., and Rosenstein, R. E. (2010). Physiology of circadian entrainment. Physiol. Rev. 90, 1063–1102. doi: 10.1152/physrev.00009.2009
Guan, X. M., Hess, J. F., Yu, H., Hey, P. J., and van der Ploeg, L. H. (1997). Differential expression of mRNA for leptin receptor isoforms in the rat brain. Mol. Cell. Endocrinol. 133, 1–7. doi: 10.1016/s0303-7207(97)00138-x
Guenthner, C. J., Luitje, M. E., Pyle, L. A., Molyneux, P. C., Yu, J. K., Li, A. S., et al. (2014). Circadian rhythms of Per2::Luc in individual primary mouse hepatocytes and cultures. PLoS One 9:e87573. doi: 10.1371/journal.pone.0087573
Gutierrez-Monreal, M. A., Cuevas-Diaz Duran, R., Moreno-Cuevas, J. E., and Scott, S. P. (2014). A role for 1alpha,25-dihydroxyvitamin d3 in the expression of circadian genes. J. Biol. Rhythms 29, 384–388. doi: 10.1177/0748730414549239
Hall, A. C., Hoffmaster, R. M., Stern, E. L., Harrington, M. E., and Bickar, D. (1997). Suprachiasmatic nucleus neurons are glucose sensitive. J. Biol. Rhythms 12, 388–400. doi: 10.1177/074873049701200501
Hamamura, K., Swarnkar, G., Tanjung, N., Cho, E., Li, J., Na, S., et al. (2012). RhoA-mediated signaling in mechanotransduction of osteoblasts. Connect Tissue Res. 53, 398–406. doi: 10.3109/03008207.2012.671398
Hankins, M. W., Peirson, S. N., and Foster, R. G. (2008). Melanopsin: an exciting photopigment. Trends Neurosci. 31, 27–36. doi: 10.1016/j.tins.2007.11.002
Hannibal, J. (2002). Neurotransmitters of the retino-hypothalamic tract. Cell Tissue Res. 309, 73–88. doi: 10.1007/s00441-002-0574-3
Hannibal, J., Brabet, P., and Fahrenkrug, J. (2008). Mice lacking the PACAP type I receptor have impaired photic entrainment and negative masking. Am. J. Physiol. Regul. Integr. Comp. Physiol. 295, R2050–R2058. doi: 10.1152/ajpregu.90563.2008
Hannibal, J., Ding, J. M., Chen, D., Fahrenkrug, J., Larsen, P. J., Gillette, M. U., et al. (1998). Pituitary adenylate cyclase activating peptide (PACAP) in the retinohypothalamic tract: a daytime regulator of the biological clock. Ann. N. Y. Acad. Sci. 865, 197–206. doi: 10.1111/j.1749-6632.1998.tb11179.x
Hannibal, J., and Fahrenkrug, J. (2004). Target areas innervated by PACAP-immunoreactive retinal ganglion cells. Cell Tissue Res. 316, 99–113. doi: 10.1007/s00441-004-0858-x
Hara, R., Wan, K., Wakamatsu, H., Aida, R., Moriya, T., Akiyama, M., et al. (2001). Restricted feeding entrains liver clock without participation of the suprachiasmatic nucleus. Genes Cell 6, 269–278. doi: 10.1046/j.1365-2443.2001.00419.x
Hashimoto, H., Shintani, N., and Baba, A. (2006). New insights into the central PACA Pergic system from the phenotypes in PACAP- and PACAP receptor-knockout mice. Ann. N. Y. Acad. Sci. 1070, 75–89. doi: 10.1196/annals.1317.038
Haspel, J. A., Chettimada, S., Shaik, R. S., Chu, J. H., Raby, B. A., Cernadas, M., et al. (2014). Circadian rhythm reprogramming during lung inflammation. Nat. Commun. 5:4753. doi: 10.1038/ncomms5753
Hastings, M. H., Reddy, A. B., and Maywood, E. S. (2003). A clockwork web: circadian timing in brain and periphery, in health and disease. Nat. Rev. Neurosci. 4, 649–661. doi: 10.1038/nrn1177
Hatori, M., Vollmers, C., Zarrinpar, A., DiTacchio, L., Bushong, E. A., Gill, S., et al. (2012). Time-restricted feeding without reducing caloric intake prevents metabolic diseases in mice fed a high-fat diet. Cell Metab. 15, 848–860. doi: 10.1016/j.cmet.2012.04.019
Hattar, S., Liao, H. W., Takao, M., Berson, D. M., and Yau, K. W. (2002). Melanopsin-containing retinal ganglion cells: architecture, projections, and intrinsic photosensitivity. Science 295, 1065–1070. doi: 10.1126/science.1069609
Herzog, E. D., Kiss, I. Z., and Mazuski, C. (2015). Measuring synchrony in the mammalian central circadian circuit. Methods Enzymol. 552, 3–22. doi: 10.1016/bs.mie.2014.10.042
Hirota, T., Kon, N., Itagaki, T., Hoshina, N., Okano, T., and Fukada, Y. (2010). Transcriptional repressor TIEG1 regulates Bmal1 gene through GC box and controls circadian clockwork. Genes Cell 15, 111–121. doi: 10.1111/j.1365-2443.2009.01371.x
Hirota, T., Okano, T., Kokame, K., Shirotani-Ikejima, H., Miyata, T., and Fukada, Y. (2002). Glucose down-regulates Per1 and Per2 mRNA levels and induces circadian gene expression in cultured Rat-1 fibroblasts. J. Biol. Chem. 277, 44244–44251. doi: 10.1074/jbc.M206233200
Holmes, M. M., and Mistlberger, R. E. (2000). Food anticipatory activity and photic entrainment in food-restricted BALB/c mice. Physiol. Behav. 68, 655–666. doi: 10.1016/s0031-9384(99)00231-0
Hughes, M. E., Hogenesch, J. B., and Kornacker, K. (2010). JTK_CYCLE: an efficient nonparametric algorithm for detecting rhythmic components in genome-scale data sets. J. Biol. Rhythms 25, 372–380. doi: 10.1177/0748730410379711
Imamura, K., Yoshitane, H., Hattori, K., Yamaguchi, M., Yoshida, K., Okubo, T., et al. (2018). ASK family kinases mediate cellular stress and redox signaling to circadian clock. Proc. Natl. Acad. Sci. U.S.A. 115, 3646–3651. doi: 10.1073/pnas.1719298115
Inagaki, N., Honma, S., Ono, D., Tanahashi, Y., and Honma, K. (2007). Separate oscillating cell groups in mouse suprachiasmatic nucleus couple photoperiodically to the onset and end of daily activity. Proc. Natl. Acad. Sci. U.S.A. 104, 7664–7669. doi: 10.1073/pnas.0607713104
Ishida, A., Mutoh, T., Ueyama, T., Bando, H., Masubuchi, S., Nakahara, D., et al. (2005). Light activates the adrenal gland: timing of gene expression and glucocorticoid release. Cell Metab. 2, 297–307. doi: 10.1016/j.cmet.2005.09.009
Isojima, Y., Nakajima, M., Ukai, H., Fujishima, H., Yamada, R. G., Masumoto, K. H., et al. (2009). CKIepsilon/delta-dependent phosphorylation is a temperature-insensitive, period-determining process in the mammalian circadian clock. Proc. Natl. Acad. Sci. U.S.A. 106, 15744–15749. doi: 10.1073/pnas.0908733106
Izumo, M., Johnson, C. H., and Yamazaki, S. (2003). Circadian gene expression in mammalian fibroblasts revealed by real-time luminescence reporting: temperature compensation and damping. Proc. Natl. Acad. Sci. U.S.A. 100, 16089–16094. doi: 10.1073/pnas.2536313100
Izumo, M., Sato, T. R., Straume, M., and Johnson, C. H. (2006). Quantitative analyses of circadian gene expression in mammalian cell cultures. PLoS Comput. Biol. 2:e136. doi: 10.1371/journal.pcbi.0020136
Jagannath, A., Butler, R., Godinho, S. I. H., Couch, Y., Brown, L. A., Vasudevan, S. R., et al. (2013). The CRTC1-SIK1 pathway regulates entrainment of the circadian clock. Cell 154, 1100–1111. doi: 10.1016/j.cell.2013.08.004
Johnson, C. H. (1999). Forty years of PRCs–what have we learned? Chronobiol. Int. 16, 711–743. doi: 10.3109/07420529909016940
Johnson, C. H., Elliott, J. A., and Foster, R. (2003). Entrainment of circadian programs. Chronobiol. Int. 20, 741–774. doi: 10.1081/cbi-120024211
Jones, C. R., Campbell, S. S., Zone, S. E., Cooper, F., DeSano, A., Murphy, P. J., et al. (1999). Familial advanced sleep-phase syndrome: a short-period circadian rhythm variant in humans. Nat. Med. 5, 1062–1065. doi: 10.1038/12502
Kalsbeek, A., Palm, I. F., La Fleur, S. E., Scheer, F. A., Perreau-Lenz, S., Ruiter, M., et al. (2006). SCN outputs and the hypothalamic balance of life. J. Biol. Rhythms 21, 458–469. doi: 10.1177/0748730406293854
Kennaway, D. J. (2004). Resetting the suprachiasmatic nucleus clock. Front. Biosci. 9, 56–62. doi: 10.2741/1200
Kettner, N. M., Mayo, S. A., Hua, J., Lee, C., Moore, D. D., and Fu, L. (2015). Circadian dysfunction induces leptin resistance in mice. Cell Metab. 22, 448–459. doi: 10.1016/j.cmet.2015.06.005
Ki, Y., Ri, H., Lee, H., Yoo, E., Choe, J., and Lim, C. (2015). Warming up your tick-tock: temperature-dependent regulation of circadian clocks. Neuroscientist 21, 503–518. doi: 10.1177/1073858415577083
Kim, D. Y., Choi, H. J., Kim, J. S., Kim, Y. S., Jeong, D. U., Shin, H. C., et al. (2005). Voltage-gated calcium channels play crucial roles in the glutamate-induced phase shifts of the rat suprachiasmatic circadian clock. Eur. J. Neurosci. 21, 1215–1222. doi: 10.1111/j.1460-9568.2005.03950.x
Kim, M., Subramanian, M., Cho, Y. H., Kim, G. H., Lee, E., and Park, J. J. (2018). Short-term exposure to dim light at night disrupts rhythmic behaviors and causes neurodegeneration in fly models of tauopathy and Alzheimer’s disease. Biochem. Biophys. Res. Commun. 495, 1722–1729. doi: 10.1016/j.bbrc.2017.12.021
Kohsaka, A., Laposky, A. D., Ramsey, K. M., Estrada, C., Joshu, C., Kobayashi, Y., et al. (2007). High-fat diet disrupts behavioral and molecular circadian rhythms in mice. Cell Metab. 6, 414–421. doi: 10.1016/j.cmet.2007.09.006
Kon, N., Hirota, T., Kawamoto, T., Kato, Y., Tsubota, T., and Fukada, Y. (2008). Activation of TGF-beta/activin signalling resets the circadian clock through rapid induction of Dec1 transcripts. Nat. Cell Biol. 10, 1463–1469. doi: 10.1038/ncb1806
Kornmann, B., Schaad, O., Bujard, H., Takahashi, J. S., and Schibler, U. (2007). System-driven and oscillator-dependent circadian transcription in mice with a conditionally active liver clock. PLoS Biol. 5:e34. doi: 10.1371/journal.pbio.0050034
Kowalska, E., Ripperger, J. A., Hoegger, D. C., Bruegger, P., Buch, T., Birchler, T., et al. (2013). NONO couples the circadian clock to the cell cycle. Proc. Natl. Acad. Sci. U.S.A. 110, 1592–1599. doi: 10.1073/pnas.1213317110
Lamia, K. A., Sachdeva, U. M., DiTacchio, L., Williams, E. C., Alvarez, J. G., Egan, D. F., et al. (2009). AMPK regulates the circadian clock by cryptochrome phosphorylation and degradation. Science 326, 437–440. doi: 10.1126/science.1172156
Lande-Diner, L., Stewart-Ornstein, J., Weitz, C. J., and Lahav, G. (2015). Single-cell analysis of circadian dynamics in tissue explants. Mol. Biol. Cell 26, 3940–3945. doi: 10.1091/mbc.E15-06-0403
Leloup, J. C., and Goldbeter, A. (2013). Critical phase shifts slow down circadian clock recovery: implications for jet lag. J. Theor. Biol. 333, 47–57. doi: 10.1016/j.jtbi.2013.04.039
Leming, M. T., Rund, S. S., Behura, S. K., Duffield, G. E., and O’Tousa, J. E. (2014). A database of circadian and diel rhythmic gene expression in the yellow fever mosquito Aedes aegypti. BMC Genomics 15:1128. doi: 10.1186/1471-2164-15-1128
LeSauter, J., Hoque, N., Weintraub, M., Pfaff, D. W., and Silver, R. (2009). Stomach ghrelin-secreting cells as food-entrainable circadian clocks. Proc. Natl. Acad. Sci. U.S.A. 106, 13582–13587. doi: 10.1073/pnas.0906426106
Levine, J. D., Funes, P., Dowse, H. B., and Hall, J. C. (2002). Signal analysis of behavioral and molecular cycles. BMC Neurosci. 3:1. doi: 10.1186/1471-2202-3-1
Lewy, A. J., Hasler, B. P., Emens, J. S., and Sack, R. L. (2001). Pretreatment circadian period in free-running blind people may predict the phase angle of entrainment to melatonin. Neurosci. Lett. 313, 158–160. doi: 10.1016/s0304-3940(01)02261-3
Li, S., Shui, K., Zhang, Y., Lv, Y., Deng, W., Ullah, S., et al. (2017). CGDB: a database of circadian genes in eukaryotes. Nucleic Acids Res. 45, D397–D403. doi: 10.1093/nar/gkw1028
Li, X., Shi, L., Zhang, K., Wei, W., Liu, Q., Mao, F., et al. (2018). CirGRDB: a database for the genome-wide deciphering circadian genes and regulators. Nucleic Acids Res. 46, D64–D70. doi: 10.1093/nar/gkx944
Liu, A. C., Welsh, D. K., Ko, C. H., Tran, H. G., Zhang, E. E., Priest, A. A., et al. (2007). Intercellular coupling confers robustness against mutations in the SCN circadian clock network. Cell 129, 605–616. doi: 10.1016/j.cell.2007.02.047
Logan, R. W., and McClung, C. A. (2019). Rhythms of life: circadian disruption and brain disorders across the lifespan. Nat. Rev. Neurosci. 20, 49–65. doi: 10.1038/s41583-018-0088-y
Loh, D. H., Dragich, J. M., Kudo, T., Schroeder, A. M., Nakamura, T. J., Waschek, J. A., et al. (2011). Effects of vasoactive intestinal peptide genotype on circadian gene expression in the suprachiasmatic nucleus and peripheral organs. J. Biol. Rhythms 26, 200–209. doi: 10.1177/0748730411401740
Lucassen, E. A., Coomans, C. P., van Putten, M., de Kreij, S. R., van Genugten, J. H., Sutorius, R. P., et al. (2016). Environmental 24-hr cycles are essential for health. Curr. Biol. 26, 1843–1853. doi: 10.1016/j.cub.2016.05.038
Manoogian, E. N. C., and Panda, S. (2017). Circadian rhythms, time-restricted feeding, and healthy aging. Ageing Res. Rev. 39, 59–67. doi: 10.1016/j.arr.2016.12.006
Martino, T. A., Oudit, G. Y., Herzenberg, A. M., Tata, N., Koletar, M. M., Kabir, G. M., et al. (2008). Circadian rhythm disorganization produces profound cardiovascular and renal disease in hamsters. Am. J. Physiol. Regul. Integr. Comp. Physiol. 294, R1675–R1683. doi: 10.1152/ajpregu.00829.2007
Matsuo, T., Yamaguchi, S., Mitsui, S., Emi, A., Shimoda, F., and Okamura, H. (2003). Control mechanism of the circadian clock for timing of cell division in vivo. Science 302, 255–259. doi: 10.1126/science.1086271
Matsu-Ura, T., Dovzhenok, A., Aihara, E., Rood, J., Le, H., Ren, Y., et al. (2016). Intercellular coupling of the cell cycle and circadian clock in adult stem cell culture. Mol. Cell 64, 900–912. doi: 10.1016/j.molcel.2016.10.015
McHill, A. W., Melanson, E. L., Higgins, J., Connick, E., Moehlman, T. M., Stothard, E. R., et al. (2014). Impact of circadian misalignment on energy metabolism during simulated nightshift work. Proc. Natl. Acad. Sci. U.S.A. 111, 17302–17307. doi: 10.1073/pnas.1412021111
Meijer, J. H., and Schwartz, W. J. (2003). In search of the pathways for light-induced pacemaker resetting in the suprachiasmatic nucleus. J. Biol. Rhythms 18, 235–249. doi: 10.1177/0748730403018003006
Menaker, M., Murphy, Z. C., and Sellix, M. T. (2013). Central control of peripheral circadian oscillators. Curr. Opin. Neurobiol. 23, 741–746. doi: 10.1016/j.conb.2013.03.003
Mendoza, J. (2007). Circadian clocks: setting time by food. J. Neuroendocrinol. 19, 127–137. doi: 10.1111/j.1365-2826.2006.01510.x
Mendoza, J., Drevet, K., Pevet, P., and Challet, E. (2008). Daily meal timing is not necessary for resetting the main circadian clock by calorie restriction. J. Neuroendocrinol. 20, 251–260. doi: 10.1111/j.1365-2826.2007.01636.x
Michel, S., and Colwell, C. S. (2001). Cellular communication and coupling within the suprachiasmatic nucleus. Chronobiol. Int. 18, 579–600. doi: 10.1081/cbi-100106074
Mieda, M. (2019). The network mechanism of the central circadian pacemaker of the SCN: do avp neurons play a more critical role than expected? Front. Neurosci. 13:139. doi: 10.3389/fnins.2019.00139
Mieda, M., Williams, S. C., Sinton, C. M., Richardson, J. A., Sakurai, T., and Yanagisawa, M. (2004). Orexin neurons function in an efferent pathway of a food-entrainable circadian oscillator in eliciting food-anticipatory activity and wakefulness. J. Neurosci. 24, 10493–10501. doi: 10.1523/jneurosci.3171-04.2004
Mikkelsen, J. D., Larsen, P. J., and Ebling, F. J. (1993). Distribution of N-methyl D-aspartate (n.d.) receptor mRNAs in the rat suprachiasmatic nucleus. Brain Res. 632, 329–333. doi: 10.1016/0006-8993(93)91171-n
Mistlberger, R. E. (1991). Effects of daily schedules of forced activity on free-running rhythms in the rat. J. Biol. Rhythms 6, 71–80. doi: 10.1177/074873049100600108
Mistlberger, R. E., Belcourt, J., and Antle, M. C. (2002). Circadian clock resetting by sleep deprivation without exercise in Syrian hamsters: dark pulses revisited. J. Biol. Rhythms 17, 227–237. doi: 10.1177/07430402017003006
Mistlberger, R. E., and Skene, D. J. (2004). Social influences on mammalian circadian rhythms: animal and human studies. Biol. Rev. Camb. Philos. Soc. 79, 533–556. doi: 10.1017/s1464793103006353
Mitsui, S., Yamaguchi, S., Matsuo, T., Ishida, Y., and Okamura, H. (2001). Antagonistic role of E4BP4 and PAR proteins in the circadian oscillatory mechanism. Genes Dev. 15, 995–1006. doi: 10.1101/gad.873501
Moga, M. M., and Moore, R. Y. (1997). Organization of neural inputs to the suprachiasmatic nucleus in the rat. J. Comp. Neurol. 389, 508–534. doi: 10.1002/(sici)1096-9861(19971222)389:3<508::aid-cne11>3.0.co;2-h
Mohawk, J. A., Green, C. B., and Takahashi, J. S. (2012). Central and peripheral circadian clocks in mammals. Annu. Rev. Neurosci. 35, 445–462. doi: 10.1146/annurev-neuro-060909-153128
Momma, T., Okayama, H., Saitou, M., Sugeno, H., Yoshimoto, N., Takebayashi, Y., et al. (2017). Expression of circadian clock genes in human colorectal adenoma and carcinoma. Oncol. Lett. 14, 5319–5325. doi: 10.3892/ol.2017.6876
Moore, A., Zielinski, T., and Millar, A. J. (2014). Online period estimation and determination of rhythmicity in circadian data, using the BioDare data infrastructure. Methods Mol. Biol. 1158, 13–44. doi: 10.1007/978-1-4939-0700-7_2
Moore, R. Y., Speh, J. C., and Leak, R. K. (2002). Suprachiasmatic nucleus organization. Cell Tissue Res. 309, 89–98. doi: 10.1007/s00441-002-0575-2
Morin, L. P., Shivers, K. Y., Blanchard, J. H., and Muscat, L. (2006). Complex organization of mouse and rat suprachiasmatic nucleus. Neuroscience 137, 1285–1297. doi: 10.1016/j.neuroscience.2005.10.030
Moriya, T., Aida, R., Kudo, T., Akiyama, M., Doi, M., Hayasaka, N., et al. (2009). The dorsomedial hypothalamic nucleus is not necessary for food-anticipatory circadian rhythms of behavior, temperature or clock gene expression in mice. Eur. J. Neurosci. 29, 1447–1460. doi: 10.1111/j.1460-9568.2009.06697.x
Morris, C. J., Yang, J. N., Garcia, J. I., Myers, S., Bozzi, I., Wang, W., et al. (2015). Endogenous circadian system and circadian misalignment impact glucose tolerance via separate mechanisms in humans. Proc. Natl. Acad. Sci. U.S.A. 112, E2225–E2234. doi: 10.1073/pnas.1418955112
Mouland, J. W., Stinchcombe, A. R., Forger, D. B., Brown, T. M., and Lucas, R. J. (2017). Responses to spatial contrast in the mouse suprachiasmatic nuclei. Curr. Biol. 27, 1633–1640.e3. doi: 10.1016/j.cub.2017.04.039
Mrosovsky, N., Lucas, R. J., and Foster, R. G. (2001). Persistence of masking responses to light in mice lacking rods and cones. J. Biol. Rhythms 16, 585–588. doi: 10.1177/074873001129002277
Mukherji, A., Kobiita, A., Ye, T., and Chambon, P. (2013). Homeostasis in intestinal epithelium is orchestrated by the circadian clock and microbiota cues transduced by TLRs. Cell 153, 812–827. doi: 10.1016/j.cell.2013.04.020
Nagoshi, E., Saini, C., Bauer, C., Laroche, T., Naef, F., and Schibler, U. (2004). Circadian gene expression in individual fibroblasts: cell-autonomous and self-sustained oscillators pass time to daughter cells. Cell 119, 693–705. doi: 10.1016/j.cell.2004.11.015
Nagy, Z., Marta, A., Butz, H., Liko, I., Racz, K., and Patocs, A. (2016). Modulation of the circadian clock by glucocorticoid receptor isoforms in the H295R cell line. Steroids 116, 20–27. doi: 10.1016/j.steroids.2016.10.002
Narasimamurthy, R., and Virshup, D. M. (2017). Molecular mechanisms regulating temperature compensation of the circadian clock. Front. Neurol. 8:161. doi: 10.3389/fneur.2017.00161
Noguchi, T., Wang, L. L., and Welsh, D. K. (2013). Fibroblast PER2 circadian rhythmicity depends on cell density. J. Biol. Rhythms 28, 183–192. doi: 10.1177/0748730413487494
Novakova, M., Sladek, M., and Sumova, A. (2010). Exposure of pregnant rats to restricted feeding schedule synchronizes the SCN clocks of their fetuses under constant light but not under a light-dark regime. J. Biol. Rhythms 25, 350–360. doi: 10.1177/0748730410377967
Ohnishi, N., Tahara, Y., Kuriki, D., Haraguchi, A., and Shibata, S. (2014). Warm water bath stimulates phase-shifts of the peripheral circadian clocks in PER2::Luciferase mouse. PLoS One 9:e100272. doi: 10.1371/journal.pone.0100272
Oike, H., Nagai, K., Fukushima, T., Ishida, N., and Kobori, M. (2010). High-salt diet advances molecular circadian rhythms in mouse peripheral tissues. Biochem. Biophys. Res. Commun. 402, 7–13. doi: 10.1016/j.bbrc.2010.09.072
Oike, H., Oishi, K., and Kobori, M. (2014). Nutrients, clock genes, and chrononutrition. Curr. Nutr. Rep. 3, 204–212. doi: 10.1007/s13668-014-0082-6
O’Keeffe, S. M., Thome, J., and Coogan, A. N. (2012). The noradrenaline reuptake inhibitor atomoxetine phase-shifts the circadian clock in mice. Neuroscience 201, 219–230. doi: 10.1016/j.neuroscience.2011.11.002
Pan, A., Schernhammer, E. S., Sun, Q., and Hu, F. B. (2011). Rotating night shift work and risk of type 2 diabetes: two prospective cohort studies in women. PLoS Med. 8:e1001141. doi: 10.1371/journal.pmed.1001141
Panda, S., Hogenesch, J. B., and Kay, S. A. (2002). Circadian rhythms from flies to human. Nature 417, 329–335. doi: 10.1038/417329a
Patel, S. A., Chaudhari, A., Gupta, R., Velingkaar, N., and Kondratov, R. V. (2016). Circadian clocks govern calorie restriction-mediated life span extension through BMAL1- and IGF-1-dependent mechanisms. FASEB J. 30, 1634–1642. doi: 10.1096/fj.15-282475
Patel, V. R., Eckel-Mahan, K., Sassone-Corsi, P., and Baldi, P. (2012). CircadiOmics: integrating circadian genomics, transcriptomics, proteomics and metabolomics. Nat. Methods 9, 772–773. doi: 10.1038/nmeth.2111
Paul, K. N., Fukuhara, C., Karom, M., Tosini, G., and Albers, H. E. (2005). AMPA/kainate receptor antagonist DNQX blocks the acute increase of Per2 mRNA levels in most but not all areas of the SCN. Brain Res. Mol. Brain Res. 139, 129–136. doi: 10.1016/j.molbrainres.2005.05.017
Peirson, S. N., Thompson, S., Hankins, M. W., and Foster, R. G. (2005). Mammalian photoentrainment: results, methods, and approaches. Methods Enzymol. 393, 697–726. doi: 10.1016/s0076-6879(05)93037-1
Pembroke, W. G., Babbs, A., Davies, K. E., Ponting, C. P., and Oliver, P. L. (2015). Temporal transcriptomics suggest that twin-peaking genes reset the clock. eLife 4:e10518. doi: 10.7554/eLife.10518
Pendergast, J. S., and Yamazaki, S. (2018). The mysterious food-entrainable oscillator: insights from mutant and engineered mouse models. J. Biol. Rhythms 33, 458–474. doi: 10.1177/0748730418789043
Penev, P. D., Kolker, D. E., Zee, P. C., and Turek, F. W. (1998). Chronic circadian desynchronization decreases the survival of animals with cardiomyopathic heart disease. Am. J. Physiol. 275, H2334–H2337. doi: 10.1152/ajpheart.1998.275.6.H2334
Peytevin, J., Aioun, J., and Chambille, I. (2000). Neurons that express the AMPA receptor GluR2/3 subunits in suprachiasmatic nuclei of Syrian hamsters colocalize either vasoactive intestinal peptide, peptide histidine isoleucine or gastrin-releasing peptide. Cell Tissue Res. 300, 345–359. doi: 10.1007/s004410000209
Pilorz, V., Tam, S. K., Hughes, S., Pothecary, C. A., Jagannath, A., Hankins, M. W., et al. (2016). Melanopsin regulates both sleep-promoting and arousal-promoting responses to light. PLoS Biol. 14:e1002482. doi: 10.1371/journal.pbio.1002482
Pizarro, A., Hayer, K., Lahens, N. F., and Hogenesch, J. B. (2013). CircaDB: a database of mammalian circadian gene expression profiles. Nucleic Acids Res. 41, D1009–D1013. doi: 10.1093/nar/gks1161
Poletini, M. O., Moraes, M. N., Ramos, B. C., Jeronimo, R., and Castrucci, A. M. (2015). TRP channels: a missing bond in the entrainment mechanism of peripheral clocks throughout evolution. Temperature 2, 522–534. doi: 10.1080/23328940.2015.1115803
Polo, A., Singh, S., Crispo, A., Russo, M., Giudice, A., Montella, M., et al. (2017). Evaluating the associations between human circadian rhythms and dysregulated genes in liver cancer cells. Oncol. Lett. 14, 7353–7359. doi: 10.3892/ol.2017.7109
Preitner, N., Damiola, F., Lopez-Molina, L., Zakany, J., Duboule, D., Albrecht, U., et al. (2002). The orphan nuclear receptor REV-ERBalpha controls circadian transcription within the positive limb of the mammalian circadian oscillator. Cell 110, 251–260. doi: 10.1016/s0092-8674(02)00825-5
Provencio, I., Rodriguez, I. R., Jiang, G., Hayes, W. P., Moreira, E. F., and Rollag, M. D. (2000). A novel human opsin in the inner retina. J. Neurosci. 20, 600–605. doi: 10.1523/jneurosci.20-02-00600.2000
Reddy, A. B., Maywood, E. S., Karp, N. A., King, V. M., Inoue, Y., Gonzalez, F. J., et al. (2007). Glucocorticoid signaling synchronizes the liver circadian transcriptome. Hepatology 45, 1478–1488. doi: 10.1002/hep.21571
Refinetti, R. (2010). Entrainment of circadian rhythm by ambient temperature cycles in mice. J. Biol. Rhythms 25, 247–256. doi: 10.1177/0748730410372074
Reid, K. J., Chang, A. M., Dubocovich, M. L., Turek, F. W., Takahashi, J. S., and Zee, P. C. (2001). Familial advanced sleep phase syndrome. Arch. Neurol. 58, 1089–1094.
Reinke, H., Saini, C., Fleury-Olela, F., Dibner, C., Benjamin, I. J., and Schibler, U. (2008). Differential display of DNA-binding proteins reveals heat-shock factor 1 as a circadian transcription factor. Genes Dev. 22, 331–345. doi: 10.1101/gad.453808
Reppert, S. M., and Weaver, D. R. (2002). Coordination of circadian timing in mammals. Nature 418, 935–941. doi: 10.1038/nature00965
Riedel, C. S., Georg, B., Jorgensen, H. L., Hannibal, J., and Fahrenkrug, J. (2018). Mice lacking EGR1 have impaired clock gene (BMAL1) oscillation, locomotor activity, and body temperature. J. Mol. Neurosci. 64, 9–19. doi: 10.1007/s12031-017-0996-8
Roenneberg, T., Daan, S., and Merrow, M. (2003). The art of entrainment. J. Biol. Rhythms 18, 183–194. doi: 10.1177/0748730403018003001
Roenneberg, T., and Foster, R. G. (1997). Twilight times: light and the circadian system. Photochem. Photobiol. 66, 549–561. doi: 10.1111/j.1751-1097.1997.tb03188.x
Roenneberg, T., and Merrow, M. (2016). The circadian clock and human health. Curr. Biol. 26, R432–R443. doi: 10.1016/j.cub.2016.04.011
Rogers, E. H., Fawcett, S. A., Pekovic-Vaughan, V., and Hunt, J. A. (2017). Comparing circadian dynamics in primary derived stem cells from different sources of human adult tissue. Stem Cell Int. 2017:2057168. doi: 10.1155/2017/2057168
Rutter, J., Reick, M., and McKnight, S. L. (2002). Metabolism and the control of circadian rhythms. Annu. Rev. Biochem. 71, 307–331. doi: 10.1146/annurev.biochem.71.090501.142857
Rutter, J., Reick, M., Wu, L. C., and McKnight, S. L. (2001). Regulation of clock and NPAS2 DNA binding by the redox state of NAD cofactors. Science 293, 510–514. doi: 10.1126/science.1060698
Saini, C., Liani, A., Curie, T., Gos, P., Kreppel, F., Emmenegger, Y., et al. (2013). Real-time recording of circadian liver gene expression in freely moving mice reveals the phase-setting behavior of hepatocyte clocks. Genes Dev. 27, 1526–1536. doi: 10.1101/gad.221374.113
Saini, C., Morf, J., Stratmann, M., Gos, P., and Schibler, U. (2012). Simulated body temperature rhythms reveal the phase-shifting behavior and plasticity of mammalian circadian oscillators. Genes Dev. 26, 567–580. doi: 10.1101/gad.183251.111
Sasaki, H., Hattori, Y., Ikeda, Y., Kamagata, M., Iwami, S., Yasuda, S., et al. (2016). Forced rather than voluntary exercise entrains peripheral clocks via a corticosterone/noradrenaline increase in PER2::LUC mice. Sci. Rep. 6:27607. doi: 10.1038/srep27607
Sato, T. K., Panda, S., Miraglia, L. J., Reyes, T. M., Rudic, R. D., McNamara, P., et al. (2004). A functional genomics strategy reveals rora as a component of the mammalian circadian clock. Neuron 43, 527–537. doi: 10.1016/j.neuron.2004.07.018
Saxena, R., Voight, B. F., Lyssenko, V., Burtt, N. P., de Bakker, P. I., Chen, H., et al. (2007). Genome-wide association analysis identifies loci for type 2 diabetes and triglyceride levels. Science 316, 1331–1336. doi: 10.1126/science.1142358
Scheer, F. A., Hilton, M. F., Mantzoros, C. S., and Shea, S. A. (2009). Adverse metabolic and cardiovascular consequences of circadian misalignment. Proc. Natl. Acad. Sci. U.S.A. 106, 4453–4458. doi: 10.1073/pnas.0808180106
Schibler, U., and Naef, F. (2005). Cellular oscillators: rhythmic gene expression and metabolism. Curr. Opin. Cell Biol. 17, 223–229. doi: 10.1016/j.ceb.2005.01.007
Schmidt, T. M., Do, M. T., Dacey, D., Lucas, R., Hattar, S., and Matynia, A. (2011). Melanopsin-positive intrinsically photosensitive retinal ganglion cells: from form to function. J. Neurosci. 31, 16094–16101. doi: 10.1523/jneurosci.4132-11.2011
Schnell, A., Chappuis, S., Schmutz, I., Brai, E., Ripperger, J. A., Schaad, O., et al. (2014). The nuclear receptor REV-ERBalpha regulates Fabp7 and modulates adult hippocampal neurogenesis. PLoS One 9:e99883. doi: 10.1371/journal.pone.0099883
Scott, E. M., Carter, A. M., and Grant, P. J. (2008). Association between polymorphisms in the Clock gene, obesity and the metabolic syndrome in man. Int. J. Obes. 32, 658–662. doi: 10.1038/sj.ijo.0803778
Segall, L. A., Perrin, J. S., Walker, C. D., Stewart, J., and Amir, S. (2006). Glucocorticoid rhythms control the rhythm of expression of the clock protein, Period2, in oval nucleus of the bed nucleus of the stria terminalis and central nucleus of the amygdala in rats. Neuroscience 140, 753–757. doi: 10.1016/j.neuroscience.2006.03.037
Sellix, M. T., Currie, J., Menaker, M., and Wijnen, H. (2010). Fluorescence/luminescence circadian imaging of complex tissues at single-cell resolution. J. Biol. Rhythms 25, 228–232. doi: 10.1177/0748730410368016
Shen, S., Spratt, C., Sheward, W. J., Kallo, I., West, K., Morrison, C. F., et al. (2000). Overexpression of the human VPAC2 receptor in the suprachiasmatic nucleus alters the circadian phenotype of mice. Proc. Natl. Acad. Sci. U.S.A. 97, 11575–11580. doi: 10.1073/pnas.97.21.11575
Sherman, H., Genzer, Y., Cohen, R., Chapnik, N., Madar, Z., and Froy, O. (2012). Timed high-fat diet resets circadian metabolism and prevents obesity. FASEB J. 26, 3493–3502. doi: 10.1096/fj.12-208868
Shim, H. S., Kim, H., Lee, J., Son, G. H., Cho, S., Oh, T. H., et al. (2007). Rapid activation of CLOCK by Ca2+-dependent protein kinase C mediates resetting of the mammalian circadian clock. EMBO Rep. 8, 366–371. doi: 10.1038/sj.embor.7400920
Smyllie, N. J., Pilorz, V., Boyd, J., Meng, Q. J., Saer, B., Chesham, J. E., et al. (2016). Visualizing and quantifying intracellular behavior and abundance of the core circadian clock protein period2. Curr. Biol. 26, 1880–1886. doi: 10.1016/j.cub.2016.05.018
So, A. Y., Bernal, T. U., Pillsbury, M. L., Yamamoto, K. R., and Feldman, B. J. (2009). Glucocorticoid regulation of the circadian clock modulates glucose homeostasis. Proc. Natl. Acad. Sci. U.S.A. 106, 17582–17587. doi: 10.1073/pnas.0909733106
Sporl, F., Schellenberg, K., Blatt, T., Wenck, H., Wittern, K. P., Schrader, A., et al. (2011). A circadian clock in HaCaT keratinocytes. J. Invest. Dermatol. 131, 338–348. doi: 10.1038/jid.2010.315
Stenvers, D. J., Scheer, F., Schrauwen, P., la Fleur, S. E., and Kalsbeek, A. (2018). Circadian clocks and insulin resistance. Nat. Rev. Endocrinol. 15, 75–89. doi: 10.1038/s41574-018-0122-1
Stepanyuk, A. R., Belan, P. V., and Kononenko, N. I. (2014). A model for the fast synchronous oscillations of firing rate in rat suprachiasmatic nucleus neurons cultured in a multielectrode array dish. PLoS One 9:e106152. doi: 10.1371/journal.pone.0106152
Stokkan, K. A., Yamazaki, S., Tei, H., Sakaki, Y., and Menaker, M. (2001). Entrainment of the circadian clock in the liver by feeding. Science 291, 490–493. doi: 10.1126/science.291.5503.490
Straume, M. (2004). DNA microarray time series analysis: automated statistical assessment of circadian rhythms in gene expression patterning. Methods Enzymol. 383, 149–166. doi: 10.1016/s0076-6879(04)83007-6
Tahara, Y., Aoyama, S., and Shibata, S. (2017). The mammalian circadian clock and its entrainment by stress and exercise. J. Physiol. Sci. 67, 1–10. doi: 10.1007/s12576-016-0450-7
Tahara, Y., Kuroda, H., Saito, K., Nakajima, Y., Kubo, Y., Ohnishi, N., et al. (2012). In vivo monitoring of peripheral circadian clocks in the mouse. Curr. Biol. 22, 1029–1034. doi: 10.1016/j.cub.2012.04.009
Tahara, Y., and Shibata, S. (2018). Entrainment of the mouse circadian clock: effects of stress, exercise, and nutrition. Free Radic. Biol. Med. 119, 129–138. doi: 10.1016/j.freeradbiomed.2017.12.026
Tahara, Y., Shiraishi, T., Kikuchi, Y., Haraguchi, A., Kuriki, D., Sasaki, H., et al. (2015). Entrainment of the mouse circadian clock by sub-acute physical and psychological stress. Sci. Rep. 5:11417. doi: 10.1038/srep11417
Takahashi, J. S. (2017). Transcriptional architecture of the mammalian circadian clock. Nat. Rev. Genet. 18, 164–179. doi: 10.1038/nrg.2016.150
Takahashi, J. S., Hong, H. K., Ko, C. H., and McDearmon, E. L. (2008). The genetics of mammalian circadian order and disorder: implications for physiology and disease. Nat. Rev. Genet. 9, 764–775. doi: 10.1038/nrg2430
Takarada, T., Xu, C., Ochi, H., Nakazato, R., Yamada, D., Nakamura, S., et al. (2017). Bone resorption is regulated by circadian clock in osteoblasts. J. Bone Miner. Res. 32, 872–881. doi: 10.1002/jbmr.3053
Tamaru, T., Hattori, M., Honda, K., Benjamin, I., Ozawa, T., and Takamatsu, K. (2011). Synchronization of circadian Per2 rhythms and HSF1-BMAL1:CLOCK interaction in mouse fibroblasts after short-term heat shock pulse. PLoS One 6:e24521. doi: 10.1371/journal.pone.0024521
Tamaru, T., Hattori, M., Ninomiya, Y., Kawamura, G., Vares, G., Honda, K., et al. (2013). ROS stress resets circadian clocks to coordinate pro-survival signals. PLoS One 8:e82006. doi: 10.1371/journal.pone.0082006
Tang, Q., Cheng, B., Xie, M., Chen, Y., Zhao, J., Zhou, X., et al. (2017). Circadian clock gene bmal1 inhibits tumorigenesis and increases paclitaxel sensitivity in tongue squamous cell carcinoma. Cancer Res. 77, 532–544. doi: 10.1158/0008-5472.can-16-1322
Travnickova-Bendova, Z., Cermakian, N., Reppert, S. M., and Sassone-Corsi, P. (2002). Bimodal regulation of mPeriod promoters by CREB-dependent signaling and CLOCK/BMAL1 activity. Proc. Natl. Acad. Sci. U.S.A. 99, 7728–7733. doi: 10.1073/pnas.102075599
Tsuchiya, Y., Minami, I., Kadotani, H., and Nishida, E. (2005). Resetting of peripheral circadian clock by prostaglandin E2. EMBO Rep. 6, 256–261. doi: 10.1038/sj.embor.7400356
Tulina, N. M., Chen, W. F., Chen, J. H., Sowcik, M., and Sehgal, A. (2014). Day-night cycles and the sleep-promoting factor, sleepless, affect stem cell activity in the Drosophila testis. Proc. Natl. Acad. Sci. U.S.A. 111, 3026–3031. doi: 10.1073/pnas.1316552111
van der Horst, G. T., Muijtjens, M., Kobayashi, K., Takano, R., Kanno, S., Takao, M., et al. (1999). Mammalian Cry1 and Cry2 are essential for maintenance of circadian rhythms. Nature 398, 627–630. doi: 10.1038/19323
van Diepen, H. C., Foster, R. G., and Meijer, J. H. (2015). A colourful clock. PLoS Biol. 13:e1002160. doi: 10.1371/journal.pbio.1002160
VanderLeest, H. T., Houben, T., Michel, S., Deboer, T., Albus, H., Vansteensel, M. J., et al. (2007). Seasonal encoding by the circadian pacemaker of the SCN. Curr. Biol. 17, 468–473. doi: 10.1016/j.cub.2007.01.048
Vilches, N., Spichiger, C., Mendez, N., Abarzua-Catalan, L., Galdames, H. A., Hazlerigg, D. G., et al. (2014). Gestational chronodisruption impairs hippocampal expression of NMDA receptor subunits Grin1b/Grin3a and spatial memory in the adult offspring. PLoS One 9:e91313. doi: 10.1371/journal.pone.0091313
Voiculescu, S. E., Le Duc, D., Rosca, A. E., Zeca, V., Chitimus, D. M., Arsene, A. L., et al. (2016). Behavioral and molecular effects of prenatal continuous light exposure in the adult rat. Brain Res. 1650, 51–59. doi: 10.1016/j.brainres.2016.08.031
Wang, J. L., Lim, A. S., Chiang, W. Y., Hsieh, W. H., Lo, M. T., Schneider, J. A., et al. (2015). Suprachiasmatic neuron numbers and rest-activity circadian rhythms in older humans. Ann. Neurol. 78, 317–322. doi: 10.1002/ana.24432
Wang, Y., Kuang, Z., Yu, X., Ruhn, K. A., Kubo, M., and Hooper, L. V. (2017). The intestinal microbiota regulates body composition through NFIL3 and the circadian clock. Science 357, 912–916. doi: 10.1126/science.aan0677
Welsh, D. K., and Kay, S. A. (2005). Bioluminescence imaging in living organisms. Curr. Opin. Biotechnol. 16, 73–78. doi: 10.1016/j.copbio.2004.12.006
Welsh, D. K., Takahashi, J. S., and Kay, S. A. (2010). Suprachiasmatic nucleus: cell autonomy and network properties. Annu. Rev. Physiol. 72, 551–577. doi: 10.1146/annurev-physiol-021909-135919
Williams, J., Yang, N., Wood, A., Zindy, E., Meng, Q. J., and Streuli, C. H. (2018). Epithelial and stromal circadian clocks are inversely regulated by their mechano-matrix environment. J. Cell. Sci. 13:jcs208223. doi: 10.1242/jcs.208223
Woods, S. C. (2005). Signals that influence food intake and body weight. Physiol. Behav. 86, 709–716. doi: 10.1016/j.physbeh.2005.08.060
Woon, P. Y., Kaisaki, P. J., Braganca, J., Bihoreau, M. T., Levy, J. C., Farrall, M., et al. (2007). Aryl hydrocarbon receptor nuclear translocator-like (BMAL1) is associated with susceptibility to hypertension and type 2 diabetes. Proc. Natl. Acad. Sci. U.S.A. 104, 14412–14417. doi: 10.1073/pnas.0703247104
Wright, K. P. Jr., Gronfier, C., Duffy, J. F., and Czeisler, C. A. (2005). Intrinsic period and light intensity determine the phase relationship between melatonin and sleep in humans. J. Biol. Rhythms 20, 168–177. doi: 10.1177/0748730404274265
Wu, X., Yu, G., Parks, H., Hebert, T., Goh, B. C., Dietrich, M. A., et al. (2008). Circadian mechanisms in murine and human bone marrow mesenchymal stem cells following dexamethasone exposure. Bone 42, 861–870. doi: 10.1016/j.bone.2007.12.226
Xiong, H., Yang, Y., Yang, K., Zhao, D., Tang, H., and Ran, X. (2018). Loss of the clock gene PER2 is associated with cancer development and altered expression of important tumor-related genes in oral cancer. Int. J. Oncol. 52, 279–287. doi: 10.3892/ijo.2017.4180
Xu, H., Gustafson, C. L., Sammons, P. J., Khan, S. K., Parsley, N. C., Ramanathan, C., et al. (2015). Cryptochrome 1 regulates the circadian clock through dynamic interactions with the BMAL1 C terminus. Nat. Struct. Mol. Biol. 22, 476–484. doi: 10.1038/nsmb.3018
Yagita, K., and Okamura, H. (2000). Forskolin induces circadian gene expression of rPer1, rPer2 and dbp in mammalian rat-1 fibroblasts. FEBS Lett. 465, 79–82. doi: 10.1016/s0014-5793(99)01724-x
Yamaguchi, S., Kobayashi, M., Mitsui, S., Ishida, Y., van der Horst, G. T., Suzuki, M., et al. (2001). View of a mouse clock gene ticking. Nature 409:684. doi: 10.1038/35055628
Yamaguchi, Y., Suzuki, T., Mizoro, Y., Kori, H., Okada, K., Chen, Y., et al. (2013). Mice genetically deficient in vasopressin V1a and V1b receptors are resistant to jet lag. Science 342, 85–90. doi: 10.1126/science.1238599
Yamamoto, T., Nakahata, Y., Tanaka, M., Yoshida, M., Soma, H., Shinohara, K., et al. (2005). Acute physical stress elevates mouse period1 mRNA expression in mouse peripheral tissues via a glucocorticoid-responsive element. J. Biol. Chem. 280, 42036–42043. doi: 10.1074/jbc.M509600200
Yamazaki, S., Numano, R., Abe, M., Hida, A., Takahashi, R., Ueda, M., et al. (2000). Resetting central and peripheral circadian oscillators in transgenic rats. Science 288, 682–685. doi: 10.1126/science.288.5466.682
Yang, N., Williams, J., Pekovic-Vaughan, V., Wang, P., Olabi, S., McConnell, J., et al. (2017). Cellular mechano-environment regulates the mammary circadian clock. Nat. Commun. 8:14287. doi: 10.1038/ncomms14287
Yeom, M., Pendergast, J. S., Ohmiya, Y., and Yamazaki, S. (2010). Circadian-independent cell mitosis in immortalized fibroblasts. Proc. Natl. Acad. Sci. U.S.A. 107, 9665–9670. doi: 10.1073/pnas.0914078107
Yoo, S. H., Yamazaki, S., Lowrey, P. L., Shimomura, K., Ko, C. H., Buhr, E. D., et al. (2004). PERIOD2::luciferase real-time reporting of circadian dynamics reveals persistent circadian oscillations in mouse peripheral tissues. Proc. Natl. Acad. Sci. U.S.A. 101, 5339–5346. doi: 10.1073/pnas.0308709101
Young, J. K., Heinbockel, T., and Gondre-Lewis, M. C. (2013). Astrocyte fatty acid binding protein-7 is a marker for neurogenic niches in the rat hippocampus. Hippocampus 23, 1476–1483. doi: 10.1002/hipo.22200
Zarrinpar, A., Chaix, A., and Panda, S. (2016). Daily eating patterns and their impact on health and disease. Trends Endocrinol. Metab. 27, 69–83. doi: 10.1016/j.tem.2015.11.007
Zeggini, E., Weedon, M. N., Lindgren, C. M., Frayling, T. M., Elliott, K. S., Lango, H., et al. (2007). Replication of genome-wide association signals in UK samples reveals risk loci for type 2 diabetes. Science 316, 1336–1341. doi: 10.1126/science.1142364
Zhang, R., Lahens, N. F., Ballance, H. I., Hughes, M. E., and Hogenesch, J. B. (2014). A circadian gene expression atlas in mammals: implications for biology and medicine. Proc. Natl. Acad. Sci. U.S.A. 111, 16219–16224. doi: 10.1073/pnas.1408886111
Zhao, J., Zhou, X., Tang, Q., Yu, R., Yu, S., Long, Y., et al. (2018). BMAL1 deficiency contributes to mandibular dysplasia by upregulating MMP3. Stem Cell Rep. 10, 180–195. doi: 10.1016/j.stemcr.2017.11.017
Zheng, B., Albrecht, U., Kaasik, K., Sage, M., Lu, W., Vaishnav, S., et al. (2001). Nonredundant roles of the mPer1 and mPer2 genes in the mammalian circadian clock. Cell 105, 683–694. doi: 10.1016/s0092-8674(01)00380-4
Zhou, X., Yu, R., Long, Y., Zhao, J., Yu, S., Tang, Q., et al. (2018). BMAL1 deficiency promotes skeletal mandibular hypoplasia via OPG downregulation. Cell Prolif. 51:e12470. doi: 10.1111/cpr.12470
Zhuang, X., Rambhatla, S. B., Lai, A. G., and McKeating, J. A. (2017). Interplay between circadian clock and viral infection. J. Mol. Med. 95, 1283–1289. doi: 10.1007/s00109-017-1592-7
Keywords: circadian rhythm, synchronization, rhythm monitoring, influence factors, disorder
Citation: Xie Y, Tang Q, Chen G, Xie M, Yu S, Zhao J and Chen L (2019) New Insights Into the Circadian Rhythm and Its Related Diseases. Front. Physiol. 10:682. doi: 10.3389/fphys.2019.00682
Received: 28 January 2019; Accepted: 13 May 2019;
Published: 25 June 2019.
Edited by:
Charlotte Helfrich-Förster, University of Würzburg, GermanyReviewed by:
Urs Albrecht, Université de Fribourg, SwitzerlandVioletta Pilorz, Luebeck University of Applied Sciences, Germany
Copyright © 2019 Xie, Tang, Chen, Xie, Yu, Zhao and Chen. This is an open-access article distributed under the terms of the Creative Commons Attribution License (CC BY). The use, distribution or reproduction in other forums is permitted, provided the original author(s) and the copyright owner(s) are credited and that the original publication in this journal is cited, in accordance with accepted academic practice. No use, distribution or reproduction is permitted which does not comply with these terms.
*Correspondence: Jiajia Zhao, emhhb2ppYWppYTAxMzBAMTYzLmNvbQ==; Lili Chen, bGlseS1jMTAzMEAxNjMuY29t
†These authors have contributed equally to this work