- State Key Laboratory of Oral Diseases, Department of Oral and Maxillofacial Surgery, National Clinical Research Center for Oral Diseases, West China Hospital of Stomatology, Sichuan University, Chengdu, China
Craniofacial muscles emerge as a developmental novelty during the evolution from invertebrates to vertebrates, facilitating diversified modes of predation, feeding and communication. In contrast to the well-studied limb muscles, knowledge about craniofacial muscle stem cell biology has only recently starts to be gathered. Craniofacial muscles are distinct from their counterparts in other regions in terms of both their embryonic origin and their injury response. Compared with somite-derived limb muscles, pharyngeal arch-derived craniofacial muscles demonstrate delayed myofiber reconstitution and prolonged fibrosis during repair. The regeneration of muscle is orchestrated by a blended source of stem/progenitor cells, including myogenic muscle satellite cells (MuSCs), mesenchymal fibro-adipogenic progenitors (FAPs) and other interstitial progenitors. Limb muscles host MuSCs of the Pax3 lineage, and FAPs from the mesoderm, while craniofacial muscles have MuSCs of the Mesp1 lineage and FAPs from the ectoderm-derived neural crest. Both in vivo and in vitro data revealed distinct patterns of proliferation and differentiation in these craniofacial muscle stem/progenitor cells. Additionally, the proportion of cells of different embryonic origins changes throughout postnatal development in the craniofacial muscles, creating a more dynamic niche environment than in other muscles. In-depth comparative studies of the stem cell biology of craniofacial and limb muscles might inspire the development of novel therapeutics to improve the management of myopathic diseases. Based on the most up-to-date literature, we delineated the pivotal cell populations regulating craniofacial muscle repair and identified clues that might elucidate the distinct embryonic origin and injury response in craniofacial muscle cells.
Introduction
The emergence of craniofacial muscles, together with the skull and sensory organs derived from the placodes and neural crest, contributed to the development of the vertebrate head, an evolutionary novelty (Gans and Northcutt, 1983; Glenn Northcutt, 2005). The muscularized pharynx and enhanced sensory abilities facilitated a more active predatory lifestyle during the transition from invertebrates to the early vertebrates (Vyas et al., 2020). In humans, the craniofacial muscles are small-to-medium-sized skeletal muscles, of which there are approximately 70, and they are involved in physiological activities such as facial expressions, food intake, respiration, and speech (Schubert et al., 2019). These muscle functions could be easily impaired by congenital deformities, tumor invasion, and traumatic injury (desJardins-Park et al., 2019). Once affected, craniofacial muscles can only be incompletely repaired with limited treatment modalities, resulting in inadequate muscle restoration and excessive fibrotic tissue production (Schreurs et al., 2020). A lack of knowledge about craniofacial muscle regeneration might contribute to the limited treatment options (Von den Hoff et al., 2019; Schreurs et al., 2020). Because skeletal muscle possesses high regenerative capacity, in that a single transplanted myofiber can generate more than one hundred new myofibers (Collins et al., 2005), therapeutic strategies to boost craniofacial regeneration could be useful.
Unlike the extensively studied limb and trunk muscles, the characteristics of craniofacial muscles have only been investigated in recent years. Researchers have gradually realized that craniofacial muscles have disparate evolutionary origins and undergo distinct developmental trajectories from limb and trunk muscles (Diogo et al., 2015; Schubert et al., 2019; Vyas et al., 2020). Moreover, their specificity in embryonic origin is accompanied by distinct muscle regeneration processes in which the muscle stem cell behavior also differs. Upon injury, the masseter muscle is likely to undergo inadequate muscle regeneration and excessive fibrosis (Ono et al., 2010; Randolph et al., 2015). Nevertheless, the underlying mechanism remains largely unknown.
Skeletal muscle regeneration is orchestrated by the interactions among multiple resident muscle cells (Wosczyna and Rando, 2018). Of these cells, myogenic muscle satellite cells (MuSCs) and mesenchymal fibroadipogenic progenitors (FAPs) have been found to be important. MuSCs play a principal role in the initiation and completion of myogenesis in response to acute or chronic injury (Dumont et al., 2015a; Wosczyna and Rando, 2018). They proliferate and differentiate to produce myogenic progenitor cells, which ultimately fuse with each other to reconstitute myofiber integrity and function (Dumont et al., 2015b). Meanwhile, FAPs have a vital function in extracellular matrix deposition, which is equally indispensable to skeletal muscle regeneration (Bentzinger et al., 2012; Yin et al., 2013; Biferali et al., 2019). Accumulating evidence indicates the distinct cellular behavior of MuSCs and FAPs in the craniofacial region. Understanding the differential regulatory mechanisms involved in muscle regeneration in the craniofacial region and other anatomic regions would support the development of novel muscle repair strategies.
In this review, we aim to provide an up-to-date discussion of the unique properties of the major cell groups in craniofacial muscles, ranging from their embryonic origin to injury response.
The Architect of Muscle Regeneration: Satellite Cells
Muscle satellite cells are peripherally located myofiber-associated stem cells wedged between the plasma membrane and basal lamina (Dumont et al., 2015a). MuSCs have a large nuclear-to-cytoplasmic ratio, which is a morphological feature typical of stem cells (Dumont et al., 2015a). The MuSC population is characterized by the expression of the transcription factor Pax7 (Dumont et al., 2015b) and can be isolated by flow cytometry via the surface markers Intergrin-α7 and Vcam1 (Dumont et al., 2015b). In healthy, unstressed muscle, MuSCs are mitotically quiescent (Baghdadi et al., 2018). When activated, MuSCs undergo either symmetric or asymmetric division (Forbes and Rosenthal, 2014). Symmetric division enables MuSCs to replenish the stem cell pool, while asymmetric division facilitates myogenic differentiation and MuSC self-renewal (Feige et al., 2018).
Craniofacial MuSCs Are of Diverse Embryonic Origins
Both the craniofacial muscles and the trunk/limb muscles derive from the mesoderm (Diogo et al., 2015; Cao et al., 2019; Schubert et al., 2019), but there are many embryonic differences. The craniofacial muscles are derivatives of the cranial mesoderm, while the trunk/limb muscles are derivatives of the lateral plate mesoderm, leading to distinct developmental patterns and genetic lineages in the two muscle groups (Vyas et al., 2020). In addition, there is substantial heterogeneity in developmental origins within the craniofacial muscles among the subgroups (Lescroart et al., 2010).
In the established model of trunk/limb muscle development, the lateral plate mesoderm is segmented into somites under local oscillations of gene expression (Chang and Kioussi, 2018; Vyas et al., 2020). The somites of the Pax3/Pax7 lineage then give rise to the corresponding trunk/limb muscles in different body segments (Harel et al., 2009). In contrast, the cranial mesoderm generates three discrete developmental units based on their general function and developmental origin (Schubert et al., 2019; Sefton and Kardon, 2019; Vyas et al., 2020): (1) Mesp1/Pitx2 lineage extraocular muscles (EOM) derived from the prechordal mesoderm (Harel et al., 2009; Keefe et al., 2015); (2) Mesp1/Isl1 lineage pharynx/cranial openings-associated muscles derived from pharyngeal arches (Harel et al., 2009; Schubert et al., 2019); (3) Mesp1/Pax3 lineage tongue muscles derived from the most anterior somites (Harel et al., 2009; Diogo et al., 2015; Heude et al., 2018). Thus, the organization of the cranial mesoderm is more precisely delineated by molecular markers than by anatomical boundaries. In particular, the Mesp1/Isl1 lineage craniofacial muscles and the cardiac muscle share the same developmental origin: the cardiopharyngeal field (Diogo et al., 2015). The critical cardiac lineage marker Isl1 also mediates craniofacial muscle myogenesis (Harel et al., 2009; Chan et al., 2013; Vyas et al., 2020).
Muscle satellite cells share the ontogeny of the muscles in which they reside (Dumont et al., 2015b). Trunk/limb MuSCs belong to the Pax3/Pax7 lineage (Nogueira et al., 2015), while the embryonic origins of craniofacial muscle MuSCs can be classified into the following three categories (Schubert et al., 2019; Vyas et al., 2020): (1) extraocular MuSCs are of the Mesp1/Pitx2 lineage; (2) MuSCs associated with the pharyngeal arch muscles, which include the jaw muscles, facial expression muscles and pharynx- and larynx-associated muscles, which are of the Mesp1/Isl1 lineage; and (3) MuSCs associated with the tongue muscles, which are of the Mesp1/Pax3 lineage (Figure 1). In addition to their different lineages, craniofacial MuSCs have distinct myogenic regulatory routes. During the embryonic myogenesis of trunk/limb muscles, Pax7 typically marks the stem cell state of MuSCs, and its expression precedes that of the myogenic commitment marker MyoD (Nogueira et al., 2015; Chang and Kioussi, 2018). In contrast, the expression of MyoD in craniofacial muscles during embryogenesis occurs earlier than that of Pax7, indicating that the cranial mesoderm is committed to myogenesis before the emergence of craniofacial MuSCs (Nogueira et al., 2015). The de novo expression of Pax7 in craniofacial MuSCs during embryonic development, which is in contrast to its consistent expression in limb and trunk MuSCs, may be a result of their different evolutionary histories (Nogueira et al., 2015).
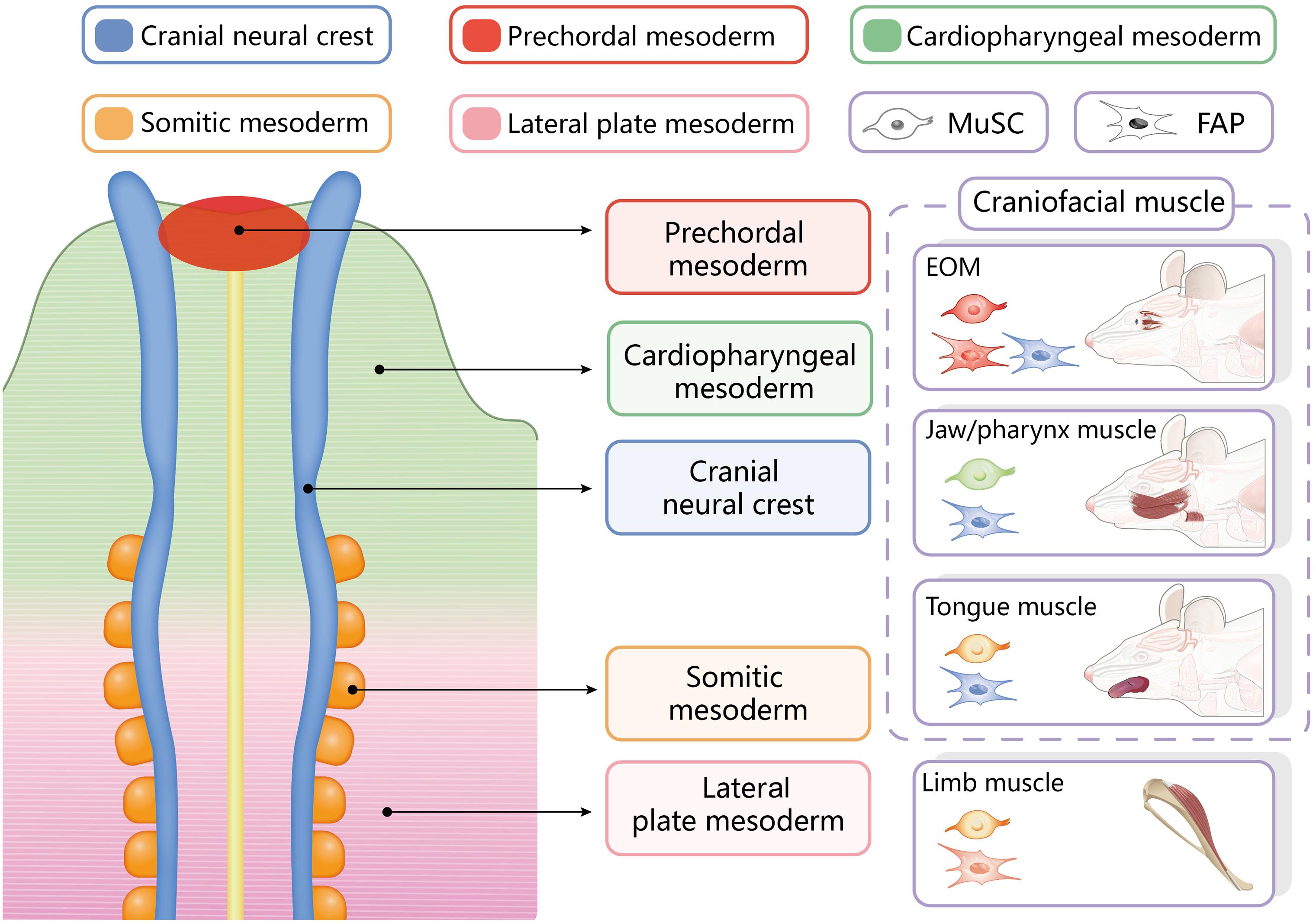
Figure 1. Embryonic origins of resident stem cells in different craniofacial and trunk/limb muscles. EOM, extraocular muscles.
Craniofacial MuSCs Behave Distinctly in Homeostasis Maintenance and Regeneration
Because skeletal muscle regeneration largely resembles embryonic muscle development, a significant disparity exists between adult myogenesis in craniofacial and limb muscle regeneration. Craniofacial MuSCs exhibit distinct behaviors both in a homeostatic state and in response to acute and chronic stimuli (Ono et al., 2010; Keefe et al., 2015; Rodriguez et al., 2020a,b).
Mesp1 Lineage Extraocular Muscle MuSCs
Extraocular muscles have long been considered a special muscle group because they are spared in Duchenne muscular dystrophy (Stuelsatz et al., 2015; Verma et al., 2017). Subsequent in vivo and in vitro analyses provided various evidence to elucidate the mechanisms underlying this observation. The proportion of MuSCs in adult uninjured EOM is five times higher than that in typical limb muscles (Stuelsatz et al., 2015; Verma et al., 2017). The elevated MuSC proportion even persists in aged individuals, and EOM are accordingly more resistant to aging-related degeneration (Formicola et al., 2014; Stuelsatz et al., 2015). Remarkably, EOM undergo more active myonuclei turnover, with nearly 80% of all myofibers undergoing MuSC fusion in the steady state, which is in sharp contrast to the 20% in limb muscles (Formicola et al., 2014). Further analysis revealed that EOM MuSCs could act as a powerful myo-engine, with strong proliferation, differentiation, and self-renewal capacities (La Rovere et al., 2014; Stuelsatz et al., 2015). The transplantation of EOM MuSCs to the limb leads to more vigorous regeneration in the host muscles than in their limb donor counterparts (Stuelsatz et al., 2015). Mechanistic studies indicated that the higher expression of trophic factors in EOM MuSCs, including brain-derived neurotrophic factors and nerve growth factors, may account for the enhanced myogenic activities (Carrero-Rojas et al., 2020).
Mesp1/Isl1 Pharyngeal Arch MuSCs
Pharyngeal arch muscles, also called branchiomeric muscles, constitute the vast majority of craniofacial muscles (Sambasivan et al., 2009; Randolph and Pavlath, 2015), the most studied of which are the first pharyngeal arch-derived masseter and the posterior pharyngeal arch-derived pharyngeal muscles (Sambasivan et al., 2009; Randolph and Pavlath, 2015; Chan et al., 2016). Again, the masseter and pharynx MuSCs each exhibit features distinct from those of trunk/limb MuSCs (Kim et al., 2020).
Unlike in EOM, the proportion of MuSCs in intact masseters is significantly lower than that in their limb counterparts (Pavlath et al., 1998; Ono et al., 2010; Keefe et al., 2015). During aging, however, the number of MuSCs in the masseter increases twofold, which is in contrast to the common decline in the number of MuSCs in aging limb muscles (Ono et al., 2010; Keefe et al., 2015). In response to acute injury, masseter muscles have relatively less efficient regeneration (Pavlath et al., 1998; Ono et al., 2010). Two weeks after a freezing injury, the limb muscles had recovered to their basal level, while the masseter was still infiltrated by a large amount of fibrous tissue. It took 40 weeks for the masseter muscle to fully recover (Pavlath et al., 1998; Yoshioka et al., 2021). The inferior regenerative capacity in the masseter has been correlated with fewer total and proliferating myoblasts during regeneration (Pavlath et al., 1998; Yoshioka et al., 2021). In fact, masseter MuSCs cultured in vitro demonstrated more active proliferation but delayed differentiation than those isolated from limb muscles (Ono et al., 2010). Fewer resident MuSCs along with prolonged proliferation and delayed differentiation may explain the inefficient regeneration of the masseter. Nevertheless, transplantation assays revealed that masseter MuSCs could regenerate the host muscles as efficiently as limb muscle MuSCs (Ono et al., 2010).
In pharynx MuSCs, the most prominent feature is more active myonuclei turnover in the absence of induced injury than limb MuSCs (Randolph et al., 2015). A larger proportion of proliferative progeny may be responsible for the elevated contribution of new myonuclei to the uninjured myofibers (Randolph et al., 2015). Another feature is that MuSCs are required for the maintenance of the myonuclear number and myofiber size in certain pharynx muscles, while limb MuSCs do not contribute to muscle mass maintenance (Randolph and Pavlath, 2015; Randolph et al., 2015). Moreover, MuSCs in the nasopharynx, oropharynx and laryngopharynx respond to chronic injury and aging differently (Randolph et al., 2014). Only laryngopharynx MuSCs are sensitive to antiaging treatments intended to combat muscle atrophy, as evidenced by the increased myofiber size and level of central nuclei, a hallmark of MuSC-mediated muscle regeneration (Randolph et al., 2014). The fact that pharynx MuSCs exhibit region-specific characteristics makes them a more complex stem cell group to study.
Apart from their particular regenerative behavior, branchiomeric MuSCs still retain plasticity in cardiogenesis, which is absent in limb MuSCs (Daughters et al., 2017; Huang et al., 2018; Schubert et al., 2019). The expression of the cardiac-specific gene Tcf21 is evident in cultured adult branchiomeric MuSC (Harel et al., 2009; Daughters et al., 2017). When exposed to Bmp4 stimuli, branchiomeric MuSCs exhibited drastically elevated expression levels of the cardiogenesis marker genes Nkx2.5 and Tbx20, which were not observed in limb MuSCs cultured under the same conditions (Harel et al., 2009). Although the possibility of manipulating branchiomeric MuSCs to regenerate the heart is still under investigation, preliminary studies have shown that they could be an alternative source for cardiomyocytes (Daughters et al., 2017; Huang et al., 2018).
Mesp1/Pax3 Tongue MuSCs
Tongue muscle regeneration is needed in patients with conditions such as oral cancer invasion into the floor of the mouth (Camacho-Alonso et al., 2020; Kinoshita et al., 2020). Investigations into the MuSC regeneration capacity of this muscle group are rare. The results from the limited studies available demonstrate that myoblasts obtained from somite-derived muscles can regenerate the tongue muscle (Cobourne et al., 2019; Kletzien et al., 2020). The common Pax3 lineage of the tongue MuSCs and the limb muscle MuSCs may mean that there are relatively more similarities between these two muscle groups. Further investigation into Mesp1/Pax3 lineage MuSCs is needed to characterize muscle stem cell behavior in this transitional region.
When compared with the trunk/limb MuSCs, all three lineages of craniofacial MuSCs have drastic differences in, homeostasis maintenance and injury response (Table 1). Comparisons among the three different craniofacial MuSCs lineages, however, are currently unavailable. Considering the existing heterogeneity in the developmental origin inside the craniofacial MuSC group, further studies to delineate the differences in MuSC behavior among different craniofacial subgroups are needed to develop a comprehensive understanding of craniofacial MuSC biology.
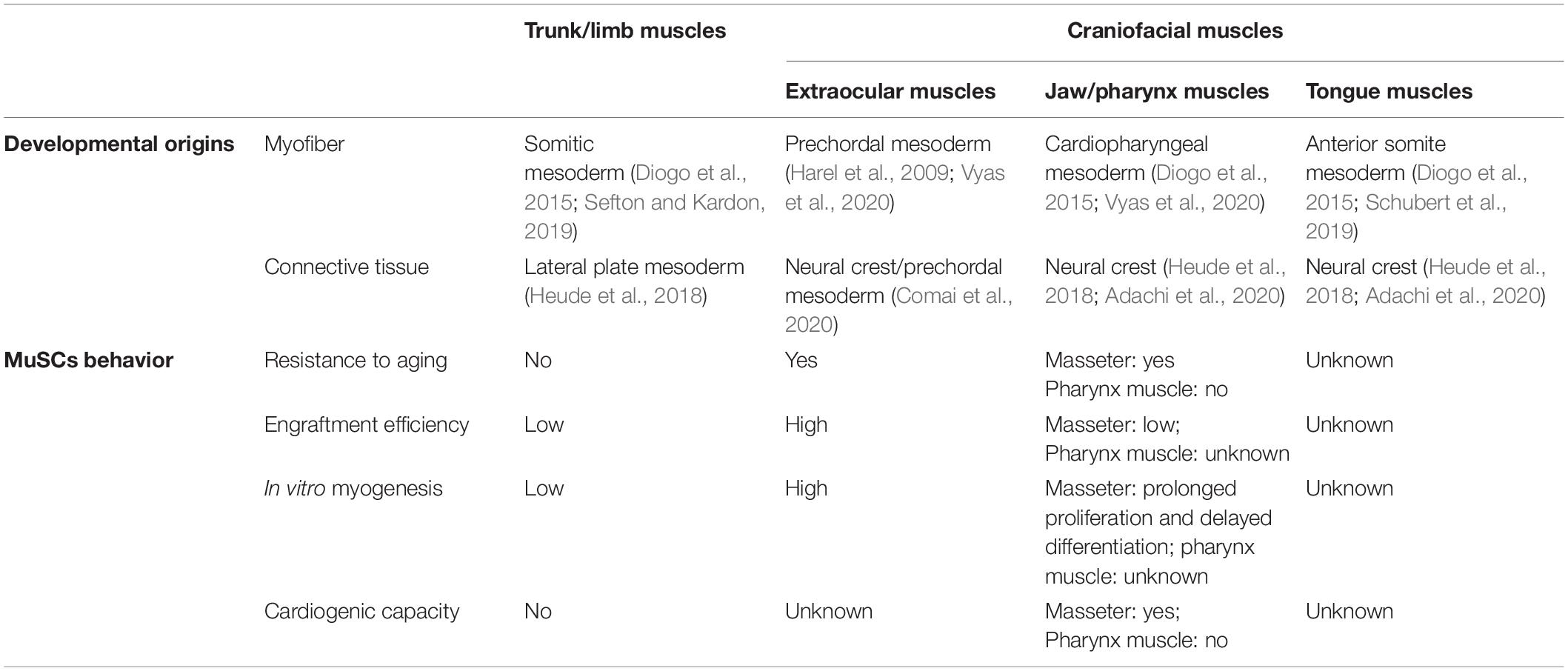
Table 1. Embryonic origins and resident muscle satellite cells (MuSCs) cell behavior among craniofacial muscles and trunk/limb muscles.
A Critical Support From the Interstitial Space: FAPs
Fibro-adipogenic progenitors are muscle stromal cells adjacent to the abluminal side of capillaries between myofibers (Lemos and Duffield, 2018). They express the mesenchymal stem cell markers Sca-1 and Pdgfrα (Wosczyna and Rando, 2018). Acting as structural support and signal guides, FAPs are important participants in the regeneration process. They serve as the progenitors of the muscle connective tissue (Vyas et al., 2020) and are the closest anatomical and functional partners of the myofiber (Sefton and Kardon, 2019). When an injury occurs, FAPs infiltrate the damaged area and synthesize extracellular matrix, which provides a framework for myofiber reconstruction (Biferali et al., 2019). Then FAPs undergo programed cell death to avoid excessive fibrogenesis (Lemos and Duffield, 2018; Wosczyna and Rando, 2018; Biferali et al., 2019). Meanwhile, normal FAPs function is indispensable to MuSCs differentiation because it provides promyogenic factors such as Wisp1 and Il-10 in a paracrine manner (Biferali et al., 2019). The temporal dynamics of FAPs and MuSCs during muscle regeneration overlap substantially, indicating close intercellular communication (Wosczyna and Rando, 2018).
Heterogenic and Dynamic Embryonic Origin of Craniofacial FAPs
From an embryogenesis perspective, FAPs share the same origin as muscle connective tissues (Lemos et al., 2012). Generally, the connective tissue within craniofacial muscle is derivative of cranial neural crest, while those within the limb and trunk muscle are lateral plate mesoderm-derived somite (Schubert et al., 2019; Sefton and Kardon, 2019; Vyas et al., 2020). Neural crest cells exert a transient promoting effect on limb muscle myogenesis, but their regulatory role in head muscle development is extensive and lasting. Although it is not necessary for the initiation of craniofacial muscle myogenesis, the cranial neural crest play a crucial role in regulating the migration, patterning and differentiation of craniofacial myogenic precursors (Rinon et al., 2007; Heude et al., 2010). Disruption of this cellular interaction may lead to developmental anomalies, as evidenced in the muscle connective tissue defect identified in patients with hemifacial microsomia (Heude et al., 2010, 2011). Craniofacial muscle patterning is regulated by the surrounding skeletogenic mesenchymal cells derived from the cranial neural crest, while patterning in the trunk/limb muscles is dependent upon signals from the lateral plate mesoderm (Rinon et al., 2007). Strikingly, Wnt signaling acted as an agonist in trunk/limb muscle myogenesis but as an antagonist in craniofacial muscle myogenesis (Rinon et al., 2007).
One distinct characteristic of craniofacial muscle connective tissue is its heterogeneity in embryonic origin (Adachi et al., 2020). Among the seven EOM, the connective tissue associated with four recti muscles is derived from mesoderm, while that associated with the rest three muscles is of neural crest origin (Comai et al., 2020). Furthermore, the composition of craniofacial FAPs undergoes temporal changes after birth (Lemos et al., 2012). Lineage tracing studies revealed that neonatal masseter muscle has the exclusive source of neural crest-derived FAPs, but the mesoderm-derived FAPs blended during subsequent development and growth (Lemos et al., 2012). The proportion of neural crest-derived FAPs in mouse masseter declined to 70% at 5 weeks of age and to 20–30% at adulthood (Lemos et al., 2012). The fluctuation in the composition of different developmental origins during postnatal growth marks another significant characteristic of craniofacial muscle FAPs.
Distinct FAPs Behavior in Craniofacial Muscle Homeostasis and Regeneration
Although less well studied, FAPs may play relatively more profound roles in craniofacial muscle regeneration. The impetus to study the functional role of FAPs in muscle regeneration only began several years ago, and investigations focusing on craniofacial FAPs are rare. Nevertheless, the putatively disparate performance of neural crest cell-derived craniofacial FAPs has been suggested (Lemos et al., 2012; Formicola et al., 2014; Paylor et al., 2014; Stuelsatz et al., 2014; Comai et al., 2020). Take the EOM muscles, for example, the proportion of FAPs in the MuSC niche in the EOM is much higher than that in the limb, and this trend is maintained in aged individuals and in dystrophic muscles (Formicola et al., 2014). The elevated FAP/MuSC ratio may contribute to the exemption of EOMs from muscular dystrophy-related pathological changes (Formicola et al., 2014). In the notexin-induced masseter regeneration model, neural crest-derived FAPs, rather than mesoderm-derived FAPs, were the main FAP population responsible for muscle regeneration (Paylor et al., 2014).
The ectoderm origin of FAPs has been correlated with a stronger fibrogenesis tendency in craniofacial muscles (Rosero Salazar et al., 2020), which constitutes the pathophysiological foundation of the impaired craniofacial muscle regeneration. Because tissue-specific resident mesenchymal stem cells emerge as targets of fibrosis therapies, as is the case in kidney, lung and skin, FAPs may play a critical role in ameliorating craniofacial muscle fibrosis (Lemos and Duffield, 2018). Because FAPs play pleiotropic roles in inducing fibrogenesis and promoting MuSC differentiation, targeting FAPs alone may suffice to combat fibrosis and enhance myogenesis at the same time.
Other Myogenic Progenitor Cells in the Stroma
In addition to the above mentioned two types of stem cells, there are other myogenic progenitor cells residing in the interstitial space of muscle. The most studied categories include Pw1+ interstitial cells and Twist2+ cells.
In the attempt to identify specific stem cells responsible for muscle regeneration, Pw1+ cells have garnered a great deal of attention. In fact, the cell stress mediator Pw1 marks two stem cell populations residing in skeletal muscle: Pw1+ /Pax7+ MuSCs and Pw1+ /Pax7- interstitial cells (PICs). PICs are bipotent progenitor cells that give rise to both smooth muscle and skeletal muscle in vitro. Although PICs are not of the Pax7 lineage, they require Pax7 for myogenic specification. In addition, a subgroup of PICs share overlapping surface markers with FAPs (Sca-1 and Pdgfrα) and possess fibrogenic and adipogenic capacity, while the Pdgfrα- PICs are myogenic (Mitchell et al., 2010; Pannerec et al., 2013).
Another type of interstitial stem cell with myogenic potential is the Twist2+ cell. Unlike MuSCs, which contribute to the formation of all types of myofibers, Twist2+ cells are type IIb/x fiber specific myogenic progenitors (Liu et al., 2017). Remarkably, the Twist2+ cells do not express the MuSCs marker Pax7 but can initiate myogenesis both in vitro and in vivo (Liu et al., 2017). Once committed to myogenic lineage, these cells downregulated Twist2 expression and began to express Pax7 (Liu et al., 2017). A notable difference between MuSC and Twsit2+ cells is their opposite roles in muscle regeneration and myofiber size maintenance. MuSCs exert minimal effects on muscle fiber size, as evidenced by the unaffected sarcopenia in Pax7-ablated mice (McCarthy et al., 2011; Fry et al., 2015). In contrast, Twist2+ cells are required for type IIb/x fiber size maintenance, but their ablation does not affect muscle regeneration (Liu et al., 2017). The hypothesis is that Twist2+ cells undergo a pre-myogenic state but are insufficient to guide full regeneration (Liu et al., 2017). It is interesting that Twist2+ cells are specifically excluded from the tongue musculature (Liu et al., 2017). Since type IIb/x fibers are the most abundant in all skeletal muscles and are the most susceptible to aging and disease in mice (Liu et al., 2017), further investigation is needed to characterize the myogenesis mechanism in type IIb/x fibers from different muscle lineages to facilitate the treatment of muscle diseases.
Summary
In contrast to trunk/limb muscles, which are ancestral muscles essential to the support and locomotion of the entire body, craniofacial muscles have long been regarded as a variation on the general body muscle scheme. This variation is manifested in many aspects, including developmental origin, myogenic regulatory trajectory, susceptibility to muscular diseases and regenerative capacity. This discrepancy between craniofacial and trunk/limb muscles may to related to their different embryonic origins and to the Hox gene, which conveys positional memory (Leucht et al., 2008). Studies comparing the bone regeneration process of neural crest-derived mandible and mesoderm-derived tibia revealed that heterogeneity in both the embryonic origin and Hox code could account for the ectopic chondrogenesis instead of osteogenesis in tibia-to-mandible periosteum stem cell transplantation assays (Leucht et al., 2008). A similar genetic marker bearing their ancestor’s phenotypic identity, perhaps HoxA and/or HoxB (Rinon et al., 2007; Vieux-Rochas et al., 2013), might also explain the trunk/limb vs. craniofacial muscle differences. Nevertheless, the mechanism underlying the different regenerative response between craniofacial and limb/trunk muscles may vary, because histological differences already exist, in contrast to the histological equivalency with cellular and molecular disparity in the comparison between mandible and tibia (Mitchell et al., 2010). The concept of positional memory should at least be taken into account. Currently, most observations of the features of craniofacial muscles remain at the level of tissue phenotype or cellular behavior. Investigation into the differences in molecular regulatory mechanisms between craniofacial muscles and trunk/limb muscles or among different subgroups of craniofacial muscles, is needed to provide targets for effective muscle regeneration modalities.
Author Contributions
XC and JL conceived the idea of writing this manuscript. XC drafted the manuscript. JL drew the figure and critically revised the manuscript. BS critically revised the manuscript. XC and BS provided the funding support. All authors contributed to the article and approved the submitted version.
Funding
Grant from West China School of Stomatology, Sichuan University to BS (Grant No. RD-03-202007) and National Natural Science Foundation of China to XC (Grant No. 82001031).
Conflict of Interest
The authors declare that the research was conducted in the absence of any commercial or financial relationships that could be construed as a potential conflict of interest.
References
Adachi, N., Bilio, M., Baldini, A., and Kelly, R. G. (2020). Cardiopharyngeal mesoderm origins of musculoskeletal and connective tissues in the mammalian pharynx. Development 147:dev185256. doi: 10.1242/dev.185256
Baghdadi, M. B., Castel, D., Machado, L., Fukada, S. I., Birk, D. E., Relaix, F., et al. (2018). Reciprocal signalling by Notch-Collagen V-CALCR retains muscle stem cells in their niche. Nature 557, 714–718. doi: 10.1038/s41586-018-0144-9
Bentzinger, C. F., Wang, Y. X., and Rudnicki, M. A. (2012). Building muscle: molecular regulation of myogenesis. Cold Spring Harb. Perspect. Biol. 4:a008342. doi: 10.1101/cshperspect.a008342
Biferali, B., Proietti, D., Mozzetta, C., and Madaro, L. (2019). Fibro-adipogenic progenitors cross-talk in skeletal muscle: the social network. Front. Physiol. 10:1074. doi: 10.3389/fphys.2019.01074
Camacho-Alonso, F., García-Carrillo, N., Buendía, A. J., Navarro, J. A., Peñarrocha-Oltra, D., Peñarrocha-Diago, M., et al. (2020). Regeneration of lingual musculature in rats using myoblasts over porcine bladder acellular matrix. Oral Dis. doi: 10.1111/odi.13674 [Online ahead of print].
Cao, J., Spielmann, M., Qiu, X., Huang, X., Ibrahim, D. M., Hill, A. J., et al. (2019). The single-cell transcriptional landscape of mammalian organogenesis. Nature 566, 496–502. doi: 10.1038/s41586-019-0969-x
Carrero-Rojas, G., Benítez-Temiño, B., Pastor, A. M., Davis López, and de Carrizosa, M. A. (2020). Muscle progenitors derived from extraocular muscles express higher levels of neurotrophins and their receptors than other cranial and limb muscles. Cells 9:747. doi: 10.3390/cells9030747
Chan, S. S., Hagen, H. R., Swanson, S. A., Stewart, R., Boll, K. A., Aho, J., et al. (2016). Development of bipotent cardiac/skeletal myogenic progenitors from MESP1+ mesoderm. Stem Cell Rep. 6, 26–34. doi: 10.1016/j.stemcr.2015.12.003
Chan, S. S., Shi, X., Toyama, A., Arpke, R. W., Dandapat, A., Iacovino, M., et al. (2013). Mesp1 patterns mesoderm into cardiac, hematopoietic, or skeletal myogenic progenitors in a context-dependent manner. Cell Stem Cell 12, 587–601. doi: 10.1016/j.stem.2013.03.004
Chang, C. N., and Kioussi, C. (2018). Location, location, location: signals in muscle specification. J. Dev. Biol. 6:11. doi: 10.3390/jdb6020011
Cobourne, M. T., Iseki, S., Birjandi, A. A., Adel Al-Lami, H., Thauvin-Robinet, C., Xavier, G. M., et al. (2019). How to make a tongue: cellular and molecular regulation of muscle and connective tissue formation during mammalian tongue development. Semin. Cell Dev. Biol. 91, 45–54. doi: 10.1016/j.semcdb.2018.04.016
Collins, C. A., Olsen, I., Zammit, P. S., Heslop, L., Petrie, A., Partridge, T. A., et al. (2005). Stem cell function, self-renewal, and behavioral heterogeneity of cells from the adult muscle satellite cell niche. Cell 122, 289–301. doi: 10.1016/j.cell.2005.05.010
Comai, G. E., Tesařová, M., Dupé, V., Rhinn, M., Vallecillo-García, P., da Silva, F., et al. (2020). Local retinoic acid signaling directs emergence of the extraocular muscle functional unit. PLoS Biol. 18:e3000902. doi: 10.1371/journal.pbio.3000902
Daughters, R. S., Keirstead, S. A., and Slack, J. M. W. (2017). Transformation of jaw muscle satellite cells to cardiomyocytes. Differentiation 93, 58–65. doi: 10.1016/j.diff.2016.11.003
desJardins-Park, H. E., Mascharak, S., Chinta, M. S., Wan, D. C., and Longaker, M. T. (2019). The spectrum of scarring in craniofacial wound repair. Front. Physiol. 10:322. doi: 10.3389/fphys.2019.00322
Diogo, R., Kelly, R. G., Christiaen, L., Levine, M., Ziermann, J. M., Molnar, J. L., et al. (2015). A new heart for a new head in vertebrate cardiopharyngeal evolution. Nature 520, 466–473. doi: 10.1038/nature14435
Dumont, N. A., Bentzinger, C. F., Sincennes, M. C., and Rudnicki, M. A. (2015a). Satellite cells and skeletal muscle regeneration. Compr. Physiol. 5, 1027–1059.
Dumont, N. A., Wang, Y. X., and Rudnicki, M. A. (2015b). Intrinsic and extrinsic mechanisms regulating satellite cell function. Development 142, 1572–1581. doi: 10.1002/cphy.c140068
Feige, P., Brun, C. E., Ritso, M., and Rudnicki, M. A. (2018). Orienting muscle stem cells for regeneration in homeostasis. Aging Dis. Cell Stem Cell. 23, 653–664. doi: 10.1016/j.stem.2018.10.006
Forbes, S. J., and Rosenthal, N. (2014). Preparing the ground for tissue regeneration: from mechanism to therapy. Nat. Med. 20, 857–869. doi: 10.1038/nm.3653
Formicola, L., Marazzi, G., and Sassoon, D. A. (2014). The extraocular muscle stem cell niche is resistant to ageing and disease. Front. Aging Neurosci. 6:328. doi: 10.3389/fnagi.2014.00328
Fry, C. S., Lee, J. D., Mula, J., Kirby, T. J., Jackson, J. R., Liu, F., et al. (2015). Inducible depletion of satellite cells in adult, sedentary mice impairs muscle regenerative capacity without affecting sarcopenia. Nat. Med. 21, 76–80. doi: 10.1038/nm.3710
Gans, C., and Northcutt, R. G. (1983). Neural crest and the origin of vertebrates: a new head. Science 220, 268–273. doi: 10.1126/science.220.4594.268
Glenn Northcutt, R. (2005). The new head hypothesis revisited. J. Exp. Zool. B Mol. Dev. Evol. 304, 274–297. doi: 10.1002/jez.b.21063
Harel, I., Nathan, E., Tirosh-Finkel, L., Zigdon, H., Guimarães-Camboa, N., Evans, S. M., et al. (2009). Distinct origins and genetic programs of head muscle satellite cells. Dev. Cell 16, 822–832. doi: 10.1016/j.devcel.2009.05.007
Heude, E., Bouhali, K., Kurihara, Y., Kurihara, H., Couly, G., Janvier, P., et al. (2010). Jaw muscularization requires Dlx expression by cranial neural crest cells. Proc. Natl. Acad. Sci. U. S. A. 107, 11441–11446. doi: 10.1073/pnas.1001582107
Heude, E., Rivals, I., Couly, G., and Levi, G. (2011). Masticatory muscle defects in hemifacial microsomia: a new embryological concept. Am. J. Med. Genet. A. 155A, 1991–1995. doi: 10.1002/ajmg.a.34095
Heude, E., Tesarova, M., Sefton, E. M., Jullian, E., Adachi, N., and Grimaldi, A. (2018). Unique morphogenetic signatures define mammalian neck muscles and associated connective tissues. Elife 7:e40179. doi: 10.7554/eLife.40179
Huang, W., Liang, J., Feng, Y., Jia, Z., Jiang, L., Cai, W., et al. (2018). Heterogeneity of adult masseter muscle satellite cells with cardiomyocyte differentiation potential. Exp. Cell Res. 371, 20–30. doi: 10.1016/j.yexcr.2018.05.028
Keefe, A. C., Lawson, J. A., Flygare, S. D., Fox, Z. D., Colasanto, M. P., Mathew, S. J., et al. (2015). Muscle stem cells contribute to myofibres in sedentary adult mice. Nat. Commun. 6:7087. doi: 10.1038/ncomms8087
Kim, E., Wu, F., Wu, X., and Choo, H. J. (2020). Generation of craniofacial myogenic progenitor cells from human induced pluripotent stem cells for skeletal muscle tissue regeneration. Biomaterials 248:119995. doi: 10.1016/j.biomaterials.2020.119995
Kinoshita, N., Sasaki, Y., Marukawa, E., Hirose, R., Sawada, S. I., Harada, H., et al. (2020). Crosslinked nanogel-based porous hydrogel as a functional scaffold for tongue muscle regeneration. J. Biomater. Sci. Polym. Ed. 31, 1254–1271. doi: 10.1080/09205063.2020.1744246
Kletzien, H., Kelm-Nelson, C. A., Wang, S., Suzuki, M., and Connor, N. P. (2020). Myogenic marker expression as a function of age and exercise-based therapy in the tongue. Exp. Gerontol. 142:111104. doi: 10.1016/j.exger.2020.111104
La Rovere, R. M., Quattrocelli, M., Pietrangelo, T., Di Filippo, E. S., Maccatrozzo, L., Cassano, M., et al. (2014). Myogenic potential of canine craniofacial satellite cells. Front. Aging Neurosci. 6:90. doi: 10.3389/fnagi.2014.00090
Lemos, D. R., and Duffield, J. S. (2018). Tissue-resident mesenchymal stromal cells: Implications for tissue-specific antifibrotic therapies. Sci. Transl. Med. 10:eaan5174. doi: 10.1126/scitranslmed.aan5174
Lemos, D. R., Paylor, B., Chang, C., Sampaio, A., Underhill, T. M., Rossi, F. M., et al. (2012). Functionally convergent white adipogenic progenitors of different lineages participate in a diffused system supporting tissue regeneration. Stem Cells 30, 1152–1162. doi: 10.1002/stem.1082
Lescroart, F., Kelly, R. G., Le Garrec, J. F., Nicolas, J. F., Meilhac, S. M., Buckingham, M., et al. (2010). Clonal analysis reveals common lineage relationships between head muscles and second heart field derivatives in the mouse embryo. Development 137, 3269–3279. doi: 10.1242/dev.050674
Leucht, P., Kim, J. B., Amasha, R., James, A. W., Girod, S., and Helms, J. A. (2008). Embryonic origin and Hox status determine progenitor cell fate during adult bone regeneration. Development 135, 2845–2854. doi: 10.1242/dev.023788
Liu, N., Garry, G. A., Li, S., Bezprozvannaya, S., Sanchez-Ortiz, E., Chen, B., et al. (2017). A Twist2-dependent progenitor cell contributes to adult skeletal muscle. Nat. Cell Biol. 19, 202–213. doi: 10.1038/ncb3477
McCarthy, J. J., Mula, J., Miyazaki, M., Erfani, R., Garrison, K., Farooqui, A. B., et al. (2011). Effective fiber hypertrophy in satellite cell-depleted skeletal muscle. Development 138, 3657–3666. doi: 10.1242/dev.068858
Mitchell, K. J., Pannérec, A., Cadot, B., Parlakian, A., Besson, V., Gomes, E. R., et al. (2010). Identification and characterization of a non-satellite cell muscle resident progenitor during postnatal development. Nat. Cell Biol. 12, 257–266. doi: 10.1038/ncb2025
Nogueira, J. M., Hawrot, K., Sharpe, C., Noble, A., Wood, W. M., Jorge, E. C., et al. (2015). The emergence of Pax7-expressing muscle stem cells during vertebrate head muscle development. Front. Aging Neurosci. 7:62. doi: 10.3389/fnagi.2015.00062
Ono, Y., Boldrin, L., Knopp, P., Morgan, J. E., and Zammit, P. S. (2010). Muscle satellite cells are a functionally heterogeneous population in both somite-derived and branchiomeric muscles. Dev. Biol. 337, 29–41. doi: 10.1016/j.ydbio.2009.10.005
Pannerec, A., Formicola, L., Besson, V., Marazzi, G., and Sassoon, D. A. (2013). Defining skeletal muscle resident progenitors and their cell fate potentials. Development 140, 2879–2891. doi: 10.1242/dev.089326
Pavlath, G. K., Thaloor, D., Rando, T. A., Cheong, M., English, A. W., Zheng, B., et al. (1998). Heterogeneity among muscle precursor cells in adult skeletal muscles with differing regenerative capacities. Dev. Dyn. 212, 495–508. doi: 10.1002/(SICI)1097-0177(199808)212:4<495::AID-AJA3>3.0.CO;2-C
Paylor, B., Joe, A. W., Rossi, F. M., and Lemos, D. R. (2014). In vivo characterization of neural crest-derived fibro/adipogenic progenitor cells as a likely cellular substrate for craniofacial fibrofatty infiltrating disorders. Biochem. Biophys. Res. Commun. 451, 148–151. doi: 10.1016/j.bbrc.2014.07.089
Randolph, M. E., and Pavlath, G. K. (2015). A muscle stem cell for every muscle: variability of satellite cell biology among different muscle groups. Front. Aging Neurosci. 7:190. doi: 10.3389/fnagi.2015.00190
Randolph, M. E., Luo, Q., Ho, J., Vest, K. E., Sokoloff, A. J., Pavlath, G. K., et al. (2014). Ageing and muscular dystrophy differentially affect murine pharyngeal muscles in a region-dependent manner. J. Physiol. 592, 5301–5315. doi: 10.1113/jphysiol.2014.280420
Randolph, M. E., Phillips, B. L., Choo, H. J., Vest, K. E., Vera, Y., Pavlath, G. K., et al. (2015). Pharyngeal satellite cells undergo myogenesis under basal conditions and are required for pharyngeal muscle maintenance. Stem Cells 33, 3581–3595. doi: 10.1002/stem.2098
Rinon, A., Lazar, S., Marshall, H., Büchmann-Møller, S., Neufeld, A., Elhanany-Tamir, H., et al. (2007). Cranial neural crest cells regulate head muscle patterning and differentiation during vertebrate embryogenesis. Development 134, 3065–3075. doi: 10.1242/dev.002501
Rodriguez, B. L., Nguyen, M. H., Armstrong, R. E., Vega-Soto, E. E., Polkowski, P. M., Larkin, L. M., et al. (2020a). A comparison of ovine facial and limb muscle as a primary cell source for engineered skeletal muscle. Tissue Eng. Part A 26, 167–177. doi: 10.1089/ten.TEA.2019.0087
Rodriguez, B. L., Vega-Soto, E. E., Kennedy, C. S., Nguyen, M. H., Cederna, P. S., Larkin, L. M., et al. (2020b). A tissue engineering approach for repairing craniofacial volumetric muscle loss in a sheep following a 2, 4, and 6-month recovery. PLoS One 15:e0239152. doi: 10.1371/journal.pone.0239152
Rosero Salazar, D. H., Carvajal Monroy, P. L., Wagener, F. A. D. T. G., Von, and den Hoff, J. W. (2020). Orofacial muscles: embryonic development and regeneration after Injury. J. Dent. Res. 99, 125–132. doi: 10.1177/0022034519883673
Sambasivan, R., Gayraud-Morel, B., Dumas, G., Cimper, C., Paisant, S., Kelly, R. G., et al. (2009). Distinct regulatory cascades govern extraocular and pharyngeal arch muscle progenitor cell fates. Dev .Cell 16, 810–821. doi: 10.1016/j.devcel.2009.05.008
Schreurs, M., Suttorp, C. M., Mutsaers, H. A. M., Kuijpers-Jagtman, A. M., Von, den Hoff, J. W., et al. (2020). Tissue engineering strategies combining molecular targets against inflammation and fibrosis, and umbilical cord blood stem cells to improve hampered muscle and skin regeneration following cleft repair. Med. Res. Rev. 40, 9–26. doi: 10.1002/med.21594
Schubert, F. R., Singh, A. J., Afoyalan, O., Kioussi, C., and Dietrich, S. (2019). To roll the eyes and snap a bite - function, development and evolution of craniofacial muscles. Semin. Cell Dev. Biol. 91, 31–44. doi: 10.1016/j.semcdb.2017.12.013
Sefton, E. M., and Kardon, G. (2019). Connecting muscle development, birth defects, and evolution: an essential role for muscle connective tissue. Curr. Top. Dev. Biol. 132, 137–176. doi: 10.1016/bs.ctdb.2018.12.004
Stuelsatz, P., Shearer, A., and Yablonka-Reuveni, Z. (2014). Ancestral Myf5 gene activity in periocular connective tissue identifies a subset of fibro/adipogenic progenitors but does not connote a myogenic origin. Dev. Biol. 385, 366–379. doi: 10.1016/j.ydbio.2013.08.010
Stuelsatz, P., Shearer, A., Li, Y., Muir, L. A., Ieronimakis, N., Shen, Q. W., et al. (2015). Extraocular muscle satellite cells are high performance myo-engines retaining efficient regenerative capacity in dystrophin deficiency. Dev. Biol. 397, 31–44. doi: 10.1016/j.ydbio.2014.08.035
Verma, M., Fitzpatrick, K., and McLoon, L. K. (2017). Extraocular muscle repair and regeneration. Curr. Ophthalmol. Rep. 5, 207–215. doi: 10.1007/s40135-017-0141-4
Vieux-Rochas, M., Mascrez, B., Krumlauf, R., and Duboule, D. (2013). Combined function of HoxA and HoxB clusters in neural crest cells. Dev. Biol. 382, 293–301. doi: 10.1016/j.ydbio.2013.06.027
Von den Hoff, J. W., Carvajal Monroy, P. L., Ongkosuwito, E. M., van Kuppevelt, T. H., and Daamen, W. F. (2019). Muscle fibrosis in the soft palate: delivery of cells, growth factors and anti-fibrotics. Adv. Drug Deliv. Rev. 146, 60–76. doi: 10.1016/j.addr.2018.08.002
Vyas, B., Nandkishore, N., and Sambasivan, R. (2020). Vertebrate cranial mesoderm: developmental trajectory and evolutionary origin. Cell Mol. Life Sci. 77, 1933–1945. doi: 10.1007/s00018-019-03373-1
Wosczyna, M. N., and Rando, T. A. (2018). A muscle stem cell support group: coordinated cellular responses in muscle regeneration. Dev. Cell 46, 135–143. doi: 10.1016/j.devcel.2018.06.018
Yin, H., Price, F., and Rudnicki, M. A. (2013). Satellite cells and the muscle stem cell niche. Physiol. Rev. 93, 23–67. doi: 10.1152/physrev.00043.2011
Keywords: myogenesis, satellite cell, fibro-adipogenic progenitor, stem cell niche, craniofacial repair
Citation: Cheng X, Shi B and Li J (2021) Distinct Embryonic Origin and Injury Response of Resident Stem Cells in Craniofacial Muscles. Front. Physiol. 12:690248. doi: 10.3389/fphys.2021.690248
Received: 02 April 2021; Accepted: 06 May 2021;
Published: 01 July 2021.
Edited by:
Jean-Pierre Saint-Jeannet, New York University, United StatesReviewed by:
Janine Ziermann, New York University School of Medicine, United StatesGiovanni Levi, Centre National de la Recherche Scientifique (CNRS), France
Copyright © 2021 Cheng, Shi and Li. This is an open-access article distributed under the terms of the Creative Commons Attribution License (CC BY). The use, distribution or reproduction in other forums is permitted, provided the original author(s) and the copyright owner(s) are credited and that the original publication in this journal is cited, in accordance with accepted academic practice. No use, distribution or reproduction is permitted which does not comply with these terms.
*Correspondence: Jingtao Li, bGlqaW5ndGFvODZAMTYzLmNvbQ==