- 1Doctoral Degree’s School, Catholic University of Valencia “San Vicente Mártir”, Valencia, Spain
- 2Department of Nutrition and Dietetics, Catholic University of Valencia “San Vicente Mártir”, Valencia, Spain
- 3Department of Basic Sciences, Catholic University of Valencia “San Vicente Mártir”, Valencia, Spain
- 4Institute for Research on Musculoskeletal Disorders, Catholic University of Valencia “San Vicente Mártir”, Valencia, Spain
- 5Department of Basic Medical Sciences, Catholic University of Valencia “San Vicente Mártir”, Valencia, Spain
Amyotrophic lateral sclerosis (ALS) is a neurodegenerative disease that produces a selective loss of the motor neurons of the spinal cord, brain stem and motor cortex. Oxidative stress (OS) associated with mitochondrial dysfunction and the deterioration of the electron transport chain has been shown to be a factor that contributes to neurodegeneration and plays a potential role in the pathogenesis of ALS. The regions of the central nervous system affected have high levels of reactive oxygen species (ROS) and reduced antioxidant defenses. Scientific studies propose treatment with antioxidants to combat the characteristic OS and the regeneration of nicotinamide adenine dinucleotide (NAD+) levels by the use of precursors. This review examines the possible roles of nicotinamide riboside and pterostilbene as therapeutic strategies in ALS.
Oxidative Stress
Most eukaryotic organisms need oxygen to ensure the normal functioning of cellular energy metabolism, which is an evolutionary advantage and a highly efficient form of energy production (Jie et al., 2013). In the electron transport chain (ETC), oxygen is partially reduced by the incorporation of an electron, generating a free radical as a secondary product (Falkowski and Godfrey, 2008; Venditti et al., 2013). Free radicals are atoms or molecules that have one or more unpaired electrons in their last orbital layer (Chandrasekaran et al., 2017), which makes them strongly reactive capable of carrying out chain reactions, responsible for the oxidative damage of cells and tissues (Solleiro-Villavicencio and Rivas-Arancibia, 2018). They are classified as ROS and reactive nitrogen species (RNS) (De Gara and Foyer, 2017). Also included are reactive iron species (RIS) (Dixon and Stockwell, 2014) and copper species (RCS) (Gyulkhandanyan et al., 2003). The main ROS are: superoxide anion radicals (O), hydroxyl radicals (OH∙) and hydrogen peroxide (H2O2) (Popa-Wagner et al., 2013). The RNS include: nitric oxide radical (NO∙), nitroxyl anion (NO–), nitrosonium cation (NO+) and peroxynitrite anions (ONOO–) (Halliwell, 2012; Rogers et al., 2014; Zuo et al., 2015).
The production of ROS is mainly secondary to enzymatic reactions involved in the respiratory chain, activity of cytochrome p-450, synthesis of prostaglandins and phagocytosis (Pizzino et al., 2017). Mitochondrial activity and metabolism of cytochrome p-450 are the most contributing sources in mammalian cells (Jha et al., 2017) (Figure 1).
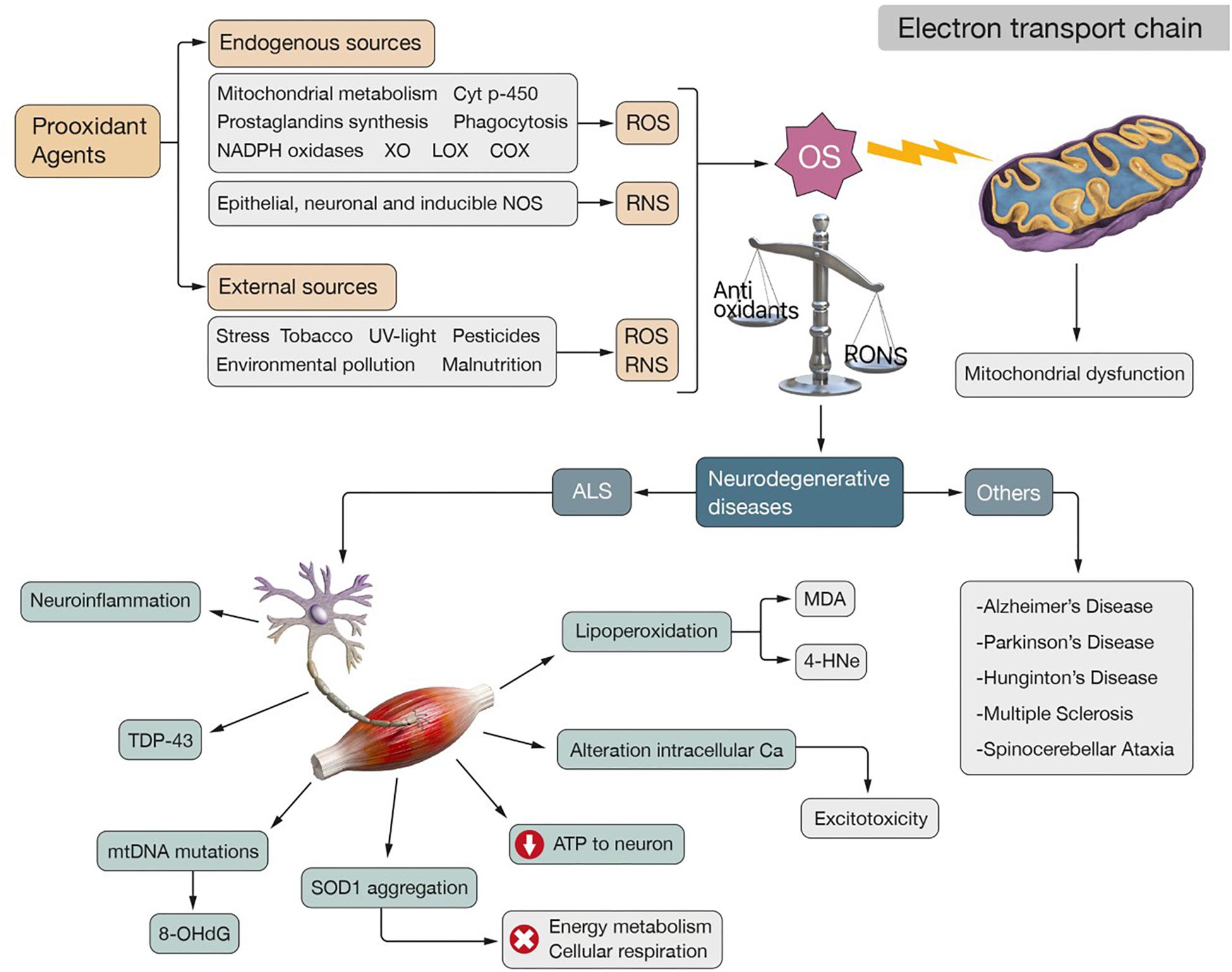
Figure 1. Physiopathogenesis of ALS. Oxidative stress is caused by an imbalance between antioxidant defenses and RONS in favor of these second ones. The endogenous production of ROS is secondary to different prooxidant agents: mitochondrial and cytochrome p-450 metabolism, prostaglandins synthesis, phagocytosis, NADPH oxidases, XO, LOX and COX. Among the endogenous sources of RNS it should be noted the activity of epithelial, neuronal and inducible NOS. Exogenous sources also can produce RONS: stress, tobacco, UV-light, pesticides, environmental pollution and malnutrition. OS affects the normal functioning of the ETC, producing mitochondrial dysfunction that generates more ROS, which leads to a “vicious-cycle” that increases metabolic stress. This situation characterizes neurodegenerative diseases such as: ALS, AD, PD, MS, HD, and SA. In the case of ALS, OS and mitochondrial dysfunction have been identified as two mechanisms involved in its pathogenesis. They are related to neuroinflammation, aggregation of TDP-43, mutations in the mtDNA that produce characteristic OS biomarkers such as 8-OHdG, aggregation of SOD1 which impairs energy metabolism and cellular respiration, affectation of ATP supply to neurons and disturbance of the intracellular calcium homeostasis that results in excitotoxicity. The formation of highly reactive end products such as MDA and 4-HNE, both secondary to the lipoperoxidation of the neuronal membranes, is also observed.
Mitochondrial production of ROS may occur on the external membrane, internal membrane or in the mitochondrial matrix, during physiological and pathological conditions (Pizzino et al., 2017). O production occurs when there is a buildup of nicotinamide adenine dinucleotide phosphate (NADPH) or when there is a reduced CoQ pool within the mitochondria (Murphy, 2009). O may also be secondary to the enzymatic activity of lipoxygenases (LOX) and cyclooxygenases (COX) (Valko et al., 2007) during the arachidonic acid metabolism and by endothelial and inflammatory cells (Al-Gubory et al., 2012). O may participate in reactions that produce H2O2 or OH∙ (Kumar and Pandey, 2015) (Figure 1).
The cytochrome p-450 enzymes present in the liver are an important source of ROS production and their function is to catalyze O producing reactions by NADPH dependent mechanisms (Liochev, 2013). The risk of ROS production here is high because it contains transition ions, oxygen and electron transfer processes (Liochev, 2013). In addition, there are a group of NOX (NADPH oxidases) enzymes located on the cell membrane of polymorphonuclear cells, macrophages and endothelial cells, that facilitate the conversion of oxygen into superoxide on biological membranes using NADPH as an electron donor with ROS released as secondary products (Atashi et al., 2015) (Figure 1). Endothelium xanthine dehydrogenase interacts with xanthine oxidase (XO) producing O and H2O2, and thus, generating another source of free radicals (Turrens, 2003) (Figure 1).
Non-enzymatic reactions may also be responsible for the production of ROS by the reaction of oxygen with organic compounds and after cellular exposure to ionizing radiation (Valko et al., 2007) (Figure 1).
The endogenous release of RNS, such as nitric oxide (NO∙), is produced from L-arginine in reactions of catalyze by three main isoforms of nitric oxide synthase (NOS): epithelial NOS, neuronal NOS and inducible NOS, which are activated in response to various endotoxin or cytokine signals (Förstermann and Sessa, 2012). Thus oxygen can react with this NO∙ and form highly reactive molecules such as ONOO– (Salisbury and Bronas, 2015; Sharina and Martin, 2017) (Figure 1).
Endogenous production of reactive oxygen and nitrogen species (RONS) can be conditioned by exogenous pro-oxidant factors: environmental and atmospheric pollution, water pollution, chemicals like pesticides or industrial solvents, heavy metals or transition metals, different types of xenobiotics, irradiation by UV-light, X-rays or gamma rays, stress, tobacco, smoked meat, the use of waste oil and malnutrition (Phaniendra et al., 2015; Niedzielska et al., 2016; Solleiro-Villavicencio and Rivas-Arancibia, 2018; Zewen et al., 2018) (Figure 1).
Reactive oxygen and nitrogen species at physiological concentrations are regulators of numerous cellular functions: cellular signaling pathway, control of cell survival, regulation of vascular tone, signal transduction by cell membrane receptors, membrane renewal, synthesis and release of hormones, increase of inflammatory cytokine transcription regulation of the immune system (Robberecht, 2000; Ray et al., 2012), phosphorylation of proteins, action on ionic channels and transcription factors, production of thyroid hormones and crosslinking on extracellular matrix (Brieger et al., 2012).
The body tries to maintain redox homeostasis between the production of RONS and the capacity for their removal by antioxidant systems (Zuo et al., 2015), which allows the redox state to be re-established after temporary exposure to high concentrations of RONS and minimize the risk of a deteriorated redox homeostasis, which is an unbalanced state known as OS (Sies, 1986; Coyle and Puttfarcken, 1993; Liguori et al., 2018) (Figure 1). However, redox homeostasis is conditioned by the magnitude and duration of exposure to free radicals, since constant exposure can have a serious impact on intracellular signals or genetic expression, resulting in irreversible pathological consequences (Rhee et al., 2003), since most reactions of the body are dependent on the redox state (Tan et al., 2018).
Diseases associated with OS, such as neurodegenerative diseases, are related to aging (Liguori et al., 2018), a physiological stage accompanied by progressive loss of tissue and organ function (Flatt, 2012), changes in regulatory processes, decrease in the antioxidant capacity of the organism and irreversible tissue damage by RONS that compromises the achievement of a redox balance (Romano et al., 2010). The damage caused by oxidation depends on the defects of the enzymes involved in the redox signaling pathways (Tan et al., 2018).
Free radicals can cross the cells and cause modifications in the main macromolecules (lipids, proteins and nucleic acids), damaging their structure and altering their normal function (Salisbury and Bronas, 2015). Lipid peroxidation is associated with different disease states and is responsible for an unstable cell membrane, oxidation of low-density lipoproteins (LDL) and poly-unsaturated fatty acids (PUFAs). The oxidation of different amino acids such as lysine, arginine, proline and threonine results in protein dysfunction. Oxidative damage of DNA generates severe mutations and adverse effects on transcription, producing an RNA more vulnerable to oxidation. Chronic persistence of this situation is capable of causing cell death (Huiyong et al., 2011; Dizdaroglu and Jaruga, 2012).
OS plays an important role in chronic, inflammatory pathologies (Kehrer and Klotz, 2015), progressive brain damage and the pathogenesis of neurodegenerative diseases such as: Amyotrophic lateral sclerosis (ALS), Alzheimer’s disease (AD), Parkinson’s disease (PD), Multiple sclerosis (MS), Huntington’s disease (HD), and Spinocerebellar ataxia (SA) (Dias et al., 2013; Islam, 2017; Zewen et al., 2018) (Figure 1).
Oxidative Stress and Neurodegenerative Diseases
The exact pathogenesis of neurodegenerative diseases remains unknown, although a complex and multifactorial origin has been established in which aspects such as genetic predisposition, certain endogenous factors and exposure to environmental factors are involved (Correia and Moreira, 2010; Correia et al., 2010; Coppedè and Migliore, 2015). ROS is a key factor in the etiology of these pathologies (Bhat et al., 2015) and in patients with a neurodegenerative disease, high levels of OS biomarkers have been observed (St-Pierre et al., 2006; Niedzielska et al., 2016). However, physiological concentrations of free radicals play an important role in normal brain function (De Silva and Miller, 2016; Scialò et al., 2017; Chen et al., 2018). Thus, ROS can contribute to: vascularization, cerebral perfusion, cellular signaling, synaptic plasticity, neurotransmitter secretion and cerebral vasodilation (Massaad and Klann, 2011; Grochowski et al., 2018; Neal and Richardson, 2018). A moderate increase of ROS secondary to mitochondrial activity produces preconditioning that leads to a neuroprotective function against harmful agents and is even preventive against massive ROS formation (Dirnagl and Meisel, 2008; Jou, 2008; Dirnagl et al., 2009). The origin of the problem lies in impaired redox homeostasis, which causes damage to cell membrane, compromising the viability and integrity of the central nervous system (CNS) (Van Horssen et al., 2011; Payne and Chinnery, 2015). The excess of ROS is related to changes in the microcirculation of the brain (Freeman and Keller, 2012; Staiculescu et al., 2014). O and H2O2 are able to cause a contraction of cerebral blood vessels (Allen and Bayraktunan, 2009). Increased levels of H2O2 are associated with increased pro-apoptotic agents in brain vascular cells (Li et al., 2003) and OS can alter cerebral vascular function through an interruption of NO∙ signaling and consequently, its vasodilatory and anti-inflammatory capacity (Miller et al., 2010; De Silva and Miller, 2016). In addition, ROS contribute to the maintenance of a proinflammatory state, secondary to secretion of cytokines and chemokines (Hsieh and Yang, 2013; Kehrer and Klotz, 2015).
The CNS is especially susceptible to oxidative damage (Cordeiro, 2014). The brain is largely formed by PUFAs with high sensitivity to lipid peroxidation (Nunomura et al., 2006), motor neurons are highly sensitive to OS (Simpkins and Dykens, 2008) and the CNS has a poor antioxidant capacity with low activity of protective enzymes such as glutathione peroxidase (GPx), catalase (CAT) or superoxide dismutase (SOD) and poor capacity for cell regeneration (Fischer and Maier, 2015; Kim et al., 2015; Formella et al., 2018). Although the brain only accounts for 2% of body weight (Mergenthaler et al., 2013) and mitochondrial density is higher in myocytes than in neuronal cells (Gandhi and Abramov, 2012), it is an organ with a high metabolic rate that can consume up to 20% of the total oxygen of the body (Moreira et al., 2009; Ronnett et al., 2009). This means it has 10 times higher energy and glucose consumption than the other tissues and a high dependence on mitochondrial energy production, responsible for the increase of ROS (Carvalho and Moreira, 2018). Taking into account the high rate of demand and energy consumption of the brain, the majority of mitochondrial mutations can affect the functioning of the CNS and have been associated with neurodegenerative diseases (Angelova and Abramov, 2018). Thus, mitochondrial dysfunction is currently seen as a “convergence point” in neurodegeneration (Correia et al., 2010; Bennett et al., 2014; Arun et al., 2016; Kurtishi et al., 2018).
Mitochondrial dysfunction compromises the energy supply of ATP to neurons, calcium homeostasis and leads to high levels of ROS that accelerate the mutation rate of mitochondrial DNA (mtDNA) and lipoperoxidation of neuronal membranes, causing decomposition of PUFAs and the formation of highly reactive end products: malondialdehyde (MDA) and 4-hydroxy-2-nonenal (HNE) (the most toxic species related to cell damage and apoptosis) (Petrozzi et al., 2007; Albarracín et al., 2012; Fritz and Petersen, 2013) (Figure 1). Accumulation of mutations in mtDNA causes an increase in oxidative damage, a decrease in energy rate and an increase in ROS. Thus, mitochondrial dysfunction generates a “vicious-cycle” responsible for neuronal damage, genetic mutations and metabolic stress, a situation that can lead to apoptosis (Van Houten et al., 2006; Selvaraji et al., 2019; Wang et al., 2019).
Mitochondrial dysfunction is considered as the main cause of neurodegenerative diseases pathogenesis (ALS, PD, AD, MS, HD) (Lin and Beal, 2006; Federico et al., 2012) (Figure 1). Postmortem studies in brains of patients with these diseases have found that mitochondrial dysfunction is a common event leading to the degeneration and death of neuronal cells (Schon and Manfredi, 2003; Bao and Swaab, 2018; Du et al., 2018).
Brain aging increases sensitivity to OS and decreases the effectiveness of antioxidant defenses, causing functional deficiencies, inflammation, decreased elasticity and greater susceptibility to the etiological factors involved in the pathogenesis of neurodegenerative disease (Federico et al., 2012; Guo et al., 2013). It also contributes to the accumulation of mutations in the mtDNA, malfunctions in the oxidative phosphorylation pathway and impaired redox homeostasis (Sarasija and Norman, 2018).
ROS have currently been proposed as one of the main contributors to the development and progression of neurodegenerative diseases (Morató et al., 2014). There is evidence that indicates that the increase in RONS concentration is a inducer of tissue damage, activation of microglia and astrocytes, neuronal dysfunction, neurodegeneration and cell death (Federico et al., 2012; Morató et al., 2014; Schieber and Chandel, 2014). Thus, a “vicious cycle” between OS, mitochondrial dysfunction, aging and neuroinflammation has been demonstrated (Witte et al., 2010; Van Giau et al., 2018). However, further studies are necessary to understand the physiopathological mechanisms implicated (Schetters et al., 2018) because it is unknown whether OS is a primary cause that induces the initiation of neurodegenerative diseases or if it is a side effect associated with the spread of damage to nerve cells (Jenner, 2003; Emerit et al., 2004).
Amyotrophic Lateral Sclerosis
ALS, also known as Charcot’s disease or Lou Gehrig’s disease, was originally described by Jean-Martin Charcot and Joffrey (Chen et al., 2013; Niedzielska et al., 2016) and is the most common motor neuron disease (Rechtmann et al., 2015). It causes a selective loss of upper motor neurons (UMN) of the motor cortex and lower motor neurons (LMN) of the brainstem and spinal cord (Brown and Al-Chalabi, 2017; Valko and Ciesla, 2019).
Patients with ALS develop weakness, muscle denervation, atrophy and progressive paralysis of all muscles (bulbar and respiratory), dysphagia (Al-Chalabi and Hardiman, 2013; D’Amico et al., 2013; Gowland et al., 2019) and respiratory muscle weakness that leads to respiratory failure and death (Sferrazza-Papa et al., 2018). The survival rate is 3–5 years after the onset of symptoms (Andersen, 2006; Gordon, 2013; Coan and Mitchell, 2015; Wier et al., 2019).
The incidence and prevalence of ALS vary according to the geography and design of the study (Marin et al., 2017; Kaye et al., 2018) and there are differences between African–American and Hispanic populations (Gordon et al., 2013). The incidence is 2–3 cases for every 1.000.000 inhabitants/year and prevalence of 4.6 – 5 for every 100.000 inhabitants in Western European countries (Chiò et al., 2013; Talbot, 2016). A significant increase in the overall prevalence of ALS to 8.58 cases per 100.000 people is expected by the year 2020 and to 9.67 per 100,000 people by 2116. In the United Kingdom prevalence in the 2010 year was 1415 cases and is projected to increase to 1701 by 2020 and 2635 by 2116 (Gowland et al., 2019).
ALS typically occurs in white adults between the ages of 50–60 (Chiò et al., 2013; Mehta et al., 2018), and cases in children are very rare (Martin, 2010; Bäumer et al., 2014; Ingre et al., 2015). The age of onset is earlier in men than in women and men are more prone to spinal involvement, whereas bulbar involvement is more common in women (McCombe and Henderson, 2010).
ALS is classified into two types: familial ALS (fALS) and sporadic ALS (sALS). fALS represents approximately 5–10% of the cases and is related to heterogeneous mutations of a set of genes with an autosomal dominant inheritance pattern (Mitchell and Borasio, 2007). Up to 20% of cases of fALS is due to a mutation of the gene that encodes the enzyme Cu-Zn superoxide dismutase (SOD1). sALS occurs in up to 90–95% of the cases but its origin is still unknown (Martin, 2010; Andersen and Al-Chalabi, 2011; Pan et al., 2012). fALS and sALS can affect any voluntary muscle but their presentation, phenotype and progression are variable (Wei et al., 2019), hindering differential diagnosis (Swinnen and Robberecht, 2014; Ghasemi, 2016; Van Es et al., 2017). ALS may manifest double onset: involvement of the extremities (80% of cases) or bulbar involvement (20% of cases) (Parakh et al., 2013).
The death of motor neurons results in a characteristic clinical feature: spasticity, muscular weakness and atrophy, hyperreflexia, cramps, fasciculation, Babinski sign, loss of coordination, paralysis of voluntary musculature, dysphagia and difficulties in swallowing, speech and respiration (Weiduschat et al., 2014; Zufiría et al., 2016; Solleiro-Villavicencio and Rivas-Arancibia, 2018). It is associated with metabolic disorders such as weight loss, hypermetabolism and hyperlipidemia (Dupuis et al., 2011). fALS and sALS do not affect the senses (sight, smell, taste, hearing and touch) (Fiala et al., 2013).
Several factors have been identified related to the pathogenesis of ALS: OS, mitochondrial dysfunction, neuroinflammation, excitotoxicity due to an increase in the neurotransmitter glutamate, defect in axonal transmission and in the metabolism of RNA, apoptosis, cytoskeletal abnormalities, disruption of membrane trafficking, endoplasmic reticulum stress, protein misfolding and aggregation (Papadimitriou et al., 2010; Blokhuis et al., 2013; Peters et al., 2015; Bond et al., 2018; Yusuf et al., 2018) and cysteine modifications like oxidation or palmitoylation that contribute to a general aberration of cysteine residues proteostasis (Valle and Carrì, 2017). A relationship has been established between environmental conditions and the epidemiology of ALS: alcohol, tobacco, sedentary lifestyle, fungal and viral infections or exposure to electromagnetic radiation (Yu et al., 2014).
There are currently no effective treatments for ALS and therapy is limited to symptomatic and palliative treatment (Bhattacharya et al., 2019). Riluzole (Miller et al., 2012) and edaravone (Rothstein, 2017) are the only approved pharmacological therapies that increase the survival rate by 2–3 months (Dorst et al., 2017; Hodgkinson et al., 2018), but edaravone is only beneficial to a subset of people with early stage ALS (Abe et al., 2017). Other therapeutic routes indicate multidisciplinary treatment involving: nurses, dieticians, nutritionists, occupational therapists, physical therapists, psychologists, social workers and speech therapists (Gordon, 2013).
Oxidative Stress and Amyotrophic Lateral Sclerosis
Presence of OS biomarkers and high levels of ROS have been determined in the CNS regions specifically in ALS patients (Golenia et al., 2014), which indicates that impaired redox homeostasis is a relevant factor and associated with the development and progression of neurodegeneration in fALS and sALS (Carrì et al., 2015; Petrov et al., 2017; Smith et al., 2017). High levels of certain types of ROS (H2O2 and O) have been observed in affected cells (Beal et al., 2005).
Loss of oxidative control and excessive ROS production are particularly linked to the mutated forms of SOD1 (Ferri et al., 2006; Hooten et al., 2015; Angelova and Abramov, 2018), which can have up to 150 different types of mutations (Gamez et al., 2006; Al-Chalabi et al., 2013). SOD1 is an antioxidant enzyme that catalyzes the conversion of O into H2O2 and O2, and is key in the regulation of OS, cell damage (Mattiazzi et al., 2002; Li et al., 2011; Pehar et al., 2014), energy metabolism and cellular respiration (Saccon et al., 2013). Oxidation and/or misfolding of SOD1 results in a deterioration in the regulation of the above processes, mitochondrial dysfunction and increase in the production of superoxides (Israelson et al., 2010; Pickles et al., 2013, 2016; Richardson et al., 2013). Its mutation predisposes cellular organelles to oxidative damage (Deng et al., 2006; Ahtoniemi et al., 2008) and excessive levels of ROS that attack astrocytes (Islam, 2017). OS contributes to the aggregation of SOD1 (Brujin et al., 2004) enhancing mitochondrial dysfunction (Liu et al., 2004; Vijayvergiya et al., 2005).
The transgenic mouse model of ALS (SOD1G93A) has enabled the identification of pathogenic mechanisms and the establishment of a “vicious cycle” between: OS, mitochondrial dysfunction and deterioration of the ETC (Jung et al., 2002; Menzies et al., 2002; Pfohl et al., 2015; Xiao et al., 2018). Most of the oxidative species that are formed in the CNS are secondary to oxidative phosphorylation (Brookes et al., 2004; Balaban et al., 2005; Karam et al., 2017) and mitochondrial dysfunction is the largest source of ROS production, so is a clear sign of affectation of motor neurons in the spinal cord and the motor cortex (Bendotti et al., 2001; Kawahara et al., 2004; Loizzo et al., 2010; Cozzolino and Carrì, 2012). Mitochondrial damage causes an alteration in intracellular calcium levels and in normal functioning of the ETC (Bond et al., 2018) that contributes to the increase of ROS and to the activation of chain reactions that lead to greater oxidative damage, reinforcing the “vicious cycle” (Harraz et al., 2008; Federico et al., 2012; Rojas et al., 2015; Loeffler et al., 2016; Van Horssen et al., 2017) and increasing cellular susceptibility to apoptosis (Guegan et al., 2001; Iverson and Orrenius, 2004).
Changes in antioxidant defense markers have been observed in patients with ALS (Cova et al., 2010). However, the results are random, due to the wide heterogeneity, form of presentation and variability of the pathogenic mechanisms of the disease (Johnson et al., 2012). Low levels of reduced glutathione (GSH) in erythrocytes (Babu et al., 2008) and in the motor cortex (Ikawa et al., 2015) of patients with ALS, a systemic pro-inflammatory state (increased levels of IL-6 and IL-8) and an impaired antioxidant system (Ehrhart et al., 2015) have been highlighted. One study compared in vivo levels of GSH in the motor cortex of 11 ALS patients with those in 11 age-matched healthy volunteers and determined that GSH levels were 31% lower in ALS patients than in healthy volunteers (Weiduschat et al., 2014). Also, decreased glutathione levels caused motor neuron degeneration in the hSOD1wt over-expressing mice model (hemizygous mice over-expressing wild-type hSOD1 at moderate levels) (Killoy et al., 2018) and accelerated motor neuron death in SOD1G93A mice (Pehar et al., 2014). Catalase (CAT) activity is decreased in erythrocytes of sALS patients (Nikolic-Kokic et al., 2006; Babu et al., 2008) and is significantly decreased compared to neurologically healthy controls (Golenia et al., 2014).
Studies conducted in vitro indicated that oxidative damage to nerve cells (astrocytes and oligodendrocytes) was related to neurodegeneration (Pollari et al., 2014). Oxidative imbalance decreases the number of glial cells and the ability to transmit the axonal signal (Park et al., 2007).
Markers for OS have been determined in the cerebrospinal fluid (CSF), tissues, blood and urine of patients with ALS (Blasco et al., 2017). After postmortem analysis of neuronal tissues in sALS and fALS patients, an increase of OS biomarkers was noted in proteins, lipids and DNA (Beal, 2002; Agar and Durham, 2003; Kim et al., 2003; Turner et al., 2013; Bozzo et al., 2016). The most frequently studied biomarkers include: carbonylated and/or glycosylated proteins, lipid peroxidation and DNA damage (Shibata et al., 2002).
In the nervous tissues of ALS patients, high levels of carbonylated proteins have been measured in the motor cortex (Sasaki et al., 2000; Guareschi et al., 2012; Tan et al., 2014) and high levels of advanced products of protein oxidation in the plasma of the erythrocytes of sALS patients (LoGerfo et al., 2014). In ALS-mouse models, non-functional proteins secondary to the oxidation of amino acid residues by peroxynitrite have been observed (Yoshino and Kimura, 2006). In addition, oxidative damage results in the aggregation of TDP-43, the major disease-associated protein involved in the pathogenesis of ALS (Moujalled et al., 2017; Oberstad et al., 2018) that promotes OS in neuronal cells (Duan et al., 2010; Cohen et al., 2012, 2015; Magrané et al., 2014) (Figure 1).
CNS lipids are susceptible to oxidative damage (Niki, 2014) and lipoperoxidation generates high levels of HNE, MDA (Radak et al., 2011) and F2-isoprostane (F2-IsoPS) (Milne et al., 2007; Milatovic et al., 2011; Van’t Erve et al., 2017) (Figure 1). In the CSF of patients with ALS, high levels of HNE and 3-nitrotyrosine (3-NT) have been obtained (Simpson et al., 2004; Duffy et al., 2011; Perluigi et al., 2012; Ayala et al., 2014; Santa-Cruz and Tapia, 2014).
The guanine contained in DNA is highly susceptible to oxidation and acts as a target for ROS (Lee et al., 2007; Chang et al., 2008). 8-oxo-deoxyguanosine (8-OHdG) can be considered as a specific biomarker of oxidation of the motor cortex in ALS (Cooke et al., 2000, 2002; Ihara et al., 2005; Mitsumoto et al., 2008) (Figure 1). Increased levels of 8-OHdG and isoprostanoids have been observed in the urine of subjects affected by the disease compared to the control group (Miller et al., 2014).
The evaluation of GPx activity, glutathione reductase (GR), SOD, total serum antioxidant status (TAS), MDA and 8-OHdG in ALS patients, found a significant decrease in TAS levels and an increase of 8-OHdG and MDA levels, together with significantly higher oxidized/reduced glutathione (GSSG/GSH) ratio and IL-6 and IL-8 (Blasco et al., 2017).
Antioxidants and Amyotrophic Lateral Sclerosis
The evidence implicates free radicals and OS as factors related to the pathogenesis of ALS. Interest has focused on substances with antioxidant potential such as: vitamin E, carotenes, flavonoids, resveratrol, epigallocatechin gallate, curcumin, Co-enzyme Q10, melatonin and edaravone as useful agents in the management of this disease (Table 1).
Vitamin E
Vitamin E (α-tocopherol) is one of the most studied lipophilic antioxidants due to its ability to cross the cell membrane and the protection that it provides against lipoperoxidation (Butterfield et al., 2002; Di Matteo and Esposito, 2003), ROS and RNS (Barber and Shaw, 2010) (Table 1). The studies on nutritional supplementation with this vitamin have obtained contradictory results.
This antioxidant has been associated with a delay in the clinical onset of the disease (Table 1) in transgenic mice that express mutant copies of the gene encoding SOD1 (an animal model of ALS) (Gurney et al., 1996). Measurements of the erythrocyte activity of the three main radical scavenging enzymes (SOD1, CAT, and GPx) indicated that vitamin E supplementation (300–3000 U/day) in 14 ALS patients did not affect the activity of the three enzymes (Przedborski et al., 1996). One study showed an accumulation of vitamin E in the spinal cord and increased MDA levels over the lifetime of the mouse compared to non-transgenic mice (Hall et al., 1998).
In a double-blind placebo-controlled randomized clinical trial, vitamin E did not appear to affect survival and motor function in ALS. However, after 3 months of treatment with vitamin E and riluzole, an increase in plasma GSH levels (Table 1) and a decrease in plasma thiobarbituric acid reactive species (TBARS) levels were observed, markers typically altered in the plasma of the patients with ALS (Desnuelle et al., 2001). Vitamin E was associated with a slower progression of ALS (Orrell et al., 2004) and in one study it was observed that regular use of vitamin E supplements was associated with a lower risk of dying by ALS (Ascherio et al., 2005) (Table 1).
Other similar studies did not detect significant differences between placebo and the group treated with α-tocopherol and indicated that there are insufficient results to claim that megadoses with vitamin E are effective in slowing the progression of the disease (Graf et al., 2005). Supplementation with 600 mg/day of vitamin E did not show differences with respect to the placebo group (Galbussera et al., 2006). In a case-control study with 132 patients suffering from ALS, a significant decrease in the risk of the disease (Table 1) was observed when the intake of vitamin E was higher than the average (Veldink et al., 2007). Supplementation with vitamin E over a prolonged period was associated with lower ALS rates (Wang H. et al., 2011) and a study that included 50 cases of male ALS patients concluded that in men with baseline serum α-tocopherol below the average, there was a non-significant decrease in ALS risk in those receiving α-tocopherol supplementation (50 mg/day) compared to those not receiving α-tocopherol. If baseline serum α-tocopherol was above the average, α-tocopherol supplementation had no effect on the risk of ALS. In this case, α-tocopherol supplementation did not have a significant protective effect on ALS risk (Michal-Freedman et al., 2013).
Carotenes
Carotenes are natural pigments, responsible for the orange, red (Krinsky and Johnson, 2005), yellow or green color of fruits and vegetables (Guest and Grant, 2016) with antioxidant and neutralizing properties against ROS (Fiedor et al., 2012; Nisar et al., 2015) (Table 1).
There is a beneficial association between ALS and the intake of carotenes (Okamoto et al., 2009; Nieves et al., 2016). Thus, their consumption could help the prevention and/or delay the onset of ALS (Fitzgerald et al., 2013) (Table 1) but in a case-controlled study with 77 Koreans diagnosed with ALS, it was determined that dietary intake of carotenes was negatively associated with ALS (Jin et al., 2014). A study conducted in 5 cohorts determined that a higher intake of these pigments was associated with a reduced risk of ALS and that high dietary intakes of ß-carotene and lutein were inversely associated with the risk of suffering from this disease. Astaxanthin and lycopene have shown a beneficial effect in ALS (Longecker et al., 2000; Isonaka et al., 2011) but in a study with the SOD1G93A mice model of ALS, a tomato-enriched food (rich in lycopene) did not affect disease onset and survival (Esposito et al., 2007). β-carotene could serve as a potential therapeutic molecule for treating neuroinflammation and apoptosis in ALS patients (Krishnaraj et al., 2016) (Table 1).
Flavonoids
Flavonoids are natural substances mainly present in fruits and vegetables (He et al., 2017) that have a protective effect against ROS (Cao et al., 1997), modulate the activity of different metabolic pathways (Mansuri et al., 2014) (Table 1) related to the reduction of cognitive damage and neuronal dysfunction (Vauzour et al., 2008) and suppress neuroinflammation (Solanki et al., 2015).
7,8-dihydroxyflavone (7,8-DHF) has neuroprotective and regulatory properties on neuromuscular transmission (Mantilla and Ermilov, 2012). Chronic administration of 7,8-DHF significantly improved motor deficits and enhanced lower neuronal survival in the transgenic ALS mouse model (Korkmaz et al., 2014) (Table 1).
Treatment with fisetin reduced intracellular ROS levels, motor neuron loss and improved motor activity and survival rate in three different hSOD1-related mutant models of ALS (Drosophila expressing hSOD1G85R, hSOD1G93ANSC34 cells and transgenic mice hSOD1G93A) (Wang et al., 2018) (Table 1).
Quercetin is an abundant flavonoid in the diet (onion, apple, broccoli and berries) (Esposito et al., 2002) that has been shown to reduce mitochondrial damage in various animal models of OS. Treatment with quercetin could be a therapeutic strategy for attenuating neuronal death against aluminum-induced neurodegeneration in the rat hippocampus (Sharma et al., 2016). Quercetin and its derivative, quercetin 3-β-D-glucoside (Q3BDG), could be therapeutic inhibitors of the aggregation and misfolding of SOD1 associated with ALS (Ip et al., 2017) (Table 1).
Resveratrol
Resveratrol (RES) can interact with mutant SOD1 (G93A) protein (a distinctive feature of ALS) (Julien, 2007; Srinivasan and Rajasekaran, 2018; Laudati et al., 2019) and has positive effects by up-regulating sirtuin 1 (SIRT1) expression in the mutant hSOD1-G93A-bearing motor neuron-like cell culture model of ALS (Wang J. et al., 2011) (Table 1). It delays the onset of ALS, increases the survival of spinal motor neurons, preserves the function of the lower and upper motor neuron (Mancuso et al., 2014a, b), attenuates the loss of motor neurons and improves muscle atrophy and mitochondrial function of muscle fibers in a SOD1G93A mouse model of ALS (Song et al., 2014) (Table 1). One study showed that bone marrow mesenchymal stem cells from ALS patients showed down-regulation of AMPK/SIRT1 signaling, which was recovered by treatment with RES (Yun et al., 2019) (Table 1).
Epigallocatechin Gallate
Epigallocatechin gallate (EGCG) is a catechin present in green tea (Chung et al., 2010) and to which an antineurodegenerative and antioxidant effect is attributed (Nie et al., 2002; Panickar et al., 2009), especially on the motor neurons (Koh et al., 2006) because it crosses the blood-brain barrier and modulates mitochondrial responses to OS (Bedlack et al., 2015) (Table 1). It also protects against lipoperoxidation (Table 1) in in vitro studies by exposing ROS to the phospholipids of the cell membrane bilayer (Terao et al., 1994). It prevents OS-induced death of mutant SOD1 motor neuron cells by alteration of cell survival and death signals (Koh et al., 2004). Treatment with EGCG could delay the outbreak or progression of ALS through changes in intracellular signals, increases survival signals (like PI3-K and Akt) and reduces death signals (like GKS-3ß, cytosolic cytochrome c, activated caspase-3 and cleaved poly ADP-ribose polymerase) (Levites et al., 2002; Mandel et al., 2003, 2005; Koh et al., 2006) (Table 1). Oral administration of 10 mg/kg of EGCG from a presymptomatic stage delays the onset of the disease and prolongs useful life, in addition to increasing the number of motor neurons, decreasing the activation of the microglia, reducing the concentration of NF-kB, caspase-3 and iNOS in a transgenic mouse model of ALS (Xu et al., 2006) (Table 1).
Curcumin
Curcumin is a natural and liposoluble dye obtained from turmeric (Table 1). It has neuroprotective effects and provides protection against OS (Kim et al., 2012), mitochondrial dysfunction, inflammation and protein aggregation (Ullah et al., 2017; Rakotoarisoa and Angelova, 2018; Ghasemi et al., 2019).
Curcumin activates nuclear factor erythroid 2-related factor (Nrf2) target genes in primary spinal cord astrocytes, decreases the level of intracellular ROS and attenuates oxidative damage and mitochondrial dysfunction (Jiang et al., 2011) (Table 1). It eliminates the excitability induced by TDP-43 (the major pathological protein in sporadic ALS) in a motor neuron-like cellular model of ALS (Mackenzie and Rademakers, 2008; Dong et al., 2014). Dimethoxy curcumin (DMC) could decrease mitochondrial dysfunction in mutated TDP-43 stably transfected cell lines (Lu et al., 2012) (Table 1). It binds to the pre-fibrillar aggregates of SOD1, altering its amyloidogenic pathway and decreasing cytotoxicity (Bhatia et al., 2015). Curcumin may improve survival in patients with ALS, especially those with bulbar involvement (Ahmadi et al., 2018). In a double-blind therapeutic trial, treatment with curcumin showed a decrease in ALS progression and a reduction of oxidative damage (Chico et al., 2018) (Table 1).
A study indicates that a drug delivery system based on curcumin will be proposed for the treatment of ALS (Tripodo et al., 2015) but others indicate that the use of curcumin as therapy in ALS has disadvantages: chemical instability and low oral bioavailability and water solubility rate (Rakotoarisoa and Angelova, 2018) (Table 1). Therefore, it is necessary to develop new technologies to overcome these limitations (Liu et al., 2016).
Co-enzyme Q10
Co-enzyme Q10 (CoQ10) is an endogenous antioxidant and a cofactor in the ETC (Petrov et al., 2017) involved in redox balance (Yamamoto, 2016) (Table 1). In the SOD1 transgenic mouse model of ALS, supplementation with CoQ10 extended survival by 6 days and increased brain mitochondrial concentration compared to controls (Matthews et al., 1998; Strong and Pattee, 2000; Beal, 2002) (Table 1). After comparing the plasma redox status of CoQ10 in 20 sALS patients with those in healthy age/sex-matched controls, a significant increase in the oxidized form of CoQ10 in sALS was observed (Sohmiya et al., 2005). A study conducted on 31 subjects, showed that treatment with megadoses of 3000 mg/day of CoQ10 over 8 months could improve mitochondrial dysfunction in ALS (Ferrante et al., 2005). However, one study suggested that serum CoQ10 concentrations are unrelated to the risk of ALS (Molina et al., 2000; Kaufmann et al., 2009).
Melatonin
Melatonin is an amphiphilic molecule that has been identified as a potent antioxidant therapeutic agent in neurodegenerative diseases (Polimeni et al., 2014) associated with mitochondrial dysfunction (Ganie et al., 2016) (Table 1). A study indicated that melatonin could be a candidate for neuroprotection in ALS (Jacob et al., 2002). In the SOD1G93A transgenic mouse model of ALS, high doses of melatonin administered orally delayed the progression of the disease and increased the survival rate (Weishaupt et al., 2006; Zhang et al., 2013) (Table 1). However, evidence suggests that intraperitoneal melatonin is not neuroprotective and may exacerbate neurodegeneration (Dardiotis et al., 2013). Clinical trials employing melatonin in the range of 50–100 mg/day are required before its relative merits as a neuroprotective agent are definitively established (Pandi-Perumal et al., 2013). Recent studies suggest that riluzole but not melatonin ameliorates acute motor neuron degeneration and moderately inhibits SOD1-mediated excitotoxicity induced disrupted mitochondrial calcium signaling in ALS (Jaiswal, 2017).
Edaravone
Edaravone is a low-molecular-weight antioxidant drug (Radicava®), administered intravenously (Petrov et al., 2017) which acts as a free radical scavenger (Jackson et al., 2019) (Table 1). In 2015, edaravone was approved for ALS treatment in Japan (Okada et al., 2018) and by the Food and Drug Administration of United States in 2017 (Watanabe et al., 2018).
Edaravone easily crosses the blood-brain-barrier and displays a high brain penetration capacity (Jin et al., 2017). Its amphiphilic capacity allows edaravone to scavenge both lipid and water soluble peroxyl radicals and chain-carrying lipid peroxyl radicals (Nagase et al., 2016) (Table 1).
The antioxidant mechanisms of edaravone are: enhancement of prostacyclin production, hydroxyl radical trapping and quenching of active oxygen (Sawada, 2017) (Table 1). Edaravone eliminates lipid peroxides and hydroxyl radicals during cerebral ischemia, protects nerve cells within or around the ischemic region from free radical damage (Abe et al., 2014), ameliorate OS and suppress degeneration of spinal motor neurons (Ikeda and Iwasaki, 2015). It is attributed anti-inflammatory (Bailly, 2019) and protective effects in neurons, microglia, astrocytes and oligodendrocytes (Banno et al., 2005; Miyamoto et al., 2013) (Table 1).
Investigation of the safety and efficacy of edaravone in 20 ALS patients who received this antioxidant intravenously indicated that this drug is safe and may delay the progression of functional motor disturbances by reducing OS (Yoshino and Kimura, 2006) (Table 1).
Nicotinamide Riboside and Neurodegenerative Diseases
NAD+ Role and Levels
NAD+ is a coenzyme that takes part in critical redox reactions (its reduced form is NADH) for the operation of mitochondrial metabolism (Berger et al., 2004; Yoshino et al., 2018). Is an important biological mediator due to its many essential functions for survival: redox reactions, signaling pathways, energy metabolism, mitochondrial function, calcium homeostasis, DNA repair, gene expression (Guarente, 2014; Yaku et al., 2018), brain metabolism, neurotransmission, learning, memory, axonal neuroprotection (Araki et al., 2004; Conforti et al., 2006; Gong et al., 2013) and participation in the NAD+/PARP/SIRT1 axis related to aging (Mendelsohn and Larrick, 2017) (Figure 2).
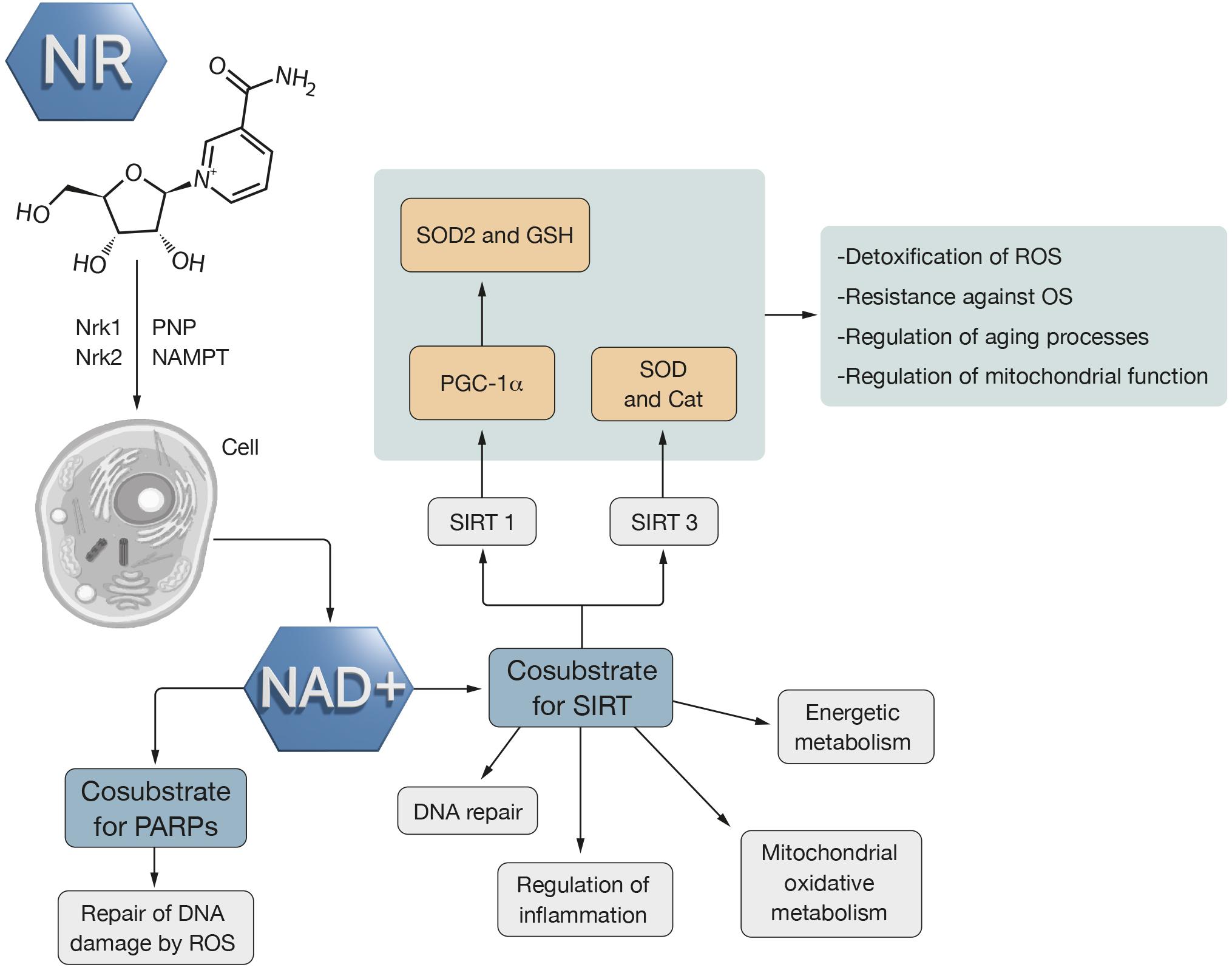
Figure 2. Nicotinamide riboside action mechanism. NR gets into the cell and there, it is converted into NAD+ through two mechanisms. One of them is Nrk1 and Nrk2 and the other is PNP and NAMPT. NAD+ is a cosubstrate of PARPS which is related to repair of DNA damage by ROS. NAD+ is also SIRT cosubstrate which is associated with energy metabolism, inflammation regulation, DNA repair and mitochondrial metabolism. The activation of SIRT increases the resistance against OS through an increase in metabolic pathways that detoxify ROS, like SOD2 and Cat. SIRT1 activates PGC-1α which involves an increase of antioxidant defense through SOD2 and GSH. SIRT3 activates SOD2 and Cat. Thus, SIRT regulates mitochondrial function and aging processes as well as is involved in ROS detoxification.
NAD+ can act as a cosubstrate for sirtuins (SIRT) (Verdin, 2015), class III histone deacetylase enzymes whose activity is dependent on NAD+ levels (Tang, 2016; Jęśko et al., 2017) and for poly(ADP-ribose) polymerases (PARPs), a family of proteins essential for repairing DNA damaged by ROS (Shen et al., 2015) (Figure 2).
Sirtuins are related to the metabolic status of cells and are key in cellular metabolism, regulation of the expression of certain genes (O’Callaghan and Vassilopoulos, 2017), energy and mitochondrial metabolism (Covington and Bajpeyi, 2016), inflammation and DNA repair (Tang, 2017). They can be found in the cytoplasm, nucleus and mitochondria (Kupis et al., 2016). In neurodegenerative diseases and in aging they organize the response to OS and damage to DNA and are related to the functionality of the respiratory machinery and the production of ROS in different tissues (Huang et al., 2010).
Activation of SIRT increases resistance to OS (Haigis and Sinclair, 2010) through an increase in metabolic pathways responsible for the detoxification of ROS (Salvatori et al., 2017) such as superoxide dismutase 2 (SOD2), isocitrate dehydrogenase 2 (IDH2) and CAT (Tennen and Chua, 2011). They improve metabolic capacity, promote mitochondrial oxidative metabolism and facilitate repair of DNA damage (Haigis and Sinclair, 2010) (Figure 2).
SIRT1 acts on the pathway of the peroxisome proliferator-activated receptor gamma coactivator 1-alpha (PGC-1α) (Alquilano et al., 2013; Tang, 2016), a fundamental regulator of energy metabolism (Bayer et al., 2017; Thirupathi and de Souza, 2017) and mitochondrial dynamics (Singh et al., 2016) associated with an increase in antioxidant defenses through SOD2 and GSH (Liang and Ward, 2006; Zhong and Xu, 2008; Wang et al., 2015) (Figure 2). Reduction of SIRT1 activity compromises oxidative metabolism and antioxidant capacity, affecting complex I of the ETC, mitochondrial function and biogenesis (Rodgers et al., 2005). This effect has been observed in aging and different pathologies: neurodegenerative diseases, metabolic disorders and cancer (Rass et al., 2007; Hou et al., 2017b; Jęśko et al., 2017). In ALS, alterations in SIRT1 levels have been determined in postmortem tissues from patients (Körner et al., 2013) and in mouse models of ALS (Han et al., 2012).
Sirtuin 3 (SIRT3) regulates mitochondrial function and the aging processes (Buck et al., 2017) by activating SOD2 and CAT (Salvatori et al., 2017) that are involved in the detoxification of ROS (Qiu et al., 2010; Kida and Goligorsky, 2016) (Figure 2).
The decrease in NAD+ levels may be secondary to a defect in its biosynthesis or to depletion (Imai and Guarente, 2014) and leads to a deficiency in the activities of SIRTs in the nucleus and mitochondria (Chang and Guarente, 2013; Gomes et al., 2013; Mendelsohn and Larrick, 2014; Frederick et al., 2016), reduces the mitochondrial unfolded protein response (Mendelsohn and Larrick, 2017), disrupts ATP biosynthesis, decreases the ability to pump calcium against the intracellular gradient (Camandola and Mattson, 2011), disrupts calcium homeostasis, increases excitotoxicity (Rzheshevsky, 2014) and has deleterious effects on muscle health (Goody and Henry, 2018).
The lower bioavailability of NAD+ levels is involved in many diseases such as neurodegenerative pathologies (Essa et al., 2013; Johnson and Imai, 2018) and affects the production of energy, lowers the levels of ATP and limits the protection of SIRT1 (Houtkooper et al., 2010; Cantó et al., 2012; Imai and Guarente, 2014), an aspect that could worsen in these diseases because of its progressive nature (Cantó et al., 2015). NAD+ levels have been shown to decrease with age, leading to low levels in the brain (Zhu et al., 2015). Several studies have demonstrated that NAD+ metabolism is involved in neuronal function and in the pathophysiology of neurodegenerative diseases (Hikosaka et al., 2019) and that NAD+ levels in tissues can produce beneficial therapeutical effects in this type of diseases (Aman et al., 2018; Kulikova et al., 2018).
The activation of metabolic pathways related to mitochondria and the production of energy through the NAD+ and SIRT1 is currently suggested as a therapeutic strategy (Yang and Sauve, 2016; Tang, 2017; Rajman et al., 2018; Zhang and Sauve, 2018).
Recovery and/or increase of NAD+ levels can protect skeletal muscle from progressive deterioration (Fletcher et al., 2017), reverse the damage to cerebral energy metabolism, increase the protection against OS (López-Otín et al., 2013; Brown et al., 2014; Verdin, 2015), promote the activity of SIRTs and proteins involved in mitochondrial function (Bonkowski and Sinclair, 2016), confers resistance against peroxide toxicity, decreases mitochondrial ROS (Harlan et al., 2016), protection against neurodegeneration (Sasaki et al., 2006) and upkeep of dependent enzymes that are involved in synaptic plasticity and neuronal stress resistance (Lautrup et al., 2019).
Therefore, the repletion of NAD+ levels using precursors can ameliorate this age-associated functional defects (Imai and Guarente, 2014), helping to reverse the pathogenic processes characteristic of neurodegenerative diseases (Zhang et al., 2016).
Precursors of NAD+
The precursors of NAD+ include nicotinamide mononucleotide (NMN), nicotinamide (NAM), nicotinic acid (NA) and nicotinamide riboside (NR) (Harlan et al., 2016). Most of the NAD+ precursors are more stable and have higher ability to enter neurons than NAD+ (Sasaki et al., 2006). NMN and NR are the most used and both have shown an increase in NAD+ levels in the cell (Nikiforov et al., 2011) (Table 2). Most evidence concludes that NR has a greater ability to stimulate a significant increase in NAD+ levels (Cantó et al., 2012) and advantages over the use of other precursors (Table 2).
NA prevents Pellagra (Prousky et al., 2011) and has positive effects on the regulation of lipids (total cholesterol, triglycerides and LDL) (Guille et al., 2008) (Tabla 2). However, produces cutaneous flushing and does not work as NAD+ precursor in the majority of cells (Davidson, 2008) (Table 2). One of the most important advantages of NR over NA is that supports neuronal NAD+ synthesis (Bogan and Brenner, 2008) and has action on several types of cultured mammalian cells, including mouse and human cells (Yang H. et al., 2007) (Table 2).
There is no consensus on how NMN is transported to the cell (Grozio et al., 2019; Schmidt and Brenner, 2019) (Table 2). However, NR is five times more effective than NMN in increasing intracellular NAD+ levels in skeletal muscles (Oakey et al., 2018) (Table 2).
After comparing NR with NA and NAM in mice, NR showed a significant higher peak of NAD+ concentration and a significant increase of intermediate NAD+ precursors including NMN, NA mononucleotide and NA adenine dinucleotide (Trammell et al., 2016) (Table 2). NR has a different time course compared to NAM and NA and produces more NAD+ than the other precursors in equivalent doses (Trammell et al., 2016). NR increases the ratio of NAD+/NADH more significantly compared NMN, NAM and NA and this can contribute to improving the oxidative capacity of mitochondria (Cantó et al., 2012) and to decrease the rate of oxidative damage against DNA and mitochondrial stress (Ramamoorthy et al., 2012; Srivastava, 2016; Dolopikou et al., 2019) (Table 2).
NR is a nucleoside that incorporates a nicotinamide and a ribose (Belenky et al., 2007; Chi and Sauve, 2013) group. It is a trace nutrient known as vitamin B3 (Lanska, 2012), available in certain foods (dairy products, fish, eggs, and vegetables) (Minto et al., 2017), nutritional supplements and fortified foods (Colbourne et al., 2013). Its effects are associated with energy metabolism and neuroprotection (Chi and Sauve, 2013). When the NR enters the cells it is converted to NAD+ by at least two types of metabolic pathway. The first requires the participation of the nicotinamide riboside kinases (NRKs) (Chi and Sauve, 2013) in two of its isoforms (NRK1 and NRK2) (Bieganowski and Brenner, 2004) and the second requires the action of purine nucleoside phosphorylase (PNP) and nicotinamide phosphoribosyltransferase (NAMPT) (Burgos et al., 2013) (Figure 2).
The conversion of NR into NAD+ has been observed in animal tissues of muscle and brain (Chi and Sauve, 2013) and treatment by exogenous supplementation of NR is able to increase intracellular concentrations of NAD+ and promote its beneficial effects (Braidy et al., 2008) (Figure 2). NR enhances protection against aging and related diseases, with positive results on longevity in multiple animal models (Mouchiroud et al., 2013; Poljsak and Milisav, 2016) and has been recently reported that NR protects against excitotoxicity induced axonal degeneration (Vaur et al., 2017). The increase of NAD+ levels by supplementation with precursors such as NR improves mitochondrial and muscular function and the function of neuronal cells in mice (Mendelsohn and Larrick, 2017). A study performed in mice supplemented nutritionally with 400 mg/kg/day of synthetic NR showed an increase in NAD+ levels in muscle and liver tissue (Cantó et al., 2012). As NR increases the NAD+/NADH ratio, it could be related to an improvement of the oxidative capacity of the mitochondrial pathway and could be a therapeutic strategy of interest in those diseases that are associated with mitochondrial dysfunction and OS such as neurodegenerative diseases (Cantó et al., 2012). Improvement of NAD+ levels provides greater mitochondrial resistance against OS (Yang T. et al., 2007) and prevents cell death due to the protective function of SIRT3 (Hafner et al., 2010), which stimulates SOD, responsible for enhancing the “detoxification” of ROS (Chen et al., 2011) (Figure 2).
Supplementation with NR is safe in mice and humans and has minimal toxicity, high bioavailability and high capacity to cross the blood-brain barrier (Trammell et al., 2016; Airhart et al., 2017; Martens et al., 2018) (Table 2).
Nicotinamide Riboside
Neurodegenerative diseases are associated with a progressive decrease in muscle mass and strength and an increase in their weakness (González et al., 2017; Van Damme et al., 2017; Dahlqvist et al., 2019). Muscle contractility dysfunction precedes the loss of motor unit connectivity in the SOD1G93A mouse model of ALS (Wier et al., 2019) with neurodegeneration being one of the determinant risk factors for muscle quality (Di Pietro et al., 2017; Moon et al., 2018). Treatment with NR improves muscle function in aged mice (Cantó et al., 2012) and has benefits on muscle strength and skeletal muscle resistance in knock out mice that have specific elimination of NAMPT and a decrease of 85% in the intramuscular content of NAD+ (Frederick et al., 2016). This would indicate that the maintenance of NAD+ homeostasis is critical for muscle mass and its contractile function (Mendelsohn and Larrick, 2014; Frederick et al., 2016). NR induces rejuvenation in muscle and brain stem cells (Ryu et al., 2016) and an increase in neurogenesis and muscle function (Zhang et al., 2016). NAD+ is a fundamental modulator of muscle senescence and after treatment with NR, there is an acceleration of muscle regeneration in both young and old mice and an improvement in running times, maximum distances, grip strength of the extremities of aged mice and increased expression of cell cycle genes (Zhang et al., 2016).
Levels of PGC-1α are decreased in Alzheimer’s disease (AD) and this is related to the formation of ß-amyloid plaques and greater deposition of this substance (main characteristics of AD) (Wu et al., 2006; Quin et al., 2009). Studies conducted in animal models of AD established a relationship between the levels of NAD+ and a decrease in the toxicity of the amyloid substance (Kim et al., 2007; Quin et al., 2009). For this reason, the therapeutic strategy based on nutritional supplementation with NR could be of interest in AD cases to stop the onset and progression of the dementia (Rodgers et al., 2005) and to improve cognitive function and synaptic plasticity by promoting the function of PGC-1α as demonstrated in Tg2576 mice (mouse model of ALS) (Gong et al., 2013). After analyzing the effect of NR on the physiopathology and cellular mechanisms in the 3xTgAD/Polß± mouse model (mice deficient in DNA repair that exacerbates the main characteristics of AD in humans) it was observed that supplementation with NR significantly increased the NAD+/NADH ratio, reduced neuroinflammation, apoptosis and damage to DNA, increased neurogenesis, restored synaptic plasticity, improved learning capacity and reversed memory deficits (Hou et al., 2017a). Treatment with NR improves cognition in transgenic mice with AD by reducing the phosphorylation of the Tau protein (pTau) (a form that accumulates in the brain of AD patients and is the hallmark of the disease) (Green et al., 2008; Rüb et al., 2017; Nizynski et al., 2017). In the case of PD, the repletion of NAD+ through the use of NR prevents aging related to the loss of dopaminergic neurons and the reduction of motor function in Drosophila fly model of PD (Sidransky et al., 2009). NR improves mitochondrial function in PD neurons, increases the markers of mitochondrial biogenesis, significantly decreases the production of mtROS (mitochondrial ROS), reduces mitochondrial membrane potential and increases the levels of NAD+ and NADH (Schöndorf et al., 2018).
Axonal degeneration occurs in most neurodegenerative diseases (AD, PD, ALS) (Raff et al., 2002; Coleman, 2005). The increase in NAD+ synthesis through the use of precursors such as NR promotes axonal protection (Wang et al., 2005; Sasaki et al., 2006). Improvement of the NAD+ recovery pathway reverses the toxicity of primary astrocytes expressing the SOD1 mutation related to ALS, decreasing the mitochondrial production of ROS and reversing the neurotoxic effects (Harlan et al., 2016). Excitotoxicity is a process that takes place in most neurodegenerative disorders like ALS and is related to strong NAD+ depletion in neurons. NR protects against excitotoxicity-induced axonal generation (Vaur et al., 2017).
In Duchenne’s muscular dystrophy disease, supplementation with NR reverses progressive wear and improves resistance in skeletal muscle NAMPT in the knock out mouse model (Ryu et al., 2016).
Pterostilbene and Neurodegenerative Diseases
Polyphenols are organic metabolites present in plants (Cory et al., 2018) and widely distributed in a variety of dietary sources (Aherne and O’Brien, 2002; Kim et al., 2009): fruits, vegetables, legumes, whole grains, seeds, nuts, extra virgin olive oil, red wine, coffee, tea, chocolate, herbs and spices (Rusconi and Conti, 2010; Fu et al., 2011; Regueiro et al., 2014; Vallverdú-Queralt et al., 2014, 2015; Talhaoui et al., 2016). Despite their zero energy contribution (Ruiz et al., 2009) they act as bioactive dietary components associated with multiple positive health effects (An-Na et al., 2014; Ganesan and Xu, 2017; Szwajgier et al., 2017; Renaud and Martinoli, 2019) due to their regulating action on metabolism, chronic diseases and cell proliferation (Tressera-Rimbau et al., 2017). Polyphenols have a chemical structure with properties that make them the compounds with the greatest antioxidant action: o-diphenolic group, 2-3 double bond conjugated with the 4-oxo function and hydroxyl groups (OH) in positions 3 and 5 (Ruiz et al., 2009). They have antioxidant (Hua and Rong, 2016; Tarique et al., 2016), anti-inflammatory (Oliviero et al., 2018), anticarcinogenic (Niedzwiecki et al., 2016), antiallergic (Juríková et al., 2015), antibiotic (Xie et al., 2017), and immunomodulatory (Nour et al., 2018) properties.
Polyphenols can be a useful therapeutic strategy in pathologies that present OS such as neurodegenerative diseases (Bhullar and Vasantha, 2013; Raskin et al., 2015; Reinisalo et al., 2015; Carvalho et al., 2018), cancer and cardiovascular diseases (Crozier et al., 2009; Bulotta et al., 2014; Lamuela-Raventós et al., 2014). Taking into account that nutrition is a factor that modulates processes such as cognition or progression of CNS diseases (Gustafson et al., 2015; Huhn et al., 2015), polyphenols have recently been associated with: prevention, repair of oxidative damage (Lange and Li, 2018), synaptic plasticity, neuronal signaling and autophagy (Miller and Shukitt-Hale, 2012; Poulose et al., 2014).
Among the polyphenols with important pharmaceutical activity are the stilbenes (Dvorakova and Landa, 2017), a group of non-flavonoid phytochemicals of polyphenolic structure with a 1,2-diphenylethylene core, which are produced naturally in plants via the phenylpropanoid pathway (Sirerol et al., 2016) to protect the plant from fungal infection and toxins (Akinwumi et al., 2018). There is a wide variety of forms because of a common structure to which various substituents can be added in different positions and they have an acidic and amphiphilic character (Neves et al., 2012). Although the trans isomer is the most common form of presentation (since it is the most stable), it can also be found in the cis isomer (Rivière et al., 2012). The following properties have been attributed to them: antioxidant, anti-inflammatory, neuroprotective, cardioprotective, anti-carcinogenic (Lange and Li, 2018), hypolipidemic and anti-diabetic (Voloshyna et al., 2013; Szkudelski and Szkudelska, 2015). They have great utility potential in the field of neurodegenerative diseases. They reduce the formation of amyloid plaques in the brain, decrease the production of ROS and could be of interest in other situations such as: ischemia-reperfusion injury, atherosclerosis, diabetes, cancer, obesity, platelet aggregation, blood pressure, depigmentation and cardiomyocyte and cardiac hypertrophy (Thandapilly et al., 2011; Gomez-Zorita et al., 2013; Zordoky et al., 2015; Guo et al., 2016; Akinwumi et al., 2018).
One of the most studied stilbenes has been Resveratrol (RES) (3,5,4’-trihydroxy-trans-stilbene), due to its benefits in cardiovascular health (Zordoky et al., 2015; Bonnefont-Rousselot, 2016; Dyck et al., 2019). However, recent studies focus their interest on pterostilbene (PTER) (trans-3,5-dimethoxy-4hydroxystilbene), a dimethylated natural stilbene (Albassam and Frye, 2019) with 1 hydroxyl group and 2 methoxy groups (Cichocki et al., 2008; Estrela et al., 2013; Traversi et al., 2016), which provides greater oral bioavailability, half-life, lipophilicity and higher permeability to targeted tissue (Kapetanovic et al., 2011; Yeo et al., 2013) with respect to RES (3 hydroxyl groups). In addition, PTER is less susceptible to phase II liver metabolism (Zhou et al., 2016). These particular characteristics improve its biological potential (Yeo et al., 2013) and its high bioavailability in vivo is an advantage over RES (Chiou et al., 2011; Carey et al., 2013; Lin et al., 2016). PTER presents a bioavailability of 80% compared to 20% for RES and plasma levels of PTER and PTER sulfate were significantly higher than plasma levels of RES and RES sulfate in a mouse model (Kapetanovic et al., 2011). After administration of PTER and RES at the same dose to male and female mice for 8 weeks, PTER reached higher concentrations in the serum and in the brain than RES (Chang et al., 2012).
The high lipophilicity of PTER allows it to easily cross the blood-brain barrier (Temsamani et al., 2015), resulting in greater neuroprotection than RES (Choo et al., 2014). In AD, PTER presents superior neuroprotection than RES (Chang et al., 2012) and is the stilbene with the highest inhibitory potential for 5-lipoxygenase (5-LOX) (Kutil et al., 2015), decreasing the levels of lipid and protein oxidation (Czpaski et al., 2012). It is a more potent anticancer and anti-inflammatory agent than RES (Chiou et al., 2011) and it distributes widely among the main target organs (brain, liver, kidney, heart and lung) for what seems to be a promising (Choo et al., 2014) and safe (Riche et al., 2013) therapeutic strategy. A study in male and female Swiss mice examined the sub-chronic toxicity and the possible adverse effects of PTER and concluded that, even with the highest dose administered, PTER was not toxic (Ruiz et al., 2009).
Molecular Mechanisms of PTER
The physiological activities of PTER include: antioxidant and anti-inflammatory activity, ability to restore intracellular calcium and cognitive function (Li Y. R. et al., 2018) (Figure 3).
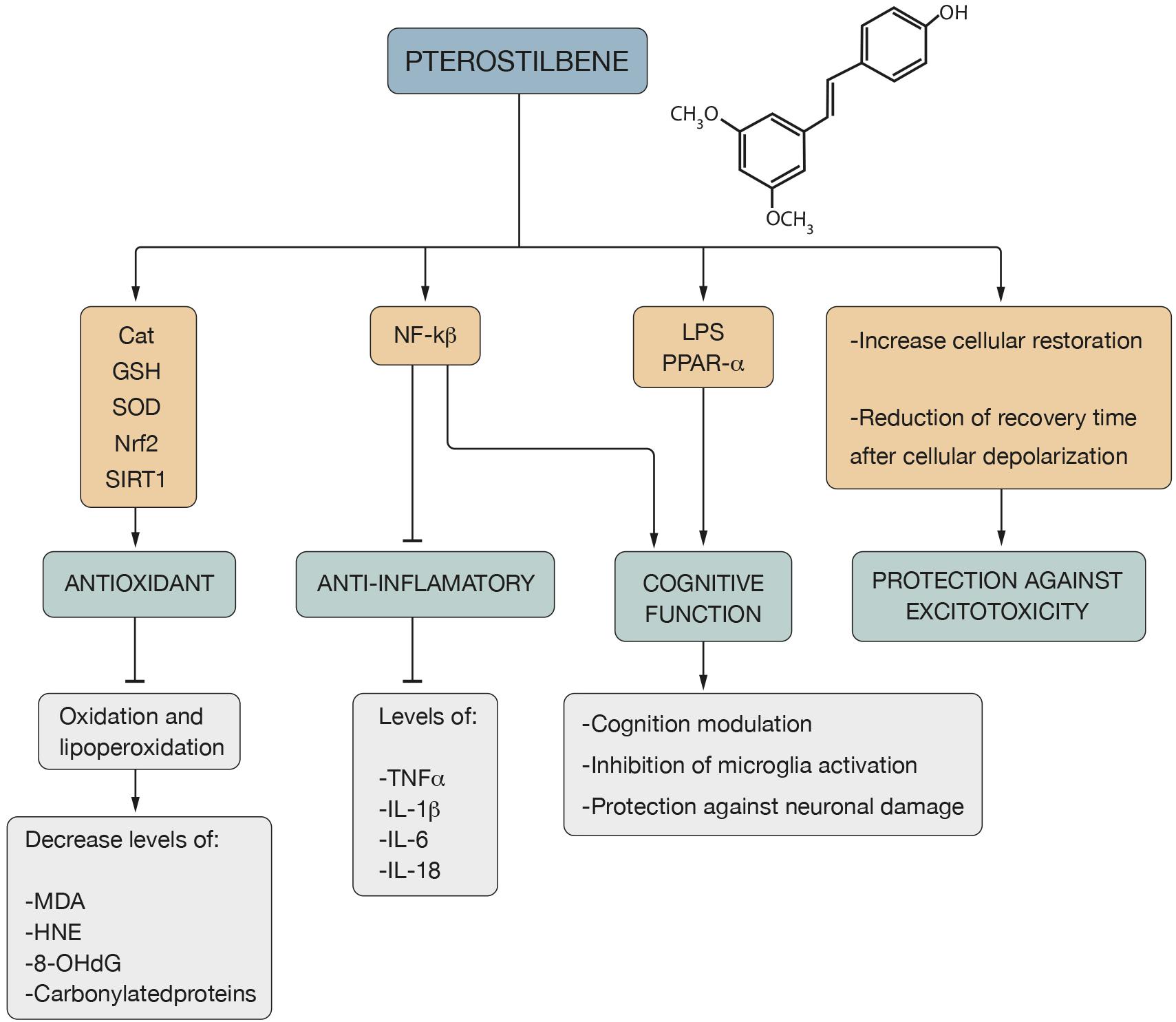
Figure 3. Pterostilbene properties and action mechanism. The antioxidant mechanism of Pter is associated with Cat, GSH, SOD, Nrf2 and SIRT1 pathways, which leads to an inhibition of the oxidation and lipoperoxidation processes, decreasing the levels of MDA, HNE, 8-OHdG and carbonilated proteins. Its action on NF-kß involves an anti-inflammatory effect due to the decrease in the levels of TNF-α and interleukins such as IL-1β, IL-6 and IL-18. Its role on NF-kß, LPS and PPAR-α mediates the cognitive function of Pter, which is expressed through cognition modulation, inhibition of microglia activation and protection against neuronal damage. In addition, Pter presents protection against excitotoxicity due to the increase in cellular restoration and the reduction of the recovery time after cellular depolarization.
Antioxidant Activity
PTER stands out for its antioxidant action against numerous types of free radicals: HO, H2O2 (Castellani et al., 2008; Mikstacka et al., 2010; Acharya and Ghaskadbi, 2013) and NO∙ (Pan et al., 2008; Zhang et al., 2012). It is able to neutralize metal-induced radicals (Rossi et al., 2013; Saw et al., 2014) and to inhibit oxidation and lipoperoxidation processes (Rimando et al., 2002) causing a decrease in carbonylated proteins and oxidation by-products: MDA, HNE and 8-OHdG (Li Y. R. et al., 2018) (Figure 3). The administration of PTER improves the antioxidant defenses of the brain by raising the levels of CAT, GSH and the activity of SOD (Naik et al., 2017) (Figure 3). In addition, diet supplemented with PTER increases the expression of SOD2 (Ding et al., 2007). Pre-treatment with PTER has a potent antioxidant function that increases SOD activity in hypoxic-ischemic brain injury in the P7 rat model (Li D. et al., 2016). Treatment with PTER reduces glutamate-induced OS injury by reducing ROS and increasing the function of SOD and GSH by activating the Nrf2 signaling pathway in neuronal cells (Wang et al., 2016), a target factor for the prevention of diseases related to aging such as neurodegenerative diseases (Bhakkiyalakshmi et al., 2016; Li Y. R. et al., 2018) (Figure 3).
The potent antioxidant mechanism of PTER is associated with SIRT1 signaling activation (Li et al., 2015; Guo et al., 2016; Liu et al., 2017) leading to the attenuation of the skeletal muscle OS injury and mitochondrial dysfunction induced by ischemia-reperfusion injury in male Sprague-Dawley rats (Cheng et al., 2016). PTER acts on Nrf2 (Saw et al., 2014; Zhang et al., 2014; Xue et al., 2017) and attenuates high glucose-induced central nervous system injury in vitro through the activation of Nrf2 signaling, displaying protective effects against mitochondrial dysfunction-derived OS (Yang et al., 2017).
Anti-inflammatory Activity
PTER has been recognized as an anti-inflammatory agent (Remsberg et al., 2008; Poulose et al., 2015; Kosuru et al., 2016) able to protect neurons against neuroinflammation due to the inhibition of ROS production (Shin et al., 2010). It acts on NF-kB and suppresses the action of various proinflammatory cytokines: TNF-α, IL-1β, IL-6 and IL-18 (Cichocki et al., 2008; Paul et al., 2009; Hou et al., 2014; El-Sayed et al., 2015) (Figure 3). PTER inhibits amyloid-β-induced neuroinflammation in microglia by inactivating the NLRP3/caspase-1 inflammasome pathway and reduces the secretion levels of IL-6, IL-1β and TNF-α, thereby attenuating the neuroinflammatory response, which would indicate that it could be a useful therapeutic strategy in neurodegenerative diseases (Li Q. et al., 2018; Seo et al., 2018).
Ability to Restore Intracellular Calcium
PTER is able to increase the capacity of intracellular calcium restoration by reducing recovery time after cell depolarization (Joseph et al., 2008). It protects against excitotoxicity, an aspect that would be interesting since it has been observed that the increase in intracellular ROS levels is related to damage in the cell membrane, impaired calcium homeostasis (Thibault et al., 2007; Joseph et al., 2011) and increased excitotoxicity (Rzheshevsky, 2014) (Figure 3). In the Sprague-Dawley rat model, PTER improves cholinergic transmission due to the decrease in the activity of acetylcholinesterase and increases the action of ATPases (Na+, K+, Ca2+, and Mg2+), which is indicative of the maintenance of the cell membrane potential (Naik et al., 2017).
Cognitive Function
After determining if PTER was effective in reversing the effects of aging in old Fischer rats, it was concluded that memory functioning was related to PTER levels in the hippocampus and that a diet supplemented with this antioxidant is effective in reversing deficits in cognitive behavior (Joseph et al., 2008). PTER attenuated lipopolysaccharide (LPS) induced learning and memory impairment, which is associated with an inhibition of the activation of microglia and therefore a protective effect against neuronal damage (Hou et al., 2014) (Figure 3). It reduces memory loss in the intracerebroventricular administered streptozotocin-induced memory decline in Sprague–Dawley rats (Naik et al., 2017) and mediates neuroprotection against oxidative toxicity via the estrogen receptor signaling pathway in human neuronal SH-SY5Y cells (Song et al., 2015).
In AD, supplementation with PTER reduces the phosphorylation of pTau (Porquet et al., 2013). The derivatives of PTER showed antioxidant and inhibitory activity against the aggregation of β-amyloid plaques, as well as cholinesterase inhibition, which would be useful in the treatment of patients with AD (Li Y. et al., 2016) and vascular dementia (Lange and Li, 2018).
PTER is a modulator of cognition and the OS due to the increased expression of peroxisome proliferator activator receptor alpha (PPAR-α) (Chang et al., 2012) (Figure 3). After comparing the effectiveness of dietary supplementation with RES and PTER in the improvement of functional deficits characteristic of AD in the SAMP8 mouse model (a model of accelerated aging that is increasingly being validated as a model of sporadic and age-related AD), it was concluded that PTER and not RES, is able to modulate the markers of cellular stress and inflammation, causing an upregulation of PPAR-α (Chang et al., 2012) and a suppression of the activation of NF-kB (Inoue et al., 2003), which is a conservative factor against the loss of cognitive function (Jeong et al., 2012; Hou et al., 2014) (Figure 3).
Due to the activation of metabolic pathways related to OS protection, inflammation, regulation of excitotoxicity and preservation of neuronal functions, PTER is a protective factor against neurodegenerative diseases (Poulose et al., 2015).
Conclusion
OS is involved in neuroinflammation, development and progression of ALS. The complex interaction between all the factors does not allow definitive determination if OS is a primary cause or if it is a secondary effect to the propagation of damage in the nervous cells. However, a clear relationship has been established between OS, mitochondrial dysfunction and neuroinflammation, aspects that promote a “vicious cycle” that causes a decrease in the capacity of ATP biosynthesis and an increase in ROS levels.
Currently, there is no cure for ALS but the use of different antioxidant substances has been proposed as a possible therapeutic strategy, the purpose of which is to increase the body’s antioxidant defenses and maintain the redox balance. However, there are cases in which the use of these antioxidants has disadvantages or requires a higher number of studies since few results are available or these are contradictory, inconclusive or scarcely significant at the statistical level. Taking into account the pathogenic mechanisms of ALS, the new therapeutic strategies have as the main goal to activate the metabolic pathways related to the mitochondria, production of energy and increase the antioxidant defense levels.
NAD+ acts on energy metabolism, mitochondrial function and is key in brain metabolism, aspects involved in the pathogenesis of ALS. Restoration of NAD+ levels by administrating the precursor NR provides greater mitochondrial resistance against impairment of redox balance and, therefore, could play a key role in those diseases that are associated with mitochondrial dysfunction and OS.
PTER has great biological potential due to its ability to activate metabolic pathways related to protection against OS, mitochondrial dysfunction, inflammation, intracellular calcium restoration and cognitive function, thus resulting in a neuroprotective function against the pathogenic mechanisms of ALS.
In a randomized, double-blind, placebo-controlled study in humans, it was determined that repeated doses of a combination therapy with NR and PTER increased NAD+ levels safely and effectively (Dellinger et al., 2017). Treatment with NR and PTER is effective and safe and therefore it could be a promising therapeutic strategy in ALS, due to its action on the pathogenesis of this disease.
Author Contributions
SC-J and MM wrote the manuscript. SC-J, MM, and ED conceived and designed the figures. CB, JR, and ED reviewed the manuscript.
Conflict of Interest
The authors declare that the research was conducted in the absence of any commercial or financial relationships that could be construed as a potential conflict of interest.
Acknowledgments
We wish to thank Universidad Católica de Valencia “San Vicente Mártir” for its financial help (Grant: 2019-802-001) in the revision and edition of the language in the manuscript and Professor José María Lajara from Universidad Católica de Valencia “San Vicente Mártir”, for his technical assistance in the design of the figures.
Abbreviations
3-NT, 3-nitrotyrosine; 5-LOX, 5-lipoxygenase; 7,8-DHF, 7,8-dihydroxyflavone; 8-OHdG, 8-oxo-deoxyguanosine; AD, Alzheimer’s disease; ALS, amyotrophic lateral sclerosis; CAT, catalase; CNS, central nervous system; CoQ10, Co-enzyme Q10; COX, cyclooxygenase; CSF, cerebrospinal fluid; DMC, dimethoxy curcumin; EGCG, epigallocatechin gallate; ETC, electron transport chain; F2-IsoPS, F2-isoprostane; fALS, familial amyotrophic lateral sclerosis; GPx, Glutathione peroxidase; GR, Glutathione reductase; GSH, Reduced glutathione; GSK-3ß, Glycogen synthase kinase 3 beta; GSSG, Glutathione disulfide; H2O2, Hydrogen peroxide; HD, Huntington’s disease; HNE, 4-hydroxy-2-nonenal; IDH2, Isocitrate dehydrogenase; IL-18, Interleukin-18; IL-1ß, Interleukin 1ß; IL-6, Interleukin-6; IL-8, Interleukin-8; iNOS, nitric oxide synthase; LDL, low-density lipoprotein; LMN, lower motor neurons; LOX, lipoxygenase; LPS, lipopolysaccharide; MDA, malondialdehyde; MPO, myeloperoxidase; MS, multiple sclerosis; mtDNA, mitochondrial DNA; mtROS, mitochondrial reactive oxygen species; NA, nicotinic acid; NAD+, nicotinamide adenine dinucleotide; NADPH, nicotinamide adenine dinucleotide phosphate; NAM, nicotinamide; NAMPT, nicotinamide phosphoribosyltransferase; NF-kB, nuclear factor kappa-light-chain-enhancer of activated B cells; NMN, nicotinamide mononucleotide; NO, nitric oxide; NO–, nitroxyl anion; NO+, nitrosonium cation; NO ∙, nitric oxide radical; NOS, nitric oxide synthase; NR, nicotinamide riboside; Nrf2, nuclear factor erythroid 2-related factor; NRK1, nicotinamide riboside kinase 1; NRK2, nicotinamide riboside kinase 2; NRKs, nicotinamide riboside kinases; O2, singlet oxygen; O, superoxide anion radical; OH, hydroxyl group; OH ∙, hydroxyl radical; ONOO–, peroxynitrite anion; OS, oxidative stress; PARPs, poly(ADP-ribose) polymerase; PD, Parkinson’s disease; PGC-1 α, Peroxisome proliferator-activated receptor gamma coactivator 1-alpha; PNP, purine nucleoside phosphorylase; PPAR- α, peroxisome proliferator activator receptor alpha; pTau, Tau protein; PTER, Pterostilbene (trans-3,5-dimethoxy-4 hydroxystilbene); PUFAs, polyunsaturated fatty acids; Q3BDG, Quercetin 3-ß-D-glucoside; RCS, reactive species of copper; RES, Resveratrol (3,5,4 ′ -trihydroxy-trans-stilbene); RIS, reactive iron species; RNA, ribonucleic acid; RNS, reactive nitrogen species; RONS, reactive oxygen and nitrogen species; ROS, reactive oxygen species; SA, spinocerebellar ataxia; sALS, sporadic amyotrophic lateral sclerosis; SIRT, sirtuin; SIRT1, sirtuin 1; SIRT3, sirtuin 3; SOD, superoxide dismutase; SOD1, superoxide dismutase 1; SOD2, superoxide dismutase 2; TAS, total antioxidant status; TBARS, thiobarbituric acid reactive species; TNF- α, tumor necrosis factor alpha; UMN, upper motor neurons; XO, xanthine oxidase.
References
Abe, K., Aoki, M., Tsuji, S., Itoyama, Y., Sobue, G., Togo, M., et al. (2017). Safety and efficacy of edaravone in well defined patients with amyotrophic lateral sclerosis: a randomized, double-blind, placebo-controlled trial. Lancet Neurol. 16, 505–512. doi: 10.1016/S1474-4422(17)30115-1
Abe, K., Itoyama, Y., Sobue, G., Tsuji, S., Aoki, M., Doyu, M., et al. (2014). Confirmatory double-blind, parallel-group, placebo-controlled study of efficacy and safety of edaravone (MCI-186) in Amyotrophic lateral sclerosis patients. Amyotroph. Lateral Scler. Frontotemporal. Degener. 15, 610–617. doi: 10.3109/21678421.2014.959024
Acharya, J. D., and Ghaskadbi, S. S. (2013). Protective effect of pterostilbene against free radical mediated oxidative damage. BMC Complement. Altern. Med. 13:238. doi: 10.1186/1472-6882-13-238
Agar, J., and Durham, H. (2003). Relevance of oxidative injury in the pathogenesis of motor neuron diseases. Amyotroph. Lateral Scler. Other Motor Neuron Disord. 4, 232–242. doi: 10.1080/14660820310011278
Aherne, S. A., and O’Brien, N. M. (2002). Dietary flavonols: chemistry, food content, and metabolism. Nutrition 18, 75–81.
Ahmadi, M., Agah, E., Nafissi, S., Jaafari, M. R., Harirchian, M. H., Sarraf, P., et al. (2018). Safety and efficacy of nanocurcumin as add-on therapy to riluzole in patients with amyotrophic lateral sclerosis: a pilot randomized clinical trial. Neurotherapeutics 15, 430–438. doi: 10.1007/s13311-018-0606-7
Ahtoniemi, T., Jaronen, M., Keksa-Goldstein, V., Goldsteins, G., and Koistiaho, J. (2008). Mutant SOD1 from spinal cord of G93A rats is destabilized and binds to inner mitochondrial membrane. Neurobiol. Dis. 32, 479–485. doi: 10.1016/j.nbd.2008.08.010
Airhart, S. E., Shireman, L. M., Risler, L. J., Anderson, G. D., Nagana-Gowda, G. A., Raftery, D., et al. (2017). An open-label, non-randomized study of the pharmacokinetics of the nutritional supplement nicotinamide riboside (NR) and its effects on blood NAD+ levels in healthy volunteers. PLoS One 12:e0186459. doi: 10.1371/journal.pone.0186459
Akinwumi, B. C., Bordu, K. A. M., and Anderson, H. D. (2018). Biological activities of stilbenoids. Int. J. Mol. Sci. 19:E792. doi: 10.3390/ijms19030792
Albarracín, S. L., Stab, B., Casas, Z., Sutachan, J. J., Samudio, I., González, J., et al. (2012). Effects of natural antioxidants in neurodegenerative diseases. Nutr. Neurosci. 15, 1–9. doi: 10.1179/1476830511Y.0000000028
Albassam, A. A., and Frye, R. F. (2019). Effect os pterostilbene on in vitro drug metabolizing enzyme activity. Saudi Pharm. J. 27, 406–412. doi: 10.1016/j.jsps.2019.01.001
Al-Chalabi, A., and Hardiman, O. (2013). The epidemiology of ALS: a conspiracy of genes, environment and time. Nat. Rev. Neurol. 9, 617–628. doi: 10.1038/nrneurol.2013.203
Al-Chalabi, A., Kwak, S., Mehler, M., Rouleau, G., Siddique, T., Strong, M., et al. (2013). Genetic and epigenetic studies of amyotrophic lateral sclerosis. Amyotroph. Lateral Scler. Frontotemporal. Degener. 14, 44–52. doi: 10.3109/21678421.2013.778571
Al-Gubory, K. H., Garrel, C., Faure, P., and Sugino, N. (2012). Roles of antioxidant enzymes in corpus luteum rescue from reactive oxygen species-induced oxidative stress. Reprod. Biomed. 25, 551–560. doi: 10.1016/j.rbmo.2012.08.004
Allen, C. L., and Bayraktunan, U. (2009). Oxidative stress and its role in the pathogenesis of ischaemic stroke. Int. J. Stroke 4, 461–470. doi: 10.1111/j.1747-4949.2009.00387.x
Alquilano, K., Baldelli, S., Paglieli, B., and Ciriolo, M. R. (2013). Extranuclear localization of SIRT1 and PGC-1α: an insight into possible roles in diseases associated with mitochondrial dysfunction. Curr. Mol. Med. 13, 140–154.
Aman, Y., Qiu, Y., Tao, J., and Fang, E. F. (2018). Therapeutic potential of boosting NAD+ in aging and age-related diseases. Transl. Med. Aging 2, 30–37. doi: 10.1016/j.tma.2018.08.003
Andersen, P. M. (2006). Amyotrophic lateral sclerosis associated with mutations in the CuZn superoxide dismutase gene. Curr. Neurol. Neurosci. Rep. 6, 37–46.
Andersen, P. M., and Al-Chalabi, A. (2011). Clinical genetics of amyotrophic lateral sclerosis: what do we really know? Nat. Rev. Neurol. 7, 603–615. doi: 10.1038/nrneurol.2011.150
Angelova, P. R., and Abramov, A. Y. (2018). Role of mitochondrial ROS in the brain: from physiology to neurodegeneration. FEBS. Lett. 592, 692–702. doi: 10.1002/1873-3468.12964
An-Na, L., Sha, L., Yu-Jie, Z., Xiang-Rong, X., Yu-Ming, C., and Hua-Bin, L. (2014). Resources and biological activities of natural polyphenols. Nutrients 6, 6020–6047. doi: 10.3390/nu6126020
Araki, T., Sasaki, Y., and Milbrandt, T. (2004). Increased nuclear NAD biosynthesis and SIRT1 activation prevent axonal degeneration. Science 305, 1010–1013. doi: 10.1126/science.1098014
Arun, S., Liu, L., and Donmez, G. (2016). Mitochondrial biology and neurological diseases. Curr. Neuropharmacol. 14, 143–154.
Ascherio, A., Weisskopf, M. G., O’reilly, E. J., Jacobs, E. J., McCullough, M. L., Calle, E. E., et al. (2005). Vitamin E intake and risk of amyotrophic lateral sclerosis. Ann. Neurol. 57, 104–110. doi: 10.1002/ana.20316
Atashi, F., Modarressi, A., and Pepper, M. S. (2015). The role of reactive oxygen species in mesenchymal stem cell adipogenic and osteogenic di?erentiation: a review. Stem Cells Dev. 24, 1150–1163. doi: 10.1089/scd.2014.0484
Ayala, A., Muñoz, M. F., and Argüelles, S. (2014). Lipid peroxidation: production, metabolism, and signaling mechanisms of malondialdehyde and 4-Hydroxy-2 nonenal. Oxid. Med. Cell. Longev. 2014:360438. doi: 10.1155/2014/360438
Babu, G. N., Kumar, A., Chandra, R., Puri, S. K., Singh, R. L., Kalita, J., et al. (2008). Oxidant-antioxidant imbalance in the erythrocytes of sporadic amyotrophic lateral sclerosis patients correlates with the progression of disease. Neurochem. Int. 52, 1284–1289. doi: 10.1016/j.neuint.2008.01.009
Bailly, C. (2019). Potential use of edaravone to reduce specific side effects of chemo-, radio- and immuno-therapy of cancers. Int. Immunopharmacol. 77:105967. doi: 10.1016/j.intimp.2019.105967
Balaban, R. S., Nemoto, S., and Finkel, T. (2005). Mitochondria, oxidants and aging. Cell 120, 483–495. doi: 10.1016/j.cell.2005.02.001
Banno, M., Mizuno, T., Kato, H., Zhang, G., Kawanokuchi, J., Wang, J., et al. (2005). The radical scavenger edaravone prevents oxidative neurotoxicity induced by peroxynitrite and activated microglia. Neuropharmacology 48, 283–290. doi: 10.1016/j.neuropharm.2004.10.002
Bao, A. M., and Swaab, D. F. (2018). The art of matching brain tissue from patients and controls for postmortem research. Handb. Clin. Neurol. 150, 197–217. doi: 10.1016/B978-0-444-63639-3.00015-3
Barber, S. C., and Shaw, P. J. (2010). Oxidative stress in ALS: key role in motor neuron injury and therpaeutic target. Free Radic. Biol. Med. 48, 629–641. doi: 10.1016/j.freeradbiomed.2009.11.018
Bäumer, D., Talbot, K., and Turner, M. R. (2014). Advances in motor neuron disease. J. R. Soc. Med. 107, 14–21. doi: 10.1177/0141076813511451
Bayer, H., Lang, K., Buck, E., Higelin, J., Barteczko, L., Pasquarelli, N., et al. (2017). ALS-causing mutations differentially affect PGC-1α expression and function in the brain vs. peripheral tissues. Neurobiol. Dis. 97(Pt A), 36–45. doi: 10.1016/j.nbd.2016.11.001
Beal, M. F. (2002). Oxidatively modified protein in aging and disease. Free Radic. Biol. Med. 32, 797–803.
Beal, M. F., Lang, A. E., and Ludolph, A. (2005). Neurodegenerative diseases: neurobiology, pathogenesis, and therapeutics. J. Neurol. Neurosurg. Psychiatry 77:284. doi: 10.1136/jnnp.2005.072710
Bedlack, R. S., Joyce, N., Carter, G. T., Pagononi, S., and Karam, C. (2015). Complementary and alternative therapies in ALS. Neurol. Clin. 33, 909–936. doi: 10.1016/j.ncl.2015.07.008
Belenky, P., Racette, F. G., Bogan, K. L., McClure, J. M., Smith, J. S., and Brenner, C. (2007). Nicotinamide riboside promotes Sir2 silencing and extends lifespan via Nrk and Urh1/Pnp1/Meu1 pathways to NAD+. Cell 129, 473–484. doi: 10.1016/j.cell.2007.03.024
Bendotti, C., Tortarolo, M., Suchak, S. K., Calvaresi, N., Carvelli, L., Bastone, A., et al. (2001). Transgenic SOD1 G93A mice develop reduced GLT-1 in spinal cord without alterations in cerebrospinal fluid glutamate levels. J. Neurochem. 79, 737–746.
Bennett, G. J., Doyle, T., and Salvemini, D. (2014). Mitotoxicity in distal symmetrical sensory peripheral neuropathies. Nat. Rev. Neurol. 10, 326–336. doi: 10.1038/nrneurol.2014.77
Berger, F., Ramírez-Hernández, M. H., and Ziegler, M. (2004). The new life of a centenarian: signalling functions of NAD(P). Trends Biochem. Sci. 29, 111–118. doi: 10.1016/j.tibs.2004.01.007
Bhakkiyalakshmi, E., Dineshkumar, K., Karthik, S., Sireesh, D., Hopper, W., Paulmurugan, R., et al. (2016). Pterostilbene-mediated Nrf2 activation: mechanistic insights on Keap1: Nrf2 interface. Bioorg. Med. Chem. 24, 3378–3386. doi: 10.1016/j.bmc.2016.05.011
Bhat, A. H., Dar, K. B., Anees, S., Zargar, M. A., Masood, A., Sofi, M. A., et al. (2015). Oxidative stress, mitocondrial dysfunction and neurodegenerative diseases; a mechanistic insight. Biomed. Pharmacother. 74, 101–110. doi: 10.1016/j.biopha.2015.07.025
Bhatia, N. K., Srivastava, A., Katyal, N., Jain, N., Khan, M. A., Kundu, B., et al. (2015). Curcumin binds to the pre-fibrillar aggregates of Cu/Zn superoxide dismutase (SOD1) and alters its amyloidogenic pathway resulting in reduced cytotoxicity. Biochim. Biophys. Acta 1854, 426–436. doi: 10.1016/j.bbapap.2015.01.014
Bhattacharya, R., Harvey, R. A., Abraham, K., Rosen, J., and Mehta, P. (2019). Amyotrophic lateral sclerosis among patients with a Medicare Advantage prescription drug plan; prevalence, survival and patient characteristics. Amyotroph. Lateral Scler. Frontotemporal. Degener. 20, 1–9. doi: 10.1080/21678421.2019.1582674
Bhullar, K. S., and Vasantha, H. P. (2013). Polyphenols: multipotent therapeutic agents in neurodegenerative diseases. Oxid. Med. Cell. Longev. 2013:891748. doi: 10.1155/2013/891748
Bieganowski, P., and Brenner, C. (2004). Discoveries of nicotinamide riboside as a nutrient and conserved NRK genes establish a Preiss-Handler independent route to NAD+ in fungi and humans. Cell 117, 495–502.
Blasco, H., Garcon, G., Patin, F., Veyrat-Durebex, C., Boyer, J., Devos, D., et al. (2017). Panel of oxidative stress and inflammatory biomarkers in ALS: a pilot study. Can. J. Neurol. Sci. 44, 90–95. doi: 10.1017/cjn.2016.284
Blokhuis, A. M., Groen, E. J. N., Koppers, M., Van Den Berg, L.-H., and Pasterkamp, R. J. (2013). Protein aggregation in amyotrophic lateral sclerosis. Acta Neuropathol. 125, 777–794. doi: 10.1007/s00401-013-1125-6
Bogan, K. L., and Brenner, C. (2008). Nicotinic acid, nicotinamide, and nicotinamide riboside: a molecular evaluation of NAD+ precursor vitamins in human nutrition. Annu. Rev. Nutr. 28, 115–130. doi: 10.1146/annurev.nutr.28.061807.155443
Bond, L., Bernhardt, K., Madria, P., Sorrentino, K., Scelsi, H., and Mitchell, C. S. (2018). A metadata analysis of oxidative stress etiology in preclinical amyotrophic lateral sclerosis: benefits of antioxidant therapy. Front. Neurosci. 12:10. doi: 10.3389/fnins.2018.00010
Bonkowski, M. S., and Sinclair, D. A. (2016). Slowing ageing by design: the rise of NAD+ and sirtuin-activation compounds. Nat. Rev. Mol. Cell Biol. 17, 679–690. doi: 10.1038/nrm.2016.93
Bonnefont-Rousselot, D. (2016). Resveratrol and cardiovascular diseases. Nutrients 8:E250. doi: 10.3390/nu8050250
Bozzo, F., Mirra, A., and Carrì, M. T. (2016). Oxidative stress and mitochondrial damage in the pathogenesis of ALS: new perspectives. Neurosci. Lett. 636, 3–8. doi: 10.1016/j.neulet.2016.04.065
Braidy, N., Guillemin, G., and Grant, R. (2008). Promotion of cellular NAD(+) anabolism: therapeutic potential for oxidative stress in ageing and Alzheimer’s disease. Neurotox. Res. 13, 173–184.
Brieger, K., Schiavone, S., Miller, F. J., and Krause, K. H. (2012). Reactive oxygen species: from health to disease. Swiss Med. Wkly. 142:w13659. doi: 10.4414/smw.2012.13659
Brookes, P. S., Yoon, Y., Robotham, J. L., Anders, M. W., and Sheu, S. S. (2004). Calcium, ATP and ROS. A mitochondrial love-hate triangle. Am. J. Physiol. Cell Physiol. 287, C817–C833. doi: 10.1152/ajpcell.00139.2004
Brown, K. D., Maqsood, S., Huang, J. Y., Pan, Y., Harkcom, W., Li, W., et al. (2014). Activation of SIRT3 by the NAD+ precursor nicotinamide riboside protects from noise-induced hearing loss. Cell Metab. 20, 1059–1068. doi: 10.1016/j.cmet.2014.11.003
Brown, R. H., and Al-Chalabi, A. (2017). Amyotrophic lateral sclerosis. N. Engl. J. Med. 377, 162–172. doi: 10.1056/NEJMra1603471
Brujin, L. I., Miller, T. M., and Cleveland, D. W. (2004). Unraveling the mechanism involved in motor neuron degeneration in ALS. Annu. Rev. Neurosci. 27, 723–749. doi: 10.1146/annurev.neuro.27.070203.144244
Buck, E., Bayer, H., Lindenberg, K. S., Hanselmann, J., Pasquarelli, N., Ludolph, A. C., et al. (2017). Comparison of sirtuin 3 levels in ALS and Huntington’s disease-differential effects in human tissue samples vs. transgenic mouse models. Front. Mol. Neurosci. 10:156. doi: 10.3389/fnmol.2017.00156
Bulotta, S., Celano, M., Lepore, S. M., Montalcini, T., Pujia, A., and Russo, D. (2014). Beneficial effects of olive oil phenolic components oleuropein and hydroxytirosol: focus on protection against cardiovascular and metabolic diseases. J. Transl. Med. 12:219. doi: 10.1186/s12967-014-0219-9
Burgos, E. S., Vetticatt, M. J., and Schramm, V. L. (2013). Recycling nicotinamide. The transition-state structure of human nicotinamide phosphoribosyltransferase. J. Am. Chem. Soc. 135, 3485–3493. doi: 10.1021/ja310180c
Butterfield, D. A., Castegna, A., Drake, J., Scapagnini, G., and Calabrese, V. (2002). Vitamin E and neurodegenerative disorders associated with oxidative stress. Nutr. Neurosci. 5, 229–239. doi: 10.1080/10284150290028954
Camandola, S., and Mattson, M. P. (2011). Aberrant subcellular neuronal calcium regulation in aging and Alzheimer’s disease. Biochim. Biophys. Acta. 1813, 965–973. doi: 10.1016/j.bbamcr.2010.10.005
Cantó, C., Houtkooper, R. H., Pirinen, E., Youn, D. Y., Oosterveer, M. H., Chen, Y., et al. (2012). The NAD+ precursor nicotinamide riboside enhances oxidative metabolism and protects against high fat diet-induced obesity. Cell Metab. 15, 838–847. doi: 10.1016/j.cmet.2012.04.022
Cantó, C., Menzies, K. J., and Auwers, J. (2015). NAD(+) metabolism and the control of energy homeostasis: a balancing act between mitochondria and the nucleus. Cell Metab. 22, 31–53. doi: 10.1016/j.cmet.2015.05.023
Cao, G., Sofic, E., and Prior, R. L. (1997). Antioxidant and prooxidant behavior of flavonoids: structure-activity relationships. Free Radic. Biol. Med. 22, 749–760.
Carey, A. N., Fisher, D. R., Rimando, A. M., Gomes, S. M., Bielinski, D. F., and Shukitt-Hale, B. (2013). Stilbenes and anthocyanins reduce stress signaling in BV-2 mouse microglia. J. Agric. Food. Chem. 61, 5979–5986. doi: 10.1021/jf400342g
Carrì, M. T., Valle, C., Bozzo, F., and Cozzolino, M. (2015). Oxidative stress and mitochondrial damage: importance in non-SOD1 ALS. Front. Cell. Neurosci. 9:41. doi: 10.3389/fncel.2015.00041
Carvalho, C., and Moreira, P. I. (2018). Oxidative stress: a major player in cerebrovascular alterations associated to neurodegenerative events. Front. Physiol. 9:806. doi: 10.3389/fphys.2018.00806
Carvalho, J. C. T., Fernandes, C. P., Daleprane, J. B., Alves, M. S., Stien, D., and Dhammika, N. P. (2018). Role of natural antioxidants from functional foods in neurodegenerative and metabolic disorders. Oxid. Med. Cell. Longev. 2018:1459753. doi: 10.1155/2018/1459753
Castellani, R. J., Lee, H. G., Zhu, X., Perry, G., and Smith, M. A. (2008). Alzheimer diseases pathology as a host response. J. Neurpathol. Exp. Neurol. 67, 523–531. doi: 10.1097/NEN.0b013e318177eaf4
Chandrasekaran, A., Idelchik, M. D. P. S., and Melendez, J. A. (2017). Redox control of senescence and age-related disease. Redox. Biol. 11, 91–102. doi: 10.1016/j.redox.2016.11.005
Chang, H. C., and Guarente, L. (2013). SIRT1 mediates central circadian control in the SCN by a mechanism that decays with aging. Cell 153, 1448–1460. doi: 10.1016/j.cell.2013.05.027
Chang, J., Rimando, A., Pallas, M., Camins, A., Porquet, D., Reeves, J., et al. (2012). Low-dose perostilbene, but not resveratrol, is a potent neuromodulator in aging and Alzheimer’s disease. Neurobiol. Aging 33, 2062–2071. doi: 10.1016/j.neurobiolaging.2011.08.015
Chang, Y., Kong, Q., Shan, X., Tian, G., Ilievea, H., Cleveland, D. W., et al. (2008). Messenger RNA oxidation occurs early in disease pathogenesis and promotes motor neuron degeneration in ALS. PLoS One 3:e2849. doi: 10.1371/journal.pone.0002849
Chen, Q., Wang, Q., Zhu, J., Xiao, Q., and Zhang, L. (2018). Reactive oxygen species: key regulators in vascular health and diseases. Br. J. Pharmacol. 175, 1279–1292. doi: 10.1111/bph.13828
Chen, S., Sayana, P., Zhang, X., and Le, W. (2013). Genetics of amyotrophic lateral sclerosis: an update. Mol. Neurodegener. 8:28. doi: 10.1186/1750-1326-8-28
Chen, Y., Zhang, J., Lin, Y., Lei, Q., Guan, K. L., Zhao, S., et al. (2011). Tumour suppressor SIRT3 deacetylates and activates manganese superoxide dismutase to scavenge ROS. EMBO Rep. 12, 534–541. doi: 10.1038/embor.2011.65
Cheng, Y., Di, S., Fan, C., Cai, L., Gao, C., Jiang, P., et al. (2016). SIRT1 activation by pterostilbene attenuates the skeletal muscle oxidative stress injury and mitochondrial dysfunction induced by ischemia reperfusion injury. Apoptosis 21, 905–916. doi: 10.1007/s10495-016-1258-x
Chi, Y., and Sauve, A. (2013). Nicotinamide riboside, a trace nutrient in foods, is a Vitamina B3 with effects on energy metabolism and neuroprotection. Curr. Opin. Clin. Nutr. Metab. Care 16, 657–661. doi: 10.1097/MCO.0b013e32836510c0
Chico, L., Lenco, E. C., Bisordi, C., Lo Gerfo, A., Petrozzi, L., Petrucci, A., et al. (2018). Amyotrophic lateral sclerosis and oxidative stress: a double-blind therapeutic trial after curcumin supplementation. CNS Neurol. Disord. Drug Targets 17, 767–779. doi: 10.2174/1871527317666180720162029
Chiò, A., Logroscino, G., Traynor, B. J., Collins, J., Simeone, J. C., Goldstein, L. A., et al. (2013). Global epidemiology of amyotrophic lateral sclerosis: a systematic review of the published literature. Neuroepidemiology 41, 118–130. doi: 10.1159/000351153
Chiou, Y. S., Tsai, M. L., Nagabhusanam, K., Wang, Y. J., Wu, C. H., Ho, C. T., et al. (2011). Pterostilbene is more potent that resveratrol in preventing azoxymethane (AOM)-induced colon tumorigenesis via activation of the NF-E2-related factor 2 (Nrfe2)-mediated antioxidant signaling pathway. J. Agric. Food Chem. 59, 2725–2733. doi: 10.1021/jf2000103
Choo, Q. Y., Ming-Yeo, S. C., Ho, P. C., Tanaka, Y., and Lin, H. S. (2014). Pterostilbene surpassed resveratrol for anti-inflammatory application: potency consideration and pharmacokinetics perspective. J. Funct. Food 11, 352–362. doi: 10.1016/j.jff.2014.10.018
Chung, S., Yao, H., Caito, S., Hwang, J. W., Arunachalam, G., and Rahman, I. (2010). Regulation of SIRT1 in cellular functions: role of polyphenols. Arch. Biochem. Biophys. 501, 79–90. doi: 10.1016/j.abb.2010.05.003
Cichocki, M., Paluszczak, J., Szaefer, H., Piechowiak, A., Rimando, A. M., and Baer-Dubowska, W. (2008). Pterostilbene is equally as potent as resveratrol in inhibiting 12-O-tetradecanoylphorbol-13-acetate activated NFkappaB, AP-1. COX-2 and iNOS in mouse epidermis. Mol. Nutr. Food Res. 52(Suppl. 1), S62–S70. doi: 10.1002/mnfr.200700466
Coan, G., and Mitchell, C. S. (2015). An assessment of possible neuropathology and clinical relationships in 46 sporadic amyotrophic lateral sclerosis patient autopsies. Neurodegener. Dis. 15, 301–312. doi: 10.1159/000433581
Cohen, T. J., Hwang, A. W., Restrepo, C. X., Yuan, J. Q., Trojanowski, J. Q., and Lee, V. M. (2015). An acetylation switch controls TDP-43 function and aggregation propensity. Nat. Commun. 6:5845. doi: 10.1038/ncomms6845
Cohen, T. J., Hwang, A. W., Unger, T., Trojanowski, J. Q., and Lee, V. M. (2012). Redox signaling directly regulates TDP-43 via cysteine oxidation and disulphide cross-linking. EMBO J. 31, 1241–1252. doi: 10.1038/emboj.2011.471
Colbourne, J., Baker, N., Wang, P., Liu, L., Tucker, C., and Roebothan, B. (2013). Adequacy of niacin, folate, and vitamin B12 intakes from foods among Newfoundland and Labrador adults. Can. J. Diet. Pract. Res. 74, 63–68. doi: 10.3148/74.2.2013.63
Coleman, M. (2005). Axon degeneration mechanisms: commonality amid diversity. Nat. Rev. Neurosci. 6, 889–898. doi: 10.1038/nrn1788
Conforti, L., Fang, G., Beirowski, B., Wang, M. S., Sorci, L., Asress, S., et al. (2006). NAD(+) and axon degeneration revisited: Nmnat 1 cannot substitute for Wld(S) to delay Wallerian degeneration. Cell Death Differ. 14, 116–127. doi: 10.1038/sj.cdd.4401944
Cooke, M. S., Evans, M. D., Herbert, K. E., and Lunec, J. (2000). Urinary 8-oxo-2’-deoxyguanosine—source, significance and supplements. Free Radic. Res. 32, 381–397.
Cooke, M. S., Lunec, J., and Evans, M. D. (2002). Progress in the analysis of urine oxidative DNA damage. Free Radic. Biol. Med. 33, 1601–1614.
Coppedè, F., and Migliore, L. (2015). DNA damage in neurodegenerative diseases. Mutat. Res. 776, 84–97. doi: 10.1016/j.mrfmmm.2014.11.010
Cordeiro, R. M. (2014). Reactive oxygen species at phospholipid bilayers: distribution, mobility and permation. Biochim. Biophys. Acta. 1838, 438–444. doi: 10.1016/j.bbamem.2013.09.016
Correia, S. C., Carvalho, C., Cardoso, S., Santos, R. X., Santos, M. S., Oliveira, C. R., et al. (2010). Mitochondrial preconditioning: a potential neuroprotective strategy. Front. Aging Neurosci. 2:138. doi: 10.3389/fnagi.2010.00138
Correia, S. C., and Moreira, P. I. (2010). Hypoxia-inducible factor 1: a new hope to counteract neurodegeneration? J. Neurochem. 112, 1–12. doi: 10.1111/j.1471-4159.2009.06443.x
Cory, H., Passarelli, S., Szeto, J., Tamez, M., and Mattei, J. (2018). The role of polyphenols in human health and food systems: a mini-review. Front. Nutr. 5:87. doi: 10.3389/fnut.2018.00087
Cova, E., Bongioanni, P., Cereda, C., Metelli, M. R., Salvaneschi, L., Bernuzzi, S., et al. (2010). Time course of oxidant markers and antioxidant defenses in subgroups of amyotrophic lateral sclerosis patients. Neurochem. Int. 56, 687–693. doi: 10.1016/j.neuint.2010.02.004
Covington, J. D., and Bajpeyi, S. (2016). The sirtuins: markers of metabolic health. Mol. Nutr. Food Res. 60, 79–91. doi: 10.1002/mnfr.201500340
Coyle, J. T., and Puttfarcken, P. (1993). Oxidative stress, glutamate and neurodegenerative disorders. Science 262, 689–695.
Cozzolino, M., and Carrì, M. T. (2012). Mitochondrial dysfunction in ALS. Prog. Neurobiol. 97, 54–66. doi: 10.1016/j.pneurobio.2011.06.003
Crozier, A., Jaganath, I. B., and Clifford, M. N. (2009). Dietary phenolics: chemistry, bioavailability and effects on health. Nat. Prod. Rep. 26, 1001–1043. doi: 10.1039/b802662a
Czpaski, G. A., Czubowicz, K., and Strosznajder, R. P. (2012). Evaluation of the antioxidative properties of lypoxygenase inhibitors. Pharmacol. Rep. 64, 1179–1188.
Dahlqvist, J. R., Oestergaard, S. T., Poulsen, N. S., Kank, K. L., Thomsen, C., and Vissing, J. (2019). Muscle contractility in spinobulbar muscular atrophy. Sci. Rep. 9:4680. doi: 10.1038/s41598-019-41240-y
D’Amico, E., Factor-Litvak, P., Santella, R. M., and Mitsumoto, H. (2013). Clinical perspective of oxidative stress in sporadic ALS. Free Radic. Biol. Med. 65, 509–527. doi: 10.1016/j.freeradbiomed.2013.06.029
Dardiotis, E., Panayiotou, E., Feldman, M. L., Hadjisavvas, A., Malas, S., Vonta, I., et al. (2013). Intraperitoneal melatonin is not neuroprotective in the g93asod1 transgenic mouse model of familial als and may exacerbate neurodegeneration. Neurosci. Lett. 548, 170–175. doi: 10.1016/j.neulet.2013.05.058
Davidson, M. H. (2008). Niacin use and cutaneous flushing: mechanisms and strategies for prevention. Am. J. Cardiol. 101, 14B–19B. doi: 10.1016/j.amjcard.2008.02.028
De Gara, L., and Foyer, C. H. (2017). Ying and Yang interplay between reactive oxygen and reactive nitrogen species controls cells functions. Plant Cell Environ. 40, 459–461. doi: 10.1111/pce.12936
De Silva, T. M., and Miller, A. A. (2016). Cerebral small vessel diseases: targeting oxidative stress as a novel therapeutic strategy? Front. Pharmacol. 17:61. doi: 10.3389/fphar.2016.00061
Dellinger, R. W., Roel-Santos, S., Morris, M., Evans, M., Alminana, D., Guarente, L., et al. (2017). Repeat dose NRPT (nicotinamide riboside and pterostilbene) increases NAD+ levels in humans safely and sustainably: a randomized, doublé-blind, placebo-controlled study. NPJ Aging Mech. Dis. 3:17. doi: 10.1038/s41514-017-0016-9
Deng, H. X., Shi, Y., Furukawa, Y., Zhai, H., Fu, R., Liu, E., et al. (2006). Conversion to the amyotrophic lateral sclerosis phenotype is associated with intermolecular linked insoluble aggregates of SOD1 in mitochondria. Proc. Natl. Acad. Sci. U.S.A. 103, 7142–7147. doi: 10.1073/pnas.0602046103
Desnuelle, C., Dib, M., Garrel, C., and Favier, A. (2001). A double-blind, placebo-controlled randomized clinical trial of alpha-tocopherol (vitamin E) in the treatment of amyotrophic lateral sclerosis. ALS riluzole-tocopherol Study Group. Amyotroph. Lateral Scler. Other Motor Neuron Disord. 2, 9–18.
Di Matteo, V., and Esposito, E. (2003). Biochemical and therapeutic effects of antioxidants in the treatment of Alzheimer’s disease, Parkinson’s disease, and amyotrophic lateral sclerosis. Curr. Drug Targets CNS Neurol. Disord. 2, 95–107.
Di Pietro, L., Baranzini, M., Berardinelli, M. G., Lattanzi, W., Monforte, M., Tasca, G., et al. (2017). Potential therapeutic targets for ALS: MIR206, MIR208b and MIR499 are modulated during disease progression in the skeletal muscle of patients. Sci. Rep. 7:9538. doi: 10.1038/s41598-017-10161-z
Dias, V., Junn, E., and Mouradian, M. (2013). The role of oxidative stress in Parkinson’s diseases. J. Parkinsons Dis. 3, 461–491. doi: 10.3233/JPD-130230
Ding, G., Fu, M., Quin, Q., Lewis, W., Kim, H. W., Fukai, T., et al. (2007). Cardiac peroxisome proliferator-activated receptor gamma is essential in protecting cardiomyocytes from oxidative damage. Cardiovasc. Res. 76, 269–279. doi: 10.1016/j.cardiores.2007.06.027
Dirnagl, U., Becker, K., and Meisel, A. (2009). Preconditioning and tolerance against cerebral ischaemia: from experimental strategies to clinical use. Lancet Neurol. 8, 398–412. doi: 10.1016/S1474-4422(09)70054-7
Dirnagl, U., and Meisel, A. (2008). Endogenous neuroprotection: mitochondria as gateways to cerebral preconditioning? Neuropharmacology 55, 334–344. doi: 10.1016/j.neuropharm.2008.02.017
Dixon, S. J., and Stockwell, B. R. (2014). The role of iron and reactive oxygen species in cell death. Nat. Chem. Biol. 10, 9–17. doi: 10.1038/nchembio.1416
Dizdaroglu, M., and Jaruga, P. (2012). Mechanism of free radical-induced damage to DNA. Free Radic. Res. 46, 382–419. doi: 10.3109/10715762.2011.653969
Dolopikou, C. F., Kourtzidis, I. A., Margaritelis, N. V., Vrabas, I. S., Koidou, I., Kyparos, A., et al. (2019). Acute nicotinamide riboside supplementation improves redox homeostasis and exercise performance in old individuals: a double-blind cross-over study. Eur. J. Nutr. doi: 10.1007/s00394-019-01919-4 [Epub ahead of print].
Dong, H., Xu, L., Wu, L., Wang, X., Duan, W., Li, H., et al. (2014). Curcumin abolishes mutant TDP-43 induced excitability in a motoneuron-like cellular model of ALS. Neuroscience 272, 141–153. doi: 10.1016/j.neuroscience.2014.04.032
Dorst, J., Ludolph, A. C., and Huebers, A. (2017). Disease-modifying and symptomatic treatment of amyotrophic lateral sclerosis. Ther. Adv. Neurol. Disord. 11:1756285617734734. doi: 10.1177/1756285617734734
Du, Y., Wu, H. T., Quin, X. Y., Cao, C., Liu, Y., Cao, Z. Z., et al. (2018). Postmortem brain, cerebrospinal fluid, and blood neurotrophic factor levels in Alzheimer’s disease: a systematic review and meta-analysis. J. Mol. Neurosci. 65, 289–300. doi: 10.1007/s12031-018-1100-8
Duan, W., Li, X., Shi, J., Guo, Y., Li, Z., and Li, C. (2010). Mutant TAR DNA-binding protein-43 induces oxidative injury in motor neuron-like cell. Neuroscience 169, 1621–1629. doi: 10.1016/j.neuroscience.2010.06.018
Duffy, L. M., Chapman, A. L., Shaw, P. J., and Grierson, A. J. (2011). Review: the role of mitochondria in the pathogenesis of amyotrophic lateral sclerosis. Neuropathol. Appl. Neurobiol. 37, 336–352. doi: 10.1111/j.1365-2990.2011.01166.x
Dupuis, L., Pradat, P. F., Ludolph, A. C., and Loeffler, J. P. (2011). Energy metabolism in amyotrophic lateral sclerosis. Lancet Neurol. 10, 75–82. doi: 10.1016/S1474-4422(10)70224-6
Dvorakova, M., and Landa, P. (2017). Anti-inflammatory activity of natural stilbenoids: a review. Pharmacol. Res. 124, 126–145. doi: 10.1016/j.phrs.2017.08.002
Dyck, G. J. B., Raj, P., Zieroth, S., Dyck, J. R. B., and Ezekowitz, J. A. (2019). The effects of resveratrol in patients with cardiovascular disease and heart failure: a narrative review. Int. J. Mol. Sci. 20:E904. doi: 10.3390/ijms20040904
Ehrhart, J., Smith, A. J., Kuzmin-Nichols, N., Zesiewicz, T. A., Jahan, I., Shytle, R. D., et al. (2015). Humoral factors in ALS patients during disease progression. J. Neuroinflammation 12:127. doi: 10.1186/s12974-015-0350-4
El-Sayed, S. M., Mansour, A. M., and Nady, M. E. (2015). Protective effects of pterostilbene against actaminophen-induced hepatotoxicity in rats. J. Biochem. Mol. Toxicol. 29, 35–42. doi: 10.1002/jbt.21604
Emerit, J., Edeas, M., and Bricaire, F. (2004). Neurodegenerative diseases and oxidative stress. Biomed. Pharmacother. 58, 39–46.
Esposito, E., Capasso, M., di Tomasso, N., Corona, C., Pellegrini, F., Uncini, A., et al. (2007). Antioxidant strategies based on tomato- enriched food or pyruvate do not affect disease onset and survival in an animal model of amyotrophic lateral sclerosis. Brain Res. 1168, 90–96. doi: 10.1016/j.brainres.2007.06.095
Esposito, E., Rotilio, D., Di Matteo, V., Di Giulio, C., Cacchio, M., and Algeri, S. (2002). A review of specific dietary antioxidants and the effects on biochemical mechanisms related to neurodegenerative processes. Neurobiol. Aging 23, 719–735.
Essa, M. M., Subash, S., Braidy, N., Adawi, S., Lim, C. K., Manivasagam, T., et al. (2013). Role of NAD+, oxidative and tryptophan metabolism in autism spectrum disorders. Int. J. Tryptophan Res. 6, 15–28. doi: 10.4137/IJTR.S11355
Estrela, J. M., Ortega, A., Mena, S., Rodriguez, M. L., and Asensi, M. (2013). Pterostilbene: biomedical applications. Crit. Rev. Clin. Lab. Sci. 50, 65–78. doi: 10.3109/10408363.2013.805182
Falkowski, P. G., and Godfrey, L. V. (2008). Electrons, life an the evolution of Earth’s oxygen cycle. Philos. Trans. R. Soc. Lond. B Biol. Sci. 363, 2705–2716. doi: 10.1098/rstb.2008.0054
Federico, A., Cardaioli, E., Da Pozzo, P., Formichi, P., Gallus, G. N., and Radi, E. (2012). Mitochondria, oxidative stress and neurodegeneration. J. Neurol. Sci. 322, 254–262. doi: 10.1016/j.jns.2012.05.030
Ferrante, K. L., Shefner, J., Zhang, H., Betensky, R., O’Brien, M., Yu, H., et al. (2005). Tolerance of high-dose (3,000 mg/day) coenzyme Q10 in ALS. Neurology 56, 1834–1836. doi: 10.1212/01.wnl.0000187070.35365.d7
Ferri, A., Cozzolino, M., Crosio, C., Nencini, M., Casciati, A., Gralla, E. B., et al. (2006). Familial ALS-superoxide dismutases associate with mitocondria and shift their redox potentials. Proc. Natl. Acad. Sci. U.S.A. 103, 13860–13865. doi: 10.1073/pnas.0605814103
Fiala, M., Mizwicki, M. T., Weitzman, R., Magpantay, L., and Nishimoto, N. (2013). Tocilizumab infusión therapy normalizes inflammation in sporadic ALS patients. Am. J. Neurodegener. Dis. 2, 129–139.
Fiedor, J., Sulikowska, A., Orzechowska, A., Fiedor, L., and Burda, K. (2012). Antioxidant effects of carotenoids in a model pigment-protein complex. Acta Biochim. Pol. 59, 61–64.
Fischer, R., and Maier, O. (2015). Interrelation of oxidative stress and inflammation in neurodegenerative disease: role of TNF. Oxid. Med. Cell. Longev. 2015:610813. doi: 10.1155/2015/610813
Fitzgerald, K. C., O’Reilly, ÉJ., Fondell, E., Falcone, G. J., McCullough, M. L., Park, Y., et al. (2013). Intakes of vitamin C and carotenoids and risk of amyotrophic lateral sclerosis: pooled results from 5 cohort studies. Ann. Neurol. 73, 236–245. doi: 10.1002/ana.23820
Fletcher, R. S., Ratajczak, J., Doig, C. L., Oakey, L. A., Callingham, R., Da Silva-Xavier, G., et al. (2017). Nicotinamide riboside kinases display redundancy in mediating nicotinamide mononucleotide and nicotinamide riboside metabolism in skeletal muscle cells. Mol. Metab. 6, 819–832. doi: 10.1016/j.molmet.2017.05.011
Formella, I., Svahn, A. J., Radford, R. A. W., Don, E. K., Cole, N. J., Hogan, A., et al. (2018). Real-time visualization of oxidateive stress-mediated neurodegeneration of individual spinal motor neurons in vivo. Redox. Biol. 19, 226–234. doi: 10.1016/j.redox.2018.08.011
Förstermann, U., and Sessa, W. C. (2012). Nitric oxide synthases: regulation and function. Eur. Heart. J. 33, 829–837. doi: 10.1093/eurheartj/ehr304
Frederick, D. W., Loro, E., Liu, L., Davila, A. J., Chellappa, K., Silverman, I. M., et al. (2016). Loss of NAD homeostasis leads to progressive and reversible degeneration of skeletal muscle. Cell Metab. 24, 269–282. doi: 10.1016/j.cmet.2016.07.005
Freeman, L. R., and Keller, J. N. (2012). Oxidative stress and cerebral endotelial cells: regulation of the blood-brain-barrier and antioxidant based interventions. Biochim. Biophys. Acta 1822, 822–829. doi: 10.1016/j.bbadis.2011.12.009
Fritz, K. S., and Petersen, D. R. (2013). An overview of the chemistry and biology of reactive aldehydes. Free. Radic. Biol. Med. 59, 85–91. doi: 10.1016/j.freeradbiomed.2012.06.025
Fu, L., Xu, B. T., Gan, R. Y., Zhang, Y. A., Xu, R., Xia, E. Q., et al. (2011). Total phenolic contents and antioxidant capacities of herbal and tea infusions. Int. J. Mol. Sci. 12, 2112–2124. doi: 10.3390/ijms12042112
Galbussera, A., Tremolizzo, L., Brighina, L., Testa, D., Lovati, R., Ferrarese, C., et al. (2006). Vitamin E intake and quality of life in amyotrophic lateral sclerosis patients: a follow-up case series study. Neurol. Sci. 27, 190–193. doi: 10.1007/s10072-006-0668-x
Gamez, J., Corbera-Bellalta, M., Nogales, G., Raguer, N., García-Arumí, E., Badia-Canto, M., et al. (2006). Mutational analysis of the Cu/Zn superoxide dismutase gen in a Catalan ALS population: should all sporadic ALS cases also be screened for SOD1? J. Neurol. Sci. 247, 21–28. doi: 10.1016/j.jns.2006.03.006
Gandhi, S., and Abramov, A. Y. (2012). Mechanism of oxidative stress in neurodegeneration. Oxid. Med. Cell. Longev. 2012:428010. doi: 10.1155/2012/428010
Ganesan, K., and Xu, B. (2017). Polyphenol-rich lentils ant their health promoting effects. Int. J. Mol. Sci. 18:E2390. doi: 10.3390/ijms18112390
Ganie, S. A., Dar, T. A., Bhat, A. H., Dar, K. B., Anees, S., Zargar, M. A., et al. (2016). Melatonin: a potential anti-oxidant therapeutic agent for mitochondrial dysfunctions and related disorders. Rejuvenation Res. 19, 21–40. doi: 10.1089/rej.2015.1704
Ghasemi, F., Bagheri, H., Barreto, G. E., Read, M. I., and Sahebkar, A. (2019). Effects of curcumin on microgial cells. Neurotox. Res. 36, 12–26. doi: 10.1007/s12640-019-00030-0
Golenia, A., Leskiewicz, M., Regulska, M., Budziszewska, B., Szczesny, E., Jagiella, J., et al. (2014). Catalase activity in blood fractions of patients with sporadic ALS. Pharmacol. Rep. 66, 704–707. doi: 10.1016/j.pharep.2014.02.021
Gomes, A. P., Price, N. L., Ling, A. J., Moslehi, J. J., Montogomery, M. K., Rajman, L., et al. (2013). Declining NAD+ induces a pseudohypoxic state disrupting nuclear-mitochondrial communication during aging. Cell 155, 1624–1638. doi: 10.1016/j.cell.2013.11.037
Gomez-Zorita, S., Tréguer, K., Mercader, J., and Carpéné, C. (2013). Resveratrol directly affects in vitro lipolysis and glucose transport in human fat cells. J. Physiol. Biochem. 69, 585–593. doi: 10.1007/s13105-012-0229-220
Gong, B., Pan, Y., Vempati, P., Zhao, W., Knable, L., Ho, L., et al. (2013). Nicotinamide riboside restores cognition through an upregulation of proliferator-activated receptor-γ coactivator 1α regulated ß-secretase 1 degradation and mitochondrial gene expression in Alzheimer’s mouse models. Neurobiol. Aging 34, 1581–1588. doi: 10.1016/j.neurobiolaging.2012.12.005
González, D., Contreras, O., Rebolledo, D. L., Espinoza, J. P., Van Zundert, B., and Brandan, E. (2017). ALS skeletal muscle shows enhanced TGF-ß signaling, fibrosis and induction of fibro/adipogenic progenitor markers. PLoS One 12:e0177649. doi: 10.1371/journal.pone.0177649
Goody, M. F., and Henry, C. A. (2018). A need for NAD+ in muscle development, homeostasis, and aging. Skelet. Muscle 8:9. doi: 10.1186/s13395-018-0154-1
Gordon, P. H. (2013). Amyotrophic lateral sclerosis: an update for 2013 clinical features, pathophysiology, management and therapeutic trials. Aging Dis. 4, 295–310. doi: 10.14336/AD.2013.0400295
Gordon, P. H., Mehal, J. M., Holamn, R. C., Rowland, L. P., Rowland, A. S., and Cheek, J. E. (2013). Incidence of amyotrophic lateral sclerosis among American Indians and Alaska natives. JAMA Neurol. 70, 476–480. doi: 10.1001/jamaneurol.2013.929
Gowland, A., Opie-Martin, S., Scott, K. M., Jones, A. R., Mehta, P. R., Batts, C. J., et al. (2019). Predicting the future of ALS: the impact of demographic change and potential new treatments on the prevalence of ALS in the United Kingdom, 2020-2116. Amyotroph. Lateral Scler. Frontotemporal. Degener. 20, 264–274. doi: 10.1080/21678421.2019.1587629
Graf, M., Ecker, D., Horowski, R., Kramer, B., Riederer, P., Gerlach, M., et al. (2005). High dose vitamin E therapy in amyotrophic lateral sclerosis as add-on therapy to riluzole: results of a placebo-controlled double-blind study. J. Neural Transm. 112, 649–660. doi: 10.1007/s00702-004-0220-1
Green, K. N., Steffan, J. S., Martínez-Coria, H., Sun, X., Schreiber, S. S., Thompson, L. M., et al. (2008). Nicotinamide restores cognition in Alzheimer’s disease transgenic mice via mechanism involving sirtuin inhibiton and selective reduction of Thr231-phosphotau. J. Neurosci. 28, 11500–11510. doi: 10.1523/JNEUROSCI.3203-08.2008
Grochowski, C., Litak, J., Kamieniak, P., and Maciejewski, R. (2018). Oxidative stress in small vessel disease. Role of reactive species. Free Radic. Res. 52, 1–13. doi: 10.1080/10715762.2017.1402304
Grozio, A., Mills, K. F., Yoshino, J., Bruzzone, S., Sociali, G., Tokizane, K., et al. (2019). Slc12a8 is a nicotinamide mononucleotide transporter. Nat. Metab. 1, 47–57. doi: 10.1038/s42255-018-0009-4
Guarente, L. (2014). Linking DNA damage, NAD(+)/SIRT1, and aging. Cell. Metab. 20, 706–707. doi: 10.1016/j.cmet.2014.10.015
Guareschi, S., Cova, E., Cereda, C., Ceroni, M., Donetti, E., Bosco, D. A., et al. (2012). An over-oxidized form of superoxide dismutase found in sporadic amyotrophic lateral sclerosis with bulbar onset shares a toxic mechanism with mutant SOD1. Proc. Natl. Acad. Sci. U.S.A. 109, 5074–5079. doi: 10.1073/pnas.1115402109
Guegan, C., Vila, M., Rosoklija, G., Hays, A. P., and Przedborski, S. (2001). Recruitment of the mitochondrial-dependent apoptotic pathway in amyotrophic lateral sclerosis. J. Neurosci. 21, 6569–6576.
Guest, J., and Grant, R. (2016). Carotenoids and neurobiological health. Adv. Neurobiol. 12, 199–228. doi: 10.1007/978-3-319-28383-8_11
Guille, A., Bodor, E. T., Ahmed, K., and Offermanns, S. (2008). Nicotinic acid: pharmacological effects and mechanisms of action. Annu. Rev. Pharmacol. Toxicol. 48, 79–106. doi: 10.1146/annurev.pharmtox.48.113006.094746
Guo, C., Sun, L., Chen, X., and Zhang, D. (2013). Oxidative stress, mitochondrial damage and neurodegenerative diseases. Neural Regen. Res. 8, 2003–2014. doi: 10.3969/j.issn.1673-5374.2013.21.009
Guo, Y., Zhang, L., Li, F., Hu, C. P., and Zhang, Z. (2016). Restoration of sirt1 function by pterostilbene attenuates hypoxia-reoxygenation injury in cardiomyocytes. Eur. J. Pharmacol. 776, 26–33. doi: 10.1016/j.ejphar.2016.02.052
Gurney, M. E., Cutting, F. B., Zhai, P., Doble, A., Taylor, C. P., Andrus, P. K., et al. (1996). Benefit of vitamin E, riuzole, and gabapentin in a transgenic model of familial amyotrophic lateral sclerosis. Ann. Neurol. 39, 147–157. doi: 10.1002/ana.410390203
Gustafson, D. R., Clare Morris, M., Scarmeas, N., Shah, R. C., Sijben, J., Yaffe, K., et al. (2015). New perspectives on Alzheimer’s disease and nutrition. J. Alzheimers Dis. 46, 1111–1127. doi: 10.3233/JAD-150084
Gyulkhandanyan, A. V., Feeney, C. J., and Pennefather, P. S. (2003). Modulation of mitochondrial membrane potential and reactive oxygen species production by copper in astrocytes. J. Neurochem. 87, 448–460.
Hafner, A. V., Dai, J., Gomes, A. P., Xiao, C. Y., Palmeira, C. M., Rosenzweig, A., et al. (2010). Regulation of the mPTP by SIRT3-mediated deacetylation of CypD at lysine 166 suppresses age-related cardiac hypertrophy. Aging 2, 914–923. doi: 10.18632/aging.100252
Haigis, M. C., and Sinclair, D. A. (2010). Mammalian sirtuins: biological insights and disease relevance. Annu. Rev. Pathol. 5, 253–295. doi: 10.1146/annurev.pathol.4.110807.092250
Hall, E. D., Andrus, P. K., Oostveen, J. A., Fleck, T. J., and Gurney, M. E. (1998). Relationship of oxygen radical-induced lipid peroxidative damage to disease onset and progression in a transgenic model of familial ALS. J. Neurosci. Res. 53, 66–77.
Halliwell, B. (2012). Free radicals and antioxidants: updating a personal view. Nutr. Rev. 70, 257–265. doi: 10.1111/j.1753-4887.2012.00476.x
Han, S., Choi, J. R., Soon-Shin, K., and Kang, S. J. (2012). Resveratrol upregulated heat shock proteins and extended the survival of G93A-SOD1 mice. Brain Res. 5, 112–117. doi: 10.1016/j.brainres.2012.09.022
Harlan, B. A., Pehar, M., Sharma, D. R., Beeson, G., Beeson, C. C., and Vargas, M. (2016). Enhancing NAD+ salvage pathway reverts the toxicity of primary astrocytes expressing amyotrophic lateral sclerosis-linked mutant SOD1. J. Biol. Chem. 291, 10836–10846. doi: 10.1074/jbc.M115.698779
Harraz, M. M., Marden, J. J., Zhou, W., Zhang, Y., Williams, A., Sharov, V. S., et al. (2008). SOD1 mutations disrupt redox-sensitive. J. Clin. Invest. 118, 659–670. doi: 10.1172/JCI34060
He, L., He, T., Farrar, S., Ji, L., Liu, T., and Ma, X. (2017). Antioxidants maintain cellular redox homeostasis by elimination of reactive oxygen species. Cell. Physiol. Biochem. 44, 532–553. doi: 10.1159/000485089
Hikosaka, K., Yaku, K., Okabe, K., and Nakagawa, T. (2019). Implications of NAD metabolism in pathophysiology and therapeutics for neurodegenerative diseases. Nutr. Neurosci. 7, 1–13. doi: 10.1080/1028415X.2019.1637504
Hodgkinson, V. L., Lounsberry, J., Mirian, A., Genge, A., Benstead, T., Briemberg, H., et al. (2018). Provincial differences in the diagnosis and care of amyotrophic lateral sclerosis. Can. J. Neurol. Sci. 45, 652–659. doi: 10.1017/cjn.2018.311
Hooten, K. G., Beers, D. R., Zhao, W., and Appel, S. H. (2015). Protective and toxic neuroinflammation in amyotrophic lateral sclerosis. Neurotherapeutics 12, 364–375. doi: 10.1007/s13311-014-0329-3
Hou, Y., Lautrup, S., Cordonnier, S., Wang, Y., Croteau, D. L., Zavala, E., et al. (2017a). NAD+ supplementation normalizes key Alzheimer’s features and DNA damage responses in a new AD mouse model with introduced DNA repair deficiency. Proc. Natl. Acad. Sci. U.S.A. 115, E1876–E1885. doi: 10.1073/pnas.1718819115
Hou, Y., Song, H., Croteau, D. L., Akbari, M., and Bohr, V. A. (2017b). Genome instability in Alzheimer disease. Mech. Ageing Dev. 161(Pt A), 83–94. doi: 10.1016/j.mad.2016.04.005
Hou, Y., Xie, G., Miao, F., Ding, L., Mou, Y., Wang, I., et al. (2014). Pterostilbene attenuates lipopolysaccharide-induced learning and memory impairment possibily via inhibiting microglia activation and protecting neuronal injury in mice. Prog. Neuropsychopharmacol. Biol. Psychiatry 54, 92–102. doi: 10.1016/j.pnpbp.2014.03.015
Houtkooper, R. H., Cantó, C., Wanders, R. J., and Auwerx, J. (2010). The secret life of NAD+: an old metabolite controlling new metabolic signaling pathways. Endocr. Rev. 31, 194–223. doi: 10.1210/er.2009-0026
Hsieh, H., and Yang, C. M. (2013). Role of redox signaling in neuroinflammation and neurodegenerative diseases. Biomed. Res. Int. 2013:484613. doi: 10.1155/2013/484613
Hua, Z., and Rong, T. (2016). Dietary polyphenols, oxidative stress and antioxidant and anti-inflammatory effects. Curr. Opin. Food Sci. 8, 33–42. doi: 10.1016/j.cofs.2016.02.002
Huang, J. Y., Hirschey, M. D., Shimazu, T., Ho, L., and Verdin, E. (2010). Mitochondrial sirtuins. Biochim. Biophys. Acta 1804, 1645–1651. doi: 10.1016/j.bbapap.2009.12.021
Huhn, S., Kharabian Masouleh, S., Stumvoll, M., Villringer, A., and Witte, V. (2015). Components of a Mediterranean diet and their impacto n cognitive functions in aging. Front. Aging Neurosci. 7:132. doi: 10.3389/fnagi.2015.00132
Huiyong, Y., Libin, X., and Porter, N. (2011). Free radical lipid peroxidation: mechanism and analysis. Chem. Rev. 111, 5944–5972. doi: 10.1021/cr200084z
Ihara, Y., Nobukuni, K., Takata, H., and Hayabara, T. (2005). Oxidative stress and metal content in blood and cerebrospinal fluid in amyotrophic lateral sclerosis patients with and without a Cu, Zn superoxide dismutase mutation. Neurol. Res. 27, 105–108. doi: 10.1179/016164105X18430
Ikawa, M., Okazawa, H., Tsujikawa, T., Muramatsu, T., Kishitani, T., Kamisawa, T., et al. (2015). Increased oxidative stress is related to disease severity in the ALS motor cortex: a PET study. Neurology 84, 2033–2039. doi: 10.1212/WNL.0000000000001588
Ikeda, K., and Iwasaki, Y. (2015). Edaravone, a free radical scavenger, delayed symptomatic and pathological progression of motor neuron disease in the wobbler mouse. PLoS One 10:e0140316. doi: 10.1371/journal.pone.0140316
Imai, S., and Guarente, L. (2014). NAD+ and sirtuins in aging and disease. Trends Cell Biol. 24, 464–471. doi: 10.1016/j.tcb.2014.04.002
Ingre, C., Roos, P. M., Piehl, F., Kamel, F., and Fang, F. (2015). Risk factors for amyotrophic lateral sclerosis. Clin. Epidemiol. 12, 181–193. doi: 10.2147/CLEP.S37505
Inoue, H., Jiang, X. F., Katayama, T., Osada, S., Umesono, K., and Namura, S. (2003). Brain protection by reeratrol and fenofibrate against stroke requieres peroxsiome proliferator-activated receptor alpha in mice. Neurosci. Lett. 352, 203–206.
Ip, P., Sharda, P. R., Cunningham, A., Chakrabartty, S., Pande, V., and Chakrabartty, A. (2017). Quercitrin and quercetin 3-β-d-glucoside as chemical chaperones for the A4V SOD1 ALS-causing mutant. Protein Eng. Des. Sel. 30, 431–440. doi: 10.1093/protein/gzx025
Islam, M. T. (2017). Oxidative stress and mitochondrial dysfunction-linked neurodegenerative disorders. Neurol. Res. 39, 73–82. doi: 10.1080/01616412.2016.1251711
Isonaka, R., Hiruma, H., Katakura, T., and Kawakami, T. (2011). Inhibition of superoxide dismutase selectively suppresses growth of rat spinal motor neurons: comparison with phosphorylated neurofilament-containing spinal neurons. Brain Res. 1425, 13–19. doi: 10.1016/j.brainres.2011.09.046
Israelson, A., Arbel, N., Da Cruz, S., Ilieva, H., Yamanaka, K., Shoshan-Barmatz, V., et al. (2010). Misfolded mutant SOD1 directly inhibits VDAC1 conductance in a mouse model of inherited ALS. Neuron 67, 575–587. doi: 10.1016/j.neuron.2010.07.019
Iverson, S. L., and Orrenius, S. (2004). The cardiolipin-cytochrome c interaction and the mitochondrial regulation of apoptosis. Arch. Biochem. Biophys. 423, 37–46.
Jackson, C., Heiman-Patterson, T., Kittrell, P., Baranovsky, T., Mcananama, G., Bower, L., et al. (2019). Radicava (edaravone) for amyotrophic lateral sclerosis: US experience at 1 year after launch. Amyotroph. Lateral Scler. Frontotemporal. Degener. 20, 1–6. doi: 10.1080/21678421.2019.1645858
Jacob, S., Poeggler, B., Weishaupt, J. H., Sirén, A. L., Hardeland, R., Bähr, M., et al. (2002). Melatonin as a candidate compound for neuroprotection in amyotrophic lateral sclerosis (ALS): high tolerability of daily oral melatonin administration in ALS patients. J. Pineal Res. 33, 186–187.
Jaiswal, M. K. (2017). Riluzole but not melatonin ameliorates acute motor neuron degeneration and moderately inhibits sod1-mediated excitotoxicity induced disrupted mitochondrial ca2+ signaling in amyotrophic lateral sclerosis. Front. Cell. Neurosci. 10:295. doi: 10.3389/fncel.2016.00295
Jeong, J. K., Moon, M. H., Bae, B. C., Lee, Y. J., Seol, J. W., Kang, H. S., et al. (2012). Autophagy induced by resveratrol prevents human prion protein-mediated neurotoxicity. Neurosci. Res. 73, 99–105. doi: 10.1016/j.neures.2012.03.005
Jęśko, H., Wencel, P., Strosznajder, R. P., and Strosznajder, J. B. (2017). Sirtuins and their roles in brain aging and neurodegenerative disorders. Neurochem. Res. 42, 876–890. doi: 10.1007/s11064-016-2110-y
Jha, N., Ryu, J. J., Choi, E. H., and Kaushik, N. K. (2017). Generation and role of reactive oxygen and nitrogen species induced by plasma, lasers, chemical agents, and other systems in dentistry. Oxid. Med. Cell. Longev. 2017:7542540. doi: 10.1155/2017/7542540
Jiang, H., Tian, X., Guo, Y., Duan, W., Bu, H., and Li, C. (2011). Activation of nuclear factor erythroid 2-related factor 2 cytoprotective signaling by curcumin protect primary spinal cord astrocytes against oxidative toxicity. Biol. Pharm. Bull. 34, 1194–1197.
Jie, L., Wuliji, O., Wei, L., Zhi-Gang, J., and Hossein, A. G. (2013). Oxidative stress and neurodegenerative disorders. Int. J. Mol. Sci. 14, 24438–24475. doi: 10.3390/ijms141224438
Jin, Q., Cai, Y., Li, S., Liu, H., Zhou, X., Lu, C., et al. (2017). Edaravone –encapsuled agonistic micelles rescue ischemic brain tissue by tuning blood-brain barrier permability. Theranostics 7, 884–898. doi: 10.7150/thno.18219
Jin, Y., Oh, K., Oh, S. I., Baek, H., Kim, S. H., and Park, Y. (2014). Dietary intake of fruits and beta-carotene is negatively associated with amyotrophic lateral sclerosis risk in Koreans: a case-control study. Nutr. Neurosci. 17, 104–108. doi: 10.1179/1476830513Y.0000000071
Johnson, S., and Imai, S. I. (2018). NAD + biosynthesis, aging, and disease. F1000Research 7:132. doi: 10.12688/f1000research.12120.1
Johnson, W. M., Wilson-Delfosse, A. L., and Mieyal, J. J. (2012). Dysregulation of glutathione homeostasis in neurodegenerative diseases. Nutrients 4, 1399–1440. doi: 10.3390/nu4101399
Joseph, J. A., Fisher, D. R., Cheng, V., Rimando, A. M., and Shukitt-Hale, B. (2008). Cellular and behavioral effects of stilbene resveratrol analogues: implications for reducing the deleterious effects of aging. J. Agric. Food Chem. 56, 10544–10551. doi: 10.1021/jf802279h
Joseph, J. A., Shukitt-Hale, B., Brewer, G. J., Weikel, K. A., Wilhelmina, K., and Fisher, D. R. (2011). Differential protection among fractionated blueberry polyphenolic families against DA-, Aβ42 and LPS-induced decrements in Ca2+ buffering in primary hippocampal cells. J. Agric. Food Chem. 58, 8196–8204. doi: 10.1021/jf100144y
Jou, M. J. (2008). Pathophysiological and pharmacological implications of mitochondria-targeted reactive oxygen species generation in astrocytes. Adv. Drug Deliv. Rev. 60, 1512–1526. doi: 10.1016/j.addr.2008.06.004
Julien, J. P. (2007). ALS: astrocytes move in as deadly neighbors. Nat. Neurosci. 10, 535–537. doi: 10.1038/nn0507-535
Jung, C., Higgins, C. M., and Xu, Z. (2002). Mitochondrial electron transport chain complex dysfunction in a transgenic mouse model for amyotrophic lateral sclerosis. J. Neurochem. 83, 535–545.
Juríková, T., Mlcek, J., Sochor, J., and Hegedusová, A. (2015). Polyphenols and their mechanism of action in allergic immune response. Glob. J. Allergy 1, 37–39. doi: 10.17352/2455-8141.000008
Kapetanovic, J. M., Muzzio, M., Huang, Z., Thompson, T. N., and McCormick, D. L. (2011). Pharmacokinetics, oral bioavailability, and metabolic profile of resveratrol and its dimethylether analog, pterostilbene, in rats. Cancer Chemother. Pharmacol. 68, 593–601. doi: 10.1007/s00280-010-1525-4
Karam, C., Yi, J., Xiao, Y., Dhakal, K., Zhang, L., Li, X., et al. (2017). Abscence of physiological Ca 2+ transients is an initial trigger for mitochondrial dysfunction in skeletal muscle following denervation. Skelet. Muscle 7:6. doi: 10.1186/s13395-017-0123-0
Kaufmann, P., Thompson, J. L., Levy, G., Buchsbaum, R., Shefner, J., Krivickas, L. S., et al. (2009). Phase II trial of CoQ10 for ALS finds insufficient evidence to justify phase III. Ann. Neurol. 66, 235–244. doi: 10.1002/ana.21743
Kawahara, Y., Ito, K., Sun, H., Aizawa, H., Kanazawa, I., and Kwak, S. (2004). Glutamate receptors: RNA editing and death of motor neurons. Nature 427:801. doi: 10.1038/427801a
Kaye, W. E., Wagner, L., Wu, R., and Metha, P. (2018). Evaluating the completeness of the national ALS registry, United States. Amyotroph. Lateral Scler. Frontotemporal. Degener. 19, 112–117. doi: 10.1080/21678421.2017.1384021
Kehrer, J. P., and Klotz, L. O. (2015). Free radicals and related reactive species as mediators of tissue injury and disease: implications for health. Crit. Rev. Toxicol. 45, 765–798. doi: 10.3109/10408444.2015.1074159
Kida, Y., and Goligorsky, M. S. (2016). Sirtuins, cell senescence, and vascular aging. Can. J. Cardiol. 32, 634–641. doi: 10.1016/j.cjca.2015.11.022
Killoy, K. M., Harlan, B. A., Pehar, M., Helke, K. L., Johnson, J. A., and Vargas, M. R. (2018). Decreased glutathione levels cause overt motor neuron degeneration in hSOD1WT over-expressing mice. Exp. Neurol. 302, 129–135. doi: 10.1016/j.expneurol.2018.01.004
Kim, D., Nguyen, M. D., Dobbin, M. M., Fischer, A., Sananbenesi, F., Rodgers, J. T., et al. (2007). SIRT1 deacetylase protects against neurodegeneration in models for Alzheimer’s disease and amyotrophic lateral sclerosis. EMBO J. 26, 3169–3179. doi: 10.1038/sj.emboj.7601758
Kim, D. S., Kim, J. Y., and Han, Y. (2012). Curcuminoids in neurodegenerative diseases. Recent Pat. CNS Drug Discov. 7, 184–204.
Kim, G. H., Kim, J. E., Rhie, S. J., and Yoon, S. (2015). The role of oxidative stress in neurodegenerative diseases. Exp. Neurobiol. 24, 325–340. doi: 10.5607/en.2015.24.4.325
Kim, J. S., Ha, T. Y., Ahn, J., Kim, H. J., and Kim, S. (2009). Pterostilbene from Vitis coignetiae rotect H2O2-induced inhibition of gap junctional intercelular communication in rat liver cell line. Food Chem. Toxicol. 47, 404–409. doi: 10.1016/j.fct.2008.11.038
Kim, S. H., Henkel, J. S., Beers, D. R., Sengun, I. S., Simpson, E. P., Goodman, J. C., et al. (2003). PARP expression is increased in astrocytes but decreased in motor neurons in the spinal cord of sporadic ALS patients. J. Neuropathol. Exp. Neurol. 62, 88–103.
Koh, S. H., Kwon, H., Kim, K. S., Kim, J., Kim, M. H., Yu, H. J., et al. (2004). Epigallocatechin gallate prevents oxidative-stress-induced death of mutant Cu/Zn-superoxide dismutase (G93A) motoneuron cells by alteration of cell survival and death signals. Toxicology 202, 213–225. doi: 10.1016/j.tox.2004.05.008
Koh, S. H., Lee, S. M., Kim, H. Y., Lee, K. Y., Kim, H. T., Kim, J., et al. (2006). The effect of epigallocatechin gallate on suppressing disease progression of ALS model mice. Neurosci. Lett. 395, 103–107. doi: 10.1016/j.neulet.2005.10.056
Korkmaz, O. T., Aytan, N., Carreras, I., Choi, J. K., Kowall, N. W., Jenkins, B. G., et al. (2014). 7,8-Dihydroxyflavone improves motor performance and enhances lower motor neuronal survival in a mouse model of amyotrophic lateral sclerosis. Neurosci. Lett. 566, 286–291. doi: 10.1016/j.neulet.2014.02.058
Körner, S., Böselt, S., Thau, N., Rath, K. J., Dengler, R., and Petri, S. (2013). Differential sirtuin expression patterns in amyotrophic lateral sclerosis (ALS) postmortem tissue: neuroprotective or neurotoxic properties of sirtuins in ALS? Neurodegener. Dis. 11, 141–152. doi: 10.1159/000338048
Kosuru, R., Rai, U., Prakash, S., Singh, A., and Singh, S. (2016). Promising therapeutical potential of pterostilbene and its mechanistic insight based on preclinical evidence. Eur. J. Pharmacol. 789, 229–243. doi: 10.1016/j.ejphar.2016.07.046
Krinsky, N. I., and Johnson, E. J. (2005). Carotenoid actions and their relation to health and disease. Mol. Aspects Med. 26, 459–516. doi: 10.1016/j.mam.2005.10.001
Krishnaraj, R. N., Kumari, S. S., and Mukhopadahyay, S. S. (2016). Antagonistic molecular interactions of photosynthetic pigments with molecular disease targets: a new approach to treat AD and ALS. J. Recept. Signal Transduct. Res. 36, 67–71. doi: 10.3109/10799893.2015.1024851
Kulikova, V. A., Gromyko, D. V., and Nikiforov, A. A. (2018). The regulatory role of NAD in human and animal cells. Biochemistry 83, 800–812. doi: 10.1134/S0006297918070040
Kumar, S., and Pandey, A. K. (2015). Free radicals: health implications and their mitigation by herbals. Br. J. Med. Med. Res. 7, 438–457. doi: 10.9734/BJMMR/2015/16284
Kupis, W., Palyga, J., Tomal, E., and Niewiadomska, E. (2016). The role of sirtuins in cellular homeostasis. J. Physiol. Biochem. 72, 371–380. doi: 10.1007/s13105-016-0492-6
Kurtishi, A., Rosen, B., Patil, K. S., Alves, G. W., and Moller, S. G. (2018). Cellular proteostasis in neurodegeneration. Mol. Neurobiol. 56, 3676–3689. doi: 10.1007/s12035-018-1334-z
Kutil, Z., Pribylova, M., Temml, V., Schuster, D., Marsik, P., Susimamani, E., et al. (2015). Effect of dietary stilbenes on 5-lipoxygenase and cyclooxygenase activities in vitro. Int. J. Food Prop. 18, 1471–1477. doi: 10.1080/10942912.2014.903416
Lamuela-Raventós, R. M., Vallverdú-Queralt, A., Jáuregui, O., Martínez-Huélamo, P., and Quifer-Rada, P. (2014). Improved characterization of polyphenols using liquid chromatography. Polyphenols Plants 14, 261–292. doi: 10.1016/B978-0-12-397934-6.00014-0
Lange, K. W., and Li, S. (2018). Resveratrol, pterostilbene and dementia. Biofactors 44, 83–90. doi: 10.1002/biof.1396
Lanska, D. J. (2012). The discovery of niacin, biotin, and panthotenic acid. Ann. Nutr. Metab. 61, 246–253. doi: 10.1159/000343115
Laudati, G., Mascolo, L., Guida, N., Sirabella, R., Pizzorusso, V., Bruzznati, S., et al. (2019). Resveratrol treatment reduces the vulnerability of SH-SY5Y cells and cortical neurons overexpressing SOD1-G93A to Thimerosal toxicity through SIRT1/DREAM/PDYN pathway. Neurotoxicology 71, 6–15. doi: 10.1016/j.neuro.2018.11.009
Lautrup, S., Sinclair, D. A., Mattson, M. P., and Fang, E. F. (2019). NAD+ in brain aging and neurodegenerative disorders. Cell Metab. 30, 630–655. doi: 10.1016/j.cmet.2019.09.001
Lee, Y. A., Yun, B. H., Kim, S. K., Margolin, Y., Dedon, P. C., Geacintov, N. E., et al. (2007). Mechanisms of oxidation of guanine in DNA by carbonate radical anion, a decomposition product of nitrosoperoxycarbonate. Chemistry 13, 4571–4581. doi: 10.1002/chem.200601434
Levites, Y., Amit, T., Youdim, M. B., and Mandel, S. (2002). Involvement of protein kinase C activation and cell survival/cell cycle genes in green tea polyphenol (-)-epigallocatechin 3-gallate neuroprotective action. J. Biol. Chem. 277, 30574–30580. doi: 10.1074/jbc.M202832200
Li, D., Song, T., Yang, L., Wang, X., Yang, C., and Jiang, Y. (2016). Neuroprotective actions of pterostilbene on hypoxic-ischemic brain damage in neonatal rats through upregulation of heme oxygenase-1. Int. J. Dev. Neurosci. 54, 22–31. doi: 10.1016/j.ijdevneu.2016.08.005
Li, J., Li, W., Su, J., Liu, W., Altura, B. T., and Altura, B. M. (2003). Hydrogen peroxide induces apoptosis in cerebral vascular smooth muscle cells: possible relation to neurodegenerative diseases and strokes. Brain Res. Bull. 62, 101–106.
Li, L., Sun, Q., Li, Y., Yang, Y., Chang, T., Man, M., et al. (2015). Overexpression of SIRT1 induced by resveratrol and inhibitor of miR-204 suppresses activation and proliferation of microglia. J. Mol. Neurosci. 56, 858–867. doi: 10.1007/s12031-015-0526-5
Li, Q., Chen, L., Liu, X., Li, X., Cao, Y., Bai, Y., et al. (2018). Pterostilbene inhibits amyloid-β-induced neuroinflammation in a microglia cell line by inactivating the NLRP3/caspase-1 inflammasome pathway. J. Cell. Biochem. 119, 7053–7062. doi: 10.1002/jcb.27023
Li, Q., Spencer, N. Y., Pantazis, N. J., and Engelhardt, J. F. (2011). Alsin and SOD1(G93A) proteins regulate endosomal reactive oxygen specie production by glial cells and proinflammatory pathways responsable for neurotoxicity. J. Biol. Chem. 286, 40151–40162. doi: 10.1074/jbc.M111.279711
Li, Y., Quiang, X., Li, Y., Yang, X., Luo, L., Xiao, G., et al. (2016). Pterostilbene-O-acetamidoalkylbenzylamines derivatives as novel dual inhibitors of cholinesterase with anti-β-amyloid aggregation and antioxidant properties for the treatment of Alzheimer’s disease. Bioorg. Med. Chem. Lett. 26, 2035–2039. doi: 10.1016/j.bmcl.2016.02.079
Li, Y. R., Li, S., and Lin, C. C. (2018). Effect of resveratrol and pterostilbene on aging and logenvity. Biofactors 44, 69–82. doi: 10.1002/biof.1400
Liang, H., and Ward, W. F. (2006). PGC-1alpha: a key regulator of energy metabolism. Adv. Physiol. Educ. 30, 145–151. doi: 10.1152/advan.00052.2006
Liguori, I., Russo, G., Curcio, F., Bulli, G., Aran, L., Della-Morte, D., et al. (2018). Oxidative stress, aging and diseases. Clin. Interv. Aging 13, 757–772. doi: 10.2147/CIA.S158513
Lin, M. T., and Beal, M. F. (2006). Mitochondrial dysfunction and oxidative stress in neurodegenerative diseases. Nature 443, 787–795. doi: 10.1038/nature05292
Lin, Y. J., Ding, Y., Wu, J., and Ning, B. T. (2016). Pterostilbene as treatment for severe acute pancreatitis. Genet. Mol. Res. 15:15038330. doi: 10.4238/gmr.15038330
Liochev, S. I. (2013). Reactive oxygen species and the free radical theory of aging. Free Radic. Biol. Med. 60, 1–4. doi: 10.1016/j.freeradbiomed.2013.02.011
Liu, J., Lillo, C., Jonsson, P. A., Vande Velde, C., Ward, C. M., Miller, T. M., et al. (2004). Toxicity of familial ALS-linked SOD1 mutants from selective recruitment to spinal mitochondria. Neuron 43, 5–17. doi: 10.1016/j.neuron.2004.06.016
Liu, W., Zhai, Y., Heng, X., Che, F. Y., Chen, W., Sun, D., et al. (2016). Oral bioavailability of curcumin: problems and advancements. J. Drug Target. 24, 694–702. doi: 10.3109/1061186X.2016.1157883
Liu, X., Yang, X., Han, L., Ye, F., Liu, M., Fan, W., et al. (2017). Pterostilbene alleviates polymicrobial sepsis-induced liver injury: possible role of SIRT1signaling. Int. Immunopharmacol. 49, 50–59. doi: 10.1016/j.intimp.2017.05.022
Loeffler, J. P., Picchiarelli, G., Dupuis, L., and Gonzalez de Aguilar, J. L. (2016). The role of skeletal muscle in amyotrophic lateral sclerosis. Brain Pathol. 26, 227–236. doi: 10.1111/bpa.12350
LoGerfo, A., Chico, L., Borgia, L., Petrozzi, L., Rocchi, A., D’Amelio, A., et al. (2014). Lack of association between nuclear factor erythroid-derived 2-like 2 promoter gene polymorphisms and oxidative stress biomarkers in amyotrophic lateral sclerosis patients. Oxid. Med. Cell. Longev. 2014:432626. doi: 10.1155/2014/432626
Loizzo, S., Pieri, M., Ferri, A., Carrì, M. T., Zona, C., Fortuna, A., et al. (2010). Dynamic NAD(P)H post-synaptic autofluorescence signals for the assessment of mitochondrial function in a neurodegenerative disease: monitoring the primary motor cortex of G93A mice, an amyotrophic lateral sclerosis model. Mitochondrion 10, 108–114. doi: 10.1016/j.mito.2009.11.001
Longecker, M. P., Kamel, F., Umbach, D. M., Munsat, T. L., Shefner, J. M., Landsell, L. W., et al. (2000). Dietary intake of calcium, magnesium and antioxidants in relation to risk of amyotrophic lateral sclerosis. Neuroepidemiology 19, 210–216. doi: 10.1159/000026258
López-Otín, C., Blasco, M. A., Partridge, L., Serrano, M., and Kroemer, G. (2013). The hallmarks of aging. Cell 153, 1194–1217. doi: 10.1016/j.cell.2013.05.039
Lu, J., Duan, W., Guo, Y., Jiang, H., Li, Z., Huang, J., et al. (2012). Mitochondrial dysfunction in human TDP-43 transfected NSC34 cell lines and the protective effect of dimethoxy curcumin. Brain Res. Bull. 89, 185–190. doi: 10.1016/j.brainresbull.2012.09.005
Mackenzie, I. R. A., and Rademakers, R. (2008). The role of TDP-43 in amyotrophic lateral sclerosis and frontotemporal dementia. Curr. Opin. Neurol. 21, 693–700. doi: 10.1097/WCO.0b013e3283168d1d
Magrané, J., Cortez, C., Gan, W. B., and Manfredi, G. (2014). Abnormal mitochondrial transport and morphology are common pathological denominators in SOD1 and TDP43 ALS mouse models. Hum. Mol. Genet. 23, 1413–1424. doi: 10.1093/hmg/ddt528
Mancuso, R., del Valle, J., Modol, L., Martinez, A., Granado-Serrano, A. B., Ramírez-Núñez, O., et al. (2014a). Resveratrol improves motoneuron function and extends survival in SOD1(G93A) ALS mice. Neurotherapeutics 11, 419–432. doi: 10.1007/s13311-013-0253-y
Mancuso, R., del Valle, J., Morell, M., Pallás, M., Osta, R., and Navarro, X. (2014b). Lack of synergistic effect of resveratrol and sigma-1 receptor agonist (PRE-084) in SOD1G93A ALS mice: overlapping effects or limited therapeutic opportunity? Orphanet J. Rare Dis. 9:78. doi: 10.1186/1750-1172-9-78
Mandel, S., Reznichenko, L., Amit, T., and Youdim, M. B. (2003). Green tea polyphenol (-)-epigallocatechin-3-gallate protects rat PC12 cells from apoptosis induced by serum withdrawal independent of P13-Akt pathway. Neurotox. Res. 5, 419–424.
Mandel, S. A., Avramovich-Tirosh, Y., Reznichenko, L., Zheng, H., Weinreb, O., Amit, T., et al. (2005). Multifunctional activities of green tea catechins in neuroprotection. Modulation of cell survival genes, iron-dependent oxidative stress and PKC signaling pathway. Neurosignals 14, 46–60. doi: 10.1159/000085385
Mansuri, M. L., Parihar, P., Solanki, I., and Parihar, M. S. (2014). Flavonoids in modulation of cell survival signalling pathways. Genes Nutr. 9:400. doi: 10.1007/s12263-014-0400-z
Mantilla, C. B., and Ermilov, L. G. (2012). The novel TrkB receptor agonist 7,8-dihydroxyflavone enhances neuromuscular transmission. Muscle Nerve 45, 274–276. doi: 10.1002/mus.22295
Marin, B., Boumedine, F., Logroscino, G., Couratier, P., Babron, M. C., Leutenegger, A. L., et al. (2017). Variation in worldwide incidence of amyotrophic lateral sclerosis: a meta-analysis. Int. J. Epidemiol. 46, 57–74. doi: 10.1093/ije/dyw061
Martens, C. R., Denman, B. A., Mazzo, M. R., Armstrong, M. L., Reisdorph, N., McQueen, M. B., et al. (2018). Chronic nicotinamide riboside supplementation is well-tolerated and elevates NAD+ in healthy middle-aged and older adults. Nat. Commun. 9:1286. doi: 10.1038/s41467-018-03421-7
Martin, L. J. (2010). Mitochondrial patohiology in Parkinson’s disease and amyotrophic lateral sclerosis. J. Alzheimers Dis. 20(Suppl. 2), S335–S356. doi: 10.3233/JAD-2010-100348
Massaad, C. A., and Klann, E. (2011). Reactive oxygen species in the regulation of synaptic plasticity and memory. Antioxid. Redox. Signal. 14, 2013–2054. doi: 10.1089/ars.2010.3208
Matthews, R. T., Yang, L., Browmne, S., Baik, M., and Beal, M. F. (1998). Coenzyme Q10 administration increases brain mitochondrial concentrations and exerts neuroprotective effects. Proc. Natl. Acad. Sci. U.S.A. 95, 8892–8897.
Mattiazzi, M., D’Aurelio, M., Gajewski, C. D., Martushova, K., Kiaei, M., Beal, M. F., et al. (2002). Mutated human SOD1 causes dysfunction of oxidative phosphorylation in mitocondria of transgenic mice. J. Biol. Chem. 277, 29626–29633. doi: 10.1074/jbc.M203065200
McCombe, P. A., and Henderson, R. D. (2010). Effects of gender in amyotrophic lateral sclerosis. Gend. Med. 7, 557–570. doi: 10.1016/j.genm.2010.11.010
Mehta, P., Kaye, W., Raymond, J., Wu, R., Larson, T., Punjani, R., et al. (2018). Prevalence of amyotrophic lateral sclerosis - United States, 2014. Morb. Mortal. Wkly. Rep. 67, 216–218. doi: 10.15585/mmwr.mm6707a3
Mendelsohn, A. R., and Larrick, J. W. (2014). Partial reversal of skeletal muscle aging by restoration of normal NAD+ levels. Rejuvenation Res. 17, 62–69. doi: 10.1089/rej.2014.1546
Mendelsohn, A. R., and Larrick, J. W. (2017). The NAD+/PARP1/SIRT1 axis in aging. Rejuvenation Res. 20, 244–247. doi: 10.1089/rej.2017.1980
Menzies, F. M., Ince, P. G., and Shaw, P. J. (2002). Mitochondrial involvement damage in patients with sporadic amyotrophic lateral sclerosis. Neurochem. Int. 40, 543–551. doi: 10.1016/S0197-0186(01)00125-5
Mergenthaler, P., Lindauer, U., Dienel, G. A., and Meisel, A. (2013). Sugar for the brain: the role of glucose in physiological and pathological brain function. Trends Neurosci. 36, 587–597. doi: 10.1016/j.tins.2013.07.001
Michal-Freedman, D., Kuncl, R. W., Weinstein, S. J., Malila, N., Virtamo, J., and Albanes, D. (2013). Vitamin E serum levels and controlled supplementation and risk of amyotrophic lateral sclerosis. Amyotroph. Lateral Scler. Frontotemporal. Degener. 14, 246–251. doi: 10.3109/21678421.2012.745570
Mikstacka, R., Rimando, A. M., and Ignatowicz, E. (2010). Antioxidant effect of trans-resveratrol, pterostilbene, quercetin and their combinations in human erythrocytes in vitro. Plant Foods Hum. Nutr. 65, 57–63. doi: 10.1007/s11130-010-0154-8
Milatovic, D., Montine, T. J., and Aschner, M. (2011). Measurement of isoprostanes as markers of oxidative stress. Methods Mol. Biol. 758, 195–204. doi: 10.1007/978-1-61779-170-3_13
Miller, A. A., Budzyn, K., and Sobey, G. G. (2010). Vascular dysfunction in cerebrovascular disease: mechanisms and therapeutic intervention. Clin. Sci. 119, 1–17. doi: 10.1042/CS20090649
Miller, E., Morel, A., Saso, L., and Saluk, J. (2014). Isoprostanes and neuroprostanes as biomarkers of oxidative stress in neurodegenerative diseases. Oxid. Med. Cell. Longev. 2014:572491. doi: 10.1155/2014/572491
Miller, M. G., and Shukitt-Hale, B. (2012). Berry fruit enhances beneficial signaling in the brain. J. Agric. Food. Chem. 60, 5709–5715. doi: 10.1021/jf2036033
Miller, R. G., Mitchell, J. D., and Moore, D. H. (2012). Riluzole for amyotrophic lateral sclerosis (ALS)/motor neuron disease (MND). Cochrane Database Syst. Rev. 14:CD001447. doi: 10.1002/14651858.CD001447.pub3
Milne, G. L., Sanchez, S. C., Musiek, E. S., and Morrow, J. D. (2007). Quantification of F2-isoprostanes as biomarker of oxidative stress. Nat. Protoc. 2, 221–226. doi: 10.1038/nprot.2006.375
Minto, C., Vecchio, M. G., Lamprecht, M., and Gregori, D. (2017). Definition of a tolerable upper intake level of niacin: a systematic review and meta-analysis of the doe-dependent effects of nicotinamide and nicotinic acid supplementation. Nutr. Rev. 75, 471–490. doi: 10.1093/nutrit/nux011
Mitchell, J., and Borasio, G. (2007). Amyotrophic lateral sclerosis. Lancet 369, 2031–2041. doi: 10.1016/S0140-6736(07)60944-1
Mitsumoto, H., Santella, R. M., Xinhau, L., Bogdanov, M., Zipprich, J., Hui-Chen, W., et al. (2008). Oxidative Stress biomarkers in sporadic ALS. Amyotroph. Lateral Scler. 9, 177–183. doi: 10.1080/17482960801933942
Miyamoto, N., Maki, T., Pham, L. D., Hayakawa, K., Seo, J. H., Mandeville, E. T., et al. (2013). Oxidative stress interferes with White matter renewal after prolonged cerebral hypoperfusion in mice. Stroke 44, 3516–3521. doi: 10.1161/STROKEAHA.113.002813
Molina, J. A., De Bustos, F., Jiménez-Jiménez, F. J., Gómez-Escalonilla, C., García-Redondo, A., Esteban, J., et al. (2000). Serum levels of coenzyme Q10 in patients with amyotrophic lateral sclerosis. J. Neural Transm. 107, 1021–1026. doi: 10.1007/s007020070050
Moon, Y., Choi, Y. J., Kim, J. O., and Han, S. H. (2018). Muscle profile and cognition in patients with Alzheimer’s disease dementia. Neurol. Sci. 39, 1861–1866. doi: 10.1007/s10072-018-3505-0
Morató, L., Bertini, E., Verrigini, D., Ardissone, A., Ruiz, M., Ferrer, I., et al. (2014). Mitochondrial dysfunction in central nervous system white matters disorders. Glia 62, 1878–1894. doi: 10.1002/glia.22670
Moreira, P. I., Duarte, A. I., Santos, M. S., Rego, A. C., and Oliveira, C. R. (2009). An integrative view of the role of oxidative stress, mitocondria and insulin in Alzheimer’s disease. J. Alzheimers. Dis. 16, 741–761. doi: 10.3233/JAD-2009-0972
Mouchiroud, L., Houtkooper, R. H., Moullan, N., Katsyuba, E., Ryu, D., Cantó, D., et al. (2013). The NAD(+)/sirtuin pathway modulates longevity through activation of mitochondrial UPR and FOXO signaling. Cell 154, 430–441. doi: 10.1016/j.cell.2013.06.016
Moujalled, D., Grubman, A., Acevedo, K., Yang, S., Ke, Y. D., Moujalled, D. M., et al. (2017). TDP-43 mutations causing amyotrophic lateral sclerosis are associated with altered expression of RNA-binding protein hnRNP K and affect the Nrf2 antioxidant pathway. Hum. Mol. Genet. 26, 1732–1746. doi: 10.1093/hmg/ddx093
Murphy, M. P. (2009). How mitochondria produce reactive oxygen species. Biochem. J. 417, 1–13. doi: 10.1042/BJ20081386
Nagase, M., Yamamoto, Y., Miyazaki, Y., and Yoshino, H. (2016). Increased oxidative stress in patients with amyotrophic lateral sclerosis and the effect of edaravone administration. Redox. Rep. 21, 104–112. doi: 10.1179/1351000215Y.0000000026
Naik, B., Nirwane, A., and Majumdar, A. (2017). Pterostilbene ameliorates intracerebroventricular streptozotocin induced memory decline in rats. Cogn. Neurodyn. 11, 35–49. doi: 10.1007/s11571-016-9413-1
Neal, M., and Richardson, J. R. (2018). Time to get personal: a framework for personalized targeting of oxidative stress in neurotoxicity and neurodegenerative disease. Curr. Opin. Toxicol. 7, 127–132. doi: 10.1016/j.cotox.2018.02.003
Neves, A. R., Lúcio, M., Lima, J. L. C., and Reis, S. (2012). Resveratrol in medicinal chemistry: a critical review of its pharmacokinetics, drug-delivery, and membrana interactions. Curr. Med. Chem. 19, 1663–1681.
Nie, G., Gao, Y., and Zhao, B. (2002). Protective effects of green tea polyphenols and their major component, (-)-epigallocatechin-3-gallate (EGCG), on 6-hydroxydopamine-induced apoptosis in PC12 cells. Redox. Rep. 7, 171–177. doi: 10.1179/135100002125000424
Niedzielska, E., Smaga, I., Gawlik, M., Moniczewski, A., Stankowicz, P., Pera, J., et al. (2016). Oxidative stress in neurodegenerative diseases. Mol. Neurobiol. 53, 4094–4125. doi: 10.1007/s12035-015-9337-5
Niedzwiecki, A., Roomi, M. W., Kalinovsky, T., and Rath, M. (2016). Anticancer efficacy of polyphenols and their combinations. Nutrients 8:552. doi: 10.3390/nu8090552
Nieves, J. W., Gennings, C., Factor- Litvak, P., Hupf, J., Singleton, J., Sharf, V., et al. (2016). Association between dietary intake and function in amyotrophic lateral sclerosis. JAMA Neurol. 73, 1425–1432. doi: 10.1001/jamaneurol.2016.3401
Niki, E. (2014). Biomarkers in lipid peroxidation in clinical material. Biochim. Biophys. Acta 1840, 809–817. doi: 10.1016/j.bbagen.2013.03.020
Nikiforov, A., Dolle, C., Niere, M., and Ziegler, M. (2011). Pathways and subcellular compartmentation of NAD biosynthesis in human cells: from entry of extracelullar precursors to mitochondrial NAD generation. J. Biol. Chem. 286, 21767–21778. doi: 10.1074/jbc.M110.213298
Nikolic-Kokic, A., Stevic, Z., Blagojevic, D., Davidovic, B., Jones, D. R., and Spasic, M. B. (2006). Alterations in anti-oxidative defence enzymes in erythrocytes sporadic amyotrophic lateral sclerosis (SALS) and familial ALS patients. Clin. Chem. Lab. Med. 44, 589–593. doi: 10.1515/CCLM.2006.111
Nisar, N., Li, L., Lu, S., Khin, N. C., and Pogson, B. J. (2015). Carotenoid metabolism in plants. Mol. Plant 8, 68–82. doi: 10.1016/j.molp.2014.12.007
Nizynski, B., Dzwolak, W., and Nieznanski, K. (2017). Amyloidogenesis of Tau protein. Protein Sci. 26, 2126–2150. doi: 10.1002/pro.3275
Nour, Y., Nawal, A., Majed, J., and Chantal, M. (2018). The immunomodulatory and anti-inflammatory role of polyphenols. Nutrients 10:1618. doi: 10.3390/nu10111618
Nunomura, A., Honda, K., Takeda, A., Hirai, K., Zhu, X., Smith, M. A., et al. (2006). Oxidative damage to RNA in neurodegenerative diseases. J. Biomed. Biotechnol. 2006:82323. doi: 10.1155/JBB/2006/82323
Oakey, L. A., Fletcher, R. S., Elhassan, Y. S., Cartwright, D. M., Doig, C. L., Garten, A., et al. (2018). Metabolic tracing reveals novel adaptations to skeletal muscle cell energy production pathways in response to NAD + depletion. Wellcome Open Res. 3:147. doi: 10.12688/wellcomeopenres.14898.2
Oberstad, M., Stieler, J., Simpong, D. L., Römuß, U., Urban, N., Schaefer, M., et al. (2018). TDP-43 self-interaction is modulated by redox-active compounds Auranofin, Chelerythrine and Riluzole. Sci. Rep. 8:2248. doi: 10.1038/s41598-018-20565-0
O’Callaghan, C., and Vassilopoulos, A. (2017). Sirtuins at the crossroads of stemness, aging, and cáncer. Aging Cell 16, 1208–1218. doi: 10.1111/acel.12685
Okada, M., Yamashita, S., Uevama, H., Ishizaki, M., Maeda, Y., and Ando, Y. (2018). Long-term effects of edaravone on survival of patients with amyotrophic lateral sclerosis. Neurol. Sci. 11, 11–14. doi: 10.1016/j.ensci.2018.05.001
Okamoto, K., Kihira, T., Kobashi, G., Washio, M., Sasaki, S., Yokoyama, T., et al. (2009). Fruit and vegetable intake and risk of amyotrophic lateral sclerosis in Japan. Neuroepidemiology 32, 251–256. doi: 10.1159/000201563
Oliviero, F., Scanu, A., Zamudio-Cuevas, Y., Punzi, L., and Spinella, P. (2018). Anti-inflammatory effects of polyphenols in arthritis. J. Sci. Food Agric. 98, 1653–1659. doi: 10.1002/jsfa.8664
Orrell, R. W., Lane, J. M., and Ross, M. A. (2004). Antioxidant treatment for amyotrophic lateral sclerosis/motor neuron disease. Cochrane Database Syst. Rev. 18:CD002829. doi: 10.1002/14651858.CD002829.pub2
Pan, L., Yoshii, Y., Otomo, A., Ogawa, H., Iwasaki, Y., Shang, H. F., et al. (2012). Different human copper-zinc superoxide dismutase mutants, SOD1, and SOD1 exert distinct harmful effect on gross phenotype in mice. PLoS One 7:e33409. doi: 10.1371/journal.pone.0033409
Pan, M. H., Chang, Y. H., Tsai, M. L., Lai, C. S., Ho, S. Y., Badmaev, V., et al. (2008). Pterostilbene suppressed lipopolysaccharide-induced up-expression of iNOS and COX-2 in murine macrophages. J. Agric. Food Chem. 56, 7502–7509. doi: 10.1021/jf800820y
Pandi-Perumal, S. R., BaHammam, A. S., Brown, G. M., Spence, D. W., Bharti, V. K., Kaur, C., et al. (2013). Melatonin antioxidative defense: therapeutical implications for aging and neurodegenerative processes. Neurotox. Res. 23, 267–300. doi: 10.1007/s12640-012-9337-4
Panickar, K. S., Polansky, M. M., and Anderson, R. A. (2009). Green tea polyphenols attenuate glial swelling and mitochondrial dysfunction following oxygen-glucose deprivation in cultures. Nutr. Neurosci. 12, 105–113. doi: 10.1179/147683009X423300
Papadimitriou, D., Le Verche, V., Jacquier, A., Ikiz, B., Przedborski, S., and Re, D. B. (2010). Inflammation in ALS and SMA: sorting out the good from the evil. Neurobiol. Dis. 37, 493–502. doi: 10.1016/j.nbd.2009.10.005
Parakh, S., Spencer, D. M., Halloran, M. A., Soo, K. Y., and Atkin, J. D. (2013). Redox regulation in amyotrophic lateral sclerosis. Oxid. Med. Cell. Longev. 2013:408681. doi: 10.1155/2013/408681
Park, J. H., Hong, Y. H., Kim, H. J., Kim, S. M., Park, K. S., Sung, J. J., et al. (2007). Pyruvate slows disease progression in a G93A SOD1 mutant transgenic mouse model. Neurosci. Lett. 413, 265–269. doi: 10.1016/j.neulet.2006.11.058
Paul, S., Rimando, A. M., Lee, H. J., Ji, Y., Reddy, B. S., and Suh, N. (2009). Anti-inflammatory action of pterostilbene is mediated through the p38 mitogen-activated protein kinase pathway in colon cancer cells. Cancer Prev. Res. 2, 650–657. doi: 10.1158/1940-6207.CAPR-08-0224
Payne, B. A., and Chinnery, P. F. (2015). Mitochondrial dysfunction in aging: much progress but many unresolved questions. Biochim. Biophys. Acta 1847, 1347–1353. doi: 10.1016/j.bbabio.2015.05.022
Pehar, M., Beeson, G., Beeson, C. C., Johnson, J. A., and Vargas, M. R. (2014). Mitochondria-targeted catalase reverts the neurotoxicity of h SOD1G(9)(3) A astrocytes without extending the survival of ALS-linked mutant hSOD1 mice. PLoS One 9:e103438. doi: 10.1371/journal.pone.0103438
Perluigi, M., Coccia, R., and Butterfield, D. A. (2012). 4-Hydroxy-2-Nonenal, a reactive product of lipid peroxidation, and neurodegenerative diseases: a toxic combination illuminated by redox proteomics studies. Antioxid. Redox Signal. 17, 1590–1609. doi: 10.1089/ars.2011.4406
Peters, O. M., Ghasemi, M., and Brown, R. H. (2015). Emerging mechanisms of molecular pathology in ALS. J. Clin. Invest. 125, 1767–1779. doi: 10.1172/JCI71601
Petrov, D., Mansfield, C., Moussy, A., and Hermine, O. (2017). ALS clinical trials review: 20 years of failure. Are we any closer to registering a new treatment? Front. Aging Neurosci. 9:68. doi: 10.3389/fnagi.2017.00068
Petrozzi, L., Ricci, G., Giglioli, N. J., Sicialiano, G., and Mancuso, M. (2007). Mitochondria and neurodegeneration. Biosci. Rep. 27, 87–104. doi: 10.1007/s10540-007-9038-z
Pfohl, S. R., Halicek, M. T., and Mitchell, C. S. (2015). Characterization of the contribution of genetic brackground and gender to disease progression in the SOD1 G93A mouse model of amyotrophic lateral sclerosis: a meta-analysis. J. Neuromuscul. Dis. 2, 137–150. doi: 10.3233/JND-140068
Phaniendra, A., Jestadi, D. B., and Periyasamy, L. (2015). Free radicals: properties, sources, targets, and their implication in various diseases. Indian J. Clin. Biochem. 30, 11–26. doi: 10.1007/s12291-014-0446-0
Pickles, S., Destroismaisons, L., Peyrard, S. L., Cadot, S., Rouleau, G. A., Brown, R. H., et al. (2013). Mitochondrial damage revealed by immunoselection for ALS-linked misfolded SOD1. Hum. Mol. Genet. 22, 3947–3959. doi: 10.1093/hmg/ddt249
Pickles, S., Semmler, H. R., Broom, L., Destroismaisons, L., Legroux, N., Arbour, E., et al. (2016). ALS-linked misfolded SOD1 species have divergent impacts on mitocondria. Acta Neuropathol. Commun. 4:43. doi: 10.1186/s40478-016-0313-8
Pizzino, G., Irrera, N., Cucinotta, M., Pallio, G., Mannino, F., Arcoraci, V., et al. (2017). Oxidative stress: harms and benefics for human health. Oxid. Med. Cell. Longev. 2017:8416763. doi: 10.1155/2017/8416763
Polimeni, G., Esposito, E., Bevelacqua, V., Guarneri, C., and Cuzzocrea, S. (2014). Role of melatonin supplementation in neurodegenerative disorders. Front. Biosci. 19, 429–446. doi: 10.2741/4217
Poljsak, B., and Milisav, I. (2016). NAD+ as the link between oxidative stress, inflammation, caloric restriction, exercise, DNA repair, longevity, and health span. Rejuvenation Res. 19, 406–413. doi: 10.1089/rej.2015.1767
Pollari, E., Goldsteins, G., Bart, G., Koistinaho, J., and Giniatullin, R. (2014). The role of oxidative stress in degeneration of the neuromuscular junction in amyotrophic lateral sclerosis. Front. Cell. Neurosci. 8:131. doi: 10.3389/fncel.2014.00131
Popa-Wagner, A., Mitran, S., Sivanesan, S., Chang, E., and Buga, A. M. (2013). ROS and brain diseases: the good, the bad and the ugly. Oxid. Med. Cell. Longev. 2013:963520. doi: 10.1155/2013/963520
Porquet, D., Casadesus, C., Bayod, S., Vicente, A., Canudas, A. M., Vilaplana, J., et al. (2013). Dietary resveratrol prevents Alzheimer’s markers and increases life span in SAMP8. Age 35, 1851–1865. doi: 10.1007/s11357-012-9489-4
Poulose, S. M., Miller, M. G., and Shukitt-Hale, B. (2014). Role of walnuts in maintaining brain health with age. J. Nutr. 144(4 Suppl.), 561S–566S. doi: 10.3945/jn.113.184838
Poulose, S. M., Thanthaeng, N., Miller, M. G., and Shukitt-Hale, B. (2015). Effects of pterostilbene and resveratrol on brain and behaviour. Neurochem. Int. 89, 227–233. doi: 10.1016/j.neuint.2015.07.017
Prousky, J., Millman, C. G., and Kikland, J. B. (2011). Pharmacologic use of niacin. J. Evid. Based Intedr. Med. 16, 91–101. doi: 10.1177/2156587211399579
Przedborski, S., Donaldson, D. M., Murphy, P. L., Hirsch, O., Lange, D., Naini, A. B., et al. (1996). Blood superoxide dismutase, catalase and glutathione peroxidase activities in familial and sporadic amyotrophic lateral sclerosis. Neurodegeneration 5, 57–64.
Qiu, X., Brown, K., Hirschey, M. D., Verdin, E., and Chen, D. (2010). Calorie restriction reduces oxidative stress by SIRT3-mediated SOD2 activation. Cell Metab. 12, 662–667. doi: 10.1016/j.cmet.2010.11.015
Quin, W., Haroutunian, V., Katsel, P., Cardozo, C. P., Ho, L., Buxbaum, J. D., et al. (2009). PGC-1 alpha expression decreases in the Alzheimer diasease brain as a function of dementia. Arch. Neurol. 66, 352–361. doi: 10.1001/archneurol.2008.588
Radak, Z., Zhao, Z., Goto, S., and Koltai, E. (2011). Age-associated neurodegeneration and oxidative damage to lipids, proteins and DNA. Mol. Aspects Med. 32, 305–315. doi: 10.1016/j.mam.2011.10.010
Raff, M. C., Whitmore, A. V., and Finn, J. T. (2002). Axonal self-destruction and neurodegeneration. Science 296, 868–871. doi: 10.1126/science.1068613
Rajman, L., Chwalek, K., and Sinclair, D. A. (2018). Therapeutic potential of NAD-boosting molecules: the in vivo evidence. Cell Metab. 27, 529–547. doi: 10.1016/j.cmet.2018.02.011
Rakotoarisoa, M., and Angelova, A. (2018). Amphiphilic nanocarrier systems for curcumin delivery in neurodegenerative disorders. Medicines 5:E126. doi: 10.3390/medicines5040126
Ramamoorthy, M., Sykora, P., Scheibye-Knudsen, M., Dunn, C., Kasmer, C., Zhang, Y., et al. (2012). Sporadic Alzheimer disease fibroblasts display an oxidative stress pehnotype. Free Radic. Biol. Med. 53, 1371–1380. doi: 10.1016/j.freeradbiomed.2012.07.018
Raskin, J., Cummings, J., Hardy, J., Schuh, K., and Dean, R. A. (2015). Neurobiology of Alzheimer’s Disease: integrated molecular, physiological, anatomical, biomarker, and cognitive dimensions. Curr. Alzheimer Res. 12, 712–722.
Rass, U., Ahel, I., and West, S. C. (2007). Defective DNA repair and neurodegenerative disease. Cell 130, 991–1004. doi: 10.1016/j.cell.2007.08.043
Ray, P. D., Huang, B. W., and Tsuji, Y. (2012). Reactive oxygen species (ROS) homeostasis and redox regulation in cellular signaling. Cell Signal. 24, 981–990. doi: 10.1016/j.cellsig.2012.01.008
Rechtmann, L., Wagner, L., Kaye, W., and Villanacci, J. (2015). Update prevalence and demographic characteristics for ALS cases in Texas, 2009-2011. South. Med. J. 108, 483–486. doi: 10.14423/SMJ.0000000000000324
Regueiro, J., Sánchez-González, C., Vallverdú-Queralt, A., Simal-Gándara, J., Lamuela-Raventós, R., and Izquierdo-Pulido, M. (2014). Comprehensive identification of walnut polyphenols by liquid chromatography coupled to linear ion trap– Orbitrap mass spectrometry. Food Chem. 152, 340–348. doi: 10.1016/j.foodchem.2013.11.158
Reinisalo, M., Karlund, A., Koskela, A., Kaarniranta, K., and Karjalainen, R. O. (2015). Polyphenol stilbenes: molecular mechanisms of defence against oxidative stress and aging-related diseases. Oxid. Med. Cell. Longev. 2015:340520. doi: 10.1155/2015/340520
Remsberg, C. M., Yáñez, J. A., Ohgami, Y., Vega-Villa, K. R., Rimando, A. M., and Davies, N. M. (2008). Pharmacometrics of pterostilbene: preclinical pharmacokinetics and metabolism, anticancer, antiinflammatory, antioxidant and analgesic activity. Phytother. Res. 22, 169–179. doi: 10.1002/ptr.2277
Renaud, J., and Martinoli, M. G. (2019). Consideration for the use of polyphenols as therapies in neurodegenerative diseases. Int. J. Mol. Sci. 20:E1883. doi: 10.3390/ijms20081883
Rhee, S. G., Chang, T. S., Bae, Y. S., Lee, S. R., and Kang, S. W. (2003). Cellular regulation by hydrogen peroxide. J. Am. Soc. Nephrol. 14(8 Suppl. 3), S211–S215. doi: 10.1097/01.asn.0000077404.45564.7e
Richardson, K., Allen, S. P., Mortiboys, H., Grierson, A. J., Wharton, S. B., Ince, P. G., et al. (2013). The effect of SOD1 mutation on cellular bioenergetics profile and viability in response to oxidative stress and influence of mutation-type. PLoS One 8:e68256. doi: 10.1371/journal.pone.0068256
Riche, D. M., McEwen, C. L., Riche, K. D., Sherman, J. J., Wofford, M. R., Deschamp, D., et al. (2013). Analysis of safety from a human clinical trial with pterostilbene. J. Toxicol. 2013:463595. doi: 10.1155/2013/463595
Rimando, A. M., Cuendet, M., Desmarchelier, C., Mehta, R. G., Pezzuto, J. M., and Duko, S. O. (2002). Cancer chemopreventive and antioxidant activities of pterostilbene, a naturally ocurring analogue of resveratrol. J. Agric. Food Chem. 50, 3453–3457.
Rivière, C., Pawlus, A. D., and Mérillon, J. M. (2012). Natural stilbenoids: distribution in the plant kingdom and chemotaxonomic interest in vitaceae. Nat. Prod. Rep. 29, 1317–1333. doi: 10.1039/c2np20049j
Robberecht, W. (2000). Oxidative stress in amyotrophic lateral sclerosis. J. Neurol. 247(Suppl. 1), 1–6.
Rodgers, J. T., Lerin, C., Haas, W., Gygi, S. P., Spiegelman, B. M., and Puigserver, P. (2005). Nutrient control of glucose homeostasis through a complex of PGC-1α and SIRT1. Nature 434, 113–118. doi: 10.1038/nature03354
Rogers, N. M., Seeger, F., Garcin, E. D., Roberts, D. D., and Isenberg, J. S. (2014). Regulation of soluble guanylate cyclase by matricellular thrombospondins: implications for blood flow. Front. Physiol. 5:134. doi: 10.3389/fphys.2014.00134
Rojas, F., González, D., Cortes, N., Ampuero, E., Hernández, D. E., Fritz, E., et al. (2015). Reactive oxygen species trigger motoneuron death in non-cell-autonmous models of ALS through activation of c-Abl signaling. Front. Cell. Neurosci. 9:203. doi: 10.3389/fncel.2015.00203
Romano, A. D., Serviddio, G., de Matthaeis, A., Bellanti, F., and Vendemiale, G. (2010). Oxidative stress and aging. J. Nephrol. 23(Suppl. 15):36.
Ronnett, G. B., Ramamurthy, S., Kleman, A. M., Landree, L. E., and Aja, S. (2009). AMPK in the brain: its roles in energy balance and neuroprotection. J. Neurochem. 109, 17–23. doi: 10.1111/j.1471-4159.2009.05916.x
Rossi, M., Caruso, F., Antonioletti, R., Viglianti, A., Traversi, G., Leone, S., et al. (2013). Scavenging of hydroxyl radical by resveratrol and related natural stilbenes after hydrogen peroxide attack on DNA. Chem. Biol. Interact. 206, 175–185. doi: 10.1016/j.cbi.2013.09.013
Rothstein, J. D. (2017). Edaravone: a new drug approved for ALS. Cell 171:725. doi: 10.1016/j.cell.2017.10.011
Rüb, U., Stratmann, K., Heinsen, H., Seidel, K., Bouzrou, M., and Korf, H. W. (2017). Alzheimer’s disease: characterization of the brain sites of the initial tau cytoskeletal pathology will improve the success of novel immunological anti-tau treatment approaches. J. Alzheimers Dis. 57, 683–696. doi: 10.3233/JAD-161102
Ruiz, M. J., Fernández, M., Picó, Y., Mañes, J., Asensi, M., Carda, C., et al. (2009). Dietary administration of high doses of pterostilbene and quercetin to mice is not toxic. J. Agric. Food Chem. 57, 3180–3186. doi: 10.1021/jf803579e
Rusconi, M., and Conti, A. (2010). Theobroma cacao L., the food of the gods: a scientific approach beyond myths and claims. Pharmacol. Res. 61, 5–13. doi: 10.1016/j.phrs.2009.08.008
Ryu, D., Zhang, H., Ropelle, E. R., Sorrentino, V., Mázala, D. A., Mouchiroud, L., et al. (2016). NAD+ repletion improves muscle function in muscular dystrophy and counters global PARylation. Sci. Transl. Med. 8:361ra139. doi: 10.1126/scitranslmed.aaf5504
Rzheshevsky, A. V. (2014). Decrease in ATP biosynthesis and dysfunction of biological membranes. Two possible key mechanisms of phenoptosis. Biochemistry 79, 1056–1068. doi: 10.1134/S0006297914100071
Saccon, R. A., Bunton-Stasyshyn, R. K., Fisher, E. M., and Fratta, P. (2013). Is SOD1 loss of function involved in amyotrophic lateral sclerosis? Brain 136(Pt 8), 2342–2358. doi: 10.1093/brain/awt097
Salisbury, D., and Bronas, U. (2015). Reactive oxygen and nitrogen species: impact on endothelial dysfunction. Nurs. Res. 64, 53–66. doi: 10.1097/NNR.0000000000000068
Salvatori, I., Valle, C., Ferri, A., and Carrì, M. T. (2017). SIRT3 and mitochondrial metabolism in neurodegenerative diseases. Neurochem. Int. 109, 184–192. doi: 10.1016/j.neuint.2017.04.012
Santa-Cruz, L. D., and Tapia, R. (2014). Role of energy metabolic déficits and oxidative stress in extcitotoxic spinal motor neuron degeneration in vivo. ANS Neuro 6:e00138. doi: 10.1042/AN20130046
Sarasija, S., and Norman, K. R. (2018). Role of presenilin in mitochondrial oxidative stress and neurodegeneration in Caenorhabditis elegans. Antioxidants 7:111. doi: 10.3390/antiox7090111
Sasaki, S., Shibata, N., Komori, T., and Iwata, T. (2000). iNOS and nitrotyrosine immunoreactivity in amyotrophic lateral sclerosis. Neurosci. Lett. 291, 44–48.
Sasaki, Y., Araki, T., and Mildbrandt, J. (2006). Stimulation of nicotinamide adenine dinucleotide biosynthetic pathways delays axonal degeneration after axotomy. J. Neurosci. 26, 8484–8491. doi: 10.1523/JNEUROSCI.2320-06.2006
Saw, C. L., Guo, Y., Yang, A. Y., Paredes-Gonzalez, X., Ramirez, C., Pung, D., et al. (2014). The berry constituents quercetin, kaempferol and pterostilbene synergistically attenuate reactive oxygen species: involvement of the Nrf2-ARE signaling pathway. Food Chem. Toxicol. 72, 303–311. doi: 10.1016/j.fct.2014.07.038
Sawada, H. (2017). Clinical efficacy of edaravone for the treatment of amyotrophic lateral sclerosis. Expert Opin. Pharmacother. 18, 735–738. doi: 10.1080/14656566.2017.1319937
Schetters, S. T. T., Gómez-Nicola, D., García-Vallejo, J. J., and Van Kooyk, Y. (2018). Neuroinflammation: microglia and T Cells Get ready to tango. Front. Immunol. 8:1905. doi: 10.3389/fimmu.2017.01905
Schieber, M., and Chandel, N. S. (2014). ROS function in redox signaling and oxidative stress. Curr. Biol. 24, R453–R462. doi: 10.1016/j.cub.2014.03.034
Schmidt, M., and Brenner, C. (2019). Absence of evidence that Slc12a8 encodes a nicotinamide mononucleotide transporter. Nat. Metab. 1, 660–661. doi: 10.1038/s42255-018-0009-4
Schon, E. A., and Manfredi, G. (2003). Neuronal degeneration and mitochondrial dysfunction. J. Clin. Invest. 111, 303–312. doi: 10.1172/JCI200317741
Schöndorf, D. C., Ivanyuk, D., Baden, P., Sánchez-Martínez, A., De Circo, S., Yu, C., et al. (2018). The NAD+ precursor nicotinamide riboside rescues mitochondrial defects and neuronal loss in iPSC and fly models of Parkinson’s disease. Cell Rep. 23, 2976–2988. doi: 10.1016/j.celrep.2018.05.009
Scialò, F., Fernández-Ayala, D. J., and Sanz, A. (2017). Role of mitochondrial reverse electron transport in ROS signaling: potential roles in health and disease. Front. Physiol. 27:428. doi: 10.3389/fphys.2017.00428
Selvaraji, S., Poh, L., Natarajan, V., Mallilankaraman, K., and Arumugan, T. V. (2019). Negative conditioning of mitochondrial dysfunction in age-related neurodegenerative diseases. Cond. Med. 2, 30–39.
Seo, E. J., Fischer, N., and Efferth, T. (2018). Phytochemicals as inhibitors of NF-kB for treatment of Alzheimer’s disease. Pharmacol. Res. 129, 262–273. doi: 10.1016/j.phrs.2017.11.030
Sferrazza-Papa, G. F., Pellegrino, G. M., Shaikh, H., Lax, A., Lorini, L., and Corbo, M. (2018). Respiratory muscle testing in amyotrophic lateral sclerosis: a practical approach. Minerva Med. 109(6 Suppl. 1), 11–19. doi: 10.23736/S0026-4806.18.05920-7
Sharina, I. G., and Martin, E. (2017). The role of reactive oxygen and nitrogen species in the expression and splicing of nitric oxide receptor. Antioxid. Redox Signal. 26, 122–136. doi: 10.1089/ars.2016.6687
Sharma, D. R., Wani, W. Y., Sunkaria, A., Kandimalla, R. J., Sharma, R. K., Verma, D., et al. (2016). Quercetin attenuates neuronal death against aluminium-induced neurodegeneration in the rat hippocampus. Neuroscience 324, 163–176. doi: 10.1016/j.neuroscience.2016.02.055
Shen, Y., Aoyagi-Scharber, M., and Wang, B. (2015). Trapping poly(ADP-Ribose) polymerase. J. Pharmacol. Exp. Ther. 353, 446–457. doi: 10.1124/jpet.114.222448
Shibata, N., Hirano, A., Hedley-Whyte, E. T., Dal Canto, M. C., Nagai, R., Uchida, K., et al. (2002). Selective formation of certain advanced glycation end products in spinal cord astrocytes of humans and mice with superoxide dismutase-1 mutation. Acta Neuropathol. 104, 171–178. doi: 10.1007/s00401-002-0537-5
Shin, J. A., Lee, H., Lim, Y. K., Koh, Y., Choi, J. H., and Park, E. M. (2010). Therapeutic effects of resveratrol during acute periods following experimental ischemic stroke. J. Neuroimmunol. 227, 93–100. doi: 10.1016/j.jneuroim.2010.06.017
Sidransky, E., Nalls, M. A., Aasly, J. O., Aharon-Peretz, J., Annesi, G., Barbosa, E. R., et al. (2009). Multicenter analysis of glucocerebroside mutations in Parkison’s disease. N. Engl. J. Med. 361, 1651–1661. doi: 10.1056/NEJMoa0901281
Sies, H. (1986). Biochemistry of oxidative stress. Angew. Chem. Int. Ed. Engl. 25, 1058–1071. doi: 10.1002/anie.198610581
Simpkins, J. W., and Dykens, J. A. (2008). Mitochondrial mechanisms of estrogen neuroprotection. Brain Res. Rev. 57, 421–430. doi: 10.1016/j.brainresrev.2007.04.007
Simpson, E. P., Henry, Y. K., Henkel, J. S., Smith, R. G., and Appel, S. H. (2004). Increased lipid peroxidation in será of ALS patients: a potential biomarker of disease burden. Neurology 62, 1758–1765.
Singh, S. P., Schragenheim, J., Cao, J., Falck, J. R., Abraham, N. G., and Bellner, L. (2016). PGC-1 alpha regulates HO-1 expression, mitochondrial dynamics and biogenesis: role of epoxyeicosatrienoic acid. Prostaglandins Other Lipid Mediat. 125, 8–18. doi: 10.1016/j.prostaglandins.2016.07.004
Sirerol, J. A., Rodríguez, M. L., Mena, S., Asensi, M. A., Estrela, J. M., and Ortega, A. L. (2016). Role of natural stilbenes in the prevention of cancer. Oxid. Med. Cell. Longev. 2016:3128951. doi: 10.1155/2016/3128951
Smith, E. F., Shaw, P. J., and De Vos, K. J. (2017). The role of mitochondria in Amyotrophic Lateral Sclerosis. Neurosci. Lett. 710:132933. doi: 10.1016/j.neulet.2017.06.052
Sohmiya, M., Tanaka, M., Susuki, Y., Tanino, Y., Okamoto, K., and Yamamoto, Y. (2005). An increase of oxidized coenzyme Q-10 occurs in the plasma of sporadic ALS patients. J. Neurol. Sci. 228, 49–53. doi: 10.1016/j.jns.2004.09.030
Solanki, I., Parihar, P., Mansuri, M. L., and Parihar, M. S. (2015). Flavonoid-based therapies in the early management of neurodegenerative diseases. Adv. Nutr. 6, 64–72. doi: 10.3945/an.114.007500
Solleiro-Villavicencio, H., and Rivas-Arancibia, S. (2018). Effect of chronic oxidative stress on neuroinflammatory response mediated by CD4+T cells in neurodegenerative diseases. Front. Cell. Neurosci. 12:114. doi: 10.3389/fncel.2018.00114
Song, L., Chen, L., Zhang, X., Li, J., and Le, W. (2014). Resveratrol ameliorates motor neuron degeneration and improves survival in SOD1(G93A) mouse model of amyotrophic lateral sclerosis. Biomed. Res. Int. 2014:483501. doi: 10.1155/2014/483501
Song, Z., Han, S., Pan, X., Gong, Y., and Wang, M. (2015). Pterostilbene mediates neuroprotection against oxidative toxicity via oestrogen receptor α signalling pathways. J. Pharm. Pharmacol. 67, 720–730. doi: 10.1111/jphp.12360
Srinivasan, E., and Rajasekaran, R. (2018). Quantum chemical and molecular mechanisms studies on the assessment of interactions between resveratrol and mutant SOD1 (G93A) protein. J. Comput. Aided Mol. Des. 32, 1347–1361. doi: 10.1007/s10822-018-0175-1
Srivastava, S. (2016). Emerging therapeutic roles for NAD(+) metabolism in mitochondrial and age-related disorders. Clin. Transl. Med. 5:25. doi: 10.1186/s40169-016-0104-7
Staiculescu, M. C., Foote, C., Meininger, G. A., and Martínez-Lemus, L. A. (2014). The role of reactive oxygen species in microvascular remodeling. Int. J. Mol. Sci. 15, 23792–23835. doi: 10.3390/ijms151223792
St-Pierre, J., Drori, S., Uldry, M., Silvaggi, J. M., Rhee, J., Jäger, S., et al. (2006). Suppression of reactive oxygen species and neurodegeneration by the PGC-1 transcriptional activators. Cell 127, 397–408. doi: 10.1016/j.cell.2006.09.024
Strong, M. J., and Pattee, G. L. (2000). Creatine and coenzyme Q10 in the treatment of ALS. Amyotroph. Lateral Scler. Other Motor Neuron Disord. 4, 17–20.
Swinnen, B., and Robberecht, W. (2014). The phenotypic variability of amyotrophic lateral sclerosis. Nat. Rev. Neurol. 10, 661–670. doi: 10.1038/nrneurol.2014.184
Szkudelski, T., and Szkudelska, K. (2015). Resveratrol and diabetes: from animal to human studies. Biochim. Biophys. Acta 1852, 1145–1154. doi: 10.1016/j.bbadis.2014.10.013
Szwajgier, D., Borowiec, K., and Pustelniak, K. (2017). The neuroprotective effects of phenolic acids: molecular mechanism of action. Nutrients 9:E477. doi: 10.3390/nu9050477
Talbot, K. (2016). Clinical tool for predicting survival in ALS: do we need one? J. Neurol. Neurosurg. Psychiatry 87:1275. doi: 10.1136/jnnp-2016-313683
Talhaoui, N., Gómez-Caravaca, A. M., León, L., De la Rosa, R., Fernández-Gutiérrez, A., and Segura-Carretero, A. (2016). From olive fruits to olive oil: phenolic compound transfer in six different olive cultivars grown under the same agronomical conditions. Int. J. Mol. Sci. 17:337. doi: 10.3390/ijms17030337
Tan, B. L., Norhaizan, M. E., Liew, W. P., and Sulaiman-Rahman, H. (2018). Antioxidant and oxidative stress: a mutual interplay in age-related diseases. Front. Pharmacol. 9:1162. doi: 10.3389/fphar.2018.01162
Tan, W., Pasinelli, P., and Trotti, D. (2014). Role of mitochondria in mutant SOD1 linked amyotrophic lateral sclerosis. Biochim. Biophys. Acta 1842, 1295–1301. doi: 10.1016/j.bbadis.2014.02.009
Tang, B. L. (2016). Sirt1 and the mitochondria. Mol. Cells 39, 87–95. doi: 10.14348/molcells.2016.2318
Tang, B. L. (2017). Could sirtuins activities modify ALS onset and progression? Cell. Mol. Neurobiol. 37, 1147–1160. doi: 10.1007/s10571-016-0452-2
Tarique, H., Tan, B., Yulong, Y., Blachier, F., Myrlene, C. B., and Najma, R. (2016). Oxidative stress and inflammation: what polyphenols can do for us? Oxid. Med. Cell. Longev. 2016:7432797. doi: 10.1155/2016/7432797
Temsamani, H. K. S., Merillon, J. M., and Richard, T. (2015). Promising neuroprotective effects of oligostilbenes. Nutr. Aging 3, 49–54. doi: 10.3233/NUA-150050
Tennen, R. I., and Chua, K. F. (2011). Chromatin regulation and genome maintenance by mammalian SIRT6. Trends Biochem. Sci. 36, 39–46. doi: 10.1016/j.tibs.2010.07.009
Terao, J., Piskula, M., and Yao, Q. (1994). Protective effect of epicatechin, epicatechin gallate, and quercetin on lipid peroxidation in phospholipid bilayers. Arch. Biochem. Biophys. 308, 278–284. doi: 10.1006/abbi.1994.1039
Thandapilly, S. J., Louis, X. L., Yang, T., Stringer, D. M., Yu, L., Zhang, S., et al. (2011). Resveratrol prevents norepinephrine induced hypertrophy in adult rat cardiomyocytes, by activating no-ampk pathway. Eur. J. Pharmacol. 668, 217–222. doi: 10.1016/j.ejphar.2011.06.042
Thibault, O., Gant, J. C., and Landfield, P. W. (2007). Expansion of the calcium hypothesis of braing aging and Alzheimer’s disease: minding the store. Aging Cell 6, 307–317. doi: 10.1111/j.1474-9726.2007.00295.x
Thirupathi, A., and de Souza, C. T. (2017). Multi-regulatory network of ROS: the interconnection of ROS, PGC-1 alpha, and AMPK-SIRT1 during exercise. J. Physiol. Biochem. 73, 487–494. doi: 10.1007/s13105-017-0576-y
Trammell, S. A., Schmidt, M. S., Weidemann, B. J., Redpath, P., Jaksch, F., Delinger, R. W., et al. (2016). Nicotinamide riboside is uniquely and orally bioavaliable in mice and humans. Nat. Commun. 7:12948. doi: 10.1038/ncomms12948
Traversi, G., Fiore, M., Leone, S., Basso, E., Di Muzio, E., Polticelli, F., et al. (2016). Resveratrol and its methoxy-derivatives as modulators of DNA damage induced by ionizing radiation. Mutagenesis 31, 433–441. doi: 10.1093/mutage/gew002
Tressera-Rimbau, A., Arranz, S., Eder, M., and Vallverdú-Queralt, A. (2017). Dietary polyphenols in the prevention of stroke. Oxid. Med. Cell. Longev. 2017:7467962. doi: 10.1155/2017/7467962
Tripodo, G., Chlapanidas, T., Perteghella, S., Vigani, B., Mandracchia, D., Trapani, A., et al. (2015). Mesenchymal stromal cells loading curcumin-INVITE-micelles: a drug delivery system for neurodegenerative diseases. Colloids Surf. B Biointerfaces 125, 300–308. doi: 10.1016/j.colsurfb.2014.11.034
Turner, M. R., Bowser, R., Bruijin, L., Dupuis, L., Ludolph, A., Mcgrath, M., et al. (2013). Mechanisms, models and biomarkers in amyotrophic lateral sclerosis. Amyotroph. Lateral Scler. Frontotemporal. Degener. 14(Suppl. 1), 19–32. doi: 10.3109/21678421.2013.778554
Turrens, J. F. (2003). Mitochondrial formation of reactive oxygen species. J. Physiol. 552(Pt 2), 335–344. doi: 10.1113/jphysiol.2003.049478
Ullah, F., Liang, A., Rangel, A., Gyengesi, E., Niedermayer, G., and Münch, G. (2017). High bioavailability curcumin: an anti-inflammatory and neurosupportive bioactive nutrient for neurodegenerative diseases characterized by chronic neuroinflammation. Arch. Toxicol. 91, 1623–1634. doi: 10.1007/s00204-017-1939-4
Valko, K., and Ciesla, L. (2019). Amyotrophic lateral sclerosis. Prog. Med. Chem. 58, 63–117. doi: 10.1016/bs.pmch.2018.12.001
Valko, M., Leibfritz, D., Moncol, J., Cronin, M. T., Mazur, M., and Telser, J. (2007). Free radicals and antioxidants in normal physiological functions and human disease. Int. J. Biochem. Cell Biol. 39, 44–84. doi: 10.1016/j.biocel.2006.07.001
Valle, C., and Carrì, M. T. (2017). Cysteine modifications in the pathogenesis of ALS. Front. Mol. Neurosci. 10:5. doi: 10.3389/fnmol.2017.00005
Vallverdú-Queralt, A., Boix, N., Piqué, E., Gómez-Catalán, J., Medina-Remon, A., Sasot, G., et al. (2015). Identification of phenolic compounds in red wine extract samples and zebrafisch embryos by HPLC-ESI-LTQ-Orbitrap-MS. Food Chem. 181, 146–151. doi: 10.1016/j.foodchem.2015.02.098
Vallverdú-Queralt, A., Regueiro, J., Martínez-Huélamo, M., Rinaldi-Alvarenga, J. F., Leal, L. N., and Lamuela-Raventos, R. M. (2014). A comprehensive study on the phenolic profile of widely used culinary herbs and spices: rosemary, thyme, oregano, cinnamon, cumin and bay. Food Chem. 154, 299–307. doi: 10.1016/j.foodchem.2013.12.106
Van Damme, P., Robberecht, W., and Van Den Bosch, L. (2017). Modelling amyotrophic lateral sclerosis: progress and possibilities. Dis. Model. Mech. 10, 537–549. doi: 10.1242/dmm.029058
Van Es, M. A., Hardiman, O., Chio, A., Al-Chalabi, A., Pasterkamp, R. J., Veldink, J. H., et al. (2017). Amyotrophic lateral sclerosis. Lancet 390, 2084–2098. doi: 10.1016/S0140-6736(17)31287-4
Van Giau, V., An, S. S. A., and Hulme, S. P. (2018). Mitochondrial therapeutic interventions in Alzheimer’s disease. J. Neurol. Sci. 395, 62–70. doi: 10.1016/j.jns.2018.09.033
Van Horssen, J., Van Schaik, P., and Witte, M. (2017). Inflammation and mitochondrial dysfunction: a vicious circle in neurodegenerative disorders? Neurosci. Lett. 710:132931. doi: 10.1016/j.neulet.2017.06.050
Van Horssen, J., Witte, M. E., Schreibelt, G., and De Vries, H. E. (2011). Radical changes in multiple sclerosis pathogenesis. Biochim. Biophys. Acta 1812, 141–150. doi: 10.1016/j.bbadis.2010.06.011
Van Houten, B., Woshner, V., and Santos, J. H. (2006). Role of mitochondrial DNA in toxic responses to oxidative stress. DNA Repair 5, 145–152. doi: 10.1016/j.dnarep.2005.03.002
Van’t Erve, T. J., Kadiiska, M. B., London, S. J., and Mason, R. P. (2017). Classifying oxidative stress by F2-isoprostane levels across human diseases: a meta-analysis. Redox Biol. 12, 582–599. doi: 10.1016/j.redox.2017.03.024
Vaur, P., Brugg, B., Mericskay, M., Li, Z., Schmidt, M. S., Vivien, D., et al. (2017). Nicotinamide riboside, a form of vitamin B3, protects againts excitotoxicity-induced axonal degeneration. FASEB J. 31, 5440–5452. doi: 10.1096/fj.201700221RR
Vauzour, D., Vafeiadou, K., Rodriguez-Mateos, A., Rendeiro, C., and Spencer, J. P. E. (2008). The neuroprotective potential of flavonoids: a multiplicity of effects. Genes Nutr. 3, 115–126. doi: 10.1007/s12263-008-0091-4
Veldink, J. H., Kalmijn, S., Groeneveld, G. J., Wunderink, W., Koster, A., de Vries, J. H. M., et al. (2007). Intake of polyunsaturated fatty acids and vitamin E reduces the risk of developing amyotrophic lateral sclerosis. J. Neurol. Neurosurg. Psychiatr. 78, 367–371. doi: 10.1136/jnnp.2005.083378
Venditti, P., Di Stefano, L., and Di Meo, S. (2013). Mitochondrial metabolism of reactive oxygen species. Mitochondrion 13, 71–82. doi: 10.1016/j.mito.2013.01.008
Verdin, E. (2015). NAD+ in aging, metabolism, and neurodegeneration. Science 350, 1208–1213. doi: 10.1126/science.aac4854
Vijayvergiya, C., Beal, M. F., Buck, J., and Manfredi, G. (2005). Mutant superoxide dismutase 1 forms aggregates in the brain mitochondrial matrix of amyotrophic lateral sclerosis mice. J. Neurosci. 25, 2463–2470. doi: 10.1523/JNEUROSCI.4385-04.2005
Voloshyna, I., Hai, O., Littlefield, M. J., Carsons, S., and Reiss, A. B. (2013). Resveratrol mediates anti-atherogenic effects on cholesterol flux in human macrophages and endothelium via pparγ and adenosine. Eur. J. Pharmacol. 698, 299–309. doi: 10.1016/j.ejphar.2012.08.024
Wang, B., Liu, H., Yue, L., Li, X., Zhao, L., Yang, X., et al. (2016). Neuroprotective effects of pterostilbene against oxidative stress injury: involvement of nuclear factor erythroid 2-related factor 2 pathway. Brain Res. 1643, 70–79. doi: 10.1016/j.brainres.2016.04.048
Wang, H., O’reilly, E. J., Weisskopf, M. G., Logroscino, G., McCullough, M. L., Schatzkin, A., et al. (2011). Vitamin E intake and risk of amyotrophic lateral sclerosis: a pooled analysis of data from 5 prospective cohort studies. Am. J. Epidemiol. 173, 595–602. doi: 10.1093/aje/kwq416
Wang, J., Zhai, Q., Chen, Y., Lin, E., Gu, W., McBurney, M. W., et al. (2005). A local mechanism mediates NAD-dependent protection of axon degeneration. J. Cell Biol. 170, 349–355. doi: 10.1083/jcb.200504028
Wang, J., Zhang, Y., Tang, L., Zhan, N., and Fan, D. (2011). Protective effects of resveratrol through the up-regulation of SIRT1 expression in the mutant hSOD1-G93A-bearing motor neuron-like cell culture model of amyotrophic lateral sclerosis. Neurosci. Lett. 503, 250–255. doi: 10.1016/j.neulet.2011.08.047
Wang, Q., Li, L., Li, C. Y., Pei, Z., Zhou, M., and Li, N. (2015). SIRT3 protects cells from hypoxia via PGC-1α- and MnSOD-dependent pathways. Neuroscience 12, 109–121. doi: 10.1016/j.neuroscience.2014.11.045
Wang, T. H., Wang, S. Y., Wang, X. D., Jiang, H. Q., Yang, Y. Q., Wang, Y., et al. (2018). Fisetin exerts antioxidant and neuroprotective effects in multiple mutant hSOD1 models of amyotrophic lateral sclerosis by activating ERK. Neuroscience 379, 152–166. doi: 10.1016/j.neuroscience.2018.03.008
Wang, Y., Xu, E., Musich, P. R., and Lin, F. (2019). Mitochondrial dysfunction in neurodegenerative diseases and the potential countermeasure. CNS Neurosci. Ther. 25, 816–824. doi: 10.1111/cns.13116
Watanabe, K., Tanaka, M., Yuki, S., Hiraj, M., and Yamamoto, Y. (2018). How is edaravone effective against acute ischemic stroke and amyotrophic lateral sclerosis? J. Clin. Biochem. Nutr. 62, 20–38. doi: 10.3164/jcbn.17-62
Wei, Q., Chen, X., Chen, Y., Ou, R., Cao, B., Hou, Y., et al. (2019). Unique characteristics of the genetics epidemiology of amyotrophic lateral sclerosis in China. Sci. China Life Sci. 62, 517–525. doi: 10.1007/s11427-018-9453-x
Weiduschat, N., Mao, X., Hupf, J., Armstrong, N., Kang, G., Lange, D. J., et al. (2014). Motor cortex glutathione déficit in ALS measured in vivo with the J-editing technique. Neurosci. Lett. 570, 102–107. doi: 10.1016/j.neulet.2014.04.020
Weishaupt, J. H., Bartels, C., Pölking, E., Dietrich, J., Rohde, G., Poeggeler, B., et al. (2006). Reduced oxidative damage in ALS by high-dose enteral melatonin treatment. J. Pineal. Res. 41, 313–323. doi: 10.1111/j.1600-079X.2006.00377.x
Wier, C. G., Crum, A. E., Reynolds, A. B., Iyer, C. C., Chugh, D., Palettas, M. S., et al. (2019). Muscle contractility dysfunction precedes loss of motor unit connectivity in SOD1(G93A) mide. Muscle Nerve 59, 254–262. doi: 10.1002/mus.26365
Witte, M. E., Geurts, J. J., de Vries, H. E., Van der Valk, P., and Van Horssen, J. (2010). Mitochondrial dysfunction: a pontential link between neuroinflammation and neurodegeneration? Mitochondrion 10, 411–418. doi: 10.1016/j.mito.2010.05.014
Wu, Z., Huang, X., Feng, Y., Handschin, C., Feng, Y., Gullicksen, P. S., et al. (2006). Transducer of regulated CREB-binding proteins (TORCs) induce PGC-1alpha transcription and mitochondrial biogénesis in muscle cells. Proc. Natl. Acad. Sci. U.S.A. 103, 14379–14384. doi: 10.1073/pnas.0606714103
Xiao, Y., Karam, C., Yi, J., Zhang, L., Li, X., Yoon, D., et al. (2018). ROS-related mitochondrial dysfunction in skeletal muscle of an ALS mouse model during the disease progression. Pharmacol. Res. 138, 25–36. doi: 10.1016/j.phrs.2018.09.008
Xie, Y., Chen, J., Xiao, A., and Liu, L. (2017). Antibacterial activity of polyphenols: structure-activity relationship and influence of hyperglycemic condition. Molecules 22:1913. doi: 10.3390/molecules22111913
Xu, Z., Chen, S., Li, X., Luo, G., Li, L., and Le, W. (2006). Neuroprotective effects of (-)-epigallocatechin-3-gallate in a transgenic mouse model of amyotrophic lateral sclerosis. Neurochem. Res. 31, 1263–1269. doi: 10.1007/s11064-006-9166-z
Xue, E. X., Lin, J. P., Zhang, Y., Sheng, S. R., Liu, H. X., Zhou, Y. L., et al. (2017). Pterostilbene inhibits inflammation and ROS production in chondrocytes by activating Nrf2 pathway. Oncotarget 8, 41988–42000. doi: 10.18632/oncotarget.16716
Yaku, K., Okabe, K., and Nakagawa, T. (2018). NAD metabolism: implications in aging and longevity. Ageing Res. Rev. 47, 1–17. doi: 10.1016/j.arr.2018.05.006
Yamamoto, Y. (2016). Coenzyme Q10 redox balance and a free radical scavenger drug. Arch. Biochem. Biophys. 595, 132–135. doi: 10.1016/j.abb.2015.11.026
Yang, H., Yang, T., Baur, J. A., Pérez, E., Matsui, T., Carmona, J. J., et al. (2007). Nutrient-sensitive mitochondrial NAD+ levels dictate cell survival. Cell 130, 1095–1107. doi: 10.1016/j.cell.2007.07.035
Yang, T., Chan, N. Y., and Sauve, A. A. (2007). Synthesis of nicotinamide riboside and derivatives: effective agents for increasing nicotinamide adenine dinucleotide concentrations in mammalian cells. J. Med. Chem. 50, 6458–6461. doi: 10.1021/jm701001c
Yang, Y., Fan, C., Wang, B., Ma, Z., Wang, D., Gong, B., et al. (2017). Pterostilbene attenuates high glucose-induced oxidative injury in hippocampal neuronal cells by activating nuclear factor erythroid 2-related factor 2. Biochim. Biophys. Acta. Mol. Basis Dis. 1863, 827–837. doi: 10.1016/j.bbadis.2017.01.005
Yang, Y., and Sauve, A. A. (2016). NAD(+) metabolism: bioenergetics, signaling and manipulation for therapy. Biochim. Biophys. Acta 1864, 1787–1800. doi: 10.1016/j.bbapap.2016.06.014
Yeo, S. C., Ho, P. C., and Lin, H. S. (2013). Pharmacokinetics of pterostilbene in Sprague-Dawley rats: the impacts of aqueous solubility, fasting, dose escalation, and dosing route on bioavailability. Mol. Nutr. Food Res. 57, 1015–1025. doi: 10.1002/mnfr.201200651
Yoshino, H., and Kimura, A. (2006). Investigation of the therapeutic effects of edaravone, a free radical scavenger, on amyotrophic lateral sclerosis (Phase II study). Amyotroph. Lateral Scler. 7, 241–245. doi: 10.1080/17482960600881870
Yoshino, J., Baur, J. A., and Imai, S. I. (2018). NAD+ intermediates: the biology and therapeutic potential of NMN and NR. Cell Metab. 27, 513–528. doi: 10.1016/j.cmet.2017.11.002
Yu, Y., Sy, F. C., Callaghan, B. C., Goutman, S. A., Batterman, S. A., and Feldman, E. L. (2014). Environmental risk factors and amyotrophic lateral sclerosis (ALS): a case-control sutdy of ALS in Michingan. PLoS One 9:e101186. doi: 10.1371/journal.pone.0101186
Yun, Y. C., Jeong, S. G., Kim, S. H., and Cho, G. W. (2019). Reduced sirtuin 1/adenosine monophosphate-activated protein kinase in amyotrophic lateral sclerosis patient-derived mesenchymal stem cells can be restored by resveratrol. J. Tissue Eng. Regen. Med. 13, 110–115. doi: 10.1002/term.2776
Yusuf, M., Khan, M., Robaian, M. A., and Khan, R. A. (2018). Biomechanistic insights into the roles of oxidative stress in generating complex neurological disorders. Biol. Chem. 399, 305–319. doi: 10.1515/hsz-2017-0250
Zewen, L., Zhangpin, R., Jun, Z., Chia-Chen, C., Eswar, K., Tingyang, Z., et al. (2018). Role of ROS and nutritional antioxidants in human diseases. Front. Physiol. 9:477. doi: 10.3389/fphys.2018.00477
Zhang, B., Wang, X. Q., Chen, H. Y., and Liu, B. H. (2014). Involvement of the Nrf2 pathway in the regulation of pterostilbene-induced apoptosis in HeLa cells via ER stress. J. Pharmacol. Sci. 126, 216–229.
Zhang, H., Ryu, D., Wu, Y., Gariani, K., Wang, X., Luan, P., et al. (2016). NAD+ repletion improves mitochondial and stem cell function and enhaces life span in mice. Science 352, 1436–1443. doi: 10.1126/science.aaf2693
Zhang, L., Zhou, G., Song, W., Tan, X., Guo, Y., Zhou, B., et al. (2012). Pterostilbene protects vascular endotelial cells against oxidized low-density lipoprotein-induced apoptosis in vitro and in vivo. Apoptosis 17, 25–36. doi: 10.1007/s10495-011-0653-6
Zhang, N., and Sauve, A. A. (2018). Regulatory effects of NAD+ metabolic pathways on sirtuin activity. Prog. Mol. Biol. Transl. Sci. 154, 71–104. doi: 10.1016/bs.pmbts.2017.11.012
Zhang, Y., Cook, A., Kim, J., Baranov, S. V., Jiang, J., Smith, K., et al. (2013). Melatonin inhibits the caspase-1/cytochrome c/caspase-3 cell death pathway, inhibits mt1 receptor loss and delays disease progression in a mouse model of amyotrophic lateral sclerosis. Neurobiol. Dis. 55, 26–35. doi: 10.1016/j.nbd.2013.03.008
Zhong, N., and Xu, J. (2008). Synergistic activation of the human MnSOD promoter by DJ-1 and PGC-1alpha: regulation by SUMOylation and oxidation. Hum. Mol. Genet. 17, 3357–3367. doi: 10.1093/hmg/ddn230
Zhou, Y., Zheng, J., Li, Y., Xu, D. P., Li, S., Chen, Y. M., et al. (2016). Natural polyphenols for prevention and treatment of cancer. Nutrients 8:E515. doi: 10.3390/nu8080515
Zhu, X. H., Lu, M., Lee, B. Y., Ugurbil, K., and Chen, W. (2015). In vivo NAD assay reveals the intracelular NAD contents and redox state in healthy human brain and their age dependences. Proc. Natl. Acad. Sci. U.S.A. 112, 2876–2881. doi: 10.1073/pnas.1417921112
Zordoky, B. N., Robertson, I. M., and Dyck, J. R. (2015). Preclinical and clinical evidence for the role of resveratrol in the treatment of cardiovascular diseases. Biochim. Biophys. Acta 1852, 1155–1177. doi: 10.1016/j.bbadis.2014.10.016
Zufiría, M., Gil-Bea, F. J., Fernández-Torrón, R., Poza, J. J., Muñoz-Blanco, J. L., Rojas-García, R., et al. (2016). ALS: a bucket of genes, environment, metabolism and unknow ingredients. Prog. Neurobiol. 142, 104–129. doi: 10.1016/j.pneurobio.2016.05.004
Keywords: amyotrophic lateral sclerosis, neurodegenerative diseases, oxidative stress, mitochondrial dysfunction, nicotinamide riboside, pterostilbene
Citation: Carrera-Juliá S, Moreno ML, Barrios C, de la Rubia Ortí JE and Drehmer E (2020) Antioxidant Alternatives in the Treatment of Amyotrophic Lateral Sclerosis: A Comprehensive Review. Front. Physiol. 11:63. doi: 10.3389/fphys.2020.00063
Received: 25 July 2019; Accepted: 21 January 2020;
Published: 06 February 2020.
Edited by:
Murugesan Velayutham, West Virginia University, United StatesReviewed by:
Houzao Chen, Chinese Academy of Medical Sciences, ChinaMarcos Lopez, University of Puerto Rico, Puerto Rico
Copyright © 2020 Carrera-Juliá, Moreno, Barrios, de la Rubia Ortí and Drehmer. This is an open-access article distributed under the terms of the Creative Commons Attribution License (CC BY). The use, distribution or reproduction in other forums is permitted, provided the original author(s) and the copyright owner(s) are credited and that the original publication in this journal is cited, in accordance with accepted academic practice. No use, distribution or reproduction is permitted which does not comply with these terms.
*Correspondence: Eraci Drehmer, ZXJhY2kuZHJlaG1lckB1Y3YuZXM=