- 1University of California, Los Angeles (UCLA) Cardiac Arrhythmia Center, David Geffen School of Medicine, Los Angeles, CA, United States
- 2UCLA Neurocardiology Research Program of Excellence, UCLA, Los Angeles, CA, United States
- 3Molecular, Cellular, and Integrative Physiology Program, UCLA, Los Angeles, CA, United States
Dysfunction of the autonomic nervous system has been implicated in the pathogenesis of cardiovascular disease, including congestive heart failure and cardiac arrhythmias. Despite advances in the medical and surgical management of these entities, progression of disease persists as does the risk for sudden cardiac death. With improved knowledge of the dynamic relationships between the nervous system and heart, neuromodulatory techniques such as cardiac sympathetic denervation and vagal nerve stimulation (VNS) have emerged as possible therapeutic approaches for the management of these disorders. In this review, we present the structure and function of the cardiac nervous system and the remodeling that occurs in disease states, emphasizing the concept of increased sympathoexcitation and reduced parasympathetic tone. We review preclinical evidence for vagal nerve stimulation, and early results of clinical trials in the setting of congestive heart failure. Vagal nerve stimulation, and other neuromodulatory techniques, may improve the management of cardiovascular disorders, and warrant further study.
Introduction
The autonomic nervous system regulates all aspects of cardiac function, including chronotropy, inoptroy, dromotropy, and lusitropy (Fukuda et al., 2015). In the last decade, it has become increasingly evident that autonomic dysregulation plays a major role in the development and progression of major cardiovascular diseases, including myocardial infarction, heart failure, and sudden cardiac death (Fukuda et al., 2015; Ardell et al., 2016; Ardell and Armour, 2016; Shivkumar et al., 2016). While pharmacologic therapy targets cardiac disease through neurohormonal blockade, results have not been as promising as hoped (Florea and Cohn, 2014; McMurray et al., 2014; Shivkumar et al., 2016). This has led to increasing interest in neuromodulation as a new therapeutic approach, with therapies such as vagal nerve stimulation (VNS) and video-assisted thoracoscopic sympathectomy showing efficacy for the treatment of heart failure and refractory ventricular arrhythmias (Schwartz et al., 2004, 2008; Wilde et al., 2008; Bourke et al., 2010; Vaseghi et al., 2014; Sharma et al., 2020). Though these early studies are promising, a better understanding of the anatomy and function of the cardiac nervous system is imperative to developing and implementing precise neuromodulatory therapies.
The anatomy of the cardiac nervous system is complex and has been categorized into: (1) central components, (2) intrathoracic extracardiac components, and (3) intrinsic cardiac components (Figure 1; Fukuda et al., 2015; Ardell et al., 2016). The intrathoracic extracardiac system connects the central components to the intrinsic cardiac nervous system (ICNS), and is composed of parasympathetic and sympathetic systems that are traditionally thought to exert opposing action on cardiac electrical and mechanical function (Armour, 2008; Palma and Benarroch, 2014). The parasympathetic component acts through the vagus nerve and its intrathoracic branches, using acetylcholine, nitric oxide, and vasoactive intestinal peptide as neurotransmitters (Hill et al., 1995; Jänig, 2006). The sympathetic component originates in the intermediolateral cell columns of the spinal cord, projects via C7 to T6 rami to the superior cervical, middle cervical, stellate, or cervicothoracic ganglia, and acts though norepinephrine and neuropeptide Y (Norris et al., 1974, 1977; Jänig, 2006; Herring et al., 2012; Hoang et al., 2020). Afferent cardiac neurons, now known to be dispersed throughout the myocardium, act as mechanoreceptors and/or chemoreceptors, completing a cardiac neural circuit (Thompson et al., 2000; Armour and Ardell, 2004; Ardell et al., 2016). These afferent neurons have multimodal transduction capabilities, capable of transducing the presence of myocardial ischemia and (Fu and Longhurst, 2009) initiating reflex sympathetic activation in the setting of myocardial infarction (Malliani et al., 1969, 1973; Malliani and Montano, 2002; Billman, 2006). The ICNS is composed of clusters of ganglia referred to as ganglionated plexi distributed in epicardial fat pads (Armour, 2008). These ganglionated plexi contain a diverse population of neurons, including neurons that that receive parasympathetic and sympathetic input, neurons that receive afferent information directly from myocytes, and local interneurons (Armour, 2008; Ardell et al., 2016). Contrary to initial studies, recent evidence has shown that the ICNS is not simply a relay station for extrinsic projections to the heart, but instead functions with higher centers to modulate regional cardiac electrical and mechanical indices on a beat-to-beat basis (Cardinal et al., 2009). Moreover, the ICNS is a dynamic neural network, which remodels with disease states (Hardwick et al., 2008; Beaumont et al., 2015; Rajendran et al., 2016). For example, in the setting of myocardial infarction, our group has shown that processing of afferent and efferent neural signals in the ICNS is impaired, with overall decreased network connectivity, suggesting an inability of neurons to respond to local myocardial stimuli (Rajendran et al., 2016). Similar remodeling occurs within intrathoracic and primary sensory ganglia (Zucker et al., 2012; Wang et al., 2014; Ardell et al., 2016; Ajijola et al., 2017; Yoshie et al., 2020). Given the complexity of these systems, and remodeling that occurs in disease states, a better understanding of the function of each component is necessary to improve therapeutic outcomes and reduce off-target consequences.
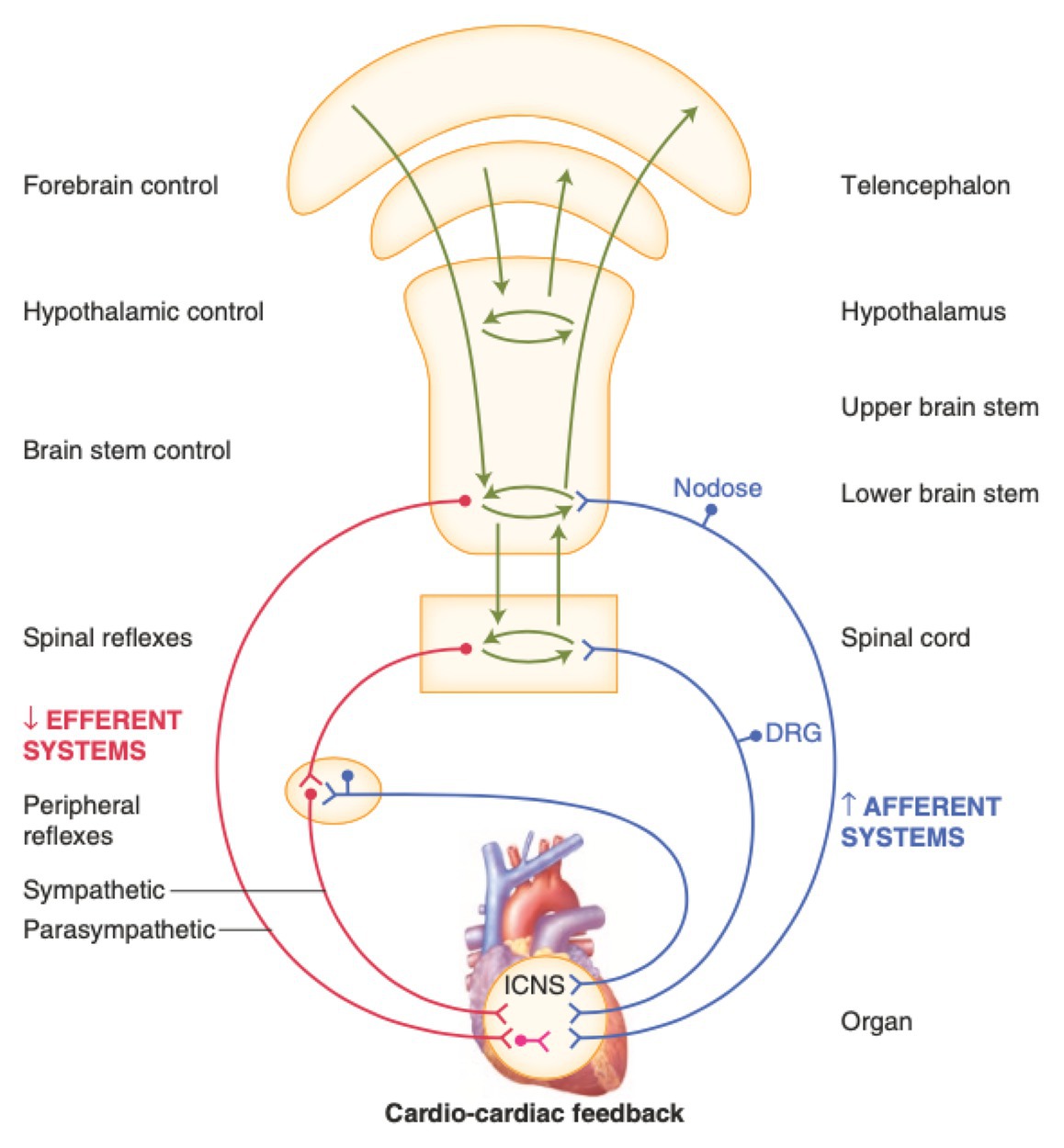
Figure 1. Neural regulation of cardiac function involves multiple nested feedback loops at the level of the heart, peripheral ganglia, and central nervous system. Afferent systems (blue) are mediated through the intrinsic cardiac nervous system (ICNS), dorsal root ganglia (DRG), and nodose ganglia. Efferent systems (red) involve sympathetic, parasympathetic, and local circuit neuron (LCNs). DRG, dorsal root ganglia. ICNS, intrinsic cardiac nervous system. Adapted from Jänig (2014) with permission.
In this review, we provide a brief foundation for understanding the structure of, and organization of the cardiac autonomic nervous system and highlight neural control of cardiovascular function, emphasizing the roles of the sympathetic, parasympathetic, and ICNSs. Based on this scientific foundation, an overview of current neuromodulatory therapies is presented, focusing on cardiac sympathetic denervation and VNS (Levy and Schwartz, 1994; Shivkumar et al., 2016; Hanna et al., 2018). We highlight preclinical studies of vagal nerve stimulation for structural heart disease and arrythmias. We conclude with a discussion on recently completed and in-process clinical trials of vagal nerve stimulation for heart disease, primarily congestive heart failure, driven by foundations established from preclinical studies.
Overview of Structural and Functional Organization of the Cardiovascular Neuraxis
Autonomic control of the cardiovascular system is mediated through afferent and efferent pathways and neural networks involving the brain stem, spinal cord, peripheral ganglia, and ICNS (Figures 1, 2; Ardell et al., 2016). Feedback systems between each of these components exist and mediate transduction of beat-to-beat information, allowing for maintenance of hemostasis and adaptation to stressors (Armour, 2008; Fukuda et al., 2015; Ardell et al., 2016). The cardiovascular neuraxis can be considered in three different levels: Level 1 is considered the heart with its own ICNS, which is comprised of cardiac ganglia that reside at the origin of the great vessels, and posteriorly along the atria and atrioventricular junction (Armour, 2008; Ardell et al., 2016; Shivkumar et al., 2016). Level 2 consists of intrathoracic components that regulate cardiac function including the middle cervical ganglia, cervicothoracic (stellate) ganglia, and the T2-T4 portions of the sympathetic chain (Kuntz and Morehouse, 1930; Armour and Janes, 1988; Ardell et al., 2016). Level 3 can be considered as the dorsal root and nodose ganglia, which mediates the majority of afferent neurotransmission, as well as spinal cord, brainstem, and higher centers (Malliani et al., 1975; Tjen et al., 1997; Armour and Kember, 2004; Jänig, 2006; Olshansky et al., 2008). Importantly, information is processed at multiple levels by interneurons, resulting in the interdependent network interactions between brainstem, spinal, intrathoracic, and cardio-cardiac reflexes (Cardinal et al., 2009; Ardell et al., 2015, 2016; Habecker et al., 2016). While initially thought to exert opposing effects on cardiac electrophysiologic and mechanical properties, interactions between the sympathetic and parasympathetic nervous systems mediate cooperative control and regulation of function (Linz et al., 2014; Fukuda et al., 2015; Ardell et al., 2016).
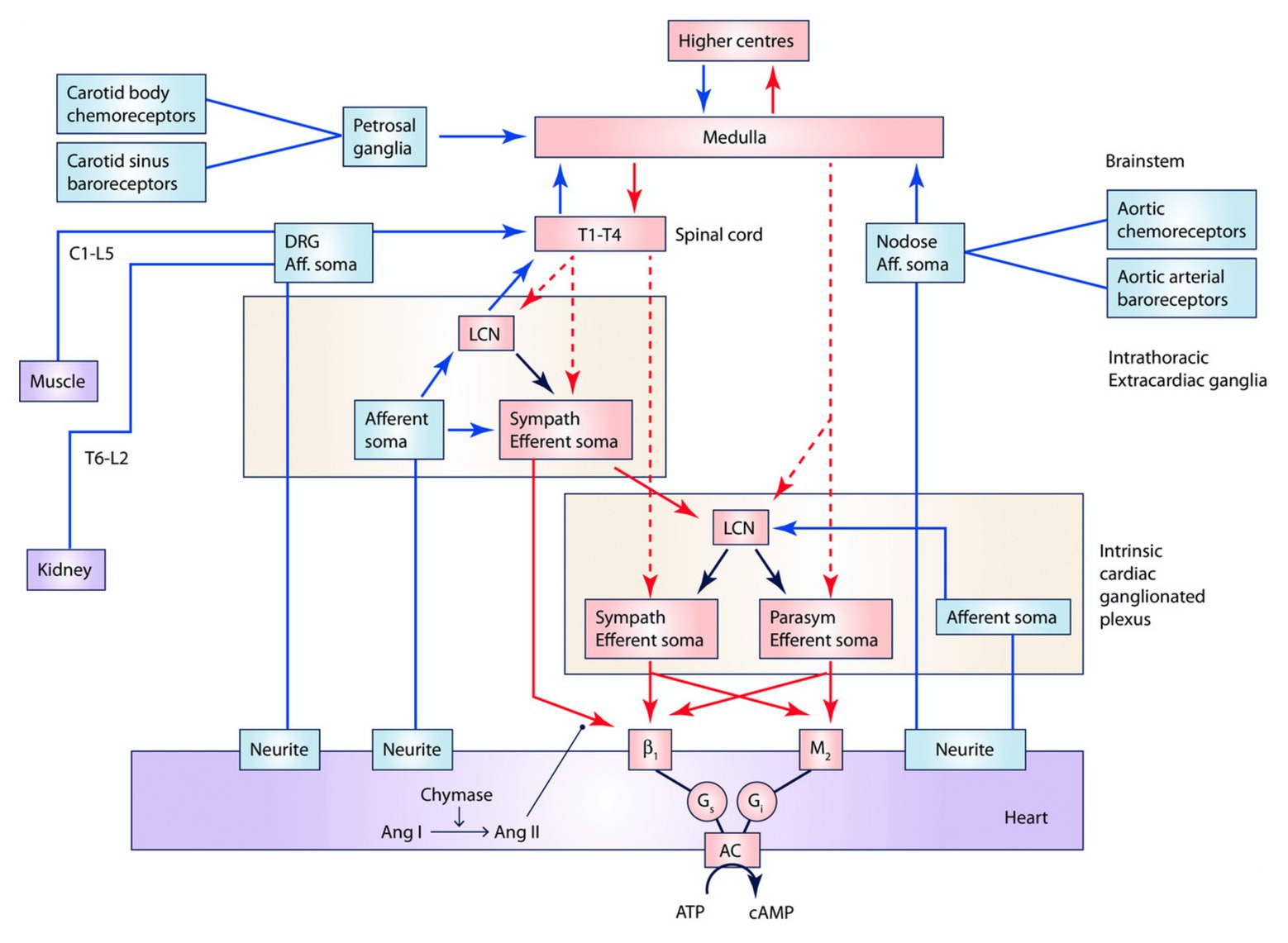
Figure 2. Interactions between central nervous system, peripheral ganglia, and the ICNS. Multiple interdependent feedback loops regulate regional cardiac mechanical and electrical function. At the level of the heart, sensory (blue) neurons provide input directly and indirectly through the ICNS, peripheral ganglia, and spinal cord, which are capable of engaging cardiocentric reflexes. Converging inputs result in activation of adrenergic or muscarinic receptors as well as receptors for peptide co-transmitters such as neuropeptide Y (not displayed). Preganglionic sympathetic and parasympathetic efferent fibers project (red, dashed) to the ICNS, including to LCNs, and postganglionic sympathetic fibers (red, solid) also directly to the myocardium. The ICNS acts as the final common pathway for cardiac control, though interactions occur at all levels. Input from chemoreceptors, baroreceptors, as well as neurohormonal factors such as angiotensin II and circulating epinephrine (not displayed), also modulate cardiac function. Ang I, angiotensin I. Ang II, angiotensin II. LCN, local circuit neuron. Adapted from Ardell et al. (2016) with permission.
Cardiac Sympathetic Innervation: In Health and Disease
Canonical organization of the sympathetic nervous system traditionally considers two sets of neuronal projections, defined by location of cell bodies and neurotransmitters mediating cell to cell communication. Preganglionic neurons with cell bodies in the intermediolateral cell column of the cervical and thoracic spinal cord (C7-T6) project to the superior, middle, cervicothoracic or stellate ganglia, and the remainder of paravertebral chain, with fibers running through ventral horns (Randall, 1994; Armour and Ardell, 2004; Jänig, 2006). Postganglionic sympathetic neurons project from these intrathoracic ganglia to atrial and ventricular myocardium, components of the conduction system, and coronary vasculature, traveling primarily as mixed cardiopulmonary nerves (Armour and Hopkins, 1981; Hopkins and Armour, 1984; Kawashima, 2005). Preganglionic neurons act through acetylcholine, binding to nicotinic acetylcholine receptors, whereas postganglionic neurons release norepinephrine. Sympathetic innervation of the heart has been well characterized using immunohistochemical studies targeting tyrosine hydroxylase, the rate-rate limiting enzyme in norepinephrine synthesis, across multiple animal models (Wharton et al., 1990; Kawano et al., 2003). Although canonical neurobiology primarily emphasizes the role of norepinephrine for postganglionic neurotransmission, several co-receptors such as neuropeptide Y and galanin have been reported to be released from postganglionic nerve terminals, especially during higher levels of neural activity (Lundberg et al., 1990; Hoover et al., 2009; Herring et al., 2012). These peptides may mediate additional efferent effects and have been implicated in various cardiovascular disorders including heart failure (Shanks and Herring, 2013; Ajijola et al., 2019). Further study of these co-transmitters may prove of value in development of novel therapeutics (Bohlender and Imboden, 2012).
In general, sympathetic activation mediates increases in chronotropy, inotropy, dromotropy, and lusitropy (Burwash et al., 1993; Fukuda et al., 2015). In more contemporary porcine studies, both right and left stellate stimulation reduced activation recovery intervals, an in vivo surrogate for action potential duration (Haws and Lux, 1990; Vaseghi et al., 2013). Notably, differential shortening of activation recovery intervals in different portions of the left ventricular (LV) by right versus left stellate stimulation was noted suggesting the presence of regional control of cardiac electrical function (Yanowitz et al., 1966; Vaseghi et al., 2012; Vaseghi et al., 2013). In the setting of chronic myocardial infarction, this regional regulation of cardiac electrical function is disrupted, with greater variation in repolarization and altered activation propagation (Ajijola et al., 2013).
In the setting of diseased states, such as myocardial infarction and heart failure, aberrant remodeling of the sympathetic nervous system occurs and is well described (Cao et al., 2000; Zhou et al., 2004; Ajijola et al., 2012, 2015). At the level of the myocardium, cardiac sympathetic nerve density, electrical excitability, and neurotransmitter content are altered in disease states (Cao et al., 2000; Gardner et al., 2015; Tapa et al., 2020). In a Langendorff-perfused mouse model of toxin-induced regional sympathetic hypoinnervation with no accompanying myocardial infarction, greater sensitivity to circulating catecholamines and blunted responses to sympathetic nerve stimulation were observed, demonstrating the direct proarrhythmogenic effects of sympathetic nerve heterogeneity (Tapa et al., 2020). Ischemia results in release of reactive oxygen species, adenosine, and other chemical mediators, that activate afferent neurons within the myocardium (Malliani et al., 1973; Dibner-Dunlap et al., 1993; Minisi and Thames, 1993; Huang et al., 1996). Reflexes at the level of the central nervous system, stellate ganglia, and possibly the ICNS, result in sympathoexcitation to mitigate in part the effects of ischemia on mechanical cardiac function (Minisi and Thames, 1991; Schwartz et al., 1992; Ardell et al., 2019). This reflex sympathoexcitation is associated with structural remodeling of portions of the ICNS as well as the stellate ganglia and an increased risk of ventricular fibrillation (Schwartz et al., 1976; Armour, 2004; Mill et al., 2011; Han et al., 2012). In porcine models of chronic ischemia, as well as humans with ischemic cardiomyopathy, histologic changes in stellate ganglia neurons have been observed resulting in inflammation, glial cell activation, and oxidative stress (Ajijola et al., 2012, 2015). Cholinergic transdifferentiation has been described following myocardial infarction, and, in animal models, co-release of acetylcholine with norepinephrine may be antiarrhythmic by reducing actional potential duration dispersion (Olivas et al., 2016; Wang et al., 2020).
From a clinical perspective, this excessive sympathoexcitation is targeted pharmacologically with beta blockers as well as inhibition of the renin-angiotensin-aldosterone system in the case of heart failure (Triposkiadis et al., 2009; Florea and Cohn, 2014; Fukuda et al., 2015). Neuromodulatory approaches to decrease sympathetic tone, such as thoracic epidural anesthesia, stellate ganglion blockade, cardiac sympathetic denervation, and renal denervation have been utilized clinically to manage these disease entities (Triposkiadis et al., 2009; Bourke et al., 2010; Schwartz, 2014; Fukuda et al., 2015; Shivkumar et al., 2016). In the research setting, another avenue considers application of electrical energy to reversibly block nerve conduction. For example, kilohertz frequency alternating current applied to the sympathetic chain mitigated sympathetic outflow to the heart, reducing electrophysiologic indices associated with sympathetic chain stimulation (Buckley et al., 2017). Similarly, in animals with chronic myocardial infarction, axonal block applied to high thoracic paravertebral chain using charge-balanced direct current produced reversible block of sympathetic nerve activity and reduced ventricular tachycardia/ventricular fibrillation potential (Chui et al., 2017). These technologies and treatment approaches warrant further study given an increasing interest of neuromodulation as a therapeutic opportunity.
Cardiac Parasympathetic Innervation: In Health and Disease
Similar to the structural organization of the sympathetic nervous system, the parasympathetic nervous system is classically described as preganglionic and postganglionic projections (Jänig, 2006; Ardell et al., 2016). Preganglionic fibers, originating in the dorsal motor nucleus and nucleus ambiguus of the medulla project near to, or to, their target organ through the vagus nerve and intrathoracic cardiopulmonary branches (Standish et al., 1994; Hopkins et al., 1996). Presynaptic fibers synapse on postsynaptic cell bodies located near or on the target organ, with neurotransmission mediated through acetylcholine and nicotinic receptors (Jänig, 2006). In the setting of cardiac autonomic system, a significant portion of postganglionic cell bodies are located within ganglia of the ICNS, and then directly project to myocardium, acting through release of acetylcholine (Yuan et al., 1993; Armour et al., 1998; Pauza et al., 2014; Petraitiene et al., 2014). These groups of neurons have been well characterized using markers such as the choline acetyltransferase, and vesicular acetylcholine transporter (Hoover et al., 2004).
In addition to mediating parasympathetic efferent input to the heart and other viscera, the vagus nerve carries afferent information from intrathoracic and intraabdominal viscera to the central nervous system (Schultz and Ustinova, 1996; Schultz, 2001; Jänig, 2006). Of particular relevance to the cardiovascular system, bipolar neurons located in the superior and inferior (nodose) ganglia of the vagus nerve transmit sensory information from the myocardium to the nucleus tractus solitarus (Kalia and Mesulam, 1980; Janes et al., 1986). Vagal afferent fibers are predominantly unmyelinated and multimodal and with reference to bioelectric interventions are thought to be preferentially activated at lower levels of current (Yoo et al., 2013; Ardell et al., 2015). Given the prevalence of afferent fibers in the vagus nerve, with some studies reporting upwards of 80% of fibers as providing afferent input to the CNS, relevance of fiber type and activation is particularly important for the use of vagal nerve stimulation for therapeutic purposes (Janes et al., 1986; Prechtl and Powley, 1990; Hoover et al., 2008).
Vagal afferent fibers carry a wide variety of information to higher centers and include atrial stretch receptors, multimodal receptors within ventricles, pulmonary stretch receptors, and cardiopulmonary chemoreceptors (Paintal, 1953, 1963, 1973). Early studies, employing recordings of the vagus nerve and nodose ganglia, identified neuronal activity that was synchronous with the cardiac cycle, and affected by hemodynamic changes such as varied preload or afterload (Thoren, 1976; Berthoud and Neuhuber, 2000; Schultz, 2001; Schultz et al., 2015). In an in vivo study in guinea pigs, epicardial application of substances such as nitroprusside, calcitonin gene-related peptide, histamine, and bradykinin elicited changes in nodose afferent neuronal activity, suggesting that this population of afferent neurons transduce to a wide variety of neurotransmitters and chemicals at the level of the heart (Thompson et al., 2000).
In experimental models, efferent activation of the vagus nerve produces a bradycardia and slows conduction through the atrioventricular node (Armour et al., 1975; Ardell and Randall, 1986). However, at low levels of current, which primarily activates afferent pathways, a tachycardia is induced, which is abolished with transection of the vagus nerve (Ardell et al., 2015). While effects of vagal nerve stimulation on the sinus and atrioventricular node are well characterized, innervation of the ventricles by the parasympathetic system has been debated. Cholinergic neurons are present and innervate the ventricles as demonstrated by immunohistologic studies, with a greater density at the base than apex (Kawano et al., 2003; Hoover et al., 2004). Functional studies in porcine models demonstrate global prolongation of activation recovery intervals with right and left sided stimulation, with no clear regional regulation by the left versus right vagus (Yamakawa et al., 2014). This coordination across all areas of the heart likely reflects the interganglionic interconnections occurring within the ICNS (Ardell et al., 2016). In addition to electrophysiologic effects, vagal nerve stimulation reduces inotropy, further supporting the existence of functional innervation of the ventricle by the vagus (Yamakawa et al., 2014). With respect to vagal nerve stimulation, transection of afferent pathways rostral to the electrode interface markedly enhancing suppression of all cardiac functions, reflecting the dynamic interactions of afferent and efferent mediated responses whenever bioelectric interventions are imposed (Schwartz et al., 1973; Ardell et al., 2015, 2017).
Recently, selective modulation of neuronal populations has been made possible through optogenetic techniques that employ light-activated ion channels to stimulate or inhibit neurons (Zemelman et al., 2002; Boyden et al., 2005). For example, in a Langendorff-perused murine model, optogenetic stimulation of tyrosine-hydrolase expressing neurons on the epicardial surface of the right atrium and right ventricle resulted in greater contractile forces and heart rate, with shortening of optically-mapped action potentials (Wengrowski et al., 2015). A similar study in a murine Langendorff-perfused model targeting cardiac parasympathetic neurons expressing choline acetyltransferase demonstrated bradycardia and heart block with illumination of the junction of the superior vena cava and right atrium, which was blocked by administration of atropine (Moreno et al., 2019). Other non-electrical approaches for neural stimulation including designer receptors specifically activated by designer drugs (DREADDs) or receptor activated solely by a synthetic ligand (RASSL), a family of engineered proteins that respond only to synthetic ligands and allow for modulation of G protein coupled receptor signaling (Sternson and Roth, 2014). Using excitatory DREADDs in a rat model of heart failure, Garrott et al. (2017) activated a population of oxytocin and glutamate co-releasing neurons in the hypothalamus that stimulated cardiac vagal neurons, which mitigated myocyte hypertrophy, collagen deposition, and ventricular dysfunction. These novel approaches highlight pre-clinical advances that may allow for subselective evaluation of specific parasympathetic neuronal populations.
In the setting of myocardial injury and disease, structural and functional remodeling occurs at multiple levels of the cardiac nervous system (Ardell et al., 2016; Shivkumar et al., 2016; Shivkumar and Ardell, 2016). While myocardial injury generally results in a net increase in sympathoexcitation, central parasympathetic tone is reduced, driving an interest in restoring parasympathetic tone to treat disease (Olshansky et al., 2008; Ardell et al., 2016; Shivkumar et al., 2016). Using a porcine model, Vaseghi et al. (2017) reported minimal changes in acetylcholine levels in the apex, anterior wall, and lateral wall of the left ventricle in healthy animals compared to those with chronic myocardial infarction. Alterations in the basal activity and input of parasympathetic nervous within the ICNS were also evident in the setting of myocardial infarction (Vaseghi et al., 2017). Specifically, neurons normally activated by vagal stimulation were reduced in the setting of myocardial infarction, while those normally suppressed became more active. Inputs to these neurons significantly changed, with increased sympathetic input in the setting of myocardial infarction (Vaseghi et al., 2017). Changes in composition of the nodose ganglia has also been reported in the setting of chronic myocardial infarction, with increased neuron size as well as an increase in tyrosine hydroxylase positive and calcitonin gene-related peptide positive cells, with a decrease in neuronal nitric oxide synthase positive cells (Salavatian et al., 2017b). Overall, these findings suggest that intra and extra-cardiac parasympathetic remodeling occurs in the setting of myocardial infarction, with reduced parasympathetic input, but intact parasympathetic pathways (Vaseghi et al., 2017). As such, one therapeutic approach may be to target these intact pathways using vagal nerve stimulation.
Aberrant sensory information is a primary driver of acute autonomic responses to ischemic events and the progression of cardiac disease. Specific chemical ablation of cardiac transient receptor potential cation channel subfamily V member 1, TRPV1, receptors with epicardial resiniferatoxin mitigates progression of heart failure in preclinical models of ischemic heart disease (Wang et al., 2014). Targeted neuromodulation has the potential to impact sensory transduction in an on-demand and reversible fashion (Ardell et al., 2016; Salavatian et al., 2017a). Figure 3 shows the increase in nodose activity in response to transient occlusion of the left anterior descending (LAD) coronary artery. Such LAD occlusions were repeated in the presence of direct (cervical vagal, VNS) and remote (thoracic spinal cord, SCS) neuromodulation (Salavatian et al., 2017a). In both cases the nodose response to transient myocardial ischemia was mitigated (Salavatian et al., 2017a). Rather than being reflective of silent ischemia, we and others has proposed that pre-emptive neuromodulation alters the myocytes, likely via altered metabolism, and that this renders them stress-resistant (McGuirt et al., 1997; Southerland et al., 2007; Wang et al., 2014; Salavatian et al., 2016). As such the milieu is stabilized during transient ischemic events and sensory afferent responses and resultant evoked autonomic reflexes mitigated.
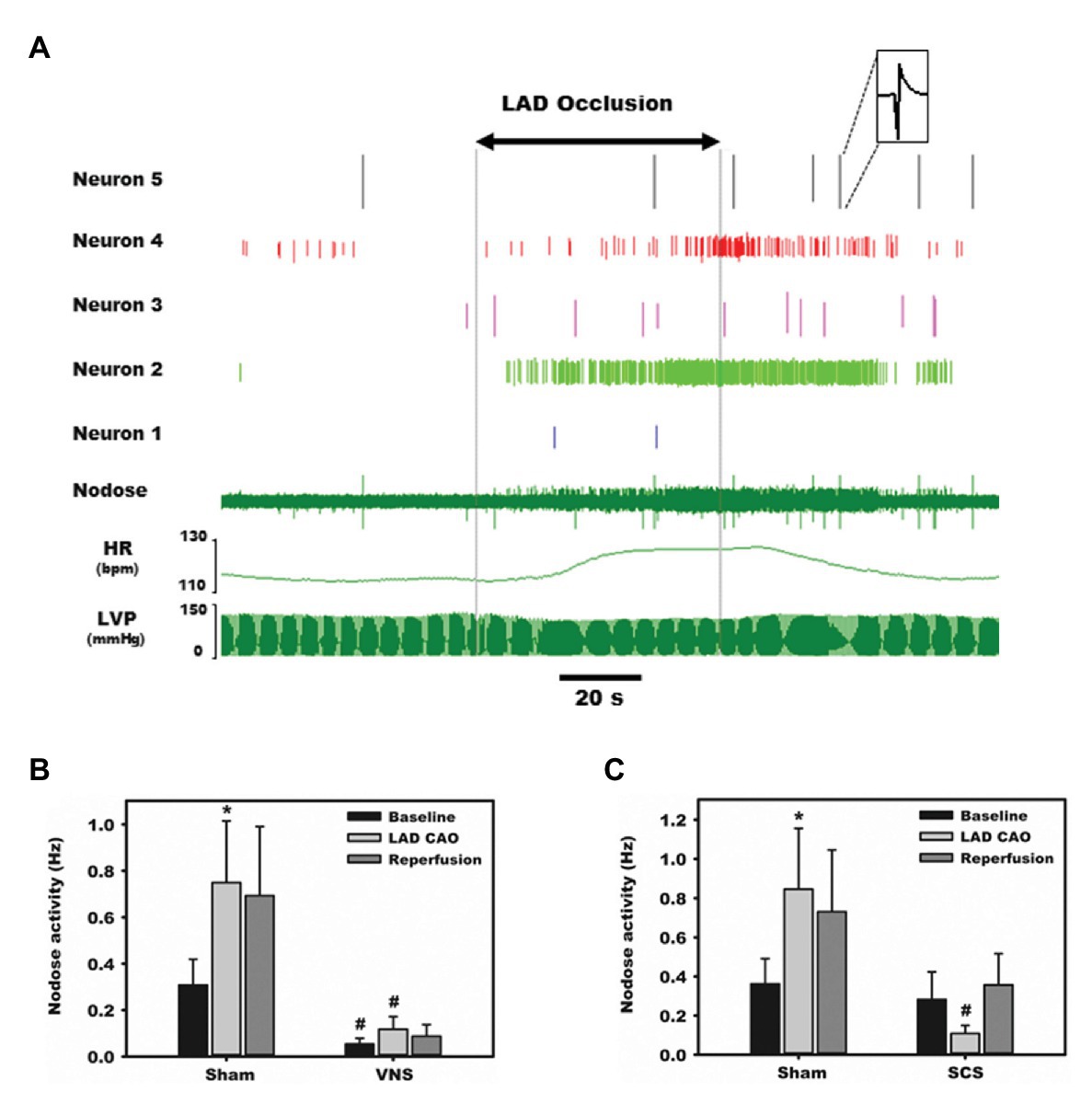
Figure 3. Afferent transduction of ischemia is modulated directly by parasympathetic input as well as remotely. Representative recordings from the nodose ganglia, one of the major parasympathetic afferent ganglia, during occlusion of the left anterior descending (LAD) coronary artery. (A) Activity from five neurons displays substantial heterogeneity in baseline firing frequency and responses to ischemia and reperfusion. (B) Normalized, summative nodose activity at baseline, with LAD artery occlusion, and reperfusion, with and without vagal nerve stimulation. In all settings, animals receiving vagal nerve stimulation (VNS) displayed marked reductions in nodose activity. (C) Spinal cord stimulation also reduces nodose neural activity in response to ischemia stress. Adapted from Salavatian et al. (2017a) with permission. *p < 0.05 from baseline; #p < 0.05 from sham.
The Intrinsic Cardiac Nervous System: Final Pathway for Cardiac Control
The ICNS is a neural network comprised of afferent, efferent, and interneurons situated in fat pads distributed along the epicardial surface of the heart (Armour, 2008). Although the ICNS has been traditionally considered a relay station for efferent input to the heart, recent studies have identified a heterogenous population of neurons with varied putative functions (Armour et al., 1990; Butler et al., 1990; Ardell et al., 1991; Armour, 2008; Cardinal et al., 2009). These afferent neurons include multimodal and nociceptive afferent neurons, identified through both functional studies and immunohistochemical evaluation using antibodies against calcitonin gene-related peptide and substance P (Rysevaite et al., 2011a,b; Pauza et al., 2014; Ardell et al., 2016). As such, the ICNS has a role in afferent sensory transduction, local network processing afferent-efferent input and can be considered as the final common pathway for input to the heart (Armour, 2008; Ardell et al., 2016). While each aggregate of neurons has a preferential sphere of influence with regards to cardiac mechanical and electrical function, there is substantial overlap in areas of the heart regulated. For example, focal activation of all ganglionated plexi results in changes in heart rate, suggesting direct and indirect input from each GP to the primary pacemaker of the heart (Figure 4; Armour, 2008; Cardinal et al., 2009). Likewise, the nicotine micro-injections of most, but not all, ganglionated plexi can result in atrioventricular block or atrial fibrillation, and most have varied effects on ventricular mechanical function and electrophysiology (Armour, 2008; Cardinal et al., 2009). These data reflects the ICNS role in overall coordination of cardiac function, as a result of local circuit neuron (LCNs) mediating intra- and interganglionic interactions, shared efferent inputs, and convergent/divergent afferent inputs (Ardell et al., 2016).
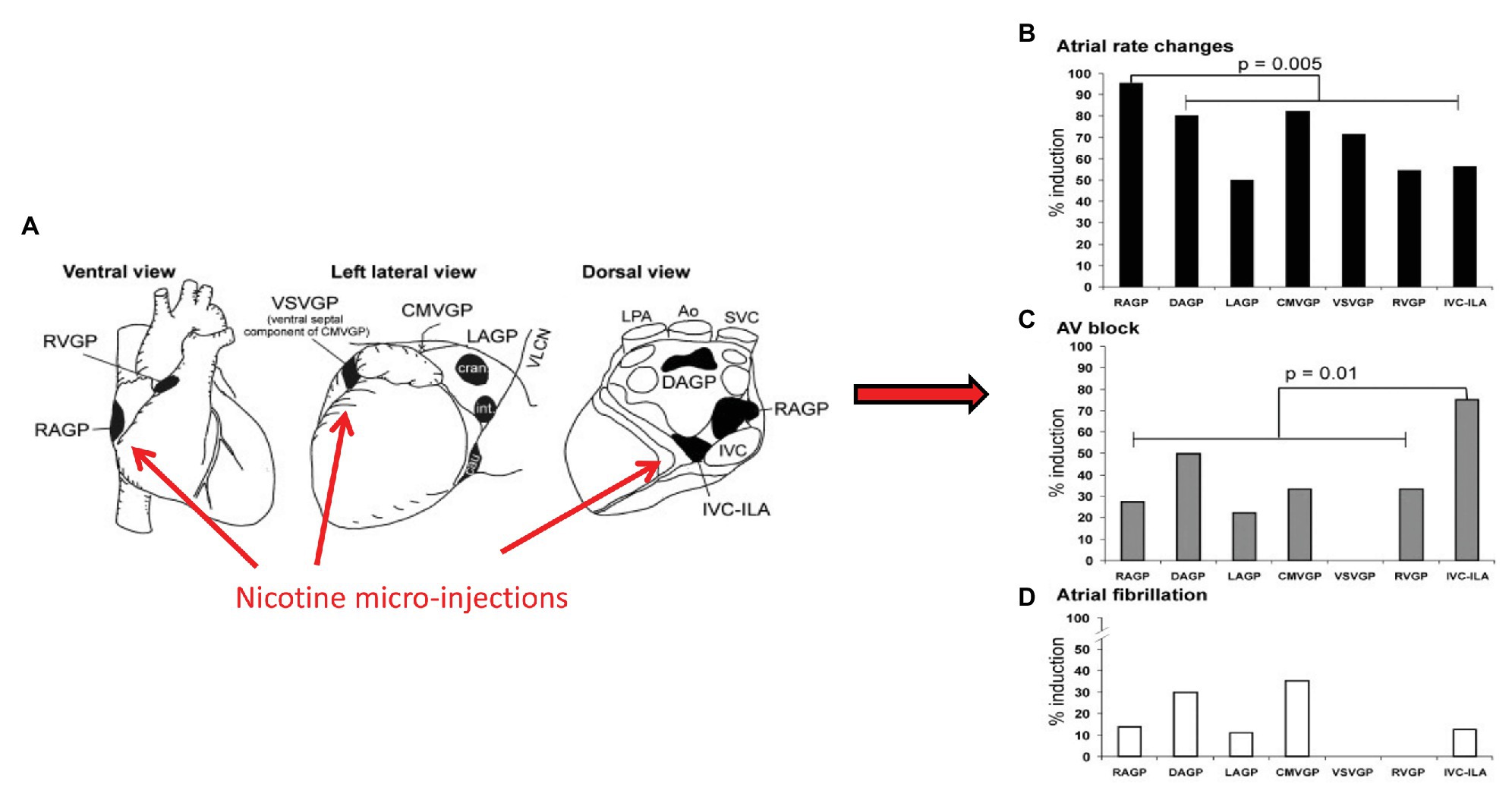
Figure 4. Aggregates of afferent, efferent, and interneurons located within epicardial fat pads comprise the ICNS. Although each ganglionated plexus has a preferred sphere of influence, substantial overlap exists. (A) Ventral, left lateral and dorsal views of the heart demonstrating common atrial and ventricular ganglionated plexi. Selected electrophysiologic effects of nicotine microinjections into major atrial and ventricular ganglionated plexi, (B) Heart rate, (C) Atrioventricular block, (D) atrial fibrillation. RVGP, right ventricular ganglionated plexus. RAGP, right atrial ganglionated plexus. VSVGP, ventral septal ventricular ganglionated plexus. CMVGP, cranial medial ventricular ganglionated plexus. LAGP, left atrial ganglionated plexus. DAGP, dorsal atrial ganglionated plexus. IVC-ILA, inferior vena cava-inferior atrial ganglionated plexus. Adapted from Cardinal et al. (2009) with permission.
Interactions between the ganglionated plexi and higher centers ultimately modulate regional cardiac function, rather than each system acting on its own (Armour, 2008; Ardell et al., 2016). The right atrial ganglionated plexus (RAGP), located posterolateral to the superior and inferior vena cava, and anterior to the right superior pulmonary vein, is one of the major atrial ganglionated plexi and mediates significant parasympathetic input to the right atrium (Ali et al., 1990; Ardell et al., 1991; Cardinal et al., 2009). In animal models, ablation of the RAGP results in near complete loss of evoked bradycardia induced by vagal nerve stimulation (Figure 5; McGuirt et al., 1997). Sequential sympathetic and combined sympathetic-parasympathetic stimulation initially results in tachycardia followed by substantial bradycardia in the intact state (McGuirt et al., 1997). As shown in Figure 5, with ablation of the RAGP, the tachycardia induced by sympathetic stimulation is preserved but returned to baseline with the addition of VNS. This residual sympathetic-parasympathetic interaction is completely abolished when atropine is added (McGuirt et al., 1997). These observations suggest that interactions occur at both the level of end-organ as well as through the ICNS, underscoring the importance of such interactions in understanding regional regulation of function (McGuirt et al., 1997; Randall et al., 2003; Ardell et al., 2016).
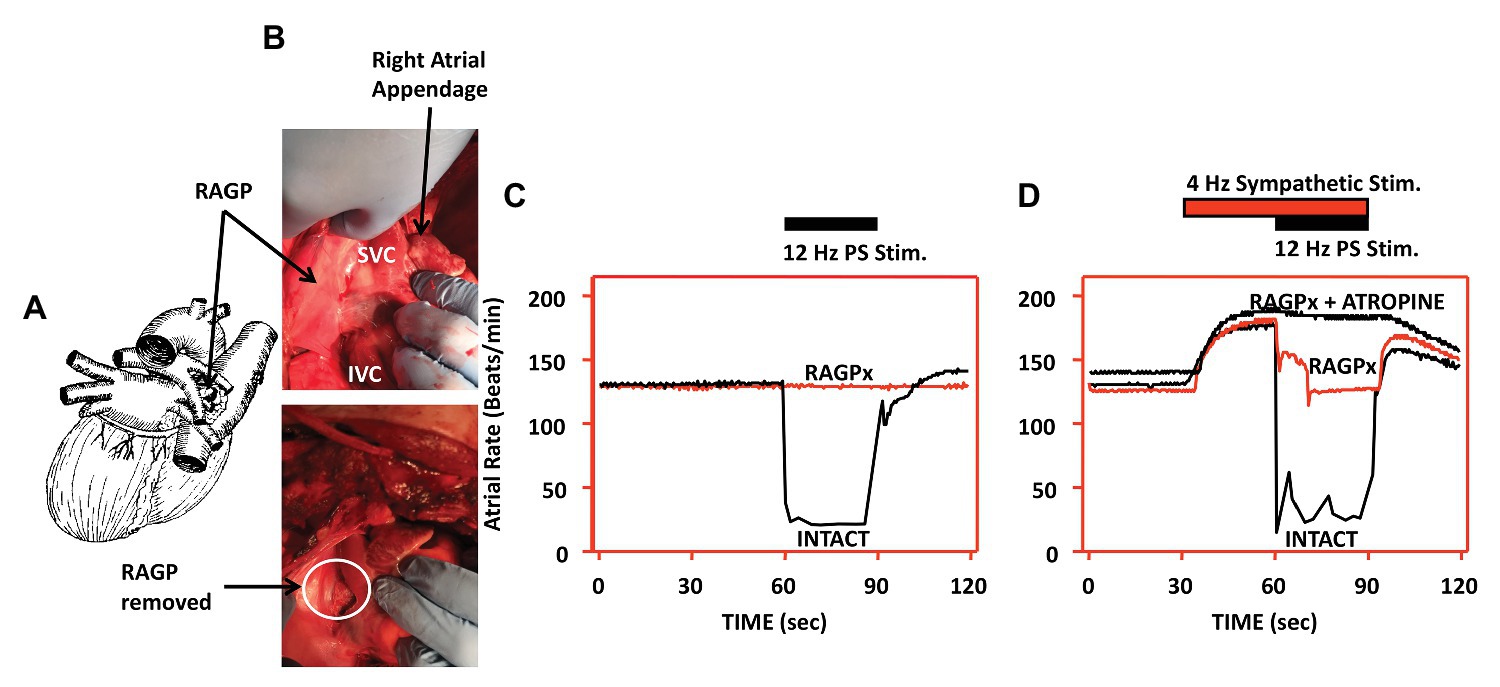
Figure 5. Interactions between sympathetic and parasympathetic system occur at end-effector as well as within the ICNS. Disruption of the RAGP alters neural control of heart rate. (A) Diagram depicting location of RAGP, posterior to the caval veins and anterior to right pulmonary artery and right superior pulmonary vein. (B) Photographs of RAGP before and after ablation. (C) Evoked bradycardia with 12 Hz parasympathetic stimulation in intact state, with loss of evoked bradycardia after RAGP ablation. (D) Combined sympathetic (4 Hz) and parasympathetic (12 Hz) stimulation in intact state, following RAGP ablation, and RAGP ablation with atropine administration. Adapted from McGuirt et al. (1997).
The ICNS has received significant attention clinically due to its accessibility with cardiac interventions or cardiac surgery (Liu et al., 2013; Linz et al., 2014; Ripplinger et al., 2016; Shivkumar et al., 2016). Although dysfunction of the ICNS has been implicated in various cardiovascular diseases, our lack of knowledge regarding interactions among ganglionated plexi and higher centers has limited the advancement of therapeutics (Ardell et al., 2016; Shivkumar et al., 2016). For example, in 2009, Nakagawa et al. (2009) reported percutaneous ablation of five accessible atrial GP in 63 patients with paroxysmal atrial fibrillation, which resulted in a marked reduction of episodes of atrial fibrillation. Further follow-up of these patients, as well as other trials, showed a greater need for pacemaker implantation, likely due to disruption of neurons projecting to the sinoatrial node, and an inadvertent increased risk of ventricular arrhythmias in the setting of ischemia (Armour et al., 1997; Lo et al., 2013).
Disruptions in autonomic inputs to the ICNS are arrhythmogenic. In a series of studies, our group developed a model for reproducible transient induction of atrial fibrillation (AF; Gibbons et al., 2012; Beaumont et al., 2013; Salavatian et al., 2016). Using this model, we recorded intrinsic cardiac neural and cardiac electrical activity in response to bursts of electrical activity delivered to cardiac mediastinal nerves during the atrial refractory period (Gibbons et al., 2012; Beaumont et al., 2013; Salavatian et al., 2016). Such bipolar stimulation reproducibly produces short periods of AF (~30 duration) with a latency of ~1 s (Figure 6). ICNS activity, derived from extracellular recording, can be functionally defined into afferent, efferent or convergent (both afferent and efferent) related. While burst stimulation of mediastinal nerves increases activity in all three functional classes of ICNS neurons, convergent neurons were the preferential target. As demonstrated in Figure 6, pre-emptive VNS reduces the evoked ICNS neural response to mediastinal nerve stimulation, with the primary target being the convergent neurons, and the atrial arrhythmia potential was reduced in 75% of stimulations. The VNS impact on ICNS network function is further exemplified by mitigating synchrony between efferent to convergent and convergent to convergent neurons (Figure 6, Panels D,E). Finally, VNS exhibits memory (Figure 7), specifically, 3 min of cervical VNS reduced the arrhythmia potential to neural imbalances produced by mediastinal nerve stimulation for approximately 30 min. This has important implications for design of stimulation protocols for VNS.
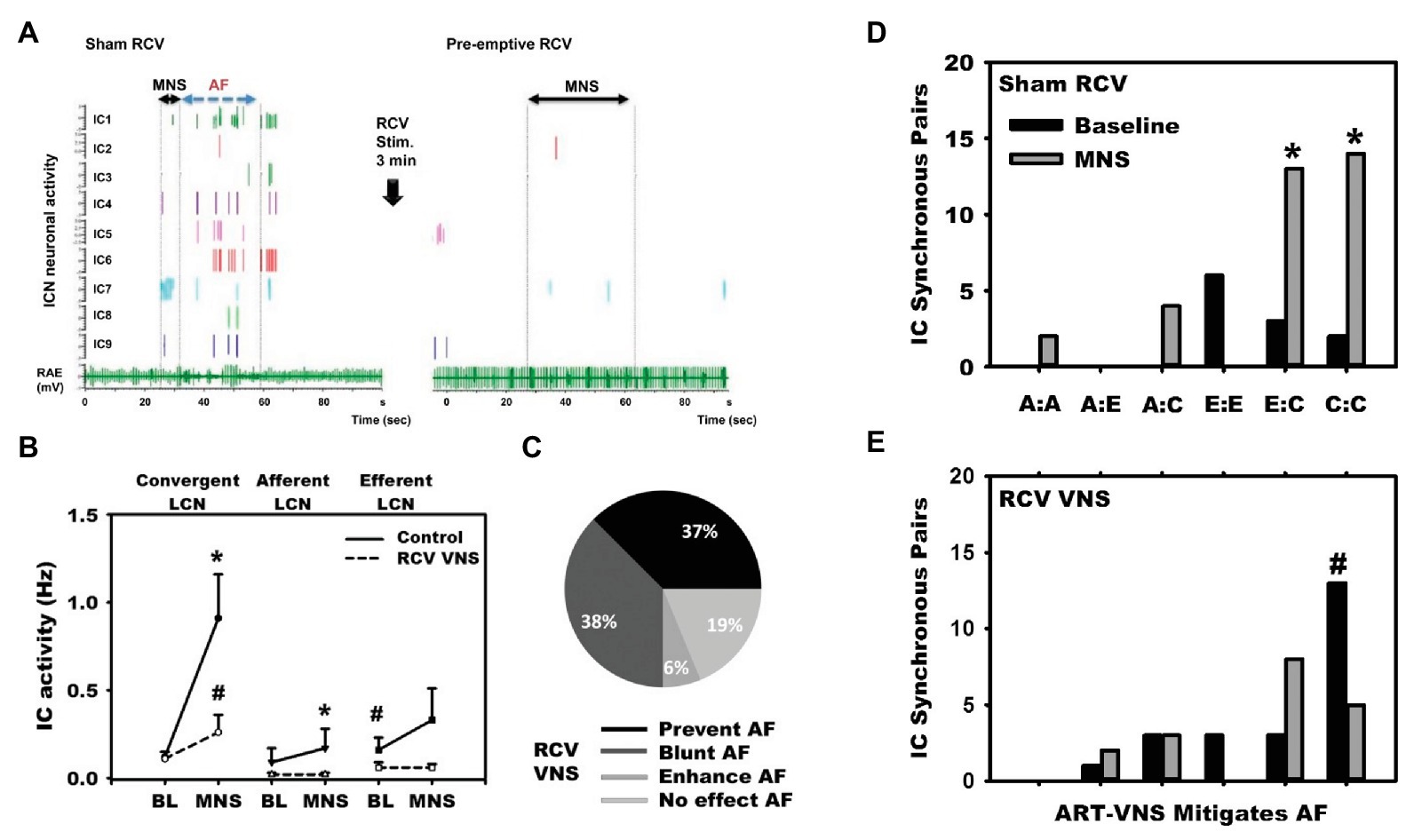
Figure 6. Vagal nerve stimulation targets LCNs within the ICNS to reduce arrhythmogenesis. (A) In response to mediastinal nerve stimulation, activity within the RAGP significantly increased and transient periods of AF were induced. (B) Increased neural activity during mediastinal nerve stimulation was primarily driven by increased activity of LCNs. (C) VNS mitigated neural activity changes in response to mediastinal nerve stimulation and prevented or blunted atrial arrhythmogenesis. (D) and (E) Intrinsic cardiac neurons were classified as afferent (A), efferent (E), or convergent (C). Mediastinal nerve stimulation resulted in increases in synchrony between efferent to convergent pairs and convergent to convergent pairs, while preemptive right cervical vagus nerve stimulation prevented such. Adapted from Salavatian et al. (2016). (B): *p < 0.05 from baseline; #p < 0.05 from control (sham VNS). (D) and (E): *p < 0.05 from baseline; #p < 0.01 sham to RCV VNS state.
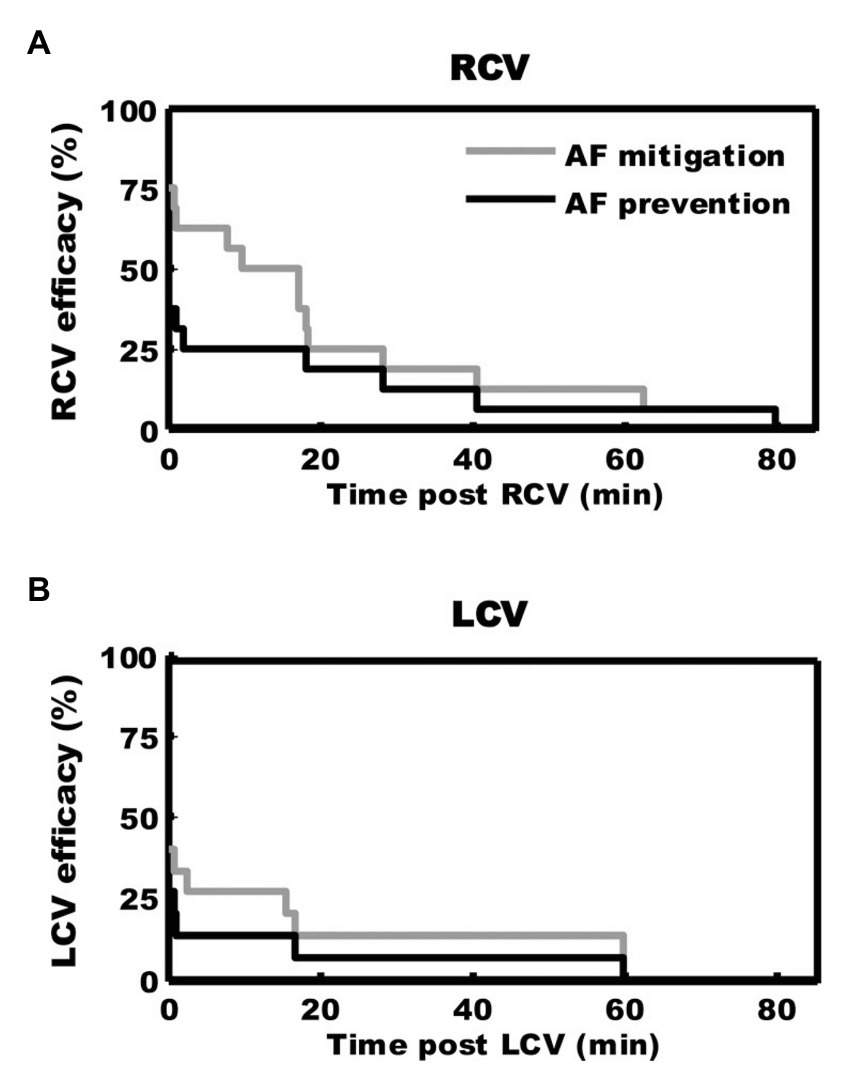
Figure 7. Kaplan-Meier curves demonstrating duration of antiarrhythmic effects of 3 min of preemptive cervical vagal nerve stimulation. After right (A) or left (B) sided vagal nerve stimulation, mediastinal nerve stimulation was less effective in inducing atrial fibrillation for approximately 30 min. Adapted from Salavatian et al. (2016).
VNS for Treatment of Cardiovascular Disease: Pre-Clinical Studies
Preclinical studies have provided substantial evidence supporting the utility of chronic VNS for the management of various cardiovascular disorders, with majority of studies focused on heart failure (Buckley et al., 2015; Schwartz et al., 2015). In a rat model of chronic heart failure induced by myocardial infarction through ligation of left coronary artery, 6 weeks of right vagal nerve stimulation ameliorated adverse ventricular remodeling and improved contractility (Li et al., 2004). Rats treated with VNS had reduced plasma norepinephrine and brain natriuretic peptide levels, and a 73% reduction in relative risk of death (Li et al., 2004). In a canine model of ventricular tachypacing-induced heart failure, concomitant right vagal nerve stimulation, at an intensity to reduce heart rate by 20 beats per minute, attenuated the development of heart failure (Zhang et al., 2009). Canine receiving concomitant VNS had improved LV ejection fraction and lower end-diastolic volumes, with greater heart rate variability and baroreflex sensitivity (Zhang et al., 2009). In a guinea pig model of chronic pressure overload designed to induce hypertension-mediated changes, chronic vagal nerve stimulation mitigated the development of cardiac hypertrophy and adverse neural remodeling (Figure 8; Beaumont et al., 2016). Compared to animals receiving sham VNS, those receiving right or left cervical vagal nerve stimulation had reduced pressure overload-induced remodeling, including a reduction in left ventricular internal diameter at diastole, left ventricular end diastolic volume, and cardiac output (Beaumont et al., 2016). At a cellular level, VNS consistently reduced pressure overload-induced myocyte hypertrophy and remodeling within intrinsic cardiac neurons (Beaumont et al., 2016). Taken together, these animal models suggest vagal nerve stimulation can modulate pathologic responses that result in heart failure, in part through improved autonomic balance, reduction of inflammation, alteration of energy utilization, and modulation of apoptotic pathways (Zhang et al., 2009; Zhao et al., 2013; Beaumont et al., 2016).
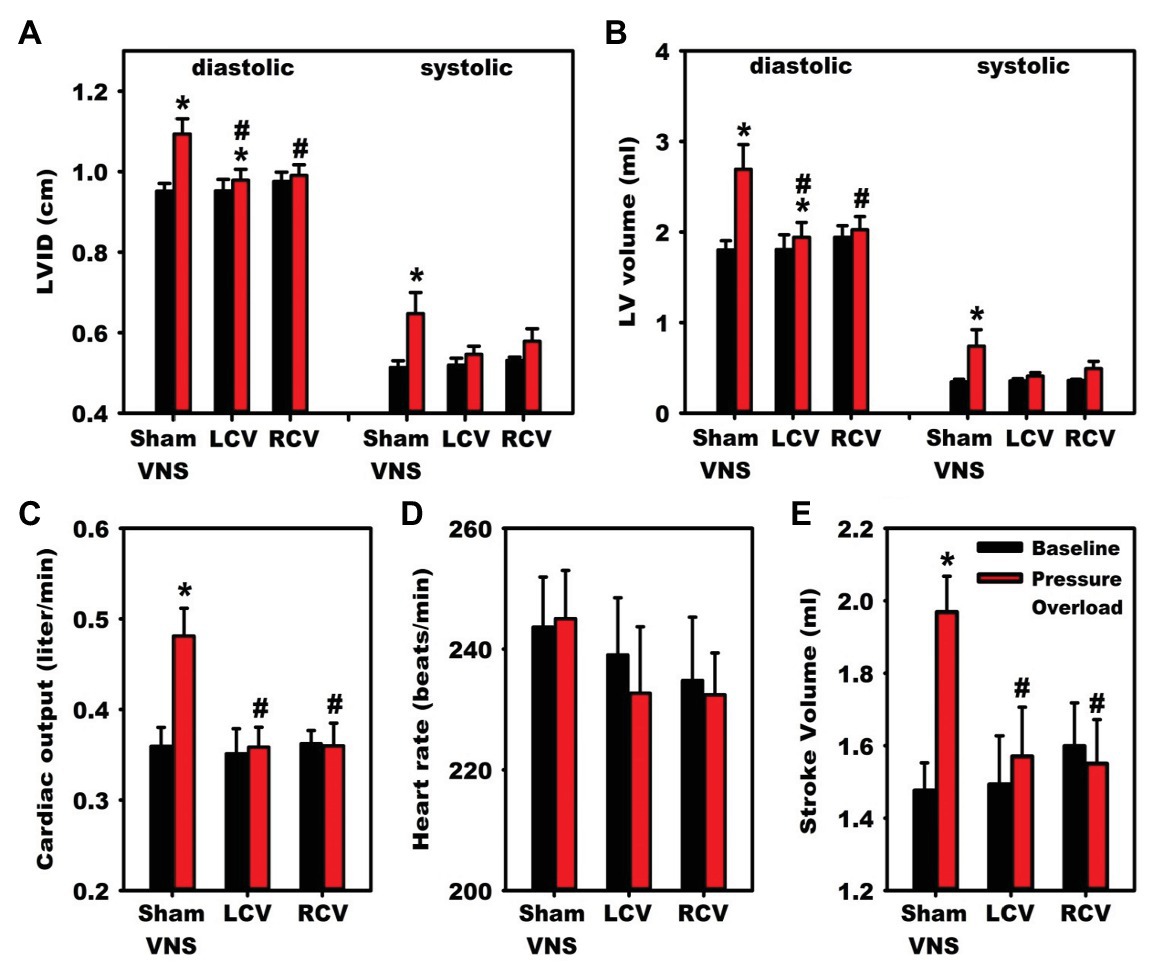
Figure 8. Vagal nerve stimulation mitigates heart failure and cardiac hypertrophy in a guinea pig model of chronic pressure-overload induced by aortic constriction. Compared to animals receiving sham vagal nerve stimulation, those receiving right or left sided VNS had improvements in indices of heart failure. Significant improvements were noted in diastolic indices of (A) left ventricular (LV) internal diameter and (B) LV volume. (C) Cardiac output and (E) stroke volume normalized to baseline with left and right vagal nerve stimulation, with no changes in (D) Heart Rate. LCV, left cervical vagus stimulation. RCV, right cervical vagus stimulation. VNS, vagus nerve stimulation. LV, left ventricular. LVID, Left ventricular internal diameter. Adapted from Beaumont et al. (2016). *p < 0.05 from baseline; #p < 0.05 to sham VNS.
Preclinical studies have demonstrated putative anti-arrhythmic effects of vagal nerve stimulation in the setting of acute (Figures 6, 7) and chronic myocardial ischemia. In a study of acute coronary artery occlusion in anesthetized cats, animals who had previously undergone bilateral vagotomy or received atropine had less ventricular arrhythmias and death, suggesting that either efferent or afferent vagal tone may reduce ventricular fibrillation and death (Corr and Gillis, 1974). Billman et al. (1982) demonstrated that high vagal reflexes, assessed through baroreflex slopes, were associated with reduced vulnerability to ventricular fibrillation during acute coronary artery occlusion in canine with a history of healed myocardial infarction. Similarly, animals that were initially susceptible to ventricular fibrillation and underwent daily exercise had improved baroreflexes and reduced susceptibility to ventricular fibrillation, attributed to improved autonomic balance (Billman et al., 1984). In a canine model of healed anterior wall myocardial infarction, acute vagal nerve stimulation reduced the frequency of ventricular fibrillation in the setting of acute coronary artery occlusion and treadmill exercise among animals who were susceptible to ventricular fibrillation (Vanoli et al., 1991). In a similar study, canine who were resistant to ventricular fibrillation received atropine prior to coronary artery occlusion, resulting in either novel occurrence of ventricular arrhythmias or worsened degree of ventricular arrhythmias (De Ferrari et al., 1991). Taken together, these early studies support a putative benefit of vagal nerve stimulation and restoration of vagal tone in the setting of acute myocardial ischemia. In a rat model of acute coronary ischemia, concomitant vagal nerve stimulation reduced ventricular tachyarrythmias, with abrogation of this effect with atropine administration (Ando et al., 2005). Immunohistochemical studies showed that the vagal nerve stimulation mitigated the MI-induced loss of Connexin-43 at gap junctions, suggesting that vagal nerve stimulation may combat electrical instability that occurs with myocardial ischemia (Ando et al., 2005). In a contemporary, porcine study utilizing neuronal recordings from the ventral interventricular ganglionated plexus (VIVGP), a portion of the ICNS that innervates the ventricular myocardium, myocardial infarction resulted in increased sympathetic input to parasympathetic neurons in the VIVGP, with maintenance of parasympathetic neuronal networks. (Vaseghi et al., 2017) In this model, vagal nerve stimulation reduced the burden of ventricular arrhythmias and reduced heterogeneity of repolarization in the scar-border zone, an area known to initiate arrhythmias clinically (Rajendran et al., 2016). This study, and others, suggests that vagal nerve stimulation may, in part, act by stabilizing myocardial electrical activity, particularly at the scar-border zone.
Although the therapeutic molecular and cellular mechanisms underlying the therapeutic efficacy of vagal nerve stimulation are incompletely understood, mechanisms are thought to be mediated through both efferent activation of muscarinic receptors at the nerve-myocyte interface, as well as interaction with higher order systems and modulation of afferent-mediated reflexes (Figure 9; Ardell et al., 2016). At the level of the brainstem, afferent input is modified resulting in reduced central drive, as well as modulation of brainstem projections to spinal sympathetic networks (Ardell et al., 2016). At the level of the heart, activation of vagal efferent fibers results in acetylcholine release, activating M2 receptors on myocytes and conduction system cells, resulting in negative chronotropy, dromotropy, and, to a lesser degree, inotropy (Janig, 2016). VNS may, in part, alter the balance between energy demand and supply in the setting of pathologic states (Sabbah et al., 2011; De Ferrari, 2014). Coronary blood flow is modulated by vagal nerve stimulation with the majority of studies demonstrating parasympathetic coronary vasodilation although direct application of acetylcholine to vascular smooth muscle cells results in vasoconstriction (Winbury and Green, 1952; Schofield and Walker, 1953; Feigl, 1983). In a canine model employing bilateral vagotomies and bilateral stellectomy, efferent vagal nerve stimulation resulted in coronary vasodilation when controlling for heart rate and aortic pressure, which was partly mitigated by atropine administration (Feigl, 1969). At the level of myocytes as well as the ICNS, vagal nerve stimulation reduces sympathetic input, which may combat sympathoexcitation induced by disease states (Levy and Martin, 1979; Ardell et al., 2016; Vaseghi et al., 2017). On a molecular level, vagal nerve stimulation has been associated with reduced pro-inflammatory cytokine release and improved nitric oxide signaling (Tracey, 2007; Ruble et al., 2010; Calvillo et al., 2011; Sabbah, 2011). In addition, factors implicated in the progression of heart failure, such as norepinephrine and angiotensin are reduced with vagal nerve stimulation (Zhang et al., 2009).
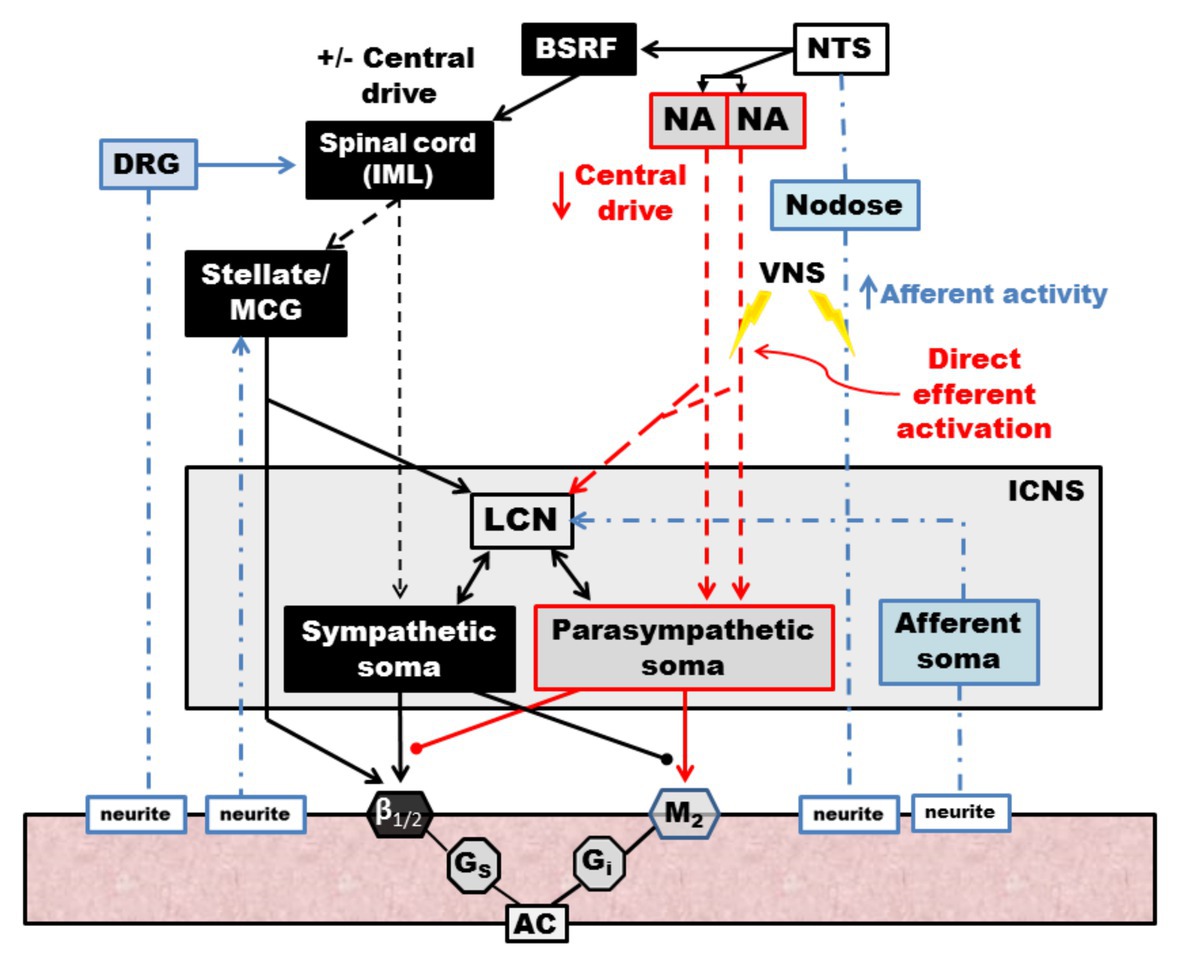
Figure 9. Schematic diagram of the effects of vagal nerve stimulation on the cardiac neuraxis. Direct efferent activation of the vagus nerve results in activation of parasympathetic postganglionic cells, located in the ICNS, which act on muscarinic receptors on the myocardium. LCNs within the ICN are activated, which modulate sympathetic reflexes with input from afferent soma, ultimately blunting reflexes within the ICNS. Afferent activation results in reduced central drive and modulates projections to spinal sympathetic networks. Sympathetic spinal reflexes are also blunted via inhibitory projections (not shown). NTS, nucleus tractus solitarus. NA, nucleus ambiguus. BSRF, brain stem reticular formation. VNS, vagal nerve stimulation. DRG, dorsal root ganglia. IML, intermediolateral nucleus. MCG, middle cervical ganglia. LCN, local circuit neuron. ICNS, intrinsic cardiac nervous system. AC, adenylyl cyclase. Adapted from Ardell et al. (2015).
VNS for Treatment of Cardiovascular Disease: Clinical Trials
Congestive heart failure is a major public health problem in the United States, with greater than 6 million Americans affected, with an increasing prevalence in the last decade (Virani et al., 2020). Despite advances in medical therapies and interventional and surgical strategies for heart failure and comorbid conditions, mortality rates remain high as do the frequency of rehospitalizations for heart failure and associated costs (Benjamin et al., 2019).
Heart failure is a heterogenous disorder, resulting from various entities including myocardial ischemia and valvular diseases, which ultimately result in an inability to meet oxygen and metabolic demands (Fukuda et al., 2015; Gloschat et al., 2016). Homeostatic humoral regulatory mechanisms, such as the renin-angiotensin-aldosterone system, are activated in an attempt to maintain cardiac output and end-organ perfusion. Importantly, imbalance the sympathetic and parasympathetic nervous systems develop and contribute to the pathogenesis of heart failure, with, in general, withdrawal of parasympathetic tone and heightening of sympathetic tone (Olshansky et al., 2008; Triposkiadis et al., 2009; Fukuda et al., 2015; Gloschat et al., 2016). Consistent with this, the Autonomic Tone and Reflexes After Myocardial Infarction (ATRAMI) study reported an association between impaired vagal reflexes and mortality among 1,284 patients with a history of myocardial infarction, independent of left ventricular ejection fraction (La Rovere et al., 1998). The majority of pharmacologic therapy for heart failure targets changes in neurohormonal axis, including beta-blockers and angiotensin-converting enzyme (ACE) inhibitors (Triposkiadis et al., 2009). Guideline directed medical therapy for most patients with heart failure with reduced ejection includes beta-blockers and ACE inhibitors or aldosterone receptor antagonists (Yancy et al., 2013). Large scale randomized trials demonstrate an improvement in survival for ACE inhibitors and beta-blockers, particularly in those with a history of myocardial infarction or reduced ejection fraction (Pfeffer et al., 1992; Vantrimpont et al., 1997; Dargie, 2001; Verdecchia et al., 2009). Although these agents have been efficacious in the management of heart failure, progression of disease generally continues, leading to renewed interest in neuromodulatory therapies (Shivkumar et al., 2016).
Vagal nerve stimulation was initially developed for and clinically utilized for the management of depression and refractory epilepsy (Aaronson et al., 2013; Arle et al., 2016). Though the mechanisms of VNS for epilepsy are poorly characterized, reduction in seizure frequency and use of anti-epileptic drugs has been noted across trials (Ben-Menachem et al., 2015). The Food and Drug Administration has already approved implantable pulse generators and leads for safe and effective treatment of epilepsy, facilitating the adaptation of similar technology to alternate diseases (Ben-Menachem et al., 2015; Anand et al., 2019). Given the relevance of autonomic dysregulation in the development and progression of heart failure and arrhythmias, trials exploring the efficacy and safety of VNS for cardiovascular disease have received significant national attention since the initial report of its use for heart failure in 2008 (Schwartz et al., 2008; De Ferrari et al., 2011; Premchand et al., 2014; Gold et al., 2016; De Ferrari et al., 2017). Initial clinical trials of VNS, in combination with medical therapy, for CHF demonstrated mixed results, attributed to variation in study design, sidedness of stimulation, and vagal nerve stimulation protocols (Anand et al., 2020). Importantly, VNS protocols represent the total dose of “therapy” delivered to the patient as a function of current intensity, frequency, pulse width, and duty cycle (time on vs. off), and thus are hypothesized to have a substantial impact on efficacy of therapy, as well as side effects (Anand et al., 2020).
The Autonomic Neural Therapy to Enhance Myocardial Function in Heart Failure (ANTHEM-HF) evaluated the adjunct use of the Demipulse (LivaNova, Houston, TX) VNS system for left or right cervical vagal nerve stimulation in a cohort of patients with heart failure with reduced ejection fraction and New York Heart Association functional class II-III symptoms (Premchand et al., 2016; Dicarlo et al., 2018; Konstam et al., 2019; Sharma et al., 2020). Improvement in primary endpoints including left ventricular ejection fraction, heart rate variability, and 6-min walk tests, were noted, with follow-up at 1 year demonstrating persistent improvement (Figure 10; Premchand et al., 2016; Dicarlo et al., 2018; Konstam et al., 2019; Sharma et al., 2020). In the setting of heart failure with preserved ejection fraction (HFpEF), a more clinically challenging entity to treat, a similar VNS protocol by the same investigators is presently enrolling (Dicarlo et al., 2018). Of existing and completed trials for CHF, ANTHEM-HF is the only trial reporting positive findings for both safety and efficacy of VNS, which has been attributed to both study design and VNS stimulation protocols (Anand et al., 2020).
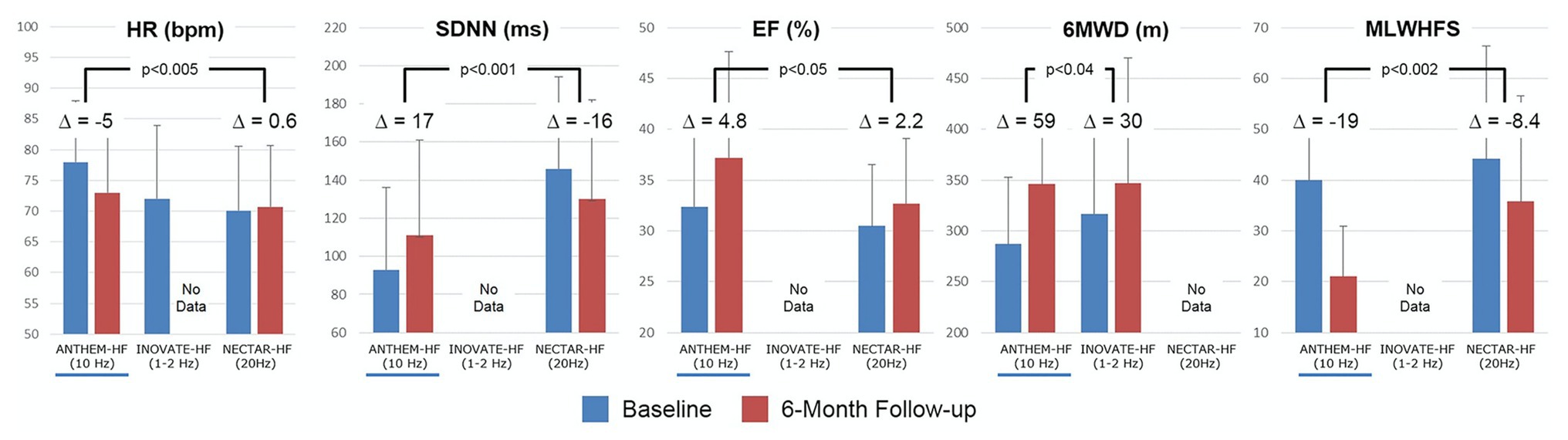
Figure 10. Clinical responses for the three major vagus nerve stimulation trials for HFrEF at baseline (blue) and 6-month follow up (red). Improvement for most parameters were noted for ANTHEM-HF, but unstudied or not met for INOVATE-HF or NECTAR-HF. Outcomes included heart rate (HR), standard deviation of NN intervals (SDNN, a heart rate variability time domain measure), ejection fraction, 6 minute walking distance test, and minnesota living with heart failure score. Adapted from Anand et al. (2020) with permission.
Two other major studies have explored the impact of VNS on cardiovascular outcomes in the setting of heart failure, with mixed results (Figure 10). The NEural Cardiac TherApy foR HF (NECTAR-HF) study randomized patients with heart failure with reduced ejection fraction (HFrEF) and left ventricular ejection fraction (LVEF) ≤ 35% to VNS therapy or sham (device without stimulation) with a different VNS protocol (intensity titrated to 20 Hz, 300 μs, proposed target current of 4 mA; Zannad et al., 2015). At 6-months, the majority of patients did not achieve the pre-specified stimulus intensity because of off-target effects and there was no significant difference in pre-specified outcomes of left ventricular end systolic diameter (Zannad et al., 2015). Importantly, NECTAR-HF targeted vagal nerve stimulation at a frequency of 20 Hz and a low current (Zannad et al., 2015). At these stimulation parameters, it is hypothesized that afferent fibers were preferentially activated, rather than efferent motor fibers, which may, in part, explain the lack of efficacy observed (De Ferrari et al., 2017; Anand et al., 2020).
The INcrease Of VAgal TonE in Heart Failure (INOVATE-HF) trial randomized 707 patients with HFrEF (LVEF ≤ 40%) with NYHA class III symptoms to medical therapy or medical therapy and VNS using an R-wave triggered pulse (Gold et al., 2016). Of note, this trial utilized a vagal nerve stimulation protocol that implemented a putative afferent block in addition to efferent activation (Gold et al., 2016). At a mean follow-up of 16 months, a composite primary endpoint of death, rehospitalization for CHF, or need for intravenous diuresis, was more frequent in the VNS group compared to control (Gold et al., 2016). About 6-min walk test and quality of life measures improved in the VNS group compared to control (Figure 10; Gold et al., 2016). The mixed findings in both of these studies may represent heterogenous cohorts and inadequate or inappropriately tailored VNS therapy delivery (Anand et al., 2020).
Our prior work has described the concept of the neural fulcrum – an equilibrium point between activation of afferent and efferent vagus nerve pathways, resulting in a null heart response to stimulation with intact neural circuits (Ardell et al., 2017). Titration of vagal nerve stimulation parameters to reach the neural fulcrum over a period of time is a feasible approach to achieve therapeutic levels of VNS (Ardell et al., 2017; Anand et al., 2020). Likewise, adjustment of parameters during a training period, off-target effects such as cough, GI discomfort, and dyspnea, can be minimized (Ardell et al., 2017; Anand et al., 2020). Figure 11 represents a graphical display of a frequency and intensity relationship for VNS, with the neural fulcrum defined as the minimal heart rate change with VNS (yellow shaded region), overlaid with parameters from the three previously discussed trials of VNS for heart failure. At a given pulse width, in this case 500 μs, notes the inverse relationship between frequency and intensity to achieve a null and minimal bradycardia (1–3 beats per minute; Figure 11). Both NECTAR-HF and INOVATE-HF trials delivered VNS at parameters that were not effective in engaging all levels of the cardiac nervous system, while ANTHEM-HF operated at the fulcrum (Ardell et al., 2017; Anand et al., 2020). ANTHEM-HF is presently the only trial has shown a net long-term benefit to VNS for heart failure, which may be related to activating the appropriate balance of afferent and efferent fibers (Ardell et al., 2016, 2017; Anand et al., 2020). Although there were significant variation in clinical characteristics, disease burden, and comorbidities including arrhythmias, in these three trials, it is likely that the choice of VNS likely impacted clinical responses (Ardell et al., 2016, 2017; Anand et al., 2020). As future trials of VNS are designed for heart failure and other cardiovascular disorders, it is imperative that structure–function relationships of the nervous system be considered.
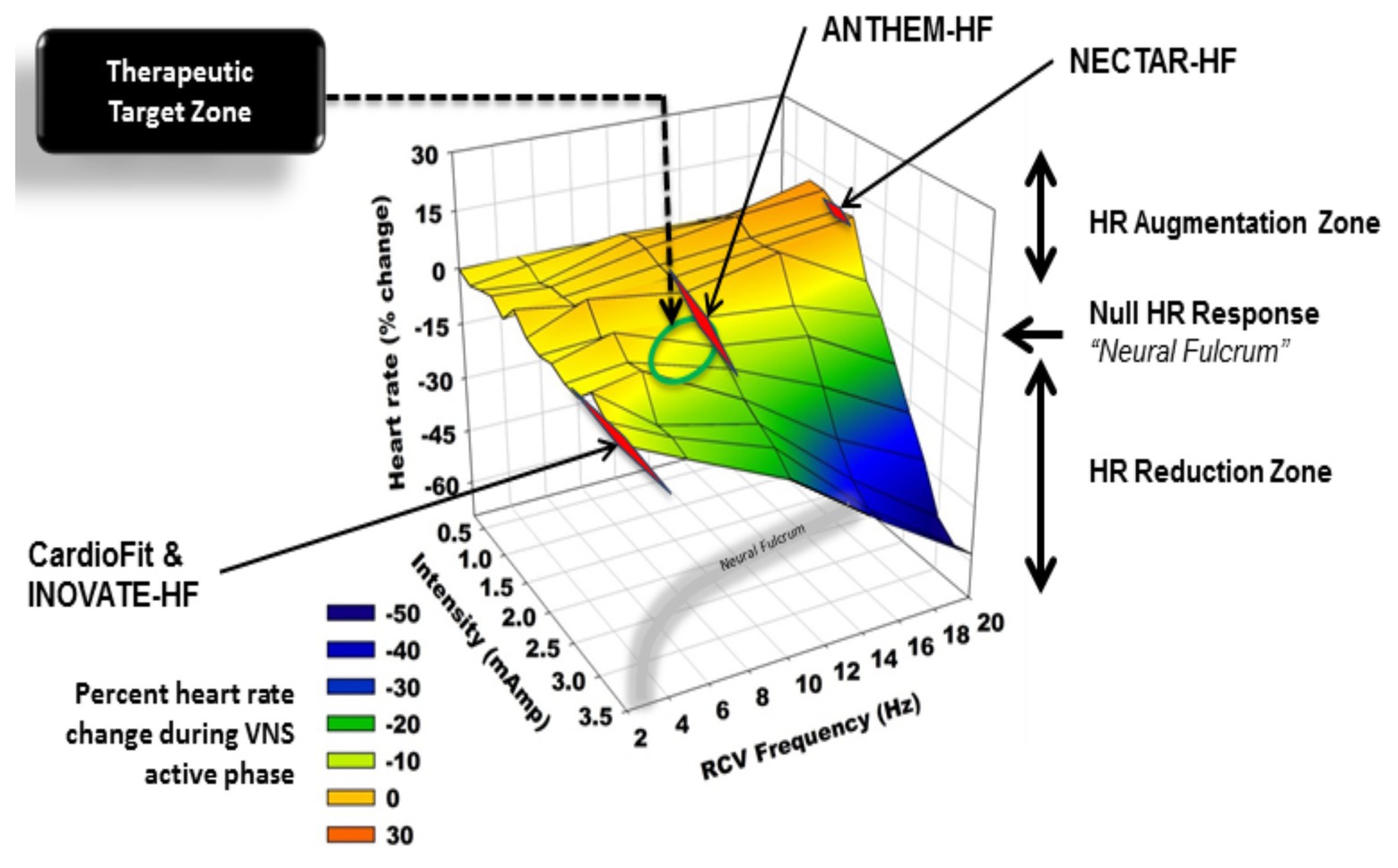
Figure 11. Heart rate responses to right cervical vagus nerve stimulation in a canine model at varying frequency and intensity (pulse width 500 μs). At higher frequencies and current, vagal nerve stimulation results in predominant activation of vagomotor efferent fibers, producing a bradycardia (blue). At higher frequencies and lower current, vagal nerve stimulation results in a relative tachycardia due to preferential activation of afferent fibers (orange, red). The area at which a null heart rate response is achieved is the neural fulcrum (yellow surface). Achieved stimulation parameters for three vagus nerve stimulation trials (ANTHEM-HF, NECTAR-HF, and INOVATE-HF) are plotted as a function of frequency and intensity. Adapted from Ardell et al. (2017) with permission.
Concluding Remarks and Future Directions
Multiple cardiovascular disorders including myocardial infarction and congestive heart failure result in excessive sympatoexcitation and a withdrawal of central parasympathetic tone (Ardell et al., 2016; Shivkumar et al., 2016). The majority of existing therapies for these disorders focus on blunting sympathetic drive to the heart, in the form of beta-adrenergic receptor blockade or inhibition of the renin-angiotensin-aldosterone axis (Shivkumar et al., 2016). Despite the widespread use of these medications, progression of disease occurs, creating a need for novel therapeutic approaches (Shivkumar et al., 2016). Therapies such as cardiac sympathetic denervation, stellate ganglion blockade, and renal artery denervation are being explored as avenues to reduce sympathetic tone associated with heart failure and ventricular arrhythmias (Shivkumar et al., 2016). In addition to the value of these therapies in the management of refractory ventricular arrhythmias, cardiac sympathetic denervation has also shown efficacy in the setting of inherited channelopathies including congenital long QT syndrome and catecholaminergic polymorphic ventricular tachycardia (Schwartz et al., 2004; Schwartz, 2014; De Ferrari et al., 2015). Vagal nerve stimulation has emerged as a promising therapeutic approach to combat the observed withdrawal of parasympathetic tone and to counteract the reflex mediated sympathoexcitation (Ardell et al., 2016; Shivkumar et al., 2016). A significant body of pre-clinical studies supports the value of vagal nerve stimulation for the management of heart failure or arrhythmias (Ardell et al., 2016; Shivkumar et al., 2016). Existing trials of vagal nerve stimulation have been confounded by substantial variation in inclusion criteria and inconsistent vagal nerve stimulation delivery (Ardell et al., 2016; Shivkumar et al., 2016; Anand et al., 2020). A mechanistic understanding of neural cardiac remodeling, where and how to target the cardiac neuraxis and follow-up with appropriate biomarkers is essential to achieve and maintain therapeutic efficacy. The goal for any of these therapies is to reduce sympathoexcitation, increase parasympathetic tone and/or blunt aberrant afferent processing. VNS achieves all three goals concurrently and remains an area of intense scientific and clinical interest.
Author Contributions
JH and JLA contributed to writing, reviewing, and editing of the manuscript. Both the authors approved the submitted version.
Funding
This work was funded by the National Institutes of Health, Office of The Director 3OT2OD023848 and U01EB025138.
Conflict of Interest
University of California, Los Angeles has patents developed by JLA relating to cardiac neural diagnostics and therapeutics. JLA is a co-founder of NeuCures, Inc.
The remaining author declares that the research was conducted in the absence of any commercial or financial relationships that could be construed as a potential conflict of interest.
References
Aaronson, S. T., Carpenter, L. L., Conway, C. R., Reimherr, F. W., Lisanby, S. H., Schwartz, T. L., et al. (2013). Vagus nerve stimulation therapy randomized to different amounts of electrical charge for treatment-resistant depression: acute and chronic effects. Brain Stimul. 6, 631–640. doi: 10.1016/j.brs.2012.09.013
Ajijola, O. A., Chatterjee, N. A., Gonzales, M. J., Gornbein, J., Liu, K., Li, D., et al. (2019). Coronary sinus neuropeptide Y levels and adverse outcomes in patients with stable chronic heart failure. JAMA Cardiol. 5, 318–325. doi: 10.1001/jamacardio.2019.4717
Ajijola, O. A., Hoover, D. B., Simerly, T. M., Brown, T. C., Yanagawa, J., Biniwale, R. M., et al. (2017). Inflammation, oxidative stress, and glial cell activation characterize stellate ganglia from humans with electrical storm. JCI Insight 2:e94715. doi: 10.1172/jci.insight.94715
Ajijola, O. A., Vaseghi, M., Zhou, W., Yamakawa, K., Benharash, P., Hadaya, J., et al. (2013). Functional differences between junctional and extrajunctional adrenergic receptor activation in mammalian ventricle. Am. J. Physiol. Heart Circ. Physiol. 304, H579–H588. doi: 10.1152/ajpheart.00754.2012
Ajijola, O. A., Wisco, J. J., Lambert, H. W., Mahajan, A., Stark, E., Fishbein, M. C., et al. (2012). Extracardiac neural remodeling in humans with cardiomyopathy. Circ. Arrhythm. Electrophysiol. 5, 1010–1116. doi: 10.1161/CIRCEP.112.972836
Ajijola, O. A., Yagishita, D., Reddy, N. K., Yamakawa, K., Vaseghi, M., Downs, A. M., et al. (2015). Remodeling of stellate ganglion neurons after spatially targeted myocardial infarction: neuropeptide and morphologic changes. Heart Rhythm 12, 1027–1035. doi: 10.1016/j.hrthm.2015.01.045
Ali, I. M., Butler, C. K., Armour, J. A., and Murphy, D. A. (1990). Modification of supraventricular tachyarrhythmias by stimulating atrial neurons. Ann. Thorac. Surg. 50, 251–256. doi: 10.1016/0003-4975(90)90744-Q
Anand, I. S., Konstam, M. A., Ardell, J. L., Libbus, I., Dicarlo, L., and Mann, D. L. (2019). Neuromodulation for drug-refractory epilepsy and chronic heart failure: targets, delivery, composition and titration. Int. J. Neurol. Neurother. 6, 1–12. doi: 10.23937/2378-3001/1410091
Anand, I. S., Konstam, M. A., Klein, H. U., Mann, D. L., Ardell, J. L., Gregory, D. D., et al. (2020). Comparison of symptomatic and functional responses to vagus nerve stimulation in ANTHEM-HF, INOVATE-HF, and NECTAR-HF. ESC Heart Fail. 7, 75–83. doi: 10.1002/ehf2.12592
Ando, M., Katare, R. G., Kakinuma, Y., Zhang, D., Yamasaki, F., Muramoto, K., et al. (2005). Efferent vagal nerve stimulation protects heart against ischemia-induced arrhythmias by preserving connexin43 protein. Circulation 112, 164–170. doi: 10.1161/CIRCULATIONAHA.104.525493
Ardell, J. L., Andresen, M. C., Armour, J. A., Billman, G. E., Chen, P. S., Foreman, R. D., et al. (2016). Translational neurocardiology: preclinical models and cardioneural integrative aspects. J. Physiol. 594, 3877–3909. doi: 10.1113/JP271869
Ardell, J. L., and Armour, J. A. (2016). Neurocardiology: structure-based function. Compr. Physiol. 6, 1635–1653. doi: 10.1002/cphy.c150046
Ardell, J. L., Butler, C. K., Smith, F. M., Hopkins, D. A., and Armour, J. A. (1991). Activity of in vivo atrial and ventricular neurons in chronically decentralized canine hearts. Am. J. Physiol. 260, H713–H721. doi: 10.1152/ajpheart.1991.260.3.H713
Ardell, J. L., Foreman, R. D., Armour, J. A., and Shivkumar, K. (2019). Cardiac sympathectomy and spinal cord stimulation attenuate reflex-mediated norepinephrine release during ischemia preventing ventricular fibrillation. JCI Insight 4:e131648. doi: 10.1172/jci.insight.131648
Ardell, J. L., Nier, H., Hammer, M., Southerland, E. M., Ardell, C. L., Beaumont, E., et al. (2017). Defining the neural fulcrum for chronic vagus nerve stimulation: implications for integrated cardiac control. J. Physiol. 595, 6887–6903. doi: 10.1113/JP274678
Ardell, J. L., Rajendran, P. S., Nier, H. A., Kenknight, B. H., and Armour, J. A. (2015). Central-peripheral neural network interactions evoked by vagus nerve stimulation: functional consequences on control of cardiac function. Am. J. Physiol. Heart Circ. Physiol. 309, H1740–H1752. doi: 10.1152/ajpheart.00557.2015
Ardell, J. L., and Randall, W. C. (1986). Selective vagal innervation of sinoatrial and atrioventricular nodes in canine heart. Am. J. Physiol. 251, H764–H773. doi: 10.1152/ajpheart.1986.251.4.H764
Arle, J. E., Carlson, K. W., and Mei, L. (2016). Investigation of mechanisms of vagus nerve stimulation for seizure using finite element modeling. Epilepsy Res. 126, 109–118. doi: 10.1016/j.eplepsyres.2016.07.009
Armour, J. A. (2004). Cardiac neuronal hierarchy in health and disease. Am. J. Phys. Regul. Integr. Comp. Phys. 287, R262–R271. doi: 10.1152/ajpregu.00183.2004
Armour, J. A. (2008). Potential clinical relevance of the ‘little brain’ on the mammalian heart. Exp. Physiol. 93, 165–176. doi: 10.1113/expphysiol.2007.041178
Armour, J. A., and Ardell, J. L. (2004). Basic and clinical neurocardiology. New York: Oxford University Press.
Armour, J. A., Collier, K., Kember, G., and Ardell, J. L. (1998). Differential selectivity of cardiac neurons in separate intrathoracic autonomic ganglia. Am. J. Physiol. 274, R939–R949. doi: 10.1152/ajpregu.1998.274.4.R939
Armour, J. A., and Hopkins, D. A. (1981). Localization of sympathetic postganglionic neurons of physiologically identified cardiac nerves in the dog. J. Comp. Neurol. 202, 169–184. doi: 10.1002/cne.902020204
Armour, J. A., and Janes, R. D. (1988). Neuronal activity recorded extracellularly from in situ canine mediastinal ganglia. Can. J. Physiol. Pharmacol. 66, 119–127. doi: 10.1139/y88-022
Armour, J. A., and Kember, G. (2004). “Cardiac sensory neurons” in Basic and clinical neurocardiology. eds. J. A. Armour and J. L. Ardell (New York: Oxford University Press), 79–117.
Armour, J. A., Murphy, D. A., Yuan, B. X., Macdonald, S., and Hopkins, D. A. (1997). Gross and microscopic anatomy of the human intrinsic cardiac nervous system. Anat. Rec. 247, 289–298. doi: 10.1002/(SICI)1097-0185(199702)247:2<289::AID-AR15>3.0.CO;2-L
Armour, J. A., Randall, W. C., and Sinha, S. (1975). Localized myocardial responses to stimulation of small cardiac branches of the vagus. Am. J. Physiol. 228, 141–148. doi: 10.1152/ajplegacy.1975.228.1.141
Armour, J. A., Yuan, B. X., and Butler, C. K. (1990). Cardiac responses elicited by peptides administered to canine intrinsic cardiac neurons. Peptides 11, 753–761. doi: 10.1016/0196-9781(90)90191-7
Beaumont, E., Salavatian, S., Southerland, E. M., Vinet, A., Jacquemet, V., Armour, J. A., et al. (2013). Network interactions within the canine intrinsic cardiac nervous system: implications for reflex control of regional cardiac function. J. Physiol. 591, 4515–4533. doi: 10.1113/jphysiol.2013.259382
Beaumont, E., Southerland, E. M., Hardwick, J. C., Wright, G. L., Ryan, S., Li, Y., et al. (2015). Vagus nerve stimulation mitigates intrinsic cardiac neuronal and adverse myocyte remodeling postmyocardial infarction. Am. J. Physiol. Heart Circ. Physiol. 309, H1198–H1206. doi: 10.1152/ajpheart.00393.2015
Beaumont, E., Wright, G. L., Southerland, E. M., Li, Y., Chui, R., Kenknight, B. H., et al. (2016). Vagus nerve stimulation mitigates intrinsic cardiac neuronal remodeling and cardiac hypertrophy induced by chronic pressure overload in Guinea pig. Am. J. Physiol. Heart Circ. Physiol. 310, H1349–H1359. doi: 10.1152/ajpheart.00939.2015
Benjamin, E. J., Muntner, P., Alonso, A., Bittencourt, M. S., Callaway, C. W., Carson, A. P., et al. (2019). Heart disease and stroke statistics-2019 update: a report from the american heart association. Circulation 139, e56–e528. doi: 10.1161/CIR.0000000000000659
Ben-Menachem, E., Revesz, D., Simon, B. J., and Silberstein, S. (2015). Surgically implanted and non-invasive vagus nerve stimulation: a review of efficacy, safety and tolerability. Eur. J. Neurol. 22, 1260–1268. doi: 10.1111/ene.12629
Berthoud, H. R., and Neuhuber, W. L. (2000). Functional and chemical anatomy of the afferent vagal system. Auton. Neurosci. 85, 1–17. doi: 10.1016/S1566-0702(00)00215-0
Billman, G. E. (2006). A comprehensive review and analysis of 25 years of data from an in vivo canine model of sudden cardiac death: implications for future anti-arrhythmic drug development. Pharmacol. Ther. 111, 808–835. doi: 10.1016/j.pharmthera.2006.01.002
Billman, G. E., Schwartz, P. J., and Stone, H. L. (1982). Baroreceptor reflex control of heart rate: a predictor of sudden cardiac death. Circulation 66, 874–880. doi: 10.1161/01.CIR.66.4.874
Billman, G. E., Schwartz, P. J., and Stone, H. L. (1984). The effects of daily exercise on susceptibility to sudden cardiac death. Circulation 69, 1182–1189. doi: 10.1161/01.CIR.69.6.1182
Bohlender, J., and Imboden, H. (2012). Angiotensinergic neurotransmission in the peripheral autonomic nervous system. Front. Biosci. 17, 2419–2432. doi: 10.2741/4062
Bourke, T., Vaseghi, M., Michowitz, Y., Sankhla, V., Shah, M., Swapna, N., et al. (2010). Neuraxial modulation for refractory ventricular arrhythmias: value of thoracic epidural anesthesia and surgical left cardiac sympathetic denervation. Circulation 121, 2255–2262. doi: 10.1161/CIRCULATIONAHA.109.929703
Boyden, E. S., Zhang, F., Bamberg, E., Nagel, G., and Deisseroth, K. (2005). Millisecond-timescale, genetically targeted optical control of neural activity. Nat. Neurosci. 8, 1263–1268. doi: 10.1038/nn1525
Buckley, U., Chui, R. W., Rajendran, P. S., Vrabec, T., Shivkumar, K., and Ardell, J. L. (2017). Bioelectronic neuromodulation of the paravertebral cardiac efferent sympathetic outflow and its effect on ventricular electrical indices. Heart Rhythm 14, 1063–1070. doi: 10.1016/j.hrthm.2017.02.020
Buckley, U., Shivkumar, K., and Ardell, J. L. (2015). Autonomic regulation therapy in heart failure. Curr. Heart Fail. Rep. 12, 284–293. doi: 10.1007/s11897-015-0263-7
Burwash, I. G., Morgan, D. E., Koilpillai, C. J., Blackmore, G. L., Johnstone, D. E., and Armour, J. A. (1993). Sympathetic stimulation alters left ventricular relaxation and chamber size. Am. J. Physiol. 264, R1–R7. doi: 10.1152/ajpregu.1993.264.1.R1
Butler, C. K., Smith, F. M., Cardinal, R., Murphy, D. A., Hopkins, D. A., and Armour, J. A. (1990). Cardiac responses to electrical stimulation of discrete loci in canine atrial and ventricular ganglionated plexi. Am. J. Physiol. 259, H1365–H1373. doi: 10.1152/ajpheart.1990.259.5.H1365
Calvillo, L., Vanoli, E., Andreoli, E., Besana, A., Omodeo, E., Gnecchi, M., et al. (2011). Vagal stimulation, through its nicotinic action, limits infarct size and the inflammatory response to myocardial ischemia and reperfusion. J. Cardiovasc. Pharmacol. 58, 500–507. doi: 10.1097/FJC.0b013e31822b7204
Cao, J. M., Chen, L. S., Kenknight, B. H., Ohara, T., Lee, M. H., Tsai, J., et al. (2000). Nerve sprouting and sudden cardiac death. Circ. Res. 86, 816–821. doi: 10.1161/01.RES.86.7.816
Cardinal, R., Page, P., Vermeulen, M., Ardell, J. L., and Armour, J. A. (2009). Spatially divergent cardiac responses to nicotinic stimulation of ganglionated plexus neurons in the canine heart. Auton. Neurosci. 145, 55–62. doi: 10.1016/j.autneu.2008.11.007
Chui, R. W., Buckley, U., Rajendran, P. S., Vrabec, T., Shivkumar, K., and Ardell, J. L. (2017). Bioelectronic block of paravertebral sympathetic nerves mitigates post-myocardial infarction ventricular arrhythmias. Heart Rhythm 14, 1665–1672. doi: 10.1016/j.hrthm.2017.06.025
Corr, P. B., and Gillis, R. A. (1974). Role of the vagus nerves in the cardiovascular changes induced by coronary occlusion. Circulation 49, 86–97. doi: 10.1161/01.CIR.49.1.86
Dargie, H. J. (2001). Effect of carvedilol on outcome after myocardial infarction in patients with left-ventricular dysfunction: the CAPRICORN randomised trial. Lancet 357, 1385–1390. doi: 10.1016/s0140-6736(00)04560-8
De Ferrari, G. M. (2014). Vagal stimulation in heart failure. J. Cardiovasc. Transl. Res. 7, 310–320. doi: 10.1007/s12265-014-9540-1
De Ferrari, G. M., Crijns, H. J., Borggrefe, M., Milasinovic, G., Smid, J., Zabel, M., et al. (2011). Chronic vagus nerve stimulation: a new and promising therapeutic approach for chronic heart failure. Eur. Heart J. 32, 847–855. doi: 10.1093/eurheartj/ehq391
De Ferrari, G. M., Dusi, V., Spazzolini, C., Bos, J. M., Abrams, D. J., Berul, C. I., et al. (2015). Clinical management of catecholaminergic polymorphic ventricular tachycardia: the role of left cardiac sympathetic denervation. Circulation 131, 2185–2193. doi: 10.1161/CIRCULATIONAHA.115.015731
De Ferrari, G. M., Stolen, C., Tuinenburg, A. E., Wright, D. J., Brugada, J., Butter, C., et al. (2017). Long-term vagal stimulation for heart failure: eighteen month results from the neural cardiac therapy for heart failure (NECTAR-HF) trial. Int. J. Cardiol. 244, 229–234. doi: 10.1016/j.ijcard.2017.06.036
De Ferrari, G. M., Vanoli, E., Stramba-Badiale, M., Hull, S. S. Jr., Foreman, R. D., and Schwartz, P. J. (1991). Vagal reflexes and survival during acute myocardial ischemia in conscious dogs with healed myocardial infarction. Am. J. Physiol. 261, H63–H69. doi: 10.1152/ajpheart.1991.261.1.H63
Dibner-Dunlap, M. E., Kinugawa, T., and Thames, M. D. (1993). Activation of cardiac sympathetic afferents: effects of exogenous adenosine and adenosine analogues. Am. J. Physiol. 265, H395–H400. doi: 10.1152/ajpheart.1993.265.1.H395
Dicarlo, L. A., Libbus, I., Kumar, H. U., Mittal, S., Premchand, R. K., Amurthur, B., et al. (2018). Autonomic regulation therapy to enhance myocardial function in heart failure patients: the ANTHEM-HFpEF study. ESC Heart Fail. 5, 95–100. doi: 10.1002/ehf2.12241
Feigl, E. O. (1969). Parasympathetic control of coronary blood flow in dogs. Circ. Res. 25, 509–519. doi: 10.1161/01.RES.25.5.509
Florea, V. G., and Cohn, J. N. (2014). The autonomic nervous system and heart failure. Circ. Res. 114, 1815–1826. doi: 10.1161/CIRCRESAHA.114.302589
Fu, L. W., and Longhurst, J. C. (2009). Regulation of cardiac afferent excitability in ischemia. Handb. Exp. Pharmacol. 194, 185–225. doi: 10.1007/978-3-540-79090-7_6
Fukuda, K., Kanazawa, H., Aizawa, Y., Ardell, J. L., and Shivkumar, K. (2015). Cardiac innervation and sudden cardiac death. Circ. Res. 116, 2005–2019. doi: 10.1161/CIRCRESAHA.116.304679
Gardner, R. T., Wang, L., Lang, B. T., Cregg, J. M., Dunbar, C. L., Woodward, W. R., et al. (2015). Targeting protein tyrosine phosphatase sigma after myocardial infarction restores cardiac sympathetic innervation and prevents arrhythmias. Nat. Commun. 6:6235. doi: 10.1038/ncomms7235
Garrott, K., Dyavanapalli, J., Cauley, E., Dwyer, M. K., Kuzmiak-Glancy, S., Wang, X., et al. (2017). Chronic activation of hypothalamic oxytocin neurons improves cardiac function during left ventricular hypertrophy-induced heart failure. Cardiovasc. Res. 113, 1318–1328. doi: 10.1093/cvr/cvx084
Gibbons, D. D., Southerland, E. M., Hoover, D. B., Beaumont, E., Armour, J. A., and Ardell, J. L. (2012). Neuromodulation targets intrinsic cardiac neurons to attenuate neuronally mediated atrial arrhythmias. Am. J. Phys. Regul. Integr. Comp. Phys. 302, R357–R364. doi: 10.1152/ajpregu.00535.2011
Gloschat, C. R., Koppel, A. C., Aras, K. K., Brennan, J. A., Holzem, K. M., and Efimov, I. R. (2016). Arrhythmogenic and metabolic remodelling of failing human heart. J. Physiol. 594, 3963–3980. doi: 10.1113/JP271992
Gold, M. R., Van Veldhuisen, D. J., Hauptman, P. J., Borggrefe, M., Kubo, S. H., Lieberman, R. A., et al. (2016). Vagus nerve stimulation for the treatment of heart failure: the INOVATE-HF trial. J. Am. Coll. Cardiol. 68, 149–158. doi: 10.1016/j.jacc.2016.03.525
Habecker, B. A., Anderson, M. E., Birren, S. J., Fukuda, K., Herring, N., Hoover, D. B., et al. (2016). Molecular and cellular neurocardiology: development, and cellular and molecular adaptations to heart disease. J. Physiol. 594, 3853–3875. doi: 10.1113/JP271840
Han, S., Kobayashi, K., Joung, B., Piccirillo, G., Maruyama, M., Vinters, H. V., et al. (2012). Electroanatomic remodeling of the left stellate ganglion after myocardial infarction. J. Am. Coll. Cardiol. 59, 954–961. doi: 10.1016/j.jacc.2011.11.030
Hanna, P., Shivkumar, K., and Ardell, J. L. (2018). Calming the nervous heart: autonomic therapies in heart failure. Card. Fail. Rev. 4, 92–98. doi: 10.15420/cfr.2018.20.2
Hardwick, J. C., Southerland, E. M., and Ardell, J. L. (2008). Chronic myocardial infarction induces phenotypic and functional remodeling in the Guinea pig cardiac plexus. Am. J. Phys. Regul. Integr. Comp. Phys. 295, R1926–R1933. doi: 10.1152/ajpregu.90306.2008
Haws, C. W., and Lux, R. L. (1990). Correlation between in vivo transmembrane action potential durations and activation-recovery intervals from electrograms. Effects of interventions that alter repolarization time. Circulation 81, 281–288. doi: 10.1161/01.cir.81.1.281
Herring, N., Cranley, J., Lokale, M. N., Li, D., Shanks, J., Alston, E. N., et al. (2012). The cardiac sympathetic co-transmitter galanin reduces acetylcholine release and vagal bradycardia: implications for neural control of cardiac excitability. J. Mol. Cell. Cardiol. 52, 667–676. doi: 10.1016/j.yjmcc.2011.11.016
Hill, M. R., Wallick, D. W., Martin, P. J., and Levy, M. N. (1995). Effects of repetitive vagal stimulation on heart rate and on cardiac vasoactive intestinal polypeptide efflux. Am. J. Physiol. 268, H1939–H1946. doi: 10.1152/ajpheart.1995.268.5.H1939
Hoang, J. D., Salavatian, S., Yamaguchi, N., Swid, M. A., and Vaseghi, M. (2020). Cardiac sympathetic activation circumvents high-dose beta blocker therapy in part through release of neuropeptide Y. JCI Insight 5:e135519. doi: 10.1172/jci.insight.135519
Hoover, D. B., Ganote, C. E., Ferguson, S. M., Blakely, R. D., and Parsons, R. L. (2004). Localization of cholinergic innervation in Guinea pig heart by immunohistochemistry for high-affinity choline transporters. Cardiovasc. Res. 62, 112–121. doi: 10.1016/j.cardiores.2004.01.012
Hoover, D. B., Isaacs, E. R., Jacques, F., Hoard, J. L., Page, P., and Armour, J. A. (2009). Localization of multiple neurotransmitters in surgically derived specimens of human atrial ganglia. Neuroscience 164, 1170–1179. doi: 10.1016/j.neuroscience.2009.09.001
Hoover, D. B., Shepherd, A. V., Southerland, E. M., Armour, J. A., and Ardell, J. L. (2008). Neurochemical diversity of afferent neurons that transduce sensory signals from dog ventricular myocardium. Auton. Neurosci. 141, 38–45. doi: 10.1016/j.autneu.2008.04.010
Hopkins, D. A., and Armour, J. A. (1984). Localization of sympathetic postganglionic and parasympathetic preganglionic neurons which innervate different regions of the dog heart. J. Comp. Neurol. 229, 186–198. doi: 10.1002/cne.902290205
Hopkins, D. A., Bieger, D., Devente, J., and Steinbusch, W. M. (1996). Vagal efferent projections: viscerotopy, neurochemistry and effects of vagotomy. Prog. Brain Res. 107, 79–96. doi: 10.1016/s0079-6123(08)61859-2
Huang, M. H., Horackova, M., Negoescu, R. M., Wolf, S., and Armour, J. A. (1996). Polysensory response characteristics of dorsal root ganglion neurones that may serve sensory functions during myocardial ischaemia. Cardiovasc. Res. 32, 503–515. doi: 10.1016/S0008-6363(96)00108-3
Janes, R. D., Brandys, J. C., Hopkins, D. A., Johnstone, D. E., Murphy, D. A., and Armour, J. A. (1986). Anatomy of human extrinsic cardiac nerves and ganglia. Am. J. Cardiol. 57, 299–309. doi: 10.1016/0002-9149(86)90908-2
Jänig, W. (2006). The integrative action of the autonomic nervous system: Neurobiology of homeostasis. New York: Cambridge University Press.
Jänig, W. (2014). Sympathetic nervous system and inflammation: a conceptual view. Auton. Neurosci. 182, 4–14. doi: 10.1016/j.autneu.2014.01.004
Janig, W. (2016). Neurocardiology: a neurobiologist’s perspective. J. Physiol. 594, 3955–3962. doi: 10.1113/JP271895
Kalia, M., and Mesulam, M. M. (1980). Brain stem projections of sensory and motor components of the vagus complex in the cat: I. the cervical vagus and nodose ganglion. J. Comp. Neurol. 193, 435–465. doi: 10.1002/cne.901930210
Kawano, H., Okada, R., and Yano, K. (2003). Histological study on the distribution of autonomic nerves in the human heart. Heart Vessels 18, 32–39. doi: 10.1007/s003800300005
Kawashima, T. (2005). The autonomic nervous system of the human heart with special reference to its origin, course, and peripheral distribution. Anat. Embryol. 209, 425–438. doi: 10.1007/s00429-005-0462-1
Konstam, M. A., Udelson, J. E., Butler, J., Klein, H. U., Parker, J. D., Teerlink, J. R., et al. (2019). Impact of autonomic regulation therapy in patients with heart failure: ANTHEM-HFrEF pivotal study design. Circ. Heart Fail. 12:e005879. doi: 10.1161/CIRCHEARTFAILURE.119.005879
Kuntz, A., and Morehouse, A. (1930). Thoracic sympathetic cardiac nerves in man. Arch. Surg. 20, 607–613. doi: 10.1001/archsurg.1930.01150100067004
La Rovere, M. T., Bigger, J. T. Jr., Marcus, F. I., Mortara, A., and Schwartz, P. J. (1998). Baroreflex sensitivity and heart-rate variability in prediction of total cardiac mortality after myocardial infarction. ATRAMI (autonomic tone and reflexes after myocardial infarction) investigators. Lancet 351, 478–484. doi: 10.1016/s0140-6736(97)11144-8
Levy, M. N., and Martin, P. J. (1979). “Neural control of the heart” in Handbook of physiology: Section 2: The cardiovascular system, Vol. 1: The heart. ed. R. M. Berne (Bethesda: The American Physiological Society), 581–620.
Levy, M. N., and Schwartz, P. J. (1994). Vagal control of the heart: Experimental basis and clinical implications. Armonk, NY: Futura Pub. Co.
Li, M., Zheng, C., Sato, T., Kawada, T., Sugimachi, M., and Sunagawa, K. (2004). Vagal nerve stimulation markedly improves long-term survival after chronic heart failure in rats. Circulation 109, 120–124. doi: 10.1161/01.CIR.0000105721.71640.DA
Linz, D., Ukena, C., Mahfoud, F., Neuberger, H. R., and Bohm, M. (2014). Atrial autonomic innervation: a target for interventional antiarrhythmic therapy? J. Am. Coll. Cardiol. 63, 215–224. doi: 10.1016/j.jacc.2013.09.020
Liu, Y., Scherlag, B. J., Fan, Y., Varma, V., Male, S., Chaudhry, M. A., et al. (2013). Inducibility of atrial fibrillation after GP ablations and "autonomic blockade": evidence for the pathophysiological role of the nonadrenergic and noncholinergic neurotransmitters. J. Cardiovasc. Electrophysiol. 24, 188–195. doi: 10.1111/j.1540-8167.2012.02449.x
Lo, L. W., Scherlag, B. J., Chang, H. Y., Lin, Y. J., Chen, S. A., and Po, S. S. (2013). Paradoxical long-term proarrhythmic effects after ablating the “head station” ganglionated plexi of the vagal innervation to the heart. Heart Rhythm 10, 751–757. doi: 10.1016/j.hrthm.2013.01.030
Lundberg, J. M., Franco-Cereceda, A., Lacroix, J. S., and Pernow, J. (1990). Neuropeptide Y and sympathetic neurotransmission. Ann. N. Y. Acad. Sci. 611, 166–174. doi: 10.1111/j.1749-6632.1990.tb48930.x
Malliani, A., Lombardi, F., Pagani, M., Recordati, G., and Schwartz, P. J. (1975). Spinal cardiovascular reflexes. Brain Res. 87, 239–246. doi: 10.1016/0006-8993(75)90421-7
Malliani, A., and Montano, N. (2002). Emerging excitatory role of cardiovascular sympathetic afferents in pathophysiological conditions. Hypertension 39, 63–68. doi: 10.1161/hy0102.099200
Malliani, A., Recordati, G., and Schwartz, P. J. (1973). Nervous activity of afferent cardiac sympathetic fibres with atrial and ventricular endings. J. Physiol. 229, 457–469. doi: 10.1113/jphysiol.1973.sp010147
Malliani, A., Schwartz, P. J., and Zanchetti, A. (1969). A sympathetic reflex elicited by experimental coronary occlusion. Am. J. Physiol. 217, 703–709. doi: 10.1152/ajplegacy.1969.217.3.703
McGuirt, A. S., Schmacht, D. C., and Ardell, J. L. (1997). Autonomic interactions for control of atrial rate are maintained after SA nodal parasympathectomy. Am. J. Physiol. 272, H2525–H2533. doi: 10.1152/ajpheart.1997.272.6.H2525
McMurray, J. J., Packer, M., Desai, A. S., Gong, J., Lefkowitz, M. P., Rizkala, A. R., et al. (2014). Angiotensin-neprilysin inhibition versus enalapril in heart failure. N. Engl. J. Med. 371, 993–1004. doi: 10.1056/NEJMoa1409077
Mill, J. G., Stefanon, I., Dos Santos, L., and Baldo, M. P. (2011). Remodeling in the ischemic heart: the stepwise progression for heart failure. Braz. J. Med. Biol. Res. 44, 890–898. doi: 10.1590/S0100-879X2011007500096
Minisi, A. J., and Thames, M. D. (1991). Activation of cardiac sympathetic afferents during coronary occlusion. Evidence for reflex activation of sympathetic nervous system during transmural myocardial ischemia in the dog. Circulation 84, 357–367. doi: 10.1161/01.cir.84.1.357
Minisi, A. J., and Thames, M. D. (1993). Distribution of left ventricular sympathetic afferents demonstrated by reflex responses to transmural myocardial ischemia and to intracoronary and epicardial bradykinin. Circulation 87, 240–246. doi: 10.1161/01.CIR.87.1.240
Moreno, A., Endicott, K., Skancke, M., Dwyer, M. K., Brennan, J., Efimov, I. R., et al. (2019). Sudden heart rate reduction upon optogenetic release of acetylcholine from cardiac parasympathetic neurons in perfused hearts. Front. Physiol. 10:16. doi: 10.3389/fphys.2019.00016
Nakagawa, H., Scherlag, B. J., Patterson, E., Ikeda, A., Lockwood, D., and Jackman, W. M. (2009). Pathophysiologic basis of autonomic ganglionated plexus ablation in patients with atrial fibrillation. Heart Rhythm 6, S26–S34. doi: 10.1016/j.hrthm.2009.07.029
Norris, J. E., Foreman, R. D., and Wurster, R. K. (1974). Responses of the canine heart to stimulation of the first five ventral thoracic roots. Am. J. Physiol. 227, 9–12. doi: 10.1152/ajplegacy.1974.227.1.9
Norris, J. E., Lippincott, D., and Wurster, R. D. (1977). Responses of canine endocardium to stimulation of the upper thoracic roots. Am. J. Physiol. 233, H655–H659. doi: 10.1152/ajpheart.1977.233.6.H655
Olivas, A., Gardner, R. T., Wang, L., Ripplinger, C. M., Woodward, W. R., and Habecker, B. A. (2016). Myocardial infarction causes transient cholinergic transdifferentiation of cardiac sympathetic nerves via gp130. J. Neurosci. 36, 479–488. doi: 10.1523/JNEUROSCI.3556-15.2016
Olshansky, B., Sabbah, H. N., Hauptman, P. J., and Colucci, W. S. (2008). Parasympathetic nervous system and heart failure: pathophysiology and potential implications for therapy. Circulation 118, 863–871. doi: 10.1161/CIRCULATIONAHA.107.760405
Paintal, A. S. (1953). The conduction velocities of respiratory and cardiovascular afferent fibres in the vagus nerve. J. Physiol. 121, 341–359. doi: 10.1113/jphysiol.1953.sp004950
Paintal, A. S. (1973). Vagal sensory receptors and their reflex effects. Physiol. Rev. 53, 159–227. doi: 10.1152/physrev.1973.53.1.159
Palma, J. A., and Benarroch, E. E. (2014). Neural control of the heart: recent concepts and clinical correlations. Neurology 83, 261–271. doi: 10.1212/WNL.0000000000000605
Pauza, D. H., Rysevaite-Kyguoliene, K., Vismantaite, J., Brack, K. E., Inokaitis, H., Pauza, A. G., et al. (2014). A combined acetylcholinesterase and immunohistochemical method for precise anatomical analysis of intrinsic cardiac neural structures. Ann. Anat. 196, 430–440. doi: 10.1016/j.aanat.2014.08.004
Petraitiene, V., Pauza, D. H., and Benetis, R. (2014). Distribution of adrenergic and cholinergic nerve fibres within intrinsic nerves at the level of the human heart hilum. Eur. J. Cardiothorac. Surg. 45, 1097–1105. doi: 10.1093/ejcts/ezt575
Pfeffer, M. A., Braunwald, E., Moye, L. A., Basta, L., Brown, E. J., Cuddy, T. E., et al. (1992). Effect of captopril on mortality and morbidity in patients with left-ventricular dysfunction after myocardial-infarction-results of the survival and ventricular enlargement trial. N. Engl. J. Med. 327, 669–677. doi: 10.1056/NEJM199209033271001
Prechtl, J. C., and Powley, T. L. (1990). The fiber composition of the abdominal vagus of the rat. Anat. Embryol. 181, 101–115. doi: 10.1007/BF00198950
Premchand, R. K., Sharma, K., Mittal, S., Monteiro, R., Dixit, S., Libbus, I., et al. (2014). Autonomic regulation therapy via left or right cervical vagus nerve stimulation in patients with chronic heart failure: results of the ANTHEM-HF trial. J. Card. Fail. 20, 808–816. doi: 10.1016/j.cardfail.2014.08.009
Premchand, R. K., Sharma, K., Mittal, S., Monteiro, R., Dixit, S., Libbus, I., et al. (2016). Extended follow-up of patients with heart failure receiving autonomic regulation therapy in the ANTHEM-HF study. J. Card. Fail. 22, 639–642. doi: 10.1016/j.cardfail.2015.11.002
Rajendran, P. S., Nakamura, K., Ajijola, O. A., Vaseghi, M., Armour, J. A., Ardell, J. L., et al. (2016). Myocardial infarction induces structural and functional remodelling of the intrinsic cardiac nervous system. J. Physiol. 594, 321–341. doi: 10.1113/JP271165
Randall, W. C. (1994). “Efferent sympathetic innervation of the heart” in Neurocardiology. eds. J. A. Armour and J. L. Ardell (New York: Oxford University Press), 77–94.
Randall, D. C., Brown, D. R., McGuirt, A. S., Thompson, G. W., Armour, J. A., and Ardell, J. L. (2003). Interactions within the intrinsic cardiac nervous system contribute to chronotropic regulation. Am. J. Phys. Regul. Integr. Comp. Phys. 285, R1066–R1075. doi: 10.1152/ajpregu.00167.2003
Ripplinger, C. M., Noujaim, S. F., and Linz, D. (2016). The nervous heart. Prog. Biophys. Mol. Biol. 120, 199–209. doi: 10.1016/j.pbiomolbio.2015.12.015
Ruble, S. B., Hamann, J. J., Gupta, R. C., Mishra, S., and Sabbah, H. N. (2010). Chronic vagus nerve stimulation impacts biomarkers of heart failure in canines. J. Am. Coll. Cardiol. 55:A16.E153. doi: 10.1016/S0735-1097(10)60154-4
Rysevaite, K., Saburkina, I., Pauziene, N., Noujaim, S. F., Jalife, J., and Pauza, D. H. (2011a). Morphologic pattern of the intrinsic ganglionated nerve plexus in mouse heart. Heart Rhythm 8, 448–454. doi: 10.1016/j.hrthm.2010.11.019
Rysevaite, K., Saburkina, I., Pauziene, N., Vaitkevicius, R., Noujaim, S. F., Jalife, J., et al. (2011b). Immunohistochemical characterization of the intrinsic cardiac neural plexus in whole-mount mouse heart preparations. Heart Rhythm 8, 731–738. doi: 10.1016/j.hrthm.2011.01.013
Sabbah, H. N. (2011). Electrical vagus nerve stimulation for the treatment of chronic heart failure. Cleve. Clin. J. Med. 78 (Suppl. 1), S24–S29. doi: 10.3949/ccjm.78.s1.04
Sabbah, H. N., Ilsar, I., Zaretsky, A., Rastogi, S., Wang, M., and Gupta, R. C. (2011). Vagus nerve stimulation in experimental heart failure. Heart Fail. Rev. 16, 171–178. doi: 10.1007/s10741-010-9209-z
Salavatian, S., Beaumont, E., Gibbons, D., Hammer, M., Hoover, D. B., Armour, J. A., et al. (2017a). Thoracic spinal cord and cervical vagosympathetic neuromodulation obtund nodose sensory transduction of myocardial ischemia. Auton. Neurosci. 208, 57–65. doi: 10.1016/j.autneu.2017.08.005
Salavatian, S., Beaumont, E., Longpre, J. P., Armour, J. A., Vinet, A., Jacquemet, V., et al. (2016). Vagal stimulation targets select populations of intrinsic cardiac neurons to control neurally induced atrial fibrillation. Am. J. Physiol. Heart Circ. Physiol. 311, H1311–H1320. doi: 10.1152/ajpheart.00443.2016
Salavatian, S., Yamaguchi, N., Hamon, D., Fishbein, M. C., Ardell, J. L., Shivkumar, K., et al. (2017b). Myocardial infarction causes both structural and functional remodeling in cardiac neurons of the inferior vagal (Nodose) ganglia: implications for mechanisms behind parasympathetic withdrawal in heart disease. Circulation 136:A17355.
Schofield, B. M., and Walker, J. M. (1953). Perfusion of the coronary arteries of the dog. J. Physiol. 122, 489–497. doi: 10.1113/jphysiol.1953.sp005016
Schultz, H. D. (2001). Cardiac vagal chemosensory afferents. Function in pathophysiological states. Ann. N. Y. Acad. Sci. 940, 59–73. doi: 10.1111/j.1749-6632.2001.tb03667.x
Schultz, H. D., Marcus, N. J., and Del Rio, R. (2015). Mechanisms of carotid body chemoreflex dysfunction during heart failure. Exp. Physiol. 100, 124–129. doi: 10.1113/expphysiol.2014.079517
Schultz, H. D., and Ustinova, E. E. (1996). Cardiac vagal afferent stimulation by free radicals during ischaemia and reperfusion. Clin. Exp. Pharmacol. Physiol. 23, 700–708. doi: 10.1111/j.1440-1681.1996.tb01762.x
Schwartz, P. J. (2014). Cardiac sympathetic denervation to prevent life-threatening arrhythmias. Nat. Rev. Cardiol. 11, 346–353. doi: 10.1038/nrcardio.2014.19
Schwartz, P. J., De Ferrari, G. M., Sanzo, A., Landolina, M., Rordorf, R., Raineri, C., et al. (2008). Long term vagal stimulation in patients with advanced heart failure: first experience in man. Eur. J. Heart Fail. 10, 884–891. doi: 10.1016/j.ejheart.2008.07.016
Schwartz, P. J., Foreman, R. D., Stone, H. L., and Brown, A. M. (1976). Effect of dorsal root section on the arrhythmias associated with coronary occlusion. Am. J. Physiol. 231, 923–928. doi: 10.1152/ajplegacy.1976.231.3.923
Schwartz, P. J., La Rovere, M. T., De Ferrari, G. M., and Mann, D. L. (2015). Autonomic modulation for the management of patients with chronic heart failure. Circ. Heart Fail. 8, 619–628. doi: 10.1161/CIRCHEARTFAILURE.114.001964
Schwartz, P. J., La Rovere, M. T., and Vanoli, E. (1992). Autonomic nervous system and sudden cardiac death. Experimental basis and clinical observations for post-myocardial infarction risk stratification. Circulation 85, I77–I191.
Schwartz, P. J., Pagani, M., Lombardi, F., Malliani, A., and Brown, A. M. (1973). A cardiocardiac sympathovagal reflex in the cat. Circ. Res. 32, 215–220. doi: 10.1161/01.RES.32.2.215
Schwartz, P. J., Priori, S. G., Cerrone, M., Spazzolini, C., Odero, A., Napolitano, C., et al. (2004). Left cardiac sympathetic denervation in the management of high-risk patients affected by the long-QT syndrome. Circulation 109, 1826–1833. doi: 10.1161/01.CIR.0000125523.14403.1E
Shanks, J., and Herring, N. (2013). Peripheral cardiac sympathetic hyperactivity in cardiovascular disease: role of neuropeptides. Am. J. Phys. Regul. Integr. Comp. Phys. 305, R1411–R1420. doi: 10.1152/ajpregu.00118.2013
Sharma, K., Premchand, R. K., Mittal, S., Monteiro, R., Libbus, I., Dicarlo, L. A., et al. (2020). Long-term follow-up of patients with heart failure and reduced ejection receiving autonomic regulation therapy in the ANTHEM-HF pilot study. Int. J. Cardiol. 323, 175–178. doi: 10.1016/j.ijcard.2020.09.072
Shivkumar, K., Ajijola, O. A., Anand, I., Armour, J. A., Chen, P. S., Esler, M., et al. (2016). Clinical neurocardiology defining the value of neuroscience-based cardiovascular therapeutics. J. Physiol. 594, 3911–3954. doi: 10.1113/JP271870
Shivkumar, K., and Ardell, J. L. (2016). Cardiac autonomic control in health and disease. J. Physiol. 594, 3851–3852. doi: 10.1113/JP272580
Southerland, E. M., Milhorn, D. M., Foreman, R. D., Linderoth, B., Dejongste, M. J., Armour, J. A., et al. (2007). Preemptive, but not reactive, spinal cord stimulation mitigates transient ischemia-induced myocardial infarction via cardiac adrenergic neurons. Am. J. Physiol. Heart Circ. Physiol. 292, H311–H317. doi: 10.1152/ajpheart.00087.2006
Standish, A., Enquist, L. W., and Schwaber, J. S. (1994). Innervation of the heart and its central medullary origin defined by viral tracing. Science 263, 232–234. doi: 10.1126/science.8284675
Sternson, S. M., and Roth, B. L. (2014). Chemogenetic tools to interrogate brain functions. Annu. Rev. Neurosci. 37, 387–407. doi: 10.1146/annurev-neuro-071013-014048
Tapa, S., Wang, L., Francis Stuart, S. D., Wang, Z., Jiang, Y., Habecker, B. A., et al. (2020). Adrenergic supersensitivity and impaired neural control of cardiac electrophysiology following regional cardiac sympathetic nerve loss. Sci. Rep. 10:18801. doi: 10.1038/s41598-020-75903-y
Thompson, G. W., Horackova, M., and Armour, J. A. (2000). Chemotransduction properties of nodose ganglion cardiac afferent neurons in Guinea pigs. Am. J. Phys. Regul. Integr. Comp. Phys. 279, R433–R439. doi: 10.1152/ajpregu.2000.279.2.R433
Thoren, P. N. (1976). Atrial receptors with nonmedullated vagal afferents in the cat. Discharge frequency and pattern in relation to atrial pressure. Circ. Res. 38, 357–362. doi: 10.1161/01.RES.38.5.357
Tjen, A. L. S., Bonham, A., and Longhurst, J. (1997). Interactions between sympathetic and vagal cardiac afferents in nucleus tractus solitarii. Am. J. Physiol. 272, H2843–H2851. doi: 10.1152/ajpheart.1997.272.6.H2843
Tracey, K. J. (2007). Physiology and immunology of the cholinergic antiinflammatory pathway. J. Clin. Invest. 117, 289–296. doi: 10.1172/JCI30555
Triposkiadis, F., Karayannis, G., Giamouzis, G., Skoularigis, J., Louridas, G., and Butler, J. (2009). The sympathetic nervous system in heart failure physiology, pathophysiology, and clinical implications. J. Am. Coll. Cardiol. 54, 1747–1762. doi: 10.1016/j.jacc.2009.05.015
Vanoli, E., De Ferrari, G. M., Stramba-Badiale, M., Hull, S. S. Jr., Foreman, R. D., and Schwartz, P. J. (1991). Vagal stimulation and prevention of sudden death in conscious dogs with a healed myocardial infarction. Circ. Res. 68, 1471–1481. doi: 10.1161/01.RES.68.5.1471
Vantrimpont, P., Rouleau, J. L., Wun, C. C., Ciampi, A., Klein, M., Sussex, B., et al. (1997). Additive beneficial effects of beta-blockers to angiotensin-converting enzyme inhibitors in the survival and ventricular enlargement (SAVE) study. SAVE investigators. J. Am. Coll. Cardiol. 29, 229–236. doi: 10.1016/s0735-1097(96)00489-5
Vaseghi, M., Gima, J., Kanaan, C., Ajijola, O. A., Marmureanu, A., Mahajan, A., et al. (2014). Cardiac sympathetic denervation in patients with refractory ventricular arrhythmias or electrical storm: intermediate and long-term follow-up. Heart Rhythm 11, 360–366. doi: 10.1016/j.hrthm.2013.11.028
Vaseghi, M., Salavatian, S., Rajendran, P. S., Yagishita, D., Woodward, W. R., Hamon, D., et al. (2017). Parasympathetic dysfunction and antiarrhythmic effect of vagal nerve stimulation following myocardial infarction. JCI Insight 2:e86715. doi: 10.1172/jci.insight.86715
Vaseghi, M., Yamakawa, K., Sinha, A., So, E. L., Zhou, W., Ajijola, O. A., et al. (2013). Modulation of regional dispersion of repolarization and T-peak to T-end interval by the right and left stellate ganglia. Am. J. Physiol. Heart Circ. Physiol. 305, H1020–H1030. doi: 10.1152/ajpheart.00056.2013
Vaseghi, M., Zhou, W., Shi, J., Ajijola, O. A., Hadaya, J., Shivkumar, K., et al. (2012). Sympathetic innervation of the anterior left ventricular wall by the right and left stellate ganglia. Heart Rhythm 9, 1303–1309. doi: 10.1016/j.hrthm.2012.03.052
Verdecchia, P., Sleight, P., Mancia, G., Fagard, R., Trimarco, B., Schmieder, R. E., et al. (2009). Effects of telmisartan, ramipril, and their combination on left ventricular hypertrophy in individuals at high vascular risk in the ongoing telmisartan alone and in combination with ramipril global end point trial and the telmisartan randomized assessment study in ACE intolerant subjects with cardiovascular disease. Circulation 120, 1380–1389. doi: 10.1161/CIRCULATIONAHA.109.865774
Virani, S. S., Alonso, A., Benjamin, E. J., Bittencourt, M. S., Callaway, C. W., Carson, A. P., et al. (2020). Heart disease and stroke statistics-2020 update: a report from the american heart association. Circulation 141, e139–e596. doi: 10.1161/CIR.0000000000000757
Wang, L., Olivas, A., Stuart, S. D. S., Tapa, S., Blake, M. R., Woodward, W. R., et al. (2020). Cardiac sympathetic nerve transdifferentiation reduces action potential heterogeneity after myocardial infarction. Am. J. Physiol. Heart Circ. Physiol. 318, H558–H565. doi: 10.1152/ajpheart.00412.2019
Wang, H. J., Wang, W., Cornish, K. G., Rozanski, G. J., and Zucker, I. H. (2014). Cardiac sympathetic afferent denervation attenuates cardiac remodeling and improves cardiovascular dysfunction in rats with heart failure. Hypertension 64, 745–755. doi: 10.1161/HYPERTENSIONAHA.114.03699
Wengrowski, A. M., Wang, X., Tapa, S., Posnack, N. G., Mendelowitz, D., and Kay, M. W. (2015). Optogenetic release of norepinephrine from cardiac sympathetic neurons alters mechanical and electrical function. Cardiovasc. Res. 105, 143–150. doi: 10.1093/cvr/cvu258
Wharton, J., Polak, J. M., Gordon, L., Banner, N. R., Springall, D. R., Rose, M., et al. (1990). Immunohistochemical demonstration of human cardiac innervation before and after transplantation. Circ. Res. 66, 900–912. doi: 10.1161/01.RES.66.4.900
Wilde, A. A., Bhuiyan, Z. A., Crotti, L., Facchini, M., De Ferrari, G. M., Paul, T., et al. (2008). Left cardiac sympathetic denervation for catecholaminergic polymorphic ventricular tachycardia. N. Engl. J. Med. 358, 2024–2029. doi: 10.1056/NEJMoa0708006
Winbury, M. M., and Green, D. M. (1952). Studies on the nervous and humoral control of coronary circulation. Am. J. Physiol. 170, 555–563. doi: 10.1152/ajplegacy.1952.170.3.555
Yamakawa, K., So, E. L., Rajendran, P. S., Hoang, J. D., Makkar, N., Mahajan, A., et al. (2014). Electrophysiological effects of right and left vagal nerve stimulation on the ventricular myocardium. Am. J. Physiol. Heart Circ. Physiol. 307, H722–H731. doi: 10.1152/ajpheart.00279.2014
Yancy, C. W., Jessup, M., Bozkurt, B., Butler, J., Casey, D. E. Jr., Drazner, M. H., et al. (2013). 2013 ACCF/AHA guideline for the management of heart failure: a report of the american college of cardiology foundation/american heart association task force on practice guidelines. J. Am. Coll. Cardiol. 62, e147–e.239. doi: 10.1016/j.jacc.2013.05.019
Yanowitz, F., Preston, J. B., and Abildskov, J. A. (1966). Functional distribution of right and left stellate innervation to the ventricles. Production of neurogenic electrocardiographic changes by unilateral alteration of sympathetic tone. Circ. Res. 18, 416–428. doi: 10.1161/01.RES.18.4.416
Yoo, P. B., Lubock, N. B., Hincapie, J. G., Ruble, S. B., Hamann, J. J., and Grill, W. M. (2013). High-resolution measurement of electrically-evoked vagus nerve activity in the anesthetized dog. J. Neural. Eng. 10:026003. doi: 10.1088/1741-2560/10/2/026003
Yoshie, K., Rajendran, P. S., Massoud, L., Mistry, J., Swid, M. A., Wu, X., et al. (2020). Cardiac TRPV1 afferent signaling promotes arrhythmogenic ventricular remodeling after myocardial infarction. JCI Insight 5:e124477. doi: 10.1172/jci.insight.124477
Yuan, B. X., Ardell, J. L., Hopkins, D. A., and Armour, J. A. (1993). Differential cardiac responses induced by nicotine sensitive canine atrial and ventricular neurones. Cardiovasc. Res. 27, 760–769. doi: 10.1093/cvr/27.5.760
Zannad, F., De Ferrari, G. M., Tuinenburg, A. E., Wright, D., Brugada, J., Butter, C., et al. (2015). Chronic vagal stimulation for the treatment of low ejection fraction heart failure: results of the neural cardiac therapy for heart failure (NECTAR-HF) randomized controlled trial. Eur. Heart J. 36, 425–433. doi: 10.1093/eurheartj/ehu345
Zemelman, B. V., Lee, G. A., Ng, M., and Miesenbock, G. (2002). Selective photostimulation of genetically charged neurons. Neuron 33, 15–22. doi: 10.1016/S0896-6273(01)00574-8
Zhang, Y., Popovic, Z. B., Bibevski, S., Fakhry, I., Sica, D. A., Van Wagoner, D. R., et al. (2009). Chronic vagus nerve stimulation improves autonomic control and attenuates systemic inflammation and heart failure progression in a canine high-rate pacing model. Circ. Heart Fail. 2, 692–699. doi: 10.1161/CIRCHEARTFAILURE.109.873968
Zhao, M., He, X., Bi, X. Y., Yu, X. J., Wier, W. G., and Zang, W. J. (2013). Vagal stimulation triggers peripheral vascular protection through the cholinergic anti-inflammatory pathway in a rat model of myocardial ischemia/reperfusion. Basic Res. Cardiol. 108:345. doi: 10.1007/s00395-013-0345-1
Zhou, S., Chen, L. S., Miyauchi, Y., Miyauchi, M., Kar, S., Kangavari, S., et al. (2004). Mechanisms of cardiac nerve sprouting after myocardial infarction in dogs. Circ. Res. 95, 76–83. doi: 10.1161/01.RES.0000133678.22968.e3
Keywords: vagus nerve, neuromodulation, neurocardiology, autonomic nervous system, heart failure, myocardial infaraction, arrhythmia, sympathectomy
Citation: Hadaya J and Ardell JL (2020) Autonomic Modulation for Cardiovascular Disease. Front. Physiol. 11:617459. doi: 10.3389/fphys.2020.617459
Edited by:
Eugene Nalivaiko, The University of Newcastle, AustraliaReviewed by:
Peter John Schwartz, Istituto Auxologico Italiano (IRCCS), ItalyDavid Mendelowitz, George Washington University, United States
Copyright © 2020 Hadaya and Ardell. This is an open-access article distributed under the terms of the Creative Commons Attribution License (CC BY). The use, distribution or reproduction in other forums is permitted, provided the original author(s) and the copyright owner(s) are credited and that the original publication in this journal is cited, in accordance with accepted academic practice. No use, distribution or reproduction is permitted which does not comply with these terms.
*Correspondence: Jeffrey L. Ardell, amFyZGVsbEBtZWRuZXQudWNsYS5lZHU=