- 1Medical Research Council Institute for Radiation Oncology, Department of Oncology, University of Oxford, Oxford, United Kingdom
- 2Genome Damage and Stability Centre, School of Life Sciences, University of Sussex, Brighton, United Kingdom
Treatment with ionizing radiation (IR) remains the cornerstone of therapy for multiple cancer types, including disseminated and aggressive diseases in the palliative setting. Radiotherapy efficacy could be improved in combination with drugs that regulate the ubiquitin-proteasome system (UPS), many of which are currently being tested in clinical trials. The UPS operates through the covalent attachment of ATP-activated ubiquitin molecules onto substrates following the transfer of ubiquitin from an E1, to an E2, and then to the substrate via an E3 enzyme. The specificity of ubiquitin ligation is dictated by E3 ligases, which select substrates to be ubiquitylated. Among the E3s, cullin ring ubiquitin ligases (CRLs) represent prototypical multi-subunit E3s, which use the cullin subunit as a central assembling scaffold. CRLs have crucial roles in controlling the cell cycle, hypoxia signaling, reactive oxygen species clearance and DNA repair; pivotal factors regulating the cancer and normal tissue response to IR. Here, we summarize the findings on the involvement of CRLs in the response of cancer cells to IR, and we discuss the therapeutic approaches to target the CRLs which could be exploited in the clinic.
Introduction: The Cullin Ring Ubiquitin Ligases
Ubiquitylation is a versatile post-translational modification to control protein levels in cells and modulate cellular states to allow adaptation under stress. Indeed, this secondary modification is crucial for the cellular response to DNA damaging agents including (Ionizing Radiation) IR (Schwertman et al., 2016). The classical process of ubiquitylation is based on the activation of a ubiquitin molecule by ATP, followed by its covalent attachment to an E1 enzyme, then its transfer from the E1 enzyme to an E2 enzyme, and finally its transfer from the E2 enzyme onto selected substrates (Hershko et al., 1983; Finley et al., 2004). Selection of substrates is operated by the E3 enzymes, which bridge substrates to the E2 enzyme for ubiquitin transfer. The human genome codes for 2 E1s, ∼35 E2s and more than 700 E3 ubiquitin ligases. The organization of the system is hierarchical which allows for substrate specificity and, at the same time, diversity in substrate modification.
Cullin ring ubiquitin ligases are multi-subunit E3 ubiquitin ligases which use a specific cullin as a central scaffold to bridge an E2 enzyme to the substrate. All the cullin complexes use a cullin C-terminal portion to recruit a RING-Box protein (Rbx1 or Rbx2), required for the interaction with an E2. The N-terminal portion of the cullin binds to a different adaptor protein containing a domain that interacts with a range of variable substrate recruitment subunits, specific to that culllin class. In general, an adaptor protein, different for each cullin, links the cullin N-terminus to the aforementioned variable substrate recruitment subunit (Sarikas et al., 2011). The specific complexes formed by Cul1, Cul2, Cul3, Cul4, Cul5, Cul7, and Cul9 are highlighted in Figure 1 and are individually discussed below.
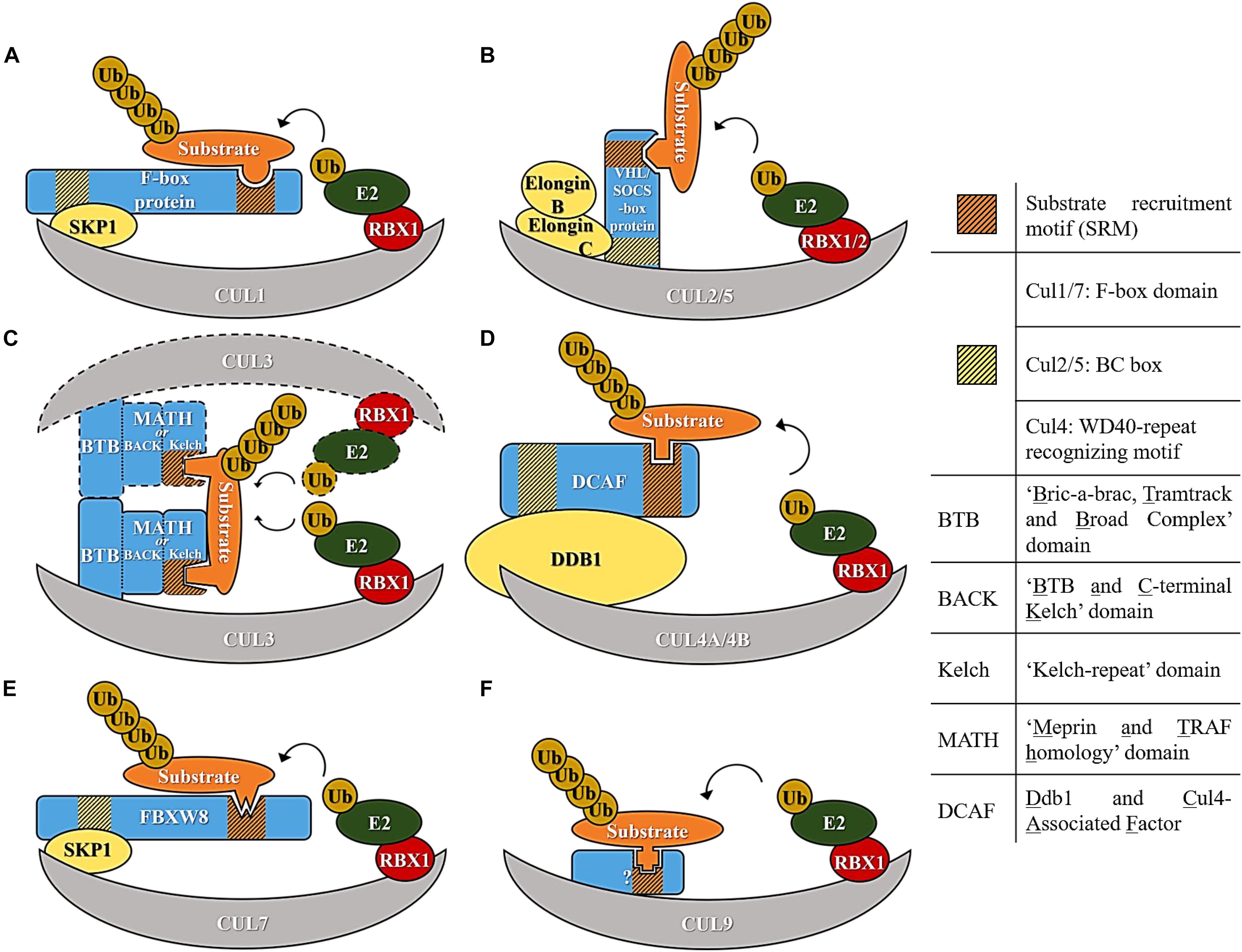
Figure 1. Diagram representing E3 ubiquitin ligase complexes of the cullin family of E3 ligases. (A) Cul1-based E3 ligase. (B) Cul2/5-based E3 ligase. (C) Cul3-based E3 ligase. (D) Cul4-based E3 ligase. (E) Cul7-based E3 ligase. (F) Cul9-based E3 ligase.
The prototypical multi-subunit E3s are Cullin1-based E3s, which assemble an SCF complex composed of Skp1, Cul1, and an F-box protein. In this particular E3 machinery, the Cul1 N-terminus binds to Skp1 (adaptor subunit), which in turn binds to a variable F-box protein (Figure 1A). F-box proteins derive their name from cyclin F in which the F-box domain, required for interaction with Skp1, was first discovered (Bai et al., 1996). After the discovery of cyclin F, about 69 F-box proteins were identified in the human genome based on their interaction with Skp1 and sequence homology within the F-box domain (Cenciarelli et al., 1999; Winston et al., 1999). F-box proteins were further sub-classified according to the protein domains they use to recruit substrates. Three F-box subfamilies exist; a subfamily that has a WD-40 domain (Fbxws), another that has a Leucine zipper (Fbxls) and finally one that has other variable domains (Fbxos) (Jin et al., 2004) (Figure 1A).
Work in the nematode C. elegans revealed the presence of cullin genes highly related and similar to Cul1. A search for EST in the human database identified Cul1, Cul2, Cul3, Cul4A, Cul4B, and Cul5 genes (Kipreos et al., 1996). Although the general organization of Cul2, Cul3, Cul4A, Cul4B, and Cul5 resemble the SCF assembly, structural studies have revealed substantial differences in the assembly and use of adaptors. These different features likely reflect different biochemical properties and mechanisms of action/ubiquitylation, however, studies on the kinetics of action comparing Cul1 to Cul2, Cul3, Cul4A, Cul4B, and Cul5 are lacking.
Cul2 and Cul5 are the most structurally related among the cullins and use elongins B and C as adaptors to engage a variable substrate recruitment protein. Among the most well-studied substrate recruitment proteins are the VHL tumor suppressor protein and VHL-like proteins, which use Cul2 as scaffold. Cul5 also recruits elongins B and C as adaptors but assembles with SOCS proteins to form a functional E3 (Figure 1B). The difference in specificity between Cul2 and Cul5 is related to the presence of a Cul2 and a Cul5 box, and these two distinct sequences mediate the interaction with the substrate recruitment subunits (Kamura et al., 2004).
In Cul3 complexes several BTB domain-containing proteins assemble directly with Cul3 and act as both an adaptor and a substrate recognition subunit. The BTB domain-containing proteins recognize substrates with their MATH (Meprin and TRAF homology) motif and Kelch beta-propeller repeats (Pintard et al., 2004; Genschik et al., 2013). A characteristic of these complexes is their intrinsic capacity for assembling homo-dimers through the BTB (Figure 1C). A quality control system regulating homo-heterodimerization of Kelch like proteins (Klhls) has recently been unveiled and depends on the activity of another E3 ubiquitin ligase of the F-box clade (Fbxl17) (Mena et al., 2018).
Cul4 machineries, which comprise Cul4A and Cul4B, use Ddb1 as an adapter. Ddb1 contains three WD40 propeller domains (BPA, BPB, and BPC) and assembles with a large family of DCAF (Ddb1 and Cul4 Associated Factor) proteins using a WDXR motif within the substrate recruitment factors (Figure 1D) (Jin et al., 2006).
Cul7 is similar to Cul1 in using Skp1 as an adaptor and recruiting Fbxw8 as a substrate receptor (Dias et al., 2002), but it can only assemble with Fbxw8 and not other F-box proteins (Figure 1E). The last member of the family and the most recently identified is Cul9 for which features of a cullin assembly are less clear (Figure 1F).
An attribute of the majority of the substrate recruitment subunits of the CRLs is that they recognize modified or unmodified short and unique amino acid sequences in substrates to initiate substrate engagement and ubiquitylation. These are collectively termed “degrons,” as they mark proteins for proteasomal degradation by the respective cullin machinery. Degrons are well-established but only for a small fraction of CRLs and novel insights have been recently made in deciphering the degron code at a system-wide level (Koren et al., 2018). Although the general organization of CRLs is conserved across different cullins there are substantial differences in complex assembly, which could dictate diverse modes of substrate engagement and modification. As more details of substrate engagement and ubiquitin chain specificity emerge, it will be important to compare the kinetics of action as well as the specificities of the different CRL complexes.
CRL complexes have been linked to many aspects of tumorigenesis as they participate in multiple biological processes. This review focuses specifically on the role of CRLs in the cellular response to IR covering also aspects of IR responses not related strictly to DSB repair.
Ionizing Radiation-Induced Damage
IR can induce a wide variety of biological effects within the cells and tissues and there is strong evidence to suggest that DNA damage is a major consequence. Indeed, a typical 2 Gy X-ray fraction used in clinical radiotherapy will result in approximately 80 DSBs, 2,000 SSBs, and over 4,000 base damages (Shrieve and Loeffler, 2011). Interestingly, the number of DNA lesions produced is low in comparison to the background level of endogenous DNA damage which corresponds to the order of 50,000 DNA lesions per day as a result of Reactive Oxygen Species (ROS) and other reactive metabolites (De Bont and van Larebeke, 2004). A critical feature of all types of IR is the deposition of energy in the form of highly structured tracks of ionization and excitation events and is therefore highly heterogeneous in space and time (Hill, 2018). This results in clusters of events not only on the nanometer scale, but in some cases on the micrometer scale, which could be responsible for the effectiveness of IR in treating cancers.
Damage to DNA is produced either by direct ionization of constituent atoms or indirectly as a result of free radicals produced close by in the surrounding water. This indirect damage is dominated by the hydroxyl (OH) radical which has a lifetime of ∼4 ns and a diffusion distance of ∼6 nm within the cell (Roots and Okada, 1975). As a result of clustering, these events not only produce simple isolated lesions such as base damage, abasic sites, SSBs, DNA-protein cross-links, but importantly are also efficient at producing combinations of these, including complex SSBs, DSBs, or complex DSBs. The increase in complexity of these DSBs results in an increase in the lifetime of these lesions and a decrease in overall repairability (Eccles et al., 2011). The relative proximity of these breaks can subsequently result in a wide range of chromosome aberrations including deletions, and for many cell types is an important driver of cell death as a result of a mitotic catastrophe.
While DNA damage produced is likely to dominate the response following clinical exposures, it is also known that IR can perturb existing intra- and inter-cellular signaling which may result in a change in the background level of ROS. These changes may be temporary but can often result in a persistent change in homeostasis of this signaling, and associated changes in oxidative stress can lead to a modulation of the background rate of endogenous DNA damage induction. Almost all solid tumors contain oxygen-deficient regions and as a result oxygen, or more specifically the absence of it, can play a significant role in the radiation response of tumors. The presence of oxygen at the time of irradiation results in an enhancement in the yield and complexity of the initial damage produced over that produced under hypoxia, as a result of the oxygen chemically “fixing” the damage thereby making it permanent (Rockwell et al., 2009). In addition to modulating the initial DNA damage produced by radiation, hypoxia results in the dysregulation of oxygen homeostasis and activates pathways which alter the phenotype of the cell, adapting the cell to the stress associated with low oxygen levels.
Roles of Cul1 in the Cellular Response to IR
Cul1 and IR-Induced Cell Cycle Arrest
Cells entering the cell cycle duplicate their DNA in S phase and complete cell division in M phase. The presence of checkpoints allows fidelity of cell cycle transitions. For instance, when cells are exposed to IR, the DNA damage generated promotes the activation of a checkpoint response. Cell cycle progression restarts only when the DNA damage is repaired.
The progression from one cell cycle phase to the next is promoted by the periodic oscillations of cyclins that partner with CDKs to phosphorylate target substrates (Murray, 2004). The activity of CDKs is halted by phosphorylation of the CDK subunit on tyrosine 15 mediated by the tyrosine kinase Wee1 and reverted by the Cdc25A phosphatase. The presence of DNA damage induced by IR activates checkpoint kinases (Chk1 and Chk2) whose role is to prevent the activity of CDKs and arrest cell cycle progression to allow DNA repair. Chk1 performs this function by controlling the ubiquitylation and subsequent degradation of the Cdc25A phosphatase (Busino et al., 2003; Jin et al., 2003). Cdc25A activates CDKs by removing the Wee1-mediated inhibitory phosphorylation on Tyr15 of CDKs. Therefore, upon activation of Chk1, the degradation of Cdc25A prevents CDK activity which in turn restrains DNA replication and progression of the cell cycle (Bartek and Lukas, 2003).
Beta Transduction Repeat-Containing Proteins β-TrCP1/Fbxw1 and β-TrCP2/Fbxw11, jointly referred to as β-TrCP, are the two Cul1 E3 ubiquitin ligases promoting the degradation of Cdc25A (Busino et al., 2003; Jin et al., 2003). A feature of the SCFβ−TrCP ubiquitin ligase is that it recognizes substrates containing the degron sequence DSGXXS in which both serines need to be phosphorylated (Guardavaccaro et al., 2003). Chk1 promotes the phosphorylation of Cdc25A and its subsequent degradation but the specific kinases responsible for Cdc25A degron phosphorylation are not completely clear. A number of kinases have been proposed to phosphorylate Cdc25A in addition to Chk1. Cdc25A, unlike the majority of other β-TrCP substrates that have the consensus degron DSGXXS, has the alternate degron 76SSESTDSG83 with serines Ser76, Ser79, and Ser82 phosphorylated (Busino et al., 2003; Jin et al., 2003). CkIα was shown to phosphorylate Ser76 of Cdc25A (Honaker and Piwnica-Worms, 2010), while Nek11 was shown to phosphorylate Ser82 (Melixetian et al., 2009). Gsk3β can carry out the priming phosphorylation of Ser76 on the Cdc25A degron (Kang et al., 2008).
The choice of kinase could be influenced by the cell cycle phase, for example Nek11-phosphorylation of Cdc25A is most active in S and G2 phases and is required for the establishment of a G2/M checkpoint promoted by IR, whereas Gsk3β and Chk1 are active in G1 and S phases. The control of Cdc25A by β-TrCP following DNA damage is crucial for normal cell cycle progression and the checkpoint response. Therefore, it represents a node with multiple control points. The lack of β-TrCP and Cdc25A degradation forces cells to undergo DNA replication in the presence of DNA damage, giving rise to a subsequent mitotic catastrophe and cell death (Busino et al., 2003; Jin et al., 2003).
In addition to Cdc25A, β-TrCP regulates other crucial components of the checkpoint response: Claspin and Wee1. Wee1 counteracts the activity of Cdc25A on CDK1. It follows that since β-TrCP is simultaneously acting on Wee1 and Cdc25A, the net effect of blocking β-TrCP should have no overall impact on cell cycle progression. However, the consequences of β-TrCP knockdown are significant. The discrepancy could be explained by the different-timed action of β-TrCP on Cdc25A and Wee1 dictated by the kinases responsible for phosphorylating the respective degrons. The kinases phosphorylating Cdc25A are acting when the checkpoint is operating, whereas the kinases phosphorylating Wee1 are Polo Like Kinase 1 (Plk1) and CDK1 itself, whose activity marks mitotic entry and, thus, checkpoint resolution (Watanabe et al., 2004).
An important sensor of DNA damage functioning to sustain the checkpoint response is Claspin. Claspin is required for Chk1 activity prompted by the ATR kinase, which detects the presence of DNA damage. Claspin levels are also regulated by β-TrCP, and Plk1 kinase triggers Claspin degradation (Mailand et al., 2006; Peschiaroli et al., 2006). This β-TrCP-mediated degradation of Claspin prevents any further activation of Chk1 during recovery from genotoxic stress. The process facilitates the restart of the cell cycle once the DNA damage has been resolved. The leftover active Chk1 is targeted for degradation by the F-box protein Fbxo6. Fbxo6 regulates Chk1 degradation to terminate the checkpoint response in S phase (Zhang et al., 2005; Zhang Y.-W. et al., 2009). From the regulation of Cdc25A, Wee1, Claspin and Chk1 by β-TrCP and Fbxo6, a temporally-ordered picture of checkpoint activation and resolution emerges where the action of kinases is enforced and coordinated by E3 ubiquitin ligases to promote irreversible cell cycle phase transitions.
Interestingly, Liu and colleagues reported that Matrix Extracellular Phosphoglycoprotein/Osteoblast Factor 45 (Mepe/Of45) is a co-factor of Chk1. Upon knocking down Mepe/Of45 in transformed rat embryo fibroblasts, Chk1 levels decrease which sensitizes cells to IR and other DNA damaging agents. It is suggested that the way Mepe/Of45 achieves that is by competing with the E3 ubiquitin ligases binding to Chk1, therefore decreasing the degradation of Chk1 by the UPS (Liu et al., 2009). However, more detailed mechanistic insights are necessary to derive a clear picture of the role of Mepe/Of45.
Another Cul1 adaptor with important roles in the IR-induced checkpoint response is cyclin F (Fbxo1). It has been shown that cyclin F prevents mitotic entry after IR by enforcing a G2 checkpoint. The mechanism relies on the capacity of cyclin F to competitively inhibit B-Myb. B-Myb activates the transcription of a number of genes required for mitotic entry, therefore the inhibition of transcriptional activity of B-Myb by cyclin F enables cyclin F to control mitotic entry after IR (Klein et al., 2015). Our laboratory has shown that the levels of cyclin F are reduced by β-TrCP at mitotic entry to facilitate the transition from G2 to M phase of the cell cycle (Mavrommati et al., 2018). However, it is yet unclear whether the regulation of cyclin F by β-TrCP has a role in the checkpoint response induced by IR in G2 as well.
It is also important to note that cyclin F ubiquitylates Exo1 which is a 5′ to 3′ exonuclease with a significant role in DNA repair after IR (Elia et al., 2015). One of the major forms of DNA damage induced by IR is the formation of DSBs. DSBs could be repaired by two main pathways: (1) simply ligating the broken ends together by non-homologous end joining (NHEJ); (2) by using a second copy of DNA as a template to initiate homologous recombination (HR). HR includes a number of steps which enable the engagement of a second copy of DNA to use as a template. An important part of the process that commits the cells to repair through HR is the resection of the broken ends of DNA to facilitate strand invasion of the homologous template. Exo1 controls long-range resection at DSBs upon IR, a process that needs to be tightly regulated to avoid hyper or hypo activity of Exo1. Thus, Exo1 levels are regulated by both phosphorylation and ubiquitylation after IR (Tomimatsu et al., 2017). Cyclin F was shown to mediate ubiquitylation of Exo1 after cells were challenged with 4NQO, a radio-mimetic drug (Elia et al., 2015), however it is unclear whether cyclin F is participating in regulating Exo1 levels and activity (and therefore resection) after IR.
Exo1 is not the only substrate of cyclin F involved in maintaining genome stability; there is also RRM2. RRM2 assembles with the RRM1 subunit to form a functional RNR, an essential enzyme in the production of deoxyribonucleotides (dNTPs) which are the building blocks of DNA (Nordlund and Reichard, 2006). The activity of RNR must be regulated in accordance with the cell cycle to maintain balanced levels of dNTPs for optimal DNA replication and DNA repair (D’Angiolella et al., 2012).
Abnormal dNTP levels have a negative effect on genomic stability and are known to promote transformation as well as an increase in the frequency of spontaneous mutations (Kunz et al., 1994). Accordingly, the overexpression of RRM2 promotes the development of lung cancer in mice (Xu et al., 2008), and elevated levels of RRM2 are often linked to poor prognosis in different cancer types (Morikawa et al., 2010a, b; Jones et al., 2011). RRM2 is targeted for degradation by cyclin F after phosphorylation of RRM2 on Thr33 by cyclin A/CDK1. This phosphorylation of RRM2 by CDK1 represents a failsafe mechanism to destroy RRM2 only in G2/M when the bulk of DNA replication has been completed (D’Angiolella et al., 2012). RRM2 alterations have been shown to impact DSB repair by HR in yeast (Moss et al., 2010) and mammalian cells (Gustafsson et al., 2018), thus cyclin F could also impact IR response by regulating RRM2.
Two other F-box proteins involved in checkpoint activation in response to IR are Fbxo4 and Fbxo31. This is due to their ability to regulate cyclin D1 levels following IR. Cyclin D1 promotes entry into the cell cycle from G0, and the regulation of cyclin D levels is important after the genotoxic stress induced by IR to promote cell survival (Lukas et al., 1994; Pagano et al., 1994; Agami and Bernards, 2000). It has been shown that the phosphorylation of Thr286 of cyclin D1 promotes the degradation of cyclin D1 by Fbxo4 in a cell cycle-dependent manner both in the absence of genotoxic stress, and in response to DNA damage (Pontano et al., 2008). Cell cycle-linked degradation of cyclin D1 by Fbxo4 requires RAS/RAF as its upstream pathway, whereas the DNA damage-induced degradation of cyclin D1 requires the ATM pathway (Pontano et al., 2008). Similarly to Fbxo4, Fbxo31 targets cyclin D1 for degradation after phosphorylation of Thr286, but in this case the presence of DNA damage is central to promote its degradation. The levels of Fbxo31 increase after DNA damage, unlike the levels of Fbxo4. Evidence indeed suggests that Fbxo31-mediated degradation of cyclin D1 following DNA damage may explain the prompt implementation of G1 arrest for checkpoint control (Santra et al., 2009).
Cul1 and p53-Mediated Checkpoint Response
Arguably, the most important regulator of cell fate upon checkpoint responses is the tumor suppressor and transcription factor p53. Upon IR, p53 is activated to promote the transcription of thousands of genes required for multiple cellular functions that range from activation of cell death and immune responses to checkpoint control and DNA repair. The major E3 ubiquitin ligase for p53 is MDM2, which targets p53 for ubiquitylation to maintain it in an inactive state (Momand et al., 1992; Oliner et al., 1993). However, given its central role, p53 is regulated by a myriad of other mechanisms. Such complex regulation is necessary, because p53 represents a central hub for cell survival, thus, multiple diverse pathways converge to regulate its function. For instance, numerous studies uncovered links between p53 and NF-κB, a family of transcription regulators with roles in regulating proliferation, apoptosis, inflammation, and the DDR (Wang et al., 2017). Interestingly, one study showed that IκB Kinase 2 (IKK2/IKKβ) phosphorylates p53 on Ser362 and Ser366, and stimulates the SCFβ−TrCP-mediated degradation of p53 (Xia et al., 2009). This is required to enforce inactivation of p53 in addition to the role of MDM2, however, biological consequences of this regulation are not yet fully elucidated.
The literature is somewhat confusing with regards to the regulation of p53 as another study suggests that MDM2 can be targeted for degradation by β-TrCP. MDM2 is phosphorylated by CkIα on multiple putative β-TrCP degrons (Inuzuka et al., 2010). This mechanism of regulation of MDM2 could be relevant after repeated DNA damage insults to control the transcriptional activity of p53. It is important to note that other researchers pointed out that what seems to be the degradation of MDM2 could just be epitope-masking by phosphorylation which blocks the epitope for the most commonly used MDM2 western blotting antibodies (Cheng and Chen, 2011).
Besides β-TrCP and MDM2, several ubiquitin ligases also target p53 for degradation like the Kelch domain-containing F-box protein, Jfk (Fbxo42). Jfk was found to inhibit p53-dependent transcription, and the depletion of Jfk was found to stabilize p53 and hence sensitizes cells to IR-induced DNA damage (Sun et al., 2009).
Among the transcriptional targets of p53, p21 has the most significant function in promoting cell cycle arrest. p21 acts as a direct inhibitor of CDKs and is required to enforce checkpoint control. Fbxl1, more widely-recognized as Skp2, targets p21 and p27 (the two CDK inhibitors) for ubiquitin-mediated proteolysis (Carrano et al., 1999). Skp2 has been linked to the regulation of a p53-dependent checkpoint response in melanoma cells through the control of p27 (Hu and Aplin, 2008). It is less clear whether Skp2 has a role in controlling checkpoint resolution through p21 degradation in G2, although Cul4-mediated degradation of p21 could also play a role (the roles of Cul4 are discussed in more detail later in this review).
Some F-box proteins are direct transcriptional targets of p53 like Fbxo22 (Vrba et al., 2008), however, the function of Fbxo22 as a p53 transcriptional target is yet unclear. It has been shown that Fbxo22 targets KDM4A for degradation. KDM4A is a histone demethylase that regulates the demethylation of histone H3 at positions K9 and K36 (Tan et al., 2011). However, a different study pointed out that Fbxo22 ubiquitylates p53 by forming a complex with KDM4A. In this case Fbxo22 was shown to recognize a methylated form of p53 and to promote the degradation of methylated p53. The degradation of p53 operated by Fbxo22 is required for the induction of senescence after DNA damage induced by IR (Johmura et al., 2016). Overall, multiple F-box proteins have been shown to regulate p53, and diversity in p53 regulation could simply reflect redundancy. However, it is also plausible that the regulation of p53 by each ligase depends on the input DNA damage signal and could lead to different transcriptional outputs and consequences for cell survival.
Cul1 and DSB Repair
Breast Cancer Type 1 Susceptibility Protein (BRCA1) is a tumor suppressor that has a significant role in HR following DNA DSBs. BRCA1, with its obligate binding partner BARD1, has been shown to act as a marker for sister chromatid availability (Nakamura et al., 2019) and to serve as a scaffold at DSB sites to recruit several proteins involved in the DNA damage repair pathway (Huen et al., 2009). BRCA1 forms a complex with BRCA2 which is facilitated by PALB2 (Sy et al., 2009; Zhang F. et al., 2009). This complex recruits the recombinase Rad51 to DSB sites and promotes the loading of Rad51 onto single-strand DNA, ultimately leading to the repair of DSBs by HR (Bhattacharyya et al., 2000; Zhang et al., 2012). The loss of BRCA2 and BRCA1 function is frequent in breast and ovarian cancers. Cancers bearing inactivating mutations in these genes are defective in the repair of DSBs by HR. It follows that forcing the use of HR by promoting the formation of unrepairable SSBs and DSBs triggers cell death. This principle is at the basis of the synthetic lethality observed between the loss of BRCA and the use of PARPi (Helleday, 2011). The striking sensitivity of tumors lacking BRCA1 and BRCA2 is exploited in the treatment of breast and ovarian cancers with PARPi.
The regulation of BRCA1 and BRCA2 is complex and far from being fully elucidated. Lu and colleagues proposed that the F-box protein Fbxo44 targets BRCA1 for its UPS-mediated degradation through the SCFFbxo44 E3 ligase complex (Lu et al., 2012). However, Fbxo44 did not localize= at damaged DNA sites and PARPi sensitivity was not tested after Fbxo44 depletion.
The F-box protein Emi1, also known as Fbxo5, has been recently discovered to be involved in controlling the BRCA1-BRCA2-Rad51 axis. In cell lines with inactivating BRCA1 mutations, SCFEmi 1 was found to target Rad51 for its ubiquitin-mediated degradation. However, under genotoxic stress Chk1 was found to inhibit Rad51 degradation by Emi1 through phosphorylation. The phosphorylation of Rad51 increases its affinity to BRCA2 and hence results in a surplus of Rad51, which restores HR in cells with faulty BRCA1 (Marzio et al., 2018). Given its role in regulating Rad51 proteolysis in cells lacking BRCA1, Emi1 was identified as a regulator of PARPi sensitivity in BRCA1-deficient triple-negative breast cancer (Marzio et al., 2018).
The Ku70/Ku80 heterodimer (Ku) is the initiating factor of the NHEJ pathway of DSB repair. Ku recognizes the ends of DSBs and recruits other components of the DNA damage repair pathway to the site of the break. After serving its role, Ku needs to be removed from the repaired DSB site but the mechanism of its removal is not clearly understood. Studying the egg extract of Xenopus laevis (African clawed frog), Postow et al. (2008) demonstrated that Ku80 is degraded in response to DSBs in a ubiquitin-dependent manner, and postulated that an SCF complex is the E3 ligase responsible for this ubiquitylation. It was shown that the K48-linked ubiquitylation step, but not the proteasomal degradation step that follows it, is required for removing Ku80 from repaired DSB sites (Postow et al., 2008). Fbxl12 was found to be the F-box protein responsible for the recruitment of the Ku80-ubiquitylating SCF complex to sites of DSBs, resulting in the degradation of Ku80 (Postow and Funabiki, 2013).
In mammalian cells other E3 ubiquitin ligases, which are not members of the CRL clade of E3s, have been shown to contribute to Ku80 removal from DSB sites. RING-Finger Protein 8 (RNF8) (Feng and Chen, 2012) and RNF126 (Ishida et al., 2017) were shown to be essential for the ubiquitylation and degradation of Ku80. RNF138 also promotes the ubiquitylation but not the degradation of Ku80 (Ismail et al., 2015).
Another F-box protein involved in DNA repair through Ku is Fbxw7. Fbxw7 has been previously found responsible for the ubiquitylation and subsequent degradation of a set of oncoproteins such as cyclin E, c-Jun and c-Myc via K48 linkage, which earned Fbxw7 recognition as a tumor suppressor (Nakayama and Nakayama, 2006; Welcker and Clurman, 2008). Adding to the functions of Fbxw7, it was recently discovered to promote DNA repair through NHEJ. Following exposure to IR, the ATM kinase phosphorylates Fbxw7 at Ser26 allowing the recruitment of the F-box protein to the DSB sites. Once at the DSB sites, Fbxw7 proceeds to K63-ubiquitylate the protein Xrcc4 at Lys296, which enhances the association of Xrcc4 with Ku and in turn promotes NHEJ. Given the ability of Fbxw7 to regulate NHEJ, inactivation of Fbxw7 promotes increased sensitivity of cancer cells to IR (Zhang et al., 2016).
Roles of Cul2 and Cul5 in the Cellular Response to IR
Cul2: Crucial Regulator of Hypoxia Response
Hypoxia-inducible factor 1 is a transcriptional factor expressed in hypoxic tumor cells and transiently induced in tumors as a result of oxidative stress following radiation (Semenza, 1999). Hif-1 transactivates several hypoxia-responsive genes, which results in the tumor acquiring malignant properties such as apoptotic resistance, enhanced tumor growth, invasion and metastasis (Semenza, 2003). Additionally, Hif-1 activates VEGFs and PDGFs which promote resistance to radiotherapy in endothelial cells, and also promote cell proliferation and blood vessel growth around tumors (Gorski et al., 1999; Moeller et al., 2004). Hif-1 is a heterodimer that consists of α and β subunits, and oxygen is responsible for the regulation of the α subunit (Hif-1α) at a post-translational level (Huang et al., 1998). In normoxia, proline residues in the ODD domain of Hif-1α get hydroxylated by oxygen-dependent prolyl hydroxylases (Bruick and McKnight, 2001; Epstein et al., 2001). The hydroxylation of Hif-1 serves as a signal to initiate the binding of Hif-1α to VHL. VHL is a Cul2 E3 ligase that assembles with Cul2, elongins B and C, and Rbx1, resulting in the proteasomal degradation of Hif-1α subunits. Under hypoxic conditions, the prolyl hydroxylases are inhibited, hindering the recognition of Hif-1α by the VHL protein and resulting in Hif-1 accumulation and a transcriptional response to hypoxia (Maxwell et al., 1999).
Given the contribution of Hif-1α and hypoxia to malignant and radiotherapy-resistant phenotypes in cancer, researchers have been trying to eliminate Hif-1α-active cells using strategies that hijack E3 ubiquitin ligases. One strategy worth noting is the development of the fusion protein TOP3 (TAT-ODD-procaspase-3) (Figure 2A) (reproduced from Harada et al., 2002). In this three-domain protein, the first domain is constituted by the HIV-Tat protein-transduction domain, required for the efficient delivery of TOP3 to cells and tissues in vitro and in vivo. The second domain is ODD (the VHL-mediated protein destruction motif of human Hif-1α), which promotes the stabilization of the peptide in hypoxia and its degradation in normoxia. The last domain is the proenzyme form of the human caspase-3, which endows TOP3 with cytocidal activity. The caspase 3 domain is activated following accumulated-TOP3 cleavage by endogenous caspases and triggers apoptosis. TOP3 is actively eliminated by VHL in normoxic cells but is stable in hypoxic cells where it promotes cell death. Since this design enables TOP3 to target hypoxic cells for elimination while sparing normoxic cells, and IR effectively targets normoxic cells but is less effective against hypoxic cells (Brown, 1999; Vaupel, 2004), the group suggested that the combination of IR and TOP3 could be a valuable therapeutic strategy. They proceeded to test their hypothesis, and found that this combinatorial approach efficiently reduces the population of tumor cells with Hif-1α induced by both hypoxia and IR, resulting in long-term suppression of tumor growth and angiogenesis compared to treatment by either IR or TOP3 alone (Harada et al., 2002).
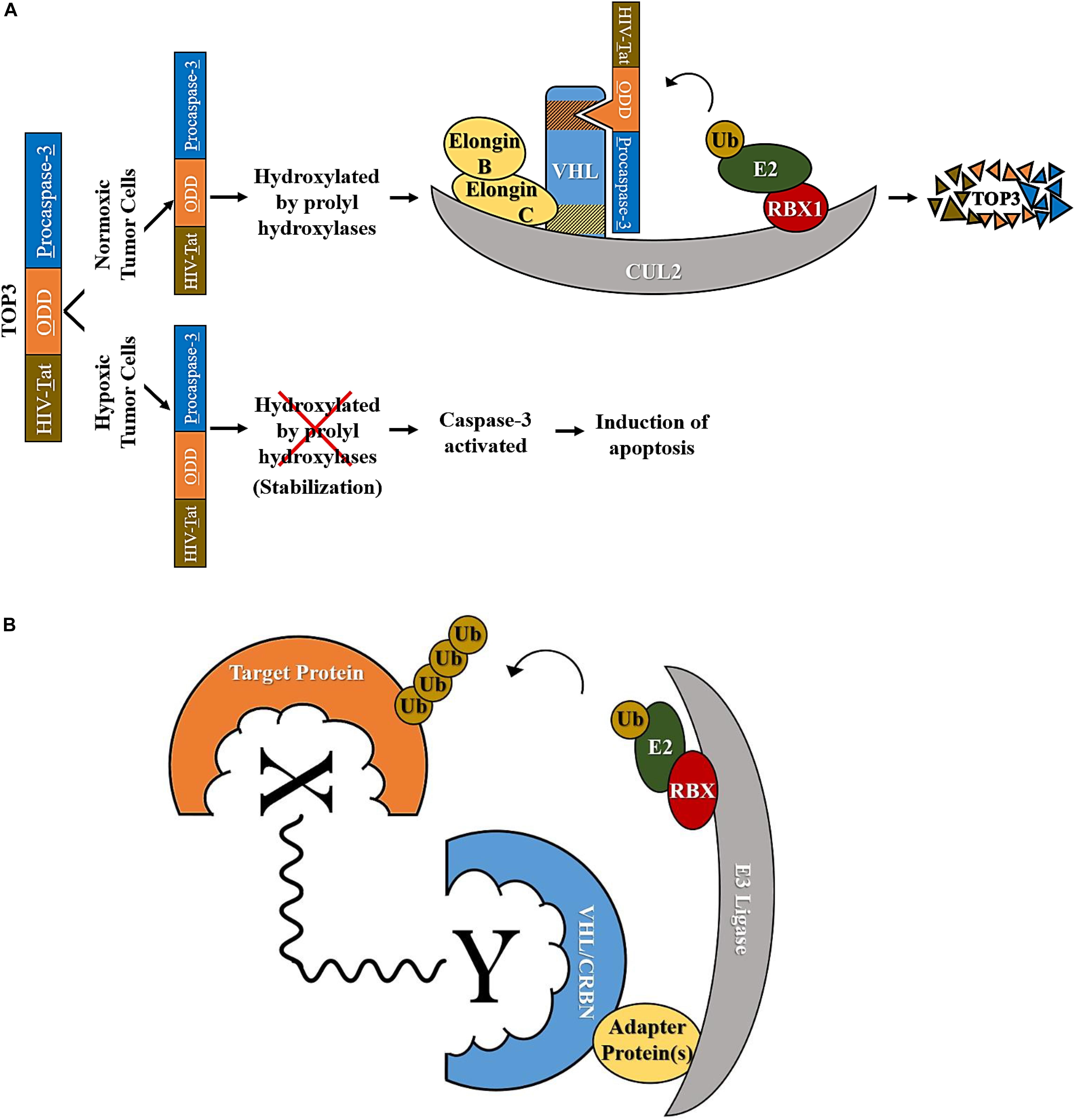
Figure 2. Examples of therapeutic approaches that exploit CRL complexes. (A) TOP3 technology; in normoxic tumor cells CRL2VHL targets TOP3 for degradation while in hypoxic tumor cells TOP3 is stabilized, caspase-3 is activated, and apoptosis is induced (adapted from Harada et al., 2005). (B) Degradation of a target protein through bringing it in close proximity to an E3 ligase using a PROTAC (represented as X∼Y where X is the target-binding ligand, Y is the E3-binding ligand, and ∼ is the linker molecule).
While additionally investigating the role of Hif-1 in the IR response, it was discovered that exposing cells to IR results in the p38 MAPK-mediated stabilization of Hif-1 under both hypoxic and normoxic conditions. It was found that upon IR, Hif-1α is stabilized by the decreased half-life of its E3 ligase subunit VHL and of its hydroxylating enzymes prolyl hydroxylases 2. Findings from this study suggest that, in combination with IR, targeting Hif-1α through its MAPK-mediated stabilization possibly has the potential to increase the efficacy of IR treatment in glioma (Kim et al., 2014). Hypoxic IR-resistant cells tend to spread, often resulting in relapse after treatment, therefore inactivation of Hif-1α could have a significant therapeutic response in glioma.
Roles of SOCS Boxes in Signaling and as Viral Adaptors
In addition to VHL, Cul2 or Cul5 can assemble with SOCS-box and ‘ankyrin repeat and SOCS box (ASB)’ proteins to form E3 ubiquitin ligase complexes that target proteins for proteasomal degradation. In complex with Cul2, elongin BC subcomplex, and Rbx1, SOCS1 accelerates the UPS-mediated degradation of Jak2 (Kamizono et al., 2001), a STAT3-activating tyrosine kinase which when inhibited using a small-molecule inhibitor sensitizes lung cancer cells to radiotherapy (Sun et al., 2011). Furthermore, SOCS1 has been shown to directly regulate the p53 response and apoptosis in T cells after IR by modulating STAT signaling (Calabrese et al., 2009). SOCS1 was also found to act as a substrate-binding motif targeting the HPV oncoprotein E7, inducing its ubiquitylation and subsequent degradation (Kamio et al., 2004). HPV is implicated in the formation of a subgroup of head and neck cancers that display high sensitivity to radiotherapy both in vitro and in vivo (Kimple et al., 2013; Dok et al., 2014; Sorensen et al., 2014). Therefore, the inhibition of SOCS1 could further enhance E7 levels to promote radiosensitivity.
Some SOCS-box-containing viral proteins such as the HIV-1 Vif have the capacity to hijack Cul5 E3 ubiquitin ligase complexes through which they acquire the ability to degrade host proteins (Yu et al., 2003). Acting as the substrate-specificity subunit of a CRL5 complex, the HIV-1 Vif protein targets the cellular intrinsic restriction factor APOBEC3G for proteasomal degradation (Yu et al., 2004). It has been reported that the inhibition of APOBEC3G activity using a Vif-derived peptide (Vif25-39) hinders DNA DSB repair in lymphoma cells, and hence sensitizes the cells to IR. The results from this study suggest that the peptide-mediated inhibition of APOBEC3G may be a potential radiosensitizing strategy (Prabhu et al., 2016), however, the mechanisms through which APOBEC3G affects DNA repair are not fully elucidated.
Another elongin BC-box protein is Wsb1. Kim et al. (2015) discovered that Wsb1 binds VHL and mediates its ubiquitylation and its subsequent proteasomal degradation. Hence, Wsb1 stabilizes Hif-1α by promoting the degradation of VHL under both hypoxic and normoxic conditions (Kim et al., 2015). Given that Hif-1 promotes resistance to radiotherapy, it is plausible that Wsb1 negatively influences the cellular response to IR by regulating Hif-1α, however, the role of Wsb1 in radiation sensitivity remains to be investigated.
Interestingly, it was found that in glioblastoma (GBM) cell lines, SOCS1 and SOCS3 are regulated reciprocally and SOCS3 is highly expressed in GBM, while SOCS1 is not. Expressing SOCS1 promotes radiosensitivity, while SOCS3 expression activates prosurvival signals in cancer cells through STAT-signaling, specifically STAT3 (Zhou et al., 2007; Sitko et al., 2008). Baek et al. (2016) evaluated the effect of the drug Resveratrol, a known suppressor of STAT3 signaling, on apoptosis induction and radiosensitization in SCCHN. They found that Resveratrol induces the expression of the SOCS1 protein and also its mRNA, which blocks the STAT3 signaling pathway, induces apoptosis and even enhances the rate of apoptosis when combined with IR (Baek et al., 2016). However, the functions of SOCS1 and SOCS3 in regulating STAT3 signaling in terms of their capacity to form Cul5 complexes are yet to be unraveled.
Roles of Cul3 in the Cellular Response to IR: DNA Repair and ROS Regulation
Bric-a-brac/tramtrack/broad complex -domain proteins serve as substrate-recruitment motifs of E3 ubiquitin ligase complexes and function as bridges to connect Cul3 and substrates. They have crucial roles in regulating two aspects of the IR response: DNA repair and ROS quenching.
An important aspect of DNA repair at DSBs is the choice between HR and NHEJ. While NHEJ is considered a fast and error prone repair mechanism, HR is considered error-free. However, while NHEJ can occur throughout the cell cycle, HR can only be carried out if a homologous template is present and thus is restricted to S and G2 phases of the cell cycle. Multiple mechanisms controlled by the ubiquitin system exist to control repair choice at specific phases of the cell cycle. For instance, Klhl15, a Cul3 adaptor, was found to accelerate the UPS-mediated degradation of the DNA endonuclease CtIP (Ferretti et al., 2016). CtIP together with the Mre11-Rad50-Nbs1 (MRN) complex control the choice of DNA DSB repair pathway by initiating DNA end-resection, which commits cells to HR by inhibiting NHEJ as processed DSB ends can no longer be targeted by the NHEJ pathway (Sartori et al., 2007; Chapman et al., 2012; Ceccaldi et al., 2016). Since IR induces DSBs, and CtIP is an essential factor of the DDR, the regulation of CtIP turnover by CRL3-Klhl15 is required to fine-tune the balance between HR and NHEJ in the cellular response to IR. Depletion of Klhl15 sensitizes cells to IR by disrupting the balance between these two repair pathways (Ferretti et al., 2016).
Another BTB protein that is crucial for responses to IR is Kelch-like ECH-Associated Protein 1 (Keap1). Keap1 has been directly implicated in the control of DSB repair; Keap1 ubiquitylates the BRCA1-interacting site of PALB2, preventing the interaction of PALB2 and BRCA1 and hence prohibits HR. The ubiquitylation by Keap1 does not lead to PALB2 proteolysis but restricts the interaction with BRCA1. The ubiquitylation of PALB2 is counteracted by USP11 during G2. This mechanism prevents the execution of HR in G1 (Orthwein et al., 2015).
The most well-studied function of CRL3-Keap1 is the regulation of the transcription factor Nf-E2-Related Factor 2 (Nrf2), a master mediator of the cellular defenses against ROS (Cullinan et al., 2004; Kobayashi et al., 2004; Zhang et al., 2004). Nrf2 binds to the ARE found within the promoters of several genes encoding proteins that have crucial roles in the anti-oxidation responses (Kensler et al., 2007). Under oxidative stress, several cysteine residues in Keap1 are covalently modified by thiol intermediates activated by the ROS radicals. The chemical modifications in Keap1 prevent the binding to Nrf2, which, as a consequence, is stabilized and can transcribe the genes required for the anti-oxidation response.
As oxidative stress is a key player in carcinogenesis, the Keap1-Nrf2 pathway serves a chemopreventive role and protects healthy cells from carcinogenesis. However, cancer cells have the ability to hijack this pathway to acquire survival advantages under high ROS, which are common events in cancer tissues (Chen and Chen, 2016). It was indeed found that Nrf2 overexpression in cancer cells decreases their sensitivity to IR and chemotherapy, while Nrf2 knockdown sensitizes the cells to such treatments (Shibata et al., 2008a; Wang et al., 2008; Solis et al., 2010; Zhang et al., 2010). Nrf2 is aberrantly overexpressed in many cancers, and one reason for this is the presence of mutations in Keap1, the ligase targeting Nrf2 (Shibata et al., 2008b; Yoo et al., 2012). Loss-of-function mutations in Keap1 were found in human lung carcinoma, NSCLC, gallbladder, ovarian, and liver cancers (Singh et al., 2006; Ohta et al., 2008; Shibata et al., 2008a; Konstantinopoulos et al., 2011; Yoo et al., 2012), whereas gain-of-function mutations in Nrf2 were found in lung, head and neck, and esophageal carcinoma (Shibata et al., 2008a, 2011).
Mutations in Nrf2 prevent binding to Keap1, leading to an increase in protein stability and constitutively high levels of Nrf2 (Tong et al., 2006). The mutational status of Keap1 and of Nrf2 have been directly connected to the success of localized radiotherapy in lung cancer (Jeong et al., 2017), underscoring the crucial role of this E3 ubiquitin ligase-substrate axis in the cellular response to IR.
Another protein worth noting is the chromatin-modifying enzyme Tip60; it is essential for DNA repair and apoptosis after IR (Ikura et al., 2000), its inhibition abolishes IR-induced G1 cell-cycle arrest (Berns et al., 2004), and it was also found to activate ATM following genotoxic stress (Sun et al., 2005). The stability and activity of Tip60 is reportedly tightly regulated by the combined action of Activating Transcription Factor-2 (ATF2) and CRL3, however, since ATF2 lacks a BTB domain, it is speculated that an unidentified BTB domain-containing protein is part of this CRL3 complex (Bhoumik et al., 2008).
Roles of Cul4A and Cul4B in the Cellular Response to IR: Controlling DNA Repair and DNA Replication
Cul4 and Ddb1, the substrate adapter for CRL4 complexes (Bondar et al., 2006; Sugasawa, 2009), are conserved from yeast to humans (Holmberg et al., 2005; Bondar et al., 2006; Kim and Kipreos, 2007). In lower organisms, the Cul4 protein is encoded by a single gene, but in mammals Cul4A and Cul4B proteins are encoded by two highly homologous genes (Iovine et al., 2011). Cul4A and Cul4B are 82% identical, and the difference between the two proteins is highlighted at their N-terminals; Cul4B is 149 amino acids longer than Cul4A as it has an N-terminal extension that contains an NLS. The NLS in Cul4B is believed to mediate the nuclear localization of Cul4B whereas Cul4A is mostly located in the cytoplasm (Zou et al., 2009; Hannah and Zhou, 2015).
Cul4A is reportedly aberrantly expressed in a plethora of cancers including breast cancer, squamous cell carcinoma, pleural mesothelioma (Shinomiya et al., 1999; Yasui et al., 2002; Melchor et al., 2009; Hung et al., 2011), and lung cancer (Wang et al., 2014), and its overexpression contributes to tumor progression, metastasis and poor prognosis (Wang et al., 2014). Compared to Cul4A, findings linking Cul4B to cancer are less common, however it was still found to be overexpressed in colon cancer (Jiang et al., 2013), cervical cancer, lung cancer, esophageal cancer, and breast cancer (Hu et al., 2012), with its overexpression closely related to tumor stage, invasion and metastasis.
Again as a leitmotiv of all E3 ubiquitin ligases, it was reported that Cul4A associates with MDM2 to degrade p53 (Nag et al., 2004), however, dissecting the role of Cul4 from all its adaptors is quite difficult and the emerging picture is that the observed activation of p53 is likely indirect.
One role of Cul4 complexes is to recognize UV-induced pyrimidine photodimers in DNA. In this case, the Ddb1 is bound to Ddb2 and directly recognizes DNA lesions. The ubiquitylation events mediated by Ddb1-Ddb2 following recognition subsequently help recruit the required proteins for the repair of the DNA lesion. A detailed explanation of the role of Cul4 complexes in UV-irradiation is outside the scope of this review and there are excellent reviews on the topic (Scrima et al., 2011). However, it is important to mention other important Cul4 adaptors that regulate genome stability, in particular Cdt2. Cdt2 is a Cul4 substrate-recognition subunit that targets p21 (Abbas et al., 2008), p27 (Li et al., 2006), Cdt1 (Jin et al., 2006) and the histone methyl-transferase Set8 (Abbas et al., 2010) for degradation. The mode of substrate engagement that Cdt2 uses is unique: Cdt2 couples substrate degradation to DNA replication by interacting with a modified PCNA Interacting Peptide (PIP) box in the substrate.
Proliferating cell nuclear antigen is an essential DNA clamp which travels with the DNA replication fork and acts as a scaffold for the recruitment of proteins required for DNA replication completion and fidelity. Proteins that interact with PCNA have in common a PIP-box motif consisting of the following amino-acid residues: Qxxψxxϑϑ. Substrates of Cdt2 contain an equivalent PIP box, but with modifications that increase the affinity for PCNA and favor a concomitant interaction with Cdt2. Thus, the recognition of Cdt2 substrates is mediated through the PIP degron: QxxψTDϑϑxxxK/R (Havens and Walter, 2009; Michishita et al., 2011). This strategy is important to control degradation of substrates present on the chromatin and traveling with DNA replication. Indeed, Cdt2 targets chromatin-bound p21 for degradation (Abbas et al., 2008; Kim et al., 2008) whilst the soluble p21 is targeted for degradation by Skp2 (Fbxl1) (Carrano et al., 1999).
Cdt2 also targets Cdt1 for degradation during S phase. Cdt1 is an important component of the Pre-RC, which defines DNA replication origins in G1. The Cdt2-dependant degradation of Cdt1 in S phase is one of several redundant mechanisms that prevent the re-initiation of DNA replication from regions of the genome which have already been replicated (Ralph et al., 2006). Interestingly, it was previously reported that Cdt1 is degraded by a CRL4 complex upon IR. This may contribute to the inhibition of replication in response to IR, although the majority of DNA replication inhibition is ascribed to checkpoint activation (Higa et al., 2003). The turnover of Cdt2 itself is also UPS-dependent and is mediated by the E3 ligase SCFFbxo11. This hints at an interesting crosstalk between CRL1 and CRL4 ligases (Abbas et al., 2013; Rossi et al., 2013).
Literature linking CRL4 complexes to IR is limited, but evidence from lower eukaryotes suggests that Cul4 based machineries could play a significant role in processing DSBs (Moss et al., 2010). More recently, Zeng et al. (2016) identified WDR70, a conserved DCAF that is recruited to DNA DSBs. WDR70 is part of a Cul4-Ddb1 ubiquitin ligase complex and promotes HR by stimulating long range resection(Zeng et al., 2016). Interestingly, knockdown of WDR70 sensitizes HEK293T cells to IR and is comparable to the survival observed upon Ddb1 depletion.
Whilst the hijacking of cullin machinery has been previously discussed, about 50% of human hepatocellular carcinomas are associated with chronic hepatitis B infection, and risk of carcinogenesis increases with viral load (El-Serag, 2011). The hepatitis B virus encodes an oncoprotein, Hepatitis B x protein (HBx) that can hijack the Cul4-Ddb1 machinery by displacing WDR70 (and likely other DCAFs) to form viral CRL4HBx. Disassembly of the CRL4WDR70 complex by HBx compromises DNA end resection and results in HR deficiency (Ren et al., 2019). Understanding the target of the CRL4WDR70 machinery may provide insights into new targets for sensitizing cancer cells to IR.
Targeting CRL Complexes for Cancer Therapy
Given the central role that CRLs have in regulating cancer pathogenesis and therapy responses, inhibitors of CRLs with limited specificity have been developed (Jang et al., 2018). MLN4924 (pevonedistat), a small-molecule inhibitor that selectively inhibits NEDD-8, was shown to inactivate CRL complexes and suppress tumor growth both in vitro and in vivo (Soucy et al., 2009). TAS4464 is another NEDDylation inhibitor, and most clinical trials involving either MLN4924 or TAS4464 are still restricted to phase I and II trials (Jang et al., 2018). The mechanism of action of MLN4924 is not selective as MLN4924 inhibits all the NEDDylation reactions in the cells. NEDDylation is essential for ubiquitylation operated by CRLs and thus NEDDylation inhibitors are effectively blocking the action of more than 400 enzymes in the cells. Despite the limited specificity, MLN4924 was shown to sensitize cancer cells to several chemotherapeutic drugs, so several of the ongoing trials are testing this small-molecule inhibitor in combination with DNA-damaging drugs such as carboplatin (Jang et al., 2018). MLN4924 was shown to act as a radiosensitizer in a mouse xenograft model of human pancreatic cancer (Wei et al., 2012), and in the human colorectal cancer cell lines HT-29 and HCT-116 (Wan et al., 2016). It was also found that MLN4924 radiosensitizes SCCHN in culture, and enhances IR-induced suppression of SCCHN xenografts in mice (Vanderdys et al., 2018).
It is possible to specifically inhibit individual CRLs or CRL-interacting proteins such as CRL substrates or CRL substrate-recruitment units, as opposed to global CRL- or proteasomal-inhibition, and this has in fact been attempted in a number of studies. For example, targeting CRL substrates that enhance the resistance of cells to radiotherapy, or targeting CRLs whose substrates sensitize cells to IR could potentially prove as an effective strategy to radiosensitize cancer cells. Substrates and substrate-recruitment members of CRLs that are involved in the cellular response to IR are summarized below in Table 1. An alternative emerging possibility to exploit CRL roles in cancer therapy is to use PROTACs (Figure 2B). PROTACs are heterobifunctional molecules made up of two different ligands and a linker; one of the two ligands binds to the target protein, and the other binds to an E3 ubiquitin ligase (An and Fu, 2018). Upon the formation of the E3 ligase-PROTAC-protein complex, E2 enzymes ubiquitylate the target protein marking it for proteasomal degradation (An and Fu, 2018). Thereby, a PROTAC molecule increases the proximity between the target protein and an E3 ligase, and acts catalytically to induce the proteolysis of this substrate.
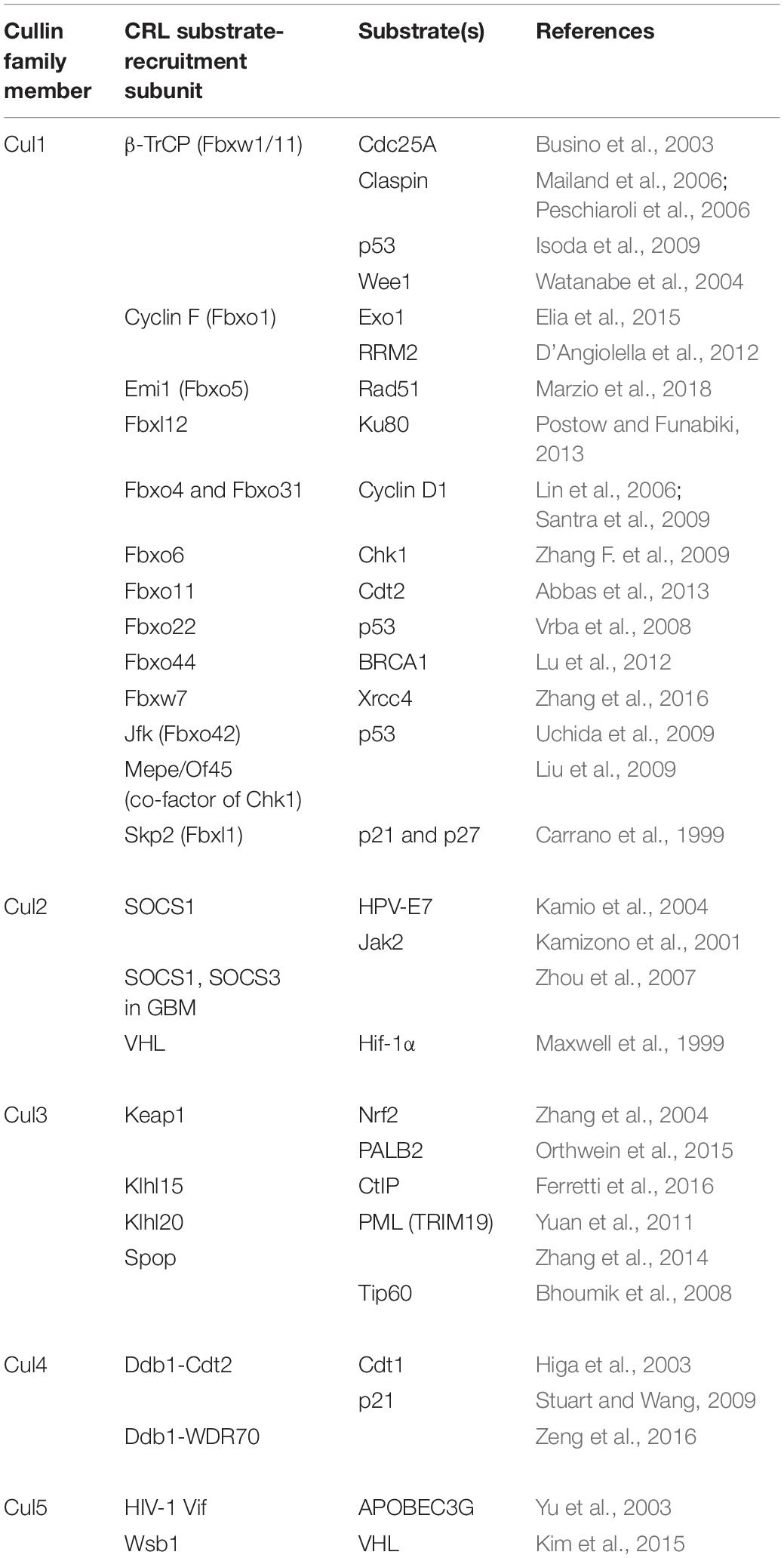
Table 1. Summary of the subunits of E3 ligase complexes of the cullin family that are involved in the IR response.
Proteolysis-targeting chimeras were designed to overcome several of the limitations that face their predecessors, small-molecule inhibitors. Small-molecule inhibitors are the most common targeted treatment method for intracellular proteins, however, they have several shortcomings. One example of such shortcomings is that small-molecule inhibitors are mainly engineered to target proteins with active sites (ex., enzymes or receptors), whereas the majority of the proteome lacks active sites and is therefore not targetable using this method (Toure and Crews, 2016). Target protein-binding ligands in PROTACs overcome this through binding to crevices on the surface of target proteins as opposed to active sites, making a much wider range of proteins druggable (An and Fu, 2018).
Through this design, and due to the nature of PROTAC-substrate binding being transient and reversible, PROTACs are also less prone than small-molecule inhibitors to drug resistance arising from mutations at the active site of the target protein (An and Fu, 2018).
Additionally, in contrast to the relatively high concentration of small-molecule inhibitors required to reach therapeutic capacity which often leads to off-target effects, PROTACs are required at much lower concentrations for two reasons. The first is that PROTACs act sub-stoichiometrically so they are involved in multiple rounds of protein degradation as opposed to small-molecule inhibitors that act in a 1:1 ratio to the substrate (Bondeson et al., 2015; Lu et al., 2015; Olson et al., 2018). And the second reason is that the hook effect applies to PROTACs, which entails that at high PROTAC concentrations the protein degradation efficiency decreases due to the direct inhibition of the E3 target (An and Fu, 2018).
Another advantage of PROTACs over small-molecule inhibitors stems from the fact that PROTACs exploit the intracellular UPS to degrade target proteins so the whole protein is lysed, as opposed to only disrupting the activity of a single domain in a protein by small-molecule inhibitors, which leaves the other domains in a multi-domain protein fully functional.
The concept of PROTACs was first introduced in 2001 (Sakamoto et al., 2001), and the first cell-permeable PROTAC was designed a few years later in 2004 (Schneekloth et al., 2004). At their infancy, PROTACs were peptide-based but that soon changed due to the poor permeability as well as low potency of the high-molecular weight peptide ligands, and the first small-molecule PROTAC was reported in 2008 (Schneekloth et al., 2008; Lai and Crews, 2017). It was reported that immunomodulatory drugs thalidomide, lenalidomide, and pomalidomide have the ability to hijack the ubiquitin ligase CRL4CRBN so small-molecule PROTACs with thalidomide (and derivatives)-based CRBN binding ligands were developed (An and Fu, 2018). Since 2015, the number of small-molecule PROTACs reported is more than 30, most of them utilizing VHL and cereblon (CRBN, a substrate recognition subunit of CRL4) E3 ligases (An and Fu, 2018). Currently, several pharmaceutical companies have programs for developing PROTACs, such companies include Arvinas, C4 Therapeutics, Kymera Therapeutics, and Captor Therapeutics (An and Fu, 2018). A large expansion of small molecules targeting E3 ligases is predicted given the significant investment from both pharma companies and academia.
Conclusion and Future Research Directions
Given the specific roles of E3 ligase adaptors in regulating multiple aspects of the cellular responses to IR, it is possible to envision that alteration of the CRLs could be exploited to improve radiotherapy response in cancer cells. For instance, the development of PROTACs targeting CRL adaptors in the DNA damage could lead to drugs that are only activated upon IR or under a specific stimulus like hypoxia. In the future it will be important to dissect the roles of the diverse CRLs at a system-wide level. It is important to emphasize the fact that studies on some subfamilies of CRLs (like CRL4 and CRL3) and DNA damage are quite limited and require further investigations that take into consideration the role of the adaptor, the alteration in selected cancer types and the impact in therapy response with radiotherapy and other DNA damaging agents.
In the search for new approaches to improve patient response to radiotherapy, all possible ways IR affects the cell need to be considered. The effect of IR is not limited to DNA damage by DSBs and SSBs, it also affects the immune system. It was recently noted that IR treatment could facilitate the immune responses by activating a cGAS/STING pathway to initiate immune signaling (Harding et al., 2017). This observation is likely at the basis of a well-known phenomena in the clinic called the abscopal effect. The abscopal effect, first defined in 1953, is the ability of localized radiotherapy to induce off-target anti-tumor effects leading to tumor regression at non-irradiated metastatic sites (Mole, 1953). Following this discovery, researchers began looking into the mechanisms guiding such effect and only 50 years later were able to make the breakthrough proposition that it was immune-system mediated (Demaria et al., 2004). With a plethora of recent studies reporting successful oncological applications of immune checkpoint inhibitors in clinical trials, researchers are becoming increasingly interested in the effects of combining immunotherapy with localized radiotherapy to best exploit the abscopal effect (Ng and Dai, 2016; Liu et al., 2018). The cullin family of E3 ligases is involved in several pathways of immune responses (Kawaida et al., 2005; Bibeau-Poirier et al., 2006; Kuiken et al., 2012; Mathew et al., 2012; Saucedo-Cuevas et al., 2014), therefore they could also be exploited to modulate the abscopal effect and/or cGAS/STING activation in combination with radiotherapy. A recent example of the involvement of E3s in immune checkpoint responses is represented by the E3 ligase Spop. In its E3 ligase complex with Cul3, Spop targets the ligand of the immune checkpoint Pd-L1 for its ubiquitin-mediated degradation (Zhang et al., 2018). Based on this mechanism, inhibiting Spop was found to result in an abundance of Pd-L1, and in combination with anti-Pd-1 immunotherapy was found to improve tumor regression and overall survival rates in murine models (Zhang et al., 2018).
In the future, CRL adaptors could be targeted with PROTACs to enhance the response of tumors to radiotherapy and elicit a systemic immune response. Given the large expansion of PROTAC-like-molecules in the last years, it is conceivable that further studies on CRLs might improve patient survival by changing the clinical approaches to cancer treatment with radiotherapy.
Author Contributions
SF worked on the main body of the review and collected the relevant literature. OW critically analyzed and reviewed the manuscript, and contributed to the description of the roles of Cul4 in IR responses. MH, as a radiation biophysics expert, wrote the paragraph on the biological effects of ionizing radiation. VD’A organized the literature, provided insightful comments, and revised the work on cullins.
Funding
This study was possible thanks to the support of a Medical Research Council (MRC) grant (MC_UU_00001/7) to VD’A. Funding provided by the MRC Strategic Partnership Funding (MC_PC_12004) for the Cancer Research UK (CRUK)/MRC Oxford Institute for Radiation Oncology is also gratefully acknowledged. This work was additionally supported by a CRUK grant (C5255/A18085) through the CRUK Oxford Centre.
Conflict of Interest Statement
The authors declare that the research was conducted in the absence of any commercial or financial relationships that could be construed as a potential conflict of interest.
Abbreviations
APOBEC3G, apolipoprotein B mRNA editing enzyme catalytic subunit 3G; ARE, antioxidant response element; ATF, activating transcription factor; ATM, ataxia telangiectasia-mutated; ATR, ataxia telangiectasia and Rad3-related; BACK, BTB and C-terminal kelch; BRCA, breast cancer susceptibility gene/protein; BTB, bric-a-brac/tramtrack/broad complex; β-TrCP, beta transduction repeat-containing protein; Cdc25, cell division cycle 25; Cdt, chromatin licensing and DNA replication factor; CDK, cyclin-dependent kinase; Chk, checkpoint kinase; CkI α, casein kinase I α; CRBN, cereblon; CRL, cullin-RING ligase; Cul, cullin; DCAF, Ddb1 and Cul4-associated factor; Ddb1, UV-damaged DNA-binding protein 1; DDR, DNA damage response; dNTPs, deoxyribonucleotides; DSB, double-strand break; Emi1, early mitotic inhibitor 1; EST, expressed sequence tag; Exo1, exonuclease 1; FDA, US Food and Drug Administration; Gsk3 β, glycogen synthase kinase 3 Beta; Hif, hypoxia-inducible factor; HIV, human immunodeficiency virus; HPV, human papilloma virus; HR, homologous recombination; IR, ionizing radiation; Jak2, Janus kinase 2; Jfk, just one F-box and kelch domain-containing protein; Kdm4A, lysine demethylase 4A; Keap1, kelch-like ECH-associated protein; Klhl, kelch-like protein; Ku, Ku70/Ku80 heterodimer; MAPK, mitogen-activated protein kinase; MATH, meprin and TRAF homology; MDM2, mouse double minute 2, human homolog of; P53, binding protein matrix extracellular; Mepe/Of, phosophoglycoprotein/osteoblast factor; MRN, Mre11-Rad50-Nbs1 complex; Nek11, NIMA (never in mitosis gene A)- related kinase 11; NF- κ B, nuclear factor kappa-light-chain-enhancer of activated B cells; NHEJ, non-homologous end joining; NLS, nuclear localization signal; Nrf2, Nf-E2-related factor 2; ODD, oxygen-dependent degradation; PALB2, partner and localizer of BRCA2; PARP, poly-ADP-ribose polymerase; PCNA, proliferating cell nuclear antigen; Pd-1, programed cell death protein 1; PDGF, platelet-derived growth factor; Pd-L1, programed cell death 1 ligand 1; Plk1, polo like kinase 1; PML, promyelocytic leukemia; PROTAC, proteolysis-targeting chimera; Rbx, RING-box protein; Rnf, RING-finger protein; RNR, ribonucleotide reductase; ROS, reactive oxygen species; RRM, ribonucleotide reductase family member; SCCHN, squamous cell carcinoma of the head and neck; SCF, Skp1/Cul1/F-box protein; Skp1, S-phase kinase-associated protein 1; SOCS, suppressor of cytokine signaling; Spop, speckle-type poxvirus and zinc-finger protein; SRM, substrate recruitment motif; SSB, single-strand break; STAT, signal transducer and activator of transcription; Tip60, 60 kDa tat-interactive protein; TOP3, TAT-ODD-procaspase-3; UPS, ubiquitin-proteasome system; Usp11, ubiquitin specific peptidase 11; VEGF, vascular endothelial growth factor; VHL, von-Hippel-Lindau; Vif, viral infectivity factor; Wsb1, WD repeat and SOCS box-containing protein 1; Xrcc4, X-ray repair cross complementing 4.
References
Abbas, T., Mueller, A. C., Shibata, E., Keaton, M., Rossi, M., and Dutta, A. (2013). Crl1-Fbxo11 promotes Cdt2 ubiquitylation and degradation and regulates Pr-Set7/Set8-mediated cellular migration. Mol. Cell 49, 1147–1158. doi: 10.1016/j.molcel.2013.02.003
Abbas, T., Shibata, E., Park, J., Jha, S., Karnani, N., and Dutta, A. (2010). Crl4(Cdt2) regulates cell proliferation and histone gene expression by targeting Pr-Set7/Set8 for degradation. Mol. Cell 40, 9–21. doi: 10.1016/j.molcel.2010.09.014
Abbas, T., Sivaprasad, U., Terai, K., Amador, V., Pagano, M., and Dutta, A. (2008). Pcna-dependent regulation of p21 ubiquitylation and degradation via the Crl4Cdt2 ubiquitin ligase complex. Genes Dev. 22, 2496–2506. doi: 10.1101/gad.1676108
Agami, R., and Bernards, R. (2000). Distinct initiation and maintenance mechanisms cooperate to induce G1 Cell cycle arrest in response to dna damage. Cell 102, 55–66. doi: 10.1016/s0092-8674(00)00010-6
An, S., and Fu, L. (2018). Small-molecule protacs: an emerging and promising approach for the development of targeted therapy drugs. EBioMedicine 36, 553–562. doi: 10.1016/j.ebiom.2018.09.005
Baek, S. H., Ko, J. H., Lee, H., Jung, J., Kong, M., Lee, J. W., et al. (2016). Resveratrol inhibits Stat3 signaling pathway through the induction of Socs-1: Role in apoptosis induction and radiosensitization in head and neck tumor cells. Phytomedicine 23, 566–577. doi: 10.1016/j.phymed.2016.02.011
Bai, C., Sen, P., Hofmann, K., Ma, L., Goebl, M., Harper, J. W., et al. (1996). Skp1 connects cell cycle regulators to the ubiquitin proteolysis machinery through a novel motif, the F-box. Cell 86, 263–274. doi: 10.1016/s0092-8674(00)80098-7
Bartek, J., and Lukas, J. (2003). Chk1 and Chk2 kinases in checkpoint control and cancer. Cancer Cell 3, 421–429. doi: 10.1016/s1535-6108(03)00110-7
Berns, K., Hijmans, E. M., Mullenders, J., Brummelkamp, T. R., Velds, A., Heimerikx, M., et al. (2004). A large-scale Rnai screen in human cells identifies new components of the p53 pathway. Nature 428, 431–437. doi: 10.1038/nature02371
Bhattacharyya, A., Ear, U. S., Koller, B. H., Weichselbaum, R. R., and Bishop, D. K. (2000). The breast cancer susceptibility gene Brca1 is required for subnuclear assembly of Rad51 and survival following treatment with the Dna cross-linking agent cisplatin. J. Biol. Chem. 275, 23899–23903. doi: 10.1074/jbc.c000276200
Bhoumik, A., Singha, N., O’connell, M. J., and Ronai, Z. A. (2008). Regulation of Tip60 by Atf2 modulates Atm activation. J. Biol. Chem. 283, 17605–17614. doi: 10.1074/jbc.M802030200
Bibeau-Poirier, A., Gravel, S. P., Clement, J. F., Rolland, S., Rodier, G., Coulombe, P., et al. (2006). Involvement of the IkappaB kinase (Ikk)-related kinases tank-binding kinase 1/Ikki and cullin-based ubiquitin ligases in Ifn regulatory factor-3 degradation. J. Immunol. 177, 5059–5067. doi: 10.4049/jimmunol.177.8.5059
Bondar, T., Kalinina, A., Khair, L., Kopanja, D., Nag, A., Bagchi, S., et al. (2006). Cul4A and Ddb1 associate with Skp2 to target p27Kip1 for proteolysis involving the Cop9 signalosome. Mol. Cell Biol. 26, 2531–2539. doi: 10.1128/mcb.26.7.2531-2539.2006
Bondeson, D. P., Mares, A., Smith, I. E., Ko, E., Campos, S., Miah, A. H., et al. (2015). Catalytic in vivo protein knockdown by small-molecule protacs. Nat. Chem. Biol. 11, 611–617. doi: 10.1038/nchembio.1858
Bruick, R. K., and McKnight, S. L. (2001). A conserved family of prolyl-4-Hydroxylases that modify hif. Science 294, 1337–1340. doi: 10.1126/science.1066373
Busino, L., Donzelli, M., Chiesa, M., Guardavaccaro, D., Ganoth, D., Dorrello, N. V., et al. (2003). Degradation of Cdc25A by beta-Trcp during S phase and in response to Dna damage. Nature 426, 87–91. doi: 10.1038/nature02082
Calabrese, V., Mallette, F. A., Deschenes-Simard, X., Ramanathan, S., Gagnon, J., Moores, A., et al. (2009). Socs1 links cytokine signaling to p53 and senescence. Mol. Cell. 36, 754–767. doi: 10.1016/j.molcel.2009.09.044
Carrano, A. C., Eytan, E., Hershko, A., and Pagano, M. (1999). Skp2 is required for ubiquitin-mediated degradation of the Cdk inhibitor p27. Nat. Cell Biol. 1, 193–199. doi: 10.1038/12013
Ceccaldi, R., Rondinelli, B., and D’andrea, A. D. (2016). Repair pathway choices and consequences at the double-strand break. Trends Cell Biol. 26, 52–64. doi: 10.1016/j.tcb.2015.07.009
Cenciarelli, C., Chiaur, D. S., Guardavaccaro, D., Parks, W., Vidal, M., and Pagano, M. (1999). Identification of a family of human F-box proteins. Curr. Biol. 9, 1177–1179.
Chapman, J. R., Sossick, A. J., Boulton, S. J., and Jackson, S. P. (2012). Brca1-associated exclusion of 53bp1 from Dna damage sites underlies temporal control of Dna repair. J. Cell Sci. 125, 3529–3534. doi: 10.1242/jcs.105353
Chen, H. Y., and Chen, R. H. (2016). Cullin 3 ubiquitin ligases in cancer biology: functions and therapeutic implications. Front. Oncol. 6:113. doi: 10.3389/fonc.2016.00113
Cheng, Q., and Chen, J. (2011). The phenotype of Mdm2 auto-degradation after Dna damage is due to epitope masking by phosphorylation. Cell Cycle 10, 1162–1166. doi: 10.4161/cc.10.7.15249
Cullinan, S. B., Gordan, J. D., Jin, J., Harper, J. W., and Diehl, J. A. (2004). The Keap1-Btb protein is an adaptor that bridges Nrf2 to a Cul3-based E3 ligase: oxidative stress sensing by a Cul3-Keap1 ligase. Mol. Cell Biol. 24, 8477–8486. doi: 10.1128/mcb.24.19.8477-8486.2004
D’Angiolella, V., Donato, V., Forrester, F. M., Jeong, Y. T., Pellacani, C., Kudo, Y., et al. (2012). Cyclin F-mediated degradation of ribonucleotide reductase M2 controls genome integrity and Dna repair. Cell 149, 1023–1034. doi: 10.1016/j.cell.2012.03.043
De Bont, R., and van Larebeke, N. (2004). Endogenous Dna damage in humans: a review of quantitative data. Mutagenesis 19, 169–185. doi: 10.1093/mutage/geh025
Demaria, S., Ng, B., Devitt, M. L., Babb, J. S., Kawashima, N., Liebes, L., and Formenti, S. C. (2004). Ionizing radiation inhibition of distant untreated tumors (abscopal effect) is immune mediated. Int. J. Radiat. Oncol. Biol. Phys. 58, 862–870. doi: 10.1016/j.ijrobp.2003.09.012
Dias, D. C., Dolios, G., Wang, R., and Pan, Z. Q. (2002). Cul7: A Doc domain-containing cullin selectively binds Skp1.Fbx29 to form an Scf-like complex. Proc. Natl. Acad. Sci. U.S.A. 99, 16601–16606. doi: 10.1073/pnas.252646399
Dok, R., Kalev, P., Van Limbergen, E. J., Asbagh, L. A., Vazquez, I., Hauben, E., et al. (2014). p16ink4a impairs homologous recombination-mediated Dna repair in human papillomavirus-positive head and neck tumors. Cancer Res. 74, 1739–1751. doi: 10.1158/0008-5472.CAN-13-2479
Eccles, L. J., O’neill, P., and Lomax, M. E. (2011). Delayed repair of radiation induced clustered Dna damage: friend or foe? Mutat. Res. 711, 134–141. doi: 10.1016/j.mrfmmm.2010.11.003
Elia, A. E., Boardman, A. P., Wang, D. C., Huttlin, E. L., Everley, R. A., Dephoure, N., et al. (2015). Quantitative proteomic atlas of ubiquitination and acetylation in the dna damage response. Mol. Cell 59, 867–881. doi: 10.1016/j.molcel.2015.05.006
Epstein, A. C. R., Gleadle, J. M., Mcneill, L. A., Hewitson, K. S., O’rourke, J., Mole, D. R., et al. (2001). C. elegans Egl-9 and mammalian homologs define a family of dioxygenases that regulate hif by prolyl hydroxylation. Cell 107, 43–54. doi: 10.1016/s0092-8674(01)00507-4
Feng, L., and Chen, J. (2012). The E3 ligase Rnf8 regulates Ku80 removal and Nhej repair. Nat. Struct. Mol. Biol. 19, 201–206. doi: 10.1038/nsmb.2211
Ferretti, L. P., Himmels, S. F., Trenner, A., Walker, C., Von Aesch, C., Eggenschwiler, A., et al. (2016). Cullin3-Klhl15 ubiquitin ligase mediates Ctip protein turnover to fine-tune Dna-end resection. Nat. Commun. 7:12628. doi: 10.1038/ncomms12628
Finley, D., Ciechanover, A., and Varshavsky, A. (2004). Ubiquitin as a central cellular regulator. Cell 116, S29–S32.
Genschik, P., Sumara, I., and Lechner, E. (2013). The emerging family of Cullin3-Ring ubiquitin ligases (Crl3s): cellular functions and disease implications. EMBO J. 32, 2307–2320. doi: 10.1038/emboj.2013.173
Gorski, D. H., Beckett, M. A., Jaskowiak, N. T., Calvin, D. P., Mauceri, H. J., Salloum, R. M., et al. (1999). Blockade of the vascular endothelial growth factor stress response increases the antitumor effects of ionizing radiation. Cancer Res. 59, 3374–3378.
Guardavaccaro, D., Kudo, Y., Boulaire, J., Barchi, M., Busino, L., Donzelli, M., et al. (2003). Control of meiotic and mitotic progression by the F box protein beta-Trcp1 in vivo. Dev. Cell 4, 799–812. doi: 10.1016/s1534-5807(03)00154-0
Gustafsson, N. M. S., Farnegardh, K., Bonagas, N., Ninou, A. H., Groth, P., Wiita, E., et al. (2018). Targeting Pfkfb3 radiosensitizes cancer cells and suppresses homologous recombination. Nat. Commun. 9:3872. doi: 10.1038/s41467-018-06287-x
Hannah, J., and Zhou, P. (2015). Distinct and overlapping functions of the cullin E3 ligase scaffolding proteins Cul4A and Cul4B. Gene 573, 33–45. doi: 10.1016/j.gene.2015.08.064
Harada, H., Hiraoka, M., and Kizaka-Kondoh, S. (2002). Antitumor effect of tat-oxygen-dependent degradation-caspase-3 fusion protein specifically stabilized and activated in hypoxic tumor cells. Cancer Res. 62, 2013–2018.
Harada, H., Kizaka-Kondoh, S., and Hiraoka, M. (2005). Optical imaging of tumor hypoxia and evaluation of efficacy of a hypoxia-targeting drug in living animals. Mol. Imaging 4, 182–193.
Harding, S. M., Benci, J. L., Irianto, J., Discher, D. E., Minn, A. J., and Greenberg, R. A. (2017). Mitotic progression following Dna damage enables pattern recognition within micronuclei. Nature 548, 466–470. doi: 10.1038/nature23470
Havens, C. G., and Walter, J. C. (2009). Docking of a specialized Pip Box onto chromatin-bound Pcna creates a degron for the ubiquitin ligase Crl4Cdt2. Mol. Cell 35, 93–104. doi: 10.1016/j.molcel.2009.05.012
Helleday, T. (2011). The underlying mechanism for the Parp and Brca synthetic lethality: clearing up the misunderstandings. Mol. Oncol. 5, 387–393. doi: 10.1016/j.molonc.2011.07.001
Hershko, A., Heller, H., Elias, S., and Ciechanover, A. (1983). Components of ubiquitin-protein ligase system. Resolution, affinity purification, and role in protein breakdown. J. Biol. Chem. 258, 8206–8214.
Higa, L. A., Mihaylov, I. S., Banks, D. P., Zheng, J., and Zhang, H. (2003). Radiation-mediated proteolysis of Cdt1 by Cul4-Roc1 and Csn complexes constitutes a new checkpoint. Nat. Cell Biol. 5, 1008–1015. doi: 10.1038/ncb1061
Hill, M. A. (2018). Track to the future: historical perspective on the importance of radiation track structure and Dna as a radiobiological target. Int. J. Radiat. Biol. 94, 759–768. doi: 10.1080/09553002.2017.1387304
Holmberg, C., Fleck, O., Hansen, H. A., Liu, C., Slaaby, R., Carr, A. M., et al. (2005). Ddb1 controls genome stability and meiosis in fission yeast. Genes Dev. 19, 853–862. doi: 10.1101/gad.329905
Honaker, Y., and Piwnica-Worms, H. (2010). Casein kinase 1 functions as both penultimate and ultimate kinase in regulating Cdc25A destruction. Oncogene 29, 3324–3334. doi: 10.1038/onc.2010.96
Hu, H., Yang, Y., Ji, Q., Zhao, W., Jiang, B., Liu, R., et al. (2012). Crl4B catalyzes H2ak119 monoubiquitination and coordinates with Prc2 to promote tumorigenesis. Cancer Cell 22, 781–795. doi: 10.1016/j.ccr.2012.10.024
Hu, R., and Aplin, A. E. (2008). Skp2 regulates G2/M progression in a p53-dependent manner. Mol. Biol. Cell. 19, 4602–4610. doi: 10.1091/mbc.E07-11-1137
Huang, L. E., Gu, J., Schau, M., and Bunn, H. F. (1998). Regulation of hypoxia-inducible factor 1α is mediated by an O2-dependent degradation domain via the ubiquitin-proteasome pathway. Proc. Natl. Acad. Sci. U.S.A. 95, 7987–7992. doi: 10.1073/pnas.95.14.7987
Huen, M. S. Y., Sy, S. M. H., and Chen, J. (2009). Brca1 and its toolbox for the maintenance of genome integrity. Nat. Rev. Mol. Cell Biol. 11, 138–148. doi: 10.1038/nrm2831
Hung, M. S., Mao, J. H., Xu, Z., Yang, C. T., Yu, J. S., Harvard, C., et al. (2011). Cul4A is an oncogene in malignant pleural mesothelioma. J. Cell Mol. Med. 15, 350–358. doi: 10.1111/j.1582-4934.2009.00971.x
Ikura, T., Ogryzko, V. V., Grigoriev, M., Groisman, R., Wang, J., Horikoshi, M., et al. (2000). Involvement of the Tip60 histone acetylase complex in Dna repair and apoptosis. Cell 102, 463–473. doi: 10.1016/s0092-8674(00)00051-9
Inuzuka, H., Tseng, A., Gao, D., Zhai, B., Zhang, Q., Shaik, S., et al. (2010). Phosphorylation by casein kinase i promotes the turnover of the Mdm2 oncoprotein via the Scfβ-Trcp Ubiquitin ligase. Cancer Cell 18, 147–159. doi: 10.1016/j.ccr.2010.06.015
Iovine, B., Iannella, M. L., and Bevilacqua, M. A. (2011). Damage-specific Dna binding protein 1 (Ddb1): a protein with a wide range of functions. Int. J. Biochem. Cell Biol. 43, 1664–1667. doi: 10.1016/j.biocel.2011.09.001
Ishida, N., Nakagawa, T., Iemura, S. I., Yasui, A., Shima, H., Katoh, Y., et al. (2017). Ubiquitylation of Ku80 by Rnf126 promotes completion of nonhomologous end joining-mediated dna repair. Mol. Cell Biol. 37:e00347-16. doi: 10.1128/MCB.00347-16
Ismail, I. H., Gagne, J. P., Genois, M. M., Strickfaden, H., Mcdonald, D., Xu, Z., et al. (2015). The Rnf138 E3 ligase displaces Ku to promote Dna end resection and regulate Dna repair pathway choice. Nat. Cell Biol. 17, 1446–1457. doi: 10.1038/ncb3259
Isoda, M., Kanemori, Y., Nakajo, N., Uchida, S., Yamashita, K., Ueno, H., et al. (2009). The extracellular signal-regulated kinase–mitogen-activated protein kinase pathway phosphorylates and targets Cdc25A for Scfβ-Trcp-dependent degradation for cell cycle arrest. Mol. Biol. Cell 20, 2186–2195. doi: 10.1091/mbc.e09-01-0008
Jang, S. M., Redon, C. E., and Aladjem, M. I. (2018). Chromatin-bound cullin-ring ligases: regulatory roles in dna replication and potential targeting for cancer therapy. Front. Mol. Biosci. 5:19. doi: 10.3389/fmolb.2018.00019
Jeong, Y., Hoang, N. T., Lovejoy, A., Stehr, H., Newman, A. M., Gentles, A. J., et al. (2017). Role of Keap1/Nrf2 and Tp53 mutations in lung squamous cell carcinoma development and radiation resistance. Cancer Discov. 7, 86–101. doi: 10.1158/2159-8290.CD-16-0127
Jiang, T., Tang, H. M., Wu, Z. H., Chen, J., Lu, S., Zhou, C. Z., et al. (2013). Cullin 4B is a novel prognostic marker that correlates with colon cancer progression and pathogenesis. Med. Oncol. 30:534. doi: 10.1007/s12032-013-0534-7
Jin, J., Arias, E. E., Chen, J., Harper, J. W., and Walter, J. C. (2006). A family of diverse Cul4-Ddb1-interacting proteins includes Cdt2, which is required for S phase destruction of the replication factor Cdt1. Mol. Cell 23, 709–721. doi: 10.1016/j.molcel.2006.08.010
Jin, J., Cardozo, T., Lovering, R. C., Elledge, S. J., Pagano, M., and Harper, J. W. (2004). Systematic analysis and nomenclature of mammalian F-box proteins. Genes Dev. 18, 2573–2580. doi: 10.1101/gad.1255304
Jin, J., Shirogane, T., Xu, L., Nalepa, G., Qin, J., Elledge, S. J., et al. (2003). Scfbeta-Trcp links Chk1 signaling to degradation of the Cdc25A protein phosphatase. Genes Dev. 17, 3062–3074. doi: 10.1101/gad.1157503
Johmura, Y., Sun, J., Kitagawa, K., Nakanishi, K., Kuno, T., Naiki-Ito, A., et al. (2016). Scf(Fbxo22)-Kdm4A targets methylated p53 for degradation and regulates senescence. Nat. Commun. 7:10574. doi: 10.1038/ncomms10574
Jones, R. J., Baladandayuthapani, V., Neelapu, S., Fayad, L. E., Romaguera, J. E., Wang, M., et al. (2011). Hdm-2 inhibition suppresses expression of ribonucleotide reductase subunit M2, and synergistically enhances gemcitabine-induced cytotoxicity in mantle cell lymphoma. Blood 118, 4140–4149. doi: 10.1182/blood-2011-03-340323
Kamio, M., Yoshida, T., Ogata, H., Douchi, T., Nagata, Y., Inoue, M., et al. (2004). Socs1 inhibits Hpv-E7-mediated transformation by inducing degradation of E7 protein. Oncogene 23, 3107–3115. doi: 10.1038/sj.onc.1207453
Kamizono, S., Hanada, T., Yasukawa, H., Minoguchi, S., Kato, R., Minoguchi, M., et al. (2001). The Socs box of Socs-1 accelerates ubiquitin-dependent proteolysis of Tel-Jak2. J. Biol. Chem. 276, 12530–12538. doi: 10.1074/jbc.m010074200
Kamura, T., Maenaka, K., Kotoshiba, S., Matsumoto, M., Kohda, D., Conaway, R. C., et al. (2004). Vhl-box and Socs-box domains determine binding specificity for Cul2-Rbx1 and Cul5-Rbx2 modules of ubiquitin ligases. Genes Dev. 18, 3055–3065. doi: 10.1101/gad.1252404
Kang, T., Wei, Y., Honaker, Y., Yamaguchi, H., Appella, E., Hung, M. C., et al. (2008). Gsk-3 beta targets Cdc25A for ubiquitin-mediated proteolysis, and Gsk-3 beta inactivation correlates with Cdc25A overproduction in human cancers. Cancer Cell 13, 36–47. doi: 10.1016/j.ccr.2007.12.002
Kawaida, R., Yamada, R., Kobayashi, K., Tokuhiro, S., Suzuki, A., Kochi, Y., et al. (2005). Cul1, a component of E3 ubiquitin ligase, alters lymphocyte signal transduction with possible effect on rheumatoid arthritis. Genes Immun. 6, 194–202. doi: 10.1038/sj.gene.6364177
Kensler, T. W., Wakabayashi, N., and Biswal, S. (2007). Cell survival responses to environmental stresses via the Keap1-Nrf2-Are pathway. Annu. Rev. Pharmacol. Toxicol 47, 89–116. doi: 10.1146/annurev.pharmtox.46.120604.141046
Kim, J. J., Lee, S. B., Jang, J., Yi, S. Y., Kim, S. H., Han, S. A., et al. (2015). Wsb1 promotes tumor metastasis by inducing pvhl degradation. Genes Dev, 29, 2244–2257. doi: 10.1101/gad.268128.115
Kim, Y., and Kipreos, E. T. (2007). Cdt1 degradation to prevent Dna re-replication: conserved and non-conserved pathways. Cell Div. 2:18. doi: 10.1186/1747-1028-2-18
Kim, Y., Starostina, N. G., and Kipreos, E. T. (2008). The Crl4Cdt2 ubiquitin ligase targets the degradation of p21Cip1 to control replication licensing. Genes Dev. 22, 2507–2519. doi: 10.1101/gad.1703708
Kim, Y. H., Yoo, K. C., Cui, Y. H., Uddin, N., Lim, E. J., Kim, M. J., et al. (2014). Radiation promotes malignant progression of glioma cells through Hif-1alpha stabilization. Cancer Lett. 354, 132–141. doi: 10.1016/j.canlet.2014.07.048
Kimple, R. J., Smith, M. A., Blitzer, G. C., Torres, A. D., Martin, J. A., Yang, R. Z., et al. (2013). Enhanced radiation sensitivity in Hpv-positive head and neck cancer. Cancer Res. 73, 4791–4800. doi: 10.1158/0008-5472.CAN-13-0587
Kipreos, E. T., Lander, L. E., Wing, J. P., He, W. W., and Hedgecock, E. M. (1996). cul-1 is required for cell cycle exit in C. elegans and identifies a novel gene family. Cell 85, 829–839. doi: 10.1016/s0092-8674(00)81267-2
Klein, D. K., Hoffmann, S., Ahlskog, J. K., O’hanlon, K., Quaas, M., Larsen, B. D., et al. (2015). Cyclin F suppresses B-Myb activity to promote cell cycle checkpoint control. Nat. Commun. 6:5800. doi: 10.1038/ncomms6800
Kobayashi, A., Kang, M. I., Okawa, H., Ohtsuji, M., Zenke, Y., Chiba, T., et al. (2004). Oxidative stress sensor Keap1 functions as an adaptor for Cul3-based E3 ligase to regulate proteasomal degradation of Nrf2. Mol. Cell Biol. 24, 7130–7139. doi: 10.1128/mcb.24.16.7130-7139.2004
Konstantinopoulos, P. A., Spentzos, D., Fountzilas, E., Francoeur, N., Sanisetty, S., Grammatikos, A. P., et al. (2011). Keap1 mutations and Nrf2 pathway activation in epithelial ovarian cancer. Cancer Res. 71, 5081–5089. doi: 10.1158/0008-5472.CAN-10-4668
Koren, I., Timms, R. T., Kula, T., Xu, Q., Li, M. Z., and Elledge, S. J. (2018). The Eukaryotic Proteome Is Shaped by E3 Ubiquitin Ligases Targeting C-Terminal Degrons. Cell 173, 1622.e14–1635.e14. doi: 10.1016/j.cell.2018.04.028
Kuiken, H. J., Egan, D. A., Laman, H., Bernards, R., Beijersbergen, R. L., and Dirac, A. M. (2012). Identification of F-box only protein 7 as a negative regulator of Nf-kappaB signalling. J. Cell Mol. Med. 16, 2140–2149. doi: 10.1111/j.1582-4934.2012.01524.x
Kunz, B. A., Kohalmi, S. E., Kunkel, T. A., Mathews, C. K., Mcintosh, E. M., and Reidy, J. A. (1994). Deoxyribonucleoside triphosphate levels: A critical factor in the maintenance of genetic stability. Mutat. Res. 318, 1–64. doi: 10.1016/0165-1110(94)90006-x
Lai, A. C., and Crews, C. M. (2017). Induced protein degradation: an emerging drug discovery paradigm. Nat. Rev. Drug Discov. 16, 101–114. doi: 10.1038/nrd.2016.211
Li, B., Jia, N., Kapur, R., and Chun, K. T. (2006). Cul4A targets p27 for degradation and regulates proliferation, cell cycle exit, and differentiation during erythropoiesis. Blood 107, 4291–4299. doi: 10.1182/blood-2005-08-3349
Lin, D. I., Barbash, O., Kumar, K. G. S., Weber, J. D., Harper, J. W., Klein-Szanto, A J. P., et al. (2006). Phosphorylation-dependent ubiquitination of cyclin D1 by the Scffbx4-αB crystallin complex. Mol. Cell 24, 355–366. doi: 10.1016/j.molcel.2006.09.007
Liu, S., Wang, H., Wang, X., Lu, L., Gao, N., Rowe, P. S., et al. (2009). Mepe/Of45 protects cells from Dna damage induced killing via stabilizing Chk1. Nucleic Acids Res. 37, 7447–7454. doi: 10.1093/nar/gkp768
Liu, Y., Dong, Y., Kong, L., Shi, F., Zhu, H., and Yu, J. (2018). Abscopal effect of radiotherapy combined with immune checkpoint inhibitors. J. Hematol. Oncol. 11:104. doi: 10.1186/s13045-018-0647-8
Lu, J., Qian, Y., Altieri, M., Dong, H., Wang, J., Raina, K., et al. (2015). Hijacking the E3 ubiquitin ligase cereblon to efficiently target Brd4. Chem. Biol. 22, 755–763. doi: 10.1016/j.chembiol.2015.05.009
Lu, Y., Li, J., Cheng, D., Parameswaran, B., Zhang, S., Jiang, Z., et al. (2012). The F-box protein Fbxo44 mediates Brca1 ubiquitination and degradation. J. Biol. Chem. 287, 41014–41022. doi: 10.1074/jbc.M112.407106
Lukas, J., Pagano, M., Staskova, Z., Draetta, G., and Bartek, J. (1994). Cyclin D1 protein oscillates and is essential for cell cycle progression in human tumour cell lines. Oncogene 9, 707–718.
Mailand, N., Bekker-Jensen, S., Bartek, J., and Lukas, J. (2006). Destruction of claspin by ScfβTrcp restrains Chk1 activation and facilitates recovery from genotoxic stress. Mol. Cell 23, 307–318. doi: 10.1016/j.molcel.2006.06.016
Marzio, A., Puccini, J., Kwon, Y., Maverakis, N. K., Arbini, A., Sung, P., et al. (2018). The F-Box domain-dependent activity of emi1 regulates parpi sensitivity in triple-negative breast cancers. Mol. Cell 73, 224.e6–237.e6. doi: 10.1016/j.molcel.2018.11.003
Mathew, R., Seiler, M. P., Scanlon, S. T., Mao, A. P., Constantinides, M. G., Bertozzi-Villa, C., et al. (2012). Btb-Zf factors recruit the E3 ligase cullin 3 to regulate lymphoid effector programs. Nature 491, 618–621. doi: 10.1038/nature11548
Mavrommati, I., Faedda, R., Galasso, G., Li, J., Burdova, K., Fischer, R., et al. (2018). beta-Trcp- and casein kinase ii-mediated degradation of cyclin F controls timely mitotic progression. Cell Rep. 24, 3404–3412. doi: 10.1016/j.celrep.2018.08.076
Maxwell, P. H., Wiesener, M. S., Chang, G. W., Clifford, S. C., Vaux, E. C., Cockman, M. E., et al. (1999). The tumour suppressor protein Vhl targets hypoxia-inducible factors for oxygen-dependent proteolysis. Nature 399, 271–275. doi: 10.1038/20459
Melchor, L., Saucedo-Cuevas, L. P., Munoz-Repeto, I., Rodriguez-Pinilla, S. M., Honrado, E., Campoverde, A., et al. (2009). Comprehensive characterization of the Dna amplification at 13q34 in human breast cancer reveals Tfdp1 and Cul4A as likely candidate target genes. Breast Cancer Res. 11:R86. doi: 10.1186/bcr2456
Melixetian, M., Klein, D. K., Sørensen, C. S., and Helin, K. (2009). Nek11 regulates Cdc25A degradation and the Ir-induced G2/M checkpoint. Nat. Cell Biol. 11, 1247–1253. doi: 10.1038/ncb1969
Mena, E. L., Kjolby, R. A. S., Saxton, R. A., Werner, A., Lew, B. G., Boyle, J. M., et al. (2018). Dimerization quality control ensures neuronal development and survival. Science 362:eaa8236. doi: 10.1126/science.aap8236
Michishita, M., Morimoto, A., Ishii, T., Komori, H., Shiomi, Y., Higuchi, Y., et al. (2011). Positively charged residues located downstream of Pip box, together with Td amino acids within Pip box, are important for Crl4(Cdt2) -mediated proteolysis. Genes Cells 16, 12–22. doi: 10.1111/j.1365-2443.2010.01464.x
Moeller, B. J., Cao, Y., Li, C. Y., and Dewhirst, M. W. (2004). Radiation activates Hif-1 to regulate vascular radiosensitivity in tumors: role of reoxygenation, free radicals, and stress granules. Cancer Cell 5, 429–441. doi: 10.1016/s1535-6108(04)00115-1
Momand, J., Zambetti, G. P., Olson, D. C., George, D., and Levine, A. J. (1992). The mdm-2 oncogene product forms a complex with the p53 protein and inhibits p53-mediated transactivation. Cell 69, 1237–1245. doi: 10.1016/0092-8674(92)90644-r
Morikawa, T., Hino, R., Uozaki, H., Maeda, D., Ushiku, T., Shinozaki, A., et al. (2010a). Expression of ribonucleotide reductase M2 subunit in gastric cancer and effects of Rrm2 inhibition in vitro. Hum. Pathol. 41, 1742–1748. doi: 10.1016/j.humpath.2010.06.001
Morikawa, T., Maeda, D., Kume, H., Homma, Y., and Fukayama, M. (2010b). Ribonucleotide reductase M2 subunit is a novel diagnostic marker and a potential therapeutic target in bladder cancer. Histopathology 57, 885–892. doi: 10.1111/j.1365-2559.2010.03725.x
Moss, J., Tinline-Purvis, H., Walker, C. A., Folkes, L. K., Stratford, M. R., Hayles, J., et al. (2010). Break-induced Atr and Ddb1-Cul4(Cdt)(2) ubiquitin ligase-dependent nucleotide synthesis promotes homologous recombination repair in fission yeast. Genes Dev. 24, 2705–2716. doi: 10.1101/gad.1970810
Nag, A., Bagchi, S., and Raychaudhuri, P. (2004). Cul4A physically associates with Mdm2 and participates in the proteolysis of p53. Cancer Res. 64, 8152–8155. doi: 10.1158/0008-5472.can-04-2598
Nakamura, K., Saredi, G., Becker, J. R., Foster, B. M., Nguyen, N. V., Beyer, T. E., et al. (2019). H4K20me0 recognition by Brca1-Bard1 directs homologous recombination to sister chromatids. Nat. Cell Biol. 21, 311–318. doi: 10.1038/s41556-019-0282-9
Nakayama, K. I., and Nakayama, K. (2006). Ubiquitin ligases: cell-cycle control and cancer. Nat. Rev. Cancer 6, 369–381. doi: 10.1038/nrc1881
Ng, J., and Dai, T. (2016). Radiation therapy and the abscopal effect: a concept comes of age. Ann. Transl. Med. 4:118. doi: 10.21037/atm.2016.01.32
Ohta, T., Iijima, K., Miyamoto, M., Nakahara, I., Tanaka, H., Ohtsuji, M., et al. (2008). Loss of Keap1 function activates Nrf2 and provides advantages for lung cancer cell growth. Cancer Res. 68, 1303–1309. doi: 10.1158/0008-5472.CAN-07-5003
Oliner, J. D., Pietenpol, J. A., Thiagalingam, S., Gyuris, J., Kinzler, K. W., and Vogelstein, B. (1993). Oncoprotein Mdm2 conceals the activation domain of tumour suppressor p53. Nature 362, 857–860. doi: 10.1038/362857a0
Olson, C. M., Jiang, B., Erb, M. A., Liang, Y., Doctor, Z. M., Zhang, Z., et al. (2018). Pharmacological perturbation of Cdk9 using selective Cdk9 inhibition or degradation. Nat. Chem. Biol. 14, 163–170. doi: 10.1038/nchembio.2538
Orthwein, A., Noordermeer, S. M., Wilson, M. D., Landry, S., Enchev, R. I., Sherker, A., et al. (2015). A mechanism for the suppression of homologous recombination in G1 cells. Nature 528, 422–426. doi: 10.1038/nature16142
Pagano, M., Theodoras, A. M., Tam, S. W., and Draetta, G. F. (1994). Cyclin D1-mediated inhibition of repair and replicative Dna synthesis in human fibroblasts. Genes Dev. 8, 1627–1639. doi: 10.1101/gad.8.14.1627
Peschiaroli, A., Dorrello, N. V., Guardavaccaro, D., Venere, M., Halazonetis, T., Sherman, N. E., et al. (2006). ScfβTrcp-mediated degradation of claspin regulates recovery from the dna replication checkpoint response. Mol. Cell 23, 319–329. doi: 10.1016/j.molcel.2006.06.013
Pintard, L., Willems, A., and Peter, M. (2004). Cullin-based ubiquitin ligases: Cul3-Btb complexes join the family. EMBO J. 23, 1681–1687. doi: 10.1038/sj.emboj.7600186
Pontano, L. L., Aggarwal, P., Barbash, O., Brown, E. J., Bassing, C. H., and Diehl, J. A. (2008). Genotoxic stress-induced cyclin D1 phosphorylation and proteolysis are required for genomic stability. Mol. Cell Biol. 28, 7245–7258. doi: 10.1128/MCB.01085-08
Postow, L., and Funabiki, H. (2013). An Scf complex containing Fbxl12 mediates Dna damage-induced Ku80 ubiquitylation. Cell Cycle 12, 587–595. doi: 10.4161/cc.23408
Postow, L., Ghenoiu, C., Woo, E. M., Krutchinsky, A. N., Chait, B. T., and Funabiki, H. (2008). Ku80 removal from Dna through double strand break-induced ubiquitylation. J. Cell Biol. 182, 467–479. doi: 10.1083/jcb.200802146
Prabhu, P., Shandilya, S. M., Britan-Rosich, E., Nagler, A., Schiffer, C. A., and Kotler, M. (2016). Inhibition of Apobec3G activity impedes double-stranded Dna repair. FEBS J. 283, 112–129. doi: 10.1111/febs.13556
Ralph, E., Boye, E., and Kearsey, S. E. (2006). Dna damage induces Cdt1 proteolysis in fission yeast through a pathway dependent on Cdt2 and Ddb1. EMBO Rep. 7, 1134–1139. doi: 10.1038/sj.embor.7400827
Ren, L., Zeng, M., Tang, Z., Li, M., Wang, X., Xu, Y., et al. (2019). The antiresection activity of the X protein encoded by hepatitis virus B. Hepatology 69, 2546–2561. doi: 10.1002/hep.30571
Rockwell, S., Dobrucki, I. T., Kim, E. Y., Marrison, S. T., and Vu, V. T. (2009). Hypoxia and radiation therapy: past history, ongoing research, and future promise. Curr. Mol. Med. 9, 442–458. doi: 10.2174/156652409788167087
Roots, R., and Okada, S. (1975). Estimation of life times and diffusion distances of radicals involved in x-ray-induced Dna strand breaks of killing of mammalian cells. Radiat. Res. 64, 306–320.
Rossi, M., Duan, S., Jeong, Y. T., Horn, M., Saraf, A., Florens, L., et al. (2013). Regulation of the Crl4(Cdt2) ubiquitin ligase and cell-cycle exit by the Scf(Fbxo11) ubiquitin ligase. Mol. Cell 49, 1159–1166. doi: 10.1016/j.molcel.2013.02.004
Sakamoto, K. M., Kim, K. B., Kumagai, A., Mercurio, F., Crews, C. M., and Deshaies, R. J. (2001). Protacs: chimeric molecules that target proteins to the Skp1-Cullin-F box complex for ubiquitination and degradation. Proc. Natl. Acad. Sci. U.S.A. 98, 8554–8559. doi: 10.1073/pnas.141230798
Santra, M. K., Wajapeyee, N., and Green, M. R. (2009). F-box protein Fbxo31 mediates cyclin D1 degradation to induce G1 arrest after Dna damage. Nature 459, 722–725. doi: 10.1038/nature08011
Sarikas, A., Hartmann, T., and Pan, Z. Q. (2011). The cullin protein family. Genome Biol. 12:220. doi: 10.1186/gb-2011-12-4-220
Sartori, A. A., Lukas, C., Coates, J., Mistrik, M., Fu, S., Bartek, J., et al. (2007). Human Ctip promotes Dna end resection. Nature 450, 509–514. doi: 10.1038/nature06337
Saucedo-Cuevas, L. P., Ruppen, I., Ximenez-Embun, P., Domingo, S., Gayarre, J., Munoz, J., et al. (2014). Cul4A contributes to the biology of basal-like breast tumors through modulation of cell growth and antitumor immune response. Oncotarget 5, 2330–2343.
Schneekloth, A. R., Pucheault, M., Tae, H. S., and Crews, C. M. (2008). Targeted intracellular protein degradation induced by a small molecule: en route to chemical proteomics. Bioorg. Med. Chem. Lett. 18, 5904–5908. doi: 10.1016/j.bmcl.2008.07.114
Schneekloth, J. S. Jr., Fonseca, F. N., Koldobskiy, M., Mandal, A., Deshaies, R., Sakamoto, K., et al. (2004). Chemical genetic control of protein levels: selective in vivo targeted degradation. J. Am. Chem. Soc. 126, 3748–3754. doi: 10.1021/ja039025z
Schwertman, P., Bekker-Jensen, S., and Mailand, N. (2016). Regulation of Dna double-strand break repair by ubiquitin and ubiquitin-like modifiers. Nat. Rev. Mol. Cell Biol. 17, 379–394. doi: 10.1038/nrm.2016.58
Scrima, A., Fischer, E. S., Lingaraju, G. M., Bohm, K., Cavadini, S., and Thoma, N. H. (2011). Detecting Uv-lesions in the genome: the modular Crl4 ubiquitin ligase does it best! FEBS Lett. 585, 2818–2825. doi: 10.1016/j.febslet.2011.04.064
Semenza, G. L. (1999). Regulation of mammalian O2 homeostasis by hypoxia-inducible factor 1. Annu. Rev. Cell Dev. Biol. 15, 551–578. doi: 10.1146/annurev.cellbio.15.1.551
Semenza, G. L. (2003). Targeting Hif-1 for cancer therapy. Nat. Rev. Cancer 3, 721–732. doi: 10.1038/nrc1187
Shibata, T., Kokubu, A., Gotoh, M., Ojima, H., Ohta, T., Yamamoto, M., et al. (2008a). Genetic alteration of Keap1 confers constitutive Nrf2 activation and resistance to chemotherapy in gallbladder cancer. Gastroenterology 135, 1358.e4–1368.e4. doi: 10.1053/j.gastro.2008.06.082
Shibata, T., Ohta, T., Tong, K. I., Kokubu, A., Odogawa, R., Tsuta, K., et al. (2008b). Cancer related mutations in Nrf2 impair its recognition by Keap1-Cul3 E3 ligase and promote malignancy. Proc. Natl. Acad. Sci. U.S.A. 105, 13568–13573. doi: 10.1073/pnas.0806268105
Shibata, T., Kokubu, A., Saito, S., Narisawa-Saito, M., Sasaki, H., Aoyagi, K., et al. (2011). Nrf2 mutation confers malignant potential and resistance to chemoradiation therapy in advanced esophageal squamous cancer. Neoplasia 13, 864–873.
Shinomiya, T., Mori, T., Ariyama, Y., Sakabe, T., Fukuda, Y., Murakami, Y., et al. (1999). Comparative genomic hybridization of squamous cell carcinoma of the esophagus: the possible involvement of the Dpi gene in the 13q34 amplicon. Genes Chromosomes Cancer 24, 337–344. doi: 10.1002/(sici)1098-2264(199904)24:4<337::aid-gcc7>3.3.co;2-f
Shrieve, D. C., and Loeffler, J. S. (2011). Human Radiation Injury, 1st Edn. Philadelphia, PA: Lippincott Williams & Wilkins.
Singh, A., Misra, V., Thimmulappa, R. K., Lee, H., Ames, S., Hoque, M. O., et al. (2006). Dysfunctional Keap1-Nrf2 interaction in non-small-cell lung cancer. PLoS Med. 3:e420. doi: 10.1371/journal.pmed.0030420
Sitko, J. C., Yeh, B., Kim, M., Zhou, H., Takaesu, G., Yoshimura, A., et al. (2008). Socs3 regulates p21 expression and cell cycle arrest in response to Dna damage. Cell Signal. 20, 2221–2230. doi: 10.1016/j.cellsig.2008.08.011
Solis, L. M., Behrens, C., Dong, W., Suraokar, M., Ozburn, N. C., Moran, C. A., et al. (2010). Nrf2 and Keap1 abnormalities in non-small cell lung carcinoma and association with clinicopathologic features. Clin. Cancer Res. 16, 3743–3753. doi: 10.1158/1078-0432.CCR-09-3352
Sorensen, B. S., Busk, M., Horsman, M. R., Alsner, J., Overgaard, J., Kyle, A. H., et al. (2014). Effect of radiation on cell proliferation and tumor hypoxia in Hpv-positive head and neck cancer in vivo models. Anticancer Res. 34, 6297–6304.
Soucy, T. A., Smith, P. G., Milhollen, M. A., Berger, A. J., Gavin, J. M., Adhikari, S., et al. (2009). An inhibitor of Nedd8-activating enzyme as a new approach to treat cancer. Nature 458, 732–736. doi: 10.1038/nature07884
Stuart, S. A., and Wang, J. Y. (2009). Ionizing radiation induces Atm-independent degradation of p21Cip1 in transformed cells. J. Biol. Chem. 284, 15061–15070. doi: 10.1074/jbc.M808810200
Sugasawa, K. (2009). Uv-Ddb: a molecular machine linking Dna repair with ubiquitination. DNA Repair 8, 969–972. doi: 10.1016/j.dnarep.2009.05.001
Sun, L., Shi, L., Li, W., Yu, W., Liang, J., Zhang, H., et al. (2009). Jfk, a Kelch domain-containing F-box protein, links the Scf complex to p53 regulation. Proc. Natl. Acad. Sci. U.S.A. 106, 10195–10200. doi: 10.1073/pnas.0901864106
Sun, Y., Jiang, X., Chen, S., Fernandes, N., and Price, B. D. (2005). A role for the Tip60 histone acetyltransferase in the acetylation and activation of Atm. Proc. Natl. Acad. Sci. U.S.A. 102, 13182–13187. doi: 10.1073/pnas.0504211102
Sun, Y., Moretti, L., Giacalone, N. J., Schleicher, S., Speirs, C. K., Carbone, D. P., et al. (2011). Inhibition of Jak2 signaling by Tg101209 enhances radiotherapy in lung cancer models. J. Thorac. Oncol. 6, 699–706. doi: 10.1097/JTO.0b013e31820d9d11
Sy, S. M., Huen, M. S., and Chen, J. (2009). Palb2 is an integral component of the Brca complex required for homologous recombination repair. Proc. Natl. Acad. Sci. U.S.A. 106, 7155–7160. doi: 10.1073/pnas.0811159106
Tan, M. K., Lim, H. J., and Harper, J. W. (2011). Scf(Fbxo22) regulates histone H3 lysine 9 and 36 methylation levels by targeting histone demethylase Kdm4A for ubiquitin-mediated proteasomal degradation. Mol. Cell Biol. 31, 3687–3699. doi: 10.1128/MCB.05746-11
Tomimatsu, N., Mukherjee, B., Harris, J. L., Boffo, F. L., Hardebeck, M. C., Potts, P. R., et al. (2017). Dna-damage-induced degradation of Exo1 exonuclease limits Dna end resection to ensure accurate Dna repair. J. Biol. Chem. 292, 10779–10790. doi: 10.1074/jbc.M116.772475
Tong, K. I., Kobayashi, A., Katsuoka, F., and Yamamoto, M. (2006). Two-site substrate recognition model for the Keap1-Nrf2 system: a hinge and latch mechanism. Biol. Chem. 387, 1311–1320.
Toure, M., and Crews, C. M. (2016). Small-molecule protacs: new approaches to protein degradation. Angew Chem. Int. Ed. Engl. 55, 1966–1973. doi: 10.1002/anie.201507978
Uchida, S., Yoshioka, K., Kizu, R., Nakagama, H., Matsunaga, T., Ishizaka, Y., et al. (2009). Stress-activated mitogen-activated protein kinases c-Jun Nh2-terminal kinase and p38 target Cdc25B for degradation. Cancer Res. 69, 6438–6444. doi: 10.1158/0008-5472.CAN-09-0869
Vanderdys, V., Allak, A., Guessous, F., Benamar, M., Read, P. W., Jameson, M. J., et al. (2018). The neddylation inhibitor pevonedistat (Mln4924) suppresses and radiosensitizes head and neck squamous carcinoma cells and tumors. Mol. Cancer Ther. 17, 368–380. doi: 10.1158/1535-7163.MCT-17-0083
Vaupel, P. (2004). Tumor microenvironmental physiology and its implications for radiation oncology. Semin. Radiat. Oncol. 14, 198–206. doi: 10.1016/j.semradonc.2004.04.008
Vrba, L., Junk, D. J., Novak, P., and Futscher, B. W. (2008). p53 induces distinct epigenetic states at its direct target promoters. BMC Genomics 9:486. doi: 10.1186/1471-2164-9-486
Wan, J., Zhu, J., Li, G., and Zhang, Z. (2016). Radiosensitization of human colorectal cancer cells by Mln4924: an inhibitor of Nedd8-activating enzyme. Technol. Cancer Res. Treat. 15, 527–534. doi: 10.1177/1533034615588197
Wang, W., Mani, A. M., and Wu, Z. H. (2017). Dna damage-induced nuclear factor-kappa B activation and its roles in cancer progression. J. Cancer Metastasis Treat. 3, 45–59. doi: 10.20517/2394-4722.2017.03
Wang, X. J., Sun, Z., Villeneuve, N. F., Zhang, S., Zhao, F., Li, Y., et al. (2008). Nrf2 enhances resistance of cancer cells to chemotherapeutic drugs, the dark side of Nrf2. Carcinogenesis 29, 1235–1243. doi: 10.1093/carcin/bgn095
Wang, Y., Wen, M., Kwon, Y., Xu, Y., Liu, Y., Zhang, P., et al. (2014). Cul4A induces epithelial-mesenchymal transition and promotes cancer metastasis by regulating Zeb1 expression. Cancer Res. 74, 520–531. doi: 10.1158/0008-5472.CAN-13-2182
Watanabe, N., Arai, H., Nishihara, Y., Taniguchi, M., Watanabe, N., Hunter, T., et al. (2004). M-phase kinases induce phospho-dependent ubiquitination of somatic Wee1 by Scfβ-Trcp. Proc. Natl. Acad. Sci. U.S.A. 101, 4419–4424. doi: 10.1073/pnas.0307700101
Wei, D., Li, H., Yu, J., Sebolt, J. T., Zhao, L., Lawrence, T. S., et al. (2012). Radiosensitization of human pancreatic cancer cells by Mln4924, an investigational Nedd8-activating enzyme inhibitor. Cancer Res. 72, 282–293. doi: 10.1158/0008-5472.CAN-11-2866
Welcker, M., and Clurman, B. E. (2008). Fbw7 ubiquitin ligase: a tumour suppressor at the crossroads of cell division, growth and differentiation. Nat. Rev. Cancer 8, 83–93. doi: 10.1038/nrc2290
Winston, J. T., Koepp, D. M., Zhu, C., Elledge, S. J., and Harper, J. W. (1999). A family of mammalian F-box proteins. Curr. Biol. 9, 1180–1182.
Xia, Y., Padre, R. C., De Mendoza, T. H., Bottero, V., Tergaonkar, V. B., and Verma, I. M. (2009). Phosphorylation of p53 by IκB kinase 2 promotes its degradation by β-Trcp. Proc. Natl. Acad. Sci. U.S.A. 106, 2629–2634. doi: 10.1073/pnas.0812256106
Xu, X., Page, J. L., Surtees, J. A., Liu, H., Lagedrost, S., Lu, Y., et al. (2008). Broad overexpression of ribonucleotide reductase genes in mice specifically induces lung neoplasms. Cancer Res. 68, 2652–2660. doi: 10.1158/0008-5472.CAN-07-5873
Yasui, K., Arii, S., Zhao, C., Imoto, I., Ueda, M., Nagai, H., et al. (2002). Tfdp1, Cul4A, and Cdc16 identified as targets for amplification at 13q34 in hepatocellular carcinomas. Hepatology 35, 1476–1484. doi: 10.1053/jhep.2002.33683
Yoo, N. J., Kim, H. R., Kim, Y. R., An, C. H., and Lee, S. H. (2012). Somatic mutations of the Keap1 gene in common solid cancers. Histopathology 60, 943–952. doi: 10.1111/j.1365-2559.2012.04178.x
Yu, X., Yu, Y., Liu, B., Luo, K., Kong, W., Mao, P., et al. (2003). Induction of Apobec3G ubiquitination and degradation by an Hiv-1 Vif-Cul5-Scf complex. Science 302, 1056–1060. doi: 10.1126/science.1089591
Yu, Y., Xiao, Z., Ehrlich, E. S., Yu, X., and Yu, X. F. (2004). Selective assembly of Hiv-1 Vif-Cul5-ElonginB-ElonginC E3 ubiquitin ligase complex through a novel Socs box and upstream cysteines. Genes Dev. 18, 2867–2872. doi: 10.1101/gad.1250204
Yuan, W. C., Lee, Y. R., Huang, S. F., Lin, Y. M., Chen, T. Y., Chung, H. C., et al. (2011). A Cullin3-Klhl20 Ubiquitin ligase-dependent pathway targets Pml to potentiate Hif-1 signaling and prostate cancer progression. Cancer Cell 20, 214–228. doi: 10.1016/j.ccr.2011.07.008
Zeng, M., Ren, L., Mizuno, K., Nestoras, K., Wang, H., Tang, Z., et al. (2016). Crl4(Wdr70) regulates H2B monoubiquitination and facilitates Exo1-dependent resection. Nat. Commun. 7:11364. doi: 10.1038/ncomms11364
Zhang, D., Wang, H., Sun, M., Yang, J., Zhang, W., Han, S., et al. (2014). Speckle-type Poz protein, Spop, is involved in the DNA damage response. Carcinogenesis 35, 1691–1697. doi: 10.1093/carcin/bgu022
Zhang, D. D., Lo, S. C., Cross, J. V., Templeton, D. J., and Hannink, M. (2004). Keap1 is a redox-regulated substrate adaptor protein for a Cul3-dependent ubiquitin ligase complex. Mol. Cell Biol. 24, 10941–10953. doi: 10.1128/mcb.24.24.10941-10953.2004
Zhang, F., Bick, G., Park, J. Y., and Andreassen, P. R. (2012). Mdc1 and Rnf8 function in a pathway that directs Brca1-dependent localization of Palb2 required for homologous recombination. J. Cell Sci. 125, 6049–6057. doi: 10.1242/jcs.111872
Zhang, F., Ma, J., Wu, J., Ye, L., Cai, H., Xia, B., et al. (2009). Palb2 links Brca1 and Brca2 in the DNA-damage response. Curr. Biol. 19, 524–529. doi: 10.1016/j.cub.2009.02.018
Zhang, Y.-W., Brognard, J., Coughlin, C., You, Z., Dolled-Filhart, M., Aslanian, A., et al. (2009). The F box protein Fbx6 regulates Chk1 stability and cellular sensitivity to replication stress. Mol. Cell 35, 442–453. doi: 10.1016/j.molcel.2009.06.030
Zhang, J., Bu, X., Wang, H., Zhu, Y., Geng, Y., Nihira, N. T., et al. (2018). Cyclin D-Cdk4 kinase destabilizes Pd-L1 via cullin 3-Spop to control cancer immune surveillance. Nature 553, 91–95. doi: 10.1038/nature25015
Zhang, P., Singh, A., Yegnasubramanian, S., Esopi, D., Kombairaju, P., Bodas, M., et al. (2010). Loss of Kelch-like Ech-associated protein 1 function in prostate cancer cells causes chemoresistance and radioresistance and promotes tumor growth. Mol. Cancer Ther. 9, 336–346. doi: 10.1158/1535-7163.MCT-09-0589
Zhang, Q., Karnak, D., Tan, M., Lawrence, T. S., Morgan, M. A., and Sun, Y. (2016). Fbxw7 facilitates nonhomologous end-joining via K63-linked polyubiquitylation of Xrcc4. Mol. Cell 61, 419–433. doi: 10.1016/j.molcel.2015.12.010
Zhang, Y.-W., Otterness, D. M., Chiang, G. G., Xie, W., Liu, Y.-C., Mercurio, F., et al. (2005). Genotoxic stress targets human Chk1 for degradation by the ubiquitin-proteasome pathway. Mol. Cell 19, 607–618. doi: 10.1016/j.molcel.2005.07.019
Zhou, H., Miki, R., Eeva, M., Fike, F. M., Seligson, D., Yang, L., et al. (2007). Reciprocal regulation of Socs 1 and Socs3 enhances resistance to ionizing radiation in glioblastoma multiforme. Clin. Cancer Res. 13, 2344–2353. doi: 10.1158/1078-0432.ccr-06-2303
Keywords: cullins, ionizing radiation (IR), double-strand breaks (DSBs), DNA-damage, cullin ring ligases (CRLs), E3-ligases
Citation: Fouad S, Wells OS, Hill MA and D’Angiolella V (2019) Cullin Ring Ubiquitin Ligases (CRLs) in Cancer: Responses to Ionizing Radiation (IR) Treatment. Front. Physiol. 10:1144. doi: 10.3389/fphys.2019.01144
Received: 03 May 2019; Accepted: 22 August 2019;
Published: 01 October 2019.
Edited by:
Fumiyo Ikeda, Institute of Molecular Biotechnology (OAW), AustriaReviewed by:
Sumit Sahni, Kolling Institute of Medical Research, AustraliaGiovanna Calabrese, University of Catania, Italy
Copyright © 2019 Fouad, Wells, Hill and D’Angiolella. This is an open-access article distributed under the terms of the Creative Commons Attribution License (CC BY). The use, distribution or reproduction in other forums is permitted, provided the original author(s) and the copyright owner(s) are credited and that the original publication in this journal is cited, in accordance with accepted academic practice. No use, distribution or reproduction is permitted which does not comply with these terms.
*Correspondence: Vincenzo D’Angiolella, vincenzo.dangiolella@oncology.ox.ac.uk