- 1Department of Human Movement Studies, Human Motion Diagnostic Centre, University of Ostrava, Ostrava, Czechia
- 2Sport Performance Research Institute New Zealand (SPRINZ), Auckland University of Technology, Auckland, New Zealand
- 3Exercise Physiology, Training and Training Therapy Research Group, Institute of Sports Science, University of Graz, Graz, Austria
Purpose: The aim of this non-randomized parallel group study was to examine the 12 week effects of a very low-carbohydrate high-fat diet (VLCHF) on maximal cardiorespiratory capacity, high-intensity interval training (HIIT) performance, and cardiac autonomic regulation.
Methods: Twenty-four recreationally trained participants allocated to either a VLCHF (N = 12) or a habitual diet (HD; N = 12) group completed 12 weeks of a diet and exercise (VLCHF) or an exercise only intervention (HD). Maximal graded exercise tests (GXT) were performed at baseline, after 4, 8, and 12 weeks. A supervised HIIT session and the 30-15 Intermittent Fitness Test (30-15IFT) were conducted once a week.
Results: Total time to exhaustion (TTE) in both GXT and 30-15IFT largely increased in both VLCHF (p = 0.005, BF10 = 11.30 and p = 0.001, BF10 ≥ 100, respectively) and HD (p = 0.018, BF10 = 3.87 and p = 0.001, BF10 ≥ 100, respectively) groups after 12 weeks. Absolute maximal oxygen uptake (O2max) was not changed in both groups but relative O2max increased in VLCHF in concert with reductions in body mass (66.7 ± 10.2–63.1 ± 8.5 kg). Cardiac autonomic regulation did not reveal any between-group differences after 12 weeks. VLCHF diet induced an increase in β-hydroxybutyrate, which tended to normalize during the intervention period.
Conclusion: The 12 week VLCHF diet did not impair high-intensity continuous or intermittent exercise lasting up to 25 min, nor did it impair maximal cardiorespiratory performance or autonomic nervous system (ANS) activity.
Introduction
The very-low carbohydrate high fat (VLCHF) diet is a nutritional approach consisting of restricted carbohydrate (CHO) consumption and increased fat intake, with protein consumption and total energy intake preserved (Feinman et al., 2015). These changes contrast a traditional Western high CHO diet and cause a shift toward using more fat as a fuel source (Paoli et al., 2015), with promising potential shown for treating chronic metabolic and cardiovascular diseases (Mckenzie et al., 2017; Miller et al., 2018).
The VLCHF diet approach may also be of interest for conditioning coaches seeking methods of enhancing sports performance. The positive effects of the VLCHF diet on aerobic performance in sufficiently VLCHF diet adapted athletes have been described (Zajac et al., 2014; Paoli et al., 2015; Volek et al., 2015), however, studies still suggest that CHO may be the more critical substrate needed for high-intensity exercise performance (Burke, 2015; Burke et al., 2017). For example, Urbain et al. (2017) showed a reduction in absolute O2peak and peak power (by 2.4 and 4.1%, respectively) during a maximal incremental cycling test following a 6 week VLCHF diet intervention. However, this study lacked a control group for comparison. Thus, consensus has not been reached regarding the longer-term impact of the VLCHF diet on high-intensity exercise performance.
Many especially believe that a VLCHF diet has a detrimental effect on performance during exercise in the very high intensity domain (i.e., above critical power). For example, Lima-Silva et al. (2013) showed in six healthy, physically active men that a short-term VLCHF diet (48 h) significantly (p < 0.05) reduced total time to exhaustion (TTE; 3.0 ± 0.2 min vs. 3.7 ± 0.3 following low-CHO vs. habitual diets, respectively) during all-out supramaximal cycling exercise (115% maximal oxygen consumption). In moderately trained men, unlike a habitual moderate- to high-CHO diet, a 6 week moderate protein, high fat diet (30% protein, 61% fat) was associated with a significant decrease in mean and peak power during the first bout of a Wingate test (538–426 W and 879–786 W, respectively) (Fleming and Sharman, 2003). In contrast, 400-m sprint performance, O2peak and one-repetition maximum back squat were not adversely influenced after adoption of a 12 week ketogenic diet in recreationally trained CrossFit trainees when compared to a control group (Kephart et al., 2018). As well, Cipryan et al. (2018) showed after a 4 week VLCHF diet that individuals were able to perform high-intensity interval training (HIIT) with similar cardiorespiratory and RPE responses as a control diet group and without any indication of a performance compromise.
High-intensity interval training is an effective training method for enhancing cardiorespiratory, metabolic, and neuromuscular performance (Buchheit and Laursen, 2013; Tschakert and Hofmann, 2013) and can be efficiently used for managing and controlling a number of common metabolic diseases (Cassidy et al., 2017; Gillen and Gibala, 2018). HIIT includes several bouts of high-intensity exercise above critical speed/power, interspersed with recovery periods, and allows athletes to maintain long periods of time above 90% of O2max (Buchheit and Laursen, 2013). Substantial dependence on anaerobic glycolytic energy contribution is required by default for HIIT. HIIT performance potential can be assessed using the 30-15 Intermittent Fitness Test (30-15IFT), which involves a graded, intermittent, shuttle field test (involving changes of direction), resulting in near maximal running velocity, with substantial energy provided by anaerobic sources during the last stages of the test (Buchheit, 2008; Buchheit and Laursen, 2014). However, the effect of longer-term carbohydrate restriction on HIIT performance and resulting physiological responses are not well documented.
The influence of dietary changes or physical interventions on physiological adaptation can be indirectly assessed through monitoring of the cardiac autonomic nervous system (ANS) activity via resting heart rate variability (HRV) measurement (Young and Benton, 2018). This non-invasive method enables assessment of the cardiac ANS status (Plews et al., 2016). To the best our knowledge, however, the effect of VLCHF diet adaptation on cardiac ANS activity has not been studied.
As mentioned, CHO availability is believed to be necessary to enable exercise performance in the high-intensity domain (e.g., >critical power) (van Loon et al., 2001). At first glance, we might assume that lowered CHO intake leads to lower CHO availability and consequent impairment of anaerobic metabolism and associated performance (Langfort et al., 1997; Yeo et al., 2011; Lima-Silva et al., 2013). In light of limited research examining prolonged adaptation periods to VLCHF diets (Cipryan et al., 2018), the purpose of the present study then was to examine the effects of a 12 week VLCHF diet intervention on HIIT performance and cardiac ANS activity, as monitored via morning resting HRV and the 30-15IFT.
Materials and Methods
Participants
In total, 30 healthy and moderately trained individuals were recruited through leaflet distribution in the local city area and via social media (Figure 1). Participants needed to be aged between 18 and 35 years, non-smokers, performing regular exercise at the recreational level (defined as having no specific sport training specialty and engaging in less than 60 min of moderate intensity exercise three times per week), and without experience to the low-carbohydrate high-fat diet. Exclusion criteria included having any overt cardiovascular, metabolic, respiratory or other chronic disease, acute or chronic musculoskeletal impairment prohibiting exercise training or testing, any medication or diet supplement, or any specific dietary restrictions (e.g., vegan) for at least 6 months prior. Participants were divided into either a very low-carbohydrate high-fat diet group (VLCHF; N = 15) or habitual diet group (HD; N = 15) according to subject preference. Alcoholic beverages and dietary supplements were restricted for the intervention period.
Experimental Design
A non-randomized, two-group, parallel design was used. Twenty-four participants allocated to either a VLCHF or a HD group completed 12 weeks of diet and exercise (VLCHF) or exercise only intervention (HD). The detailed participants’ characteristics are presented in Table 1. Participants in the HD group were instructed to maintain their habitual diet (which was controlled by food diaries). Four participants (two from each group) were not able to meet the study requirements and did not complete the study (Figure 1). Diet, exercise and HRV were monitored 1 month before the intervention to obtain baseline levels. The laboratory graded exercise test (GXT) was performed at baseline, after 4, 8, and 12 weeks. A supervised HIIT session and 30-15 Intermittent Fitness Test (30-15IFT), separated by at least 48 h, were conducted each week (Figure 2).
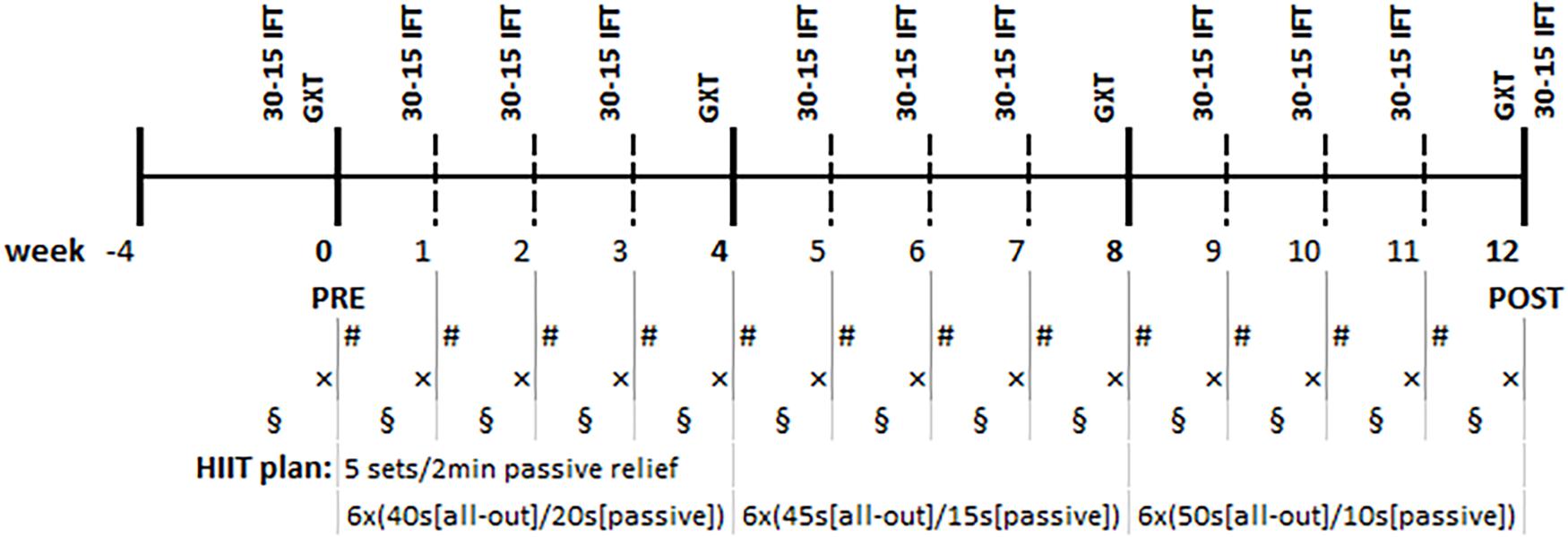
Figure 2. Research timeline. GXT, Graded Exercise Test; 30-15 IFT, 30-15 Intermittent Fitness Test (every Thursday/Friday); #, supervised High-Intensity Interval Training (HIIT) session (every Monday/Tuesday), ×, capillary blood draw for the glucose and β-hydroxybutyrate analysis (every Thursday/Friday), §, heart rate variability measurement (at least three times per week).
Diet Intervention
Participants in the VLCHF group were instructed to restrict carbohydrate consumption to a maximum 50 g/day (Feinman et al., 2015). Protein and fat were permitted to be consumed ad libitum. However, participants were encouraged to focus on increasing fat consumption in order to compensate for the reduced energy caused by carbohydrate restriction. Participants received detailed guidance concerning the VLCHF diet and its practical application from an experienced dietitian prior to the trial. Participants were provided with a sample diet and unlimited access to diet consultancy at any time during the experimental period. Participants from both groups were instructed to track their self-reported diet daily using a dietary analysis application1. A capillary blood sample was drawn from a finger for the measurement of β-hydroxybutyrate (FreeStyleOptium Neo, Oxon, United Kingdom) once a week to confirm compliance with the dietary regimen.
Exercise Intervention
Participants were instructed to perform 3–5 training sessions per week. Two of these included a supervised HIIT session and the 30-15IFT. The remaining required training sessions (1–3 per week) were home-based and of an endurance format (e.g., running, cycling, sport games). All training sessions were recorded by heart rate monitor (Polar M400, Kempele, Finland).
The supervised HIIT sessions lasted approximately 40 min in total and consisted of a 4 min warm-up followed by 5 × 6 min sets separated by 2 min recovery. Each training set was composed of three short interval exercises and three strength/core exercises. Each 1 min bout was formed by progressively increasing the work/rest ratio as follows: weeks 1–4, work/rest ratio = 40 s/20 s; weeks 5–8, 45 s/15 s; weeks 9–12, 50 s/10 s (Figure 2).
Graded Exercise Test
Prior to the start of the intervention, and then again in 1 month intervals (four times in total), participants underwent a laboratory GXT on a motorized treadmill (Rodby RL 2000E) in order to determine maximum aerobic power (O2max), the second ventilatory threshold (VT2), total time to exhaustion (TTEGXT), and respiratory exchange ratio (RER). The GXT protocol started at 7.0 km/h and running speed was increased by 1.5 km/h every 4 min (inclination remaining at 1%) conducted to volitional exhaustion. Expired air was continuously monitored to analyze O2 and CO2 concentrations during the GXT by the use of a breath-by-breath system (Blue Cherry, Geratherm Medical AG, Germany). O2max was determined as the highest average O2 consumption measured over a 30 s period. Gas-exchange measurements were also used to quantify the second ventilatory threshold (VT2). VT2 was defined as a sharp increase in ventilation (VE) accompanied with an increase in both VE/VO2 and VE/VCO2. HR was measured using a chest belt monitor (Polar Electro H9, Kempele, Finland). Peak lactate was analyzed from capillary blood obtained from a finger immediately after GXT cessation. Participants also expressed their perceived effort on the 20-point Borg scale. All sessions were conducted in the morning, at least 3 h after their last meal and in a thermally controlled laboratory (21°C, 40% relative humidity). Each participant performed their laboratory sessions during similar morning hours (±30 min), and were advised not to participate in vigorous activity 24 h prior. Body mass and composition were determined using a bioelectrical impedance analyser (InBody770, Seoul, South Korea) before the GXT.
30-15 Intermittent Fitness Test
The 30-15 Intermittent Fitness Test (30-15IFT) was performed once a week (at least 6 days apart) in indoor conditions (Buchheit, 2008). The test consists of 30 s shuttle runs interspersed by 15 s passive recovery periods. Run speed began at 8 km/h for the first 30 s run and was increased by 0.5 km/h every 45 s stage thereafter. Heart rate was monitored throughout the test. Participants evaluated their perceived exertion using the same 20-point Borg scale immediately after exercise cessation.
Heart Rate Variability
Participants performed a 60 s HRV measurement in a daily manner upon waking up whilst breathing spontaneously in a supine position (Plews et al., 2017). Participants were obliged to remain resting in a supine position at least 2 min before the HRV measurements. A Bluetooth chest belt (Polar H7 Bluetooth, Kempele, Finland) paired with a smartphone application Elite HRV (Ashville, NC, United States) were used for daily HRV measurement. The raw beat-to-beat (RR) data were acquired from Elite HRV and further processed for counting rMSSD variable as follows: RR intervals were corrected for artifacts according to two criteria. First, consecutive RR intervals were removed when they differed by more than 75% from the previous one. Additionally, outliers were removed by including only RR intervals that were within less than 25% of the first quartile and within more than 25% of the third quartile. This technique avoids over-correcting, a problem of the widely employed removal of consecutive RR intervals differing by more than 25% for individuals with very high beat-to-beat variability (Plews et al., 2017). The calculated rMSSD data (Camm et al., 1996) were log-transformed (ln) as a non-uniformity of error was expected (Plews et al., 2012). A 7 days ln rMSSD average was used for the cardiac autonomic regulation assessment, where at least three HRV measurements were recorded (Plews et al., 2016). The participants began to measure HRV 1 month before the intervention. However, the baseline rMSSD level was based on the last ten HRV measurements taken immediately prior to the intervention.
Statistical Analysis
Descriptive statistics are displayed as mean ± SD. All parameters were tested for normal distribution using the Shapiro–Wilk test. Data were assessed for practical significance using standardized differences in the mean (Cohen’s d) which were calculated using the pooled standard deviation. Threshold values for the Cohen’s d were <0.20 (trivial), 0.20–0.49 (small), 0.50–0.79 (medium), ≥0.80 (large) (Cohen, 1988). 95% Confidence Limits were expressed for Cohen’s d. The two-tailed paired Student’s t-test (or Mann–Whitney test when significant deviation from normality) was used for the within-group time comparisons. The two-tailed independent Student’s t-test (or Wilcoxon signed-rank test when significant deviation from normality) was used for the between-group comparisons. In all cases, p < 0.05 were taken as the level of statistical significance.
Bayes factor (BF10) were calculated for differences between groups as well as for the before vs. during intervention comparisons using Cauchy prior (location 0, scale 0.707). Visual prior vs. posterior check and Bayes factor robustness check were considered. Bayes factor quantify relative evidence for the null and alternative hypothesis. Evidence categories for the BF10 were <1/100 decisive evidence for H0, 1/100-1/30 very strong evidence for H0, 1/30-1/10 strong evidence for H0, 1/10-1/3 moderate evidence for H0, 1/3-1 anecdotal evidence for H0, 1 no evidence, 1–3 anecdotal evidence for H1, 3–10 moderate evidence for H1, 10–30 strong evidence for H1, 30–100 very strong evidence for H1, >100 decisive evidence for H1 (Jeffreys, 1961). Statistical analyses were performed using JASP (version 0.9.1.0).
Results
The between-group differences of the diet regime are shown in Table 2. The total energy and macronutrients intake were similar in both groups before the intervention. The macronutrient intake did not change in the HD group during the intervention period. Although the total energy significantly decreased (ES ± 95% CI: 0.69 ± 0.63, p = 0.035) in the HD group there were no between-group differences in total energy intake during the 12 week intervention period. According to the study aim, participants in the VLCHF group substantially reduced their CHO intake and increased their lipid intake (both decisive evidence for the large change). Changes in the body weight and body composition in both groups are shown in Table 1. Although energy intake slightly increased in the VLCHF group, body weight decreased by 6.4% and remained constant in the HD group. Body composition significantly changed only in the VLCHF diet group. Fat mass and trunk fat mass significantly decreased by 20.2 and 23.7%, respectively, after 12 weeks of diet in the VLCHF group only (Table 1).
The concentration of β-hydroxybutyrate (βHB) increased substantially in the VLCHF group within the first days of the diet intervention. The βHB concentration achieved its highest peak at the end of the 2 weeks (1.1 ± 0.7 mmol/l) but tended to normalize toward the end of the 12 week intervention period (Figure 3).
Total number of HIIT sessions (including 30-15IFT) through the experimental part of the research was 22 sessions. The smallest number of training sessions across all participants was 17 (N = 1). Average attendance was 20.4 ± 1.4 (92.8 ± 6.5%) training sessions for the VLCHF group and 20.1 ± 1.2 (91.3 ± 1.3%) training sessions for the HD group. The usual reasons for missed training sessions were lack of time or other personal reasons. The volume and exercise intensity were similar in the between-group comparison before as well as during the intervention period. However, there was very strong to decisive evidence for a large increase of the exercise intensity during the intervention period for both the VLCHF and the HD group (Table 2).
Graded Exercise Test
We found very strong evidence (BF10 = 11.30) for a large Total Time to Exhaustion (TTEGXT) increase in the VLCHF group (ES ± 95 CI: 1.03 ± 0.70, p = 0.005) and moderate evidence (BF10 = 3.87) for a large TTEGXT increase in the HD group (ES ± 95 CI: 0.86 ± 0.70, p = 0.018) after 12 weeks. O2max (ml/kg/min) also increased largely (ES ± 95 CI: 0.91 ± 0.67, p = 0.009, BF10 = 6.25) in the VLCHF group despite a moderate evidence for no significant (p = 0.591) change in the HD group. However, there was moderate evidence (BF10 = 0.29 and 0.32 for the VLCHF and HD group, respectively) for no changes in both groups when O2max was expressed in absolute values (l/min). RER at maximal running speed decreased largely (ES ± 95 CI: -1.11 ± 0.76, p = 0.004, BF10 = 12.29) in the VLCHF group whereas it did not change in the HD group (ES ± 95 CI: -0.06 ± 0.57, p = 0.835, BF10 = 0.29) at the end of the intervention. The complete GXT results are shown in Figure 4 and Table 3. Average group values of lactate in the rest state decreased by 40% from 1.2 ± 0.3 (mmol/l) at the start of the intervention to 0.8 ± 0.3 (mmol/l) in VLCHF and no difference in HD at the end of the 12 weeks intervention. Average group values of lactate after the GXT decreased by 4.5% from 9.3 ± 2.2 (mmol/l) at the start of the intervention to 8.9 ± 2.4 (mmol/l) in VLCHF and by 16% from 9.6 ± 3.0 (mmol/l) to 8.1 ± 3.1 (mmol/l) at the end of the 12 weeks intervention.
30-15 Intermittent Fitness Test (30-15IFT)
We found decisive evidence that TTEIFT largely increased in both VLCHF and HD groups after 12 weeks (ES ± 95% CI: 1.93 ± 0.97 and 1.50 ± 0.84, respectively, p = 0.001 and BF10 ≥ 100 for both groups). Simultaneously with the TTEIFT increase, HRmean largely decreased in both VLCHF and HD groups after 12 weeks (ES ± 95 CI: -1.86 ± 1.00 and -1.26 ± 0.77, p = 0.001 and 0.001, BF10 ≥ 100 and 35.42, respectively) (Figure 5). There was decisive evidence for an RPE increase in both VLCHF (Mean ± SD: PRE 16.83 ± 1.85, 12 w 19.42 ± 0.79, ES ± 95% CI: 1.00 ± 0.00, p = 0.002, BF10 ≥ 100) (note for VLCHF group: p-value is from Wilcoxon signed-rank; effect size is given by the matched rank biserial correlation due to deviation from normality) and HD (Mean ± SD: PRE 16.67 ± 1.30, 12 w 19.08 ± 0.67, ES ± 95% CI: 1.61 ± 0.89, p = 0.001, BF10 ≥ 100) groups.
Heart Rate Variability
The vagal related HRV parameter rMSSD did not change during the monitored period. There is moderate evidence for a trivial effect in both study groups (ES ± 95 CI: -0.06 ± 0.60 and -0.16 ± 0.59, p = 0.856 and 0.611, BF10 = 0.30 and 0.33, respectively) (Figure 6).
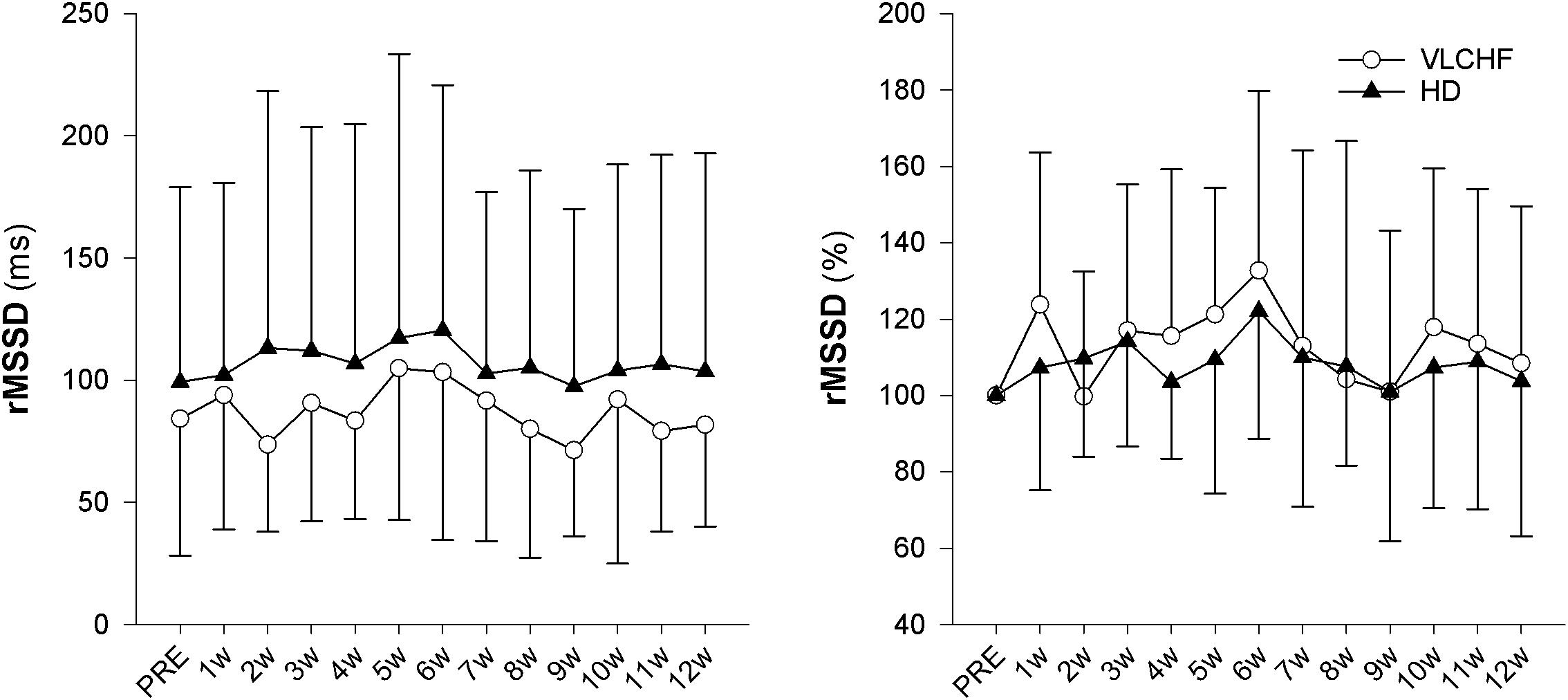
Figure 6. The time course of rMSSD during the intervention period, expressed in absolute units (ms) or as change (%) from the PRE score.
Discussion
The purpose of this controlled non-randomized study was to evaluate the effects of a 12 week non-energy-restricted VLCHF diet in healthy young individuals on maximal cardiorespiratory and HIIT performance as well as cardiac autonomic regulation. The adequate compliance to our diet intervention is highlighted by increased resting capillary blood βHB concentrations (Figure 3) and reduced RER during the GXT (Table 3). We found that the 12 week CHO intake restriction and fat-rich diet did not adversely impact on high-intensity continuous or intermittent performance. Interestingly, ßHB tended to normalize toward the end of the 12 week intervention period, potentially reflecting enhanced cellular uptake subsequent to adaptation. Additionally, as far as we know, this is the first study to report on resting cardiac autonomic response to the VLCHF diet intervention across 12 weeks. We showed that this predictor of training status (Buchheit, 2014) remained unaffected by the VLCHF diet intervention. Another interesting effect was the decrease in body mass, and specifically trunk fat mass, in the VLCHF diet group, despite unchanged energy intake (slight increase).
The VLCHF-Induced Metabolic Shift
The 12 weeks exposure to the VLCHF diet in the present investigation is considered sufficient for inducing nutritional ketosis and also achieving an adequate adaptation to the VLCHF diet, which typically takes about 3–4 weeks (Volek et al., 2015). The rapid increase in capillary blood βHB concentration during the first 2 weeks (from a baseline value of ≤0.1–1.03 ± 0.74 mmol/l) and the maintenance of the elevated βHB level in the range of 0.5–3.0 mmol/L (Volek and Phinney, 2012) throughout the entire VLCHF diet intervention, provides evidence of established nutritional ketosis (Figure 3). These βHB concentration changes and subsequent dynamics correspond with previously reported studies (Mckenzie et al., 2017; McSwiney et al., 2017). The gradual decrease in βHB concentration shown after the 2 weeks of the VLCHF diet intervention, which tended to normalize, may be the result of enhanced mitochondrial function and capacity for ketone body utilization (Miller et al., 2018). Another possible explanation for the tendency for normalizing βHB concentration toward baseline conditions is an increase in gluconeogenetic glucose production (Veldhorst et al., 2009) supported by an increased protein intake during the intervention period (Table 2). Additionally, some suggest that this increase in gluconeogenesis leads to higher energy expenditure due to a diet-induced thermogenic increase of 20–30% (Keller, 2011). In contrast, this effect was absent from the study of Webster et al. (2016) in trained cyclists performing a 6 month high-fat diet. Nevertheless, such a thermogenesis effect is suggested to explain the weight loss shown in the VLCHF diet group even despite their higher energy intake compared to the pre-intervention period. The strong evidence for the large decrease of maximal RER during GXT at maximal running speed in the VLCHF group (Table 3) also indicates increasing whole-body rates of fat oxidation and accompanying reductions in CHO utilization. Previous studies examining VLCHF diets have reported similar findings for the RER response (Burke et al., 2017; Urbain et al., 2017). The increased utilization of fat and concomitant decrease in CHO oxidation during exercise can be associated with a number of different mechanisms, including the ability of skeletal muscle to transport, store, and oxidize free fatty acids, or alternatively, suppression of pyruvate dehydrogenase activity and glycogenolysis (Yeo et al., 2011).
Performance Outcomes
Total time to exhaustion in both GXT and 30-15IFT largely increased in both VLCHF and HD group after the 12 weeks period. Additionally, our data showed no between-group differences in the TTE increase percentage changes between PRE vs. 12 week time points across the VLCHF and HD group for GXT (p = 0.530, BF10 = 0.44; Table 3) and 30-15IFT (p = 0.428, BF10 = 0.47). These results are in line with Heatherly et al. (2018) who showed no significant difference in a 5 km time trial performance between their low- and high-carbohydrate diet groups. Further, average values of peak GXT lactate in VLCHF at the end of the 12 week intervention were similar to average peak values at the beginning; a finding which is in line with the study by Burke et al. (2017). A possible explanation may be that individuals can adapt to this type of diet by synthesizing glucose from others substrates (Volek et al., 2016). Similar to the study of McSwiney et al. (2017), we found no negative effects of the VLCHF diet on exercise during the 12 week intervention period, which is in contrast to the results of Burke et al. (2017), who indicate a negative impact on 10 km race time in elite walkers after 3 weeks of a VLCHF compared to a HD. Our results are also in conflict with general sports nutrition recommendations that emphasize the necessity for dietary CHO intake and sufficient availability to enable high-intensity exercise performance (Burke and Hawley, 2018). The glycogen content of the working muscle has been considered for several decades as a determinant for the capacity to perform high-intensity exercise (Bergström et al., 1967). When fat oxidation is intentionally increased through a shift of the macronutrient intake, CHO substrate pools are reduced. It has been shown that exercise economy (increased oxygen demand for a given speed) might also be diminished when conforming to a VLCHF diet (Burke et al., 2017). Of note, the findings of impaired exercise performance have usually been presented after only short periods on the VLCHF diet, which unlikely allows participants fully to adapt sufficiently to the dietary CHO reduction and higher fat intake (>4 weeks). Nevertheless, the long-term adaptation to a fat-rich diet is a crucial prerequisite for maintaining the capacity to perform endurance or high-intensity intermittent exercise (Helge, 2002; Volek et al., 2016). This is also apparent in our data from the 30-15IFT where a considerable drop in TTE was found after 2 weeks on the VLCHF diet intervention (Figure 5); a response that was absent toward the end of the intervention period, suggesting normalization of glycogen stores due to enhanced gluconeogenesis (Veldhorst et al., 2009; Keller, 2011). The ability to maintain or even improve performance in the GXT or 30-15IFT in the VLCHF group (Figures 7, 8) might be explained, first, by the fact that the endogenous CHO stores are not necessarily completely depleted when CHO intake is reduced (Volek et al., 2016), evidenced by the normal lactate response. Second, blood glucose concentration can also be maintained through glucose synthesis via a variety of substrates (Soeters et al., 2012). However, we prescribed a high-intensity but relatively short (up to 25 min) exercise bout that may not have been long enough to exhaust the glycogen stores, regardless of how much glycogen stores were diminished through CHO intake reduction. Therefore, it remains an open question whether this effect of VLCHF diet holds true for longer exercise (>25 min) bouts with high-intensity periods.
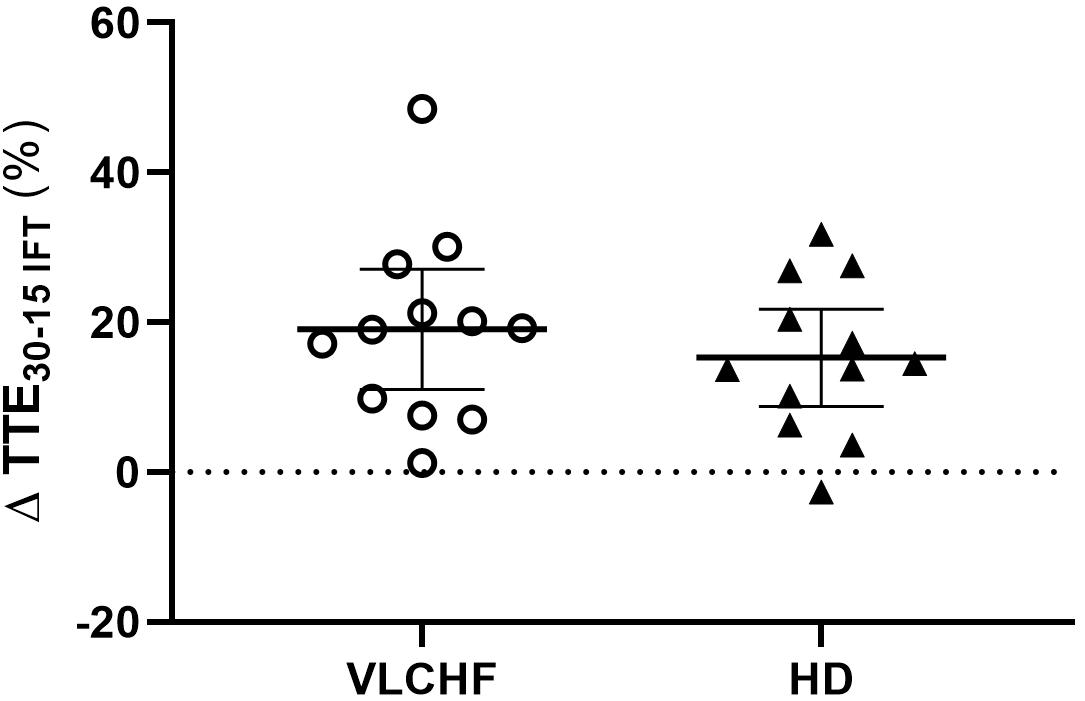
Figure 8. Individual responses of total time to exhaustion in 30-15IFT (TTE30-15IFT). The means and 95% CI are shown. The outlier at this was not included in the group statistics (Table 3).
We also analyzed the possible association between the level of capillary βHB concentrations and changes of TTE in both GXT and 30-15IFT. Due to substantial inter-individual diversity in capillary βHB concentrations, it might be expected that we can define responders vs. non-responders to the VLCHF diet within our investigated population. We found moderate evidence for a positive association between the mean level of βHB from the entire VLCHF diet intervention period and the PRE vs. 12 week change (%) in TTEGXT [r = 0.69 (95% CL: 0.19, 0.91), p = 0.013, BF10 = 5.63]. However, there was only anecdotal evidence for this association with the performance change in 30-15IFT (TTE30-15IFT; p = 0.185, BF10 = 0.78) (Figure 9). Ketone bodies (including βHB) are alternative energy substrates for brain and working muscles when the endogenous and exogenous CHO stores are reduced (Cox and Clarke, 2014). This might explain why participants with higher capillary βHB concentrations tended to be able to achieve the most substantial improvements in GXT performance. However, this explanation has limitations, since the capillary βHB concentration is a function of both βHB synthesis and utilization. Therefore, the association between βHB and its positive effect cannot be explained unequivocally by our data and warrants further research.
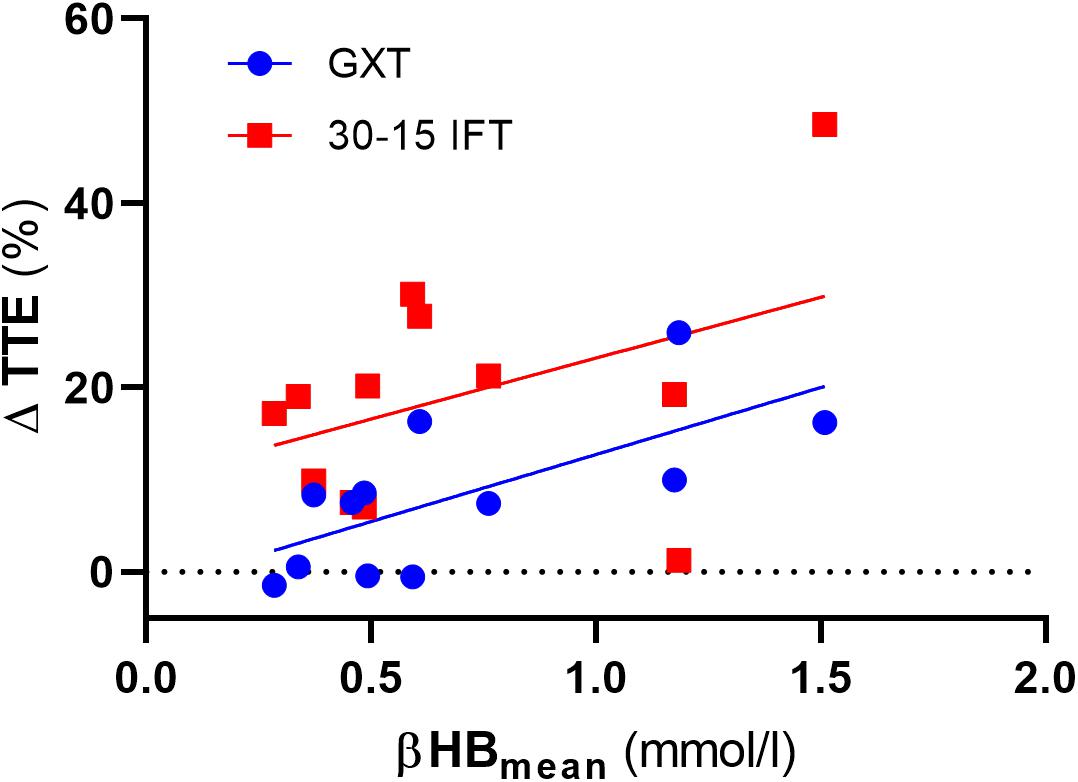
Figure 9. The association between total time to exhaustion (TTE) changes (%) and mean capillary β-hydroxybutyrate (βHB) concentration across the entire VLCHF diet intervention period. GXT, Graded Exercise Test; 30-15 IFT, 30-15 Intermittent Fitness Test.
The obvious between-group difference was found in changes of relative O2max (ml/kg/min). The O2max largely increased in the VLCHF group despite a trivial change in the HD group. However, there was moderate evidence that O2max was not changed in both groups when we expressed the marker in absolute values (l/min). Therefore, we ascribe this between-group difference in relative O2max solely to the body mass reduction (Table 1) and not to potential maximal aerobic capacity enhancement in the VLCHF group. These findings correspond with our previous study, which showed similar O2max changes in moderately trained individuals after a 4 week VLCHF diet intervention (Cipryan et al., 2018), and is in line with others (Zajac et al., 2014; Paoli et al., 2015), but contrary results have also been presented (Burke et al., 2017).
RPE and HRV
Higher mental effort to sustain the scheduled exercise sessions, while consuming a short-term fat-rich diet compared with consuming a high CHO diet, was presented earlier by Helge (2002) for submaximal exercise until exhaustion. The significant between-group differences in the RPE data shown in this study did not occur until 45 min of the exercise (Helge, 2002). We showed that the RPE in both GXT and 30-15IFT increased in both study groups but with no clear between-group differences (Table 3 for GXT, data for 30-15IFT in Figure 5). Helge (2002) explained findings through the significantly higher catecholamine and heart rate response during submaximal exercise in subjects consuming a fat-rich diet. Those changes might be related to changes in ANS activity at rest and in response to exercise after a short-term reduction in CHO intake (increased sympathetic and possibly decreased parasympathetic response) (Pellizzer et al., 1999; Helge, 2002). The autonomic imbalance is a predictor of high blood pressure, hyperglycaemia, and a diagnosis of diabetes, cardiovascular disease and early mortality (Wulsin et al., 2016). As far as we know, we present novel data of the 12 week resting cardiac ANS activity in relation to the VLCHF diet. However, neither HR response to exercise (Figure 5) nor resting rMSSD (Figure 6) in our study showed any between-group differences. The vagal-related HRV parameter rMSSD remained stable throughout the experimental period, but a slight drop in the 2 week (also accompanied by performance reduction, see Figure 5) may support the necessity of an adequate adaptation to the VLCHF diet (Figure 6).
Conclusion
A 12 week dietary CHO reduction with concomitant increases in fat intake in healthy young individuals did not impair high-intensity continuous or intermittent exercise lasting up to 25 min. The HRV assessment indicated, for the first time, that a 12 week exposure to the VLCHF diet combined with HIIT did not adversely affect ANS activity. Further, there was no evidence that individuals in the VLCHF group needed to raise their mental effort to achieve maximal performance. Finally, the similar PRE-POST average values of peak lactate indicate that the anaerobic energy contribution to performance was preserved in our adapted VLCHF group, and lowered RER values indicate enhancements in lipid metabolism throughout the exercise-intensity spectrum.
Data Availability
The datasets generated for this study are available on request to the corresponding author.
Ethics Statement
All participants provided written informed consent for the study protocol, which was approved by the local ethics committee and conformed to the principles outlined in the Declaration of Helsinki.
Author Contributions
TD and LC designed the study, collected, analyzed, and interpreted the data, drafted, revised, and submitted the manuscript. DP, PH, and PL designed the study, interpreted the data, drafted, and revised the manuscript.
Funding
This work was supported by the Czech Science Foundation (Grant 18-08358S) and the University of Ostrava (Grant SGS PdF2017).
Conflict of Interest Statement
The authors declare that the research was conducted in the absence of any commercial or financial relationships that could be construed as a potential conflict of interest.
Footnotes
References
Bergström, J., Hermansen, L., Hultman, E., and Saltin, B. (1967). Diet, muscle glycogen and physical performance. Acta physiol. Scand. 71, 140–150. doi: 10.1111/j.1748-1716.1967.tb03720.x
Buchheit, M. (2008). The 30-15 intermittent fitness test:accuracy for individualizing interval training of young intermittent sport players. J. Strength Cond. Res. 22, 365–374. doi: 10.1519/JSC.0b013e3181635b2e
Buchheit, M. (2014). Monitoring training status with HR measures: do all roads lead to Rome? Front. Physiol. 5:73. doi: 10.3389/fphys.2014.00073
Buchheit, M., and Laursen, P. B. (2013). High-intensity interval training, solutions to the programming puzzle: part I: cardiopulmonary emphasis. Sports Med. 43, 313–338. doi: 10.1007/s40279-013-0029-x
Buchheit, M., and Laursen, P. B. (2014). Dr. Boullosa’s forgotten pieces don’t fit the puzzle. Sports Med. 44, 1171–1175. doi: 10.1007/s40279-014-0191-9
Burke, L. M. (2015). Re-examining high-fat diets for sports performance: did we call the ‘nail in the coffin’too soon? Sports Med. 45, 33–49. doi: 10.1007/s40279-015-0393-9
Burke, L. M., and Hawley, J. A. (2018). Swifter, higher, stronger: what ’ s on the menu? Science 362, 781–787. doi: 10.1126/science.aau2093
Burke, L. M., Ross, M. L., Garvican-lewis, L. A., Welvaert, M., Heikura, I. A., Forbes, S. G., et al. (2017). Low carbohydrate, high fat diet impairs exercise economy and negates the performance benefit from intensified training in elite race walkers. J. Physiol. 595, 2785–2807. doi: 10.1113/JP273230
Camm, M., Malik, J. T., Bigger, G., Breithardt, S., Cerutti, R. J., Cohen, P., et al. (1996). Heart rate variability: standards of measurement, physiological interpretation and clinical use. task force of the european society of cardiology and the north american society of pacing and electrophysiology. Eur. Heart J. 17, 354–381.
Cassidy, S., Thoma, C., Houghton, D., and Trenell, M. I. (2017). High-intensity interval training?: a review of its impact on glucose control and cardiometabolic health. Diabetologia 60, 7–23. doi: 10.1007/s00125-016-4106-1
Cipryan, L., Plews, D. J., Ferretti, A., Maffetone, P. B., and Laursen, P. B. (2018). Effects of a 4-week very low-carbohydrate diet on high-intensity interval training responses. J. Sports Sci. Med. 17, 259–267.
Cohen, J. (1988). Statistical Power Analysis for the Behavioural Sciences (2nd Erlbau). Hillsdale, NJ: Lawrence Erlbaum Associates, Publishers.
Cox, P. J., and Clarke, K. (2014). Acute nutritional ketosis: implications for exercise performance and metabolism. Extrem. Physiol. Med. 3, 1–9. doi: 10.1186/2046-7648-3-17
Feinman, R. D., Pogozelski, W. K., Astrup, A., Bernstein, R. K., Fine, E. J., Westman, E. C., et al. (2015). Dietary carbohydrate restriction as the first approach in diabetes management: critical review and evidence base. Nutrition 31, 1–13. doi: 10.1016/j.nut.2014.06.011
Fleming, J., and Sharman, M. (2003). Endurance capacity and high-intensity exercise performance responses to a high fat diet. Int. J. Sport Nutr. Exerc. Metab. 13, 466–478. doi: 10.1123/ijsnem.13.4.466
Gillen, J. B., and Gibala, M. J. (2018). Interval training: a time-efficient exercise strategy to improve cardiometabolic health. Appl. Physiol. Nutr. Metab. 43, 3–4. doi: 10.1139/apnm-2013-0187
Heatherly, A. J., Killen, L. G., Smith, A. F., Waldman, H. S., Seltmann, C. L., Hollingsworth, A., et al. (2018). Effects of Ad libitum low-carbohydrate high-fat dieting in middle-age male runners. Med. Sci. Sports Exerc. 50, 570–579. doi: 10.1249/MSS.0000000000001477
Helge, J. W. (2002). Long-term fat diet adaptation effects on performance, training capacity, and fat utilization. Med. Sci. Sports Exerc. 34, 1499–1504. doi: 10.1249/01.MSS.0000027691.95769.B5
Keller, U. (2011). Dietary proteins in obesity and in diabetes. Int. J. Vitam. Nutr. Res. 81, 125–133. doi: 10.1024/0300-9831/a000059
Kephart, W., Pledge, C., Roberson, P., Mumford, P., Romero, M., Mobley, C., et al. (2018). The three-month effects of a ketogenic diet on body composition, blood parameters, and performance metrics in crossfit trainees: a pilot study. Sports 6:1. doi: 10.3390/sports6010001
Langfort, J., Zarzeczny, R., Pilis, W., Nazar, K., and Kaciuba-Uścitko, H. (1997). The effect of a low-carbohydrate diet on performance, hormonal and metabolic responses to a 30-s bout of supramaximal exercise. Eur. J. Appl. Physiol. Occup. Physiol. 76, 128–133. doi: 10.1007/s004210050224
Lima-Silva, A. E., Pires, F. O., Bertuzzi, R., Silva-Cavalcante, M. D., Oliveira, R. S. F., Kiss, M. A., et al. (2013). Effects of a low- or a high-carbohydrate diet on performance, energy system contribution, and metabolic responses during supramaximal exercise. Appl. Physiol. Nutr. Metab. 38, 928–934. doi: 10.1139/apnm-2012-0467
Mckenzie, A. L., Hallberg, S. J., Creighton, B. C., Volk, B. M., Link, M., Abner, M. K., et al. (2017). A novel intervention including individualized nutritional recommendations reduces hemoglobin A1c level, medication use, and weight in type 2 diabetes. JMIR Diabetes 2:e5. doi: 10.2196/diabetes.6981
McSwiney, F. T., Wardrop, B., Hyde, P. N., Lafountain, R. A., Volek, J. S., and Doyle, L. (2017). Keto-adaptation enhances exercise performanceand body composition responses to training inendurance athletes. Metabolism 81, 25–34. doi: 10.1016/j.metabol.2017.10.010
Miller, V. J., Villamena, F. A., and Volek, J. S. (2018). Nutritional ketosis and mitohormesis: potential implications for mitochondrial function and human health. J. Nutr. Metab. 2018:5157645. doi: 10.1155/2018/5157645
Paoli, A., Bianco, A., and Grimaldi, K. A. (2015). The ketogenic diet and sport: a possible marriage? Exerc. Sport Sci. Rev. 43, 153–162. doi: 10.1249/JES.0000000000000050
Pellizzer, A., Straznicky, N. E., Lim, S., Kamen, P. W., and Krum, H. (1999). Reduced dietary fat intake increases parasympathetic activity in healthy premenopausal women. Clin. Exp. Pharmacol. Physiol. 26, 656–660. doi: 10.1046/j.1440-1681.1999.03103.x
Plews, D. J., Laursen, P. B., and Buchheit, M. (2016). Day-to-day heart rate variability (HRV) recordings in world champion rowers: appreciating unique athlete characteristics. Int. J. Sports Physiol. Perform. 12, 697–703. doi: 10.1123/ijspp.2016-0343
Plews, D. J., Laursen, P. B., Kilding, A. E., and Buchheit, M. (2012). Heart rate variability in elite triathletes, is variation in variability the key to effective training? a case comparison. Eur. J. Appl. Physiol. 112, 3729–3741. doi: 10.1007/s00421-012-2354-4
Plews, D. J., Scott, B., Altini, M., Wood, M., Kilding, A. E., and Laursen, P. B. (2017). Comparison of heart-rate-variability recording with smartphone photoplethysmography, polar H7 chest strap, and electrocardiography. Int. J. Sports Physiol. Perform. 12, 1324–1328. doi: 10.1123/ijspp.2016-0668
Soeters, M. R., Soeters, P. B., Schooneman, M. G., Houten, S. M., and Romijn, J. A. (2012). Adaptive reciprocity of lipid and glucose metabolism in human short-term starvation. Am. J. Physiol. Endocrinol. Metab. 303, 1397–1407. doi: 10.1152/ajpendo.00397.2012
Tschakert, G., and Hofmann, P. (2013). High-intensity intermittent exercise: methodological and physiological aspects. Int. J. Sports Physiol. Perform. 8, 600–610. doi: 10.1123/ijspp.8.6.600
Urbain, P., Strom, L., Morawski, L., Wehrle, A., Deibert, P., and Bertz, H. (2017). Impact of a 6-week non-energy-restricted ketogenic diet on physical fitness, body composition and biochemical parameters in healthy adults. Nutr. Metab. 14:17. doi: 10.1186/s12986-017-0175-5
van Loon, L. J., Greenhaff, P. L., Constantin-Teodosiu, D., Saris, W. H., and Wagenmakers, A. J. (2001). The effects of increasing exercise intensity on muscle fuel utilisation in humans. J. Physiol. 536, 295–304. doi: 10.1111/j.1469-7793.2001.00295.x
Veldhorst, M. A., Westerterp-Plantenga, M. S., and Westerterp, K. R. (2009). Gluconeogenesis and energy expenditure after a high-protein, carbohydrate-free diet. Am. J. Clin. Nutr. 90, 519–526. doi: 10.3945/ajcn.2009.27834.1
Volek, J. S., and Phinney, S. D. (2012). The Art and Science of Low Carbohydrate Performance (39798th ed). Miami: Beyond Obesity LLC.
Volek, J. S., Freidenreich, D. J., Saenz, C., Kunces, L. J., Creighton, B. C., Bartley, J. M., et al. (2016). Metabolic characteristics of keto-adapted ultra-endurance runners. Metabolism 65, 100–110. doi: 10.1016/j.metabol.2015.10.028
Volek, J. S., Noakes, T., and Phinney, S. D. (2015). Rethinking fat as a fuel for endurance exercise. Eur. J. Sport Sci. 15, 13–20. doi: 10.1080/17461391.2014.959564
Webster, C. C., Noakes, T. D., Chacko, S. K., Swart, J., Kohn, T. A., and Smith, J. A. (2016). Gluconeogenesis during endurance exercise in cyclists habituated to a long-term low carbohydrate high-fat diet. J. Physiol. 594, 4389–4405. doi: 10.1113/JP271934
Wulsin, L. R., Horn, P. S., Perry, J. L., Massaro, J. M., and Agostino, R. B. D. (2016). Autonomic imbalance as a predictor of metabolic risks, cardiovascular disease, diabetes, and mortality. J. Clin. Endocrinol. Metab. 100, 2443–2448. doi: 10.1210/jc.2015-1748
Yeo, W. K., Carey, A. L., Burke, L., Spriet, L. L., and Hawley, J. A. (2011). Fat adaptation in well-trained athletes: effects on cell metabolism. Appl. Physiol. Nutr. Metab. 36, 12–22. doi: 10.1139/H10-089
Young, H. A., and Benton, D. (2018). Heart-rate variability: a biomarker to study the influence of nutrition on physiological and psychological health? Behav. Pharmacol. 29, 140–151. doi: 10.1097/FBP.0000000000000383
Keywords: nutritional ketosis, graded exercise test, 30-15 intermittent fitness test, β-hydroxybutyrate, heart rate variability
Citation: Dostal T, Plews DJ, Hofmann P, Laursen PB and Cipryan L (2019) Effects of a 12-Week Very-Low Carbohydrate High-Fat Diet on Maximal Aerobic Capacity, High-Intensity Intermittent Exercise, and Cardiac Autonomic Regulation: Non-randomized Parallel-Group Study. Front. Physiol. 10:912. doi: 10.3389/fphys.2019.00912
Received: 08 April 2019; Accepted: 03 July 2019;
Published: 17 July 2019.
Edited by:
Jun Sugawara, National Institute of Advanced Industrial Science and Technology (AIST), JapanReviewed by:
Jonathan Peter Little, University of British Columbia, CanadaCarl J. Lavie, Ochsner Medical Center, United States
Copyright © 2019 Dostal, Plews, Hofmann, Laursen and Cipryan. This is an open-access article distributed under the terms of the Creative Commons Attribution License (CC BY). The use, distribution or reproduction in other forums is permitted, provided the original author(s) and the copyright owner(s) are credited and that the original publication in this journal is cited, in accordance with accepted academic practice. No use, distribution or reproduction is permitted which does not comply with these terms.
*Correspondence: Lukas Cipryan, lukas.cipryan@osu.cz