- 1Functional Ecology, Institute of Zoology, University of Hamburg, Hamburg, Germany
- 2School of Biology and Ecology, University of Maine, Orono, ME, United States
- 3School of Biological and Environmental Sciences, Liverpool John Moores University, Liverpool, United Kingdom
Hibernation and daily torpor (heterothermy) allow endotherms to cope with demanding environmental conditions. The depth and duration of torpor bouts vary considerably between tropical and temperate climates, and tropical hibernators manage to cope with a wider spectrum of ambient temperature (Ta) regimes during heterothermy. As cycles in Ta can have profound effects on activity and torpor patterns as well as energy expenditure, we examined how these characteristics are affected by daily fluctuating versus constant Ta in a tropical hibernator, the lesser hedgehog tenrec (Echinops telfairi). Throughout the study, regardless of season, the tenrecs became torpid every day. In summer, E. telfairi used daily fluctuations in Ta to passively rewarm from daily torpor, which led to synchrony in the activity phases and torpor bouts between individuals and generally decreased energy expenditure. In contrast, animals housed at constant Ta showed considerable variation in timing and they had to invest more energy through endogenous heat production. During the hibernation season (winter) E. telfairi hibernated for several months in constant, as well as in fluctuating Ta and, as in summer, under fluctuating Ta arousals were much more uniform and showed less variation in timing compared to constant temperature regimes. The timing of torpor is not only important for its effective use, but synchronization of activity patterns could also be essential for social interactions, and successful foraging bouts. Our results highlight that Ta cycles can be an effective zeitgeber for activity and thermoregulatory rhythms throughout the year and that consideration should be given to the choice of temperature regime when studying heterothermy under laboratory conditions.
Introduction
Limited food and water supply and unfavorable climatic conditions often require mammals to find means to reduce their dependency on energy and water availability. This holds particularly true for small animals in harsh climates as their small surface area to volume ratios lead to greater heat transfer and water loss. Hibernation, prolonged and daily torpor (combined here as heterothermy) are physiological strategies that allow endotherms to cope with such demanding conditions (Lyman et al., 1982). These physiological states are characterized by an active depression of metabolic rate (MR) and a change in set point of body temperature (Tb, Geiser, 2004; Heldmaier et al., 2004), providing high savings in energy and water and a lowered Tb that can be close to ambient temperature (Ta) during deep torpor (Ruf and Geiser, 2015). Daily torpor is limited to short bouts of less than 24 h, prolonged torpor bouts last for a few days, whereas hibernation consists of a series of approximately 1–2 week-long torpor bouts interspersed by active arousals, usually totaling several months (Geiser, 2004; Heldmaier et al., 2004; Nowack et al., 2020). Although heterothermy is best known, and probably more common in arctic and temperate climates, it also occurs in the tropics (Cossins and Barnes, 1996; Ruf and Geiser, 2015; Nowack et al., 2020). With increasing numbers of ecophysiological field studies there is more and more evidence of heterothermy in tropical mammals: e.g., bats (Stawski and Geiser, 2011; Reher et al., 2018), cheirogaleid lemurs (Dausmann, 2014), the bushbaby Galago moholi (Nowack et al., 2010), loris (Ruf et al., 2015; Streicher et al., 2017), tenrecs (Lovegrove and Génin, 2008; Oelkrug et al., 2013; Levesque et al., 2014), birds (McKechnie and Mzilikazi, 2011), echidnas, and marsupials (Grigg and Beard, 2000; Geiser and Körtner, 2010; Körtner et al., 2010). In fact, there are more mammalian orders with heterotherms than without, and it is likely that the capacity for heterothermy is the ancestral state in mammals (Grigg et al., 2004; Lovegrove, 2012a, b).
In contrast to arctic or temperate regions, the ultimate triggers of heterothermy in the tropics might not be as straight forward, and are more multifaceted than low temperature and low availability of food (see Geiser and Brigham, 2012; Nowack et al., 2017, 2020). The costs of endothermy might be less pronounced in many parts of the tropics, however, food and water can be scarce during all or some periods of the year. Thus, the water saving potential of hibernation and daily torpor becomes more important in the tropics (Macmillen, 1965; Cryan and Wolf, 2003; Schmid and Speakman, 2009). Reductions in MR, Tb, food requirements and activity are accompanied by reductions in evaporative, fecal and urinary water loss, leading to substantial water savings (Cooper et al., 2005; Withers et al., 2012). Similarly, the time course and pattern of Tb during hibernation varies considerably between tropical and temperate climates. Arctic and temperate hibernators encounter very low Ta and subsequently exhibit very low Tb during hibernation, sometimes even below the freezing point (Barnes, 1989; Pretzlaff and Dausmann, 2012). Additionally, hibernacula of arctic and temperate hibernators are generally well insulated and temperature fluctuations are small within the hibernaculum (Arnold et al., 1991; Buck and Barnes, 1999). Tropical hibernators, on the other hand, use hibernacula with very variable degrees of insulation capacities, e.g., hollows in trees of varying heights and thicknesses, or underground sites at varying depths (Dausmann et al., 2004; Körtner et al., 2010; Blanco et al., 2013; Levesque et al., 2014; Lovegrove et al., 2014a). Therefore, depending on the choice of hibernaculum or resting site, i.e., well versus poorly insulated, tropical hibernators manage to cope with a wide spectrum of temperature regimes during hibernation: from constant to highly fluctuating temperatures, with elevated temperatures possibly enhanced by tropical solar radiation during the day. As Tb usually approximates Ta during hibernation, this flexibility is also reflected in Tb (Dausmann et al., 2004; Kobbe and Dausmann, 2009; Canale et al., 2012; Levesque et al., 2014; Reher et al., 2018). For example, the range of daily Ta fluctuations affects the hibernation pattern in the lemur Cheirogaleus medius (Dausmann et al., 2005) and many heterotherms use the daily Ta fluctuations to assist warming up from daily and prolonged torpor or hibernation bouts (Ortmann et al., 1997; Schmid, 2000; Mzilikazi et al., 2002; Turbill and Geiser, 2008; Warnecke et al., 2008; Kobbe and Dausmann, 2009; Warnecke and Geiser, 2010; Thompson et al., 2015). However, Ta cycles not only help rewarming, they also act as a zeitgeber, influencing activity patterns (Pohl, 1998; Vivanco et al., 2010).
We therefore sought to characterize the effects of differing Ta patterns on the thermophysiology of a tropical hibernator, the lesser hedgehog tenrec (Echinops telfairi). We aimed to evaluate how the choice of hibernaculum (i.e., insulation capacity) influences hibernation parameters in the wild by examining how daily fluctuating Ta versus constant Ta affects patterns of daily torpor and hibernation and energy expenditure. As the previous measures of cost of hibernation under constant Ta conditions may have overestimated the total frequency and cost of rewarming in tropical hibernators, we measured metabolic rate to test if Ta fluctuations are used to assist with warming during arousals. Finally, by simulating a range of summer and winter temperatures, we aimed to analyse how hibernation patterns and energy expenditure are affected by variable and changing temperatures during hibernation.
Materials and Methods
Study Species
Echinops telfairi (Martin, 1838) is a small (135 g) nocturnal insectivorous member of the family Tenrecidae and endemic to Madagascar (Eisenberg and Gould, 1969). It uses daily torpor during the austral summer, and hibernates during the winter. It has one of the lowest reported euthermic Tb of any eutherian mammal and is highly thermally labile (Scholl, 1974; Clarke and Rothery, 2008; Lovegrove and Génin, 2008). In Madagascar, E. telfairi rests and hibernates in tree hollows, dead trees or under leaf litter (Eisenberg and Gould, 1969; Soarimalala and Goodman, 2011). Neither offer a particularly well-insulated resting site and it can thus be assumed that they experience fluctuating Ta year-round.
Eighteen female and nine male adult, laboratory-bred lesser Malagasy hedgehog-tenrecs (E. telfairi; 3–5 years old during the experiments) were used for the experiments over a two year time period. The animals were acquired from the Ludwig-Maximilians-University Munich, where they had been bred for over 30 years and fully acclimated to northern hemisphere seasonal rhythms (e.g., Künzle, 1998). All animals were earmarked or marked with an injectable micro transponder (ID-100, Trovan, Usling GmbH, Weilerswist, Germany), to unambiguously identify individuals.
Experimental Setup
The animals were kept in separate cages (35 × 21 × 35 cm, L × W × H) in a climate chamber (Type TCR + 2, Weiss Technik, Reiskirchen, Germany) during the experiments and weighed regularly. The cages were equipped with wooden nest boxes (14 × 20 × 14 cm), wood chips, a hamster wheel and other environmental enrichment. Food (mealworms, cockroaches, wet canned cat food, dry dog food, dry hedgehog food, boiled egg and fresh fruit) and water were provided ad libitum. To test for the effects of Ta on torpor patterns the animals were exposed to five different temperature treatments, two during the animals’ summer and three in their winter. Temperatures were chosen to match actual climatic conditions of E. telfairi in their natural resting sites (Jury, 2003; Dausmann and Blanco, 2016). During summer, day length (simulated by ambient lighting) and humidity were adjusted to 13 h and 70%, respectively. Ta was either held constant at 24°C (Sconst24) or fluctuating between 19°C during the dark phase and 28°C during the light phase (Sfluc19–28). In winter day length was reduced to 11 h and humidity to 40%, constant Ta was set at 18 or 12°C (Wconst18 and Wconst12), and fluctuating Ta varied from 14°C during the dark phase to 24°C during the light phase (Wfluc14–24). The constant Tas 24 and 18°C were chosen as the middle between the minimum and maximum of the fluctuating Ta of the respective season (as would be found in a very well insulated resting site). Additionally, a constant Ta of 12°C was included during winter to investigate responses and limitations of E. telfairi to a constant temperature regime at the lower end of temperatures in their resting sites. Animals were randomly assigned to the different experimental treatments and were used in multiple experiments. Each treatment lasted for a minimum of two weeks and the sequence of experimental treatments within each season was randomized.
Measurement of Ambient and Skin Temperature
Skin temperature (Tskin) and Ta were measured with temperature data loggers (3.3 g; iButton, DS1922L, Maxim Integrated Products, Inc., Sunnyvale, United States) set to logging intervals of 15 min and a resolution of 0.0625°C. The data loggers were taped to the shaved animals’ abdominal regions with medical tape (Fixomull stretch, BSN medical, Hamburg, Germany), which did not restrict the tenrecs’ movements in any way and remained in close contact to the skin during activity. External temperature loggers give reliable approximation of Tb, especially during resting and torpor phases, when the animals are curled up with the logger positioned inside (Barclay et al., 1996; Dausmann, 2005). When loggers fell off (mainly during activity phases), they were re-taped to the animals without any apparent disturbance before they became torpid again. As the tenrecs (and cages) were checked daily, we found detached loggers within 24 h and the corresponding data were omitted from analyses.
Loggers for recording Ta were fixed to the inside of each cage (to control the preciseness of the climate chamber) and each nest box (Tn in the analyses). Temperature readings were averaged for every hour. As Tskin of the tenrecs is very flexible and sometimes low even in the non-torpid state, it was not possible to define a torpor/non-torpor threshold for Tskin. However, Tskin was always either almost at Ta, or distinctly above it. Thus, the animals were considered to be torpid when Tskin was at or only slightly above Tn (Tskin–Tn ≤ 2°C), as confirmed by the obvious drop and increase in MR at the beginning and end of each torpor bout and of each activity phase (summer) or arousal (winter) (see Figure 1). The term “arousals” in this study thus includes the (active) rewarming phase as well as periods of activity with normothermic Tskin (especially during summer) and parts of the cooling phase.
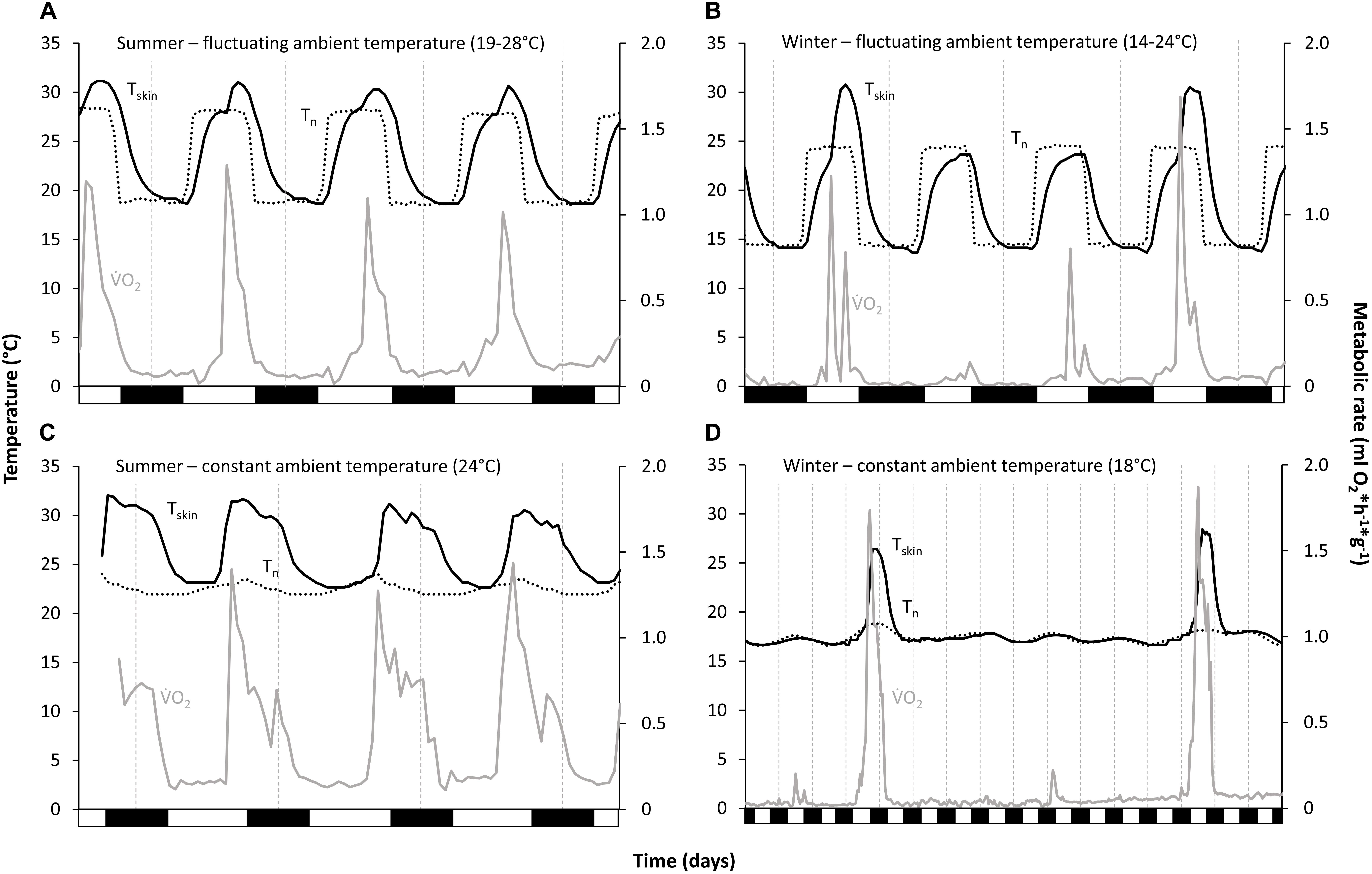
Figure 1. Torpor and arousal/activity phase pattern of Echinops telfairi for four experimental treatments. (A,B) fluctuating temperature conditions (summer and winter), (C,D) constant temperature conditions (summer and winter). Tenrecs became torpid every day; under fluctuating temperature conditions, they used these fluctuations for passively warming from torpor until maximum nest box temperature, whereas under constant temperature conditions they had to rely on endogenous heat production. Black line: skin temperature; dotted line: nest box temperature; gray line: metabolic rate; vertical dashed lines indicate midnight, black boxes on the x-axis the scotophase.
Measurement of Metabolic Rate
Metabolic rate was measured via oxygen consumption with two differential oxygen analyzers (OxBox, FIWI, Vienna University, Vienna, Austria). Air was drawn at a rate of 50 L/h from the nest boxes, which served as respiratory chambers and were connected to the oxygen analyzers with airtight tubes (Tygon tubing ST, Saint-Gobain, Charny, France). Dividers in the middle of the nest boxes lessened air mixing between ambient air and the inside of the nest boxes. A gas splitter (RM Gas Flow Multiplexer, Sable Systems, Las Vegas, NV, United States) was used to rotate air flow amongst four different nest boxes. Thus, a total of eight animals could be measured at the same time using the two oxygen analyzers. During a 1-h long rotation cycle, all nest boxes were measured for 15 min and data stored every minute, and once per hour reference air was measured for 5 min to obtain a baseline value. Depending on the start of the measurement, the reference air measurement fell into the measurement period of different animals. Air leaving the nest boxes was dried with silica gel (Silica Gel Orange, 2–5 mm, Carl Roth, Karlsruhe, Germany) before entering the analyzer and the flow meter. The oxygen analyzers were calibrated with calibration gas mixtures produced with a gas-mixing pump (2KM300/a, H. Wösthoff GmbH, Bochum, Germany) and values were corrected for the CO2 effect with: ΔVol%O2 = ΔO2 + 0.0818∗ΔO2. Oxygen consumption was calculated using the following equation: [ml O2∗h–1] = ΔVol%O2 ∗ flow [L∗h–1] ∗ 10 (Heldmaier and Steinlechner, 1981) and was converted to per gram body mass (ml O2∗g–1*h–1). Mass used in the analysis was calculated as the average of the body mass before and after the experimental periods. Mean hourly values of oxygen consumption were calculated and synchronized with corresponding temperature readings. For each daily torpor bout, hibernation bout, arousal and activity phase minimum, maximum and average oxygen consumption and Tskin were calculated. Additionally, the average oxygen consumption for the warming phase of each torpor bout was calculated. Oxygen consumption could only be measured as torpid or resting metabolic rate (RMR) in most cases, as measurements were only possible when the animals were in the nest boxes and therefore total energy budgets, including activity could not be calculated. Due to technical problems, is not available for Wconst12.
Statistical Methods
If not stated otherwise, values are given as mean ± standard deviation, with N denoting the number of individuals tested per treatment, n the number of observations. As the aim of our study was to identify responses on a population level and due to the uneven distribution of sexes within and across treatments, the data from both sexes were pooled. All statistical procedures were done using R (R Development Core Team, 2018). Differences in weight were tested with a t-test after the data were checked with a Kolmogorov–Smirnov test for normal distribution. To account for an unbalanced data set, differences in minimum and Tskin during torpor, torpor bout duration (TBD), maximum , average and Tskin during arousal and length of the activity period were tested via generalized estimation equations with a Gaussian error structure and an autoregressive, AR1, correlation structure (“geeglm” in library “geepack,” Yan, 2002; Yan and Fine, 2004; Halekoh et al., 2006), followed by type 1 ANOVA. Individual ID was included as a random effect to adjust for repeated measurements. To test for statistical differences of , we used total per animal as the response variable and adjusted for body mass by including it as a covariate. Mass-specific metabolic rates are given for descriptive purposes but were not used in statistical analyses. Post hoc analyses were performed as Tukey tests (“glht” in library “multcomp,” Hothorn et al., 2008).
Rayleigh tests were used to determine whether circular data (timing of arousals) differed significantly from random (“rayleigh.test” in library “circular,” Jammalamadaka and Sengupta, 2001). Watson two-tailed tests were performed to test differences between the timing of arousals between treatments (“watson.two.test” in “circular”). To examine differences in the variance of arousal timing, we performed generalized estimation equations as described above. Variance was calculated as the individual deviation from the mean time of arousal start (minutes from midnight) per treatment. Resultant probability values were compared to an α-value of 0.05.
Results
Behavior and Body Mass
Corresponding with their nocturnal lifestyle, all animals were active during the late day and early night throughout summer and used short bouts of torpor during the rest phase on a daily basis. Only one female animal showed an activity phase exceeding 24 h on two occasions during Sconst24 (28 and 40 h long). During winter, all E. telfairi hibernated for several months (N = 27) and spent most time inside their nest boxes, even during arousals, although some individuals briefly left their boxes to drink. Most animals did not eat during winter (see below). Average body mass during summer was 156 ± 28 g (N = 27). Mean body mass fell to 126 ± 24 g (N = 23) during the winter (t-test, t = 10.616, p < 0.001).
In a separate study, food intake of the study individuals across the seasons was measured. During winter, most tenrecs did not eat. If they ate, it was only a small fraction of the amount they ate during summer (about 2.7 g mealworms and 1.4 g banana (dry weights) per month in summer vs. 0.2 g mealworms and 0.3 g banana per month in winter (N = 6, n = 18; t-test, t = −26.393, p < 0.001 for mealworms; t-test, t = −2.696, p = 0.043 for banana, Lund, 2009).
Torpor Duration, Minimum Tskin, and Oxygen Consumption
Torpor bout length (TBD), minimum oxygen consumption (), and minimum Tskin were significantly different between the five experimental conditions (for data and statistical analyses see Tables 1, 2). During both summer temperature treatments E. telfairi entered short bouts of torpor every day during the first part of the resting phase (Figures 1A,C). Minimum Tskin closely resembled minimum Tn under both summer conditions (Sconst24 and Sfluc19–28) and hourly mean minimum Tskin thus was significantly lower during Sfluc19–28 than during Sconst24 (Tables 1, 2). Furthermore, TBD was almost double under the fluctuating condition and thus significantly longer than during Sconst24 (Tables 1, 2).
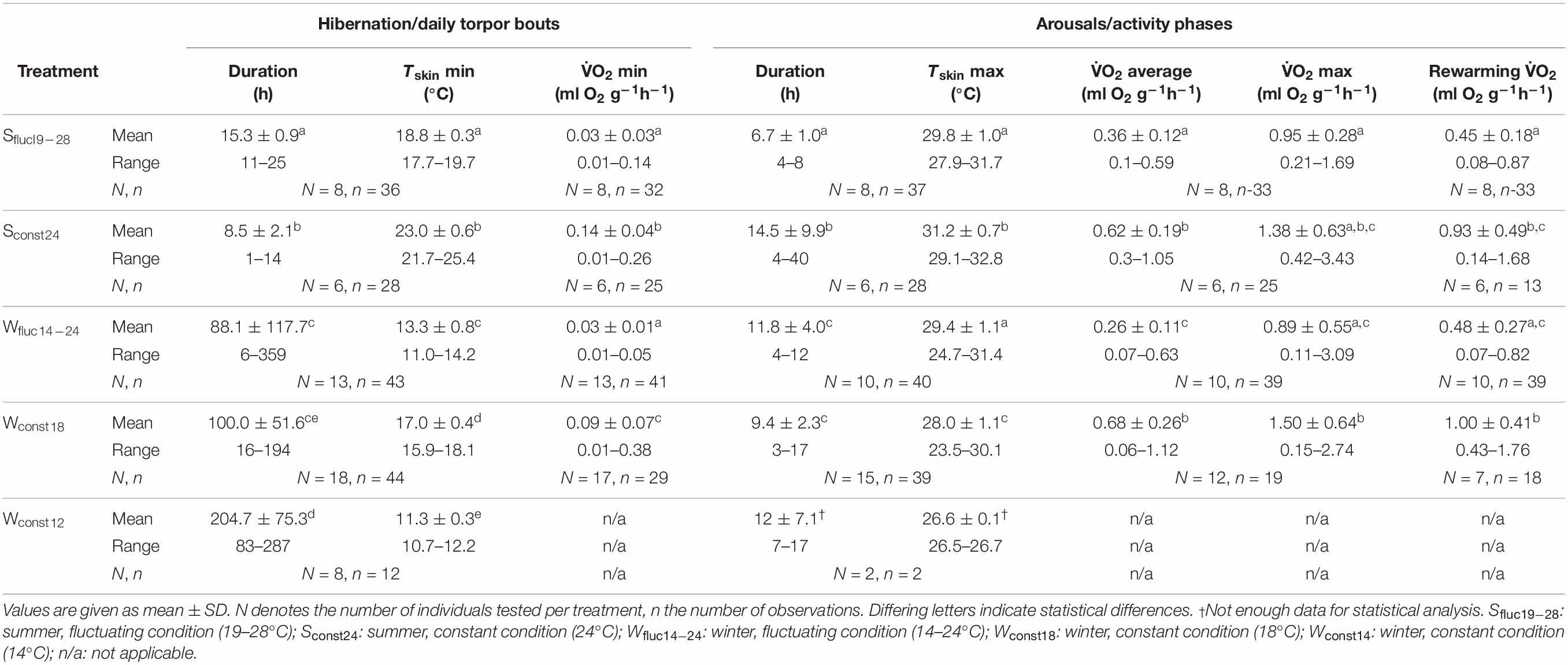
Table 1. Torpor and arousal/activity phase characteristics of Echinops telfairi for all five experimental treatments.
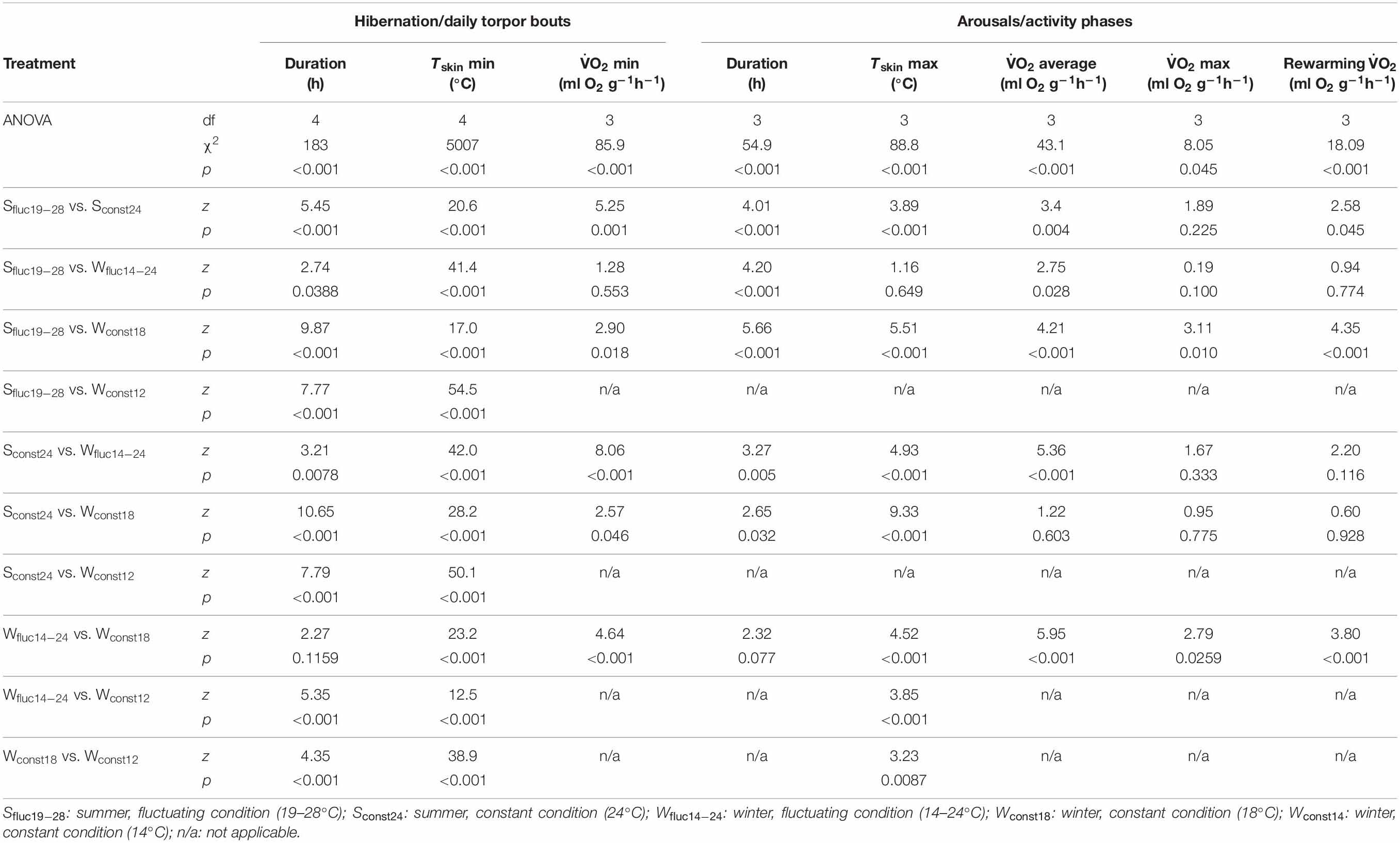
Table 2. Statistical parameters for ANOVA and Tukey post hoc tests for torpor and arousal/activity phase characteristics of Echinops telfairi for all five experimental treatments.
Animals hibernated in all winter conditions. The general hibernation pattern was similar during the Wconst12 and Wconst18 treatments. Animals entered torpor bouts of varying lengths during which Tskin was fairly constant and close to Tn, alternating with periodic arousals (Figure 1B). Torpor bouts during winter were significantly longer than during both summer conditions (Tables 1, 2). Some torpor bouts were interrupted by the end of the experiment and therefore could have even been longer. Torpor bouts lasted twice as long and significantly longer in Wconst12 as in Wconst18 (Tables 1, 2). In fluctuating winter conditions, Tskin closely tracked the Tn cycle during hibernation bouts passively, with the lowest Tskin recorded as 10.7°C under Wconst12 (Figure 1D and Table 1; climate chambers did sometimes deviate a little bit from the set temperature, in this case Ta was slightly cooler than 12°C). Arousals were more frequent in fluctuating than in constant winter temperatures and the hibernation bouts were highly variable in length (range: 6–359 h) and significantly different to the other treatments (except Wconst18; Tables 1, 2). The differences in hourly mean minimum Tskin were significantly different for all five experimental groups (Tables 1, 2).
Minimum during torpor was significantly related to the minimum experimental temperature, i.e., lowest at Wfluc14–24 and Sfluc19–28 (0.03 ml O2∗g–1*h–1 for both) and more than four-fold higher under Sconst24 (0.14 ml O2∗g–1*h–1; Tables 1, 2; Figure 1). Minimum differed significantly between all treatments except between both fluctuating conditions (Tables 1, 2).
Arousals, Maximum Tskin, and Oxygen Consumption
Animals used daily Tn fluctuation for passively rewarming from torpor and only activated endogenous heating after Tskin reached the high daytime Tn passively (Figures 1A,B). At the end of a torpor bout (which coincided with the start of the active rewarming and the initiation of activity phase), increased sharply and remained high until the end of the activity phase when it quickly dropped again (Figure 1). Maximum during arousals or activity phases, average and maximum Tskin reached during arousals and the length of the arousal/activity phase differed significantly between the temperature conditions (Tables 1, 2). Maximum and average during arousals were highest for Sconst24 and Wconst18 ( max: 1.38 ml O2∗g–1*h–1 and 1.50 ml O2∗g–1*h–1; ave: 0.62 ml O2∗g–1*h–1 and 0.68 ml O2∗g–1*h–1; no significant differences between the two for either variable, Table 2), where all heating had to be endogenously initiated, and lowest during Wfluc14–24, where passive heating over most of the Tskin increase reduced energy expenditure (0.89 ml O2∗g–1*h–1), followed by Sfluc19–28 (0.95 ml O2∗g–1*h–1). While Wfluc14–24 and Wconst18 were significantly different for maximum , the difference between Sfluc19–28 and Sconst24 was not significant (Table 2). All other treatments except Sconst24 and Wconst18 were significantly different from each other (Tables 1, 2). Thus, the high levels of maximum during active heating under constant temperature conditions carried over into average during arousals and activity phases.
during rewarming in the fluctuating temperature conditions (0.45 ml O2∗g–1*h–1 in summer and 0.48 ml O2∗g–1*h–1 in winter), including passive heating phases, was only about half, and significantly lower than that of the active heating phases observed under the constant conditions which relied exclusively on endogenous heat production (Tables 1, 2). Arousal rewarming during Sconst24 and Wconst18 was significantly higher than Sfluc19–28 and Wfluc14–24, respectively (Tables 1, 2) indicating energy saved by the use of passive heating. There were, however, no difference between the two fluctuating conditions (Sfluc19–28 and Wfluc14–24) and the two constant conditions (Sconst24 and Wconst18, Table 2).
Tskin followed the pattern of with a lag time that depended on the experimental condition (Figure 1). Hourly mean maximal Tskin during arousals was highest for Sconst24 (Table 1); the highest overall recorded Tskin was 32.8°C. Tskin in the other treatments was slightly, but significantly lower (Tables 1, 2). In the Sconst24 condition, the animals maintained elevated Tskin for about 14 h per day during the activity phase, but only for about 9 h, and significantly shorter, under Sfluc19–28, due to the passive heating phase under the fluctuating conditions (Table 2). Arousal or activity phases were significantly shortest in the Sfluc19–28 treatment (about 7 h; Table 2) and intermediate in the Wfluc14–24 treatment (Figure 2).
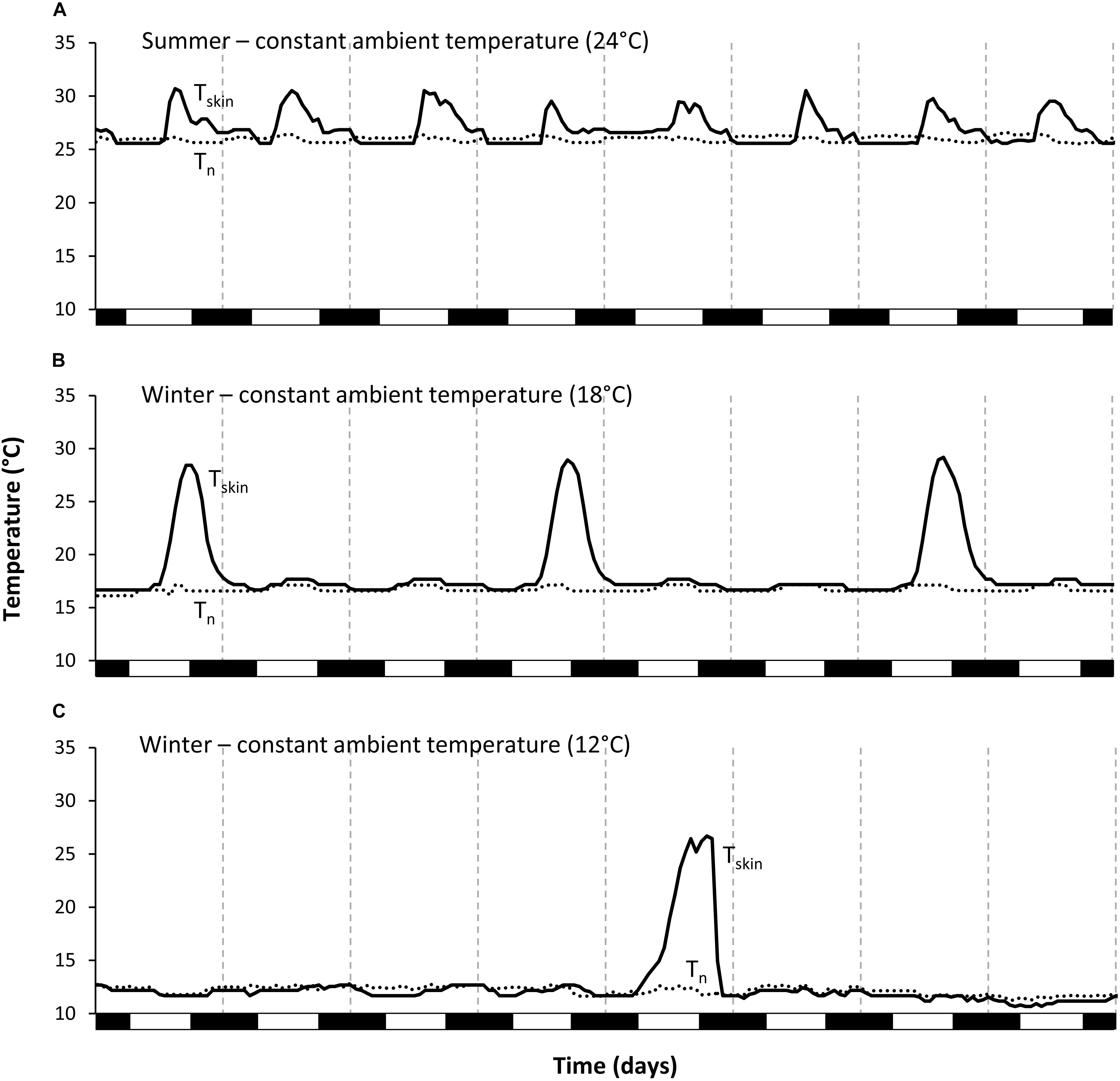
Figure 2. Examples of typical sequences of skin temperature of one individual Echinops telfairi during the different constant treatments over eight days. (A) Summer 24°C, (B) winter 18°C, and (C) winter 12°C. During summer, tenrecs aroused daily to normothermic skin temperature; during winter, torpor bouts became longer, even more so at the lower ambient temperature. Black line: skin temperature; dotted line: nest box temperature; vertical dashed lines indicate midnight, black boxes on the x-axis the scotophase.
The timing of the arousals differed significantly from a random distribution for all treatments (Figure 3). We excluded Wcost12 from arousal data analysis as only two full arousals were recorded. However, there were three unsuccessful attempts at rewarming under this condition (Figure 4), which were never observed in any other treatment. In summer, animals under Sconst24 started to rewarm at 18:43 ± 02:24 h (N = 6, n = 28; Rayleigh test: r = 0.7372, p < 0.001) and reached their maximum Tskin about 2 h later. Animals under Sfluc19–28 used daily Tn fluctuation for passively rewarming from torpor and only activated endogenous heating on average at 11:34 ± 00:35 h (N = 8, n = 37; Rayleigh test: r = 0.978, p < 0.001; Figure 3), which was significantly earlier than under the constant summer treatment (Watson’s test: x = 1.3308, p < 0.001; Figure 3).
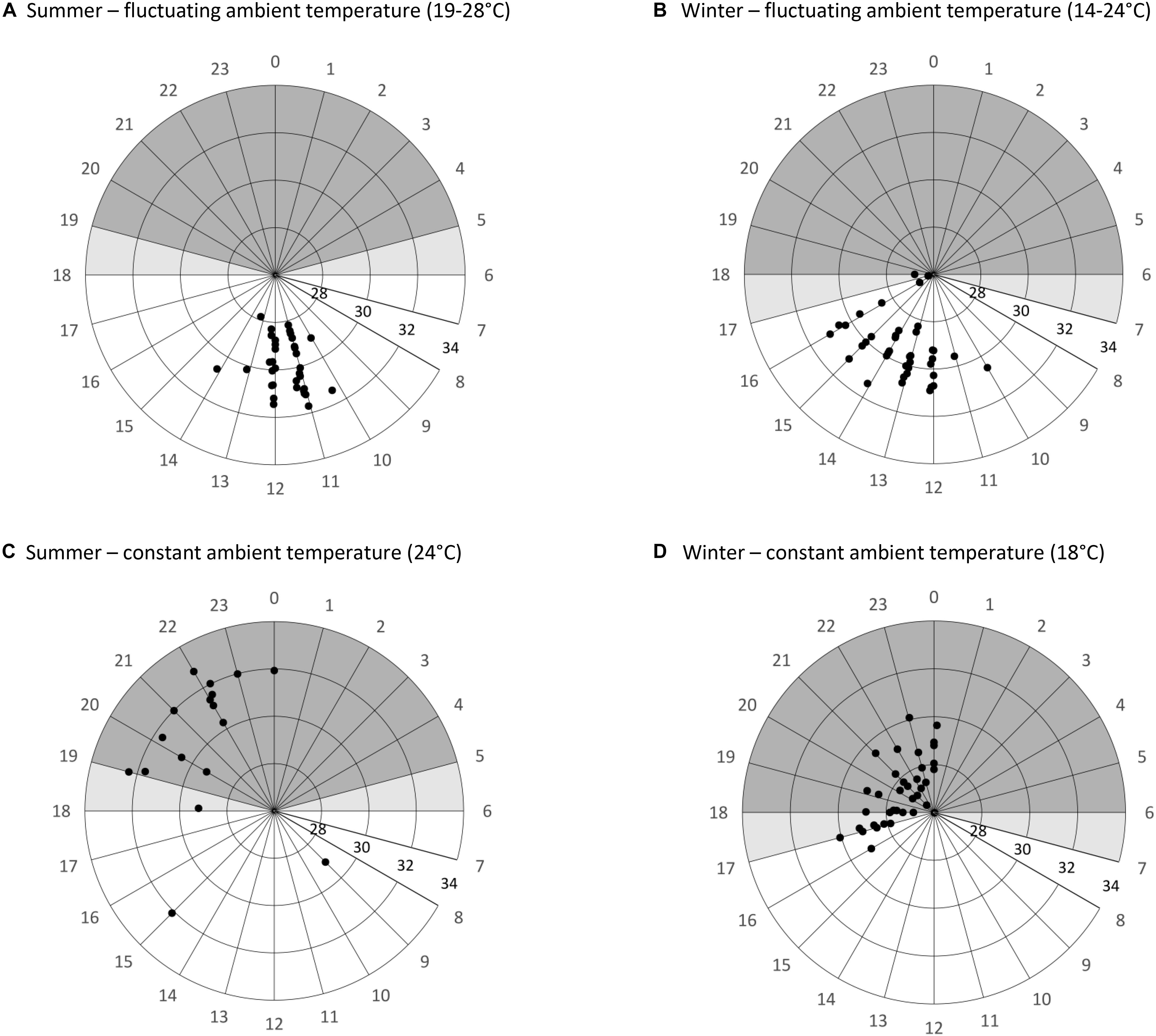
Figure 3. Timing of arousals (initiation of endogenous heat production) and maximum skin temperature during arousals/activity phases of Echinops telfairi for four experimental treatments (A–D). Data shown indicate the time of day (degrees) and level of maximum skin temperature (°C; radial distance). Shaded areas illustrate the scotophase, lighter shaded areas the transitional times between night and day phase. Under fluctuating conditions (A,B), arousals are more synchronous and initiated when Tskin passively reaches maximal Tn well before the beginning of the scotophase. Under constant conditions (C,D), the start of arousals is scattered over a longer time period, but almost always only after the beginning of the scotophase. Maximal Tskin is highest during the activity phases under constant summer condition (24°C), and lowest and least variable during the arousals under constant winter conditions (18°C).
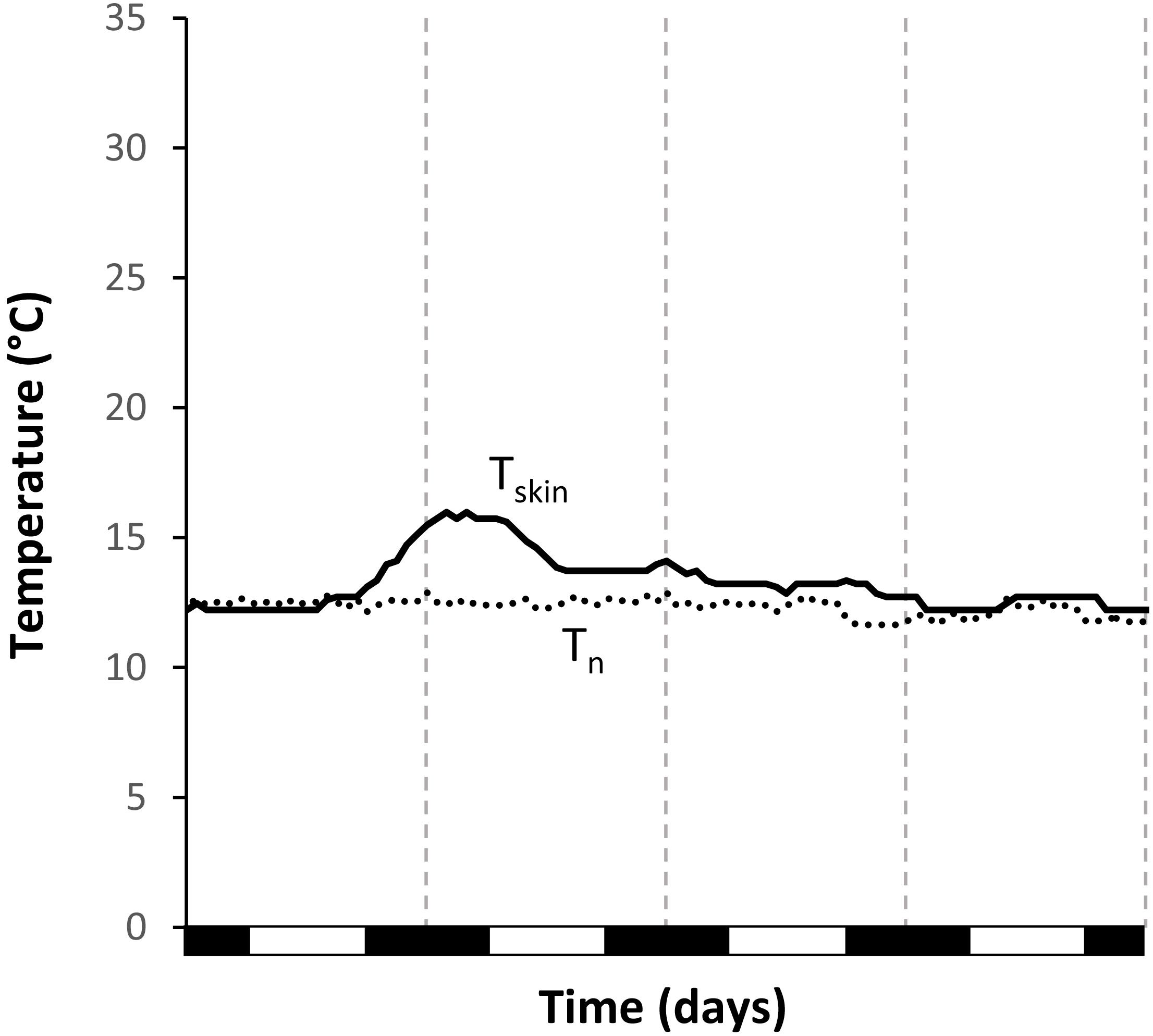
Figure 4. Unsuccessful attempt at rewarming from torpor of Echinops telfairi under constant temperature treatment in winter (Wconst12). During this event, skin temperature reached a maximum of only 14.7°C and only slowly returned to the level of nest box temperature over two days. Black line: skin temperature; dotted line: nest box temperature; vertical dashed lines indicate midnight, black boxes on the x-axis the scotophase.
During winter, arousals were less frequent, and even more so during the constant treatments. During Wconst12 only two arousals were recorded and those varied considerably in timing (15:00 and 22:00 h). There was considerable variation in the timing of arousals under Wconst18. Arousals for Wconst18 started at 19:54 ± 04:27 h (N = 16, n = 40; Rayleigh test: r = 0.896, p < 0.001) and animals needed between four to 6 h to rewarm. For Wfluc14–24 arousals started at 13:51 ± 01:20 h, after Tskin had passively reached high daytime Tn (N = 10, n = 41; Rayleigh test, r = 0.896, p < 0.001; Figure 3). Arousals started more synchronously during both fluctuating treatments than under constant Ta, i.e., the start time of the arousals was less variable (ANOVA, df = 3, χ2 = 51.3, p > 0.001; z > 2.82, p < 0.0212; Figure 3); while arousals were even more synchronized during Sfluc24 than Wfluc18 (deviation from mean 0.43 ± 0.35 min (N = 9, n = 38) and 1.06 ± 0.82 min (N = 10, n = 41), respectively; z = 4.08, p > 0.001), there were no significant differences between the two constant treatments (deviation from mean Sconst24 1.62 ± 0.79 min (N = 7, n = 29) and Wconst18 2.43 ± 1.72 min (N = 16, n = 40); z = 0.20, p = 0.9970). In general, in all analyzed parameters, was always higher in the constant than in the fluctuating conditions (Table 1).
Discussion
Temperature regime was found to have a significant effect on hibernation patterns of the highly heterothermic E. telfairi highlighting the importance of incorporating realistic temperatures in the study of hibernation. The tenrecs entered torpor every day and fluctuating temperatures acted as a zeitgeber that allowed for more synchrony in activity between individuals as well as lower costs of arousal from torpor. Under fluctuating summer Ta (Sfluc19–28) conditions, E. telfairi was able to lower its during daily resting phases to the same low levels as during winter hibernation bouts under fluctuating conditions (both 0.03 ml O2∗g–1*h–1). In both cases torpid metabolic rates were much lower than would be expected from mere Q10 effects when comparing torpid with maximum at maximum Tskin (0.95–0.89 ml O2∗g–1*h–1; decrease in Tb from about 30°C to about 20°C and drop in to less than 5% of resting rates, instead of about 50% predicted by a Q10 of 2–3; Table 1). Thus, during the resting phases of fluctuating summer conditions, the decrease in was not “only” due to the suppression of thermogenesis leading to the highly labile thermoregulation tenrecs are famous for (Nicoll, 1986; Stephenson and Racey, 1994; Lovegrove and Génin, 2008; Levesque et al., 2014), but also an indication of active metabolic inhibition. Only one female, in Sconst24, remained normothermic for longer than 24 h on two occasions. Furthermore, under the constant summer Ta (Sconst24), elevated environmental temperatures precluded the attainment of the lowest levels of torpid , however, was still lower than would be expected by Q10 effects alone (from 1.38 to 0.14 ml O2∗g–1*h–1; about 10% of RMR). However, at least during summer, the tenrecs needed temperature fluctuations, which included lower temperatures, to take advantage of the lowest, most energy saving levels of torpid . In general, fluctuating temperature conditions proved to be energetically more efficient for E. telfairi. As temperatures are predicted to continue to increase with global climate change, this could pose a significant increase in energetic costs during torpor (Lovegrove et al., 2014a).
Despite often occurring at higher Ta (but not always, see Nowack et al., 2020), tropical hibernation and daily torpor has the potential to realize significant energy savings as well as its beneficial effect on water usage (Cooper et al., 2005; Withers et al., 2012). E. telfairi in our study reached minimal levels of metabolism during torpor episodes comparable to that of temperate and arctic species (0.03 ml O2∗g–1*h–1, Heldmaier et al., 2004). However, as active metabolic rates of tenrecs are lower, the relative energy savings are less pronounced. Apparently, this level of torpid metabolism seems to be at a threshold for mammalian minimal MR (see Frappell and Butler, 2004). The average torpid of E. telfairi was 0.06 ml O2∗g–1*h–1, the same as in the Malagasy lemur C. medius (Dausmann et al., 2009), and the greater hedgehog tenrec (0.08–0.1 O2∗g–1*h–1, Levesque and Lovegrove, 2014), indicating a general, or at least Malagasy, level for tropical hibernation. When E. telfairi were hibernating under the fluctuating Ta regime, correlated with Ta and was lower during the colder night phase compared to the warmer day phase. Together with the longer duration of hibernation bouts at lower Ta and thus fewer, energetically costly arousals, it is likely that it is energetically favorable for E. telfairi to hibernate at lower Ta, at least down to a certain limit (see below).
In general, tenrec species have been reported to be highly heterothermic, except when they are pregnant or lactating (Stephenson and Racey, 1993a, b; Poppitt et al., 1994; Levesque and Lovegrove, 2014; Levesque et al., 2014). In our study individuals were not reproducing and became or continued to be torpid every day throughout the study. Flexible thermoregulation reduces general energy expenditure, however, also limits the ambient temperature breadth, over which an organism can function (Treat et al., 2018). Torpor, on the other hand, might counterbalance this disadvantage, broadening the temperature niche. Indeed, torpor use has been found to lower the risk of extinction in highly variable and quickly changing environments (Geiser and Turbill, 2009; Liow et al., 2009). Maximal Tskin during activity and arousal phases was similar in the two summer treatments and the two warmer winter treatments, and was in the same range as body temperature reported for intraperitoneally implanted temperature loggers by Lovegrove and Génin (2008), emphasizing the validity of our Tskin measurements. Maximal Tskin was lowest during the two arousals in the Wconst12 conditions, suggesting limitations of the endogenous heating capacities at constantly low temperatures. In addition, there were also some “unsuccessful” arousals during Wconst12 conditions, suggesting a lower limit for active arousal at around this temperature. Indeed, there seems to be a lower thermal limit for hibernation in E. telfairi. Support for this comes from Scholl (1974), who noted that E. telfairi was not able to arouse successfully at a Ta of 11°C. This sets an ultimate lower limit of Ta for long-term survival in this species and restricts its potential habitats. Although temperatures do drop below 10°C within the range of E. telfairi during winter nights, this does not occur very frequently and will be buffered even in hibernacula with low insulation capacity. More importantly, even on those coldest days, Ta will usually increase above 20°C during the day (Dausmann and Blanco, 2016), ensuring passive rewarming (contrary to Scholl, 1974).
It has been proposed that high costs of rewarming from torpor could limit the efficient employment of heterothermy, reducing energy savings achieved during torpor episodes (Wang, 1979; Humphries et al., 2003), especially for short torpor bouts. However, most terrestrial animals do not live in constant environments, but experience daily and seasonal fluctuations in Ta (Dillon and Woods, 2016; Dillon et al., 2016). Depending on the type and the insulation properties of their resting sites, environmental temperature fluctuations also translate into variable temperatures within the resting sites (Dausmann et al., 2004; Turner, 2020). These fluctuations in Ta can be used for assisted warming from torpor, especially in species living in tropical areas, where daily maximal temperatures tend to be higher than potential torpid Tb set-points, particularly during the winter seasons. Exogenous, mainly passive heating is known from several tropical heterotherms (e.g., Microcebus murinus, Ortmann et al., 1997; Sminthopsis macroura, Lovegrove et al., 1999; Elephantulus myurus, Mzilikazi et al., 2002; Geiser and Drury, 2003; C. medius, Dausmann et al., 2009; Thompson et al., 2015), and makes rewarming comparatively inexpensive with 60–85% reductions when compared to active warming (Lovegrove et al., 1999; Schmid et al., 2000; Geiser and Drury, 2003; Warnecke et al., 2008). The benefit from passive heating, either by daily fluctuations of Ta or by radiant heat, might lead to laboratory studies underestimating the energetic advantage of torpor in free-ranging mammals (Mzilikazi et al., 2002), might explain why daily torpor is common in sunny regions and might occur more frequently at low latitudes than hitherto believed (Geiser and Drury, 2003).
Previous laboratory studies with E. telfairi have used constant Ta for their experiments (Scholl, 1974; Poppitt et al., 1994; Künzle, 1998; Künzle et al., 2007; Oelkrug et al., 2013). In our study, fluctuating Ta treatments mimicked natural conditions. Under these conditions, E. telfairi also used daily Ta fluctuations for passively warming from daily torpor in summer as well as during arousals between hibernation bouts in winter. In both seasons, during rewarming from torpor under the intermediate, constant conditions was about double that of fluctuating temperature indicating an energetic advantage of passive heating. Under fluctuating conditions, the animals only activated endogenous heat production and became active after Tskin reached the high daytime Tn passively around noon, as evident by the sharp rise in MR above this threshold. Ta cycles lead to more uniform and synchronized Tskin patterns. In this way, Ta cycles not only help rewarming, but also synchronize the activity phases and torpor bouts of the animals, in contrast to constant Ta conditions where there was considerable variation in timing. In addition, arousals started about 6 h later under constant conditions, shortly after the lights had gone off. Possibly, for animals under constant temperature conditions light, or rather darkness, was taken as a (less stringent) cue to initiate exogenous heating.
The timing of torpor is not only important for its effective use (Körtner and Geiser, 2000), but synchronization of activity patterns could also be essential for social interactions, successful foraging bouts, and other important activities. Additional external stimuli, such as photoperiod, also affect the timing of torpor (Heldmaier et al., 1982, 1989; Aujard et al., 1998), but in the field these are often coupled to Ta. Furthermore, some species (e.g., the small marsupial dunnarts, Sminthopsis sp.) have been shown to be insensitive to photoperiodic cues (Holloway and Geiser, 1996). A study on E. telfairi in an enclosure in Madagascar with natural climatic parameters by Lovegrove and Génin (2008) also found that the tenrecs used passive exogenous heating by Ta before initiating active heat production. Interestingly, they only found daily and prolonged torpor (maximum of 4 days), but not longer hibernation bouts, possibly due to the timing of the study (beginning of winter), constant supply of food, or lack of suitable hibernacula (tree hollows) in the enclosure. In our study, E. telfairi hibernated for several months in constant, as well as in fluctuating Ta during the hibernation season (winter). Our results show that Ta cycles can be an effective zeitgeber for activity and thermoregulatory rhythms, even during hibernation, and that careful consideration should be given to the choice of temperature regime under laboratory conditions.
During deep hibernation, arctic and temperate species typically display hibernation bouts of about or exceeding two weeks in length (Heldmaier et al., 2004), possibly because of the usually constant, low temperatures that arctic animals are exposed to in their hibernacula (Arnold et al., 1991; Buck and Barnes, 1999). Hibernation bout length is more flexible in tropical hibernators. Some species can exhibit very brief hibernation bouts (Reher et al., 2018), whereas mouse and dwarf lemurs can hibernate for several months without arousals, if they use poorly insulated hibernacula and Tb fluctuates passively with Ta above 30°C at least every couple of days, thus forgoing the need for active arousals with endogenous heating (Dausmann, 2014). Interestingly, fluctuating Ta triggered more frequent arousals during hibernation in E. telfairi in our study, therefore reducing average hibernation bout length from over eight (Wconst12) and four (Wconst18), to about 3.5 days. It is possible that the maximum Tskin that could be attained passively during the fluctuating Ta treatment was not high enough to satisfy physiological demands and thus to be settled during active arousals. This suggests a threshold temperature, below which hibernators have to actively terminate hibernation bouts after a certain time and which may vary between species, individually as well as temporally (Körtner and Geiser, 2000; Dausmann et al., 2005; Turbill et al., 2008; Lovegrove et al., 2014b). An increase in length of hibernation bouts with decreasing Ta has also been found in temperate and arctic species, e.g., the golden-mantled ground squirrel Callospermophilus saturatus (Geiser and Kenagy, 1988), suggesting that this temperature-dependence in hibernation bout length is universal in tropical as well as temperate and arctic hibernators. We found hibernation bout lengths comparable or even above those described by Scholl (1974) for E. telfairi, but contrary to the study by Lovegrove and Génin (2008), hibernation was maintained for several months. Interestingly, the only Tb available from a free-ranging tenrec (Setifer setosus) hibernating in variable ambient temperatures showed no evidence of periodic arousals, although in that study tree hole temperature did not drop below 18°C (Levesque et al., 2014).
As environmental temperature variations are the norm, rather than the exception (Dillon et al., 2016), this highlights the importance of incorporating temperature variability in laboratory evaluations of animal thermoregulation. The importance of incorporating realistic temperature variability in laboratory physiology has been receiving increasing attention as we seek to predict the effects of increasingly varying climates on animal survival (Vasseur et al., 2014; Dillon and Woods, 2016; Levesque et al., 2016). Our findings show that fluctuating Ta cycles not only affect the timing of arousals and the duration of hibernation bouts in the tropical hibernator E. telfairi, but also have an impact on the timing and shape of activity phase and torpor bouts during the non-hibernation season, as well as dramatically influencing energy expenditure in all seasons. Therefore, attempts to understand the energetics and thermoregulation of hibernators would be best served by the inclusion of more realistic temperature cycles to provide a true understanding of the conditions faced by heterotherms in the wild, especially under tropical conditions.
Data Availability Statement
The datasets generated for this study are available on request to the corresponding author.
Ethics Statement
The animal study was reviewed and approved by the Behörde für Gesundheit und Verbraucherschutz (BGV).
Author Contributions
KD and JW conceived the idea and carried out the experiments. All authors contributed to the analyses of the data. JN performed the statistical analyses. KD, DL, and JN wrote the manuscript.
Conflict of Interest
The authors declare that the research was conducted in the absence of any commercial or financial relationships that could be construed as a potential conflict of interest.
Acknowledgments
We want to thank Dr. Karina Montero for sophisticated statistical advice and help in “R,” Stephanie Reher for being a wizard for figures in “R,” Dr. Julian Glos for valuable comments on the manuscript and Klaus Rupp for help with animal husbandry.
References
Arnold, W., Heldmaier, G., Ortmann, S., Pohl, H., Ruf, T., and Steinlechner, S. (1991). Ambient temperatures in hibernacula and their energetic consequences for alpine marmots (Marmota marmota). J. Therm. Biol. 16, 223–226. doi: 10.1016/0306-4565(91)90029-2
Aujard, F., Perret, M., and Vannier, G. (1998). Thermoregulatory responses to variations of photoperiod and ambient temperature in the male lesser mouse lemur: a primitive or an advanced adaptive character? J. Compar. Pyhsiol. B 168, 540–548. doi: 10.1007/s003600050175
Barclay, R. M. R., Kalcounis, M. C., Crampton, L. H., Stefan, C., Vonhof, M. J., Wilkinson, L., et al. (1996). Can external radiotransmitters be used to assess body temperature and torpor in bats. J. Mammal. 77, 1102–1106. doi: 10.2307/1382791
Barnes, B. M. (1989). Freeze avoidance in a mammal - body temperatures below 0°C in an arctic hibernator. Science 244, 1593–1595. doi: 10.1126/science.2740905
Blanco, M. B., Dausmann, K. H., Ranaivoarisoa, J. F., and Yoder, A. D. (2013). Underground hibernation in a primate. Sci. Rep. 3:1768.
Buck, C. L., and Barnes, B. M. (1999). Temperatures of hibernacula and changes in body composition of arctic ground squirrels over winter. J. Mammal. 80, 1264–1276. doi: 10.2307/1383177
Canale, C. I., Levesque, D. L., and Lovegrove, B. G. (2012). “Tropical heterothermy: does the exception prove the rule or force a re-definition?,” in Living in a Seasonal World: Thermoregulatory and Metabolic Adaptations, eds T. Ruf, C. Bieber, W. Arnold, and E. Millesi (Berlin: Springer), 29–40. doi: 10.1007/978-3-642-28678-0_3
Clarke, A., and Rothery, P. (2008). Scaling of body temperature in mammals and birds. Funct. Ecol. 22, 58–67.
Cooper, C., Mcallan, B., and Geiser, F. (2005). Effect of torpor on the water economy of an arid-zone marsupial, the stripe-faced dunnart (Sminthopsis macroura). J. Compar. Physiol. B 175, 323–328. doi: 10.1007/s00360-005-0488-y
Cossins, A. R., and Barnes, B. M. (1996). Southern discomfort. Nature 382, 582–583. doi: 10.1038/382582a0
Cryan, P. M., and Wolf, B. O. (2003). Sex differences in the thermoregulation and evaporative water loss of a heterothermic bat. Lasiurus cinereus, during its spring migration. J. Exp. Biol. 206, 3381–3390. doi: 10.1242/jeb.00574
Dausmann, K. H. (2005). Measuring body temperature in the field-evaluation of external vs. implanted transmitters in a small mammal. J. Ther. Biol. 30, 195–202. doi: 10.1016/j.jtherbio.2004.11.003
Dausmann, K. H. (2014). Flexible patterns in energy savings: heterothermy in primates. J. Zool. 292, 101–111. doi: 10.1111/jzo.12104
Dausmann, K. H., and Blanco, M. B. (2016). “Possible causes and consequences of different hibernation patterns in Cheirogaleus species: Mitovy fatsy sahala,” in Dwarf and Mouse Lemurs of Madagascar: Biology, Behavior and Conservation Biogeography of the Cheirogaleidae, eds S. M. Lehman, U. Radespiel, and E. Zimmermann (Cambridge: Cambridge University Press), 335–349.
Dausmann, K. H., Glos, J., Ganzhorn, J. U., and Heldmaier, G. (2004). Hibernation in a tropical primate. Nature 429, 825–826. doi: 10.1038/429825a
Dausmann, K. H., Glos, J., Ganzhorn, J. U., and Heldmaier, G. (2005). Hibernation in the tropics: lessons from a primate. J. Compar. Physiol. B 175, 147–155. doi: 10.1007/s00360-004-0470-0
Dausmann, K. H., Glos, J., and Heldmaier, G. (2009). Energetics of tropical hibernation. J. Compar. Physiol. B 179, 345–357. doi: 10.1007/s00360-008-0318-0
Dillon, M. E., and Woods, H. A. (2016). Introduction to the symposium: beyond the mean: biological impacts of changing patterns of temperature variation. Integr. Compar. Biol. 56, 11–13. doi: 10.1093/icb/icw020
Dillon, M. E., Woods, H. A., Wang, G., Fey, S. B., Vasseur, D. A., Telemeco, R. S., et al. (2016). Life in the frequency domain: the biological impacts of changes in climate variability at multiple time scales. Integr. Compar. Biol. 56, 14–30. doi: 10.1093/icb/icw024
Eisenberg, J. F., and Gould, E. (1969). The tenrecs: a study in mammalian behavior and evolution. Smithsonian Contribut. Zool. 27, 1–156.
Frappell, P. B., and Butler, P. J. (2004). Minimal metabolic rate, what it is, its usefulness, and its relationship to the evolution of endothermy: a brief synopsis. Physiol. Biochem. Zool. 77, 865–868. doi: 10.1086/425191
Geiser, F. (2004). Metabolic rate and body temperature reduction during hibernation and daily torpor. Annu. Rev. Physiol. 66, 239–274. doi: 10.1146/annurev.physiol.66.032102.115105
Geiser, F., and Brigham, R. M. (2012). “The other functions of torpor,” in Living in a Seasonal World: Thermoregulatory and Metabolic Adaptations, eds T. Ruf, C. Bieber, W. Arnold, and E. Millesi (Heidelberg: Springer).
Geiser, F., and Drury, R. L. (2003). Radiant heat affects thermoregulation and energy expenditure during rewarming from torpor. J. Compar. Physiol. B 173, 55–60. doi: 10.1007/s00360-002-0311-y
Geiser, F., and Kenagy, G. (1988). Torpor duration in relation to temperature and metabolism in hibernating ground squirrels. Physiol. Zool. 61, 442–449. doi: 10.1086/physzool.61.5.30161266
Geiser, F., and Körtner, G. (2010). Hibernation and daily torpor in Australian mammals. Austr. Zool. 35, 204–215. doi: 10.7882/az.2010.009
Geiser, F., and Turbill, C. (2009). Hibernation and daily torpor minimize mammalian extinctions. Naturwissenschaften 96, 1235–1240. doi: 10.1007/s00114-009-0583-0
Grigg, G. C., and Beard, L. A. (2000). “Hibernation by echidnas in mild climates: hints about the evolution of endothermy?,” in Life in the Cold: Eleventh Internation Hibernation Symposium, eds G. Heldmaier and M. Klingenspor (New York, NY: Springer), 5–20.
Grigg, G. C., Beard, L. A., and Augee, M. L. (2004). The evolution of endothermy and its diversity in mammals and birds. Physiol. Biochem. Zool. 77, 982–997. doi: 10.1086/425188
Halekoh, U., Højsgaard, S., and Yan, J. (2006). The R package geepack for generalized estimating equations. J. Statist. Softw. 15, 1–11.
Heldmaier, G., Ortmann, S., and Elvert, R. (2004). Natural hypometabolism during hibernation and daily torpor in mammals. Respir. Physiol. Neurobiol. 141, 317–329. doi: 10.1016/j.resp.2004.03.014
Heldmaier, G., and Steinlechner, S. (1981). Seasonal control of energy requirements for thermoregulation in the Djungarian hamster (Phodopus sungorus), living in natural photoperiod. J. Compar. Physiol. 142, 429–437. doi: 10.1007/bf00688972
Heldmaier, G., Steinlechner, S., Rafael, J., and Latteier, B. (1982). Photoperiod and ambient temperature as environmental cues for seasonal thermogenic adaptation in the Djungarian hamster, Phodopus sungorus. Int. J. Biometeorol. 26, 339–345. doi: 10.1007/bf02219505
Heldmaier, G., Steinlechner, S., Ruf, T., Wiesinger, H., and Klingenspor, M. (1989). Photoperiod and thermoregulation in vertebrates: body temperature rhythms and thermogenic acclimation. J. Biol. Rhythms 4, 139–153. doi: 10.1177/074873048900400211
Holloway, J. C., and Geiser, F. (1996). Reproductive status and torpor of the marsupial Sminthopsis crassicaudata: effect of photoperiod. J. Ther. Biol. 21, 373–380. doi: 10.1016/s0306-4565(96)00023-x
Hothorn, T., Bretz, F., and Westfall, P. (2008). Simultaneous inference in general parametric models. Biomet. J. 50, 346–363. doi: 10.1002/bimj.200810425
Humphries, M. M., Thomas, D. W., and Kramer, D. L. (2003). The role of energy availability in mammalian hibernation: a cost-benefit approach. Physiol. Biochem. Zool. 76, 165–179. doi: 10.1086/367950
Jammalamadaka, S. R., and Sengupta, A. (2001). Topics in Circular Statistics. Singapore: World Scientific.
Jury, M. (2003). “The climate of madagascar,” in The Natural History of Madagascar, eds S. M. Goodman and J. P. Benstead (Chicago, IL: The University of Chicago Press), 75–87.
Kobbe, S., and Dausmann, K. H. (2009). Hibernation in Malagasy mouse lemurs as a strategy to counter environmental challenge. Naturwissenschaften 96, 1221–1227. doi: 10.1007/s00114-009-0580-3
Körtner, G., and Geiser, F. (2000). The temporal organization of daily torpor and hibernation: circadian and circannual rhythms. Chronobiol. Int. 17, 103–128. doi: 10.1081/cbi-100101036
Körtner, G., Rojas, A. D., and Geiser, F. (2010). Thermal biology, torpor use and activity patterns of a small diurnal marsupial from a tropical desert: sexual differences. J. Compar. Physiol. B 180, 869–876. doi: 10.1007/s00360-010-0459-9
Künzle, H. (1998). Care and breeding of the Madagascan hedgehog tenrec, Echinops telfairi, under laboratory conditions. Der Tierschutzbeauftragte 7:98.
Künzle, H., Nautrup, C. P., and Schwarzenberger, F. (2007). High inter-individual variation in the gestation length of the hedgehog tenrec, Echinops telfairi (Afrotheria). Anim. Reproduct. Sci. 97, 364–374. doi: 10.1016/j.anireprosci.2006.02.011
Levesque, D. L., Lobban, K. D., and Lovegrove, B. G. (2014). Effects of reproductive status and high ambient temperatures on the body temperature of a free-ranging basoendotherm. J. Compar. Physiol. B 184, 1041–1053. doi: 10.1007/s00360-014-0858-4
Levesque, D. L., and Lovegrove, B. G. (2014). Increased homeothermy during reproduction in a basal placental mammal. J. Exp. Biol. 217, 1535–1542. doi: 10.1242/jeb.098848
Levesque, D. L., Nowack, J., and Stawski, C. (2016). Modelling mammalian energetics: the heterothermy problem. Clim. Change Responses 3:7.
Liow, L. H., Fortelius, M., Lintulaakso, K., Mannila, H., and Stenseth, N. C. (2009). Lower extinction risk in sleep-or-hide mammals. Am. Natur. 173, 264–272. doi: 10.1086/595756
Lovegrove, B. G. (2012a). The evolution of endothermy in Cenozoic mammals: a plesiomorphic-apomorphic continuum. Biol. Rev. 87, 128–162. doi: 10.1111/j.1469-185x.2011.00188.x
Lovegrove, B. G. (2012b). “A single origin of heterothermy in mammals,” in Living in a Seasonal World: Thermoregulatory and Metabolic Adaptations, eds T. Ruf, C. Bieber, W. Arnold, and E. Millesi (Berlin: Springer), 3–11. doi: 10.1007/978-3-642-28678-0_1
Lovegrove, B. G., Canale, C. I., Levesque, D. L., Fluch, G., Øeháková-Petrù, M., and Ruf, T. (2014a). Are tropical small mammals physiologically vulnerable to Arrhenius effects and climate change? Physiol. Biochem. Zool. 87, 30–45. doi: 10.1086/673313
Lovegrove, B. G., Lobban, K. D., and Levesque, D. L. (2014b). Mammal survival at the Cretaceous–Palaeogene boundary: metabolic homeostasis in prolonged tropical hibernation in tenrecs. Proc. R. Soc. B Biol. Sci. 281, 20141304. doi: 10.1098/rspb.2014.1304
Lovegrove, B. G., and Génin, F. (2008). Torpor and hibernation in a basal placental mammal, the Lesser Hedgehog Tenrec, Echinops telfairi. J. Compar. Physiol. B 178, 691–698. doi: 10.1007/s00360-008-0257-9
Lovegrove, B. G., Körtner, G., and Geiser, F. (1999). The energetic cost of arousal from torpor in the marsupial Sminthopsis macroura: benefits of summer ambient temperature cycles. J. Compar. Physiol. B 169, 11–18. doi: 10.1007/s003600050188
Lund, S. (2009). Die Verdauungseffizienz des Kleinen Madegassichen Igeltenreks Echinops telfairi im jahreszeitlichen Verlauf. Bachelorthesis: University Hamburg.
Lyman, C. P., Willis, J. S., Malan, A., and Wang, L. C. H. (1982). Hibernation and Torpor in Mammals and Birds. New York, NY: Academic Press.
Macmillen, R. E. (1965). Aestivation in the cactus mouse, Peromyscus eremicus. Compar. Biochem. Physiol. 16, 227–248. doi: 10.1016/0010-406x(65)90062-9
McKechnie, A. E., and Mzilikazi, N. (2011). Heterothermy in afrotropical mammals and birds: a review. Integr. Compar. Biol. 51, 349–363. doi: 10.1093/icb/icr035
Mzilikazi, N., Lovegrove, B. G., and Ribble, D. O. (2002). Exogenous passive heating during torpor arousal in free-ranging rock elephant shrews, Elephantulus myurus. Oecologia 133, 307–314. doi: 10.1007/s00442-002-1052-z
Nicoll, M. E. (1986). Diel variation in body temperature in Tenrec ecaudatus during seasonal hypothermia. J. Mammal. 67, 759–762. doi: 10.2307/1381143
Nowack, J., Levesque, D. L., Reher, S., and Dausmann, K. H. (2020). Variable climates lead to varying phenotypes: ‘weird’ mammalian torpor and lessons from lower latitudes. Front. Ecol. Evol. 8:60. doi: 10.3389/fevo.2020.00060
Nowack, J., Mzilikazi, N., and Dausmann, K. H. (2010). Torpor on demand: heterothermy in the non-lemur primate Galago moholi. PLoS ONE 5:e10797. doi: 10.1371/journal.pone.0010797
Nowack, J., Stawski, C., and Geiser, F. (2017). More functions of torpor and their roles in a changing world. J. Compar. Physiol. B 187, 889–897. doi: 10.1007/s00360-017-1100-y
Oelkrug, R., Goetze, N., Exner, C., Lee, Y., Ganjam, G. K., Kutschke, M., et al. (2013). Brown fat in a protoendothermic mammal fuels eutherian evolution. Nat. Commun. 4:e2140.
Ortmann, S., Heldmaier, G., Schmid, J., and Ganzhorn, J. (1997). Spontaneous daily torpor in Malagasy mouse lemurs. Naturwissenschaften 84, 28–32. doi: 10.1007/s001140050344
Pohl, H. H. (1998). Temperature cycles as zeitgeber for the circadian clock of two burrowing rodents, the normothermic antelope ground squirrel and the heterothermic Syrian hamster. Biol. Rhythm Res. 29, 311–325. doi: 10.1076/brhm.29.3.311.1436
Poppitt, S. D., Speakman, J. R., and Racey, P. A. (1994). Energetics of reproduction in the lesser hedgehog tenrec, Echinops telfairi (Martin). Physiol. Zool. 67, 976–994. doi: 10.1086/physzool.67.4.30163874
Pretzlaff, I., and Dausmann, K. H. (2012). “Impact of climatic variation on the hibernation physiology of Muscardinus avellanarius,” in Living in a Seasonal World, eds T. Ruf, C. Bieber, W. Arnold, and E. Millesi (Berlin: Springer), 85–97. doi: 10.1007/978-3-642-28678-0_8
R Development Core Team (2018). R: A Language and Environment for Statistical Computing. Vienna: R Foundation for Statistical Computing.
Reher, S., Ehlers, J., Rabarison, H., and Dausmann, K. H. (2018). Short and hyperthermic torpor responses in the Malagasy bat Macronycteris commersoni reveal a broader hypometabolic scope in heterotherms. J. Compar. Physiol. B 188, 1015–1027. doi: 10.1007/s00360-018-1171-4
Ruf, T., and Geiser, F. (2015). Daily torpor and hibernation in birds and mammals. Biol. Rev. 90, 891–926. doi: 10.1111/brv.12137
Ruf, T., Streicher, U., Stalder, G. L., Nadler, T., and Walzer, C. (2015). Hibernation in the pygmy slow loris (Nycticebus pygmaeus): multiday torpor in primates is not restricted to Madagascar. Sci. Rep. 5:17392.
Schmid, J. (2000). Daily torpor in the gray mouse lemur (Microcebus murinus) in Madagascar: energetic consequences and biological significance. Oecologia 123, 175–183. doi: 10.1007/s004420051003
Schmid, J., Ruf, T., and Heldmaier, G. (2000). Metabolism and temperature regulation during daily torpor in the smallest primate, the pygmy mouse lemur (Microcebus myoxinus) in Madagascar. J. Compar. Physiol. B 170, 59–68. doi: 10.1007/s003600050008
Schmid, J., and Speakman, J. R. (2009). Torpor and energetic consequences in free-ranging grey mouse lemurs (Microcebus murinus): a comparison of dry and wet forests. Naturwissenschaften 96, 609–620. doi: 10.1007/s00114-009-0515-z
Scholl, P. (1974). Temperatuuregulation beim madagassischen Igeltanrek Echinops telfairi (Martin, 1838). J. Compar. Physiol. 89, 175–195. doi: 10.1007/bf00694790
Soarimalala, V., and Goodman, S. (2011). Les petits mammifères de Madagascar. Antananarivo: Association Vahatra.
Stawski, C., and Geiser, F. (2011). Do season and distribution affect thermal energetics of a hibernating bat endemic to the tropics and subtropics? Am. J. Physiol. Regul. Integr. Compar. Physiol. 301, R542–R547.
Stephenson, P. J., and Racey, P. A. (1993a). Reproductive energetics of the Tenrecidae (Mammalia: Insectivora). I. The large-eared tenrec, Geogale aurita. Physiol. Zool. 66, 643–663. doi: 10.1086/physzool.66.5.30163816
Stephenson, P. J., and Racey, P. A. (1993b). Reproductive energetics of the Tenrecidae (Mammalia: Insectivora). II. The shrew-tenrecs, Microgale spp. Physiol. Zool. 66, 664–685. doi: 10.1086/physzool.66.5.30163817
Stephenson, P. J., and Racey, P. A. (1994). Seasonal variation in resting metabolic rate and body temperature of streaked tenrecs, Hemicentetes nigriceps and H. semispinosus (Insectivora: Tenrecidae). J. Zool. 232, 285–294. doi: 10.1111/j.1469-7998.1994.tb01573.x
Streicher, U., Nowack, J., Stalder, G., Walzer, C., Nadler, T., and Ruf, T. (2017). Hibernation in pygmy lorises (Nycticebus pygmaeus)–what does it mean? Vietnam. J. Primatol. 5, 51–57.
Thompson, M. L., Mzilikazi, N., Bennett, N. C., and Mckechnie, A. E. (2015). Solar radiation during rewarming from torpor in elephant shrews: supplementation or substitution of endogenous heat production? PLoS ONE 10:e0120442. doi: 10.1371/journal.pone.0120442
Treat, M. D., Scholer, L., Barrett, B., Khachatryan, A., Mckenna, A. J., Reyes, T., et al. (2018). Extreme physiological plasticity in a hibernating basoendothermic mammal, Tenrec ecaudatus. J. Exper. Biol. 221:jeb185900. doi: 10.1242/jeb.185900
Turbill, C., and Geiser, F. (2008). Hibernation by tree-roosting bats. J. Compar. Physiol. B 178, 597–605. doi: 10.1007/s00360-007-0249-1
Turbill, C., Körtner, G., and Geiser, F. (2008). Timing of the daily temperature cycle affects the critical arousal temperature and energy expenditure of lesser long-eared bats. J. Exp. Biol. 211, 3871–3878. doi: 10.1242/jeb.023101
Turner, J. M. (2020). The interrelationship between torpor expression and nest site use of western and eastern pygmy-possums (Cercartetus spp.). Austr. Mammal. 42, 85–95.
Vasseur, D. A., Delong, J. P., Gilbert, B., Greig, H. S., Harley, C. D. G., Mccann, K. S., et al. (2014). Increased temperature variation poses a greater risk to species than climate warming. Proc. R. Soc. B Biol. Sci. 281:20132612. doi: 10.1098/rspb.2013.2612
Vivanco, P., Rol, M. Á., and Madrid, J. A. (2010). Temperature cycles trigger nocturnalism in the diurnal homeotherm Octodon degus. Chronobiol. Int. 27, 517–534. doi: 10.3109/07420521003743660
Wang, L. C. H. (1979). Time patterns and metabolic rates of natural torpor in Richardson’s ground squirrel. Can. J. Zool. 57, 149–155. doi: 10.1139/z79-012
Warnecke, L., and Geiser, F. (2010). The energetics of basking behaviour and torpor in a small marsupial exposed to simulated natural conditions. J. Compar. Physiol. B 180, 437–445.
Warnecke, L., Turner, J. M., and Geiser, F. (2008). Torpor and basking in a small arid zone marsupial. Naturwissenschaften 95, 73–78.
Withers, P. C., Cooper, C. E., and Nespolo, R. F. (2012). Evaporative water loss, relative water economy and evaporative partitioning of a heterothermic marsupial, the monito del monte (Dromiciops gliroides). J. Exp. Biol. 215, 2806–2813.
Keywords: hibernation, torpor, body temperature, zeitgeber, tropics, Echinops telfairi
Citation: Dausmann KH, Levesque DL, Wein J and Nowack J (2020) Ambient Temperature Cycles Affect Daily Torpor and Hibernation Patterns in Malagasy Tenrecs. Front. Physiol. 11:522. doi: 10.3389/fphys.2020.00522
Received: 04 February 2020; Accepted: 28 April 2020;
Published: 28 May 2020.
Edited by:
Dehua Wang, Institute of Zoology (CAS), ChinaReviewed by:
Viviana Carmen Lo Martire, University of Bologna, ItalyStefano Bastianini, University of Bologna, Italy
Copyright © 2020 Dausmann, Levesque, Wein and Nowack. This is an open-access article distributed under the terms of the Creative Commons Attribution License (CC BY). The use, distribution or reproduction in other forums is permitted, provided the original author(s) and the copyright owner(s) are credited and that the original publication in this journal is cited, in accordance with accepted academic practice. No use, distribution or reproduction is permitted which does not comply with these terms.
*Correspondence: Kathrin H. Dausmann, a2F0aHJpbi5kYXVzbWFubkB1bmktaGFtYnVyZy5kZQ==