- 1Department of Biochemistry and Molecular Biology, Mayo Clinic, Rochester, MN, United States
- 2Department of Cardiovascular Medicine, Mayo Clinic, Rochester, MN, United States
- 3Clinical Center for Gene Diagnosis and Therapy, The Second Xiangya Hospital of Central South University, Changsha, China
Cardiomyopathies are a highly heterogeneous group of heart muscle disorders. More than 100 causative genes have been linked to various cardiomyopathies, which explain about half of familial cardiomyopathy cases. More than a dozen candidate therapeutic signaling pathways have been identified; however, precision medicine is not being used to treat the various types of cardiomyopathy because knowledge is lacking for how to tailor treatment plans for different genetic causes. Adult zebrafish (Danio rerio) have a higher throughout than rodents and are an emerging vertebrate model for studying cardiomyopathy. Herein, we review progress in the past decade that has proven the feasibility of this simple vertebrate for modeling inherited cardiomyopathies of distinct etiology, identifying effective therapeutic strategies for a particular type of cardiomyopathy, and discovering new cardiomyopathy genes or new therapeutic strategies via a forward genetic approach. On the basis of this progress, we discuss future research that would benefit from integrating this emerging model, including discovery of remaining causative genes and development of genotype-based therapies. Studies using this efficient vertebrate model are anticipated to significantly accelerate the implementation of precision medicine for inherited cardiomyopathies.
Introduction
Cardiomyopathy refers to a group of heterogeneous heart muscle disorders that cause cardiac dysfunction. Cardiomyopathy is the most common cause of heart failure and afflicts millions of people worldwide (Lipshultz et al., 2019). Non-ischemic cardiomyopathy can be broadly classified, based on clinical features, into hypertrophic cardiomyopathy (HCM), dilated cardiomyopathy (DCM), restrictive cardiomyopathy (RCM), and arrhythmogenic cardiomyopathy (ACM) (Maron et al., 2006). HCM, the most common form of genetic heart disease, is characterized by left ventricular (LV) hypertrophy (thickening) with diastolic dysfunction (estimated incidence, 1 in 500 persons) (Makavos et al., 2019). Major diagnostic criteria for HCM is a wall thickness ≥15 mm in one or more LV myocardial segments. Characteristic changes on electrocardiography may include repolarization changes, T-wave inversions, and abnormal Q waves (Gersh et al., 2011; Authors/Task Force members, Elliott et al., 2014). DCM, the most common type of non-ischemic cardiomyopathy, is characterized by an enlarged ventricular chamber, thinned ventricular walls, and systolic dysfunction (estimated prevalence, 1 in 250 persons) (Rosenbaum et al., 2020). Clinical diagnosis of DCM is based on two major criteria: (1) LV fractional shortening is <25% and/or LV ejection fraction is <45%; and (2) LV end-diastolic diameter is >117% of predicated value corrected for age and body surface area (Bozkurt et al., 2016; Pinto et al., 2016). RCM, the rarest form of cardiomyopathy, is characterized by diastolic dysfunction but normal or near normal systolic function and restrictive ventricular filling pattern on echocardiography (estimated prevalence, 1 in 5,000 persons) (Muchtar et al., 2017). ACM, another rare cardiac muscle disorder, is characterized by structural abnormalities occurring mainly in the right ventricle and ventricular arrhythmia (estimated prevalence, between 1 in 1,000 and 1 in 5,000 persons) (Mattesi et al., 2020). Major diagnostic characteristics of ACM include global or regional dysfunction and structural alterations, fibrous replacement of the right ventricle (RV)-free wall myocardium with or without fatty replacement of tissue on endomyocardial biopsy, repolarization abnormalities, depolarization/conduction abnormalities, arrhythmias, and family history (Marcus et al., 2010; Towbin et al., 2019).
Distinct phenotypes of cardiomyopathies could be partially explained by genetic heterogeneity. Since the discovery of MYH7, the first causative gene for HCM, more than 100 genes have been linked to cardiomyopathies (Geisterfer-Lowrance et al., 1990; Tanigawa et al., 1990; Marian and Braunwald, 2017; Muchtar et al., 2017; Yotti et al., 2019; James et al., 2020). Beyond a monogenic disease, findings from next-generation sequencing (NGS) suggest multiple genetic hits could contribute to phenotypic severity of cardiomyopathies (Xu et al., 2010; Harakalova et al., 2015; Kolokotronis et al., 2019). Both phenotypic and genetic heterogeneity strongly suggest that precision medicine should be practiced to treat cardiomyopathies of various causes (Fatkin et al., 2019). However, standard treatments are still the norm because insufficient knowledge exists to individualize treatment with precision medicine.
Animal models of cardiomyopathy are needed for comparing phenotypes of cardiomyopathies of various causes, deciphering the mechanisms of disease, and developing effective therapeutic strategies. Rodents are classic vertebrate models that have contributed substantially to our knowledge of cardiomyopathies. More than a dozen potential therapeutic pathways and related compounds have been identified, some of which have already been translated clinically (Ashrafian et al., 2011; Van Berlo et al., 2013; Kieserman et al., 2019; Pinilla-Vera et al., 2019; Repetti et al., 2019). However, the high cost and low throughput associated with rodent models has impeded their use in developing precision medicine. For example, it would be a daunting task to assess all known candidate therapies systematically in each rodent cardiomyopathy model of a particular genetic cause. Therefore, a reliable vertebrate model with higher throughput was needed.
Zebrafish are small tropical fish that are highly similar genetically to humans (Howe et al., 2013). Many unique advantages of zebrafish embryos, including transparency, make the zebrafish a useful and important model for genetic studies. Early studies using this model focused mainly on cardiac development and congenital heart disease (Bournele and Beis, 2016). The first reports describing embryonic zebrafish as cardiomyopathy models were published in 2002, when positional cloning of two mutations, pickwick and silent heart, identified titin and tnnt2, both known causative genes for cardiomyopathy (Sehnert et al., 2002; Xu et al., 2002). Additional studies using zebrafish embryos helped in the discovery of other cardiomyopathy genes and new therapeutic strategies (Bakkers, 2011; Gut et al., 2017). However, the studies were limited because embryo studies cannot mimic the age dependent penetrance of cardiomyopathies that often end in overt heart failure in adulthood. In 2009, cardiac remodeling phenotypes were first reported in an anemia mutant zebrafish, owing to a band3 mutation, which underscored the feasibility of adult zebrafish as a simple vertebrate model for cardiomyopathy (Sun et al., 2009). Additional models have been generated for acquired cardiomyopathies, including those caused by doxorubicin, a common chemotherapy drug that can induce dose-dependent cardiotoxicity (Ding et al., 2011), and by phenylhydrazine hydrochloride, which can cause acute hemolysis and chronic anemic stress on the heart (Sun et al., 2009; Jopling et al., 2012). The advent of transgenic technology and genome editing technology further led to the generation of a panel of adult zebrafish models for inherited cardiomyopathies, which are the focus of the present review (Table 1).
Different from lower model organisms such as Drosophila, a zebrafish heart has conserved cardiomyocytes with those in humans, as well as intact endocardium and epicardium. Electrophysiology of a zebrafish heart has higher conservation than mouse with humans (Bakkers, 2011; Macrae and Peterson, 2015; Gut et al., 2017). Heart rate is around 100 beats per minute (bpm), which resembles to human better than the mouse. Because a zebrafish heart is only sized about 1–2 mm in diameter, novel phenotyping toolkits, such as high-frequency echocardiography, the Langendorff ex vivo system, ECG, and single-myofibril contractility analysis, have been developed to define progression of cardiac remodeling in zebrafish (Dvornikov et al., 2014; Lin et al., 2015; Koth et al., 2017; Merrifield et al., 2017; Wang et al., 2017; Stoyek et al., 2018; Zhang et al., 2018; Wakamatsu et al., 2019; Yan et al., 2020). Because these toolkits have already been comprehensively reviewed recently (Dvornikov et al., 2018; Lin et al., 2020), we only list some new techniques in Table 2. Partially because these new technologies are not readily accessible, many published adult zebrafish inherited cardiomyopathy models have not been sufficiently phenotyped to be categorized into a certain type of cardiomyopathy. Therefore, we decided to group these models based on the type of cardiomyopathy that is associated with its human ortholog, such as HCM, DCM, ACM, and cardiomyopathy genes associated with complex syndromes. We did not include the RCM group because no adult zebrafish model for a human RCM gene has been reported yet.
Known Inherited Cardiomyopathy Genes
HCM Genes
Because most causative genes for HCM encode sarcomeric proteins, HCM is also considered a disease of the sarcomere (Seidman and Seidman, 2011). Pathogenic sequence variants in MYH7 and myosin-binding protein C (MYBPC3) account for approximately 75% of all inherited cases of HCM (Burke et al., 2016). Patients harboring sequence variants in genes that lead to lysosomal storage disease, such as lysosomal-associated membrane protein 2 (LAMP2), also manifest HCM phenotypes (Fu et al., 2016). To date, adult zebrafish models for the following three HCM genes have been reported.
MYL3
Essential myosin light chain 3 (MYL3), encoding a ventricular/cardiac isoform myosin essential light chain, has been identified as a causative gene of familial HCM, accounting for up to 5% of HCM cases (Olson et al., 2002; Keren et al., 2008). Scheid et al. (2016) reported phenotypic characterization of a heterozygous lazy susan (lazm647) mutant, which harbors a nonsense mutation in myl3. Like pickwick and silent heart, lazm647 was originally identified as an embryonic lethal mutant from a large-scale mutagenesis screen (Stainier et al., 1996). Reduced cardiac contraction was noted in homozygous laz–/–mutants (Meder et al., 2009). By using non-invasive echocardiography and swimming exercise assays, Scheid et al. (2016) reported systolic dysfunction in adult heterozygous laz (laz±) zebrafish at a basal level, which became more severe upon physical stress, as reflected by increased sudden death. S195 phosphorylation of essential light chain is required for adaptation of cardiac function to augmented physical stress. Thus, heterozygous laz was the first inherited cardiomyopathy model reported in adult zebrafish. However, more detailed phenotyping of this model is needed to determine whether laz is a HCM model.
MYH6
In contrast to the MYH7 gene that encodes the ventricular/slow myosin heavy chain β isoform, MYH6 encodes the myosin heavy chain α isoform that is predominantly expressed in human cardiac atrium. Mutations in MYH6, although relatively rare, have been associated with both HCM and DCM (Schiaffino and Reggiani, 1996; Parker and Peckham, 2020). In a zebrafish heart, there are three major MYH homologs: atrial myosin heavy chain (amhc, also termed myh6), ventricular myosin heavy chain (vmhc), and ventricular myosin heavy chain-like (vmhcl) (Auman et al., 2007). While amhc specifically expresses in the atrium, the latter two genes express mostly in the ventricle (Shih et al., 2015). Sarantis et al. (2019) reported the characterization of the adult cardiac phenotype of weak atrium mutants that harbor frameshift mutations in amhc. They reported that atria remained hypoplastic and showed elastin deposition, whereas ventricles exhibited increased chamber size due to hyperplasia. Similar to laz, more detailed phenotyping of this model is needed to determine whether weak atrium exhibits HCM phenotypes.
LAMP2
Lysosome-associated membrane protein 2 (LAMP2) encodes a type I integral membrane protein that is localized to lysosomes and late endosomes. LAMP2 was initially identified as a causative gene for Danon disease, manifesting with peripheral skeletal myopathy, intellectual disability, hepatic involvement, and retinopathy. Later, mutations in LAMP2 were found in about 1% of patients with HCM (Danon et al., 1981; Maron et al., 2009). Using adult zebrafish as a vertebrate model, Dvornikov et al. (2019) introduced a truncational mutation into exon 2 of the lamp2 gene by using transcription activator-like effector nuclease (TALEN)–based genome editing technology. In a lamp2e2/e2 homozygous mutant at 10 months of age, there was increased density of the trabecular muscle, a thickened compact layer, fibrosis, and reduced ejection fraction at low flow that was ascribed to decreased end diastolic volume/body weight ratio. Also described were increased maximal isometric tension and accelerated actomyosin activation kinetics at the single-myofibril level, suggesting myofibrillar “hypercontractility.” Because many of these phenotypes are characteristic features of HCM in human patients and mammalian models, this study provided a starting point to define HCM-like phenotypic traits in adult zebrafish.
DCM Genes
To date, more than 50 genes have been linked to human DCM. They can be broadly categorized into genes encoding sarcomere, cytoskeletal, mitochondrial, desmosomal, nuclear membrane, and RNA-binding proteins (Yotti et al., 2019). The three DCM genes described below have been modeled in adult zebrafish.
TTN
Truncation mutations in the TTN truncating variants (TTNtv) are the most common causative genetic lesion for inherited DCM, presenting in 15 to 20% of cases (Herman et al., 2012; Schafer et al., 2017). Allelic heterogeneity has been noted in TTNtv DCM. Whereas TTNtv in the C-terminal A-band region are pathogenic, TTNtv in the N-terminal Z-disk region are likely benign. In zebrafish, 2 TTN homologs are located in tandem on chromosome 14, termed ttn.1 and ttn.2 (Seeley et al., 2007). Huttner et al. (2018) generated zebrafish mutants harboring A-band ttn.2 truncations that mimicked mutations found in two unrelated human probands with familial DCM. Whereas the homozygous ttn.2 mutants exhibited severe cardiac dysmorphogenesis and premature death, heterozygous mutants (ttn.2tv/+) survived into adulthood and spontaneously developed DCM-like phenotypes, including reduced baseline ventricular systolic function, prolonged isovolumic relaxation, and increased diastolic passive stiffness in the absence of myocardial fibrosis. Thus, both systolic and diastolic dysfunction were noted in this zebrafish model of TTNtv cardiomyopathy.
BAG3
The BCL2-associated athanogene 3 (BAG3) gene encodes a co-chaperone protein that regulates unfolded protein aggregation and autophagy (Meriin et al., 2018). Mutations in the BAG3 gene account for 2 to 7% of DCM cases, representing a common DCM causative gene (Norton et al., 2011; Franaszczyk et al., 2014; Dominguez et al., 2018). We recently generated zebrafish bag3 gene knockout mutants by introducing frameshift mutations through TALEN-based genome editing technology (Ding et al., 2019). We characterized phenotypic traits of this model thoroughly by combining emerging technologies of high-frequency echocardiography, ex vivo heart pump function assays, and biophysical assays at the single-myofibril level. In bag3e2/e2 homozygous mutants at 6 months of age, we detected ventricular chamber enlargement, reduced trabecular muscle density, and reduced ejection fraction ascribed to increased end-systolic volume/body weight that resembled the eccentric hypertrophy characteristic of DCM in mammals (Merlo et al., 2018). We also detected decreased maximal isometric tension and reduced activation of myofibril kinetics at the single-myofibril level, suggesting “hypocontractility.” Because many phenotypic traits are characteristic features of DCM in human patients and mammalian models, this study provides a starting point to define DCM-like phenotypic traits in adult zebrafish models.
GATAD1
The GATA zinc finger domain containing 1 (GATAD1) gene encodes a transcription factor that is more highly expressed in women than men with DCM (Heidecker et al., 2010). A homozygous recessive missense mutation, S102P, was discovered by locus mapping and whole exome sequencing in a family with adult-onset DCM (Theis et al., 2011). Yang et al. (2016) generated a gatad1 knockout zebrafish mutant by TALEN technology as well as a transgenic fish line overexpressing the human GATAD1-S102P variant in cardiomyocytes. While gatad1 knockout fish exhibited a mild heart failure–like phenotype after stress, the cardiomyocyte-specific overexpressing GATAD1-S102P transgenic fish have increased mortality, concurrent with a substantially enlarged ventricular chamber and decreased papillary muscle density, suggesting cardiac remodeling.
Cardiac phenotypes for many existing cardiomyopathy models have not been characterized in detail, partially because phenotyping tools are not readily available. Two of the most comprehensively characterized cardiomyopathy models in adult zebrafish are lamp2e2/e2 and bag3e2/e2 mutants. Common cardiomyopathy traits have been identified with this model, such as reduced ejection fraction, reduced exercise capacity, and aberrant expression of molecular markers, as have unique phenotypic traits that can discern DCM from HCM-like phenotypes. First, trabecular muscle density is reduced in the bag3 knockout zebrafish, whereas it is increased in the lamp2 knockout model. Similarly, Abdul-Wajid et al. (2018) recently used the percentage of ventricle covered in trabeculation as an index to determine HCM phenotype in the jag2b mutant adult zebrafish. Whether trabecular muscle density can indeed serve as a surrogate index of ventricular wall thickness in mammals, a key phenotypic trait that discerns DCM from HCM, remains to be established. Second, the lamp2 knockout model, but not the bag3 knockout model, had a rounded heart shape. Third, at the single-myofibril level, myofibrils from the bag3 knockout model manifested hypocontractility, but myofibrils from the lamp2 knockout model manifested hypercontractility. This phenomenon is consistent with that seen via biophysical analysis in mammalian DCM and HCM models (Davis et al., 2016; Spudich et al., 2016). In the Table 3, we summarized major phenotypic traits of human cardiomyopathies and compared with features and surrogate indices in corresponding adult zebrafish models. More inherited cardiomyopathy models need to be developed and comprehensively phenotyped, which will better define phenotypic traits among cardiomyopathies of different genetic causes.
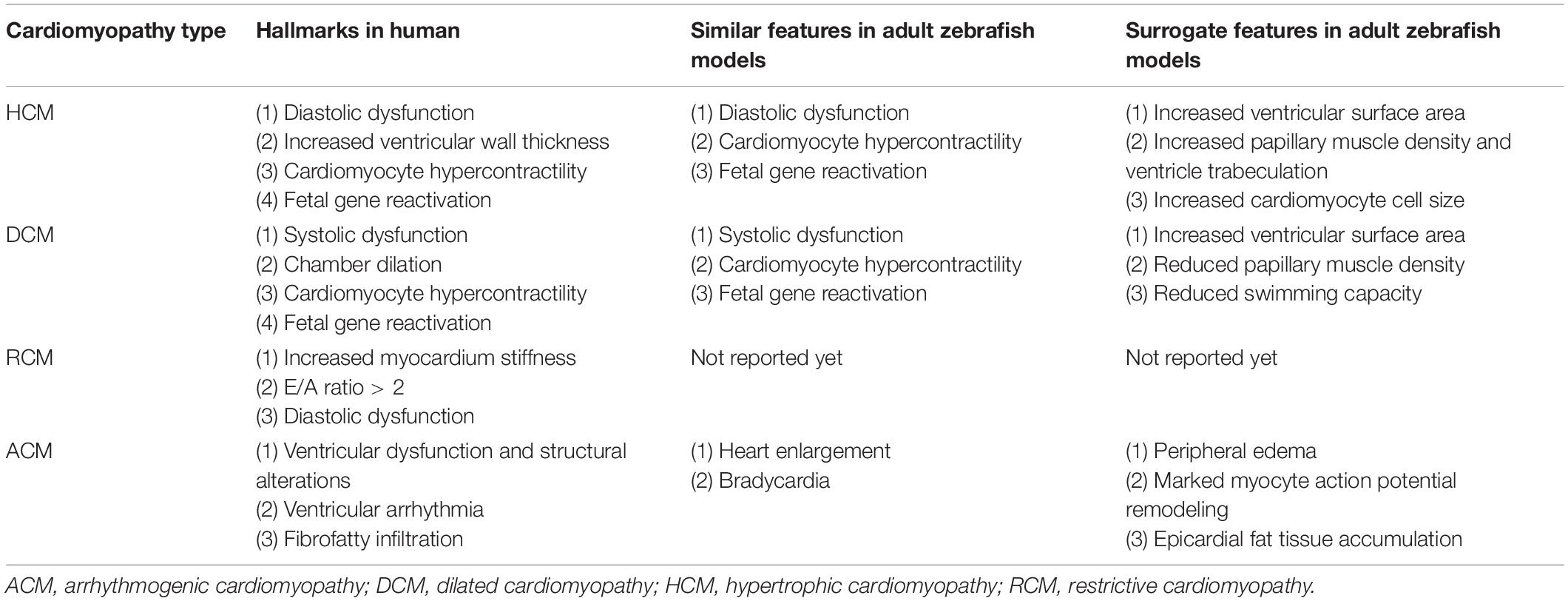
Table 3. Phenotypic characteristics of inherited cardiomyopathies between human and adult zebrafish models.
ACM Genes
To date, more than 15 genes have been linked to ACM, of which five genes encode desmosomal proteins located in the intercalated disk, including desmoplakin (DSP), desmoglein-2 (DSG2), desmocollin-2 (DSC2), plakophilin-2 (PKP2), and plakoglobin (JUP) (McKoy et al., 2000; Rampazzo et al., 2002; Gerull et al., 2004; Pilichou et al., 2006; Syrris et al., 2006; Vimalanathan et al., 2018; Roberts et al., 2019). Mutations in PKP2, DSP, and DSG2 are the most common causes, accounting for about 45% of all ACM probands, and all other genes are relatively rare (Padron-Barthe et al., 2017). Thus far, JUP and integrin-linked kinase (ILK) are the two human ACM genes that have been modeled in adult zebrafish.
JUP
Plakoglobin encodes plakoglobin, a member of the catenin protein family. A homozygous 2-base pair deletion (2057del2) in JUP results in premature truncation of plakoglobin protein and was the first sequence variant linked to ACM (McKoy et al., 2000). Asimaki et al. (2014a) generated a cardiomyocyte-specific transgenic fish that harbored human JUP cDNA containing the 2057del2 mutation. The transgenic mutant fish survived to adulthood and exhibited cardiac phenotypes, including an enlarged chamber size, wall thinning, bradycardia, and increased mortality rate. There were marked abnormalities in action potentials and ionic currents in ventricular myocytes, which likely contributed to arrhythmogenesis in this model. Asimaki et al., further conducted a high-throughput screening to search for bioactive compounds that attenuated disease phenotypes. They successfully identified SB216763, an activator of the canonical Wnt/β-catenin signaling pathway, which suppresses the ACM-like phenotypes.
ILK
Integrin-linked kinase encodes a serine/threonine protein kinase which participates in cell matrix interactions and induction of biomechanical signals for cytoskeleton remodeling, cell survival, differentiation, and proliferation (Wu, 2001). Brodehl et al. (2019) characterized phenotypes of transgenic fish overexpression of human ILK variants p.H77Y and p.P70L identified from cohorts with arrhythmogenic cardiomyopathy. Because fewer than 20% of p.P70L and p.H77Y zebrafish could survive after 15 days, the authors mainly performed phenotypic characterization at the embryonic stage such as 3 days post-fertilization. Significant reduction of fractional shortening (FS) and mild reduction of action potential were noted. Adult histology and cardiac morphology studies showed epicardial fat tissue accumulation in the transgenic fish hearts, replicating the phenotypic trait of fibrofatty infiltration in human ACM.
Cardiomyopathy Genes With Complex Syndromes
Because cardiomyopathy is a highly heterogeneous disease, overlapping phenotypes often coexist. For example, ventricular arrhythmias occur in up to 40% of patients diagnosed with DCM (Spezzacatene et al., 2015). Several adult zebrafish models for other inherited cardiac syndromes concurrent with cardiomyopathy are described below.
SCN5A
The SCN5A gene encodes the alpha subunit of the cardiac sodium channel, NaV1.5, which has a central role in controlling cardiac excitability. Variants in SCN5A have been linked to a spectrum of different human cardiac arrhythmia syndromes (Wilde and Amin, 2018). For example, a missense mutation, D1275N, in the SCN5A gene has been associated with various cardiac phenotypes, including atrial standstill and enlargement, sinus node dysfunction, tachyarrhythmias, conduction disease, and DCM (McNair et al., 2004). Huttner et al. (2013) generated a transgenic zebrafish line harboring an SCN5A-D1275N mutation Tg(SCN5A-D1275N) and then characterized the cardiac phenotypes by using video microscopy and electrocardiography (ECG). They found that the adult zebrafish had bradycardia, conduction system abnormalities, and premature death. Later, Yan et al. (2020) used an iWorx-based ECG system (iWorx) to study episodes of sinus arrest in Tg(SCN5A-D1275N) as an inherited model for sick sinus syndrome. Interestingly, they also noted sinus arrest episodes in a small subpopulation of wildtype fish. This subpopulation of wildtype fish manifests unique phenotypic traits of sick sinus syndrome, such as increased QRS/P ratio and chronotropic incompetence, that are different from the Tg(SCN5A-D1275N) model.
KCNJ8
The KCNJ8 gene encodes the pore-forming subunit of adenosine triphosphate (ATP)–sensitive potassium channels (KATP). The V65M variant in the KCNJ8 gene was associated with Cantú syndrome, a rare genetic condition characterized by congenital hypertrichosis and cardiovascular abnormalities including an enlarged, hypercontractile heart (Brownstein et al., 2013; Cooper et al., 2014). Tessadori et al. (2018) reported a KCNJ8 V65M knock-in model in adult zebrafish. The heterozygous knock-in zebrafish had substantially enlarged ventricles and enhanced cardiac output and contractile function, which was similar to the characteristic signs in patients with Cantú syndrome. Through confirming the causality of the KCNJ8 V65M mutation, this study provided the feasibility of modeling patient-specific mutations in adult zebrafish via knock-in technology.
PITX2
PITX2, located in locus 4q25, encodes a transcription factor that is critical in establishing the left-right axis and in the asymmetrical development of internal organs and, thus, confers major susceptibility to atrial fibrillation. Collins et al. (2018, 2019) generated a loss-of-function allele of pitx2c using site-specific transcription activator-like effector nucleases (TALEN) in zebrafish and assessed its adult cardiac phenotypes. By using bright-field mode imaging, pulsed-wave Doppler imaging of blood flow, and ECG, the authors detected cardiac dysfunction, arrhythmias, and atrial conduction defects in homozygous pitx2c mutant fish and a subset of heterozygous pitx2c mutant fish. They further showed that changes in sarcomeric and metabolic gene expression and function preceded the onset of cardiac arrhythmia in the pitx2c mutant fish, suggesting that developmental perturbations predispose to functional defects in the adult heart. This is the first attempt to utilize adult zebrafish to model atrial fibrillation–like diseases of humans.
New Inherited Cardiomyopathy Genes
More than 100 genes have been linked to cardiomyopathies, but these genes can only explain about 75% of HCM, 50% of DCM, 40% of RCM, and 60% of ACM (Groeneweg et al., 2015; Harakalova et al., 2015; Gallego-Delgado et al., 2016; Makavos et al., 2019; Rosenbaum et al., 2020). More genetic factors need to be discovered. Historically, most causative genes were identified from linkage analysis of cardiomyopathy patient cohorts. More recently, NGS has become the main technology for discovery of novel genetic loci associated with cardiomyopathy (Norton et al., 2012; Ho et al., 2015; Shah et al., 2020). As a supplement to these human genetics-based approaches, Ding et al. (2013, 2016) developed a novel mutagenesis screen-based forward genetic approach in adult zebrafish for identifying genetic factors of cardiomyopathy. DNAJB6 and SORBS2 were identified as new candidate cardiomyopathy susceptibility genes, which were supported by evidence from sequencing data from human patients.
DNAJB6
The DNAJB6 gene encodes a member of the J protein family that functions as a molecular chaperone to facilitate protein folding and protein quality control (Hageman et al., 2010; Kampinga and Craig, 2010). In mammals, there are two DNAJB6 isoforms that result from alternative splicing of the same gene. The short DNAJB6(S) isoform is encoded by cDNA that includes the first 6 exons and manifests a cytoplasmic expression pattern. In contrast, the longer DNAJB6(L) isoform is encoded by cDNA that includes an additional two exons at the 3′-end of the gene and manifests a nuclei and/or endoplasmic reticulum-specific expression pattern (Hanai and Mashima, 2003; Ding et al., 2016). Mutations in DNAJB6(S) have been previously linked to limb-girdle muscular dystrophy type 1D (LGMD1D) in humans (Sarparanta et al., 2012). Different from the short DNAJB6(S) isoform, DNAJB6(L) is a cardiac-enriched isoform that is primarily disrupted in GBT411. Whereas the heterozygous GBT411 exaggerates DIC phenotypes, homozygous GBT411 mutant exhibits cardiac chamber enlargement and cardiac muscle disarray phenotypes at 1 year of age. The latter observation prompted Ding et al to scan human cardiomyopathy patients, leading to identification of several rare variants at the C-terminus of the DNAJB6(L) gene. Pathogenicity of the p.S316W variant has been confirmed by generation of a transgenic fish line harboring the p.S316W variant, which exaggerates DIC. In summary, the DNAJB6(L) was the first cardiomyopathy gene discovered from a mutagenesis screen in adult zebrafish.
SORBS2
SORBS2 is an intercalated disk protein that expresses predominantly in cardiac muscle. Prompted by the modifying effects of GBT002 on DIC, Ding et al. (2020) studied cardiac phenotypes in a Sorbs2 knockout mouse. Whereas heterozygous Sorbs2± exerts deleterious modifying effects on DIC, homozygous Sorbs2–/– manifests ACM-like phenotypes, including enlarged right ventricle, arrhythmia, and fibrosis, yielding a new ACM model. Furthermore, five rare SORBS2 variants were identified in a cohort of 59 patients with ACM, among which two splice variants were classified as likely to be pathogenic. Although more human genetic evidence from larger cohorts is needed, these data suggested SORBS2 was a candidate gene for ACM susceptibility.
Use of the Adult Zebrafish Model for Cardiomyopathy Research
Since the first report of cardiac remodeling in an adult zebrafish heart, (Sun et al., 2009) the adult zebrafish has emerged as an alternate vertebrate model for studying cardiomyopathies. The development of clustered regularly interspaced short palindromic repeats (CRISPR) Cas9 technology has revolutionized precise genome editing, making for ready generation of knockout, knock-in, and/or transgenic lines harboring a particular sequence variant (Shah et al., 2015; Cornet et al., 2018; Wu et al., 2018). The Table 1 shows the various current zebrafish models for inherited cardiomyopathies. Many phenotyping toolkits have been developed for the adult zebrafish heart (Table 2), which enable differentiating causative cardiomyopathy phenotypes. Below we list three research fields that may benefit from integrating the adult zebrafish model.
Genotype-Based Therapeutics for Inherited Cardiomyopathies
To implement genotype-based precision medicine, it is desirable to systematically generate models for each of those cardiomyopathy genes and then to assess and prioritize candidate therapeutic pathways. While the induced pluripotent stem cell (iPSC) might be a more appropriate in vitro model for drug screening, this type of research in the in vivo animal systems such as rodent models can be prohibitively expensive. The efficient zebrafish model shall accelerate this research direction. Besides the existing panel of inherited cardiomyopathy models in adult zebrafish (Table 1), more models will likely be generated in the upcoming decade. Shih et al. (2015) systematically searched zebrafish homologs of 51 DCM genes and identified corresponding homologs for 49 genes, which shall contribute to this research direction.
Among well-known therapeutic pathways, some broadly target various forms of cardiomyopathy, whereas others target cardiomyopathies with a known specific cause. For example, a series of genetic studies uncovered the therapeutic effects of inhibiting the mammalian target of rapamycin (mTOR) in anemia-induced cardiomyopathy, DIC, a bag3 knockout cardiomyopathy model, and a lamp2 cardiomyopathy knockout model (Ding et al., 2011, 2016, 2019; Dvornikov et al., 2019). Possibly, dysregulated mTOR signaling is a common pathologic event among cardiomyopathies of different etiology, as suggested by other reports of studies in mammalian cardiomyopathy models, and mTOR inhibition is a common therapeutic strategy (McMullen et al., 2004; Marin et al., 2011; Ramos et al., 2012; Sciarretta et al., 2018). In the desmoplakin-based ACM model, canonical Wnt/beta-catenin signaling was reduced. Activation of Wnt signaling through the administration of a GSK3b inhibitor, SB216763, reversed the ACM disease phenotype in ACM models including the zebrafish model (Asimaki et al., 2014b; Chelko et al., 2019). The roles of Wnt/beta-catenin in other adult zebrafish models of cardiomyopathies remain to be investigated. It is expected that more research on genotype-based therapeutics in zebrafish will inform the development of precision medicine for each type of inherited cardiomyopathy.
Assessing Sequence Variants to Find Novel Cardiomyopathy Genes
To advance genotype-based precision medicine, the remaining unknown causative cardiomyopathy genes need to be identified. With the rapid advent of NGS techniques, human genetic studies of cardiomyopathy cohorts are identifying many new candidate genes. Moreover, results from human genomic studies are identifying large numbers of sequence variants from known causative genes; however, most identified sequence variants have not been characterized, making them of variants of unknown significance (VUS). The zebrafish will likely be used increasingly as an in vivo model for testing candidate genes and those VUS because of its smaller size, lower cost, and higher throughput than rodent models. Because many sequence variants are missense mutations, loss-of-function knockout mutants could result in negative conclusions or provide misleading information. As shown by recent studies of sequence variants from GATAD1, KCNJ8, and DNAJB6(L) genes (Ding et al., 2016; Yang et al., 2016; Tessadori et al., 2018), knock-in or transgenic technology can be used to precisely model missense mutations.
Modifier Screening for Isolating Novel Cardiomyopathy Genes and Therapeutic Targets
In addition to validating candidate genes identified in human genetic studies, genetic studies in adult zebrafish can uncover new susceptibility genes. A forward mutagenesis screening approach has been shown to be effective for identifying modifier genes for DIC (Ding et al., 2016). Interestingly, all three deleterious mutants identified from that approach, ANO5, DNAJB6(L), and SORBS2, were either causative or susceptibility genes for cardiomyopathies (Wahbi et al., 2013; Ding et al., 2020). Indeed, the border between modifier genes and causative genes could be blurred-it has been increasingly recognized that multiple genetic lesions might coexist in cardiomyopathy patients and contribute synergistically to the severity of pathogenesis (Ingles et al., 2005; Kelly and Semsarian, 2009; Gifford et al., 2019).
From a modifier screen, two types of modifier mutants could be identified: those that exerted deleterious modifying effects and those that exerted salutary modifying effects. Whereas the former type identifies susceptibility genes, the latter type could suggest therapeutic target genes. As shown by studies of GBT419/rxraa, Ma et al. (2020) established retinoid X receptor alpha a (rxraa) as a new therapeutic target gene for DIC and reported the underlying mechanism. Through leveraging the integrated loxP sites in the insertional vector, they uncovered a spatiotemporally predominant mechanism of rxraa-based therapy, i.e., that endothelial-specific rxraa activation, but not myocardial- or epicardial-rxraa activation, conferred therapeutic effects on DIC (Ma et al., 2020). Besides identifying therapeutic target genes via harnessing salutary modifiers, mechanistic studies of deleterious modifiers could also identify a particular way to manipulate the modifier gene to exert therapeutic effects. Ding et al. (2016) conducted detailed studies of GBT411/dnajb6b(L) and found that while dnajb6b(L) loss-of-function exerts deleterious effects, overexpression of dnajb6b(L) in cardiomyocytes exerts therapeutic effects on DIC. The therapeutic effects of dnajb6b(L) overexpression were confirmed in a mouse DIC model by using an adeno-associated virus (AAV) 9–based gene delivery system.
Limitations
Although adult zebrafish can be a model for cardiomyopathy studies, this lower vertebrate model is not without limitations. Despite a much more economic model than mouse, an adult zebrafish reaches sexual maturity in 3 months, which is actually longer than mouse. Different from the 4-chambered heart structure in humans, a zebrafish heart consists of two chambers. Its large atrium has a more active role during the cardiac cycle than atria of mammals. On Doppler echocardiography, the ratio between the E-wave and the A-wave in an adult zebrafish is less than one, suggestive of higher active filling (A-wave) than passive filling (E-wave) (Lee et al., 2014). A zebrafish heart does not have a clearly defined ventricular wall. Instead, it consists of highly trabeculated ventricular myocardium surrounded by a small compact layer of cardiomyocytes (Hu et al., 2000). Therefore, differences in hemodynamic properties of zebrafish vs. human myocardium must be considered in using this model. Throughout their lifecycle, zebrafish maintain considerable capacity for generating cardiomyocytes, whereas mammals lose this capability within a few days of birth (Poss et al., 2002; Doppler et al., 2017). This contribution of cardiomyocyte proliferation to cardiac remodeling needs to be considered (Sun et al., 2009).
Although about 70% of human genes have at least one obvious zebrafish ortholog, the remaining 30% of human genes do not have an obvious zebrafish ortholog, making it impossible to study these genes in zebrafish (Howe et al., 2013). Zebrafish have also undergone an additional whole-genome duplication (i.e., teleost-specific genome duplication); therefore, about 15% of human genes are associated with more than one zebrafish gene (average, 2.3) (Meyer and Schartl, 1999; Howe et al., 2013). However, cardiomyopathy genes might have a higher degree of conservation, as indicated by human DCM genes, 96% of which have a zebrafish ortholog (Shih et al., 2015). Shih et al. (2015) were able to prioritize one of many homologs for most DCM genes for further genetic manipulation based on their relative expression in the embryonic heart, adult heart, and adult somites.
Conclusion
Despite these limitations, in the last decade, adult zebrafish have emerged as an important vertebrate model for studying inherited cardiomyopathies. The use of zebrafish is not limited to modeling known genotype-based cardiomyopathies for therapeutic development and has already been extended to discovering new susceptibility genes and therapeutic target genes (Figure 1). In the future, this small tropical fish holds the promise of contributing substantially to implementing genotype-based precision medicine for inherited cardiomyopathies.
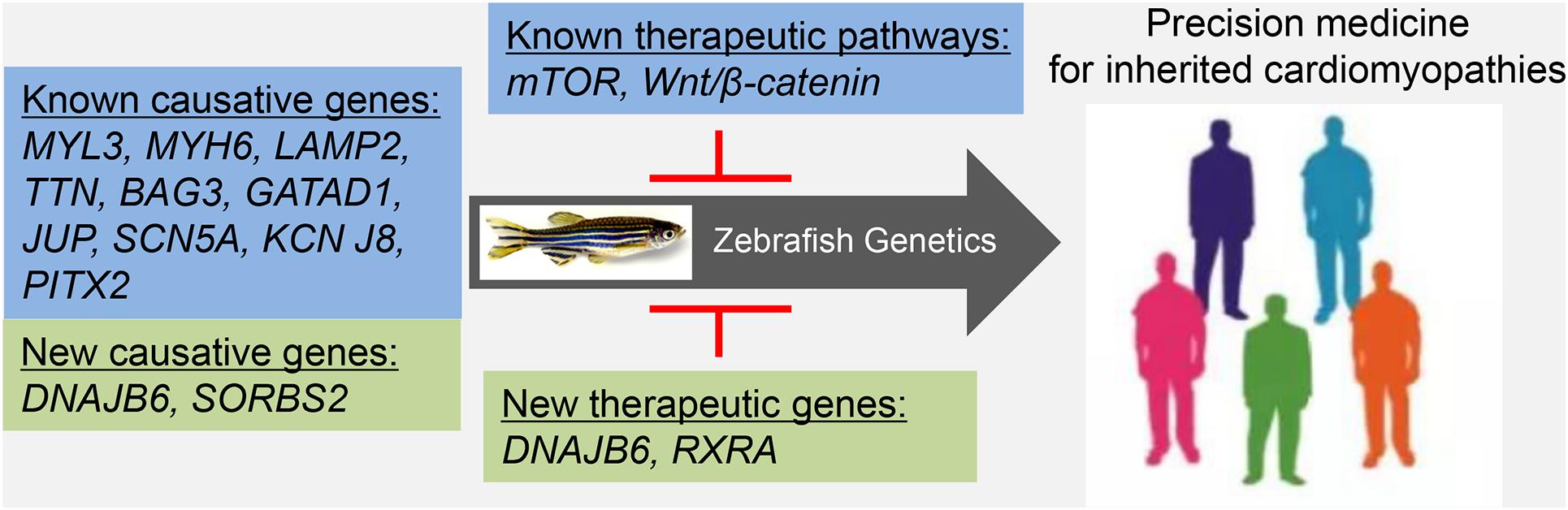
Figure 1. Future perspective. Adult zebrafish can be used to generate genotype-specific cardiomyopathy models, to identify genotype-specific therapeutic pathways, to discover new causative genes, and to discover new therapeutic genes. Discoveries from this efficient vertebrate model might inform the development of precision medicine for inherited cardiomyopathies.
Author Contributions
YD and XX designed the review outline and wrote the review. YD and HB did the literature search, data extraction, and interpretation. All authors contributed and approved the final version of the manuscript.
Funding
This work was supported by grants from National Institutes of Health (RO1-HL107304 and RO1-HL081753) and the Mayo Foundation to XX.
Conflict of Interest
The authors declare that the research was conducted in the absence of any commercial or financial relationships that could be construed as a potential conflict of interest.
References
Abdul-Wajid, S., Demarest, B. L., and Yost, H. J. (2018). Loss of embryonic neural crest derived cardiomyocytes causes adult onset hypertrophic cardiomyopathy in zebrafish. Nat. Commun. 9:4603.
Ashrafian, H., Mckenna, W. J., and Watkins, H. (2011). Disease pathways and novel therapeutic targets in hypertrophic cardiomyopathy. Circ. Res. 109, 86–96. doi: 10.1161/circresaha.111.242974
Asimaki, A., Kapoor, S., Plovie, E., Karin Arndt, A., Adams, E., Liu, Z., et al. (2014a). Identification of a new modulator of the intercalated disc in a zebrafish model of arrhythmogenic cardiomyopathy. Sci. Transl. Med. 6:240ra274.
Asimaki, A., Kleber, A. G., Macrae, C. A., and Saffitz, J. E. (2014b). Arrhythmogenic cardiomyopathy – new insights into disease mechanisms and drug discovery. Prog. Pediatr. Cardiol. 37, 3–7. doi: 10.1016/j.ppedcard.2014.10.001
Auman, H. J., Coleman, H., Riley, H. E., Olale, F., Tsai, H. J., and Yelon, D. (2007). Functional modulation of cardiac form through regionally confined cell shape changes. PLoS Biol. 5:e53. doi: 10.1371/journal.pbio.0050053
Authors/Task Force members, Elliott, P. M., Anastasakis, A., Borger, M. A., Borggrefe, M., Cecchi, F., et al. (2014). 2014 ESC guidelines on diagnosis and management of hypertrophic cardiomyopathy: the task force for the diagnosis and management of hypertrophic cardiomyopathy of the European Society of Cardiology (ESC). Eur. Heart J. 35, 2733–2779. doi: 10.1093/eurheartj/ehu284
Bakkers, J. (2011). Zebrafish as a model to study cardiac development and human cardiac disease. Cardiovasc. Res. 91, 279–288. doi: 10.1093/cvr/cvr098
Bournele, D., and Beis, D. (2016). Zebrafish models of cardiovascular disease. Heart Fail. Rev. 21, 803–813. doi: 10.1007/s10741-016-9579-y
Bozkurt, B., Colvin, M., Cook, J., Cooper, L. T., Deswal, A., Fonarow, G. C., et al. (2016). Current diagnostic and treatment strategies for specific dilated cardiomyopathies: a scientific statement from the American Heart Association. Circulation 134, e579–e646.
Brodehl, A., Rezazadeh, S., Williams, T., Munsie, N. M., Liedtke, D., Oh, T., et al. (2019). Mutations in ILK, encoding integrin-linked kinase, are associated with arrhythmogenic cardiomyopathy. Transl. Res. 208, 15–29. doi: 10.1016/j.trsl.2019.02.004
Brownstein, C. A., Towne, M. C., Luquette, L. J., Harris, D. J., Marinakis, N. S., Meinecke, P., et al. (2013). Mutation of KCNJ8 in a patient with Cantu syndrome with unique vascular abnormalities – support for the role of K(ATP) channels in this condition. Eur. J. Med. Genet. 56, 678–682. doi: 10.1016/j.ejmg.2013.09.009
Burke, M. A., Cook, S. A., Seidman, J. G., and Seidman, C. E. (2016). Clinical and mechanistic insights into the genetics of cardiomyopathy. J. Am. Coll. Cardiol. 68, 2871–2886. doi: 10.1016/j.jacc.2016.08.079
Chelko, S. P., Asimaki, A., Lowenthal, J., Bueno-Beti, C., Bedja, D., Scalco, A., et al. (2019). Therapeutic modulation of the immune response in arrhythmogenic cardiomyopathy. Circulation 140, 1491–1505. doi: 10.1161/circulationaha.119.040676
Collins, M. M., Ahlberg, G., Hansen, C. V., Guenther, S., Marin-Juez, R., Sokol, A. M., et al. (2019). Early sarcomere and metabolic defects in a zebrafish pitx2c cardiac arrhythmia model. Proc. Natl. Acad. Sci. U.S.A. 116, 24115–24121. doi: 10.1073/pnas.1913905116
Collins, M. M., Maischein, H. M., Dufourcq, P., Charpentier, M., Blader, P., and Stainier, D. Y. (2018). Pitx2c orchestrates embryonic axis extension via mesendodermal cell migration. Elife 7:e34880.
Cooper, P. E., Reutter, H., Woelfle, J., Engels, H., Grange, D. K., Van Haaften, G., et al. (2014). Cantu syndrome resulting from activating mutation in the KCNJ8 gene. Hum. Mutat. 35, 809–813. doi: 10.1002/humu.22555
Cornet, C., Di Donato, V., and Terriente, J. (2018). Combining zebrafish and CRISPR/cas9: toward a more efficient drug discovery pipeline. Front. Pharmacol. 9:703. doi: 10.3389/fphar.2018.00703
Danon, M. J., Oh, S. J., Dimauro, S., Manaligod, J. R., Eastwood, A., Naidu, S., et al. (1981). Lysosomal glycogen storage disease with normal acid maltase. Neurology 31, 51–57. doi: 10.1212/wnl.31.1.51
Davis, J., Davis, L. C., Correll, R. N., Makarewich, C. A., Schwanekamp, J. A., Moussavi-Harami, F., et al. (2016). A tension-based model distinguishes hypertrophic versus dilated cardiomyopathy. Cell 165, 1147–1159. doi: 10.1016/j.cell.2016.04.002
Ding, Y., Dvornikov, A. V., Ma, X., Zhang, H., Wang, Y., Lowerison, M., et al. (2019). Haploinsufficiency of mechanistic target of rapamycin ameliorates bag3 cardiomyopathy in adult zebrafish. Dis. Model Mech. 12:dmm040154.
Ding, Y., Liu, W., Deng, Y., Jomok, B., Yang, J., Huang, W., et al. (2013). Trapping cardiac recessive mutants via expression-based insertional mutagenesis screening. Circ. Res. 112, 606–617. doi: 10.1161/circresaha.112.300603
Ding, Y., Long, P. A., Bos, J. M., Shih, Y. H., Ma, X., Sundsbak, R. S., et al. (2016). A modifier screen identifies DNAJB6 as a cardiomyopathy susceptibility gene. JCI Insight 1:e94086.
Ding, Y., Sun, X., Huang, W., Hoage, T., Redfield, M., Kushwaha, S., et al. (2011). Haploinsufficiency of target of rapamycin attenuates cardiomyopathies in adult zebrafish. Circ. Res. 109, 658–669. doi: 10.1161/circresaha.111.248260
Ding, Y., Yang, J., Chen, P., Lu, T., Jiao, K., Tester, D. J., et al. (2020). Knockout of SORBS2 protein disrupts the structural integrity of intercalated disc and manifests features of arrhythmogenic cardiomyopathy. J. Am. Heart Assoc. 9:e017055.
Dominguez, F., Cuenca, S., Bilinska, Z., Toro, R., Villard, E., Barriales-Villa, R., et al. (2018). Dilated cardiomyopathy due to BLC2-associated athanogene 3 (BAG3) mutations. J. Am. Coll. Cardiol. 72, 2471–2481.
Doppler, S. A., Deutsch, M. A., Serpooshan, V., Li, G., Dzilic, E., Lange, R., et al. (2017). Mammalian heart regeneration: the race to the finish line. Circ. Res. 120, 630–632. doi: 10.1161/circresaha.116.310051
Dvornikov, A. V., De Tombe, P. P., and Xu, X. (2018). Phenotyping cardiomyopathy in adult zebrafish. Prog. Biophys. Mol. Biol. 138, 116–125. doi: 10.1016/j.pbiomolbio.2018.05.013
Dvornikov, A. V., Dewan, S., Alekhina, O. V., Pickett, F. B., and De Tombe, P. P. (2014). Novel approaches to determine contractile function of the isolated adult zebrafish ventricular cardiac myocyte. J. Physiol. 592, 1949–1956. doi: 10.1113/jphysiol.2014.270678
Dvornikov, A. V., Wang, M., Yang, J., Zhu, P., Le, T., Lin, X., et al. (2019). Phenotyping an adult zebrafish lamp2 cardiomyopathy model identifies mTOR inhibition as a candidate therapy. J. Mol. Cell. Cardiol. 133, 199–208. doi: 10.1016/j.yjmcc.2019.06.013
Fatkin, D., Huttner, I. G., Kovacic, J. C., Seidman, J. G., and Seidman, C. E. (2019). Precision medicine in the management of dilated cardiomyopathy: JACC state-of-the-art review. J. Am. Coll. Cardiol. 74, 2921–2938.
Franaszczyk, M., Bilinska, Z. T., Sobieszczanska-Malek, M., Michalak, E., Sleszycka, J., Sioma, A., et al. (2014). The BAG3 gene variants in polish patients with dilated cardiomyopathy: four novel mutations and a genotype-phenotype correlation. J. Transl. Med. 12:192. doi: 10.1186/1479-5876-12-192
Fu, L., Luo, S., Cai, S., Hong, W., Guo, Y., Wu, J., et al. (2016). Identification of LAMP2 mutations in early-onset danon disease with hypertrophic cardiomyopathy by targeted next-generation sequencing. Am. J. Cardiol. 118, 888–894. doi: 10.1016/j.amjcard.2016.06.037
Gallego-Delgado, M., Delgado, J. F., Brossa-Loidi, V., Palomo, J., Marzoa-Rivas, R., Perez-Villa, F., et al. (2016). Idiopathic restrictive cardiomyopathy is primarily a genetic disease. J. Am. Coll. Cardiol. 67, 3021–3023. doi: 10.1016/j.jacc.2016.04.024
Geisterfer-Lowrance, A. A., Kass, S., Tanigawa, G., Vosberg, H. P., Mckenna, W., Seidman, C. E., et al. (1990). A molecular basis for familial hypertrophic cardiomyopathy: a beta cardiac myosin heavy chain gene missense mutation. Cell 62, 999–1006. doi: 10.1016/0092-8674(90)90274-i
Gersh, B. J., Maron, B. J., Bonow, R. O., Dearani, J. A., Fifer, M. A., and Link, M. S. (2011). 2011 ACCF/AHA guideline for the diagnosis and treatment of hypertrophic cardiomyopathy: executive summary: a report of the American College of Cardiology Foundation/American Heart Association task force on practice guidelines. Circulation 124, 2761–2796. doi: 10.1161/cir.0b013e318223e230
Gerull, B., Heuser, A., Wichter, T., Paul, M., Basson, C. T., Mcdermott, D. A., et al. (2004). Mutations in the desmosomal protein plakophilin-2 are common in arrhythmogenic right ventricular cardiomyopathy. Nat. Genet. 36, 1162–1164. doi: 10.1038/ng1461
Gifford, C. A., Ranade, S. S., Samarakoon, R., Salunga, H. T., De Soysa, T. Y., Huang, Y., et al. (2019). Oligogenic inheritance of a human heart disease involving a genetic modifier. Science 364, 865–870. doi: 10.1126/science.aat5056
Groeneweg, J. A., Bhonsale, A., James, C. A., Te Riele, A. S., Dooijes, D., Tichnell, C., et al. (2015). Clinical presentation, long-term follow-up, and outcomes of 1001 arrhythmogenic right ventricular dysplasia/cardiomyopathy patients and family members. Circ. Cardiovasc. Genet. 8, 437–446.
Gut, P., Reischauer, S., Stainier, D. Y. R., and Arnaout, R. (2017). Little fish, big data: zebrafish as a model for cardiovascular and metabolic disease. Physiol. Rev. 97, 889–938. doi: 10.1152/physrev.00038.2016
Hageman, J., Rujano, M. A., Van Waarde, M. A., Kakkar, V., Dirks, R. P., Govorukhina, N., et al. (2010). A DNAJB chaperone subfamily with HDAC-dependent activities suppresses toxic protein aggregation. Mol. Cell 37, 355–369. doi: 10.1016/j.molcel.2010.01.001
Hanai, R., and Mashima, K. (2003). Characterization of two isoforms of a human DnaJ homologue, HSJ2. Mol. Biol. Rep. 30, 149–153.
Harakalova, M., Kummeling, G., Sammani, A., Linschoten, M., Baas, A. F., Van Der Smagt, J., et al. (2015). A systematic analysis of genetic dilated cardiomyopathy reveals numerous ubiquitously expressed and muscle-specific genes. Eur. J. Heart Fail. 17, 484–493. doi: 10.1002/ejhf.255
Heidecker, B., Lamirault, G., Kasper, E. K., Wittstein, I. S., Champion, H. C., Breton, E., et al. (2010). The gene expression profile of patients with new-onset heart failure reveals important gender-specific differences. Eur. Heart J. 31, 1188–1196. doi: 10.1093/eurheartj/ehp549
Herman, D. S., Lam, L., Taylor, M. R., Wang, L., Teekakirikul, P., Christodoulou, D., et al. (2012). Truncations of titin causing dilated cardiomyopathy. N. Engl. J. Med. 366, 619–628.
Ho, C. Y., Charron, P., Richard, P., Girolami, F., Van Spaendonck-Zwarts, K. Y., and Pinto, Y. (2015). Genetic advances in sarcomeric cardiomyopathies: state of the art. Cardiovasc. Res. 105, 397–408. doi: 10.1093/cvr/cvv025
Howe, K., Clark, M. D., Torroja, C. F., Torrance, J., Berthelot, C., Muffato, M., et al. (2013). The zebrafish reference genome sequence and its relationship to the human genome. Nature 496, 498–503.
Hu, N., Sedmera, D., Yost, H. J., and Clark, E. B. (2000). Structure and function of the developing zebrafish heart. Anat. Rec. 260, 148–157. doi: 10.1002/1097-0185(20001001)260:2<148::aid-ar50>3.0.co;2-x
Huttner, I. G., Trivedi, G., Jacoby, A., Mann, S. A., Vandenberg, J. I., and Fatkin, D. (2013). A transgenic zebrafish model of a human cardiac sodium channel mutation exhibits bradycardia, conduction-system abnormalities and early death. J. Mol. Cell. Cardiol. 61, 123–132. doi: 10.1016/j.yjmcc.2013.06.005
Huttner, I. G., Wang, L. W., Santiago, C. F., Horvat, C., Johnson, R., Cheng, D., et al. (2018). A-band titin truncation in zebrafish causes dilated cardiomyopathy and hemodynamic stress intolerance. Circ. Genom. Precis. Med. 11:e002135.
Ingles, J., Doolan, A., Chiu, C., Seidman, J., Seidman, C., and Semsarian, C. (2005). Compound and double mutations in patients with hypertrophic cardiomyopathy: implications for genetic testing and counselling. J. Med. Genet. 42:e59. doi: 10.1136/jmg.2005.033886
James, C. A., Syrris, P., Van Tintelen, J. P., and Calkins, H. (2020). The role of genetics in cardiovascular disease: arrhythmogenic cardiomyopathy. Eur. Heart J. 41, 1393–1400. doi: 10.1093/eurheartj/ehaa141
Jopling, C., Sune, G., Faucherre, A., Fabregat, C., and Izpisua Belmonte, J. C. (2012). Hypoxia induces myocardial regeneration in zebrafish. Circulation 126, 3017–3027. doi: 10.1161/circulationaha.112.107888
Kampinga, H. H., and Craig, E. A. (2010). The HSP70 chaperone machinery: J proteins as drivers of functional specificity. Nat. Rev. Mol. Cell Biol. 11, 579–592. doi: 10.1038/nrm2941
Kelly, M., and Semsarian, C. (2009). Multiple mutations in genetic cardiovascular disease: a marker of disease severity? Circ. Cardiovasc. Genet. 2, 182–190. doi: 10.1161/circgenetics.108.836478
Keren, A., Syrris, P., and Mckenna, W. J. (2008). Hypertrophic cardiomyopathy: the genetic determinants of clinical disease expression. Nat. Clin. Pract. Cardiovasc. Med. 5, 158–168. doi: 10.1038/ncpcardio1110
Kieserman, J. M., Myers, V. D., Dubey, P., Cheung, J. Y., and Feldman, A. M. (2019). Current landscape of heart failure gene therapy. J. Am. Heart Assoc. 8:e012239.
Kolokotronis, K., Kuhnisch, J., Klopocki, E., Dartsch, J., Rost, S., Huculak, C., et al. (2019). Biallelic mutation in MYH7 and MYBPC3 leads to severe cardiomyopathy with left ventricular noncompaction phenotype. Hum. Mutat. 40, 1101–1114.
Koth, J., Maguire, M. L., Mcclymont, D., Diffley, L., Thornton, V. L., Beech, J., et al. (2017). High-resolution magnetic resonance imaging of the regenerating adult zebrafish heart. Sci. Rep. 7:2917.
Lee, J., Cao, H., Kang, B. J., Jen, N., Yu, F., Lee, C. A., et al. (2014). Hemodynamics and ventricular function in a zebrafish model of injury and repair. Zebrafish 11, 447–454. doi: 10.1089/zeb.2014.1016
Lin, E., Craig, C., Lamothe, M., Sarunic, M. V., Beg, M. F., and Tibbits, G. F. (2015). Construction and use of a zebrafish heart voltage and calcium optical mapping system, with integrated electrocardiogram and programmable electrical stimulation. Am. J. Physiol. Regul. Integr. Comp. Physiol. 308, R755–R768.
Lin, E., Shafaattalab, S., Gill, J., Al-Zeer, B., Craig, C., Lamothe, M., et al. (2020). Physiological phenotyping of the adult zebrafish heart. Mar. Genomics 49:100701. doi: 10.1016/j.margen.2019.100701
Lin, M. H., Chou, H. C., Chen, Y. F., Liu, W., Lee, C. C., Liu, L. Y., et al. (2018). Development of a rapid and economic in vivo electrocardiogram platform for cardiovascular drug assay and electrophysiology research in adult zebrafish. Sci. Rep. 8:15986. doi: 10.1038/s41598-018-33577-7
Lipshultz, S. E., Law, Y. M., Asante-Korang, A., Austin, E. D., Dipchand, A. I., Everitt, M. D., et al. (2019). Cardiomyopathy in children: classification and diagnosis: a scientific statement from the American Heart Association. Circulation 140, e9–e68.
Ma, X., Zhu, P., Ding, Y., Zhang, H., Qiu, Q., Dvornikov, A. V., et al. (2020). Retinoid X receptor alpha is a spatiotemporally predominant therapeutic target for anthracycline-induced cardiotoxicity. Sci. Adv. 6:eaay2939. doi: 10.1126/sciadv.aay2939
Macrae, C. A., and Peterson, R. T. (2015). Zebrafish as tools for drug discovery. Nat. Rev. Drug Discov. 14, 721–731. doi: 10.1038/nrd4627
Makavos, G., Kappaairis, C., Tselegkidi, M. E., Karamitsos, T., Rigopoulos, A. G., Noutsias, M., et al. (2019). Hypertrophic cardiomyopathy: an updated review on diagnosis, prognosis, and treatment. Heart Fail. Rev 24, 439–459. doi: 10.1007/s10741-019-09775-4
Marcus, F. I., Mckenna, W. J., Sherrill, D., Basso, C., Bauce, B., and Bluemke, D. A. (2010). Diagnosis of arrhythmogenic right ventricular cardiomyopathy/dysplasia: proposed modification of the task force criteria. Circulation 121, 1533–1541.
Marian, A. J., and Braunwald, E. (2017). Hypertrophic cardiomyopathy: genetics, pathogenesis, clinical manifestations, diagnosis, and therapy. Circ. Res. 121, 749–770. doi: 10.1161/circresaha.117.311059
Marin, T. M., Keith, K., Davies, B., Conner, D. A., Guha, P., and Kalaitzidis, D. (2011). Rapamycin reverses hypertrophic cardiomyopathy in a mouse model of LEOPARD syndrome-associated PTPN11 mutation. J. Clin. Invest. 121, 1026–1043. doi: 10.1172/jci44972
Maron, B. J., Roberts, W. C., Arad, M., Haas, T. S., Spirito, P., Wright, G. B., et al. (2009). Clinical outcome and phenotypic expression in LAMP2 cardiomyopathy. JAMA 301, 1253–1259. doi: 10.1001/jama.2009.371
Maron, B. J., Towbin, J. A., Thiene, G., Antzelevitch, C., Corrado, D., Arnett, D., et al. (2006). Contemporary definitions and classification of the cardiomyopathies: an American Heart Association scientific statement from the council on clinical cardiology, heart failure and transplantation committee; quality of care and outcomes research and functional genomics and translational biology interdisciplinary working groups; and council on epidemiology and prevention. Circulation 113, 1807–1816. doi: 10.1161/circulationaha.106.174287
Mattesi, G., Zorzi, A., Corrado, D., and Cipriani, A. (2020). Natural history of arrhythmogenic cardiomyopathy. J. Clin. Med. 9:878. doi: 10.3390/jcm9030878
McKoy, G., Protonotarios, N., Crosby, A., Tsatsopoulou, A., Anastasakis, A., Coonar, A., et al. (2000). Identification of a deletion in plakoglobin in arrhythmogenic right ventricular cardiomyopathy with palmoplantar keratoderma and woolly hair (Naxos disease). Lancet 355, 2119–2124. doi: 10.1016/s0140-6736(00)02379-5
McMullen, J. R., Sherwood, M. C., Tarnavski, O., Zhang, L., Dorfman, A. L., Shioi, T., et al. (2004). Inhibition of mTOR signaling with rapamycin regresses established cardiac hypertrophy induced by pressure overload. Circulation 109, 3050–3055. doi: 10.1161/01.cir.0000130641.08705.45
McNair, W. P., Ku, L., Taylor, M. R., Fain, P. R., Dao, D., Wolfel, E., et al. (2004). SCN5A mutation associated with dilated cardiomyopathy, conduction disorder, and arrhythmia. Circulation 110, 2163–2167. doi: 10.1161/01.cir.0000144458.58660.bb
Meder, B., Laufer, C., Hassel, D., Just, S., Marquart, S., Vogel, B., et al. (2009). A single serine in the carboxyl terminus of cardiac essential myosin light chain-1 controls cardiomyocyte contractility in vivo. Circ. Res. 104, 650–659. doi: 10.1161/circresaha.108.186676
Meriin, A. B., Narayanan, A., Meng, L., Alexandrov, I., Varelas, X., Cisse, I. I., et al. (2018). Hsp70-Bag3 complex is a hub for proteotoxicity-induced signaling that controls protein aggregation. Proc. Natl. Acad. Sci. U.S.A. 115, E7043–E7052.
Merlo, M., Cannata, A., Gobbo, M., Stolfo, D., Elliott, P. M., and Sinagra, G. (2018). Evolving concepts in dilated cardiomyopathy. Eur. J. Heart Fail. 20, 228–239. doi: 10.1002/ejhf.1103
Merrifield, G. D., Mullin, J., Gallagher, L., Tucker, C., Jansen, M. A., Denvir, M., et al. (2017). Rapid and recoverable in vivo magnetic resonance imaging of the adult zebrafish at 7T. Magn. Reson. Imaging 37, 9–15. doi: 10.1016/j.mri.2016.10.013
Meyer, A., and Schartl, M. (1999). Gene and genome duplications in vertebrates: the one-to-four (-to-eight in fish) rule and the evolution of novel gene functions. Curr. Opin. Cell Biol. 11, 699–704. doi: 10.1016/s0955-0674(99)00039-3
Muchtar, E., Blauwet, L. A., and Gertz, M. A. (2017). Restrictive cardiomyopathy: genetics, pathogenesis, clinical manifestations, diagnosis, and therapy. Circ. Res. 121, 819–837. doi: 10.1161/circresaha.117.310982
Norton, N., Li, D., and Hershberger, R. E. (2012). Next-generation sequencing to identify genetic causes of cardiomyopathies. Curr. Opin. Cardiol. 27, 214–220. doi: 10.1097/hco.0b013e328352207e
Norton, N., Li, D., Rieder, M. J., Siegfried, J. D., Rampersaud, E., Zuchner, S., et al. (2011). Genome-wide studies of copy number variation and exome sequencing identify rare variants in BAG3 as a cause of dilated cardiomyopathy. Am. J. Hum. Genet. 88, 273–282. doi: 10.1016/j.ajhg.2011.01.016
Olson, T. M., Karst, M. L., Whitby, F. G., and Driscoll, D. J. (2002). Myosin light chain mutation causes autosomal recessive cardiomyopathy with mid-cavitary hypertrophy and restrictive physiology. Circulation 105, 2337–2340. doi: 10.1161/01.cir.0000018444.47798.94
Padron-Barthe, L., Dominguez, F., Garcia-Pavia, P., and Lara-Pezzi, E. (2017). Animal models of arrhythmogenic right ventricular cardiomyopathy: what have we learned and where do we go? Insight for therapeutics. Basic Res. Cardiol. 112:50.
Parker, F., and Peckham, M. (2020). Disease mutations in striated muscle myosins. Biophys. Rev. 12, 887–894. doi: 10.1007/s12551-020-00721-5
Pilichou, K., Nava, A., Basso, C., Beffagna, G., Bauce, B., Lorenzon, A., et al. (2006). Mutations in desmoglein-2 gene are associated with arrhythmogenic right ventricular cardiomyopathy. Circulation 113, 1171–1179.
Pinilla-Vera, M., Hahn, V. S., and Kass, D. A. (2019). Leveraging signaling pathways to treat heart failure with reduced ejection fraction. Circ. Res. 124, 1618–1632. doi: 10.1161/circresaha.119.313682
Pinto, Y. M., Elliott, P. M., Arbustini, E., Adler, Y., Anastasakis, A., Bohm, M., et al. (2016). Proposal for a revised definition of dilated cardiomyopathy, hypokinetic non-dilated cardiomyopathy, and its implications for clinical practice: a position statement of the ESC working group on myocardial and pericardial diseases. Eur. Heart J. 37, 1850–1858. doi: 10.1093/eurheartj/ehv727
Poss, K. D., Wilson, L. G., and Keating, M. T. (2002). Heart regeneration in zebrafish. Science 298, 2188–2190. doi: 10.1126/science.1077857
Ramos, F. J., Chen, S. C., Garelick, M. G., Dai, D. F., Liao, C. Y., Schreiber, K. H., et al. (2012). Rapamycin reverses elevated mTORC1 signaling in lamin A/C-deficient mice, rescues cardiac and skeletal muscle function, and extends survival. Sci. Transl. Med. 4:144ra103. doi: 10.1126/scitranslmed.3003802
Rampazzo, A., Nava, A., Malacrida, S., Beffagna, G., Bauce, B., Rossi, V., et al. (2002). Mutation in human desmoplakin domain binding to plakoglobin causes a dominant form of arrhythmogenic right ventricular cardiomyopathy. Am. J. Hum. Genet. 71, 1200–1206. doi: 10.1086/344208
Repetti, G. G., Toepfer, C. N., Seidman, J. G., and Seidman, C. E. (2019). Novel therapies for prevention and early treatment of cardiomyopathies. Circ. Res. 124, 1536–1550. doi: 10.1161/circresaha.119.313569
Roberts, J. D., Murphy, N. P., Hamilton, R. M., Lubbers, E. R., James, C. A., Kline, C. F., et al. (2019). Ankyrin-B dysfunction predisposes to arrhythmogenic cardiomyopathy and is amenable to therapy. J. Clin. Invest. 129, 3171–3184.
Rosenbaum, A. N., Agre, K. E., and Pereira, N. L. (2020). Genetics of dilated cardiomyopathy: practical implications for heart failure management. Nat. Rev. Cardiol. 17, 286–297. doi: 10.1038/s41569-019-0284-0
Sarantis, P., Gaitanaki, C., and Beis, D. (2019). Ventricular remodeling of single-chambered myh6(-/-) adult zebrafish hearts occurs via a hyperplastic response and is accompanied by elastin deposition in the atrium. Cell Tissue Res. 378, 279–288. doi: 10.1007/s00441-019-03044-4
Sarparanta, J., Jonson, P. H., Golzio, C., Sandell, S., Luque, H., Screen, M., et al. (2012). Mutations affecting the cytoplasmic functions of the co-chaperone DNAJB6 cause limb-girdle muscular dystrophy. Nat. Genet. 44, 450–455; S451–S452.
Schafer, S., De Marvao, A., Adami, E., Fiedler, L. R., Ng, B., Khin, E., et al. (2017). Titin-truncating variants affect heart function in disease cohorts and the general population. Nat. Genet. 49, 46–53. doi: 10.1038/ng.3719
Scheid, L. M., Mosqueira, M., Hein, S., Kossack, M., Juergensen, L., Mueller, M., et al. (2016). Essential light chain S195 phosphorylation is required for cardiac adaptation under physical stress. Cardiovasc. Res. 111, 44–55. doi: 10.1093/cvr/cvw066
Schiaffino, S., and Reggiani, C. (1996). Molecular diversity of myofibrillar proteins: gene regulation and functional significance. Physiol. Rev. 76, 371–423. doi: 10.1152/physrev.1996.76.2.371
Sciarretta, S., Forte, M., Frati, G., and Sadoshima, J. (2018). New insights into the role of mTOR signaling in the cardiovascular system. Circ. Res. 122, 489–505. doi: 10.1161/circresaha.117.311147
Seeley, M., Huang, W., Chen, Z., Wolff, W. O., Lin, X., and Xu, X. (2007). Depletion of zebrafish titin reduces cardiac contractility by disrupting the assembly of Z-discs and A-bands. Circ. Res. 100, 238–245. doi: 10.1161/01.res.0000255758.69821.b5
Sehnert, A. J., Huq, A., Weinstein, B. M., Walker, C., Fishman, M., and Stainier, D. Y. (2002). Cardiac troponin T is essential in sarcomere assembly and cardiac contractility. Nat. Genet. 31, 106–110. doi: 10.1038/ng875
Seidman, C. E., and Seidman, J. G. (2011). Identifying sarcomere gene mutations in hypertrophic cardiomyopathy: a personal history. Circ. Res. 108, 743–750. doi: 10.1161/circresaha.110.223834
Shah, A. N., Davey, C. F., Whitebirch, A. C., Miller, A. C., and Moens, C. B. (2015). Rapid reverse genetic screening using CRISPR in zebrafish. Nat. Methods 12, 535–540. doi: 10.1038/nmeth.3360
Shah, S., Henry, A., Roselli, C., Lin, H., Sveinbjornsson, G., Fatemifar, G., et al. (2020). Genome-wide association and Mendelian randomisation analysis provide insights into the pathogenesis of heart failure. Nat. Commun. 11:163.
Shih, Y. H., Zhang, Y., Ding, Y., Ross, C. A., Li, H., Olson, T. M., et al. (2015). Cardiac transcriptome and dilated cardiomyopathy genes in zebrafish. Circ. Cardiovasc. Genet. 8, 261–269. doi: 10.1161/circgenetics.114.000702
Spezzacatene, A., Sinagra, G., Merlo, M., Barbati, G., Graw, S. L., Brun, F., et al. (2015). Arrhythmogenic phenotype in dilated cardiomyopathy: natural history and predictors of life-threatening arrhythmias. J. Am. Heart Assoc. 4:e002149.
Spudich, J. A., Aksel, T., Bartholomew, S. R., Nag, S., Kawana, M., Yu, E. C., et al. (2016). Effects of hypertrophic and dilated cardiomyopathy mutations on power output by human beta-cardiac myosin. J. Exp. Biol. 219, 161–167. doi: 10.1242/jeb.125930
Stainier, D. Y., Fouquet, B., Chen, J. N., Warren, K. S., Weinstein, B. M., Meiler, S. E., et al. (1996). Mutations affecting the formation and function of the cardiovascular system in the zebrafish embryo. Development 123, 285–292.
Stoyek, M. R., Rog-Zielinska, E. A., and Quinn, T. A. (2018). Age-associated changes in electrical function of the zebrafish heart. Prog. Biophys. Mol. Biol. 138, 91–104. doi: 10.1016/j.pbiomolbio.2018.07.014
Sun, X., Hoage, T., Bai, P., Ding, Y., Chen, Z., Zhang, R., et al. (2009). Cardiac hypertrophy involves both myocyte hypertrophy and hyperplasia in anemic zebrafish. PLoS One 4:e6596. doi: 10.1371/journal.pone.0006596
Syrris, P., Ward, D., Evans, A., Asimaki, A., Gandjbakhch, E., Sen-Chowdhry, S., et al. (2006). Arrhythmogenic right ventricular dysplasia/cardiomyopathy associated with mutations in the desmosomal gene desmocollin-2. Am. J. Hum. Genet. 79, 978–984. doi: 10.1086/509122
Tanigawa, G., Jarcho, J. A., Kass, S., Solomon, S. D., Vosberg, H. P., Seidman, J. G., et al. (1990). A molecular basis for familial hypertrophic cardiomyopathy: an alpha/beta cardiac myosin heavy chain hybrid gene. Cell 62, 991–998. doi: 10.1016/0092-8674(90)90273-h
Tessadori, F., Roessler, H. I, Savelberg, S. M. C., Chocron, S., Kamel, S. M., Duran, K. J., et al. (2018). Effective CRISPR/Cas9-based nucleotide editing in zebrafish to model human genetic cardiovascular disorders. Dis. Model Mech. 11:dmm035469. doi: 10.1242/dmm.035469
Theis, J. L., Sharpe, K. M., Matsumoto, M. E., Chai, H. S., Nair, A. A., Theis, J. D., et al. (2011). Homozygosity mapping and exome sequencing reveal GATAD1 mutation in autosomal recessive dilated cardiomyopathy. Circ. Cardiovasc. Genet. 4, 585–594. doi: 10.1161/circgenetics.111.961052
Towbin, J. A., Mckenna, W. J., Abrams, D. J., Ackerman, M. J., Calkins, H., Darrieux, F. C. C., et al. (2019). 2019 HRS expert consensus statement on evaluation, risk stratification, and management of arrhythmogenic cardiomyopathy: executive summary. Heart Rhythm 16, e373–e407.
Van Berlo, J. H., Maillet, M., and Molkentin, J. D. (2013). Signaling effectors underlying pathologic growth and remodeling of the heart. J. Clin. Invest. 123, 37–45. doi: 10.1172/jci62839
Vimalanathan, A. K., Ehler, E., and Gehmlich, K. (2018). Genetics of and pathogenic mechanisms in arrhythmogenic right ventricular cardiomyopathy. Biophys. Rev. 10, 973–982. doi: 10.1007/s12551-018-0437-0
Wahbi, K., Behin, A., Becane, H. M., Leturcq, F., Cossee, M., Laforet, P., et al. (2013). Dilated cardiomyopathy in patients with mutations in anoctamin 5. Int. J. Cardiol. 168, 76–79. doi: 10.1016/j.ijcard.2012.09.070
Wakamatsu, Y., Ogino, K., and Hirata, H. (2019). Swimming capability of zebrafish is governed by water temperature, caudal fin length and genetic background. Sci. Rep. 9:16307.
Wang, L. W., Huttner, I. G., Santiago, C. F., Kesteven, S. H., Yu, Z. Y., Feneley, M. P., et al. (2017). Standardized echocardiographic assessment of cardiac function in normal adult zebrafish and heart disease models. Dis. Model Mech. 10, 63–76. doi: 10.1242/dmm.026989
Wilde, A. A. M., and Amin, A. S. (2018). Clinical spectrum of SCN5A mutations: long QT syndrome, brugada syndrome, and cardiomyopathy. JACC Clin. Electrophysiol. 4, 569–579.
Wu, R. S., Lam, I. I., Clay, H., Duong, D. N., Deo, R. C., and Coughlin, S. R. (2018). A rapid method for directed gene knockout for screening in G0 zebrafish. Dev. Cell 46, 112–125e4.
Xu, T., Yang, Z., Vatta, M., Rampazzo, A., Beffagna, G., Pilichou, K., et al. (2010). Compound and digenic heterozygosity contributes to arrhythmogenic right ventricular cardiomyopathy. J. Am. Coll. Cardiol. 55, 587–597. doi: 10.1016/j.jacc.2009.11.020
Xu, X., Meiler, S. E., Zhong, T. P., Mohideen, M., Crossley, D. A., Burggren, W. W., et al. (2002). Cardiomyopathy in zebrafish due to mutation in an alternatively spliced exon of titin. Nat. Genet. 30, 205–209. doi: 10.1038/ng816
Yan, J., Li, H., Bu, H., Jiao, K., Zhang, A. X., Le, T., et al. (2020). Aging-associated sinus arrest and sick sinus syndrome in adult zebrafish. PLoS One 15:e0232457. doi: 10.1371/journal.pone.0232457
Yang, J., Shah, S., Olson, T. M., and Xu, X. (2016). Modeling GATAD1-associated dilated cardiomyopathy in adult zebrafish. J. Cardiovasc. Dev. Dis. 3:6. doi: 10.3390/jcdd3010006
Yotti, R., Seidman, C. E., and Seidman, J. G. (2019). Advances in the genetic basis and pathogenesis of sarcomere cardiomyopathies. Annu. Rev. Genomics Hum. Genet. 20, 129–153. doi: 10.1146/annurev-genom-083118-015306
Keywords: cardiomyopathy, adult zebrafish, causative gene, animal model, precision medicine
Citation: Ding Y, Bu H and Xu X (2020) Modeling Inherited Cardiomyopathies in Adult Zebrafish for Precision Medicine. Front. Physiol. 11:599244. doi: 10.3389/fphys.2020.599244
Received: 26 August 2020; Accepted: 30 October 2020;
Published: 19 November 2020.
Edited by:
Thao P. Nguyen, David Geffen School of Medicine at UCLA, United StatesReviewed by:
Brenda Gerull, University Hospital Würzburg, GermanyJau-Nian Chen, UCLA MCDB, United States
Giuseppe Limongelli, Second University of Naples, Italy
Copyright © 2020 Ding, Bu and Xu. This is an open-access article distributed under the terms of the Creative Commons Attribution License (CC BY). The use, distribution or reproduction in other forums is permitted, provided the original author(s) and the copyright owner(s) are credited and that the original publication in this journal is cited, in accordance with accepted academic practice. No use, distribution or reproduction is permitted which does not comply with these terms.
*Correspondence: Xiaolei Xu, eHUueGlhb2xlaUBtYXlvLmVkdQ==
†These authors have contributed equally to this work