- 1Institute for Cardiogenetics, University of Lübeck, Lübeck, Germany
- 2DZHK (German Research Centre for Cardiovascular Research), Partner Site Hamburg/Lübeck/Kiel, Lübeck, Germany
- 3University of Lübeck, Lübeck, Germany
- 4Institute of Neurogenetics, University of Lübeck, Lübeck, Germany
Soluble guanylyl cyclase (sGC) protein is a heterodimer formed by two subunits encoded by GUCY1A1 and GUCY1B1 genes. The chromosomal locus 4q32.1 harbors both of these genes, which has been previously significantly associated with coronary artery disease, myocardial infarction, and high blood pressure. Blood pressure is influenced by both the environment and genetics and is complemented by several biological pathways. The underlying mechanisms associated with this locus and its genes still need to be investigated. In the current study, we aimed to establish the zebrafish as a model organism to investigate the mechanisms surrounding sGC activity and blood pressure. A zebrafish mutant gucy1a1 line was generated using the CRISPR-Cas9 system by inducing a 4-bp deletion frameshift mutation. This mutation resulted in a reduction of gucy1a1 expression in both heterozygote and homozygote zebrafish. Blood flow parameters (blood flow, arterial pulse, linear velocity, and vessel diameter) investigated in the gucy1a1 mutants showed a significant increase in blood flow and linear velocity, which was augmented in the homozygotes. No significant differences were observed for the blood flow parameters measured from larvae with individual morpholino downregulation of gucy1a1 and gucy1b1, but an increase in blood flow and linear velocity was observed after co-morpholino downregulation of both genes. In addition, the pharmacological sGC stimulator BAY41-2272 rescued the impaired cGMP production in the zebrafish gucy1a1± mutant larvae. Downregulation of cct7 gene did not show any significant difference on the blood flow parameters in both wild-type and gucy1a1± background larvae. In summary, we successfully established a zebrafish platform for investigating sGC-associated pathways and underlying mechanisms in depth. This model system will have further applications, including for potential drug screening experiments.
Background
High blood pressure (BP), or hypertension, is one of the most prominent heritable and preventable risk factors leading to premature death and the major cause for chronic heart failure, stroke, coronary artery disease (CAD), and kidney disease (Mills et al., 2016). According to the updated hypertension guideline, the estimated global financial burden of hypertension will reach approximately $200 billion by 2030 (DePalma et al., 2018). Currently, more than 1.3 billion people are estimated to have hypertension, which is expected to extend to 1.56 billion worldwide by 2025 (Kearney et al., 2005; Mills et al., 2016). Both the environment and genetics are contributing factors for BP (Evangelou et al., 2018). A recent genome-wide association study (GWAS) identified 535 novel loci associated with BP traits (systolic and diastolic blood pressure, and pulse pressure) in people of European ancestry drawn from the UK Biobank (UKB) and the International Consortium of BP-GWAS (ICBP) (Evangelou et al., 2018). The GUCY1A1-GUCY1B1 locus (4q32.1) was associated with systolic and diastolic blood pressure by the ICBP (Ehret et al., 2011), and it was one of the five loci reported by the CARDIoGRAMplusC4D consortium showing significant association with BP along with CAD (Deloukas et al., 2013; Wobst et al., 2015).
Mechanisms catalyzing vascular retention of atherogenic lipoproteins and stimulated inflammation due to high blood pressure are well understood, along with impairment of nitric oxide (NO)-mediated vasodilation (Rippe et al., 2017). The latter plays a crucial role in regulating BP, and interference can lead to several clinical manifestations, such as stroke and chronically elevated BP, and in severe cases, myocardial infarction (Rippe et al., 2017). In hypertension, a reduction of NO-dependent vasodilation is partially attributed to decreased soluble guanylyl cyclase (sGC) protein levels that are recognized as a key NO receptor in the vascular wall of blood vessels (Rippe et al., 2017).
The sGC protein is a heterodimer comprised of α1 and β1 subunits, which are encoded by the genes GUCY1A1 and GUCY1B1 (previously known as GUCY1A3 and GUCY1B3, respectively) (Erdmann et al., 2013). The sGC complex acts as key receptor for NO and promotes the generation of cyclic guanosine 3′, 5′-monophosphate (cGMP) (Erdmann et al., 2013; Wobst et al., 2015). cGMP regulates several physiological processes, such as proliferation and migration of vascular smooth muscle cells, neurotransmission, and inhibition of platelet aggregation (Erdmann et al., 2013; Wobst et al., 2015).
We previously conducted an extended family study to investigate the prevalence of premature CAD or myocardial infarction (MI) and identified a disease-causing variant in the GUCY1A1 gene, which encodes for a subunit of sGC (Erdmann et al., 2013). The GUCY1A1 frame shift mutation leads to a premature stop codon. Approximately 50% of carriers suffered with CAD and MI. In the same family, another rare mutation was identified in the CCT7 gene encoding for a chaperonin stabilizing sGC (Erdmann et al., 2013). Individuals harboring both mutations were severely affected by CAD, suggesting a causal role of di-genic mutations. Further investigations revealed impaired sGC activity and significantly lower cGMP levels from platelets isolated from di-genic mutation carriers (Erdmann et al., 2013). In support of our findings, the α1- and β1-subunit protein levels were reduced in vitro after silencing CCT7, and in vivo, α1-subunit mutant mice showed an acceleration of occluding thrombus formation in the microcirculation after trauma (Erdmann et al., 2013).
In the past decade, synthetic sGC stimulators have entered the pharmaceutical market and proven beneficial in clinical trials for pulmonary arterial hypertension (Gheorghiade et al., 2015), chronic thromboembolic pulmonary hypertension (Ghofrani et al., 2013a), and heart failure, due to a reduced ejection fraction during heart contraction (Ghofrani et al., 2013b; Wobst et al., 2016). Previously, we identified a dose-dependent effect of the sGC stimulator BAY 41-2272 in the presence of NO on the inhibition of platelet aggregation. In cell culture setting (human embryonic kidney cells), different single nucleotide polymorphisms of the α1-subunit with significantly reduced protein levels were investigated by addition of BAY 41-2272 (Wobst et al., 2016). Treatment with BAY 41-2272 increased the production of cGMP without having an impact on protein levels (Wobst et al., 2016). Although there were several limitations to this study, these results provided a basis for impairment of sGC function in the mutated carriers of the GUCY1A1 gene (Wobst et al., 2016).
A plethora of research has been conducted to investigate the different underlying mechanisms or characteristics of the sGC subunits, specifically the α1- and β1-subunits encoding for GUCY1A1 and GUCY1B1, respectively, which have been previously associated by GWAS with hypertension, CAD, MI, and other diseases. However, there is still a demand for an animal model to perform high-throughput drug screening of potential drug candidates, which are currently only investigated in vitro.
The zebrafish (Danio rerio) is a well-established model organism used for disease modeling and drug screening because of several important characteristics: a high offspring output, genetic homology to the human genome, cheap and easy maintenance, optical transparency, and ease in genetic manipulation. Therefore, we determined that the zebrafish is a well-fitting candidate to study sGC and its associated phenotypes. So far, GUCY-1A1 and GUCY-1B1 have been mostly studied using live cell lines, mice for disease phenotypic studies, or in clinical trials on humans. In the current study, we aim to establish the zebrafish as a model for future sGC subunits studies by investigating different phenotypes associated with the two subunits.
Materials and Methods
Zebrafish Maintenance
All zebrafish experiments were ethically approved and conducted in accordance with the guidelines of the Animal Studies Committee in Schleswig-Holstein, Germany. AB-wild-type zebrafish were purchased from European Zebrafish Resource Center (EZRC, Germany) and maintained at the Fraunhofer Institute of Marine Biotechnology (EMB, Lübeck, Germany) as described in The Zebrafish book (Westerfield et al., 1997; Westerfield, 2000).
Generation of Zebrafish gucy1a1 Knockout Line
To generate gucy1a1 knockout line, one-cell zebrafish embryos were co-injected with 200 ng of in vitro synthesized guide RNA (gRNA) targeting the GGGCGAATGCCCCTTTTCTA sequence in the first exon of the gucy1a1 gene (Figure 1A) and 400 ng of in vitro synthesized Cas9 mRNA. After 2 months, fish were fin-clipped and genomic DNA was extracted. The targeted genomic locus was amplified using the following primers: Forward GTTTGGAGAGGACCATTACGC and Reverse TTTACCTCTGGACACACCAGC, designed to anneal upstream and downstream from the targeted region and sequenced using Sanger sequencing (Figure 1Aa’). Only fish carrying indels (classified as potential founders) were kept to reach sexual maturity. Each potential founder was crossed with the AB-wild-type zebrafish. Four days post-fertilization, 20 embryos from each potential founder were lysed individually with 25 μl of the proteinase K buffer (1 μg/μl proteinase K in 1xPCR buffer) and incubated for 50 min at 55°C, followed by inactivation at 95°C for 10 min. Extracted DNA was screened for the presence of indel mutations by PCR using the above-mentioned primers, followed by Sanger sequencing.
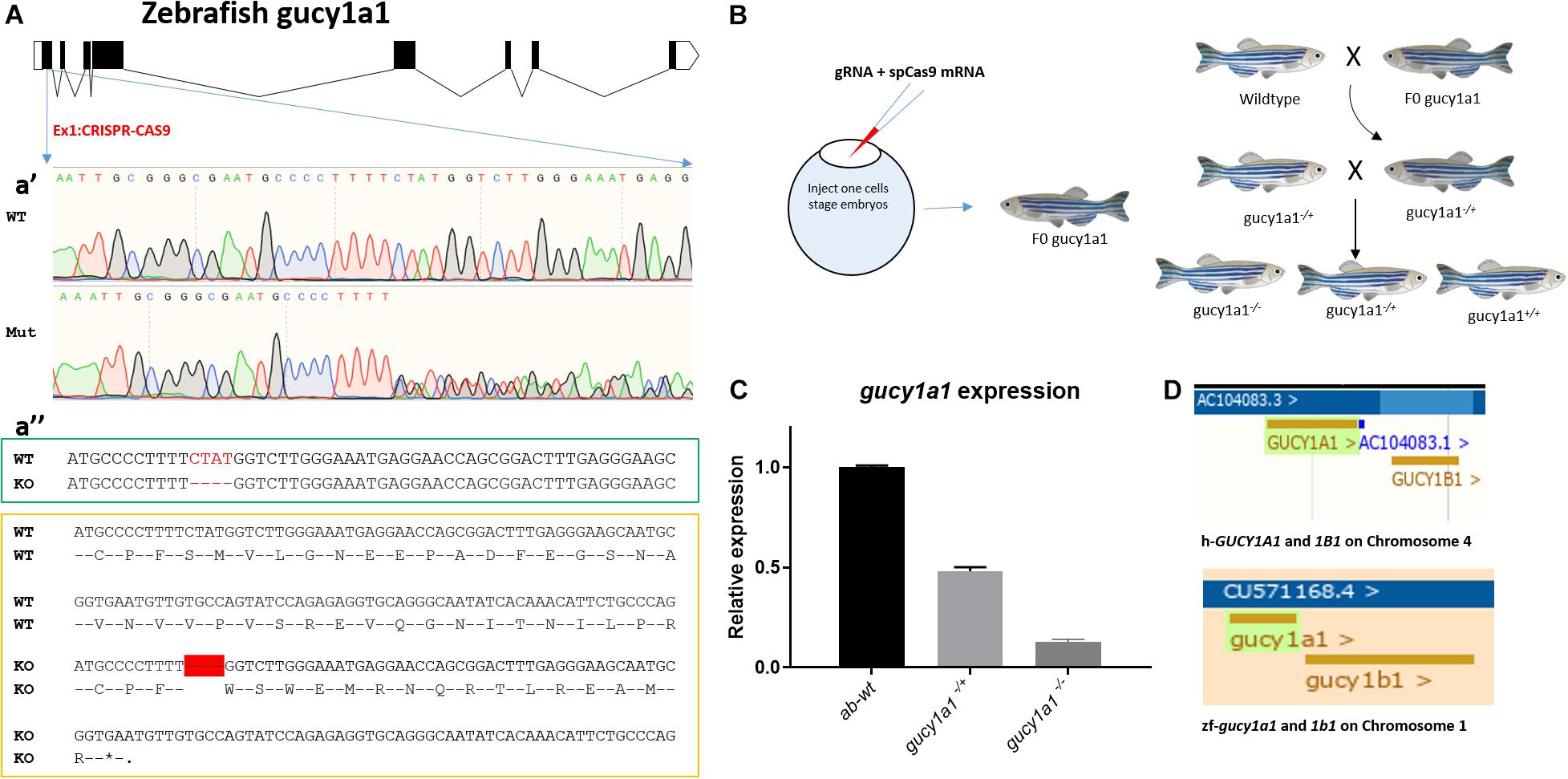
Figure 1. (A) Schematic of zebrafish gucy1a1 gene presenting all the exons in box forms and introns in the form of connecting lines, a’ showing sequencing result comparison of zebrafish wild-type allele (WT) vs. mutant (Mut) at gRNA target site on gucy1a1 gene exon 1, a” (in the green box) highlights 4 base pair deletion (highlighted as red dashes) at the mRNA level in the knockout zebrafish compared to the wild types, and a” (in the yellow box) shows the deletion of 4 base pair leading to premature stop codon at the amino acid level after 16 amino acids. (B) Schematic of gRNA + spCas9 mRNA in one-cell stage zebrafish embryos leading to identification of F0 founder fish, which were out crossed with AB wild-types following which heterozygotes were identified and in crossed. (C) mRNA relative expression of gucy1a1 gene was observed significantly reduced in the gucy1a1+/– (±50%) and gucy1a1– /– (±90%), error bars represent ±SD. (D) Schematic represents the conserved genomic positioning of both gucy1a1 and gucy1b1 genes in human and zebrafish genome, screen shots taken from Ensemble genome browser.
Finally, the offspring of the confirmed founder were maintained for 2 months and fin-clipped to identify animals carrying identical frameshift mutations in the gucy1a1 gene. As presented in the schematic in Figure 1B, gucy1a1± were crossed to obtain gucy1a1+/+, gucy1a1±, and gucy1a1–/– for all the further experiments.
Morpholino Downregulation
One-cell zebrafish embryos of both AB-wild-type and gucy1a1 mutants were injected with approximately 5 ng of transcription splice modification morpholinos of gucy1a1-e2i2 (AAAAGAACATCTTACCAACTCGTGT), gucy1b1-e2i2 (TATTTTTGAACTCACTTAATGTCCT), and cct7-e2i2 (ATACAGAATTGCTGACTAACCTCGG) along with a gene-specific control morpholino as previously described (Westerfield, 2000). Morpholinos were injected individually or in conjunction with other morpholinos as per the requirements of the experiment.
Following blood flow measurements from individual larvae, the morpholino downregulation efficiency at the mRNA level was assessed using qPCR analysis. Total RNA was extracted using RNeasy kit (Qiagen, Germany) following the manufacturer’s protocol. EF1α and β-actin were used as internal housekeeping genes, and the custom-designed primer sequences are described in Table 1. qPCR data were analyzed using the comparative threshold cycle method (2–Δ Δ Ct) to compare expression levels in samples and controls, where control samples were set to a value of 1.
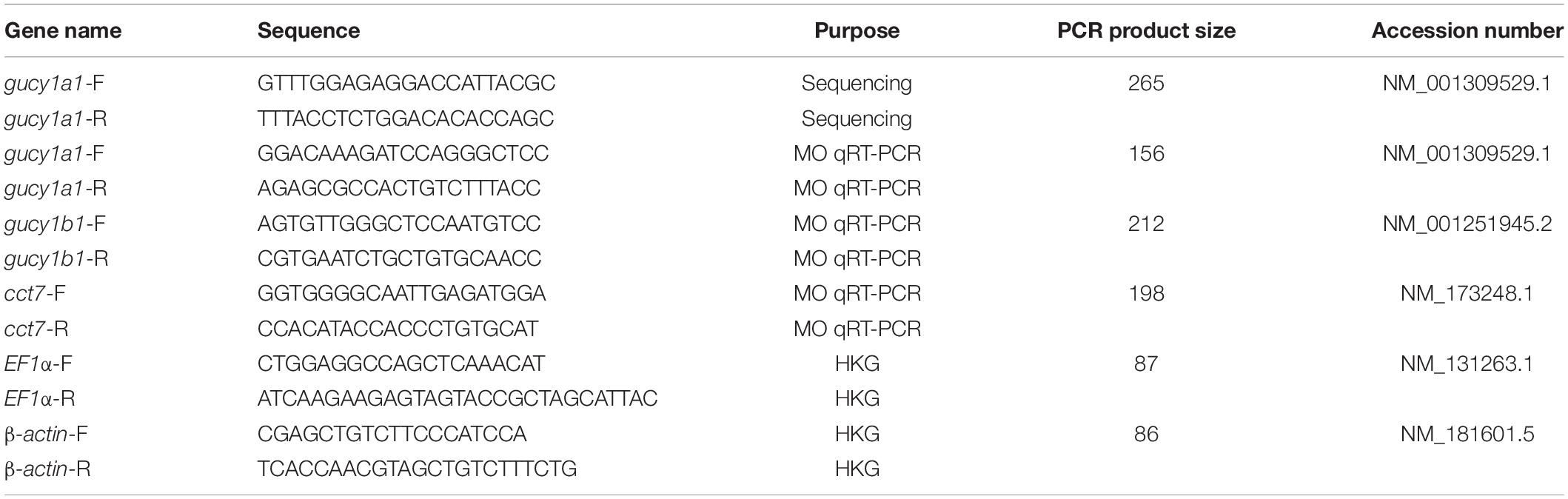
Table 1. Sequences for all the primers (both forward and reverse) along with their respective purpose in the present study.
Hypertension Parameters
Blood flow parameters, i.e., arterial pulse, blood flow, linear velocity, and vessel diameter (surrogate parameters for blood pressure), were measured following the same protocol as in previous publication (Parker et al., 2014; Vishnolia et al., 2020). Briefly, 72 h post fertilization (hpf) zebrafish larvae were mounted on glass coverslips using 0.1% low melting agarose gel maintained at 17°C. Twenty-minute video files were recorded at 120 frames per second (fps) at room temperature using an inverted microscope (Zeiss stereo, Discovery V20, Germany), mounted with high-speed video cameras (GRAS-03K2M-C, Point Grey Research Inc., Richmond, Canada) focused on dorsal aorta, caudal to swim bladder.
Video files from the positive genotyped (n = 12) and control zebrafish (n = 10) larvae were analyzed using MicroZebraLab software (Version 3.6, ViewPoint, Lyon, France). After software calibration using a video of a hemocytometer grid, the software detects pixel density along with vessel diameter to compute the flow rate (nl/sec) per frame. An area of interest on the aortic blood vessel was selected carefully, avoiding nearby capillaries as they might interfere in determining the blood flow in the main vessel. Vessel diameter was determined every 20 s by the software following manual selection of vessel and confirmation of two vessel edges. Blood flow was computed by the software by determining the movement of erythrocytes within the region of interest. To let the zebrafish larvae acclimatize to the conditions, analysis from the first 3 min of every video file was excluded from the results as demonstrated in previous literature (Parker et al., 2014) and reported in our publication previously (Vishnolia et al., 2020).
Bay41-2272 Stimulation and cGMP Measurements
Cyclic guanosine 3′, 5′-monophosphate levels were measured from the homogenized lysate of zebrafish larvae using the cGMP ELISA kit (Cyclic GMP ELISA kit-581021, Cayman chemicals) following the manufacturer’s protocol. Zebrafish gucy1a1± and wild-type larvae (n = 30 larvae per group) at 4 days post-fertilization were synergistically treated for 1 h with H2O, 1, 5, and 10 μM of BAY 41-2272 (B8810, Sigma-Aldrich, Germany) sGC stimulator. All larvae were snap frozen in liquid nitrogen (in groups of 10) before being homogenized in the ELISA buffer using a hand-held motorized homogenizer. Tissue sample lysates along the standards were carefully acetylated following the manufacturer’s protocol to enhance sensitivity and avoid interference from other contaminants. Following the instructions of the kit, the ELISA plate was prepared, developed, read at a 405 nm absorbance wavelength (BioTek instruments, United States), and cGMP values were calculated.
Statistical Analysis
Statistical analysis was performed using the GraphPad Prism 6 program. Data were tested for normal distribution following which non-parametric Student’s t-test was used to analyze the data (represented as mean ± SD) from experiments with only two groups. A two-way ANOVA with multiple comparisons and without corrections was used when experiments contained three or more groups for analysis. The significance alpha p-value was adjusted by dividing it with the total number of tests performed.
Results
Conservation of Human GUCY Subunits in Zebrafish
Both the GUCY-1A1 and GUCY-1B1 subunits of sGC are located at locus 4q32.1 on chromosome 1. Data mining on the ensemble genome browser database revealed identical genomic positioning of both gucy1a1 and gucy1b1 genes between human and zebrafish genomes, respectively, as shown in Figure 1D. Both the genes were also identified to be highly conserved in human and zebrafish such as gucy1a1 gene having 95% coverage and 58% identical protein (Browser, 2020a), whereas gucy1b1 having 98% coverage and 88% identical protein (Browse, 2020b) (as of Ensemble release 102-November 2020).
Generation of Zebrafish gucy1a1 Mutant Line
We generated a gucy1a1 mutant using AB-wild-type background zebrafish by inducing a 4-base-pair frame-shift deletion mutation in the exon 1 of the gucy1a1 gene using the clustered regularly interspaced short palindromic repeat (CRISPR)-Cas9 technology (Figures 1A,B). Sequencing chromatograph shown in Figure 1Aa’ represents a mixture of different sequences after “TTTT,” i.e., wild-type, mutant sequences.
The frame-shift deletion of 4 base pairs led to the formation of a stop codon after 16 amino acids as shown in Figure 1A. mRNA expression analysis showed a 50% ± 10% and 90% ± 10% reduction in gucy1a1 heterozygous and homozygous mutation carriers, respectively, when compared with the wild-type siblings (Figure 1C). We observed no gross morphological differences between wild-type and gucy1a1 mutant fish at either larval or adult stage. We did not had success in generating the gucy1b1 and cct7 zebrafish mutant line using same approach, and therefore, we employed the morpholino downregulation approach for gucy1b1 and cct7 gene in the current study.
Increased Blood Flow Measurements in gucy1a1 Mutants
We observed a significant increase in blood flow, arterial pulse, linear velocity, and vessel diameter, measured after 72 hpf, in both gucy1a1± (n = 12) and gucy1a1–/– (n = 12) mutants compared with AB-wild-type controls (n = 10) (Figure 2). Both blood flow and linear velocity parameters were significantly higher in the homozygotes than in the heterozygotes. However, no significant differences were found in the arterial pulse or vessel diameter parameters between heterozygotes and homozygotes, as shown in Figure 2.

Figure 2. Blood flow parameters measured from AB-wild types, heterozygote and homozygote for gucy1a1 gene in zebrafish larvae. Blood flow (nL/sec), arterial pulse (beats per minute), linear velocity (μm/sec), and vessel diameter (μm) were measured from gucy1a1+/– (n = 12), gucy1a1– /– (n = 12), and AB-wild-type (n = 10) larvae per group. Data are presented as mean ± standard deviation, significance was set at p < 0.05, and stars denote the significant differences.
gucy1a1, gucy1b1, and cct7 Morpholino Downregulation
qPCR analysis showed approximately 60–65% knockdown of the gucy1a1-, gucy1b1-, and cct7- injected morpholinos, relative to control. No cross-reactivity of the morpholinos was observed. In particular, no downregulation was observed of the gucy1b1 subunit in the gucy1a1-injected morpholino larvae or gucy1a1 subunit in the gucy1b1-injected morpholino (Figure 3A).
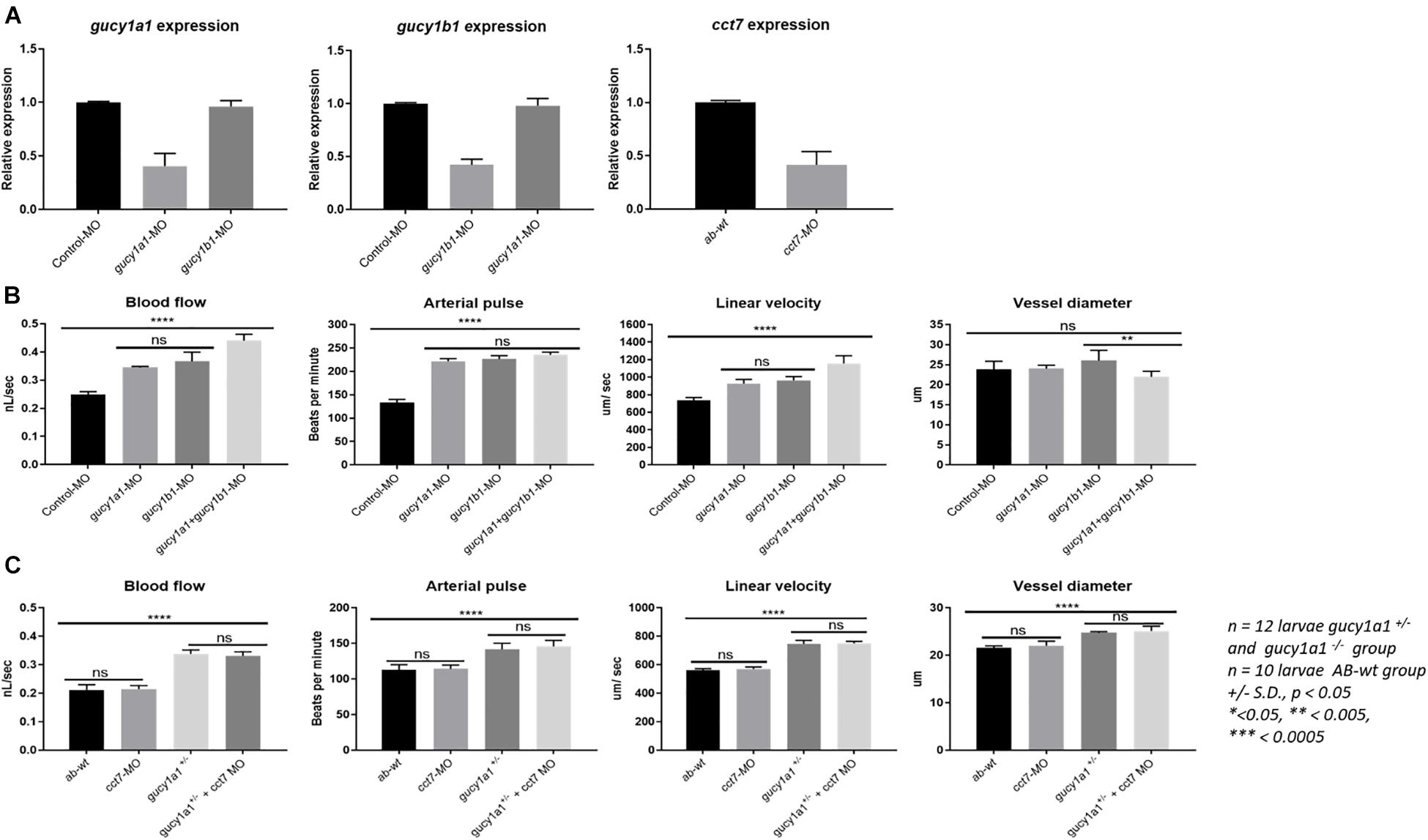
Figure 3. (A) Graphs represent mRNA downregulation of gucy1a1, gucy1b1, and cct7 genes using morpholino (MO) in zebrafish. No cross reactivity of morpholinos was observed between the two subunits of gucy gene in the mRNA expression. (B) Graphs represent comparison of blood flow parameters measured from gucy1a1 (n = 12), gucy1b1 (n = 12), gucy1a1+gucy1b1 (n = 12), and control-MO (n = 10). (C) Graphs presenting comparison of blood flow parameters measured from AB-wild-type (n = 10), cct7-MO (n = 12), gucy1a1+/– (n = 12), and gucy1a1+/– + cct7-MO (n = 12). Data are presented as mean ± standard deviation, significance was set at p < 0.05, and stars denote the significant differences.
gucy1a1 and gucy1b1-Morpholino Combined Effect
Although there was significant increase in the blood flow parameters in morpholino-injected gucy1a1 and gucy1b1 subunits compared to control morpholino-injected larvae, no significant differences were observed in the blood flow parameters among the two morpholino downregulated gucy1a1 and gucy1b1 (n = 12 larvae per group) subunits injected individually as shown in Figure 3B. Interestingly, when morpholinos for both subunits were co-injected, we observed a significant increase in the blood flow and linear velocity, but a decrease in the larval vessel diameter compared to individually morpholino downregulated subunit (Figure 3B). Decrease in vessel diameter was not significant between the control morpholino-injected and gucy1a1 and gucy1b1 morpholino co-injected larvae. Arterial pulse did not change in larvae co-injected with both morpholinos compared to individual injected morpholino for both subunits; however, consistent significant increase was observed in morpholino co-injected larvae compared to control morpholino-injected larvae.
Effect of cct7 Downregulation in gucy1a1± Mutants on Blood Flow Parameter
Injection of cct7 morpholinos into AB-wild-type zebrafish embryos presented no differences in the blood flow parameters (Figure 3C). Interestingly contrary to the familial study previously published by our group (Erdmann et al., 2013), gucy1a1± zebrafish embryos injected with the cct7 morpholino induced no change in blood flow parameters, in contrast to the gucy1a1± control (Figure 3C).
sGC Stimulator Dependent Increment in cGMP Levels
Cyclic guanosine 3’, 5’-monophosphate levels were measured from AB-wild-type zebrafish larvae treated with either H2O or 1, 5, or 10 μM concentrations of BAY 41-2272 stimulator. The cGMP levels of the larvae increased significantly in a dose-dependent manner (Figure 4A). The cGMP level was also observed to increase in a dose-dependent manner in gucy1a1± zebrafish mutants (Figure 4B). However, the cGMP levels were approximately 10-fold higher in the wild-type than in mutants.
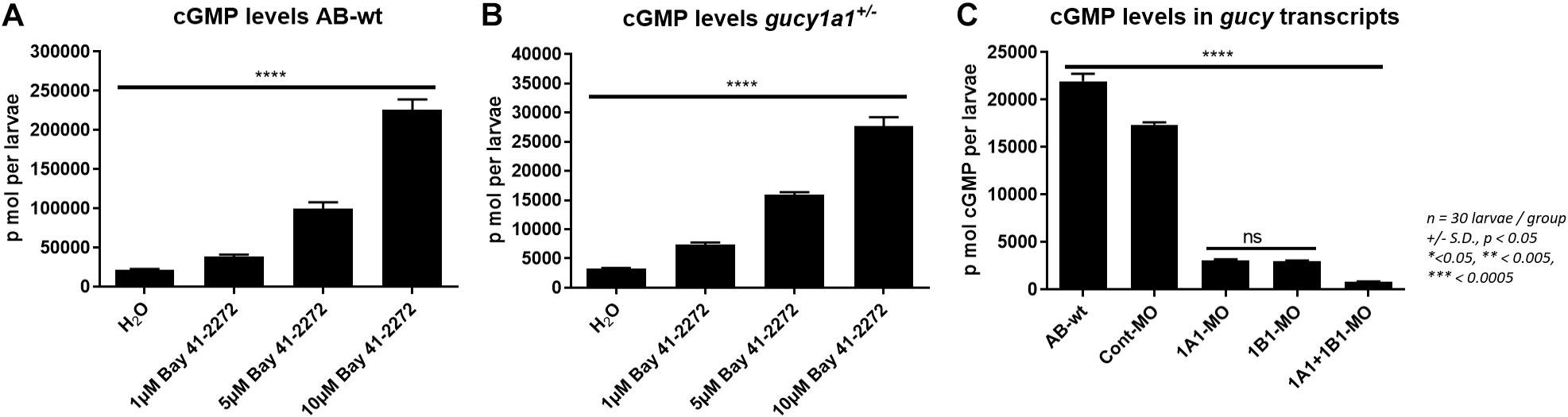
Figure 4. (A) Graph presents cGMP levels (p mol per larvae) measured from AB-wild-type larvae at 4 dpf treated with H2O, 1, 5, and 10 μM BAY41-2272 concentration. (B) cGMP levels measured and compared from gucy1a1+/– treated with H2O, 1, 5, and 10 μM BAY41-2272 concentration. (C) cGMP levels measured and compared between AB-wild types, morpholino injected controls, gucy1a1-MO, gucy1b1-MO, and gucy1a1+gucy1b1-MO. Data are presented as mean ± standard deviation from n = 30 larvae per group, significance was set at p < 0.05, and stars denote the significant differences.
Further, we found no significant difference in the cGMP levels between the two knockdowns of the gucy1a1 and gucy1b1 morpholinos (Figure 4C). We noticed a significantly lower cGMP level when morpholinos for both the subunits were co-injected in the zebrafish larvae (Figure 4C).
Discussion
In the current study, we aimed to establish zebrafish as a model system for studying the underlying mechanisms for sGC and its subunits. The zebrafish gucy1a1 mutant line was created to explicitly study the phenotypes associated with this gene, with specific interest in the blood flow parameters. Significantly increased blood flow, arterial pulse, linear velocity, and vessel dilation were observed in the mutant larvae compared with the wild type. The increased blood flow parameters in the gucy1a1 mutants are consistent with predicted associations from GWAS (Ehret et al., 2011; Evangelou et al., 2018) and previously published data (Wallace et al., 2016).
It is further warranted to investigate the effect of the gene encoding β-subunit, i.e., gucy1b1, in the sGC heterodimer. As we were unsuccessful in creating the zebrafish mutant line for gucy1b1, we employed the morpholino approach. Successful significant downregulation (50–60%), without any cross-reactivity among the two subunit morpholinos, was observed, leading to significantly increased blood flow parameters when injected individually. By comparison, larvae co-injected with both the gucy1a1 and gucy1b1 morpholinos significantly increased blood flow and linear velocity; however, no such significant difference was observed for arterial pulse. Surprisingly, we did not observe any significant difference in vessel diameter between larvae injected individually with morpholinos compared with controls. By comparison, larvae co-injected with both subunit morpholinos showed a reduced vessel diameter compared with those injected with gucy1b1 morpholino.
Impaired physiological function of the vascular wall is a very common characteristic in the diseased state, leading to either vasodilation or vasoconstriction due to anaphylactic shock or hypertension (Lerman and Burnett, 1992). Endothelial dysfunction is a crucial factor in CADs such as hypertension, MI, and atherosclerosis, which predominantly lead to impaired NO-sGC-cGMP signaling (John and Schmieder, 2003). Several factors could be accountable for the effect on the NO-sGC-cGMP pathway, such as (1) reduced NOS activity due to reduced NO availability, (2) increased PDE activity that could fast-track cGMP degradation (Wilcox, 2002; John and Schmieder, 2003; Stasch et al., 2006), (3) oxidative stress leading to reduced free radicals to scavenge NO (Wilcox, 2002), or (4) downregulation of sGC or cGMP targets (Boerrigter and Burnett Jr., 2007). Therefore, we aimed to investigate if the well-established pharmacological sGC modulator (BAY 41-2272) could rescue the impaired cGMP formation in our gucy1a1± zebrafish system. Indeed, treatment of gucy1a1± mutants with BAY 41-2272 presented a significant increase in the cGMP levels respective to the treatment concentration. This result is supported by previous in vitro studies (Boerrigter and Burnett Jr., 2007; Wobst et al., 2016) and provides evidence that the zebrafish is an ideal in vivo model system to examine the mechanisms associated with sGC and its subunits.
Mutations in the GUCY1A1 gene leads to truncated or non- functional proteins, which has been linked with higher genetic risk for CAD/MI. Individuals carrying a cct7 mutation, a gene that encodes the chaperone protein, leads to premature CAD/MI in a previously reported extended family study (Erdmann et al., 2013). In our study, injection of the gucy1a1± zebrafish larvae with the cct7 morpholino did not show any significant differences in the BP parameters, compared with the gucy1a1± larvae. Nevertheless, the cct7 morpholino-injected zebrafish larvae did not show any change in the blood flow parameters compared with controls. We believe that this needs to be further investigated to understand the effect of cct7 downregulation; however, a complete cct7 knockout mutant line should be used. To investigate and understand the mechanisms relating to atherosclerosis, the zebrafish mutants (gucy1a1, gucy1a1, gucy1a1 + cct7, gucy1a1 + gucy1b1, gucy1b1 + cct7, and gucy1a1 + gucy1b1 + cct7) could be treated with high cholesterol diets to measure the effects on cholesterol levels, lipid deposits, and downstream effects of the PDEs.
In summary, we have shown that the zebrafish model system can successfully recapitulate the sGC-associated phenotypes including blood flow parameter alterations. The gucy1a1–/+ zebrafish mutants demonstrated impaired cGMP formation and a combined effect of the α1- and β1-subunits on the blood flow parameters when downregulated by morpholino injection. Nevertheless, further investigation is warranted to produce a complete knockout mutant line for the cct7 and gucy1b1 genes in the zebrafish. Overall, this model system could serve as an ideal platform for future studies to investigate the underlying mechanisms of sGC activity and have further applications for potential drug screening experiments.
Data Availability Statement
The raw data supporting the conclusions of this article will be made available by the authors, without undue reservation.
Ethics Statement
Ethical approval of the animal study was waived in accordance with the recommendations of guidelines, EU Directive 2010/63/EU, set by the European Commission, and according to this legislation, embryos and larvae up to 5 days old are excepted. The legislation criterion is independently feeding larval forms (Directive 2010/63/EU), in other words, when the larvae are able to move through the water column independently, when their digestive tract is functional, and when they are beginning to hunt for prey which has also been simplified and published in PMID: 21726626. For generation of zebrafish gucy1a1 mutant line, approval was granted by the Animal Ethical Committee of Schleswig-Holstein, Germany, and the reference number for that is V 242-7224.122-39 (77-6/14).
Author Contributions
KV conceived, designed, performed, and collected the data, and wrote the manuscript. AR contributed to generating the gucy1a1 knockout line. CH and KT contributed to critical analysis of data and manuscript revisions. ZA and JE supervised the project, critically analyzed the data, reviewed the manuscript, and helped in designing the initial concept of the project. All authors contributed to the article and approved the submitted version.
Funding
This work was supported by the DZHK (Deutsches Zentrum für Herz-Kreislauf-Forschung e.V.) and the Excellence Cluster “Inflammation at Interfaces.”
Conflict of Interest
The authors declare that the research was conducted in the absence of any commercial or financial relationships that could be construed as a potential conflict of interest.
Acknowledgments
We would like to acknowledge all the technical assistance provided by Maren Behrensen, Petra Bruse, and Sandra Wrobel. We would also like to acknowledge the support provided by our collaborating partner Fraunhofer EMB, Lübeck, for maintenance of the zebrafish lines.
References
Boerrigter, G., and Burnett, J. C. Jr. (2007). Nitric oxide–independent stimulation of soluble guanylate cyclase with BAY 41-2272 in cardiovascular disease. Cardiovasc. Drug. Rev. 25, 30–45. doi: 10.1111/j.1527-3466.2007.00003.x
Browser, E. (2020a). Available online at: http://Nov2020.archive.ensembl.org/Danio_rerio/Gene/Compara_Ortholog/Alignment?db=core;g=ENSDARG00000013787;g1=ENSG00000164116;hom_id=333095657;r=1:25783801-25799051 (accessed February 2, 2021).
Browse, E. (2020b). Available online at: http://Nov2020.archive.ensembl.org/Danio_rerio/Gene/Compara_Ortholog/Alignment?db=core;g=ENSDARG00000086790;g1=ENSG00000061918;hom_id=333096747;r=1:25801648-25841659 (accessed February 2, 2021).
Deloukas, P., Kanoni, S., Willenborg, C., Farrall, M., Assimes, T. L., Thompson, J. R., et al. (2013). Large-scale association analysis identifies new risk loci for coronary artery disease. Nat. Genet. 45, 25–33.
DePalma, S. M., Himmelfarb, C. D., MacLaughlin, E. J., and Taler, S. J. (2018). Hypertension guideline update: a new guideline for a new era. J. Am. Acad. PAs 31, 16–22. doi: 10.1097/01.jaa.0000533656.93911.38
Ehret, G. B., Munroe, P. B., Rice, K. M., Bochud, M., Johnson, A. D., Chasman, D. I., et al. (2011). Genetic variants in novel pathways influence blood pressure and cardiovascular disease risk. Nature 478:103. doi: 10.1038/nature10405
Erdmann, J., Stark, K., Esslinger, U. B., Rumpf, P. M., Koesling, D., de Wit, C., et al. (2013). Dysfunctional nitric oxide signalling increases risk of myocardial infarction. Nature 504, 432–436.
Evangelou, E., Warren, H. R., Mosen-Ansorena, D., Mifsud, B., Pazoki, R., Gao, H., et al. (2018). Genetic analysis of over 1 million people identifies 535 new loci associated with blood pressure traits. Nat. Genet. 50, 1412–1425.
Gheorghiade, M., Greene, S. J., Butler, J., Filippatos, G., Lam, C. S., Maggioni, A. P., et al. (2015). Effect of vericiguat, a soluble guanylate cyclase stimulator, on natriuretic peptide levels in patients with worsening chronic heart failure and reduced ejection fraction: the SOCRATES-REDUCED randomized trial. JAMA 314, 2251–2262. doi: 10.1001/jama.2015.15734
Ghofrani, H.-A., D’Armini, A. M., Grimminger, F., Hoeper, M. M., Jansa, P., Kim, N. H., et al. (2013a). Riociguat for the treatment of chronic thromboembolic pulmonary hypertension. N. Engl. J. Med. 369, 319–329.
Ghofrani, H.-A., Galiè, N., Grimminger, F., Grünig, E., Humbert, M., Jing, Z.-C., et al. (2013b). Riociguat for the treatment of pulmonary arterial hypertension. N. Engl. J. Med. 369, 330–340.
John, S., and Schmieder, R. E. (2003). Potential mechanisms of impaired endothelial function in arterial hypertension and hypercholesterolemia. Curr. Hypertens. Rep. 5, 199–207. doi: 10.1007/s11906-003-0021-1
Kearney, P. M., Whelton, M., Reynolds, K., Muntner, P., Whelton, P. K., and He, J. (2005). Global burden of hypertension: analysis of worldwide data. Lancet 365, 217–223. doi: 10.1016/s0140-6736(05)17741-1
Lerman, A., and Burnett, J. J. (1992). Intact and altered endothelium in regulation of vasomotion. Circulation 86, III12–III19.
Mills, K. T., Bundy, J. D., Kelly, T. N., Reed, J. E., Kearney, P. M., Reynolds, K., et al. (2016). Global disparities of hypertension prevalence and control: a systematic analysis of population-based studies from 90 countries. Circulation 134, 441–450. doi: 10.1161/circulationaha.115.018912
Parker, T., Libourel, P.-A., Hetheridge, M. J., Cumming, R. I., Sutcliffe, T. P., Goonesinghe, A. C., et al. (2014). A multi-endpoint in vivo larval zebrafish (Danio rerio) model for the assessment of integrated cardiovascular function. J. Pharmacol. Toxicol. Methods 69, 30–38. doi: 10.1016/j.vascn.2013.10.002
Rippe, C., Zhu, B., Krawczyk, K. K., Van Bavel, E., Albinsson, S., Sjölund, J., et al. (2017). Hypertension reduces soluble guanylyl cyclase expression in the mouse aorta via the Notch signaling pathway. Sci. Rep. 7, 1–13.
Stasch, J.-P., Schmidt, P. M., Nedvetsky, P. I., Nedvetskaya, T. Y., Hs, A. K., Meurer, S., et al. (2006). Targeting the heme-oxidized nitric oxide receptor for selective vasodilatation of diseased blood vessels. J. Clin. Invest. 116, 2552–2561. doi: 10.1172/jci28371
Vishnolia, K. K., Hoene, C., Tarhbalouti, K., Revenstorff, J., Aherrahrou, Z., Erdmann, J. J. F., et al. (2020). Studies in zebrafish demonstrate that CNNM2 and NT5C2 are most likely the causal genes at the blood pressure-associated locus on human chromosome 10q24. Front. Cardiovasc. Med. 32:135. doi: 10.3389/fcvm.2020.00135
Wallace, S., Guo, D. C., Regalado, E., Mellor-Crummey, L., Bamshad, M., Nickerson, D. A., et al. (2016). Disrupted nitric oxide signaling due to GUCY1A3 mutations increases risk for moyamoya disease, achalasia and hypertension. Clin. Genet. 90, 351–360. doi: 10.1111/cge.12739
Westerfield, M. (2000). A Guide for the Laboratory Use of Zebrafish (Danio rerio) The Zebrafish Book. Eugene, OR: Zebrafish International Resource Center.
Westerfield, M., Doerry, E., Kirkpatrick, A. E., Driever, W., and Douglas, S. A. (1997). “An on-line database for zebrafish development and genetics research,” in Seminars in Cell & Developmental Biology, ed. J. Davey (Amsterdam: Elsevier), 477–488. doi: 10.1006/scdb.1997.0173
Wilcox, C. S. (2002). Reactive oxygen species: roles in blood pressure and kidney function. Curr. Hypertens. Rep. 4, 160–166. doi: 10.1007/s11906-002-0041-2
Wobst, J., Kessler, T., Dang, T. A., Erdmann, J., and Schunkert, H. (2015). Role of sGC-dependent NO signalling and myocardial infarction risk. J. Mol. Med. 93, 383–394. doi: 10.1007/s00109-015-1265-3
Keywords: gucy1a1, GUCY1A3, zebrafish, blood flow, soluble guanylate cyclase
Citation: Vishnolia KK, Rakovic A, Hoene C, Tarhbalouti K, Aherrahrou Z and Erdmann J (2021) sGC Activity and Regulation of Blood Flow in a Zebrafish Model System. Front. Physiol. 12:633171. doi: 10.3389/fphys.2021.633171
Received: 25 November 2020; Accepted: 08 February 2021;
Published: 25 February 2021.
Edited by:
Eduardo Nava, University of Castilla-La Mancha, SpainReviewed by:
Liqing Zang, Mie University, JapanSharath C. Kandhi, New York Medical College, United States
Juan Llopis, University of Castilla-La Mancha, Spain
Copyright © 2021 Vishnolia, Rakovic, Hoene, Tarhbalouti, Aherrahrou and Erdmann. This is an open-access article distributed under the terms of the Creative Commons Attribution License (CC BY). The use, distribution or reproduction in other forums is permitted, provided the original author(s) and the copyright owner(s) are credited and that the original publication in this journal is cited, in accordance with accepted academic practice. No use, distribution or reproduction is permitted which does not comply with these terms.
*Correspondence: Krishan K. Vishnolia, YnVudHkua3Jpc0BnbWFpbC5jb20=; Jeanette Erdmann, SmVhbmV0dGUuZXJkbWFubkB1bmktbHVlYmVjay5kZQ==