- 1Department of Kinesiology, The University of Texas at Arlington, Arlington, TX, United States
- 2Department of Kinesiology and Health Education, The University of Texas at Austin, Austin, TX, United States
Emerging evidence suggests that type 2 diabetes (T2D) may impair the ability to properly adjust the circulation during exercise with augmented blood pressure (BP) and an attenuated contracting skeletal muscle blood flow (BF) response being reported. This review provides a brief overview of the current understanding of these altered exercise responses in T2D and the potential underlying mechanisms, with an emphasis on the sympathetic nervous system and its regulation during exercise. The research presented support augmented sympathetic activation, heightened BP, reduced skeletal muscle BF, and impairment in the ability to attenuate sympathetically mediated vasoconstriction (i.e., functional sympatholysis) as potential drivers of neurovascular dysregulation during exercise in T2D. Furthermore, emerging evidence supporting a contribution of the exercise pressor reflex and central command is discussed along with proposed future directions for studies in this important area of research.
Introduction
Type 2 diabetes (T2D) negatively impacts cardiovascular health, contributing to a high risk of premature mortality (Raghavan et al., 2019). Notably, ~60% of T2D patients also have hypertension, which suggests that alterations in blood pressure (BP) control are common in this population (Colosia et al., 2013). This is all very important because more than 34 million Americans currently live with T2D, a number projected to increase nearly 50% by 2050 (Boyle et al., 2010; Prevention, 2020). Notably, the prevalence of T2D and prediabetes in young adults is also increasing (Mayer-Davis et al., 2017; Prevention, 2020), which is alarming considering that an earlier disease onset is associated with high lifetime risk of cardiovascular disease (Song, 2016). These rates are, in part, attributable to the accelerated rates of sedentary lifestyle in our society (Mayer-Davis and Costacou, 2001). Indeed, physical inactivity has been shown to be an important modifiable risk factor that contributes to the development of T2D (Bowden Davies et al., 2019; Antwi et al., 2020). Although exercise is a well-recognized tool in the management of T2D due to its many cardiometabolic benefits, accumulating evidence suggests that T2D negatively affects cardiovascular responses to exercise. The most alarming consequence is an exaggerated exercise-induced BP (Scott et al., 2008; Regensteiner et al., 2009; Pinto et al., 2014; O'Connor et al., 2015; Holwerda et al., 2016a), a response associated with a heightened risk of acute adverse cerebral- and cardiovascular events (Kurl et al., 2001; Laukkanen et al., 2006). Furthermore, several studies also report reductions in contracting skeletal muscle blood flow (BF) in T2D, which likely hinders the ability to sustain exercise contributing to the well-known exercise intolerance in this population (O'Connor et al., 2012; Reusch et al., 2013; Sacre et al., 2015; Senefeld et al., 2019). Therefore, understanding the underlying mechanisms contributing to altered cardiovascular responses to exercise in T2D is crucial to identifying strategies that can reduce the heightened cardiovascular risk in this population, while at the same time improving their ability to perform and sustain physical activity.
The sympathetic nervous system plays an integral role in controlling the cardiovascular adjustments to exercise. Increases in sympathetic nerve activity (SNA) to the heart facilitate increases in cardiac output and sympathetic outflow to periphery and viscera elicits vasoconstriction of inactive skeletal muscle and tissue beds, respectively. These actions contribute to elevations in BP while facilitating increases in BF to contracting muscles (Fisher et al., 2015). Moreover, within contracting muscles, the interaction between sympathetically mediated vasoconstrictor drive and local vasodilatory factors determines active skeletal muscle BF. In this regard, locally released vasoactive compounds attenuate sympathetically mediated vasoconstriction (i.e., functional sympatholysis) (Remensnyder et al., 1962) to further facilitate increases in active muscle BF. Several neural mechanisms work in concert to facilitate these adjustments. Feedback signals from the contracting skeletal muscle (i.e., exercise pressor reflex) and feedforward signals from higher brain centers (i.e., central command) both contribute to increase SNA during exercise (Alam and Smirk, 1937; Goodwin et al., 1972; McCloskey and Mitchell, 1972). These signals also contribute to the resetting of the arterial and cardiopulmonary baroreflex, which play important roles in modulating exercise-induced increases in BP via alterations in SNA (Scherrer et al., 1990; Fadel et al., 2001; Joyner, 2006). Thus, proper neural adjustments are essential to ensuring the appropriate sympathetic and thus cardiovascular responses to exercise.
Emerging evidence suggests that T2D impairs the ability to adjust the circulation during exercise, which is highlighted by reports of exaggerated muscle SNA (MSNA) (Holwerda et al., 2016a; Vranish et al., 2020), BP (Scott et al., 2008; Regensteiner et al., 2009; Pinto et al., 2014; O'Connor et al., 2015; Holwerda et al., 2016a), and attenuated increases in contracting skeletal muscle BF (Menon et al., 1992; Kingwell et al., 2003; Lalande et al., 2008; Mac Ananey et al., 2011; Kiely et al., 2014; O'Connor et al., 2015; Groen et al., 2019; Bock et al., 2020). Therefore, the purpose of this review is to provide a brief update on the current understanding of these altered exercise responses in T2D and the potential underlying mechanisms, with an emphasis on the sympathetic nervous system and its regulation during exercise. Furthermore, we discuss emerging evidence supporting a contribution of the exercise pressor reflex and central command along with proposed future directions for studies in this important area of research.
Cardiovascular Responses to Exercise in Type 2 Diabetes
The first data identifying an exaggerated exercise-induced BP response in T2D patients came from studies assessing cardiovascular responses to maximal and submaximal exercise testing (Kingwell et al., 2003; Petrofsky et al., 2005; Scott et al., 2008; Regensteiner et al., 2009; Karavelioglu et al., 2013; Pinto et al., 2014; O'Connor et al., 2015). For example, Scott et al. (2008) reported an ~50% greater prevalence of an exaggerated BP response to a graded maximal treadmill test in normotensive middle-aged T2D patients relative to controls. Furthermore, an augmented BP during submaximal steady-state and short-duration constant load cycling exercise has also been reported (Andresen and Kunze, 1994; O'Connor et al., 2015). Notably, these responses were also present at an early age in which studies have shown augmented BP responses to exercise in adolescents with T2D (Pinto et al., 2014; Yardley et al., 2015). Thus, these responses highlight that T2D patients are more likely to experience exaggerated exercise-induced BP responses, which is a prognostic indicator for an augmented risk of acute myocardial infarction and stroke (Kurl et al., 2001; Laukkanen et al., 2006). A recent study by Holwerda et al. (2016a) linked the augmented BP responses to exercise in T2D with elevated SNA demonstrating, for the first time, that T2D patients, independent of coexisting hypertension, had exaggerated MSNA responses to isometric handgrip exercise compared to nondiabetic controls (Figure 1).
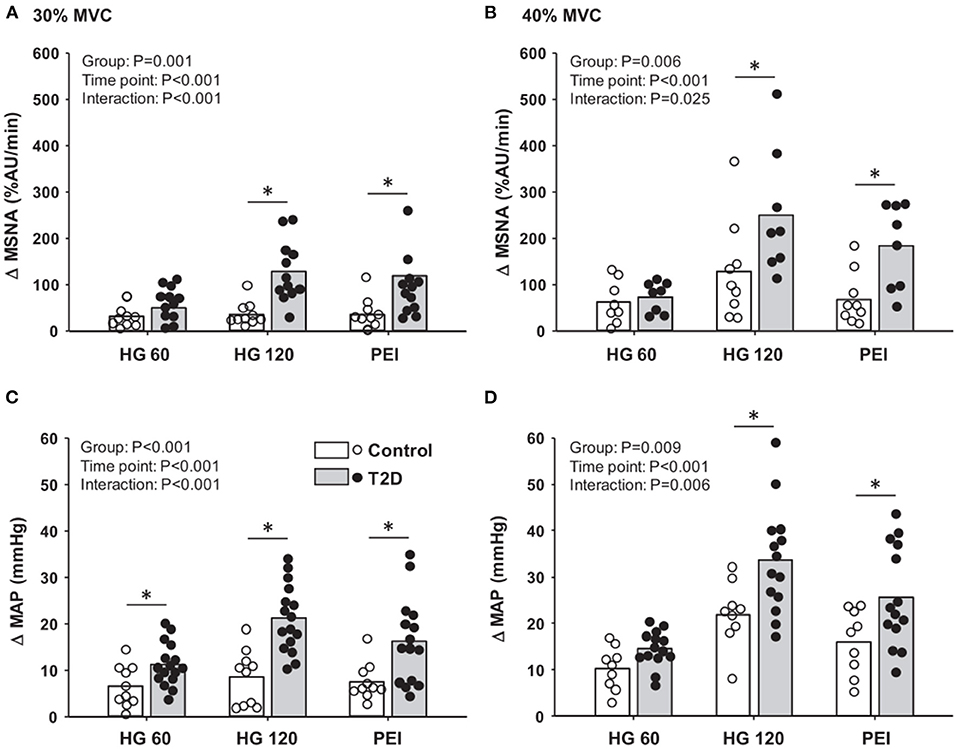
Figure 1. Mean data and individual data showing changes in muscle sympathetic nerve activity (MSNA; A,B) and mean arterial pressure (MAP; C,D) at 60 and 120 s of 30 and 40% maximal voluntary contraction (MVC) handgrip followed by subsequent periods of post-exercise ischemia (PEI) in type 2 diabetes (T2D) patients and control subjects. *P < 0.05 vs. control. Modified from Holwerda et al. (2016a) with permission.
Another common feature reported in studies examining exercise responses in T2D has been reductions in contracting skeletal muscle BF (Menon et al., 1992; Kingwell et al., 2003; Lalande et al., 2008; Mac Ananey et al., 2011; Kiely et al., 2014; O'Connor et al., 2015; Groen et al., 2019; Bock et al., 2020). For example, seminal work by Kingwell et al. (2003) showed attenuated leg BF responses to submaximal supine cycling exercise (60% VO2 peak) in normotensive T2D patients. Consequently, leg vascular resistance was substantially greater in the T2D patients. Similarly, a more recent study (Groen et al., 2019) reported attenuated increases in leg BF and vascular conductance in T2D patients during single-leg knee extension exercise. However, it should be noted that conflicting findings have also been reported (Martin et al., 1995; Copp et al., 2010; Poitras et al., 2015). For example, Poitras et al. (2015) found similar increases in forearm muscle BF during a forearm critical force test in T2D patients and controls. Nonetheless, there are substantial data reporting that T2D significantly impacts the ability to properly adjust the circulation during exercise, leading to an augmented BP and reduced contracting skeletal muscle BF. For complementary details on exercise impairments in T2D, the reader is referred to other excellent reviews (Reusch et al., 2013; Green et al., 2015; Poitras et al., 2018; Kim et al., 2020; Nesti et al., 2020).
Neurovascular Regulation During Exercise in Health
In this section, we will provide a brief overview of appropriate neurovascular regulatory mechanisms during exercise to set the stage for future sections on what is known about the potential impairments in neurovascular control mechanisms in T2D. Several neural mechanisms work in concert to regulate BP and adjust the circulation during exercise. The exercise pressor reflex is a feedback mechanism that responds to mechanical (i.e., mechanoreflex) and metabolic (i.e., metaboreflex) stimuli produced by the contracting skeletal muscle and reflexively increases MSNA, BP, HR, and respiration (Alam and Smirk, 1937; McCloskey and Mitchell, 1972; Strange et al., 1993). The afferent arm of this reflex is comprised of thinly myelinated group III (predominantly mechanically sensitive) and unmyelinated group IV (predominantly metabolically sensitive) muscle afferents (McCloskey and Mitchell, 1972; Kaufman et al., 1983). However, these afferents exhibit polymodal characteristics (Rotto and Kaufman, 1988; Rotto et al., 1990). Central command is a feedforward mechanism referring to descending signals originating from higher brain areas that simultaneously increase motor efferent drive and autonomic neural outflow that, in turn, contributes to the cardiorespiratory responses to exercise (Goodwin et al., 1972; Eldridge et al., 1981, 1985). Furthermore, both the exercise pressor reflex and central command contribute to the resetting of the arterial and cardiopulmonary baroreflex (Bevegard and Shepherd, 1966; Papelier et al., 1994; Gallagher et al., 2006). The arterial baroreflex is a negative feedback mechanism that modulates BP at rest and during exercise by making rapid cardiovascular adjustments in response to beat-to-beat changes in BP. On the other hand, the cardiopulmonary baroreflex responds to changes in central pressure and volume by reflexively adjusting MSNA at rest and during exercise (Ray et al., 1993; Ogoh et al., 2007). These neural mechanisms all converge centrally in the nucleus tractus solitarius of the medulla oblongata (Andresen and Kunze, 1994; Potts et al., 2002) and ultimately adjust sympathetic outflow via neurons in the rostral ventral lateral medulla. It is worth noting that skeletal muscle afferents also increase SNA via direct projections to the rostral ventral lateral medulla (Potts, 2006). For more in-depth discussions on neurovascular control, we refer the reader to several comprehensive reviews (Fadel and Raven, 2012; Fisher et al., 2015; Holwerda et al., 2015; Michelini et al., 2015; Nyberg et al., 2015; Grotle et al., 2020).
The resultant increases in SNA directed to visceral and peripheral blood vessels have powerful vasoconstrictor and BP-raising effects during exercise (Fairfax et al., 2013). Indeed, sympathetic vasoconstriction of inactive tissue and skeletal muscle vascular beds increases to effectively redistribute cardiac output to active skeletal muscle (Joyner et al., 1992; Rowell, 1997; Saltin et al., 1998). At the same time, sympathetically mediated vasoconstriction is attenuated in active skeletal muscle beds by local vasoactive compounds (i.e., functional sympatholysis) (Remensnyder et al., 1962) to further facilitate increases in contracting skeletal muscle BF. Although extensively studied, the sympatholytic compounds responsible for functional sympatholysis and their mechanism(s) of action are not well understood. Nevertheless, accumulating evidence supports a significant contribution played by ATP (Rosenmeier et al., 2004; Saltin and Mortensen, 2012), which appears to mediate its effect, in part, by attenuating the sensitivity of α-adrenergic receptors (Mortensen et al., 2009). Although less clear, NO also appears to contribute (Thomas and Victor, 1998), but its role may depend on the presence of other compounds such as prostacyclin (Dinenno and Joyner, 2004; Mortensen et al., 2007). Regardless, numerous studies demonstrate the presence of functional sympatholysis and its importance for increasing contracting skeletal muscle BF. Of note, functional sympatholysis does not mean complete “lysis” of sympathetic vasoconstriction but rather an attenuation that allows for increases in active skeletal muscle BF while still contributing to the maintenance of BP.
In addition to sympathetic control, non-adrenergic vasoconstrictor and vasodilatory compounds also contribute to the regulation of contracting skeletal muscle BF (Saltin and Mortensen, 2012; Holwerda et al., 2015). These can be released by skeletal muscle, endothelial cells, nerve terminals, and circulating erythrocytes in response to increased mechanical stimuli and metabolic activity during exercise. The interplay between these factors is complex and incompletely understood due, in part, to significant redundancy. Moreover, the participation of each pathway or compound may change from the onset to steady-state exercise (Clifford and Hellsten, 2004). Vasodilatory compounds include nitric oxide (NO), prostacyclin, adenosine, potassium, and ATP (Clifford and Hellsten, 2004; Clifford, 2007), which can act through endothelial-dependent or independent pathways. Additionally, non-adrenergic vasoconstrictors such as interstitial ATP, neuropeptide Y, endothelin 1, and angiotensin II also contribute to the vasoconstrictive influence during exercise (Holwerda et al., 2015); however, it should be noted that an attenuation of non-adrenergic vasoconstrictor pathways in active skeletal muscle has been reported (Brothers et al., 2006; Wray et al., 2007). Of note, the contribution of non-adrenergic vasoactive compounds to BF responses during exercise may not be as prominent in healthy individuals; however, it appears to increase with aging and disease (Schreuder et al., 2014; Barrett-O'Keefe et al., 2015; Nyberg et al., 2015).
Neurovascular Dysregulation During Exercise in Type 2 Diabetes
Until recently, little was known regarding the underlying mechanisms for the impaired cardiovascular responses to exercise observed in T2D. In this regard, emerging evidence suggests that the exercise pressor reflex plays a prominent role in evoking exaggerated MSNA and BP responses to exercise in T2D (Figure 2). For example, T2D rats have exaggerated renal SNA and BP responses to electrically evoked static muscle contractions in the absence of central command (Grotle et al., 2019; Kim et al., 2019). Both the mechanoreflex and metaboreflex have been shown to contribute to the exaggerated exercise pressor reflex in T2D. Indeed, T2D rats exhibit augmented BP responses to isolated mechanical stimuli (i.e., tendon stretch), suggesting an augmented mechanoreflex (Grotle et al., 2019). Furthermore, studies in humans suggest T2D also augments the metaboreflex (Holwerda et al., 2016a; Roberto et al., 2019). Specifically, Holwerda et al. (2016a) showed exaggerated MSNA and BP responses to postexercise ischemia (PEI) following isometric handgrip exercise (30 and 40% maximal voluntary contraction: MVC; Figure 1). This maneuver traps the metabolites produced during exercise and isolates the muscle metaboreflex. Interestingly, the magnitude of MSNA response to PEI was positively correlated with metabolic markers of disease severity (glucose, HbA1c, and HOMA-IR), which suggests that the severity of disease has significant impact on the enhanced expression of the exercise pressor reflex in T2D. In this regard, a recent study (Roberto et al., 2019) that reported a normal BP response to PEI following rhythmic handgrip exercise (30% MVC) had a cohort of T2D patients with relatively well-controlled blood glucose (106.41 ± 11.2 mg/dl; HbA1c 7.05 ± 0.10%). Although it is possible that the lower-intensity rhythmic exercise did not produce sufficient metabolic stimuli to unmask a difference in metaboreflex-induced BP responses between groups, notably T2D patients in this study exhibited exaggerated vasoconstriction during PEI. Interestingly, a recent study showed enhanced BP responses to ischemic rhythmic handgrip exercise (30% MVC) in nondiabetic individuals with greater insulin resistance than those with lower insulin resistance (Hotta et al., 2020). Overall, the existing literature supports that both components of the exercise pressor reflex (i.e., mechano- and metabo-reflex) may be enhanced in T2D (Figure 2).
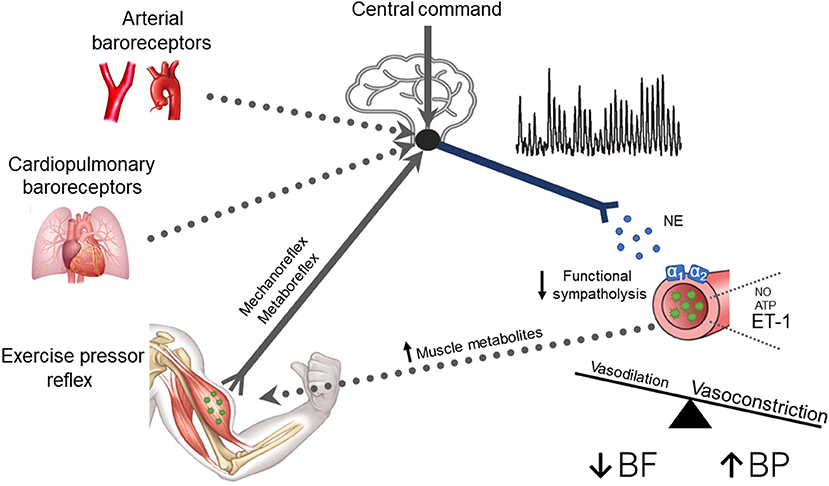
Figure 2. A schematic of potential neural mechanisms responsible for neurovascular dysregulation in type 2 diabetes (T2D). Current evidence supports a contribution of the exercise pressor reflex and central command (solid lines), whereas the contribution of the arterial and cardiopulmonary baroreflex remains unclear (dotted lines). Briefly, T2D patients exhibit exaggerated muscle sympathetic nerve activity (MSNA) responses, which increases the release of norepinephrine (NE) binding to α1 and α2 adrenergic receptors causing constriction of peripheral vascular beds. T2D may also impair the normal ability to attenuate sympathetically mediated vasoconstriction in active skeletal muscle (i.e., reduced functional sympatholysis). Additionally, enhanced endothelin-1-mediated vasoconstriction and reduced nitric oxide (NO) and adenosine triphosphate (ATP) mediated vasodilation may also contribute to the reduced exercise hyperemia in T2D. These alterations favor vasoconstriction, leading to reduced active skeletal muscle blood flow and exaggerated BP responses to exercise. Thus, it is plausible that T2D leads to a positive feedback scenario where enhanced MSNA and reduced functional sympatholysis combined with other impaired local vascular control pathways augment metabolic distress, which in turn further enhances MSNA by stimulating the muscle metaboreflex.
The underlying mechanisms for an augmented exercise pressor reflex in T2D remain unknown. Specifically, it will be important to determine whether these mechanisms involve greater metabolite accumulation during skeletal muscle contraction, enhanced afferent sensitivity, or increased expression of mechanically or metabolically sensitive receptors and/or channels, or abnormal central integration of afferent feedback. It is possible that a slowed and attenuated hyperemic response to contracting skeletal muscle during exercise enhances muscle metabolite buildup, thereby increasing the activation of the metaboreflex and simultaneously sensitizing the mechanoreflex (Adreani and Kaufman, 1998; Holwerda et al., 2016a; Nesti et al., 2020). Additionally, there are reports of increased reliance on carbohydrate metabolism (Martin et al., 1995; Scheuermann-Freestone et al., 2003), reduced capillary density and recruitment, increased fast twitch muscle fiber type recruitment (Marin et al., 1994; Padilla et al., 2006; Womack et al., 2009), and increased leg lactate output during exercise (Martin et al., 1995) in T2D. These alterations may indicate a greater propensity for metabolite production during exercise in T2D. Furthermore, oxidative stress may also contribute as it is an important mediator of well-known complications of T2D (e.g., vascular dysfunction and peripheral neuropathy) (Giacco and Brownlee, 2010) and may directly influence thin fiber afferent activity (Delliaux et al., 2009). Indeed, studies have reported that reactive oxygen species may play a role in evoking the exaggerated exercise pressor reflex in common comorbidities of T2D (e.g., hypertension, peripheral artery disease, and heart failure) (Koba et al., 2009, 2013; Wang et al., 2009; Muller et al., 2012; Harms et al., 2017). Thus, it is plausible that the increased presence of metabolites and/or reactive oxygen species may contribute to the exaggerated exercise pressor reflex in T2D (Grotle and Stone, 2019). However, additional studies are needed.
Recent evidence suggests that insulin and glucose may also play a role in augmenting thin fiber afferent activity. Specifically, Hotta et al. (2019) used a whole-cell patch-clamp preparation to show that local application of insulin to small dorsal root ganglion in healthy mice decreased their mechanical threshold and augmented the amplitude of mechanically activated currents, whereas antagonizing insulin receptors attenuated this response. Furthermore, they used an isolated muscle-nerve preparation to show that application of insulin decreased the threshold, but not the magnitude, of mechanically evoked group IV afferent activity. In terms of glucose, a recent study showed that acutely infusing glucose into the hindlimb to concentrations observed in T2D rats did not affect the exercise pressor reflex or either of its two components (i.e., mechanoreflex and metaboreflex) in healthy rats (Huo et al., 2020). However, these responses may be different in T2D rats. Notably, Ishizawa et al. (2021) recently reported an augmented pressor and renal SNA response to capsaicin (chemically sensitive TRPV1 receptor agonist) administration in the hindlimb of T2D rats compared to controls. Furthermore, group IV muscle afferents isolated from T2D rats exhibited exaggerated capsaicin-induced discharge frequency, which was related to blood glucose concentrations. This aligns with findings in T2D patients showing a positive association between the MSNA response to isolated muscle metaboreflex activation and fasting blood glucose and HbA1c (Holwerda et al., 2016a). Thus, together these findings suggest that both insulin and glucose may play a role in augmenting the exercise pressor reflex in T2D. However, further studies are warranted.
Whether central command is impacted by T2D has received less attention. However, a recent study by Vranish et al. (2020) reported augmented MSNA and BP responses in T2D patients at the early onset of exercise, evident as early as 10 s into isometric handgrip. Although by no means direct evidence for central command involvement, these data at least suggest the possibility of heightened central command responses in T2D. A recent study (Kim et al., 2019) using a high-fat diet and streptozotocin-induced T2D model in rats supports a contribution of central command in evoking exaggerated SNA and BP responses to exercise (Figure 2). These investigators reported that neural stimulation of the mesencephalic locomotor region, a putative area for the central command pathway, resulted in greater renal SNA, HR, and BP responses in T2D rats compared to controls. The underlying mechanisms for these responses are not known but could involve direct alterations to brain areas responsible for central command, or the central integration of signals. Possible contributors include enhanced central angiotensin II or oxidative stress-mediated reductions in NO within the NTS, both of which have been proposed as contributors to augmented SNA reactivity to exercise in hypertension, a common comorbidity in T2D (Song et al., 1994; Zimmerman et al., 2002; Leal et al., 2012, 2013). T2D also appears to impair dynamic cerebral autoregulation during exercise, which suggests the potential for direct cerebral vascular contributions (Vianna et al., 2015). For more information on the effects of T2D on cerebral vascular regulation during exercise, the reader is directed to a recent review (Kim et al., 2020).
It is also plausible that enhanced SNA responses during exercise in T2D include an altered interaction between central command and the exercise pressor reflex (Amann et al., 2008). Painful peripheral diabetic neuropathy is a common feature in T2D and affects similar sensory afferents as those evoking the exercise pressor reflex (Davies et al., 2006). Thus, considering that effort sense influences central command (Williamson et al., 2001), it is possible that activation of sensory afferents (i.e., ergoreceptors and nociceptors) evoking augmented SNA and muscle pain also increases central command by influencing one's perceived effort during exercise. Indeed, ischemia-induced muscle pain has been shown to increase effort sense during light resistance exercise in healthy individuals (Hollander et al., 2010). Moreover, attenuating afferent feedback during dynamic exercise lowers ratings of perceived exertion during exercise, a marker for central command activation (Amann et al., 2010). Notably, there is some evidence of higher perceived effort during exercise in T2D (Huebschmann et al., 2009; Kim et al., 2015). However, these interactions are complex and require further investigation.
Whether T2D impairs arterial and cardiopulmonary baroreflex function during exercise has not been directly tested (Figure 2). Several studies indicate that T2D attenuates cardiac baroreflex sensitivity at rest (Holwerda et al., 2016b; Cseh et al., 2020; Kuck et al., 2020). However, this may not be specific to T2D, as weight-matched controls also exhibit impaired cardiac baroreflex control at rest compared to lean controls suggesting that obesity rather than T2D impairs arterial baroreflex control of HR (Holwerda et al., 2016b). In contrast, arterial baroreflex control of MSNA appears to be preserved at rest in T2D (Holwerda et al., 2016b; Moura-Tonello et al., 2016). However, whether this is true also during exercise has not been directly tested. This is important because the arterial baroreflex plays an essential role in restraining sympathetic outflow during exercise (Joyner, 2006). Likewise, to our knowledge, no study has investigated the effect of T2D on cardiopulmonary baroreflex control of MSNA (Figure 2), which also has restraining effects on MSNA responses during exercise. There is a clear need for further studies.
In addition to an exaggerated MSNA response to exercise, recent research suggests T2D also appears to impair the ability to attenuate sympathetically mediated vasoconstriction in active skeletal muscle (i.e., functional sympatholysis; Figure 2). Specifically, Bock et al. (2020) demonstrated greater vasoconstrictor responses to intra-arterial infusion of α1 and α2–adrenergic receptor agonists in active muscle during rhythmic handgrip exercise in T2D patients compared to nondiabetic controls. It is important to note that these findings contrast with Thaning et al. (2011), demonstrating preserved functional sympatholysis in T2D. However, in this study, the T2D patients were relatively healthy and had normal vasodilatory responses to acetylcholine, suggesting normal endothelial function. This is interesting because recent work (Hearon et al., 2020) suggests that the degree of functional sympatholysis may be dependent on endothelial function and thus, could, in part, explain these disparate findings.
Non-adrenergic pathways may also contribute to the reduced BF responses in active skeletal muscle reported in T2D patients. Indeed, an imbalance favoring blunted endothelial-dependent vasodilation and enhanced non-adrenergic vasoconstrictors may be involved (Figure 2) (Mather et al., 2004; Malik et al., 2005; Frisbee et al., 2019). Although limited studies have been performed, there is some evidence that warrants discussion. For example, one study (Kingwell et al., 2003) showed that attenuated leg BF responses to dynamic exercise were significantly correlated with vasodilatory responses to acetylcholine but not to sodium nitroprusside infusion, suggesting that an impaired endothelial-dependent but not independent NO-mediated vasodilation may contribute. Furthermore, impaired ATP-mediated vasodilation, perhaps due to reduced ATP bioavailability or purinergic receptor sensitivity, may also be involved (Thaning et al., 2010; Groen et al., 2019). In terms of vasoconstrictors, blocking endothelin-1 receptors during rhythmic handgrip exercise has been shown to enhance muscle BF responses in T2D patients but not in healthy controls, indicating augmented endothelin 1-mediated vasoconstriction during exercise in T2D (Schreuder et al., 2014). Collectively, these findings suggest that attenuated vasodilation via reduced NO and ATP, in combination with enhanced vasoconstrictor influence via endothelin-1, may also impair the ability to appropriately regulate contracting skeletal muscle BF in T2D. Notably, these changes in non-adrenergic pathways, along with the heightened MSNA and impaired functional sympatholysis, likely also contribute to the exaggerated BP response to exercise reported in T2D patients. Additionally, recent work suggests that augmented exercise-induced increases in arterial stiffness may also contribute to the exaggerated BP response to exercise in T2D (Cooke et al., 2020).
Considerations and Future Directions
T2D is a multifactorial disease with many potential contributing factors to the neural vascular dysregulation reported during exercise (e.g., diet, physical inactivity, aging, family history, sex, ethnicity, comorbidities, etc.). This likely contributes to variations in diabetic phenotypes (Stidsen et al., 2018) as well as potentially differential etiologies for exercise impairments (Nesti et al., 2020), making T2D a complex population to study. It is also important to note that differences in T2D characteristics (e.g., duration of diabetes, glycemic control, and varying medications), animal models used, and exercise modality employed (e.g., type, duration, intensity of exercise) may also influence the observed neural cardiovascular responses to exercise in T2D. In addition, the majority of studies discussed in this review include relatively well-controlled T2D patients and exclude those with diabetic complications (e.g., neuropathy, uncontrolled diabetes, coronary heart disease etc.). Thus, examining neural cardiovascular responses to exercise in T2D patients with associated complications warrants future investigation. Likewise, although an augmented BP response to exercise is observed in several age groups and in both males and females, the influence of age and sex on neurovascular regulation during exercise in T2D has not been comprehensively assessed. It would be particularly interesting to determine the influence of sex hormones and menopausal status as estrogen has been shown to affect the cardiovascular responses to exercise (Schmitt and Kaufman, 2003; Fadel et al., 2004; Jarvis et al., 2011). Studies are also needed to further understand the contribution of factors such as insulin resistance, obesity, and physical inactivity. Notably, two studies indicate that insulin resistance may be a stronger predictor of an augmented metaboreflex than obesity in nondiabetic individuals (Milia et al., 2015; Hotta et al., 2020). However, others have demonstrated that obesity may drive negative effects on neural cardiovascular control mechanisms in T2D patients (Holwerda et al., 2016b). Moreover, the influence of physical inactivity on neural cardiovascular control in T2D is unclear and also warrants future investigation. Thus, overall, we have a lot more to learn about cardiovascular responses to exercise and underlying mechanisms in T2D and elucidating the role of the above mentioned factors in future studies will be important to consider as this field moves forward.
Conclusion
T2D patients are at a twofold higher risk of premature mortality, contributing to a significantly shorter life expectancy (Raghavan et al., 2019). Emerging evidence suggests that neurovascular dysregulation leading to enhanced SNA and BP reactivity to exercise or daily physical activities (e.g., carrying groceries, walking up stairs) may increase the already heightened risk for adverse cardiac events and stroke in this population. Although mechanisms for this are just starting to emerge, evidence supports a contribution of the exercise pressor reflex and central command. However, further studies are needed and elucidation of potential roles for the arterial and cardiopulmonary baroreflex requires investigation. Additionally, augmented α1 and α2 adrenergic receptor sensitivity, endothelin-1-mediated vasoconstriction and blunted NO, and ATP-mediated vasodilation may also contribute. Indeed, T2D may lead to a scenario where enhanced MSNA, via the exercise pressor reflex and central command, along with impaired local vascular control mechanisms (adrenergic and non-adrenergic) attenuates increases in exercising muscle BF and augments the BP response to exercise. Nonetheless, there is a clear need for future studies to investigate the impact of T2D on neural and vascular control mechanisms and their interaction during exercise.
Author Contributions
A-KG drafted the manuscript, which was critically evaluated by JK, AS, and PF. All authors approved the submitted version. All authors contributed to the article and approved the final version.
Funding
PF is funded, in part, by the National Heart, Lung, and Blood Institute HL-127071. AS is funded by the National Heart, Lung, and Blood Institute HL-144723.
Conflict of Interest
The authors declare that the research was conducted in the absence of any commercial or financial relationships that could be construed as a potential conflict of interest.
References
Adreani, C. M., and Kaufman, M. P. (1998). Effect of arterial occlusion on responses of group III and IV afferents to dynamic exercise. J. Appl. Physiol. (1985) 84, 1827–1833. doi: 10.1152/jappl.1998.84.6.1827
Alam, M., and Smirk, F. H. (1937). Observations in man upon a blood pressure raising reflex arising from the voluntary muscles. J. Physiol. 89, 372–383. doi: 10.1113/jphysiol.1937.sp003485
Amann, M., Blain, G. M., Proctor, L. T., Sebranek, J. J., Pegelow, D. F., and Dempsey, J. A. (2010). Group III and IV muscle afferents contribute to ventilatory and cardiovascular response to rhythmic exercise in humans. J. Appl. Physiol. (1985) 109, 966–976. doi: 10.1152/japplphysiol.00462.2010
Amann, M., Proctor, L. T., Sebranek, J. J., Eldridge, M. W., Pegelow, D. F., and Dempsey, J. A. (2008). Somatosensory feedback from the limbs exerts inhibitory influences on central neural drive during whole body endurance exercise. J. Appl. Physiol. (1985) 105, 1714–1724. doi: 10.1152/japplphysiol.90456.2008
Andresen, M. C., and Kunze, D. L. (1994). Nucleus tractus solitarius–gateway to neural circulatory control. Annu. Rev. Physiol. 56, 93–116. doi: 10.1146/annurev.ph.56.030194.000521
Antwi, J., Lavin, R., Sullivan, S., and Bellavia, M. (2020). Perception of and risk factors for type 2 diabetes among students attending an upstate New York college: a pilot study. Diabetol. Metab. Syndr. 12:25. doi: 10.1186/s13098-020-00535-1
Barrett-O'Keefe, Z., Ives, S. J., Trinity, J. D., Morgan, G., Rossman, M. J., Donato, A. J., et al. (2015). Endothelin-A-mediated vasoconstriction during exercise with advancing age. J. Gerontol. A Biol. Sci. Med. Sci. 70, 554–565. doi: 10.1093/gerona/glu065
Bevegard, B. S., and Shepherd, J. T. (1966). Circulatory effects of stimulating the carotid arterial stretch receptors in man at rest and during exercise. J. Clin. Invest. 45, 132–142. doi: 10.1172/JCI105317
Bock, J. M., Hughes, W. E., Ueda, K., Feider, A. J., Hanada, S., Kruse, N. T., et al. (2020). Greater alpha1-adrenergic-mediated vasoconstriction in contracting skeletal muscle of patients with type 2 diabetes. Am. J. Physiol. Heart Circ. Physiol. 319, H797–H807. doi: 10.1152/ajpheart.00532.2020
Bowden Davies, K. A., Pickles, S., Sprung, V. S., Kemp, G. J., Alam, U., Moore, D. R., et al. (2019). Reduced physical activity in young and older adults: metabolic and musculoskeletal implications. Ther. Adv. Endocrinol. Metab. 10:2042018819888824. doi: 10.1177/2042018819888824
Boyle, J. P., Thompson, T. J., Gregg, E. W., Barker, L. E., and Williamson, D. F. (2010). Projection of the year 2050 burden of diabetes in the US adult population: dynamic modeling of incidence, mortality, and prediabetes prevalence. Popul. Health Metr. 8:29. doi: 10.1186/1478-7954-8-29
Brothers, R. M., Haslund, M. L., Wray, D. W., Raven, P. B., and Sander, M. (2006). Exercise-induced inhibition of angiotensin II vasoconstriction in human thigh muscle. J. Physiol. 577, 727–737. doi: 10.1113/jphysiol.2006.113977
Clifford, P. S. (2007). Skeletal muscle vasodilatation at the onset of exercise. J. Physiol. 583, 825–833. doi: 10.1113/jphysiol.2007.135673
Clifford, P. S., and Hellsten, Y. (2004). Vasodilatory mechanisms in contracting skeletal muscle. J. Appl. Physiol. (1985) 97, 393–403. doi: 10.1152/japplphysiol.00179.2004
Colosia, A. D., Palencia, R., and Khan, S. (2013). Prevalence of hypertension and obesity in patients with type 2 diabetes mellitus in observational studies: a systematic literature review. Diabetes Metab. Syndr. Obes. 6, 327–338. doi: 10.2147/DMSO.S51325
Cooke, A. B., Dasgupta, K., Spronck, B., Sharman, J. E., and Daskalopoulou, S. S. (2020). Adults with type 2 diabetes mellitus exhibit a greater exercise-induced increase in arterial stiffness and vessel hemodynamics. Hypertension 75, 1565–1573. doi: 10.1161/HYPERTENSIONAHA.120.14778
Copp, S. W., Hageman, K. S., Behnke, B. J., Poole, D. C., and Musch, T. I. (2010). Effects of type II diabetes on exercising skeletal muscle blood flow in the rat. J. Appl. Physiol. (1985) 109, 1347–1353. doi: 10.1152/japplphysiol.00668.2010
Cseh, D., Climie, R. E., Offredo, L., Guibout, C., Thomas, F., Zanoli, L., et al. (2020). Type 2 diabetes mellitus is independently associated with decreased neural baroreflex sensitivity: the paris prospective Study III. Arterioscler. Thromb. Vasc. Biol. 40, 1420–1428. doi: 10.1161/ATVBAHA.120.314102
Davies, M., Brophy, S., Williams, R., and Taylor, A. (2006). The prevalence, severity, and impact of painful diabetic peripheral neuropathy in type 2 diabetes. Diabetes Care 29, 1518–1522. doi: 10.2337/dc05-2228
Delliaux, S., Brerro-Saby, C., Steinberg, J. G., and Jammes, Y. (2009). Reactive oxygen species activate the group IV muscle afferents in resting and exercising muscle in rats. Pflugers Arch. 459, 143–150. doi: 10.1007/s00424-009-0713-8
Dinenno, F. A., and Joyner, M. J. (2004). Combined NO and PG inhibition augments alpha-adrenergic vasoconstriction in contracting human skeletal muscle. Am. J. Physiol. Heart. Circ. Physiol. 287, H2576–2584. doi: 10.1152/ajpheart.00621.2004
Eldridge, F. L., Millhorn, D. E., Kiley, J. P., and Waldrop, T. G. (1985). Stimulation by central command of locomotion, respiration and circulation during exercise. Respir. Physiol. 59, 313–337. doi: 10.1016/0034-5687(85)90136-7
Eldridge, F. L., Millhorn, D. E., and Waldrop, T. G. (1981). Exercise hyperpnea and locomotion: parallel activation from the hypothalamus. Science 211, 844–846. doi: 10.1126/science.7466362
Fadel, P. J., Ogoh, S., Watenpaugh, D. E., Wasmund, W., Olivencia-Yurvati, A., Smith, M. L., et al. (2001). Carotid baroreflex regulation of sympathetic nerve activity during dynamic exercise in humans. Am. J. Physiol. Heart. Circ. Physiol. 280, H1383–1390. doi: 10.1152/ajpheart.2001.280.3.H1383
Fadel, P. J., and Raven, P. B. (2012). Human investigations into the arterial and cardiopulmonary baroreflexes during exercise. Exp. Physiol. 97, 39–50. doi: 10.1113/expphysiol.2011.057554
Fadel, P. J., Wang, Z., Watanabe, H., Arbique, D., Vongpatanasin, W., and Thomas, G. D. (2004). Augmented sympathetic vasoconstriction in exercising forearms of postmenopausal women is reversed by oestrogen therapy. J. Physiol. 561, 893–901. doi: 10.1113/jphysiol.2004.073619
Fairfax, S. T., Padilla, J., Vianna, L. C., Davis, M. J., and Fadel, P. J. (2013). Spontaneous bursts of muscle sympathetic nerve activity decrease leg vascular conductance in resting humans. Am. J. Physiol. Heart Circ. Physiol. 304, H759–766. doi: 10.1152/ajpheart.00842.2012
Fisher, J. P., Young, C. N., and Fadel, P. J. (2015). Autonomic adjustments to exercise in humans. Compr. Physiol. 5, 475–512. doi: 10.1002/cphy.c140022
Frisbee, J. C., Lewis, M. T., Kasper, J. D., Chantler, P. D., and Wiseman, R. W. (2019). Type 2 diabetes mellitus in the Goto-Kakizaki rat impairs microvascular function and contributes to premature skeletal muscle fatigue. J. Appl. Physiol. (1985) 126, 626–637. doi: 10.1152/japplphysiol.00751.2018
Gallagher, K. M., Fadel, P. J., Smith, S. A., Stromstad, M., Ide, K., Secher, N. H., et al. (2006). The interaction of central command and the exercise pressor reflex in mediating baroreflex resetting during exercise in humans. Exp. Physiol. 91, 79–87. doi: 10.1113/expphysiol.2005.032110
Giacco, F., and Brownlee, M. (2010). Oxidative stress and diabetic complications. Circ. Res. 107, 1058–1070. doi: 10.1161/CIRCRESAHA.110.223545
Goodwin, G. M., Mccloskey, D. I., and Mitchell, J. H. (1972). Cardiovascular and respiratory responses to changes in central command during isometric exercise at constant muscle tension. J. Physiol. 226, 173–190. doi: 10.1113/jphysiol.1972.sp009979
Green, S., Egana, M., Baldi, J. C., Lamberts, R., and Regensteiner, J. G. (2015). Cardiovascular control during exercise in type 2 diabetes mellitus. J. Diabetes Res. 2015:654204. doi: 10.1155/2015/654204
Groen, M. B., Knudsen, T. A., Finsen, S. H., Pedersen, B. K., Hellsten, Y., and Mortensen, S. P. (2019). Reduced skeletal-muscle perfusion and impaired ATP release during hypoxia and exercise in individuals with type 2 diabetes. Diabetologia 62, 485–493. doi: 10.1007/s00125-018-4790-0
Grotle, A. K., Crawford, C. K., Huo, Y., Ybarbo, K. M., Harrison, M. L., Graham, J., et al. (2019). Exaggerated cardiovascular responses to muscle contraction and tendon stretch in UCD type-2 diabetes mellitus rats. Am. J. Physiol. Heart Circ. Physiol 317, H479–H486. doi: 10.1152/ajpheart.00229.2019
Grotle, A. K., Macefield, V. G., Farquhar, W. B., O'leary, D. S., and Stone, A. J. (2020). Recent advances in exercise pressor reflex function in health and disease. Auton. Neurosci. 228:102698. doi: 10.1016/j.autneu.2020.102698
Grotle, A. K., and Stone, A. J. (2019). Exaggerated exercise pressor reflex in type 2 diabetes: potential role of oxidative stress. Auton. Neurosci. 222:102591. doi: 10.1016/j.autneu.2019.102591
Harms, J. E., Kuczmarski, J. M., Kim, J. S., Thomas, G. D., and Kaufman, M. P. (2017). The role played by oxidative stress in evoking the exercise pressor reflex in health and simulated peripheral artery disease. J. Physiol. 595, 4365–4378. doi: 10.1113/JP273816
Hearon, C. M. Jr., Richards, J. C., Racine, M. L., Luckasen, G. J., Larson, D. G., and Dinenno, F. A. (2020). Augmentation of endothelium-dependent vasodilatory signalling improves functional sympatholysis in contracting muscle of older adults. J. Physiol. 598, 2323–2336. doi: 10.1113/JP279462
Hollander, D. B., Reeves, G. V., Clavier, J. D., Francois, M. R., Thomas, C., and Kraemer, R. R. (2010). Partial occlusion during resistance exercise alters effort sense and pain. J. Strength Cond. Res. 24, 235–243. doi: 10.1519/JSC.0b013e3181c7badf
Holwerda, S. W., Restaino, R. M., and Fadel, P. J. (2015). Adrenergic and non-adrenergic control of active skeletal muscle blood flow: implications for blood pressure regulation during exercise. Auton. Neurosci. 188, 24–31. doi: 10.1016/j.autneu.2014.10.010
Holwerda, S. W., Restaino, R. M., Manrique, C., Lastra, G., Fisher, J. P., and Fadel, P. J. (2016a). Augmented pressor and sympathetic responses to skeletal muscle metaboreflex activation in type 2 diabetes patients. Am. J. Physiol. Heart Circ. Physiol. 310, H300–309. doi: 10.1152/ajpheart.00636.2015
Holwerda, S. W., Vianna, L. C., Restaino, R. M., Chaudhary, K., Young, C. N., and Fadel, P. J. (2016b). Arterial baroreflex control of sympathetic nerve activity and heart rate in patients with type 2 diabetes. Am. J. Physiol. Heart Circ. Physiol 311, H1170–H1179. doi: 10.1152/ajpheart.00384.2016
Hotta, N., Hori, A., Okamura, Y., Baba, R., Watanabe, H., Sugawara, J., et al. (2020). Insulin resistance is associated with an exaggerated blood pressure response to ischemic rhythmic handgrip exercise in nondiabetic older adults. J. Appl. Physiol. (1985) 129, 144–151. doi: 10.1152/japplphysiol.00247.2020
Hotta, N., Katanosaka, K., Mizumura, K., Iwamoto, G. A., Ishizawa, R., Kim, H. K., et al. (2019). Insulin potentiates the response to mechanical stimuli in small dorsal root ganglion neurons and thin fibre muscle afferents in vitro. J. Physiol. 597, 5049–5062. doi: 10.1113/JP278527
Huebschmann, A. G., Reis, E. N., Emsermann, C., Dickinson, L. M., Reusch, J. E., Bauer, T. A., et al. (2009). Women with type 2 diabetes perceive harder effort during exercise than nondiabetic women. Appl. Physiol. Nutr. Metab. 34, 851–857. doi: 10.1139/H09-074
Huo, Y., Grotle, A.-K., Ybarbo, K. M., Lee, J., Harrison, M. L., and Stone, A. J. (2020). Effects of acute hyperglycemia on the exercise pressor reflex in healthy rats. Auton. Neurosci. 229:102739. doi: 10.1016/j.autneu.2020.102739
Ishizawa, R., Kim, H.-K., Hotta, N., Iwamoto, G.A., Mitchell, J.H., Smith, S.A., et al. (2021). TRPV1 (Transient Receptor Potential Vanilloid 1) sensitization of skeletal muscle afferents in Type 2 diabetic rats with hyperglycemia. Hypertension. 75, 1072–1081.
Jarvis, S. S., Vangundy, T. B., Galbreath, M. M., Shibata, S., Okazaki, K., Reelick, M. F., et al. (2011). Sex differences in the modulation of vasomotor sympathetic outflow during static handgrip exercise in healthy young humans. Am. J. Physiol. Regul. Integr. Comp. Physiol 301, R193–200. doi: 10.1152/ajpregu.00562.2010
Joyner, M. J. (2006). Baroreceptor function during exercise: resetting the record. Exp. Physiol. 91, 27–36. doi: 10.1113/expphysiol.2005.032102
Joyner, M. J., Nauss, L. A., Warner, M. A., and Warner, D. O. (1992). Sympathetic modulation of blood flow and O2 uptake in rhythmically contracting human forearm muscles. Am. J. Physiol. 263, H1078–1083. doi: 10.1152/ajpheart.1992.263.4.H1078
Karavelioglu, Y., Karapinar, H., Gul, I., Kucukdurmaz, Z., Yilmaz, A., Akpek, M., et al. (2013). Blood pressure response to exercise is exaggerated in normotensive diabetic patients. Blood Press. 22, 21–26. doi: 10.3109/08037051.2012.701045
Kaufman, M. P., Longhurst, J. C., Rybicki, K. J., Wallach, J. H., and Mitchell, J. H. (1983). Effects of static muscular contraction on impulse activity of groups III and IV afferents in cats. J. Appl. Physiol. Respir. Environ. Exerc. Physiol. 55, 105–112. doi: 10.1152/jappl.1983.55.1.105
Kiely, C., O'connor, E., O'shea, D., Green, S., and Egana, M. (2014). Hemodynamic responses during graded and constant-load plantar flexion exercise in middle-aged men and women with type 2 diabetes. J. Appl. Physiol. (1985) 117, 755–764. doi: 10.1152/japplphysiol.00555.2014
Kim, H. K., Hotta, N., Ishizawa, R., Iwamoto, G. A., Vongpatanasin, W., Mitchell, J. H., et al. (2019). Exaggerated pressor and sympathetic responses to stimulation of the mesencephalic locomotor region and exercise pressor reflex in type 2 diabetic rats. Am. J. Physiol. Regul. Integr. Comp. Physiol. 317, R270–R279. doi: 10.1152/ajpregu.00061.2019
Kim, Y. S., Seifert, T., Brassard, P., Rasmussen, P., Vaag, A., Nielsen, H. B., et al. (2015). Impaired cerebral blood flow and oxygenation during exercise in type 2 diabetic patients. Physiol. Rep. 3:e12430. doi: 10.14814/phy2.12430
Kim, Y. S., Van Der Ster, B. J. P., Brassard, P., Secher, N. H., and Van Lieshout, J. J. (2020). Cerebral vs. cardiovascular responses to exercise in type 2 diabetic patients. Front. Physiol. 11:583155. doi: 10.3389/fphys.2020.583155
Kingwell, B. A., Formosa, M., Muhlmann, M., Bradley, S. J., and Mcconell, G. K. (2003). Type 2 diabetic individuals have impaired leg blood flow responses to exercise: role of endothelium-dependent vasodilation. Diabetes Care 26, 899–904. doi: 10.2337/diacare.26.3.899
Koba, S., Gao, Z., and Sinoway, L. I. (2009). Oxidative stress and the muscle reflex in heart failure. J. Physiol. 587, 5227–5237. doi: 10.1113/jphysiol.2009.177071
Koba, S., Watanabe, R., Kano, N., and Watanabe, T. (2013). Oxidative stress exaggerates skeletal muscle contraction-evoked reflex sympathoexcitation in rats with hypertension induced by angiotensin II. Am. J. Physiol. Heart Circ. Physiol 304, H142–153. doi: 10.1152/ajpheart.00423.2012
Kuck, J. L., Bonhof, G. J., Strom, A., Zaharia, O. P., Mussig, K., Szendroedi, J., et al. (2020). Impairment in Baroreflex sensitivity in recent-onset type 2 diabetes without progression over 5 years. Diabetes 69, 1011–1019. doi: 10.2337/db19-0990
Kurl, S., Laukkanen, J. A., Rauramaa, R., Lakka, T. A., Sivenius, J., and Salonen, J. T. (2001). Systolic blood pressure response to exercise stress test and risk of stroke. Stroke 32, 2036–2041. doi: 10.1161/hs0901.095395
Lalande, S., Gusso, S., Hofman, P. L., and Baldi, J. C. (2008). Reduced leg blood flow during submaximal exercise in type 2 diabetes. Med. Sci. Sports Exerc. 40, 612–617. doi: 10.1249/MSS.0b013e318161aa99
Laukkanen, J. A., Kurl, S., Rauramaa, R., Lakka, T. A., Venalainen, J. M., and Salonen, J. T. (2006). Systolic blood pressure response to exercise testing is related to the risk of acute myocardial infarction in middle-aged men. Eur. J. Cardiovasc. Prev. Rehabil. 13, 421–428. doi: 10.1097/01.hjr.0000198915.83234.59
Leal, A. K., Mitchell, J. H., and Smith, S. A. (2013). Treatment of muscle mechanoreflex dysfunction in hypertension: effects of L-arginine dialysis in the nucleus tractus solitarii. Exp. Physiol. 98, 1337–1348. doi: 10.1113/expphysiol.2012.071563
Leal, A. K., Murphy, M. N., Iwamoto, G. A., Mitchell, J. H., and Smith, S. A. (2012). A role for nitric oxide within the nucleus tractus solitarii in the development of muscle mechanoreflex dysfunction in hypertension. Exp. Physiol. 97, 1292–1304. doi: 10.1113/expphysiol.2012.065433
Mac Ananey, O., Malone, J., Warmington, S., O'shea, D., Green, S., and Egana, M. (2011). Cardiac output is not related to the slowed O2 uptake kinetics in type 2 diabetes. Med. Sci. Sports. Exerc. 43, 935–942. doi: 10.1249/MSS.0b013e3182061cdb
Malik, R. A., Schofield, I. J., Izzard, A., Austin, C., Bermann, G., and Heagerty, A. M. (2005). Effects of angiotensin type-1 receptor antagonism on small artery function in patients with type 2 diabetes mellitus. Hypertension 45, 264–269. doi: 10.1161/01.HYP.0000153305.50128.a1
Marin, P., Andersson, B., Krotkiewski, M., and Bjorntorp, P. (1994). Muscle fiber composition and capillary density in women and men with NIDDM. Diabetes Care 17, 382–386. doi: 10.2337/diacare.17.5.382
Martin, I. K., Katz, A., and Wahren, J. (1995). Splanchnic and muscle metabolism during exercise in NIDDM patients. Am. J. Physiol. 269, E583–590. doi: 10.1152/ajpendo.1995.269.3.E583
Mather, K. J., Lteif, A., Steinberg, H. O., and Baron, A. D. (2004). Interactions between endothelin and nitric oxide in the regulation of vascular tone in obesity and diabetes. Diabetes 53, 2060–2066. doi: 10.2337/diabetes.53.8.2060
Mayer-Davis, E. J., and Costacou, T. (2001). Obesity and sedentary lifestyle: modifiable risk factors for prevention of type 2 diabetes. Curr. Diab. Rep. 1, 170–176. doi: 10.1007/s11892-001-0030-x
Mayer-Davis, E. J., Dabelea, D., and Lawrence, J. M. (2017). Incidence trends of type 1 and type 2 diabetes among youths, 2002-2012. N. Engl. J. Med. 377:301. doi: 10.1056/NEJMoa1610187
McCloskey, D. I., and Mitchell, J. H. (1972). Reflex cardiovascular and respiratory responses originating in exercising muscle. J. Physiol. 224, 173–186. doi: 10.1113/jphysiol.1972.sp009887
Menon, R. K., Grace, A. A., Burgoyne, W., Fonseca, V. A., James, I. M., and Dandona, P. (1992). Muscle blood flow in diabetes mellitus. Evidence of abnormality after exercise. Diabetes Care 15, 693–695. doi: 10.2337/diacare.15.5.693
Michelini, L. C., O'leary, D. S., Raven, P. B., and Nobrega, A. C. (2015). Neural control of circulation and exercise: a translational approach disclosing interactions between central command, arterial baroreflex, and muscle metaboreflex. Am. J. Physiol. Heart Circ. Physiol. 309, H381–392. doi: 10.1152/ajpheart.00077.2015
Milia, R., Velluzzi, F., Roberto, S., Palazzolo, G., Sanna, I., Sainas, G., et al. (2015). Differences in hemodynamic response to metaboreflex activation between obese patients with metabolic syndrome and healthy subjects with obese phenotype. Am. J. Physiol. Heart Circ. Physiol 309, H779–789. doi: 10.1152/ajpheart.00250.2015
Mortensen, S. P., Gonzalez-Alonso, J., Damsgaard, R., Saltin, B., and Hellsten, Y. (2007). Inhibition of nitric oxide and prostaglandins, but not endothelial-derived hyperpolarizing factors, reduces blood flow and aerobic energy turnover in the exercising human leg. J. Physiol. 581, 853–861. doi: 10.1113/jphysiol.2006.127423
Mortensen, S. P., Gonzalez-Alonso, J., Nielsen, J. J., Saltin, B., and Hellsten, Y. (2009). Muscle interstitial ATP and norepinephrine concentrations in the human leg during exercise and ATP infusion. J. Appl. Physiol. (1985) 107, 1757–1762. doi: 10.1152/japplphysiol.00638.2009
Moura-Tonello, S. C., Porta, A., Marchi, A., De Almeida Fagundes, A., Francisco Cde, O., Rehder-Santos, P., et al. (2016). Cardiovascular variability analysis and baroreflex estimation in patients with type 2 diabetes in absence of any manifest neuropathy. PLoS ONE 11:e0148903. doi: 10.1371/journal.pone.0148903
Muller, M. D., Drew, R. C., Blaha, C. A., Mast, J. L., Cui, J., Reed, A. B., et al. (2012). Oxidative stress contributes to the augmented exercise pressor reflex in peripheral arterial disease patients. J. Physiol. 590, 6237–6246. doi: 10.1113/jphysiol.2012.241281
Nesti, L., Pugliese, N. R., Sciuto, P., and Natali, A. (2020). Type 2 diabetes and reduced exercise tolerance: a review of the literature through an integrated physiology approach. Cardiovasc. Diabetol. 19:134. doi: 10.1186/s12933-020-01109-1
Nyberg, M., Gliemann, L., and Hellsten, Y. (2015). Vascular function in health, hypertension, and diabetes: effect of physical activity on skeletal muscle microcirculation. Scand. J. Med. Sci. Sports 25(Suppl. 4), 60–73. doi: 10.1111/sms.12591
O'Connor, E., Green, S., Kiely, C., O'shea, D., and Egana, M. (2015). Differential effects of age and type 2 diabetes on dynamic vs. peak response of pulmonary oxygen uptake during exercise. J. Appl. Physiol. (1985) 118, 1031–1039. doi: 10.1152/japplphysiol.01040.2014
O'Connor, E., Kiely, C., O'shea, D., Green, S., and Egana, M. (2012). Similar level of impairment in exercise performance and oxygen uptake kinetics in middle-aged men and women with type 2 diabetes. Am. J. Physiol. Regul. Integr. Comp. Physiol. 303, R70–76. doi: 10.1152/ajpregu.00012.2012
Ogoh, S., Fisher, J. P., Fadel, P. J., and Raven, P. B. (2007). Increases in central blood volume modulate carotid baroreflex resetting during dynamic exercise in humans. J. Physiol. 581, 405–418. doi: 10.1113/jphysiol.2006.125112
Padilla, D. J., Mcdonough, P., Behnke, B. J., Kano, Y., Hageman, K. S., Musch, T. I., et al. (2006). Effects of Type II diabetes on capillary hemodynamics in skeletal muscle. Am. J. Physiol. Heart Circ. Physiol. 291, H2439–2444. doi: 10.1152/ajpheart.00290.2006
Papelier, Y., Escourrou, P., Gauthier, J. P., and Rowell, L. B. (1994). Carotid baroreflex control of blood pressure and heart rate in men during dynamic exercise. J. Appl. Physiol. (1985) 77, 502–506. doi: 10.1152/jappl.1994.77.2.502
Petrofsky, J. S., Stewart, B., Patterson, C., Cole, M., Al Malty, A., and Lee, S. (2005). Cardiovascular responses and endurance during isometric exercise in patients with Type 2 diabetes compared to control subjects. Med. Sci. Monit. 11, CR470–477. Available online at: https://www.medscimonit.com/abstract/index/idArt/430308
Pinto, T. E., Gusso, S., Hofman, P. L., Derraik, J. G., Hornung, T. S., Cutfield, W. S., et al. (2014). Systolic and diastolic abnormalities reduce the cardiac response to exercise in adolescents with type 2 diabetes. Diabetes Care 37, 1439–1446. doi: 10.2337/dc13-2031
Poitras, V. J., Bentley, R. F., Hopkins-Rosseel, D. H., Lahaye, S. A., and Tschakovsky, M. E. (2015). Lack of independent effect of type 2 diabetes beyond characteristic comorbidities and medications on small muscle mass exercising muscle blood flow and exercise tolerance. Physiol. Rep. 3:e12487. doi: 10.14814/phy2.12487
Poitras, V. J., Hudson, R. W., and Tschakovsky, M. E. (2018). Exercise intolerance in Type 2 diabetes: is there a cardiovascular contribution? J. Appl. Physiol. (1985) 124, 1117–1139. doi: 10.1152/japplphysiol.00070.2017
Potts, J. T. (2006). Inhibitory neurotransmission in the nucleus tractus solitarii: implications for baroreflex resetting during exercise. Exp. Physiol. 91, 59–72. doi: 10.1113/expphysiol.2005.032227
Potts, J. T., Lee, S. M., and Anguelov, P. I. (2002). Tracing of projection neurons from the cervical dorsal horn to the medulla with the anterograde tracer biotinylated dextran amine. Auton. Neurosci. 98, 64–69. doi: 10.1016/S1566-0702(02)00034-6
Prevention, C. F. D. C.A. (2020). National Diabetes Statistics Report. Atlanta, GA: U.S. Dept of Health and Human Services.
Raghavan, S., Vassy, J. L., Ho, Y. L., Song, R. J., Gagnon, D. R., Cho, K., et al. (2019). Diabetes mellitus-related all-cause and cardiovascular mortality in a National cohort of adults. J. Am. Heart. Assoc. 8:e011295. doi: 10.1161/JAHA.118.011295
Ray, C. A., Rea, R. F., Clary, M. P., and Mark, A. L. (1993). Muscle sympathetic nerve responses to dynamic one-legged exercise: effect of body posture. Am. J. Physiol. 264, H1–7. doi: 10.1152/ajpheart.1993.264.1.H1
Regensteiner, J. G., Bauer, T. A., Reusch, J. E., Quaife, R. A., Chen, M. Y., Smith, S. C., et al. (2009). Cardiac dysfunction during exercise in uncomplicated type 2 diabetes. Med. Sci. Sports Exerc. 41, 977–984. doi: 10.1249/MSS.0b013e3181942051
Remensnyder, J. P., Mitchell, J. H., and Sarnoff, S. J. (1962). Functional sympatholysis during muscular activity. Observations on influence of carotid sinus on oxygen uptake. Circ. Res. 11, 370–380. doi: 10.1161/01.RES.11.3.370
Reusch, J. E., Bridenstine, M., and Regensteiner, J. G. (2013). Type 2 diabetes mellitus and exercise impairment. Rev. Endocr. Metab. Disord 14, 77–86. doi: 10.1007/s11154-012-9234-4
Roberto, S., Milia, R., Doneddu, A., Pinna, V., Palazzolo, G., Serra, S., et al. (2019). Hemodynamic abnormalities during muscle metaboreflex activation in patients with type 2 diabetes mellitus. J. Appl. Physiol. (1985) 126, 444–453. doi: 10.1152/japplphysiol.00794.2018
Rosenmeier, J. B., Hansen, J., and Gonzalez-Alonso, J. (2004). Circulating ATP-induced vasodilatation overrides sympathetic vasoconstrictor activity in human skeletal muscle. J. Physiol. 558, 351–365. doi: 10.1113/jphysiol.2004.063107
Rotto, D. M., and Kaufman, M. P. (1988). Effect of metabolic products of muscular contraction on discharge of group III and IV afferents. J. Appl. Physiol. (1985) 64, 2306–2313. doi: 10.1152/jappl.1988.64.6.2306
Rotto, D. M., Schultz, H. D., Longhurst, J. C., and Kaufman, M. P. (1990). Sensitization of group III muscle afferents to static contraction by arachidonic acid. J. Appl. Physiol. (1985) 68, 861–867. doi: 10.1152/jappl.1990.68.3.861
Rowell, L. B. (1997). Neural control of muscle blood flow: importance during dynamic exercise. Clin. Exp. Pharmacol. Physiol. 24, 117–125. doi: 10.1111/j.1440-1681.1997.tb01793.x
Sacre, J. W., Jellis, C. L., Haluska, B. A., Jenkins, C., Coombes, J. S., Marwick, T. H., et al. (2015). Association of exercise intolerance in type 2 diabetes with skeletal muscle blood flow reserve. JACC Cardiovasc. Imaging 8, 913–921. doi: 10.1016/j.jcmg.2014.12.033
Saltin, B., and Mortensen, S. P. (2012). Inefficient functional sympatholysis is an overlooked cause of malperfusion in contracting skeletal muscle. J. Physiol. 590, 6269–6275. doi: 10.1113/jphysiol.2012.241026
Saltin, B., Radegran, G., Koskolou, M. D., and Roach, R. C. (1998). Skeletal muscle blood flow in humans and its regulation during exercise. Acta Physiol. Scand. 162, 421–436. doi: 10.1046/j.1365-201X.1998.0293e.x
Scherrer, U., Pryor, S. L., Bertocci, L. A., and Victor, R. G. (1990). Arterial baroreflex buffering of sympathetic activation during exercise-induced elevations in arterial pressure. J. Clin. Invest. 86, 1855–1861. doi: 10.1172/JCI114916
Scheuermann-Freestone, M., Madsen, P. L., Manners, D., Blamire, A. M., Buckingham, R. E., Styles, P., et al. (2003). Abnormal cardiac and skeletal muscle energy metabolism in patients with type 2 diabetes. Circulation 107, 3040–3046. doi: 10.1161/01.CIR.0000072789.89096.10
Schmitt, P. M., and Kaufman, M. P. (2003). Estrogen attenuates the exercise pressor reflex in female cats. J. Appl. Physiol. (1985) 95, 1418–1424. doi: 10.1152/japplphysiol.00368.2003
Schreuder, T. H., Van Lotringen, J. H., Hopman, M. T., and Thijssen, D. H. (2014). Impact of endothelin blockade on acute exercise-induced changes in blood flow and endothelial function in type 2 diabetes mellitus. Exp. Physiol. 99, 1253–1264. doi: 10.1113/expphysiol.2013.077297
Scott, J. A., Coombes, J. S., Prins, J. B., Leano, R. L., Marwick, T. H., and Sharman, J. E. (2008). Patients with type 2 diabetes have exaggerated brachial and central exercise blood pressure: relation to left ventricular relative wall thickness. Am. J. Hypertens. 21, 715–721. doi: 10.1038/ajh.2008.166
Senefeld, J. W., Limberg, J. K., Lukaszewicz, K. M., and Hunter, S. K. (2019). Exercise-induced hyperemia is associated with knee extensor fatigability in adults with type 2 diabetes. J. Appl. Physiol. (1985) 126, 658–667. doi: 10.1152/japplphysiol.00854.2018
Song, K., Kurobe, Y., Kanehara, H., Okunishi, H., Wada, T., Inada, Y., et al. (1994). Quantitative localization of angiotensin II receptor subtypes in spontaneously hypertensive rats. Blood Press. (Suppl. 5), 5, 21–26.
Song, S. H. (2016). Early-onset type 2 diabetes: high lifetime risk for cardiovascular disease. Lancet Diabetes Endocrinol. 4, 87–88. doi: 10.1016/S2213-8587(15)00390-3
Stidsen, J. V., Henriksen, J. E., Olsen, M. H., Thomsen, R. W., Nielsen, J. S., Rungby, J., et al. (2018). Pathophysiology-based phenotyping in type 2 diabetes: a clinical classification tool. Diabetes Metab. Res. Rev. 34:e3005. doi: 10.1002/dmrr.3005
Strange, S., Secher, N. H., Pawelczyk, J. A., Karpakka, J., Christensen, N. J., Mitchell, J. H., et al. (1993). Neural control of cardiovascular responses and of ventilation during dynamic exercise in man. J. Physiol. 470, 693–704. doi: 10.1113/jphysiol.1993.sp019883
Thaning, P., Bune, L. T., Hellsten, Y., Pilegaard, H., Saltin, B., and Rosenmeier, J. B. (2010). Attenuated purinergic receptor function in patients with type 2 diabetes. Diabetes 59, 182–189. doi: 10.2337/db09-1068
Thaning, P., Bune, L. T., Zaar, M., Saltin, B., and Rosenmeier, J. B. (2011). Functional sympatholysis during exercise in patients with type 2 diabetes with intact response to acetylcholine. Diabetes Care 34, 1186–1191. doi: 10.2337/dc10-2129
Thomas, G. D., and Victor, R. G. (1998). Nitric oxide mediates contraction-induced attenuation of sympathetic vasoconstriction in rat skeletal muscle. J. Physiol. 506 (Pt 3), 817–826. doi: 10.1111/j.1469-7793.1998.817bv.x
Vianna, L. C., Deo, S. H., Jensen, A. K., Holwerda, S. W., Zimmerman, M. C., and Fadel, P. J. (2015). Impaired dynamic cerebral autoregulation at rest and during isometric exercise in type 2 diabetes patients. Am. J. Physiol. Heart Circ. Physiol. 308, H681–687. doi: 10.1152/ajpheart.00343.2014
Vranish, J. R., Holwerda, S. W., Kaur, J., and Fadel, P. J. (2020). Augmented pressor and sympathoexcitatory responses to the onset of isometric handgrip in patients with type 2 diabetes. Am. J. Physiol. Regul. Integr. Comp. Physiol. 318, R311–R319. doi: 10.1152/ajpregu.00109.2019
Wang, H. J., Pan, Y. X., Wang, W. Z., Zucker, I. H., and Wang, W. (2009). NADPH oxidase-derived reactive oxygen species in skeletal muscle modulates the exercise pressor reflex. J. Appl. Physiol. (1985) 107, 450–459. doi: 10.1152/japplphysiol.00262.2009
Williamson, J. W., Mccoll, R., Mathews, D., Mitchell, J. H., Raven, P. B., and Morgan, W. P. (2001). Hypnotic manipulation of effort sense during dynamic exercise: cardiovascular responses and brain activation. J. Appl. Physiol. (1985) 90, 1392–1399. doi: 10.1152/jappl.2001.90.4.1392
Womack, L., Peters, D., Barrett, E. J., Kaul, S., Price, W., and Lindner, J. R. (2009). Abnormal skeletal muscle capillary recruitment during exercise in patients with type 2 diabetes mellitus and microvascular complications. J. Am. Coll. Cardiol. 53, 2175–2183. doi: 10.1016/j.jacc.2009.02.042
Wray, D. W., Nishiyama, S. K., Donato, A. J., Sander, M., Wagner, P. D., and Richardson, R. S. (2007). Endothelin-1-mediated vasoconstriction at rest and during dynamic exercise in healthy humans. Am. J. Physiol. Heart Circ. Physiol 293, H2550–2556. doi: 10.1152/ajpheart.00867.2007
Yardley, J. E., Macmillan, F., Hay, J., Wittmeier, K., Wicklow, B., Macintosh, A. C., et al. (2015). The blood pressure response to exercise in youth with impaired glucose tolerance and type 2 diabetes. Pediatr. Exerc. Sci. 27, 120–127. doi: 10.1123/pes.2014-0062
Keywords: sympathetic nerve activity, blood pressure, blood flow, exercise pressor reflex, central command, baroreflex, functional sympatholysis
Citation: Grotle A-K, Kaur J, Stone AJ and Fadel PJ (2021) Neurovascular Dysregulation During Exercise in Type 2 Diabetes. Front. Physiol. 12:628840. doi: 10.3389/fphys.2021.628840
Received: 13 November 2020; Accepted: 05 March 2021;
Published: 13 April 2021.
Edited by:
Johannes Van Lieshout, University of Amsterdam, NetherlandsReviewed by:
Celena Scheede-Bergdahl, McGill University, CanadaMasaki Mizuno, University of Texas Southwestern Medical Center, United States
Copyright © 2021 Grotle, Kaur, Stone and Fadel. This is an open-access article distributed under the terms of the Creative Commons Attribution License (CC BY). The use, distribution or reproduction in other forums is permitted, provided the original author(s) and the copyright owner(s) are credited and that the original publication in this journal is cited, in accordance with accepted academic practice. No use, distribution or reproduction is permitted which does not comply with these terms.
*Correspondence: Paul J. Fadel, cGF1bC5mYWRlbEB1dGEuZWR1