- 1Sanya Maternity and Child Care Hospital, Sanya, China
- 2Proteomic Core Facility, Oxford University, Oxford, United Kingdom
- 3New York State Institute for Basic Research in Developmental Disabilities, Staten Island, NY, United States
Introduction: Spontaneous preterm birth (sPTB), which predominantly presents as spontaneous preterm labor (sPTL) or prelabor premature rupture of membranes (PPROM), is a syndrome that accounts for 5–10% of live births annually. The long-term morbidity in surviving preterm infants is significantly higher than that in full-term neonates. The causes of sPTB are complex and not fully understood. Human placenta, the maternal and fetal interface, is an environmental core of fetal intrauterine life, mediates fetal oxygen exchange, nutrient uptake, and waste elimination and functions as an immune-defense organ. In this study, the molecular signature of preterm birth placenta was assessed and compared to full-term placenta by proteomic profiling.
Materials and Methods: Four groups of fetal membranes (the amniochorionic membranes), with five cases in each group in the discovery study and 30 cases in each group for validation, were included: groups A: sPTL; B: PPROM; C: full-term birth (FTB); and D: full-term premature rupture of membrane (PROM). Fetal membranes were dissected and used for proteome quantification study. Maxquant and Perseus were used for protein quantitation and statistical analysis. Both fetal membranes and placental villi samples were used to validate proteomic discovery.
Results: Proteomics analysis of fetal membranes identified 2,800 proteins across four groups. Sixty-two proteins show statistical differences between the preterm and full-term groups. Among these differentially expressed proteins are (1) proteins involved in inflammation (HPGD), T cell activation (PTPRC), macrophage activation (CAPG, CD14, and CD163), (2) cell adhesion (ICAM and ITGAM), (3) proteolysis (CTSG, ELANE, and MMP9), (4) antioxidant (MPO), (5) extracellular matrix (ECM) proteins (APMAP, COL4A1, LAMA2, LMNB1, LMNB2, FBLN2, and CSRP1) and (6) metabolism of glycolysis (PKM and ADPGK), fatty acid synthesis (ACOX1 and ACSL3), and energy biosynthesis (ATP6AP1 and CYBB).
Conclusion: Our molecular signature study of preterm fetal membranes revealed inflammation as a major event, which is inconsistent with previous findings. Proteolysis may play an important role in fetal membrane rupture. Extracellular matrix s have been altered in preterm fetal membranes due to proteolysis. Metabolism was also altered in preterm fetal membranes. The molecular changes in the fetal membranes provided a significant molecular signature for PPROM in preterm syndrome.
Introduction
Preterm birth refers to infants born alive before 37 weeks of gestation (Quinn et al., 2016). Preterm birth accounts for 5–12% of all live births worldwide (Gezer et al., 2018). As a populated country, China has about 1.17 million preterm infants birth every year (World Health Organization [WHO], 2018). In particular, since 2016, when the Chinese government relaxed the one-child policy and implemented its two-children policy, many women of high maternal age rushed to have a second child (Cheng and Duan, 2016). It has been demonstrated that pregnancy at 40 years of age and older is strongly associated with preterm birth and other disorders of pregnancy (Fuchs et al., 2018). In preterm newborns, the brain, lung, liver, and other organs are not fully developed, and there is a high incidence of brain injury, neonatal respiratory distress syndrome, bilirubin encephalopathy, and multiple organ failure (Fraser et al., 2004; Bhutani and Wong, 2013; Paton et al., 2017). It is estimated by the WHO that preterm birth is the most common cause of children’s death under the age of 5 years (World Health Organization [WHO], 2018). Preterm birth brings a great economic burden to families, communities, and societies.
The placenta serves as the interface between the pregnant mother and the intrauterine fetus, with the main function of exchanging the material between the fetus and the mother, providing the oxygen and nutrients required for embryonic, as well as fetal, development, and excreting metabolic waster and CO2 (Gude et al., 2004). In addition, the placenta also serves as a barrier to bacteria, pathogens, and drugs because of the existence of the placental barrier (Zeldovich et al., 2013). The placenta can also synthesize chorionic gonadotropin, human placental lactogen (hPL), estrogen, progesterone, cytokine, and growth factors (Costa, 2016). In addition, the placenta has immune tolerance to the fetus (Guleria and Sayegh, 2007). Therefore, abnormal placental function has a direct correlation with the occurrence of preterm birth. So far, estrogens, hPL, placenta growth factor (PLGF), human chorionic gonadotrophin (hCG), plasma protein A (PAPP-A), placental protein 13 (PP-13), pregnancy-specific glycoproteins, and progesterone metabolites have been employed as surrogate markers of placental function (Heazell et al., 2015). Despite recent progress on the study of these markers associated with function of placenta, a comprehensive and systemic understanding of the pathophysiology of the placenta is lacking, particularly little is known about the pathogenic role of fetal membrane and its involvement in the development of spontaneous preterm birth (sPTB). Here, we studied the protein expression of fetal membrane, with the aim to understand the pathophysiology of fetal membrane and to identify novel molecules associated with preterm birth in the fetal membrane. This study not only provides theoretical support for the occurrence of preterm birth, but also provides reference for the early diagnosis and early intervention of premature birth.
Materials and Methods
Specimens
The study was approved by the Hospital Ethics Committee of Sanya Maternity and Child Care Hospital and the informed consent was obtained from pregnant women to permit the use of placentas in research studies. A retrospective study was designed to investigate the pathological alteration of protein expression with a pre-banked birth cohort of 20 placentas, which were grouped as (A): spontaneous preterm labor (sPTL), defined as a non-medical and/or non-selective spontaneous birth delivered between 20+1 and 36+6 gestational week (GW) in which regular contractions of the uterus result in changes in the cervix before 37 weeks of pregnancy, (B): prelabor premature rupture of membranes (PPROM) that occurred between 20+1 and 36+6 GW, (C): full-term birth (FTB) between 39+1 and 40+6 GW, and (D): full-term premature rupture of membrane (PROM). There were five placentas in each group. The placentas were collected from fetal membranes through full layers to the decidua. Fetal membranes were dissected within 2 cm of the edge where the membrane naturally ruptured during labor. However, if a rupture hole could be identified in the premature rupture (PPROM and PROM) cases, the membrane would be collected within 1 cm around the hole where the premature rupture occurred.
Criteria for Inclusion and Exclusion
Tissues selected from prebanked samples had to meet the following criteria: (i) age of the pregnant woman is 18–45 years, (ii) no clinically recognized infection/inflammation (INF) before and/or during pregnancy (INF is determined by phenotypically notable fever, increased counts of peripheral white blood cells, and/or increased IL6 and/or TNFα), (iii) primipara and singleton without history of miscarriage or abortion, (iv) vaginal delivery with (PPROM and PROM) or without (FTB and sPTL) premature rupture of chorioamniotic membrane, (v) no vaginal bleeding during the pregnancy, (vi) no other pregnancy-related complication(s) and no clinical intervention with antibiotics, steroids, or tocolytics during the pregnancy, (vii) no family history of birth defects, and (viii) no consanguinity. Any cases not meeting the above criteria were excluded. Details of demographic and clinical information for the samples studied are provided in Table 1.
Protein Extraction From Placenta Membrane for Proteomic Analysis
The human placental amniochorionic membrane, or fetal membrane, was dissected (20- to 30-mg cross-sections) and first washed with cold PBS containing protease inhibitor (Sigma-Aldrich, United Kingdom), then placed in beads-beater tubes containing RIPA lysis buffer (Thermo Fisher Scientific, United Kingdom) to make it 50 mg/ml. Tissues were homogenized four times at 6,500 Hz for 40 s in a beads-beater (Stretton, United Kingdom) (Figure 1). The samples were centrifuged at 10,000 g for 5 min at 4°C to remove insoluble tissue debris. The protein concentration in the homogenates was determined by BCA assay (Thermo Fisher Scientific, United Kingdom), and 100 μg of total proteins were added to a 30-kDa filter (Merck Millipore, United Kingdom). Proteins were reduced by 10 mM Dithiothreitol (DTT) (Sigma, United Kingdom) at 37°C for 1 h and then alkylated with 40 mM iodoacetamide (IAA, Sigma, United Kingdom) for 45 min in the dark, at room temperature. Samples were centrifuged for 20 min at 14,000 g to remove DTT and IAA, followed by buffer exchange with 8 M urea twice and 50 mM ammonia bicarbonate three times. One hundred microliters of trypsin were added at a trypsin/protein ratio of 1:50 for digestion at 37°C overnight. Digested peptides were collected by upside down spin, and membrane filters were washed twice with 0.5 M NaCl and water, respectively. The peptides were purified by a SepPak C18 cartridge (Waters, United Kingdom), dried by SpeedVac centrifugation, and resuspended in buffer A (2% acetonitrile, 0.1% formic acid) for LC-MS/MS analysis.
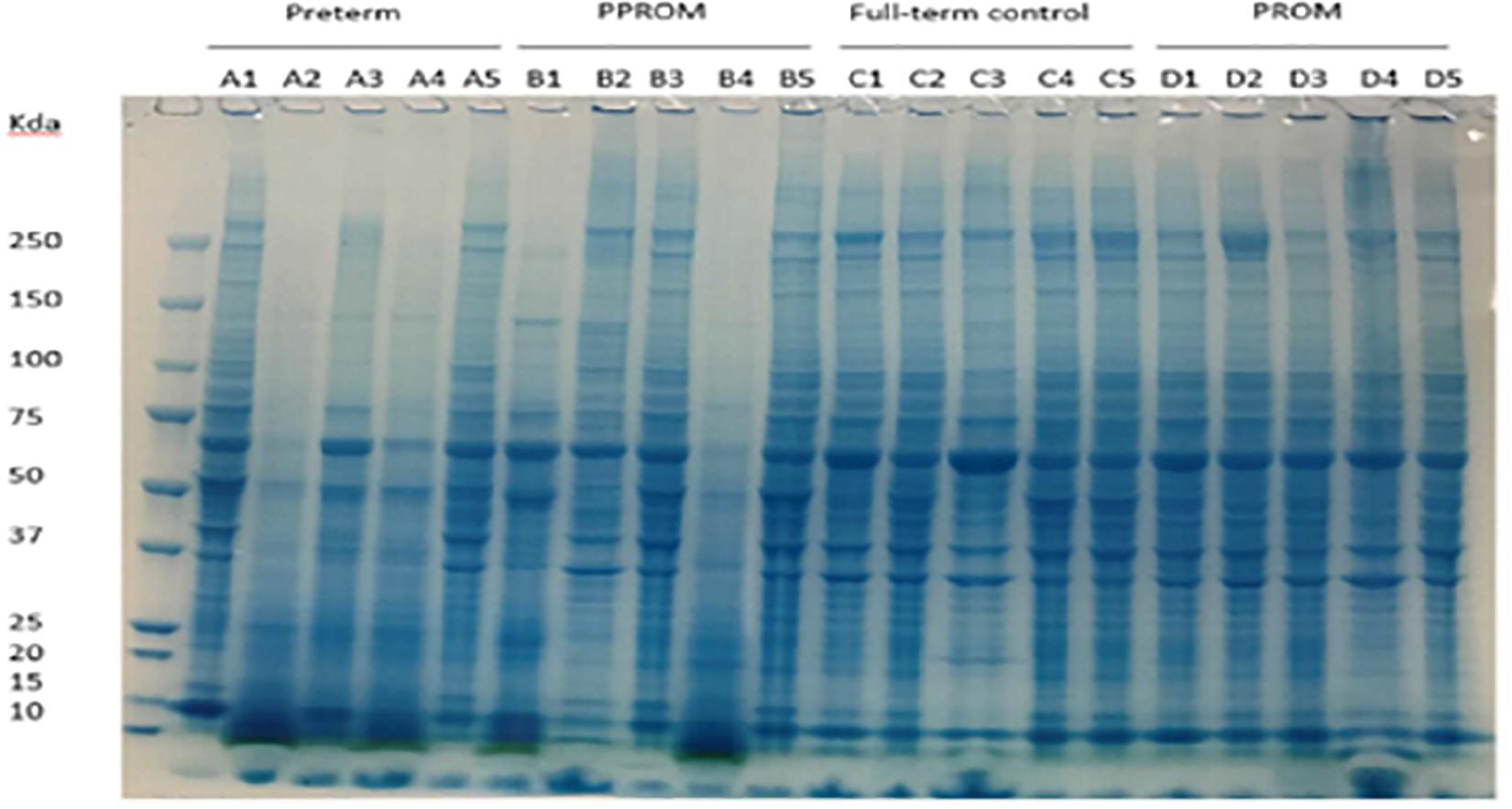
Figure 1. Protein expression pattern on SDS-PAGE gel from four groups of fetal membrane. Group A: sPTL (preterm), group B: PPROM, group C: FTB, and group D: PROM. Five individual samples in each group were analyzed on an 8% SDS-PAGE stained with a dye of Instantblue.
Peptide Measurement by Mass Spectrometry
LC-MS/MS analysis was carried out by nano-ultra performance liquid chromatography tandem mass spectrometry analysis using a 75-μm-inner diameter × 25 cm C18 nanoAcquity UPLC column (1.7-μm particle size, Waters, United Kingdom). Peptides were separated with a 120-min gradient of 3–40% solvent B (solvent A: 99.9% H2O, 0.1% formic acid; solvent B: 99.9% ACN, 0.1% formic acid) at 250 nl/min and injected into a Q Exactive High Field (HF) Hybrid Quadrupole-Orbitrap Mass Spectrometer (Thermo Fisher Scientific, United Kingdom) acquiring data in electron spray ionization (ESI) positive mode. The MS survey was set with a resolution of 60,000 FWHM, with a recording window between 300 and 2,000 m/z. A maximum of 20 MS/MS scans were triggered in data-dependent acquisition (DDA) mode.
Protein Identification and Quantification
MaxQuant software (v1.5.8.3, Max Planck Institute of Biochemistry, Germany) was used for peptide and protein identification and quantitation. Data generated from MS/MS spectra were searched against the Uniprot human database (version 2017); 20,205 entries were used for peptide homology identification. The false discovery rate (FDR) was set to 1% for protein and peptide identification. Proteins were quantified by at least one unique peptide, and match between run was selected to increase the quantifiable value cross samples (Figure 1). Label-free quantitation (LFQ) intensity data were used for further analysis and comparisons across the variant groups. Statistical analysis was assessed by using Persus software (version 1.5.5.3, Max Planck Institute of Biochemistry). Statistical comparisons between the groups were performed by using two-sided unpaired Student’s t-test. Firstly, threshold p-value was used to define statistical significance. Secondly, permutation-based FDR was used to assess truncate data.
Validation of Differentially Expressed Proteins With Western Blot
Ten milligrams of fetal membranes or placental villi were dissected and lysed in RIPA buffer containing protease inhibitors (Roche, United States). Western blot analysis was performed by loading 15 μg of proteins on 4–12% pre-cast Bis-Tris gels (Bio-Rad, United States) and transferred to PVDF membranes (Merck Millipore, United States). Membranes were incubated with mouse anti-human MPO monoclonal antibody (Santa Cruz, United States, 1:200 diluted in 2% milk), mouse anti-human elastase, neutrophil expressed (ELANE) monoclonal antibody (Santa Cruz, United States, 1:200 diluted in 2% milk), mouse anti-human GAPDH monoclonal antibody (Thermo Fisher Scientific, United Kingdom, 1:2,000 diluted in 2% milk), rabbit anti-human beta-actin antibody (Abcam, United Kingdom, 1:1,000 diluted in 2% milk). Dye-800-conjugated secondary antibodies were applied and visualized with an Odyssey Clx (Li-Cor, United States). Image studio (Li-Cor, United States) and Image J (Schneider et al., 2012) were used for Western blot quantitation, and one-way ANOVA was used for statistical significance test.
Results
Quality Control of Proteins Isolated From the Fetal Membrane Tissues
Protein lysates of fetal membranes were run on SDS-PAGE and visualized with InstantBlue (Figure 1). Protein partial degradation was detected in samples of A2, A4 and B1, B4. The protein patterns of the rest of samples did not show a clear difference.
Differentially Expressed Proteins Identified From Mass Spectra
After LC-MS/MS measurement, Maxquant analysis identified a total of 2,880 proteins from fetal membrane samples. The hierarchical clustering analysis indicated protein abundance alteration between individual samples. In addition to comparisons of the preterm group (A + B) with the full-term control group (C + D), individual comparisons were applied to identified specific protein(s) that are associated with a specific condition (Figure 2). Protein differential expression was defined by the criteria of p < 0.01, fold change (FC) ≥ 2, and permutation-based FDR 0.05, with which, 62 (38 up-regulated and 24 down-regulated) proteins were identified to be differentially expressed (Tables 2a,b). Among these 62 proteins, 20 were identified to be the top-listed FC (four were down-regulated and 16 were up-regulated). The FCs of 8 of 20 up-regulated proteins were >11, among which MMP9 showed 318.64 FC as the highest (Table 3). All differentially expressed proteins identified from fetal membranes in sPTB (sPTL and PPROM) were subjected to Kyoto Encyclopedia of Genes and Genomes (KEGG) analysis to identify the pathways that may be associated with the sPTB. The top-scoring pathways were infection and inflammation, protein degradation and proteolysis, extracellular matrix (ECM), cell adhesion, antioxidant, glycolysis, and fatty acid (FA) oxidation (Table 4).
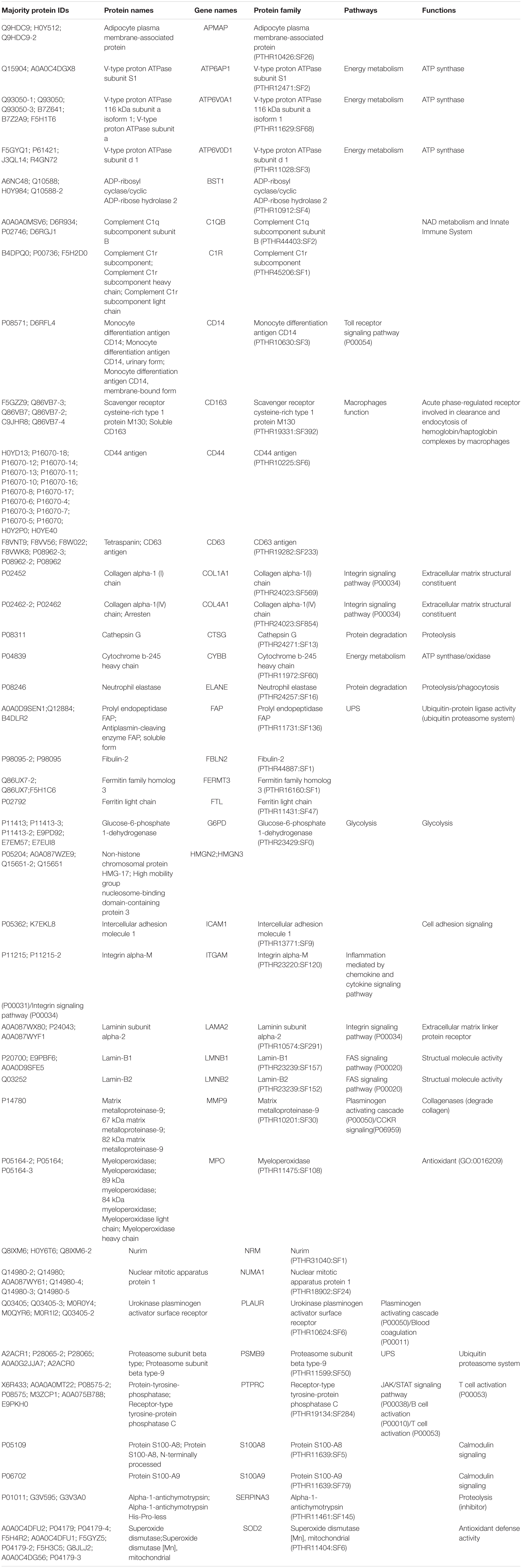
Table 2a. Proteins identified from fetal membranes associated with preterm birth (AB vs. CD): up-regulated.
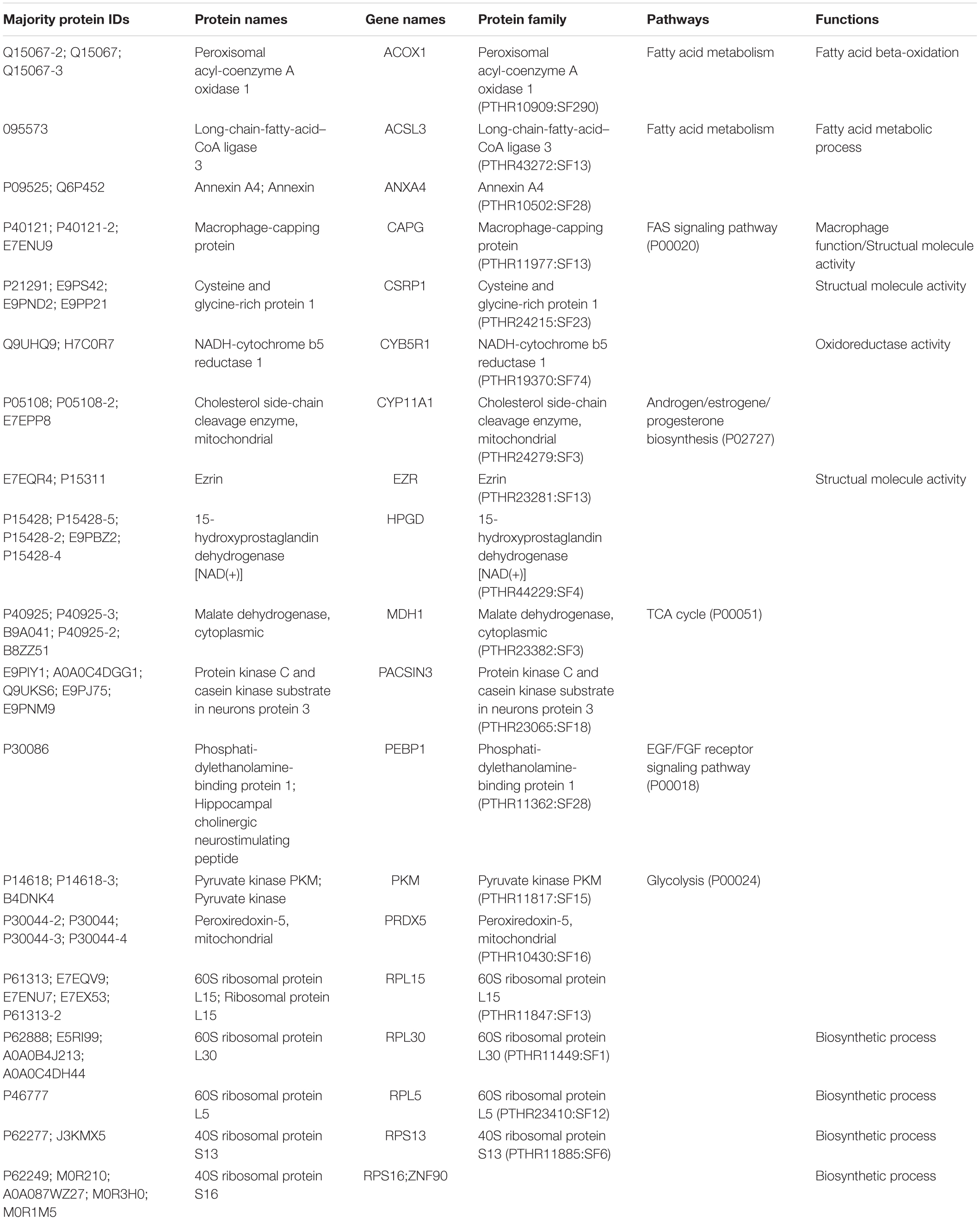
Table 2b. Proteins identified from fetal membranes associated with preterm birth (AB vs. CD): Down-regulated.
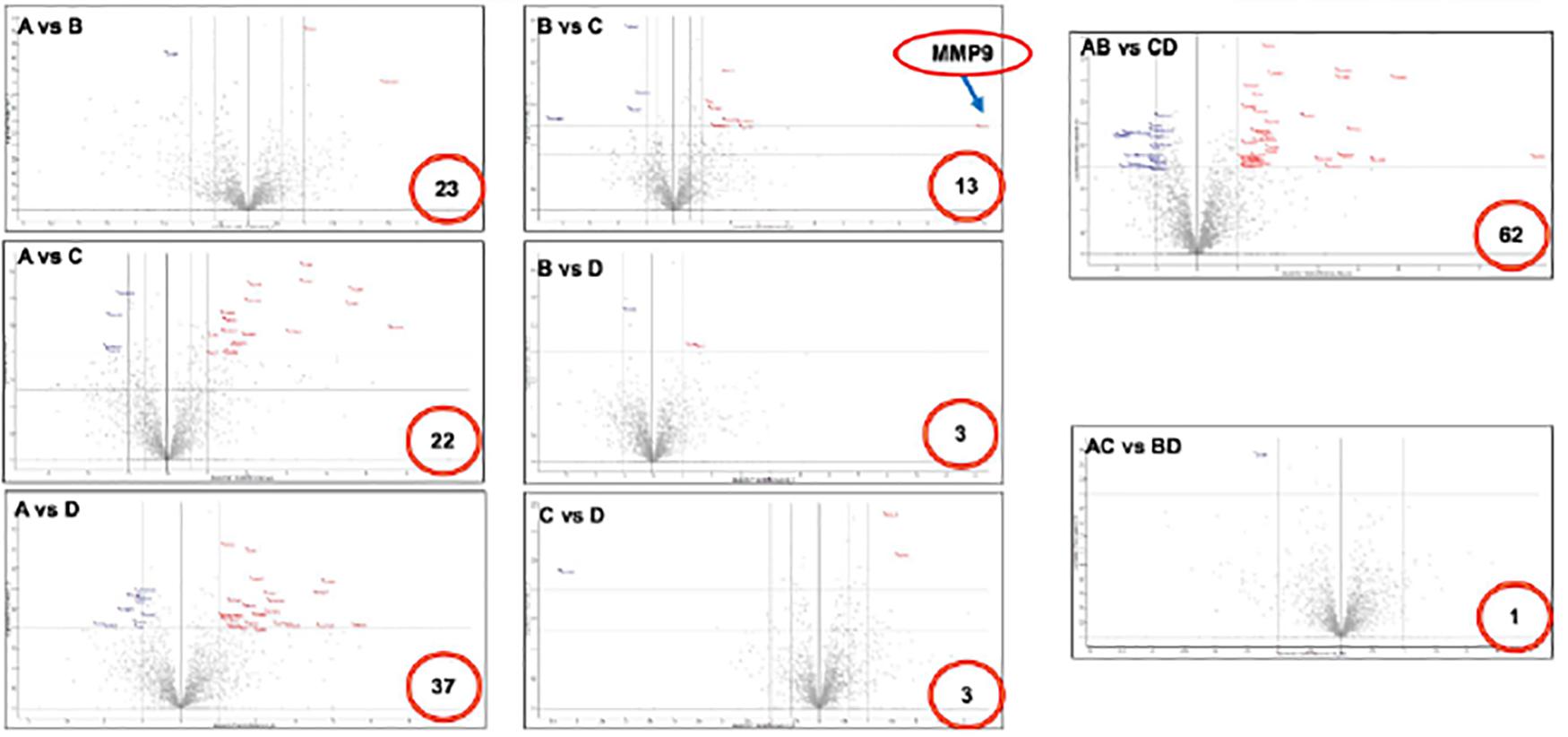
Figure 2. Volcano plot of differentially expressed proteins in fetal membranes. Various comparisons identified specific protein(s) that associated with the premature condition. Number in the red circle indicates the number of differential proteins with statistical significance. X-axis indicates fold changes and Y-axis indicates p value-log Student t-test. A: sPTL, B: PPROM, C: FTB, and D: PROM.
Validation of Differentially Expressed Proteins With Western Blots
Three proteins, MPO, ELANE, and GAPDH, which were shown by LC-MS/MS to be differentially expressed in fetal membrane, were randomly selected to validate the differential expression value of protein with Western blots. Westerns blot of MPO and ELANE showed the same protein expression pattern as proteome data with statistical significance. While GAPDH followed a similar increase of protein expression in fetal membrane compared to placental villi, it had a decreased expression in fetal membrane of preterm cases, including both sPTL and PPROM, compared to FTB and PROM (groups C and D) (Figure 3).
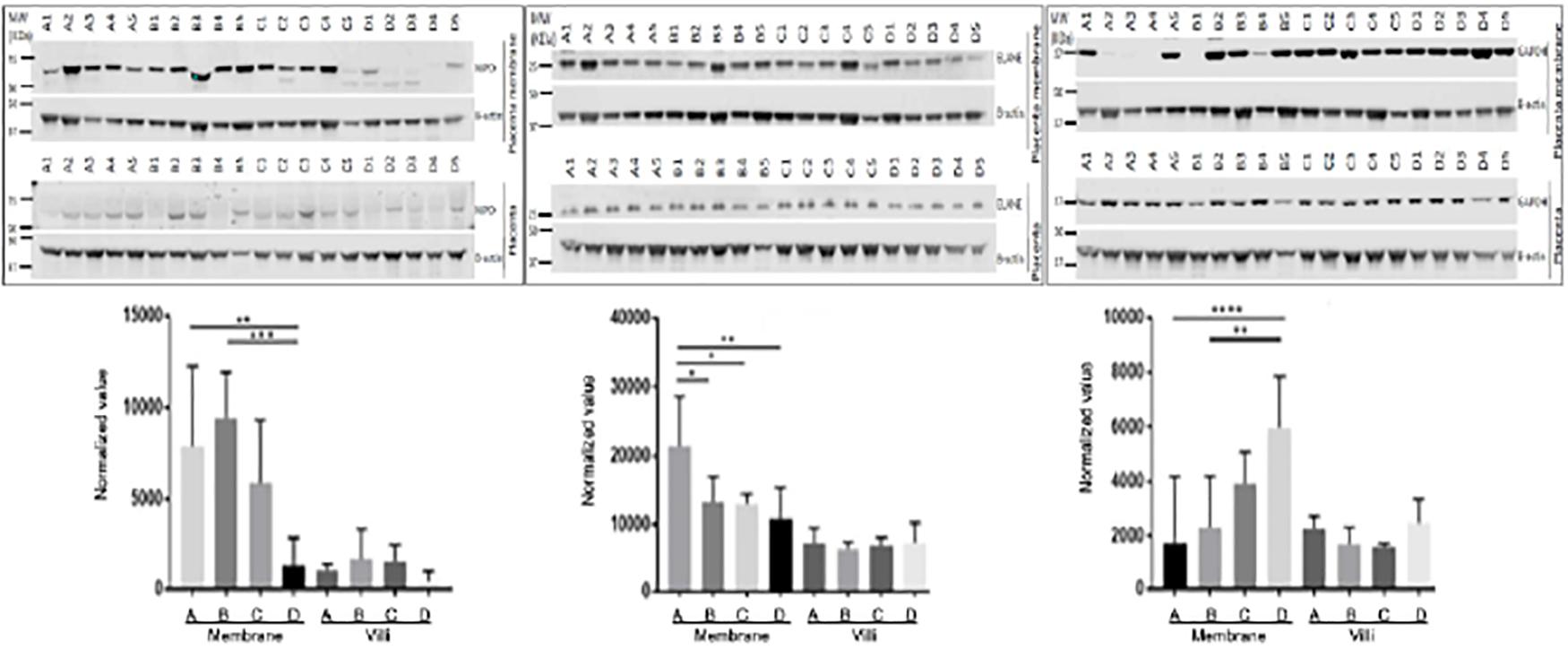
Figure 3. Validation of differentially expressed proteins with placenta villi and fetal membranes. Randomly selected proteins MPO (left panel), ELANE (middle panel), and GAPDH (right panel) that were identified to be differentially expressed in fetal membrane (FM) vs. placental villi (PV) by MS/MS were validated with Western blots. Generally, three proteins were up-regulated in fetal membranes when compared to placental villi. MPO and ELANE were significantly increased in preterm pregnancies of sPTL (A) & PPROM (B) compared to full-term pregnancies of FTB (C) and PROM (D) fetal membranes. However, GAPDH was decreased in A + B. *p < 0.05, **p < 0.01, ***p < 0.005, and ****p < 0.0001. Beta-Actin was used as an internal control for normalizing the protein expression value.
Discussion
Placental Function and Preterm Birth
PTB plays a significant adverse impact on the increased mortality and mobility of preterm-born neonates. The etiology of sPTB is multi-factorial; however, placental dysfunction has been identified as the leading cause of premature birth due to it pivotal role between the fetus and mother during pregnancy (Audette and Kingdom, 2018). As a result of the pathophysiological changes in placental dysfunction, including poor trophoblast uterine invasion and impaired transformation of the uterine spiral arteries to high capacity and low impedance vessels, which eventually leads to lower blood flow to the placenta (Ilekis et al., 2016; Cuffe et al., 2017), the placenta was unable to sustain fetal development requirements and thus preterm birth occurred. Searches for molecular markers to predict preterm birth have been conducted mainly in the maternal blood due to its richness of information and easy accessibility. It has been reported that PP-13 and PAPP-A are good predictors of preterm birth (Stout et al., 2013). Elevated maternal serum–soluble fms-like tryrosine 1 (sFlt1), inflammation marker [cysteine-rich protein (CRP)], and PIGF are associated with preterm birth (Bastek et al., 2011; Straughen et al., 2012). However, these markers have little clinical use for the prediction of preterm birth or for understanding the pathophysiology of placental dysfunction. Therefore, we conducted an unbiased proteomic analysis of the placenta in preterm birth compared to in full-term controls, followed by various comparisons to generate differentially expressed proteins specific to one single variation. Such comparisons include group B vs. group C, for example, in which the outcome of differentially expressed proteins was influenced only by premature rupture of fetal membrane in PPROM (B) vs. FTB without rupture of membrane (C). In addition, such comparisons may reduce non-specific noise, such as the comparison of group A vs. group D, in which the differentially expressed proteins would likely have resulted from the premature labor that was related to muscle contraction of uterine but not to rupture of membrane.
Proteins Involved in Infection and Inflammation
Based on molecular function, the top-scoring proteins differentially expressed in fetal membranes of sPTB have been clustered into five groups via their involvement in pathophysiological pathways (Table 3). The main pathophysiological changes in the fetal membrane from preterm birth were inflammation. Numerous studies indicated that placental intrauterine infection was strongly associated with sPTL or PPROM (Salafia et al., 1991; Helmig et al., 2002; Morgan, 2016; Pugni et al., 2016; Chisholm et al., 2018).
A study in humans demonstrated that HPGD expression in preterm-labor placenta tissues was decreased in comparison with a full-term labor group (He et al., 2015). HPGD is a member of the short-chain non-metalloenzyme alcohol dehydrogenases protein family and is responsible for the degradation of prostaglandins, hormones that modulate the inflammatory response (Aoki and Narumiya, 2012; Seo and Oh, 2017). Another study in an animal model detected high expression levels of HPGD at the beginning and at normal term of pregnancy, indicating that HPGD may play a role during the establishment and termination of gestation (von Hof et al., 2017). Our data are consistent with previously findings. The lower level of HPGD found in fetal membranes suggested a higher level of inflammatory states in the preterm group (sPTL + PPROM), possibly through negative regulation of inflammatory molecule prostaglandins.
PTPRC is a receptor-type protein tyrosine phosphatase that regulates cell growth, differentiation, and mitosis. PTPRC is essential to regulate T- and B-cell antigen receptor signaling by direct interaction with antigen receptor complexes or by activating Src family kinases (Pike and Tremblay, 2013). PTPRC also regulates cytokine receptor signaling by suppressing JAK kinase (Porcu et al., 2012). It has been reported that PTPRC is dysregulated in human miscarriage (Lorenzi et al., 2012). Increased levels of PTPRC in the fetal membranes of sPTB indicated activation of T- and B-cell antigen receptor signaling and suggested that sPTB may share a common pathophysiological mechanism with miscarriage.
Macrophage activation (CAPG, CD14, and CD163): CAPG is a member of the gelsolin/villin family of actin-regulatory proteins. CAPG reversibly blocks the barbed ends of F-actin filaments in a Ca2+-dependent manner, thus capping the barbed ends of actin filaments and controlling actin-based motility of macrophages (Young et al., 1994). CD14 is a surface protein preferentially expressed on macrophages or monocytes. It mediates the innate immune response to bacterial lipopolysaccharide (Mogensen, 2009). A study in an animal model indicated that increased expression of TLR2 and CD14 was correlated with urea plasma parvum–induced fetal inflammatory response syndrome-like pathology (Allam et al., 2014). CD163 is a member of the scavenger receptor cysteine-rich superfamily and functions as an innate immune sensor for bacteria and an inducer of local inflammation (Fabriek et al., 2009). High levels of CD163 are associated with an increased risk of preterm delivery in pregnant women (Vogel et al., 2005). The detection of CAPG, CD14, and CD163 suggested the activation of macrophage or monocyte, even though we do not know what pathogen causes are.
Extracellular Matrix, Proteolysis, and Cell Adhesion
COL4A1, LAMA2, FBLN2, and APMAP are the ECM proteins. COL4A1 is an integral component of all basement membranes; it not only provides structural support, regulating adhesion, migration, and survival of cells, but also plays a key role in early placentation by modeling trophoblast cell invasion to remodel maternal spiral arteries and ensure sufficient blood flow to the developing fetus (Oefner et al., 2015). LAMA2 is a major component of the basement membrane and mediates the attachment and migration of cells into tissues during embryonic development by interacting with other ECMs. FBLN2 is an ECM protein belonging to the fibulin family. APMAP is a novel regulator of ECM components that may serve as a potential target to mitigate obesity-associated insulin resistance (Pessentheiner et al., 2017). The exact functions of LAMA2, FBLN2, and APMAP in the placenta are not clear; the expression level changes may indicate ECM degradation in PPROM and PROM. CSRP1 is a membrane of the CRP family, which may regulate cellular development and differentiation.
MMP9, ELANE, and chymotrypsin C (CTSG) are the proteins involved in proteolysis. MMP9 has been reported to break down the ECM, such as type IV and V collagens. MMP9 is mainly expressed in amnion epithelia, chorion leave trophoblast, decidua parietalis, and placental syncytiotrophoblasts. The expression level of MMP9 was increased in fetal membranes from preterm and term labor as compared to non-labors (Xu et al., 2002). Moreover, fetuses with PPROM have higher concentrations of MMP-9 than those with preterm labor with intact membrane, indicating the pathogenic role of MMP-9 during a rupture of fetal membrane in sPTB (Romero et al., 2002). The level of MMP-9 has been used as a risk factor for preterm births (Di Ferdinando et al., 2010; Sorokin, 2010). In agreement with published data, an increase in MMP-9 expression may contribute to degradation of the ECM in the fetal membrane and in placentas, thus initiating sPTB. ELANE, a multifunctional serine protease stored in azurophilic granules of mature neutrophils, is able to degrade the ECM of connective tissue during an inflammatory process. It has been implicated that PPROM, microbial invasion of the amniotic cavity, and parturition at term and preterm are associated with a significant increase in the concentration of ELANE in the amniotic fluid. Another study suggested that ELANE levels in amniotic fluid may serve as a useful marker for predicting the duration of continued pregnancy after cervical cerclage (Hatakeyama et al., 2016). CTSG is a member of the peptidase S1 protein family in azurophilic granules of neutrophilic polymorphonuclear leukocytes. CTSG has a cleavage specificity similar to chymotrypsin C and may involve connective tissue remodeling at the site of inflammation. It has been shown that intra-amniotic inflammation (IAI) is associated with increased CTSG concentration in the amniotic fluid in PPROM (Musilova et al., 2017). It has been reported that the ubiquitin–proteasome–collagen (CUP) pathway is involved in collagen degradation in PPROM (Zhao et al., 2017). In addition, the CUP pathway was epigenetically regulated by IncRNA in PROM and PPROM (Luo et al., 2015). Even though we did not identify proteins belonging to the CUP pathway, we did identify the most significant proteolytic enzymes associated with PPROM and PTB, mainly MMP-9, serine protease (ELANE), and CTSG.
Our proteome data detected over-expression of cell adhesion molecules ICAM and ITGAM in sPTB. ICAM-1 is a cell surface glycoprotein expressed on endothelial cells and cells of the immune system (Egal et al., 2014). It has been reported that ICAM-1 was overexpressed in villous trophoblasts during placental infection (Juliano et al., 2006). Another study indicated that expression of ICAM-1 by the human choriodecidua was elevated with preterm birth, together with increased leukocyte infiltration (Marvin et al., 1999). ITGAM plays an important role in the adherence of neutrophils and monocytes to stimulated endothelium. The level of ITGAM was significantly elevated in the amniotic fluid of women with preterm labor with IAI compared without IAI (Romero et al., 2010). Again, increased levels of ICAM-1 and ITGAM suggest activation of white blood cells and immune response in preterm birth placenta membrane.
Oxidative Stress Proteins MPO, LMNB1, and LMNB2
MPO is a heme protein synthesized and released from activated monocytes and neutrophils. Traditionally, it was described as a microbicidal enzyme. New evidence indicates that MPO generates hypochlorite-modified proteins, activates metalloproteinase (Prokopenko et al., 2002), and oxidatively consumes endothelium-derived nitric oxide in humans during normal pregnancy as well as during pathophysiologic processes (pre-eclampsia) (Hammer et al., 2001; Gandley et al., 2008). Higher cord blood levels of MPO are associated with preterm delivery (Yang et al., 2004). LMNB1 and LMNB2 are B-type nuclear lamin located in the inner nuclear membrane and play an important role in nuclear stability, chromatin structure, and gene expression. Overexpression of LMNB1 was found through a mitochondrial reactive oxygen species (ROS) in vitro model to increase the proliferation rate and to delay the onset of senescence (Shimi et al., 2011). Oxidative stress was higher and antioxidant enzymes were lower in PPROM compared with sPTB (Dutta et al., 2016). Our data suggest a higher level of oxidative stress, which may induce premature cellular senescence, inflammation, and proteolysis, eventually leading to membrane rupture and PPROM.
Glycolysis, Fatty Acid Synthesis
Differentially expressed proteins involved in glycolysis and FA synthesis were down-regulated in the fetal membranes of sPTB (Table 3). PKM, a catalytic enzyme involved in the last step of glycolysis, is responsible for dephosphorylating phosphoenolpyruvate to pyruvate and producing ATP under hypoxic conditions. PKM is also involved in angiogenesis in embryo development. It has been reported that the expression of PKM is higher in pre-eclampsia at delivery than in normal pregnancy (Bahr et al., 2014). ADPGK is a rate-limiting enzyme within the first step of glycolysis; it catalyzes the phosphorylation of D-glucose to D-glucose 6-phosphate by using ADP as the phosphate donor. GAPDH is an enzyme responsible for glyceraldehyde dehydration in the process of glycolysis. The placenta is a main source of high lactate levels during gestation. In later gestation, the concentration of lactate can reach 10 mmol/l, as compared to 1–2 mmol/l in the newborn and 0.5 mmol/l in maternal plasma. Fatty acids are essential substances for the construction of cell membrane and development of the nervous system. The disruption of FAs’ metabolism in the maternal-placental interface would result in malnutrition of the fetus and in preterm birth (Bobinski and Mikulska, 2015). Blood levels of FAs are 20 times lower in the fetal circulation than in newborns’ circulation (Makinde et al., 1998). This indicates that glucose/lactate provides the main energy source in fetal development. Typically, lactate is described as a waste product catalyzed by LDH under anaerobic metabolism. A metabolism study in various cell types indicated a model whereby lactate generated in the cytosol compartment can be oxidized into pyruvate in mitochondria by LDH; pyruvate is subsequently transported into the inner membrane of the matrix and is then oxidized to acetyl CoA by pyruvate dehydrogenate (Kane, 2014). Acetyl CoA can then be fed into the TCA cycle and maintains mitochondrial function. Our data showed an increase in the level of ADPGK in the first step of glycolysis and a reduced level of PKM in the last step of glycolysis, suggesting an imbalance of glycolysis and the generation of less pyruvate through the conventional glycolysis pathway. To maintain metabolic functionality, a lactate oxidation process in mitochondria to produce pyruvate to sustain the TCA cycle is preferred. Reduction of the proteins involved in glycolysis and fatty acid synthesis may suggest that energy synthesis might be reduced in the fetal membrane tissues in sPTB. To confirm this, further investigation is needed to understand better the role of lactate’s and FAs’ metabolism in sPTB.
Tissue Specificity
Differential expression patterns of GAPDH were documented between the fetal membranes and placental villi. As shown in the right panel of Figure 3, GAPDH was found to be down-regulated in premature birth groups A and B, as compared to full-term control groups C and D with (PROM) or without (FTB) rupture of membrane in samples of fetal membrane. This finding is in agreement with the results generated from a discovery study with MS/MS. However, in the tissue of placental villi, Western blot for validation showed up-regulation, compared to FTB, which resulted in no significant change in placenta specimens between A + B vs. C + D. GAPDH is an enzyme involved in anaerobic energy metabolism through glycolysis. We speculated that the glycolysis pathway may be affected in fetal membrane and weakened the structure of fetal membrane but not in placental villi. To confirm this, further investigation should be performed to provide biochemical evidence.
Conclusion
By applying a proteomic approach, along with validation of proteomic results with Western blots, to study sPTL and PPROM with the capacity of distinguishing between the sPTL and PPROM groups, and of distinguishing the premature groups sPTL and PPROM from the mature groups FTB and PROM, we demonstrated a unique signature for each of the conditions, which is the strength this study. In addition, our proteomic data provide systemic insights into pathophysiological changes in the fetal membranes on the molecular level. Our data support the theory of inflammation/infection in sPTL and PPROM, even though we have not identified the source of infection. Inflammation increased the oxidative stress–triggered pyrolytic process that changes ECM structure, eventually rupturing the placental membrane and resulting in preterm birth. Metabolic function was also altered, in particular, imbalanced glycolysis and unconventional lactate oxidation, which may be associated with preterm birth. We are also aware of the limitation that applying the proteomic approach to identifying the differentially expressed protein(s) that are associated with clinical features and to identify biomarker(s) for the disease condition could be influenced by post-translational modification. Therefore, a larger sample size will need to be used for validation. In fact, studies on the inflammation-triggered proteolysis of ECM has been undertaken to verify a key pathogenic pathway for sPTB.
Data Availability Statement
The datasets presented in this study can be found in online repositories. The names of the repository/repositories and accession number(s) can be found in the article/supplementary material.
Ethics Statement
The study was approved by the Hospital Ethics Committee of Sanya Maternity and Child Care Hospital. Written informed consent was obtained from pregnant women to permit the use of placentas in research studies.
Author Contributions
JP, XT, and HH contributed to the sample collection, processing and preparation, data acquisition, and laboratory work. NZ contributed to, and was responsible for, conceptual research design, initiating and coordinating the studies and experiments, data analysis, and drafting, finalizing, and submitting the manuscript. All authors contributed to the article and approved the submitted version.
Funding
This project was supported in part by the Sanya Maternity and Child Care Hospital, the Major Science and Technology Program of Hainan Province (ZDKJ2017007), Hainan Bureau of Science and Technology (KJHZ2015-18), the New York State Office for People With Developmental Disabilities, and NIH/NICHD 1R01HD094381.
Conflict of Interest
The authors declare that the research was conducted in the absence of any commercial or financial relationships that could be construed as a potential conflict of interest.
References
Allam, A. B., von Chamier, M., Brown, M. B., and Reyes, L. (2014). Immune profiling of BALB/C and C57BL/6 mice reveals a correlation between Ureaplasma parvum-Induced fetal inflammatory response syndrome-like pathology and increased placental expression of TLR2 and CD14. Am. J. Reprod. Immunol. 71, 241–251. doi: 10.1111/aji.12192
Aoki, T., and Narumiya, S. (2012). Prostaglandins and chronic inflammation. Trends Pharmacol Sci. 33, 304–311.
Audette, M. C., and Kingdom, J. C. (2018). Screening for fetal growth restriction and placental insufficiency. Semin. Fetal. Neonatal. Med. 23, 119–125. doi: 10.1016/j.siny.2017.11.004
Bahr, B. L., Price, M. D., Merrill, D., Mejia, C., Call, L., Bearss, D., et al. (2014). Different expression of placental pyruvate kinase in normal, preeclamptic and intrauterine growth restriction pregnancies. Placenta 35, 883–890. doi: 10.1016/j.placenta.2014.09.005
Bastek, J. A., Brown, A. G., Anton, L., Srinivas, S. K., D’addio, A., and Elovitz, M. A. (2011). Biomarkers of inflammation and placental dysfunction are associated with subsequent preterm birth. J. Matern. Fetal. Neonatal. Med. 24, 600–605. doi: 10.3109/14767058.2010.511340
Bhutani, V. K., and Wong, R. J. (2013). Bilirubin neurotoxicity in preterm infants: risk and prevention. J. Clin. Neonatol. 2, 61–69.
Bobinski, R., and Mikulska, M. (2015). The ins and outs of maternal-fetal fatty acid metabolism. Acta Biochim. Pol. 62, 499–507. doi: 10.18388/abp.2015_1067
Cheng, P. J., and Duan, T. (2016). China’s new two-child policy: maternity care in the new multiparous era. BJOG 123(Suppl.), 7–9. doi: 10.1111/1471-0528.14290
Chisholm, K. M., Norton, M. E., Penn, A. A., and Heerema-McKenney, A. (2018). Classification of preterm birth with placental correlates. Pediatr. Dev. Pathol. 21, 548–560. doi: 10.1177/1093526618775958
Costa, M. A. (2016). The endocrine function of human placenta: an overview. Reprod. Biomed. Online 32, 14–43. doi: 10.1016/j.rbmo.2015.10.005
Cuffe, J. S. M., Holland, O., Salomon, C., Rice, G. E., and Perkins, A. V. (2017). Review: placental derived biomarkers of pregnancy disorders. Placenta 54, 104–110. doi: 10.1016/j.placenta.2017.01.119
Di Ferdinando, A., Patacchiola, F., Perilli, M. G., Amicosante, G., and Carta, G. (2010). Expression of matrix metalloproteinase-9 (MMP-9) in human midpregnancy amniotic fluid and risk of preterm labor. Clin. Exp. Obstet. Gynecol. 37, 193–196.
Dutta, E. H., Behnia, F., Boldogh, I., Saade, G. R., Taylor, B. D., Kacerovský, K., et al. (2016). Oxidative stress damage-associated molecular signaling pathways differentiate spontaneous preterm birth and preterm premature rupture of the membranes. Mol. Hum. Reprod. 22, 143–157. doi: 10.1093/molehr/gav074
Egal, E. S., Mariano, F. V., Blotta, M. H., Piña, A. R., Montalli, V. A., Almeida, O. P., et al. (2014). ICAM-1 expression on immune cells in chronic villitis. Placenta 35, 1021–1026. doi: 10.1016/j.placenta.2014.10.004
Fabriek, B. O., van Bruggen, R., Deng, D. M., Ligtenberg, A. J., Nazmi, K., Schornagel, K., et al. (2009). The macrophage scavenger receptor CD163 functions as an innate immune sensor for bacteria. Blood 113, 887–892. doi: 10.1182/blood-2008-07-167064
Fraser, J., Walls, M., and McGuire, W. (2004). Respiratory complications of preterm birth. BMJ 329, 962–965. doi: 10.1136/bmj.329.7472.962
Fuchs, F., Monet, B., Ducruet, T., Chaillet, N., and Audibert, F. (2018). Effect of maternal age on the risk of preterm birth: a large cohort study. PLoS One 13:e0191002. doi: 10.1371/journal.pone.0191002
Gandley, R. E., Rohland, J., Zhou, Y., Shibata, E., Harger, G. F., and Rajakumar, A. (2008). Increased myeloperoxidase in the placenta and circulation of women with preeclampsia. Hypertension 52, 387–393. doi: 10.1161/hypertensionaha.107.107532
Gezer, C., Ekin, A., Solmaz, U., Yildirim, A. G. S., Dogan, A., and Ozeren, M. (2018). Identification of preterm birth in women with threatened preterm labour between 34 and 37 weeks of gestation. J. Obstet. Gynaecol. 38, 652–657. doi: 10.1080/01443615.2017.1399990
Gude, N. M., Roberts, C. T., Kalionis, B., and King, R. G. (2004). Growth and function of the normal human placenta. Thromb. Res. 114, 397–407. doi: 10.1016/j.thromres.2004.06.038
Guleria, I., and Sayegh, M. H. (2007). Maternal acceptance of the fetus: true human tolerance. J. Immunol. 178, 3345–3351. doi: 10.4049/jimmunol.178.6.3345
Hammer, A., Desoye, G., Dohr, G., Sattler, W., and Malle, E. (2001). Myeloperoxidase-dependent generation of hypochlorite-modified proteins in human placental tissues during normal pregnancy. Lab. Invest. 81, 543–554. doi: 10.1038/labinvest.3780263
Hatakeyama, Y., Miura, H., Sato, A., Onodera, Y., Sato, N., Shimizu, D., et al. (2016). Neutrophil elastase in amniotic fluid as a predictor of preterm birth after emergent cervical cerclage. Acta Obstet. Gynecol. Scand. 95, 1136–1142. doi: 10.1111/aogs.12928
He, P., Li, Y., Ding, X., Sun, Q., Huang, Y., Gu, H., et al. (2015). Expression of 15-hydroxyprostaglandin dehydrogenase in human chorion is associated with peroxisome proliferator-activated receptor isoform expression in term labor. Am. J. Pathol. 185, 1981–1990. doi: 10.1016/j.ajpath.2015.03.021
Heazell, A. E., Whitworth, M., Duley, L., and Thornton, J. G. (2015). Use of biochemical tests of placental function for improving pregnancy outcome. Cochrane Database. Syst. Rev. 11:CD011202. doi: 10.1002/14651858.CD011202.pub2
Helmig, B. R., Romero, R., Espinoza, J., Chaiworapongsa, T., Bujold, E., Gomez, R., et al. (2002). Neutrophil elastase and secretory leukocyte protease inhibitor in prelabor rupture of membranes, parturition and intra-amniotic infection. J. Matern. Fetal Neonatal. Med. 12, 237–246.
Ilekis, J. V., Tsilou, E., Fisher, S., Abrahams, V. M., Soares, M. J., Cross, J. C., et al. (2016). Placental origins of adverse pregnancy outcomes: potential molecular targets: an executive workshop summary of the eunice kennedy shriver national institute of child health and human development. Am. J. Obstet. Gynecol. 215(Suppl.), S1–S46.
Juliano, P. B., Blotta, M. H., and Altemani, A. M. (2006). ICAM-1 is overexpressed by villous trophoblasts in placentitis. Placenta 27, 750–757. doi: 10.1016/j.placenta.2005.07.008
Kane, D. A. (2014). Lactate oxidation at the mitochondria: a lactate-malate-aspartate shuttle at work. Front. Neurosci. 8:366. doi: 10.3389/fnins.2014.00366
Lorenzi, T., Turi, A., Lorenzi, M., Paolinelli, F., Mancioli, F., La Sala, L., et al. (2012). Placental expression of CD100, CD72 and CD45 is dysregulated in human miscarriage. PLoS One 7:e35232. doi: 10.1371/journal.pone.0035232
Luo, X., Luo, X., Pan, J., Wang, L., Wang, P., Zhang, M., et al. (2015). -inflammation in preterm births and preterm premature rupture of membranes. BMC Pregnancy Childbirth 15:35. doi: 10.1186/s12884-015-0460-0
Makinde, A. O., Kantor, P. F., and Lopaschuk, G. D. (1998). Maturation of fatty acid and carbohydrate metabolism in the newborn heart. Mol. Cell. Biochem. 188, 49–56. doi: 10.1007/978-1-4615-5763-0_6
Marvin, K. W., Keelan, J. A., Sato, T. A., Coleman, M. A., McCowan, L. M., and Mitchell, M. D. (1999). Expression of intercellular adhesion molecule-1 (ICAM-1) in choriodecidua with labour and delivery at term and preterm. Reprod. Fertil. Dev. 11, 255–262.
Mogensen, T. H. (2009). Pathogen recognition and inflammatory signaling in innate immune defenses. Clin. Microbiol. Rev. 22, 240–273. doi: 10.1128/cmr.00046-08
Morgan, T. K. (2016). Role of the placenta in preterm birth: a review. Am. J. Perinatol. 33, 258–266. doi: 10.1055/s-0035-1570379
Musilova, I., Andrys, C., Drahosova, M., Soucek, O., Pliskova, L., Stepan, M., et al. (2017). Amniotic fluid cathepsin-G in pregnancies complicated by the preterm prelabor rupture of membranes. J. Matern. Fetal. Neonatal. Med. 30, 2097–2104. doi: 10.1080/14767058.2016.1237499
Oefner, C. M., Sharkey, A., Gardner, L., Critchley, H., Oyen, M., and Moffett, A. (2015). Collagen type IV at the fetal-maternal interface. Placenta 36, 59–68. doi: 10.1016/j.placenta.2014.10.012
Paton, M. C. B., McDonald, C. A., Allison, B. J., Fahey, M. C., Jenkin, G., and Miller, S. L. (2017). Perinatal brain injury as a consequence of preterm birth and intrauterine inflammation: designing targeted stem cell therapies. Front. Neurosci. 11:200. doi: 10.3389/fnins.2017.00200
Pessentheiner, A. R., Huber, K., Pelzmann, H. J., Prokesch, A., Radner, F., Wolinski, H., et al. (2017). APMAP interacts with lysyl oxidase-like proteins, and disruption of Apmap leads to beneficial visceral adipose tissue expansion. FASEB J. 31, 4088–4103. doi: 10.1096/fj.201601337r
Pike, K. A., and Tremblay, M. L. (2013). Tremblay, Regulating naive and memory CD8 T cell homeostasis–a role for protein tyrosine phosphatases. FEBS J. 280, 432–444. doi: 10.1111/j.1742-4658.2012.08587.x
Porcu, M., Kleppe, M., Gianfelici, V., Geerdens, E., De Keersmaecker, K., Tartaglia, M., et al. (2012). Mutation of the receptor tyrosine phosphatase PTPRC (CD45) in T-cell acute lymphoblastic leukemia. Blood 119, 4476–4479. doi: 10.1182/blood-2011-09-379958
Prokopenko, V. M., Aleshina, G. M., Frolova, E. V., Anan’eva, V. V., Kokriakov, V. N., and Arutiunian, A. V. (2002). [Myeloperoxidase in the human placenta at preterm labor]. Vopr Med. Khim 48, 378–380.
Pugni, L., Pietrasanta, C., Acaia, B., Merlo, D., Ronchi, A., Ossola, M. W., et al. (2016). Chorioamnionitis and neonatal outcome in preterm infants: a clinical overview. J. Matern. Fetal. Neonatal. Med. 29, 1525–1529. doi: 10.3109/14767058.2015.1053862
Quinn, J. A., Munoz, F. M., Gonik, B., Frau, L., Cutland, C., Mallett-Moore, T., et al. (2016). Preterm birth: case definition & guidelines for data collection, analysis, and presentation of immunisation safety data. Vaccine 34, 6047–6056.
Romero, R., Chaiworapongsa, T., Espinoza, J., Gomez, R., Yoon, B. H., Edwin, S., et al. (2002). Fetal plasma MMP-9 concentrations are elevated in preterm premature rupture of the membranes. Am. J. Obstet. Gynecol. 187, 1125–1130. doi: 10.1067/mob.2002.127312
Romero, R., Kusanovic, J. P., Gotsch, F., Erez, O., Vaisbuch, E., Mazaki-Tovi, S., et al. (2010). Isobaric labeling and tandem mass spectrometry: a novel approach for profiling and quantifying proteins differentially expressed in amniotic fluid in preterm labor with and without intra-amniotic infection/inflammation. J Matern Fetal Neonatal Med. 23, 261–280. doi: 10.3109/14767050903067386
Salafia, C. M., Vogel, C. A., Vintzileos, A. M., Bantham, K. F., Pezzullo, J., and Silberman, L. (1991). Placental pathologic findings in preterm birth. Am. J. Obstet. Gynecol. 165(Pt 1), 934–938. doi: 10.1016/0002-9378(91)90443-u
Schneider, C. A., Rasband, W. S., and Eliceiri, K. W. (2012). NIH Image to Image J: 25 years of image analysis. Nat. Methods 9, 671–675. doi: 10.1038/nmeth.2089
Seo, M. J., and Oh, D. K. (2017). Prostaglandin synthases: molecular characterization and involvement in prostaglandin biosynthesis. Prog Lipid Res. 66, 50–68. doi: 10.1016/j.plipres.2017.04.003
Shimi, T., Butin-Israeli, V., Adam, S. A., Hamanaka, R. B., Goldman, A. E., Lucas, C. A., et al. (2011). The role of nuclear lamin B1 in cell proliferation and senescence. Genes Dev. 25, 2579–2593. doi: 10.1101/gad.179515.111
Sorokin, Y. (2010). Maternal serum interleukin-6, C-reactive protein, and matrix metalloproteinase-9 concentrations as risk factors for preterm birth <32 weeks and adverse neonatal outcomes. Am. J. Perinatol. 27, 631–640. doi: 10.1055/s-0030-1249366
Stout, M. J., Romero, R., Mele, L., Wapner, R. J., Iams, J. D., Dudley, D. J., et al. (2013). First trimester serum analytes, maternal characteristics and ultrasound markers to predict pregnancies at risk for preterm birth. Placenta 34, 14–19. doi: 10.1016/j.placenta.2012.10.013
Straughen, J. K., Kumar, P., and Misra, V. K. (2012). The effect of maternal soluble FMS-like tyrosine kinase 1 during pregnancy on risk of preterm delivery. J. Matern. Fetal. Neonatal. Med. 25, 1879–1883. doi: 10.3109/14767058.2012.666589
Vogel, I., Grove, J., Thorsen, P., Moestrup, S. K., Uldbjerg, N., and Møller, H. J. (2005). Preterm delivery predicted by soluble CD163 and CRP in women with symptoms of preterm delivery. BJOG 112, 737–742. doi: 10.1111/j.1471-0528.2005.00557.x
von Hof, J., Sprekeler, N., Schuler, G., Boos, A., and Kowalewski, M. P. (2017). Uterine and placental expression of HPGD in cows during pregnancy and release of fetal membranes. Prostaglandins Other Lipid Mediat. 12, 17–26. doi: 10.1016/j.prostaglandins.2016.12.003
Xu, P., Alfaidy, N., and Challis, J. R. (2002). Expression of matrix metalloproteinase (MMP)-2 and MMP-9 in human placenta and fetal membranes in relation to preterm and term labor. J. Clin. Endocrinol. Metab. 87, 1353–1361. doi: 10.1210/jcem.87.3.8320
Yang, K. D., Wang, C. L., Huang, L. T., Chang, H., Huang, H. C., Hsu, T. Y., et al. (2004). Implication of cord blood myeloperoxidase but not of soluble p-selectin levels in preterm deliveries. J. Perinat. Med. 32, 49–52.
Young, C. L., Feierstein, A., and Southwick, F. S. (1994). Calcium regulation of actin filament capping and monomer binding by macrophage capping protein. J. Biol. Chem. 269, 13997–14002.
Zeldovich, V. B., Clausen, C. H., Bradford, E., Fletcher, D. A., Maltepe, E., Robbins, J. R., et al. (2013). Placental syncytium forms a biophysical barrier against pathogen invasion. PLoS Pathog. 9:e1003821. doi: 10.1371/journal.ppat.1003821
Keywords: fetal membrane, preterm birth, prelabor premature rupture of membrane, inflammation, extracellular matrix
Citation: Pan J, Tian X, Huang H and Zhong N (2020) Proteomic Study of Fetal Membrane: Inflammation-Triggered Proteolysis of Extracellular Matrix May Present a Pathogenic Pathway for Spontaneous Preterm Birth. Front. Physiol. 11:800. doi: 10.3389/fphys.2020.00800
Received: 02 April 2020; Accepted: 16 June 2020;
Published: 21 July 2020.
Edited by:
Ramkumar Menon, University of Texas Medical Branch at Galveston, United StatesReviewed by:
Martha Lappas, The University of Melbourne, AustraliaPallavi Kshetrapal, Translational Health Science and Technology Institute (THSTI), India
Copyright © 2020 Pan, Tian, Huang and Zhong. This is an open-access article distributed under the terms of the Creative Commons Attribution License (CC BY). The use, distribution or reproduction in other forums is permitted, provided the original author(s) and the copyright owner(s) are credited and that the original publication in this journal is cited, in accordance with accepted academic practice. No use, distribution or reproduction is permitted which does not comply with these terms.
*Correspondence: Nanbert Zhong, nanbert.zhong@opwdd.ny.gov
†These authors have contributed equally to this work