- 1Laboratory for Clinical Cardiovascular Physiology, Amsterdam UMC, University of Amsterdam, Amsterdam, Netherlands
- 2Department of Medical Biology, Amsterdam UMC, University of Amsterdam, Amsterdam, Netherlands
- 3Department of Internal Medicine, Medisch Centrum Leeuwarden, Leeuwarden, Netherlands
- 4Department of Anesthesiology, Amsterdam UMC, University of Amsterdam, Amsterdam, Netherlands
- 5Department of Kinesiology, Faculty of Medicine, Research Center of the Institut Universitaire de Cardiologie et de Pneumologie de Québec, Université Laval, Québec, QC, Canada
- 6Department of Anesthesia, The Copenhagen Muscle Research Center, University of Copenhagen, Copenhagen, Denmark
- 7MRC/Arthritis Research UK Centre for Musculoskeletal Ageing Research, School of Life Sciences, University of Nottingham Medical School, Queen’s Medical Centre, Nottingham, United Kingdom
- 8Department of Internal Medicine, Amsterdam UMC, University of Amsterdam, Amsterdam, Netherlands
The human brain is constantly active and even small limitations to cerebral blood flow (CBF) may be critical for preserving oxygen and substrate supply, e.g., during exercise and hypoxia. Exhaustive exercise evokes a competition for the supply of oxygenated blood between the brain and the working muscles, and inability to increase cardiac output sufficiently during exercise may jeopardize cerebral perfusion of relevance for diabetic patients. The challenge in diabetes care is to optimize metabolic control to slow progression of vascular disease, but likely because of a limited ability to increase cardiac output, these patients perceive aerobic exercise to be more strenuous than healthy subjects and that limits the possibility to apply physical activity as a preventive lifestyle intervention. In this review, we consider the effects of functional activation by exercise on the brain and how it contributes to understanding the control of CBF with the limited exercise tolerance experienced by type 2 diabetic patients. Whether a decline in cerebral oxygenation and thereby reduced neural drive to working muscles plays a role for “central” fatigue during exhaustive exercise is addressed in relation to brain’s attenuated vascular response to exercise in type 2 diabetic subjects.
Introduction
Animals like the Crucian carp and the aquatic turtle can survive anoxia for extended periods of time (Sick et al., 1982; Lutz et al., 1985; Hochachka and Lutz, 2001; Nilsson and Lutz, 2004), but human brain function depends on continuous delivery of oxygen and nutrients. Thus, interruption of blood supply to the brain for only a few seconds results in loss of consciousness (Rossen et al., 1943; Finnerty et al., 1954; Smith et al., 2011). Accordingly, even minor limitations to cerebral blood flow (CBF) may be critical in preserving oxygen and substrate supply to the brain and in that regard the human brain is challenged by exercise and hypoxia (Kim, 2014). When the brain is activated to perform exercise, the increment in CBF enhances brain oxygenation whereas skeletal muscle oxygenation decreases progressively with work rate. Thus, functional activation of the brain initially leads to hyperperfusion, while the large increase in skeletal muscle blood flow during exercise may be taken to be insufficient (Quistorff et al., 2008).
Reduced exercise tolerance in type 2 diabetes mellitus (T2DM) is incompletely understood (Estacio et al., 1998; Fang et al., 2005), and has been attributed to cardiac insufficiency and impaired muscle metabolism (Poirier et al., 2000; Taegtmeyer et al., 2002; Scheuermann-Freestone et al., 2003; Stephens et al., 2007). We consider the effects of functional activation by exercise on the brain and how it contributes to understanding the control of CBF in relation to the limited exercise tolerance experienced by type 2 diabetic patients. Analogies and differences between the cerebral vs. skeletal muscle blood flow responses to exercise are highlighted with emphasis on the dependency of the human brain on the distribution of the available blood flow. A decline in cerebral oxygenation in the later stages of exhaustive exercise may reduce the motor drive to working muscles similar to what is observed during exercise in hypoxia (Rasmussen et al., 2010). Whether a decline in cerebral oxygenation with following reduced neural drive to working muscles plays a role in the development of “central” fatigue during exhaustive exercise is addressed in relation to the altered brain vascular response to exercise in type 2 diabetic patients and their accentuated perceived exertion.
Autonomic Neural Control of CBF During Exercise
The large increase in systolic blood pressure during exhaustive exercise challenges CBF control mechanisms including cerebrovascular or cerebral autoregulation, the cerebrovascular responsiveness (CVRCO2) to carbon dioxide (CO2) and oxygen (O2) partial pressures, matching of local cerebral blood supply to the metabolic demand (i.e., neurovascular coupling), neurogenic control (Immink et al., 2014; Ritz et al., 2014; Willie et al., 2014; Phillips et al., 2016), and maintenance of cardiac output (Ide et al., 1998, 1999a; Van Lieshout et al., 2001, 2003; Ogoh et al., 2005a; Bronzwaer et al., 2014, 2017). During exercise, CBF increases as quantified by several methods (for review, see Secher et al., 2008; Smith and Ainslie, 2017). Dynamic exercise enhances the transcranial Doppler ultrasound determined middle cerebral artery blood velocity (MCA V) and the 133Xe clearance determined CBF (Jorgensen et al., 1992) and also the blood flow in the internal carotid and vertebral arteries (Sato et al., 2011). Notably, the increase in CBF during cerebral activation is such that cerebral oxygenation is enhanced as expressed by blood-oxygen-level (BOLD) dependent imaging (Laughlin et al., 2012) and for whole-body exercise, a similar increase in cerebral oxygenation is demonstrated by near-infrared spectroscopy (Ide et al., 1999b). Changes in CBF in response to exercise are restricted to specific areas of the brain and, therefore, blood flow in a single brain artery or vein cannot be considered to fully represent flow to or from the brain as a whole, reflecting that the effects of exercise on brain metabolism are heterogeneous. For example, regulation of internal carotid and vertebral artery flow seems different not only during exercise (Sato et al., 2011) but also during simulated orthostatic stress (Ogoh et al., 2015b). Constancy of diameter of an insonated large cerebral artery is required to link changes in cerebral blood velocity to those in CBF (Coverdale et al., 2014; Verbree et al., 2014, 2017).
Sympathetic activity is proposed to enhance cerebral vascular tone to counteract the increase in cerebral perfusion pressure beyond what is designated as the cerebral autoregulatory range (Purkayastha et al., 2013; Ogoh et al., 2015a), with cerebral perfusion pressure defined as the difference between blood pressure at the level of the circle of Willis and the critical closing pressure, the pressure inside a blood vessel below which it collapses and blood flow ceases. Both sympathetic and cholinergic mechanisms are considered important for restricting the exercise-induced increase in CBF without affecting the cerebral metabolic rate for oxygen (Seifert et al., 2010; Purkayastha et al., 2013; Willie et al., 2014; Ogoh et al., 2015a). Of note, erythropoietin has been applied to improve athletic performance and endurance but it actually reduces cerebrovascular conductance during exercise both under normoxic and hypoxic conditions (Rasmussen et al., 2012). The contribution of autonomic neural control of CBF during exercise remains difficult to detangle (Van Lieshout and Secher, 2008; Mitchell et al., 2009; Willie et al., 2014). The presently available evidence for neurogenic CBF control from rest to exercise is mainly from direct sympathetic ganglion blockade studies. At rest, unilateral trigeminal ganglion stimulation reduces CBF as evaluated by transcranial Doppler ultrasound and by single-photon emission computed tomography (Seifert and Secher, 2011). During exercise, β-adrenergic receptor blockade restricts the increase in cardiac output and in MCA V whereas this attenuation is eliminated by stellate ganglion blockade (Ide et al., 1998). Intrinsic cerebrovascular sympathetic activity is indicated by jugular venous “spillover” of norepinephrine from the brain in healthy humans but not in patients with autonomic failure who lack sympathetic vasomotor control (Harms et al., 2000; Mitchell et al., 2009). Apart from these selective investigations numerous studies have manipulated CBF pharmacologically by e.g., angiotensin, α-adrenergic receptor agonists and antagonists, nitric oxide donors, and anesthetic agents (Purkayastha et al., 2013; Willie et al., 2014) but the effects of these interventions on cerebrovascular tone remain controversial (Van Lieshout and Secher, 2008; Willie et al., 2014). For instance, the similarity of reductions in arterial pressure and pulsatile change in MCA V before vs. during ganglion blockade while maintaining arterial pressure with phenylephrine was taken to suggest that sympathetic vasoconstriction, mediated through α2-adrenergic receptor activation, is not the underlying mechanism for the reduction in CBF during central hypovolemia (Zhang and Levine, 2007). Yet, it should be considered that phenylephrine may lower CBF while increasing mean arterial pressure (Stewart et al., 2013). In diabetic patients, both cerebral autoregulatory capacity (Kim et al., 2008a; Kim, 2014; Vianna et al., 2015) and CVRCO2 as the major operative mechanisms maintaining CBF may have become impaired (Dandona et al., 1978; Fulesdi et al., 1997), rendering diabetic patients more susceptible to ischemic episodes (Dandona et al., 1978; Kim et al., 2011).
Brain Vs. Skeletal Muscle Blood Flow Response to Exercise
A major difference between brain and skeletal muscle is that the brain is active under all living conditions and uses ~15% of cardiac output at rest (Ide et al., 1999a, 2000; Immink et al., 2009; Willie et al., 2014). The effects of exercising in the upright vs. seated position on cardiac preload are exemplified by a higher heart rate in the upright position (Yoshiga and Higuchi, 2002). Equally, the change to the upright posture accompanying the majority of exercise modalities affects both the arterial supply to and the venous drainage from the brain (Van Lieshout et al., 2003; Dawson et al., 2004; Gisolf et al., 2004). When assuming the upright position, global CBF and frontal cortical oxygenation decrease, seemingly at odds with the concept of cerebral autoregulation implicating constancy of CBF for a range of cerebral perfusion pressures. The “constant flow” autoregulation plateau has been constructed from data across different studies rather than quantifying the pressure-flow relationship within individual subjects that, however, is difficult given that the range of blood pressures required for relating flow to pressure remains effectively limited by autonomic cardiovascular reflex activity. Yet, maintaining CBF constant would require an autoregulatory efficacy with an infinite gain, which generally does not apply to biological systems (Van Lieshout et al., 2003; Willie et al., 2014). Obviously, the arterioles rather than large arteries represent the main side of vascular resistance, but also larger arteries contribute to vascular control (Iversen et al., 1995). For the brain, the large extracranial vessels and surface vessels contribute importantly to cerebrovascular resistance, thus being at least passively involved in regulation of CBF (Faraci and Heistad, 1990; Ritz et al., 2014; Willie et al., 2014).
The brain with its small vascular bed being tightly controlled takes up to ~25% of whole-body oxygen consumption at rest (Braz and Fisher, 2016). The vulnerability of the brain is exemplified by the fact that its function deteriorates when cerebral oxygenation is reduced by more than about 10% from the resting level, in contrast to skeletal muscles, that continue their activity despite an O2 desaturation below 10% (Quistorff et al., 2008; Secher et al., 2008). Continued exhaustive exercise evokes a competition for the supply of oxygenated blood between the brain and the working muscles. The brain activates the muscles, but from then on, the large increase in muscle blood flow and thus skeletal muscle vascular conductance represents a major competitor for continuous provision of oxygen and substrate upon which the brain relies (Secher et al., 2008). Heavy exercise with large muscle groups requests more blood than the heart can provide and thus requires tight sympathetic vasomotor control to maintain arterial pressure (Calbet et al., 2004). When humans exercise at maximal intensity, up to ~80–90% of total cardiac output is being distributed to skeletal and cardiac muscle (Laughlin et al., 2012). At the same time, an increase in regional CBF has to match the enhanced neuronal metabolism exemplified by an elevated cerebral metabolic rate for oxygen at that stage of exercise (Laughlin et al., 2012). Within the brain, in contrast to skeletal muscles, there is no capillary recruitment and creating and maintaining an elevated O2 gradient is a prerequisite given that the efficacy for O2 extraction by the brain compared to skeletal muscle is small.
Cardiac Output Supports CBF During Exercise
The size of cardiac output is important for regulation of CBF beyond arterial pressure both at rest and during exercise (Hellstrøm et al., 1994, 1996; Magnusson et al., 1997; Ide et al., 1998, 1999a,b, 2000; Gruhn et al., 2001; Van Lieshout et al., 2001; Ogoh et al., 2005a; Secher et al., 2008; Braz and Fisher, 2016). In consequence, an incompetence to increase cardiac output sufficiently during exercise may jeopardize cerebral perfusion and thereby the ability of the central nervous system to recruit and adequately drive the motoneurons. The role of cardiac output for distribution of flow is illustrated in patients with moderate heart failure for whom peak skeletal muscle perfusion is maintained, provided that the activated muscle mass is small. Involvement of a larger muscle mass, however, reduces peak leg blood flow, perfusion, and oxygen uptake (Magnusson et al., 1997). Similarly in these patients during one-legged exercise, MCA V is maintained but declines with two-legged exercise and exposes a competition between brain and skeletal muscle (Hellstrøm et al., 1996). Thus, the traditional concept that the brain is at the top of the hierarchy of competing physiological needs is challenged when cardiac output no longer matches tissue O2 requirements. Under these circumstances, exercise evokes cerebral deoxygenation, metabolic changes, and indices of fatigue similar to those observed during exercise in hypoxia (Secher et al., 2008; Rasmussen et al., 2010). Thus, reduced cerebral oxygenation may play a role for the development of central fatigue as an exercise capacity limiting factor (Rasmussen et al., 2010; Kim et al., 2015).
Exercise and Brain Vascular Control in Type 2 Diabetes
Physiological aging is associated with a decline in resting cerebral metabolism, global CBF, and gray matter flow but does not in itself implicate affected CBF control (for review, see Braz and Fisher, 2016). Specifically, the normal development of an initial increase in CBF in response to exercise is well maintained in the elderly (Laughlin et al., 2012; Fisher et al., 2013; Braz and Fisher, 2016). During maximal exercise in healthy humans, fatigue is preceded by reductions in systemic and skeletal muscle blood flow, and O2 delivery and uptake (Gonzalez-Alonso et al., 2004).
In middle-aged type 2 diabetic patients, the cardiac output reserve and work capacity are low and the increase in CBF that is present in healthy young and elderly does not develop (Figures 1, 2; Kim et al., 2015). Accordingly, these patients demonstrate an early reduction in cerebral oxygenation despite a larger brain O2 extraction, and they express enhanced perceived exertion, signifying a fundamental problem in brain vascular control during exercise (Kim et al., 2008a; Vianna et al., 2015). Yet, for these patients, the brain uptake of lactate and glucose is similar to what is found in healthy reference subjects (Kim, 2014; Kim et al., 2015), which points to cerebrovascular rather than brain metabolic derangement. In contrast to the vast amount of studies on the muscle blood flow response to exercise in type 2 diabetic patients, data on the CBF response to exercise in these patients are very sparse. In diabetic patients, progression of microvascular disease interferes with the physiological nocturnal decline in blood pressure, coinciding with a persistently increased arterial pulse pressure and reduced baroreflex sensitivity, contributing to their increased cardiovascular risk (Kim et al., 2019). Treatment of hypertension as a common comorbidity in type 2 diabetes is required to reduce the risk of hypertensive surges during strenuous exercise that challenge the brain vasculature, but intensive blood pressure control may, in contrast to nondiabetic hypertensive patients, reduce their CBF (Kim et al., 2011).
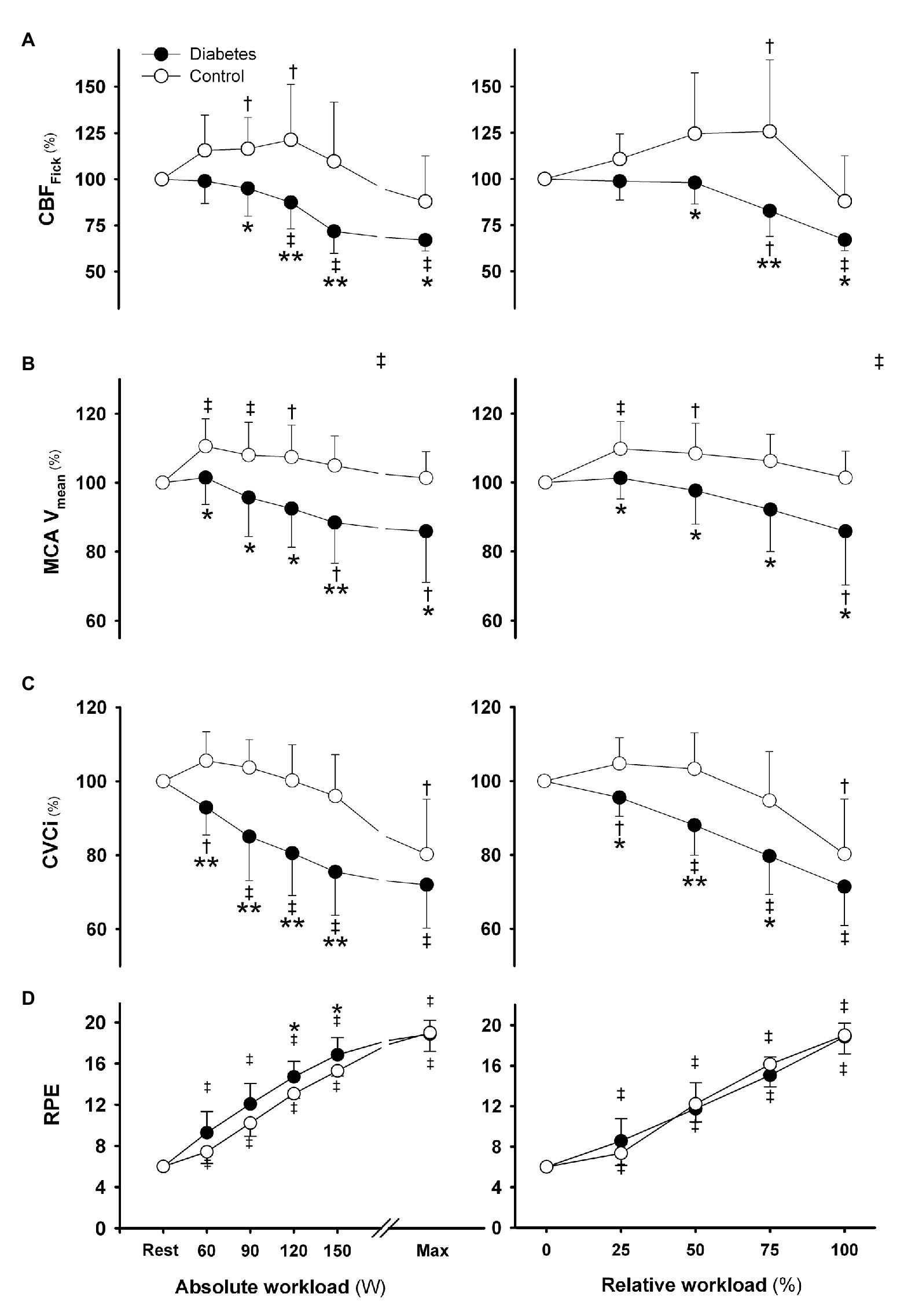
Figure 1. Cerebrovascular response to exercise in eight male type 2 diabetic patients without symptomatic cardio-vascular disease (closed circles) vs. seven age and gender matched healthy subjects (open circles) at the same absolute (left panels) and relative workload (right panels). (A) Cerebral blood flow derived from the Fick principle (CBFFick) from inverse arterial-jugular venous oxygen difference, (B) middle cerebral artery mean blood flow velocity (MCA Vmean), (C) cerebrovascular conductance index (CVCi), and (D) rating of perceived exertion (RPE; Borg scale). The patients demonstrated a decline in cerebral perfusion and oxygenation during incremental exercise associated with attenuated increases in cerebral and systemic vascular conductance compared with healthy controls. Cerebral oxygenation reached its lowest level at exhaustion at a 20% lower workload in type 2 diabetes mellitus (T2DM) patients than healthy controls and patients expressed a higher RPE than healthy controls. †p < 0.05 and ‡p < 0.01 vs. rest; *p < 0.05 and **p < 0.01 vs. control subjects. Values are mean ± SD (modified from Kim et al., 2015).
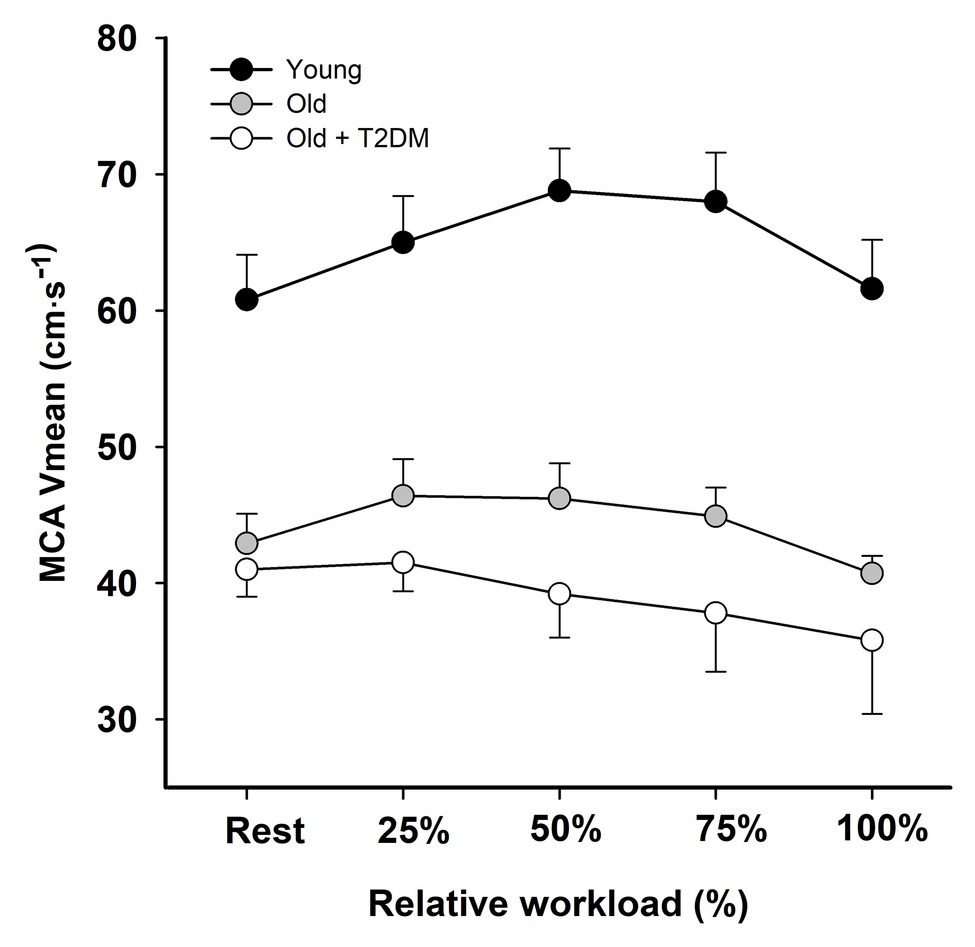
Figure 2. Middle cerebral artery mean blood flow velocity (MCA Vmean) at rest and during exercise at the same relative workload in young individuals (black circles), and in middle-aged subjects without (gray circles), and with type 2 diabetes (T2DM; open circles). During submaximal and during maximal exercise, cerebral perfusion was reduced in older individuals compared with young individuals, and the more so in the older diabetic patients. Values are mean ± SEM (adapted from Fisher et al., 2013; Kim et al., 2015).
From Deconditioning to Physical Exercise – a Challenge of Brain Vascular Control
Loss of skeletal muscle mass is a main factor for the increased incidence of type 2 diabetes with aging. Deconditioning as a result of physical inactivity vs. resistance exercise is associated with opposing adaptive responses. Resistance exercise provides better metabolic control (Baldi and Snowling, 2003), mitigates disuse-associated tendon stiffness, maintains or increases skeletal muscle mass, and improves whole body glucose disposal (Fenicchia et al., 2004). Thus, a focus on resistance exercise has been recommended for type 2 diabetic patients, specifically for the subgroup of sarcopenic or severely deconditioned older patients (Sigal et al., 2006). Resistance vs. endurance exercise has different cardiovascular effects. Resistance-type activities produce a considerably larger increase in arterial pressure, because of the mechanical compression of blood vessels together with repeated Valsalva-like maneuvers (MacDougall et al., 1985). Unlike aerobic exercise, resistance training affects central arterial compliance in healthy men (Miyachi et al., 2004).
In healthy young adults, isometric resistance exercise with vs. without concomitant straining produces a greater cerebrovascular challenge (Perry et al., 2020), whereas straining dominates the central and cerebral hemodynamic response to intense static exercise (Pott et al., 2003). Although acute changes in arterial blood pressure during physiological challenges are transmitted to the cerebral circulation, under normal conditions, CBF returns to its baseline value within a few seconds (Panerai et al., 2001; Pott et al., 2003; Immink et al., 2005; Labrecque et al., 2020). Cerebral vasoconstriction constantly plays a protective role during exercise of moderate to heavy intensity, in particular when pulse pressure exceeds the autoregulatory range (Ogoh et al., 2005b). When autoregulatory mechanisms are failing (Immink et al., 2005; Kim et al., 2008a; Frosch et al., 2017; Vranish et al., 2020) or overwhelmed by acute blood pressure surges beyond the autoregulatory range, e.g., grave hypertension, CBF becomes more directly related to its perfusion pressure, resulting in cerebral hyperperfusion manifested by retinal edema and encephalopathy (Immink et al., 2004).
Perspective
In the European Union, 55 million individuals suffer from type 2 diabetes and 66 million have impaired glucose tolerance, with an estimated ~4% annual increase. Optimizing metabolic control by behavioral modification including regular physical activity, thus slowing down progression of vascular disease is a task for diabetes care. From that point of view, physical activity represents a “medicine” for metabolic disease (Pedersen and Saltin, 2015; Pedersen, 2019). The challenge to optimize metabolic control in individuals with type 2 diabetes may be achieved at least in part by behavioral modification including regular physical activity (Kim et al., 2008b; Pedersen, 2017). Indeed, physical activity by patients with type 2 diabetes markedly improves the impaired insulin action and is considered a cornerstone in the treatment along with diet and medication. Unfortunately, however, type 2 diabetic patients perceive sustained aerobic exercise to be more strenuous than healthy, non-diabetic subjects. This sets a limit to the effectiveness of physical activity as a preventive lifestyle intervention for this patient population (Praet and van Loon, 2008, 2009; Huebschmann et al., 2009, 2015; Nadeau et al., 2009; Regensteiner et al., 2014; Senefeld et al., 2020). Left ventricular diastolic dysfunction may be an early manifestation of diabetic cardiomyopathy. When cardiac function deteriorates, the blood supply to the brain seems no longer safeguarded, pointing to the hitherto underexposed functional connection between heart and brain. Aerobic exercise itself may reveal arterial dysfunction associated with latent and overt cerebrovascular disease (Robertson et al., 2019).
In mice, exercise training increased brain mitochondrial biogenesis (Steiner et al., 2011) and a liver-to-brain axis was identified by which plasma glycosylphosphatidylinositol-specific phospholipase could transfer the benefits of exercise on neurogenesis in the brain from young to old mice (Ansere and Freeman, 2020; Horowitz et al., 2020). Nevertheless, regular physical exercise arguably continues to remain the most consistently effective health-enhancing strategy to attenuate the deterioration in brain structure and function related to aging and type 2 diabetes (Hillman et al., 2008; Mayhan et al., 2011; Espeland et al., 2018; Pedersen, 2019). When applying the concept that failure in regulation at multiple levels is common in diseases like diabetes (Pedersen and Saltin, 2015), a limited ability to increase cardiac output together with reduced systemic and cerebral vasodilatory capacity become primary targets for prevention and treatment, challenging integrative physiologists and clinicians alike.
Author Contributions
Y-SK contributed to the experimental design, data acquisition, data analysis, and writing the manuscript. BS contributed to data analysis and manuscript revision. PB contributed to manuscript writing. NS contributed to experimental design of studies and writing. JL supervised the study and contributed to the experimental design, data analysis, and writing the manuscript. All authors contributed to the article and approved the submitted version.
Funding
BS is supported by an educational grant from Edwards Lifesciences (2010B0797).
Conflict of Interest
The authors declare that the research was conducted in the absence of any commercial or financial relationships that could be construed as a potential conflict of interest.
References
Ansere, V. A., and Freeman, W. M. (2020). Exercising your mind. Science 369, 144–145. doi: 10.1126/science.abc8830
Baldi, J. C., and Snowling, N. (2003). Resistance training improves glycaemic control in obese type 2 diabetic men. Int. J. Sports Med. 24, 419–423. doi: 10.1055/s-2003-41173
Braz, I. D., and Fisher, J. P. (2016). The impact of age on cerebral perfusion, oxygenation and metabolism during exercise in humans. J. Physiol. 594, 4471–4483. doi: 10.1113/JP271081
Bronzwaer, A. S., Stok, W. J., Westerhof, B. E., and van Lieshout, J. J. (2014). Arterial pressure variations as parameters of brain perfusion in response to central blood volume depletion and repletion. Front. Physiol. 5:157. doi: 10.3389/fphys.2014.00157
Bronzwaer, A. G. T., Verbree, J., Stok, W. J., Daemen, M., van Buchem, M. A., van Osch, M. J. P., et al. (2017). Aging modifies the effect of cardiac output on middle cerebral artery blood flow velocity. Physiol. Rep. 5:e13361. doi: 10.14814/phy2.13361
Calbet, J. A., Jensen-Urstad, M., van Hall, G., Holmberg, H. C., Rosdahl, H., and Saltin, B. (2004). Maximal muscular vascular conductances during whole body upright exercise in humans. J. Physiol. 558, 319–331. doi: 10.1113/jphysiol.2003.059287
Coverdale, N. S., Gati, J. S., Opalevych, O., Perrotta, A., and Shoemaker, J. K. (2014). Cerebral blood flow velocity underestimates cerebral blood flow during modest hypercapnia and hypocapnia. J. Appl. Physiol. 117, 1090–1096. doi: 10.1152/japplphysiol.00285.2014
Dandona, P., James, I. M., Newbury, P. A., Woollard, M. L., and Beckett, A. G. (1978). Cerebral blood flow in diabetes mellitus: evidence of abnormal cerebrovascular reactivity. Br. Med. J. 2, 325–326. doi: 10.1136/bmj.2.6133.325
Dawson, E. A., Secher, N. H., Dalsgaard, M. K., Ogoh, S., Yoshiga, C. C., Gonzalez-Alonso, J., et al. (2004). Standing up to the challenge of standing: a siphon does not support cerebral blood flow in humans. Am. J. Physiol. Regul. Integr. Comp. Physiol. 287, R911–R914. doi: 10.1152/ajpregu.00196.2004
Espeland, M. A., Luchsinger, J. A., Neiberg, R. H., Carmichael, O., Laurienti, P. J., Pi-Sunyer, X., et al. (2018). Long term effect of intensive lifestyle intervention on cerebral blood flow. J. Am. Geriatr. Soc. 66, 120–126. doi: 10.1111/jgs.15159
Estacio, R. O., Regensteiner, J. G., Wolfel, E. E., Jeffers, B., Dickenson, M., and Schrier, R. W. (1998). The association between diabetic complications and exercise capacity in NIDDM patients. Diabetes Care 21, 291–295. doi: 10.2337/diacare.21.2.291
Fang, Z. Y., Sharman, J., Prins, J. B., and Marwick, T. H. (2005). Determinants of exercise capacity in patients with type 2 diabetes. Diabetes Care 28, 1643–1648. doi: 10.2337/diacare.28.7.1643
Faraci, F. M., and Heistad, D. D. (1990). Regulation of large cerebral arteries and cerebral microvascular pressure. Circ. Res. 66, 8–17. doi: 10.1161/01.res.66.1.8
Fenicchia, L. M., Kanaley, J. A., Azevedo, J. L. Jr., Miller, C. S., Weinstock, R. S., Carhart, R. L., et al. (2004). Influence of resistance exercise training on glucose control in women with type 2 diabetes. Metabolism 53, 284–289. doi: 10.1016/j.metabol.2003.10.007
Finnerty, F. A. Jr., Witkin, L., and Fazekas, J. F. (1954). Cerebral hemodynamics during cerebral ischemia induced by acute hypotension. J. Clin. Invest. 33, 1227–1232. doi: 10.1172/JCI102997
Fisher, J. P., Hartwich, D., Seifert, T., Olesen, N. D., McNulty, C. L., Nielsen, H. B., et al. (2013). Cerebral perfusion, oxygenation and metabolism during exercise in young and elderly individuals. J. Physiol. 591, 1859–1870. doi: 10.1113/jphysiol.2012.244905
Frosch, O. H., Yau, P. L., Osorio, R. S., Rusinek, H., Storey, P., and Convit, A. (2017). Insulin resistance among obese middle-aged is associated with decreased cerebrovascular reactivity. Neurology 89, 249–255. doi: 10.1212/WNL.0000000000004110
Fulesdi, B., Limburg, M., Bereczki, D., Michels, R. P., Neuwirth, G., Legemate, D., et al. (1997). Impairment of cerebrovascular reactivity in long-term type 1 diabetes. Diabetes 46, 1840–1845. doi: 10.2337/diab.46.11.1840
Gisolf, J., van Lieshout, J. J., van Heusden, K., Pott, F., Stok, W. J., and Karemaker, J. M. (2004). Human cerebral venous outflow pathway depends on posture and central venous pressure. J. Physiol. 560, 317–327. doi: 10.1113/jphysiol.2004.070409
Gonzalez-Alonso, J., Dalsgaard, M. K., Osada, T., Volianitis, S., Dawson, E. A., Yoshiga, C. C., et al. (2004). Brain and central hemodynamics and oxygenation during maximal exercise in humans. J. Physiol. 557, 331–342. doi: 10.1113/jphysiol.2004.060574
Gruhn, N., Larsen, F. S., Boesgaard, S., Knudsen, G. M., Mortensen, S. A., Thomsen, G., et al. (2001). Cerebral blood flow in patients with chronic heart failure before and after heart transplantation. Stroke 32, 2530–2533. doi: 10.1161/hs1101.098360
Harms, M. P., Colier, W. N., Wieling, W., Lenders, J. W., Secher, N. H., and van Lieshout, J. J. (2000). Orthostatic tolerance, cerebral oxygenation, and blood velocity in humans with sympathetic failure. Stroke 31, 1608–1614. doi: 10.1161/01.str.31.7.1608
Hellstrøm, G., Magnusson, G., Saltin, B., and Wahlgren, N. G. (1994). Cerebral haemodynamic effects of physical exercise in patients with chronic heart failure. Cerebrovasc. Dis. 4, 256–261.
Hellstrøm, G., Magnusson, G., Wahlgren, N. G., and Saltin, B. (1996). Physical exercise may impair cerebral perfusion in patients with chronic heart failure. Cardiol. Elder. 4, 191–194. doi: 10.1152/japplphysiol.00817.2016
Hillman, C. H., Erickson, K. I., and Kramer, A. F. (2008). Be smart, exercise your heart: exercise effects on brain and cognition. Nat. Rev. Neurosci. 9, 58–65. doi: 10.1038/nrn2298
Hochachka, P. W., and Lutz, P. L. (2001). Mechanism, origin, and evolution of anoxia tolerance in animals. Comp. Biochem. Physiol. B Biochem. Mol. Biol. 130, 435–459. doi: 10.1016/s1096-4959(01)00408-0
Horowitz, A. M., Fan, X., Bieri, G., Smith, L. K., Sanchez-Diaz, C. I., Schroer, A. B., et al. (2020). Blood factors transfer beneficial effects of exercise on neurogenesis and cognition to the aged brain. Science 369, 167–173. doi: 10.1126/science.aaw2622
Huebschmann, A. G., Kohrt, W. M., Herlache, L., Wolfe, P., Daugherty, S., Reusch, J. E., et al. (2015). Type 2 diabetes exaggerates exercise effort and impairs exercise performance in older women. BMJ Open Diabetes Res. Care 3:e000124. doi: 10.1136/bmjdrc-2015-000124
Huebschmann, A. G., Reis, E. N., Emsermann, C., Dickinson, L. M., Reusch, J. E., Bauer, T. A., et al. (2009). Women with type 2 diabetes perceive harder effort during exercise than nondiabetic women. Appl. Physiol. Nutr. Metab. 34, 851–857. doi: 10.1139/H09-074
Ide, K., Boushel, R., Sorensen, H. M., Fernandes, A., Cai, Y., Pott, F., et al. (2000). Middle cerebral artery blood velocity during exercise with beta-1 adrenergic and unilateral stellate ganglion blockade in humans. Acta Physiol. Scand. 170, 33–38. doi: 10.1046/j.1365-201x.2000.00757.x
Ide, K., Gullov, A. L., Pott, F., Van Lieshout, J. J., Koefoed, B. G., Petersen, P., et al. (1999a). Middle cerebral artery blood velocity during exercise in patients with atrial fibrillation. Clin. Physiol. 19, 284–289. doi: 10.1046/j.1365-2281.1999.00178.x
Ide, K., Horn, A., and Secher, N. H. (1999b). Cerebral metabolic response to submaximal exercise. J. Appl. Physiol. 87, 1604–1608. doi: 10.1152/jappl.1999.87.5.1604
Ide, K., Pott, F., Van Lieshout, J. J., and Secher, N. H. (1998). Middle cerebral artery blood velocity depends on cardiac output during exercise with a large muscle mass. Acta Physiol. Scand. 162, 13–20. doi: 10.1046/j.1365-201X.1998.0280f.x
Immink, R. V., Pott, F. C., Secher, N. H., and van Lieshout, J. J. (2014). Hyperventilation, cerebral perfusion, and syncope. J. Appl. Physiol. 116, 844–851. doi: 10.1152/japplphysiol.00637.2013
Immink, R. V., Truijen, J., Secher, N. H., and Van Lieshout, J. J. (2009). Transient influence of end-tidal carbon dioxide tension on the postural restraint in cerebral perfusion. J. Appl. Physiol. 107, 816–823. doi: 10.1152/japplphysiol.91198.2008
Immink, R. V., van den Born, B. J., van Montfrans, G. A., Koopmans, R. P., Karemaker, J. M., and van Lieshout, J. J. (2004). Impaired cerebral autoregulation in patients with malignant hypertension. Circulation 110, 2241–2245. doi: 10.1161/01.CIR.0000144472.08647.40
Immink, R. V., van Montfrans, G. A., Stam, J., Karemaker, J. M., Diamant, M., and van Lieshout, J. J. (2005). Dynamic cerebral autoregulation in acute lacunar and middle cerebral artery territory ischemic stroke. Stroke 36, 2595–2600. doi: 10.1161/01.STR.0000189624.06836.03
Iversen, H. K., Madsen, P., Matzen, S., and Secher, N. H. (1995). Arterial diameter during central volume depletion in humans. Eur. J. Appl. Physiol. Occup. Physiol. 72, 165–169. doi: 10.1007/BF00964132
Jorgensen, L. G., Perko, G., and Secher, N. H. (1992). Regional cerebral artery mean flow velocity and blood flow during dynamic exercise in humans. J. Appl. Physiol. 73, 1825–1830. doi: 10.1152/jappl.1992.73.5.1825
Kim, Y. S. (2014). Cerebral blood flow control in small vessel disease. In Universiteit van Amsterdam [Host].
Kim, Y. S., Davis, S., Stok, W. J., van Ittersum, F. J., and van Lieshout, J. J. (2019). Impaired nocturnal blood pressure dipping in patients with type 2 diabetes mellitus. Hypertens. Res. 42, 59–66. doi: 10.1038/s41440-018-0130-5
Kim, Y. S., Davis, S. C., Truijen, J., Stok, W. J., Secher, N. H., and van Lieshout, J. J. (2011). Intensive blood pressure control affects cerebral blood flow in type 2 diabetes mellitus patients. Hypertension 57, 738–745. doi: 10.1161/HYPERTENSIONAHA.110.160523
Kim, Y. S., Immink, R. V., Stok, W. J., Karemaker, J. M., Secher, N. H., and van Lieshout, J. J. (2008a). Dynamic cerebral autoregulatory capacity is affected early in type 2 diabetes. Clin. Sci. 115, 255–262. doi: 10.1042/CS20070458
Kim, Y. S., Secher, N. H., and van Lieshout, J. J. (2008b). Resistance exercise and control of cerebral blood flow in type 2 diabetes. Diabetologia 51, 1755–1756. doi: 10.1007/s00125-008-1068-y
Kim, Y. S., Seifert, T., Brassard, P., Rasmussen, P., Vaag, A., Nielsen, H. B., et al. (2015). Impaired cerebral blood flow and oxygenation during exercise in type 2 diabetic patients. Physiol. Rep. 3:e12430. doi: 10.14814/phy2.12430
Labrecque, L., Drapeau, A., Rahimaly, K., Imhoff, S., Billaut, F., and Brassard, P. (2020). Comparable blood velocity changes in middle and posterior cerebral arteries during and following acute high-intensity exercise in young fit women. Physiol. Rep. 8:e14430. doi: 10.14814/phy2.14430
Laughlin, M. H., Davis, M. J., Secher, N. H., van Lieshout, J. J., Arce-Esquivel, A. A., Simmons, G. H., et al. (2012). Peripheral circulation. Compr. Physiol. 2, 321–447. doi: 10.1002/cphy.c100048
Lutz, P., Rosenthal, M., and Sick, T. (1985). Living without oxygen: turtle brain as a model of anaerobic metabolism. Mol. Physiol. 8, 411–425.
MacDougall, J. D., Tuxen, D., Sale, D. G., Moroz, J. R., and Sutton, J. R. (1985). Arterial blood pressure response to heavy resistance exercise. J. Appl. Physiol. 58, 785–790. doi: 10.1152/jappl.1985.58.3.785
Magnusson, G., Kaijser, L., Sylven, C., Karlberg, K. E., Isberg, B., and Saltin, B. (1997). Peak skeletal muscle perfusion is maintained in patients with chronic heart failure when only a small muscle mass is exercised. Cardiovasc. Res. 33, 297–306. doi: 10.1016/s0008-6363(96)00249-0
Mayhan, W. G., Arrick, D. M., Patel, K. P., and Sun, H. (2011). Exercise training normalizes impaired NOS-dependent responses of cerebral arterioles in type 1 diabetic rats. Am. J. Physiol. Heart Circ. Physiol. 300, H1013–H1020. doi: 10.1152/ajpheart.00873.2010
Mitchell, D. A., Lambert, G., Secher, N. H., Raven, P. B., van Lieshout, J., and Esler, M. D. (2009). Jugular venous overflow of noradrenaline from the brain: a neurochemical indicator of cerebrovascular sympathetic nerve activity in humans. J. Physiol. 587, 2589–2597. doi: 10.1113/jphysiol.2008.167999
Miyachi, M., Kawano, H., Sugawara, J., Takahashi, K., Hayashi, K., Yamazaki, K., et al. (2004). Unfavorable effects of resistance training on central arterial compliance: a randomized intervention study. Circulation 110, 2858–2863. doi: 10.1161/01.CIR.0000146380.08401.99
Nadeau, K. J., Zeitler, P. S., Bauer, T. A., Brown, M. S., Dorosz, J. L., Draznin, B., et al. (2009). Insulin resistance in adolescents with type 2 diabetes is associated with impaired exercise capacity. J. Clin. Endocrinol. Metab. 94, 3687–3695. doi: 10.1210/jc.2008-2844
Nilsson, G. E., and Lutz, P. L. (2004). Anoxia tolerant brains. J. Cereb. Blood Flow Metab. 24, 475–486. doi: 10.1097/00004647-200405000-00001
Ogoh, S., Brothers, R. M., Barnes, Q., Eubank, W. L., Hawkins, M. N., Purkayastha, S., et al. (2005a). The effect of changes in cardiac output on middle cerebral artery mean blood velocity at rest and during exercise. J. Physiol. 569, 697–704. doi: 10.1113/jphysiol.2005.095836
Ogoh, S., Dalsgaard, M. K., Yoshiga, C. C., Dawson, E. A., Keller, D. M., Raven, P. B., et al. (2005b). Dynamic cerebral autoregulation during exhaustive exercise in humans. Am. J. Physiol. Heart Circ. Physiol. 288, H1461–H1467. doi: 10.1152/ajpheart.00948.2004
Ogoh, S., Hirasawa, A., Raven, P. B., Rebuffat, T., Denise, P., Lericollais, R., et al. (2015a). Effect of an acute increase in central blood volume on cerebral hemodynamics. Am. J. Physiol. Regul. Integr. Comp. Physiol. 309, R902–R911. doi: 10.1152/ajpregu.00137.2015
Ogoh, S., Sato, K., Okazaki, K., Miyamoto, T., Hirasawa, A., Sadamoto, T., et al. (2015b). Blood flow in internal carotid and vertebral arteries during graded lower body negative pressure in humans. Exp. Physiol. 100, 259–266. doi: 10.1113/expphysiol.2014.083964
Panerai, R. B., Dawson, S. L., Eames, P. J., and Potter, J. F. (2001). Cerebral blood flow velocity response to induced and spontaneous sudden changes in arterial blood pressure. Am. J. Physiol. Heart Circ. Physiol. 280, H2162–H2174. doi: 10.1152/ajpheart.2001.280.5.H2162
Pedersen, B. K. (2017). Anti-inflammatory effects of exercise: role in diabetes and cardiovascular disease. Eur. J. Clin. Investig. 47, 600–611. doi: 10.1111/eci.12781
Pedersen, B. K. (2019). The physiology of optimizing health with a focus on exercise as medicine. Annu. Rev. Physiol. 81, 607–627. doi: 10.1146/annurev-physiol-020518-114339
Pedersen, B. K., and Saltin, B. (2015). Exercise as medicine—evidence for prescribing exercise as therapy in 26 different chronic diseases. Scand. J. Med. Sci. Sports 25(Suppl. 3), 1–72. doi: 10.1111/sms.12581
Perry, B. G., De Hamel, T., Thomas, K. N., Wilson, L. C., Gibbons, T. D., and Cotter, J. D. (2020). Cerebrovascular haemodynamics during isometric resistance exercise with and without the Valsalva manoeuvre. Eur. J. Appl. Physiol. 120, 467–479. doi: 10.1007/s00421-019-04291-7
Phillips, A. A., Chan, F. H., Zheng, M. M., Krassioukov, A. V., and Ainslie, P. N. (2016). Neurovascular coupling in humans: physiology, methodological advances and clinical implications. J. Cereb. Blood Flow Metab. 36, 647–664. doi: 10.1177/0271678X15617954
Poirier, P., Garneau, C., Bogaty, P., Nadeau, A., Marois, L., Brochu, C., et al. (2000). Impact of left ventricular diastolic dysfunction on maximal treadmill performance in normotensive subjects with well-controlled type 2 diabetes mellitus. Am. J. Cardiol. 85, 473–477. doi: 10.1016/s0002-9149(99)00774-2
Pott, F., Van Lieshout, J. J., Ide, K., Madsen, P., and Secher, N. H. (2003). Middle cerebral artery blood velocity during intense static exercise is dominated by a Valsalva maneuver. J. Appl. Physiol. 94, 1335–1344. doi: 10.1152/japplphysiol.00457.2002
Praet, S. F., and van Loon, L. J. (2008). Exercise: the brittle cornerstone of type 2 diabetes treatment. Diabetologia 51, 398–401. doi: 10.1007/s00125-007-0910-y
Praet, S. F., and van Loon, L. J. (2009). Exercise therapy in type 2 diabetes. Acta Diabetol. 46, 263–278. doi: 10.1007/s00592-009-0129-0
Purkayastha, S., Saxena, A., Eubank, W. L., Hoxha, B., and Raven, P. B. (2013). Alpha1-adrenergic receptor control of the cerebral vasculature in humans at rest and during exercise. Exp. Physiol. 98, 451–461. doi: 10.1113/expphysiol.2012.066118
Quistorff, B., Secher, N. H., and Van Lieshout, J. J. (2008). Lactate fuels the human brain during exercise. FASEB J. 22, 3443–3449. doi: 10.1096/fj.08-106104
Rasmussen, P., Kim, Y. S., Krogh-Madsen, R., Lundby, C., Olsen, N. V., Secher, N. H., et al. (2012). Both acute and prolonged administration of EPO reduce cerebral and systemic vascular conductance in humans. FASEB J. 26, 1343–1348. doi: 10.1096/fj.11-193508
Rasmussen, P., Nielsen, J., Overgaard, M., Krogh-Madsen, R., Gjedde, A., Secher, N. H., et al. (2010). Reduced muscle activation during exercise related to brain oxygenation and metabolism in humans. J. Physiol. 588, 1985–1995. doi: 10.1113/jphysiol.2009.186767
Regensteiner, J. G., Bauer, T. A., Huebschmann, A. G., Herlache, L., Weinberger, H. D., Wolfel, E. E., et al. (2014). Sex differences in the effects of type 2 diabetes on exercise performance. Med. Sci. Sports Exerc. 47, 58–65. doi: 10.1249/MSS.0000000000000371
Ritz, K., Denswil, N. P., Stam, O. C., van Lieshout, J. J., and Daemen, M. J. (2014). Cause and mechanisms of intracranial atherosclerosis. Circulation 130, 1407–1414. doi: 10.1161/CIRCULATIONAHA.114.011147
Robertson, A. D., Atwi, S., Kostoglou, K., Verhoeff, N., Oh, P. I., Mitsis, G. D., et al. (2019). Cerebrovascular pulsatility during rest and exercise reflects hemodynamic impairment in stroke and cerebral small vessel disease. Ultrasound Med. Biol. 45, 3116–3127. doi: 10.1016/j.ultrasmedbio.2019.08.019
Rossen, R., Kabat, H., and Anderson, J. P. (1943). Acute arrest of cerebral circulation in man. Arch. Neurol. Psychiatr. 50, 510–528.
Sato, K., Ogoh, S., Hirasawa, A., Oue, A., and Sadamoto, T. (2011). The distribution of blood flow in the carotid and vertebral arteries during dynamic exercise in humans. J. Physiol. 589, 2847–2856. doi: 10.1113/jphysiol.2010.204461
Scheuermann-Freestone, M., Madsen, P. L., Manners, D., Blamire, A. M., Buckingham, R. E., Styles, P., et al. (2003). Abnormal cardiac and skeletal muscle energy metabolism in patients with type 2 diabetes. Circulation 107, 3040–3046. doi: 10.1161/01.CIR.0000072789.89096.10
Secher, N. H., Seifert, T., and Van Lieshout, J. J. (2008). Cerebral blood flow and metabolism during exercise: implications for fatigue. J. Appl. Physiol. 104, 306–314. doi: 10.1152/japplphysiol.00853.2007
Seifert, T., Fisher, J. P., Young, C. N., Hartwich, D., Ogoh, S., Raven, P. B., et al. (2010). Glycopyrrolate abolishes the exercise-induced increase in cerebral perfusion in humans. Exp. Physiol. 95, 1016–1025. doi: 10.1113/expphysiol.2010.054346
Seifert, T., and Secher, N. H. (2011). Sympathetic influence on cerebral blood flow and metabolism during exercise in humans. Prog. Neurobiol. 95, 406–426. doi: 10.1016/j.pneurobio.2011.09.008
Senefeld, J. W., Harmer, A. R., and Hunter, S. K. (2020). Greater lower limb fatigability in people with prediabetes than controls. Med. Sci. Sports Exerc. 52, 1176–1186. doi: 10.1249/MSS.0000000000002238
Sick, T. J., Lutz, P. L., LaManna, J. C., and Rosenthal, M. (1982). Comparative brain oxygenation and mitochondrial redox activity in turtles and rats. J. Appl. Physiol. 53, 1354–1359. doi: 10.1152/jappl.1982.53.6.1354
Sigal, R. J., Kenny, G. P., Wasserman, D. H., Castaneda-Sceppa, C., and White, R. D. (2006). Physical activity/exercise and type 2 diabetes: a consensus statement from the American Diabetes Association. Diabetes Care 29, 1433–1438. doi: 10.2337/dc06-9910
Smith, K. J., and Ainslie, P. N. (2017). Regulation of cerebral blood flow and metabolism during exercise. Exp. Physiol. 102, 1356–1371. doi: 10.1113/EP086249
Smith, B. A., Clayton, E. W., and Robertson, D. (2011). Experimental arrest of cerebral blood flow in human subjects: the red wing studies revisited. Perspect. Biol. Med. 54, 121–131. doi: 10.1353/pbm.2011.0018
Steiner, J. L., Murphy, E. A., McClellan, J. L., Carmichael, M. D., and Davis, J. M. (2011). Exercise training increases mitochondrial biogenesis in the brain. J. Appl. Physiol. 111, 1066–1071. doi: 10.1152/japplphysiol.00343.2011
Stephens, F. B., Constantin-Teodosiu, D., and Greenhaff, P. L. (2007). New insights concerning the role of carnitine in the regulation of fuel metabolism in skeletal muscle. J. Physiol. 581, 431–444. doi: 10.1113/jphysiol.2006.125799
Stewart, J. M., Medow, M. S., DelPozzi, A., Messer, Z. R., Terilli, C., and Schwartz, C. E. (2013). Middle cerebral O(2) delivery during the modified Oxford maneuver increases with sodium nitroprusside and decreases during phenylephrine. Am. J. Physiol. Heart Circ. Physiol. 304, H1576–H1583. doi: 10.1152/ajpheart.00114.2013
Taegtmeyer, H., McNulty, P., and Young, M. E. (2002). Adaptation and maladaptation of the heart in diabetes: part I: general concepts. Circulation 105, 1727–1733. doi: 10.1161/01.cir.0000012466.50373.e8
Van Lieshout, J. J., Pott, F., Madsen, P. L., van Goudoever, J., and Secher, N. H. (2001). Muscle tensing during standing: effects on cerebral tissue oxygenation and cerebral artery blood velocity. Stroke 32, 1546–1551. doi: 10.1161/01.str.32.7.1546
Van Lieshout, J. J., and Secher, N. H. (2008). Point:counterpoint: sympathetic activity does/does not influence cerebral blood flow. Point: sympathetic activity does influence cerebral blood flow. J. Appl. Physiol. 105, 1364–1366. doi: 10.1152/japplphysiol.90597.2008
Van Lieshout, J. J., Wieling, W., Karemaker, J. M., and Secher, N. H. (2003). Syncope, cerebral perfusion, and oxygenation. J. Appl. Physiol. 94, 833–848. doi: 10.1152/japplphysiol.00260.2002
Verbree, J., Bronzwaer, A. S., Ghariq, E., Versluis, M. J., Daemen, M. J., van Buchem, M. A., et al. (2014). Assessment of middle cerebral artery diameter during hypocapnia and hypercapnia in humans using ultra-high-field MRI. J. Appl. Physiol. 117, 1084–1089. doi: 10.1152/japplphysiol.00651.2014
Verbree, J., Bronzwaer, A., van Buchem, M. A., Daemen, M., van Lieshout, J. J., and van Osch, M. (2017). Middle cerebral artery diameter changes during rhythmic handgrip exercise in humans. J. Cereb. Blood Flow Metab. 37, 2921–2927. doi: 10.1177/0271678X16679419
Vianna, L. C., Deo, S. H., Jensen, A. K., Holwerda, S. W., Zimmerman, M. C., and Fadel, P. J. (2015). Impaired dynamic cerebral autoregulation at rest and during isometric exercise in type 2 diabetes patients. Am. J. Physiol. Heart Circ. Physiol. 308, H681–H687. doi: 10.1152/ajpheart.00343.2014
Vranish, J. R., Holwerda, S. W., Kaur, J., and Fadel, P. J. (2020). Augmented pressor and sympathoexcitatory responses to the onset of isometric handgrip in patients with type 2 diabetes. Am. J. Physiol. Regul. Integr. Comp. Physiol. 318, R311–R319. doi: 10.1152/ajpregu.00109.2019
Willie, C. K., Tzeng, Y. C., Fisher, J. A., and Ainslie, P. N. (2014). Integrative regulation of human brain blood flow. J. Physiol. 592, 841–859. doi: 10.1113/jphysiol.2013.268953
Yoshiga, C. C., and Higuchi, M. (2002). Heart rate is lower during ergometer rowing than during treadmill running. Eur. J. Appl. Physiol. 87, 97–100. doi: 10.1007/s00421-002-0599-z
Keywords: cardiac output, cerebral blood flow, cerebral oxygenation, cerebral metabolism, diabetes, vascular conductance
Citation: Kim Y-S, van der Ster BJP, Brassard P, Secher NH and van Lieshout JJ (2021) Cerebral vs. Cardiovascular Responses to Exercise in Type 2 Diabetic Patients. Front. Physiol. 11:583155. doi: 10.3389/fphys.2020.583155
Edited by:
Heikki Olavi Tikkanen, University of Eastern Finland, FinlandReviewed by:
Marko S. Laaksonen, Mid Sweden University, SwedenZeljko Dujic, University of Split, Croatia
Copyright © 2021 Kim, van der Ster, Brassard, Secher and van Lieshout. This is an open-access article distributed under the terms of the Creative Commons Attribution License (CC BY). The use, distribution or reproduction in other forums is permitted, provided the original author(s) and the copyright owner(s) are credited and that the original publication in this journal is cited, in accordance with accepted academic practice. No use, distribution or reproduction is permitted which does not comply with these terms.
*Correspondence: Johannes J. van Lieshout, ai5qLnZhbmxpZXNob3V0QGFtc3RlcmRhbXVtYy5ubA==, orcid.org/0000-0002-3646-2122