- 1Inter-University Laboratory of Human Movement Science (LIBM), University Savoie Mont Blanc, Chambéry, France
- 2Laboratoire de Pharm-Ecologie Cardiorespiratoire (LAPEC EA4278), Avignon University, Avignon, France
- 3HP2 Laboratory, INSERM, Grenoble Alpes University, Grenoble, France
- 4RSRM EA7442, ID17-ESRF, Grenoble Alpes University, Grenoble, France
- 5Extremes Research Group, School of Sport, Health and Exercise Sciences, Bangor University, Bangor, United Kingdom
- 6Pôle Anesthésie Réanimation, Grenoble Alpes University Hospital, Grenoble, France
Purpose: Positive expiratory pressure (PEP) breathing has been shown to increase arterial oxygenation during acute hypoxic exposure but the underlying mechanisms and consequences on symptoms during prolonged high-altitude exposure remain to be elucidated.
Methods: Twenty-four males (41 ± 16 years) were investigated, at sea level and at 5,085 m after 18 days of trekking from 570 m. Participants breathed through a face-mask with PEP = 0 cmH2O (PEP0, 0–45th min) and with PEP = 10 cmH2O (PEP10, 46–90th min). Arterial (SpO2), quadriceps and prefrontal (near infrared spectroscopy) oxygenation was measured continuously. Middle cerebral artery blood velocity (MCAv, transcranial Doppler), cardiac function (2D-echocardiography), extravascular lung water accumulation (UsLC, thoracic ultrasound lung comets) and acute mountain sickness (Lake Louise score, LLS) were assessed during PEP0 and PEP10.
Results: At 5,085 m with PEP0, SpO2 was 78 ± 4%, UsLC was 8 ± 5 (a.u.) and the LLS was 2.3 ± 1.7 (all P < 0.05 versus sea level). At 5,085 m, PEP10 increased significantly SpO2 (+9 ± 5%), quadriceps (+2 ± 2%) and prefrontal cortex (+2 ± 2%) oxygenation (P < 0.05), and decreased significantly MCAv (−16 ± 14 cm.s–1) and cardiac output (−0.7 ± 1.2 L.min–1) together with a reduced stroke volume (−9 ± 15 mL, all P < 0.05) and no systemic hypotension. PEP10 decreased slightly the number of UsLC (−1.4 ± 2.7, P = 0.04) while the incidence of acute mountain sickness (LLS ≥ 3) fell from 42% with PEP0 to 25% after PEP10 (P = 0.043).
Conclusion: PEP10 breathing improved arterial and tissue oxygenation and symptoms of acute mountain sickness after trekking to very high altitude, despite reduced cerebral perfusion and cardiac output. Further studies are required to establish whether PEP-breathing prophylactic mechanisms also occur in participants with more severe acute mountain sickness.
Introduction
At high-altitude, the low barometric pressure reduces inspired oxygen partial pressure (PiO2) in mountaineers, workers and travellers, leading to reduced arterial oxygenation that can be responsible for symptoms of acute mountain sickness (AMS). As a countermeasure, researchers have investigated a non-pharmacological, lightweight and relatively easy to implement method to prevent AMS by using positive expiratory pressure (PEP) in healthy awake (Savourey et al., 1998; Tannheimer et al., 2009; Agostoni et al., 2010; Nespoulet et al., 2013) and asleep (Lipman et al., 2015) participants. Breathing with a PEP is used in critical care medicine to improve pulmonary gas exchange and compliance (Wang et al., 2016) and besides, has been shown to increase arterial oxygen saturation (SpO2) at high altitude (Nespoulet et al., 2013). It is well known that the severity of hypoxia-induced disabilities is closely correlated with the degree of SpO2 reduction. Burtscher et al. (2004) showed that, for a given altitude (>2,500 m) or equivalent normobaric hypoxic level, a difference of about 4–5% of SpO2 is a key factor that distinguishes people who develop symptoms of altitude intolerance and those remaining clinically healthy. Hence, the ability to reduce the hypoxemic stress may directly decrease the probability of subsequent altitude illness as well as mental and physical performance alterations (Hackett and Roach, 2001). Under heterogeneous experimental designs (e.g., subject characteristics, altitude level, exposure duration, day-time/night-time evaluation, level/type of PEP, simulated vs. terrestrial altitude), 0–23% increase in SpO2 have been reported with PEP breathing (Savourey et al., 1998; Tannheimer et al., 2009; Nespoulet et al., 2013; Lipman et al., 2015; Rupp et al., 2019). However, whether PEP breathing would be a safe and efficient method to improve SpO2 and AMS symptoms remains to be assessed in participants reaching very high altitude (>5,000 m) after several days of trekking, as performed nowadays by an increasing amount of people in the Himalaya and Andean Cordillera.
Together with the reduction in arterial oxygenation during progressive ascents to high altitude, changes in muscle and brain perfusion and/or oxygenation are known to be of critical importance and potentially involved in subject’s functional impairment and AMS (Bailey et al., 2009; Wilson et al., 2009). Hence, whether an improvement in arterial oxygenation with PEP breathing at very high altitude would be associated with beneficial changes in muscle and cerebral oxygenation needs to be determined. Also, cardiac and macrohemodynamic adverse effects (e.g., pulmonary hypertension and systemic hypotension, impaired cardiac filling pressure, depression of cardiac output and subsequent cerebral hypoperfusion) resulting from an important increase in intrathoracic pressure with resembling modalities of airway pressure (e.g., continuous positive airway pressure, CPAP; positive end-expiratory positive pressure, PEEP; forced expiratory manoeuvres) have previously raised important concerns at sea level (Muench et al., 2005) and deserve attention at high altitude. Echocardiographic and transcranial Doppler evaluations during PEP breathing at high altitude would allow determining PEP effects on cardiac and cerebrovascular functions.
Symptoms of AMS may progress to high altitude pulmonary oedema (HAPE), which is the most common cause of death from high altitude sickness (Hackett and Roach, 2001; Luks et al., 2017). HAPE is associated with increased pulmonary arterial pressure, increased shunts and areas of low ventilation/perfusion ratio and probable damage of alveolar-capillary membranes, resulting in the accumulation of extravascular lung water (Korzeniewski et al., 2015). Thoracic ultrasonography (e.g., ultrasound lung comets, UsLC) in studies conducted at high altitude has shown that extravascular pulmonary fluid shift has an inverse relationship with oxygen saturation (Fagenholz et al., 2007). Although diffuse subclinical extravascular fluid accumulation (i.e., increased UsLC) is frequent in healthy lowlander climbers (Pratali et al., 2010; Bouzat et al., 2013), UsLC have been shown to be significantly greater in patients diagnosed with HAPE than in healthy controls, and resolve with treatment (Pratali et al., 2010; Agostoni et al., 2013; Korzeniewski et al., 2015). Breathing with PEP is thought to improve gas exchange and blood oxygenation mainly due to the recruitment of collapsed alveoli and increased alveolar pressure (Duncan et al., 1986; Di Marco et al., 2010). Hence, it can be hypothesized that PEP may reduce lung fluid accumulation, not only in patients with pulmonary oedema at sea level (e.g., acute respiratory distress syndrome) (Di Marco et al., 2010) but also in participants ascending to very high altitude. Whether high altitude-induced UsLC may be reduced with PEP breathing remains to be investigated.
The present study aimed to comprehensively assess the effects of PEP on arterial and tissue oxygen saturation, cardiac alteration, interstitial lung fluid accumulation and AMS symptoms in trekkers exposed to high altitude for a prolonged period. We hypothesized that, in such an extremely challenging environment, PEP breathing would (i) improve systemic as well as muscle and cerebral oxygenation with minor negative effects on cardiac function, (ii) decrease subclinical signs of pulmonary oedema, and (iii) reduce AMS incidence.
Materials and Methods
Participants and Ethical Approval
The study group consisted of 24 healthy male trekkers (mean ± SD; age 41.0 ± 15.6 years, body weight 72.0 ± 11.9 kg, body mass index 23.3 ± 3.8 kg/m2, maximal oxygen consumption 59.3 ± 9.8 mL.kg–1.min–1) with no known cardiovascular, respiratory or cerebral disorders. Inclusion criteria included adult participants over 18 years of age who planned to take part to the Manaslu trek organized by MEDEX Medical Expeditions in 2015, and those willing to provide free, verbal and written informed and on-going consent. Participants’ maximum living altitude was 450 m and were non-acclimatized nor recently exposed to altitude (>1,500 m within the last 3 months) and prophylactic medication for AMS was not allowed before and during the expedition. The study was approved by the National Institute for Social Care and Health Research Wales Research Ethics Service (14/WA/1260) and conformed with the standards set by the latest revision of the Declaration of Helsinki, except for registration in a database.
Study Design
The study design consisted in a first visit to the laboratory of Bangor University, North Wales (65 m above sea level; SL) where participants completed the baseline anthropological measurements and were familiarized with PEP breathing and evaluation procedures. One month later, participants performed 16 to 18 days of trekking from an initial altitude of 570 m to a high altitude base camp (HABC) where a field laboratory was set at 5,085 m (Larkye Pass, Manaslu Circuit in the Nepali Himalaya). Participants were divided into five groups with similar ascent profiles but delayed departure, to allow the field testing to be done in each subject the day after arrival to HABC. The precise ascent profile of the different groups can be found in a previous paper (Sutherland et al., 2017) and in Figure 1. It has not been designed to induce exacerbated risks of altitude sickness but to mimic real exposure in actual trekkers on that tour.
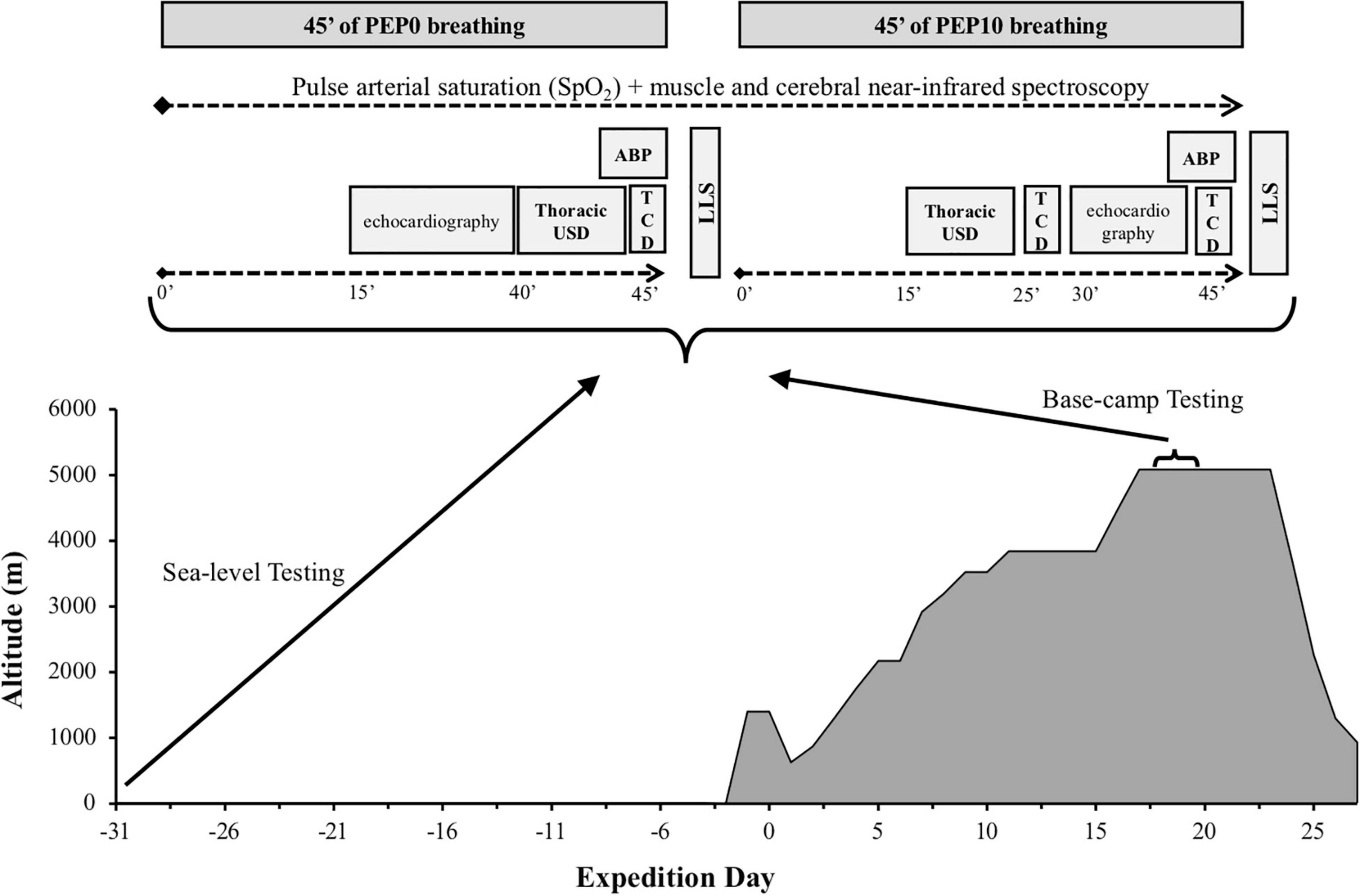
Figure 1. Schematic view of the experimental design and measurements performed at different time points at sea level and high altitude base camp. ABP, systolic-diastolic and mean arterial blood pressure; LLS, Lake Louise Score; TCD, transcranial ultrasound doppler; USD, ultrasonography.
Hence, a repeated measures design was used whereby all participants were exposed to two altitudes in the following order: sea level and high altitude. At each altitude participants were exposed to two conditions in the following order: breathing without any expiratory pressure and breathing with positive expiratory pressure (Figure 1). We choose not to randomize the order in which each modality was performed to avoid previously reported late effects of breathing with expiration against a resistance (Tannheimer et al., 2009), that may have led our data to be misinterpreted.
Experimental Session
At sea level and at HABC, participants laid comfortably in supine position for 90 min, breathing through a facemask (V2maskTM, Hans Rudolph Inc., Shawnee, KS, United States) connected to a three-way valve. The inspiratory side of the valve was open to ambient air while a mechanical resistance (Ambu®, Ballerup, Denmark) was added to the expiratory side of the valve, allowing breathing with PEP = 0 cmH2O (sham, PEP0) from the 1st to the 45th min and breathing with PEP = 10 cmH2O (PEP10) from the 46th to the 90th min. The latter level of pressure was chosen as it appeared to be the most efficient in a previous study (Nespoulet et al., 2013) to improve both SpO2 and muscle oxygenation in healthy participants exposed to acute normobaric hypoxia without significant discomfort.
Measurements
Experimental design and measurements made at different time points at sea level and base camp are summarized in Figure 1.
Clinical Examination
SpO2 was continuously recorded during the tests by finger-pulse oximetry (WristOx2 3150, Nonin Medical, Inc., Plymouth, MA, United States). One-minute averaged values were calculated at the end of the PEP0 period and after 15, 25, and 45 min of PEP10. Particular attention was given to keep participants comfortably warm (e.g., blankets and gloves when necessary) throughout the experimental sessions. Systolic, diastolic and mean arterial blood pressures (SABP, DABP, and MABP, respectively) were assessed non-invasively (Dinamap, GE Medical Systems Inc., Milwaukee, WI, United States) at the end of the PEP0 (45th min) and PEP10 (90th min) periods. Before and after PEP10 at HABC only, participants were asked to complete a self-reported questionnaire for AMS evaluation according to the Lake Louise Score (LLS, 5 items, i.e., “headache,” “gastrointestinal distress,” “fatigue/weakness,” “dizzy/light-headedness,” “sleep disturbance”) (Hackett and Roach, 2001). The presence of AMS was defined as LLS ≥ 3. In addition, a visual analog scale was used at SL and HABC to assess subject’s headache (from no to extreme headache) and breathing discomfort (from no to extreme breathlessness).
Echocardiographic Data Acquisition
Echocardiographic assessments, including standard (morphologic and functional) and 2-D strain parameters and tissue Doppler imaging, were obtained at SL and at HABC, during PEP0 (15–30th min) and after 30 min at PEP10 (70–85th min). A fully trained operator (CM) used a commercially available system (Vivid Q, GE Healthcare, Horten, Norway) with a 3.5-MHz sector scanning electronic transducer and participants in the left lateral decubitus position, according to the recommendations of the American Society of Echocardiography. Two-dimensional grayscale harmonic images were obtained at a rate of 65 to 90 frames/s, and colour tissue velocity images were acquired at a rate of 120 to 140 frames/s. Images were acquired in cine loops triggered to the QRS complex and saved digitally for subsequent blinded off-line analysis with dedicated software (EchoPac 6.0, GE Healthcare). Heart rate (HR), stroke volume (SV) and cardiac output (Qc) were calculated from an apical 5-chamber view. Specific recommendations of the American Society of Echocardiography were used to assess systolic tricuspid regurgitation gradient as surrogate of pulmonary artery systolic pressure (PASP) with the modified Bernoulli equation as described previously: Doppler-estimated PASP = 4 × . Examination of the inferior vena cava (IVC) was also performed in the supine position, in a longitudinal plane with the cardiac transducer in the subxyphoid position. The maximum anterior-posterior IVC diameter at end-expiration was measured 3 to 4 cm from the junction of the IVC and right atrium as an estimate of central venous pressure (Ciozda et al., 2016). Analysis of LV longitudinal and circumferential strains were conducted with speckle tracking imaging as previously described (Maufrais et al., 2017). Left and right ventricle systolic longitudinal strain rates and systolic basal circumferential strain rates were also used as indices of myocardial contractility. Due to poor echogenicity and technical issues at HABC, echocardiographic data are presented for 18 to 20 participants out of 24.
Thoracic Ultrasonography
Thoracic ultrasonography has been used to show extravascular lung water accumulation at high altitude (Fagenholz et al., 2007; Wimalasena et al., 2013). In the present study, UsLC were assessed by one trained operator (GW) via transthoracic sonography performed with a portable ultrasound (CX-50, Phillips, Eindhoven, Netherlands) and using an abdominal 5–2 MHz probe (curvature 40R, field-of-view 75°) as described previously (Bouzat et al., 2013). At SL and at HABC, measurements were made after echocardiography at PEP0 (40th min) and after 15 min at PEP10 (60th min). With participants in the supine position, the 28 intercostal lung fields located at the upper, medium and lower parts of the anterior and lateral regions of the two chest walls were sequentially examined (i.e., video loop recorded for post processing). The total number of UsLC identified was checked after the expedition, from the video sequences, by another trained operator (PB) blinded for the subject identification code, the session (SL or HABC) and the condition/time point (PEP0 or PEP10). An UsLC was defined as an echogenic, coherent, wedge-shaped signal that originated from the hyperechoic pleural line (Picano et al., 2006) and extended to the edge of the screen. This ultrasound sign correlates with alveolar-interstitial oedema assessed by chest radiography, wedge pressure and extravascular lung water measured by thermodilution (Agricola et al., 2005). A number of up to 4–5 UsLC is a normal echographic chest pattern since healthy participants may present a small number of UsLC, especially confined laterally to the last intercostal spaces above the diaphragm (Picano et al., 2006). All participants were within this range at SL, only participants presenting elevated UsLC at HABC PEP0 (n = 19) were considered to assess the effect of PEP10 on UsLC at very high altitude.
Cerebral Perfusion
Middle cerebral artery blood flow velocity (MCAv) was measured at SL and HABC using a 2-MHz pulsed Doppler ultrasound system (CX-50, Phillips, Eindhoven, Netherlands). Measurements were performed after transthoracic ultrasonography at PEP0 (45th min) and after 25 min (70th min) and 45 min (90th min) at PEP10. Due to poor echogenicity and technical issues, transcranial Doppler data are presented for n = 23 at SL and n = 19 at HABC. The Doppler ultrasound probe was positioned over the right temporal window. Signal quality was optimized using an M-mode screen shot and probe location and insonation depth were marked to ensure within-subject repeatability. MCAv was used as an index of cerebral blood flow (CBF). The pulsatility index (PI), an indirect measure of cerebrovascular resistance believed to be positively influenced by intracranial pressure (ICP), was estimated from transcranial Doppler measurements as the difference in flow velocities measured during systole (sysMCAv) and diastole (diaMCAv), divided by the mean flow velocity (MCAv): PI = (diaMCAv – sysMCAv)/ MCAv (Wakerley et al., 2015). End tidal partial pressure of carbon dioxide (EtCO2) was measured and averaged during each MCAv measurement (at HABC only) from a cannula connected to the face-mask (iPM9800, Mindray, China).
Muscle and Cerebral Oxygenation
Cerebral oxygenation in the left prefrontal (PFC) cortex and muscle oxygenation from the right vastus lateralis (at mid-thigh) were assessed by monitoring changes in oxy- and deoxy- haemoglobin concentration (O2Hb and HHb, respectively) obtained with portable spatially resolved, continuous wave near-infrared spectroscopy (NIRS) (Portalites, Artinis, Zetten, Netherlands). Theoretical and performance details of NIRS have been previously described (Perrey, 2008). PFC NIRS probe was centred between Fp1 and F3 locations according to the international 10–20 EEG system. PFC and muscle probe holders (3.5 cm interoptode distance) were secured to the skin using double-sided adhesive tape and covered with black sweatbands for them to be shield from ambient light. Total haemoglobin change (THb = O2Hb+HHb) was calculated to reflect changes in tissue blood volume within the illuminated areas. Tissue saturation index (TSI, expressed in %) as an absolute measure of oxygenated-haemoglobin saturation was provided by the equipment based on spatially resolved spectroscopy (Hoshi et al., 2001). NIRS data were recorded at 10 Hz and filtered with a 2-s moving Gaussian smoothing algorithm. THb changes were expressed as relative changes (Δμmol) from the beginning of the PEP0 period and reported after 15 and 25 min of PEP10 (average over 60-s periods). Because TSI is less sensitive than Hb concentrations to movements (associated with echocardiographic and thoracic ultrasonography evaluations between 30 and 45 min of PEP10), it was reported after 15, 25, and 45 min of PEP10.
Statistics
Data are reported as means and standard deviations (SD). The statistical analyses were performed with Statistica (version 8, Tulsa, United States). Data were tested for equality of variance (Fisher-Snedecor F-test) and for normality (Shapiro-Wilk test).
The effect of altitude on dependent variables at baseline (i.e., SL versus HABC with PEP0) and the effect of PEP10 versus PEP0 (either at SL or HABC) on arterial pressure, subjective feelings, echocardiographic and transthoracic ultrasound variables were tested with parametric paired Student t-test or non-parametric Wilcoxon tests when required. Variables with several measurement time points (SpO2, HR, EtCO2, transcranial Doppler, NIRS) were analysed in each condition (SL or HABC) with one-way ANOVAs with repeated measures (time points: PEP0 and PEP10 at 15th, 25th, and 45th min). When significant main or interaction effects were found, Tukey HSD post hoc tests were used to localize differences. Null hypothesis was rejected at P < 0.05.
Results
Clinical Examination
SpO2 was decreased at HABC compared to SL (P < 0.001, Table 1) and increased with PEP10 compared to PEP0 at HABC (+8.8 to 9.4% on average depending on the time point throughout the 45 min, P < 0.001; Figure 2A). SABP tended to be increased by altitude (P = 0.087) and by PEP10 (P = 0.048 at SL and P = 0.070 at HABC). DABP and MABP were both higher at HABC compared to SL and increased with PEP10 compared to PEP0, whatever the altitude condition (all P < 0.001). PASP was increased at HABC compared to SL (P < 0.001). PEP10-induced decrease in PASP was significant at SL (P = 0.010) and did not reach significance at HABC (P = 0.086). EtCO2 did not differ between PEP0 and PEP10 at HABC (P = 0.39).
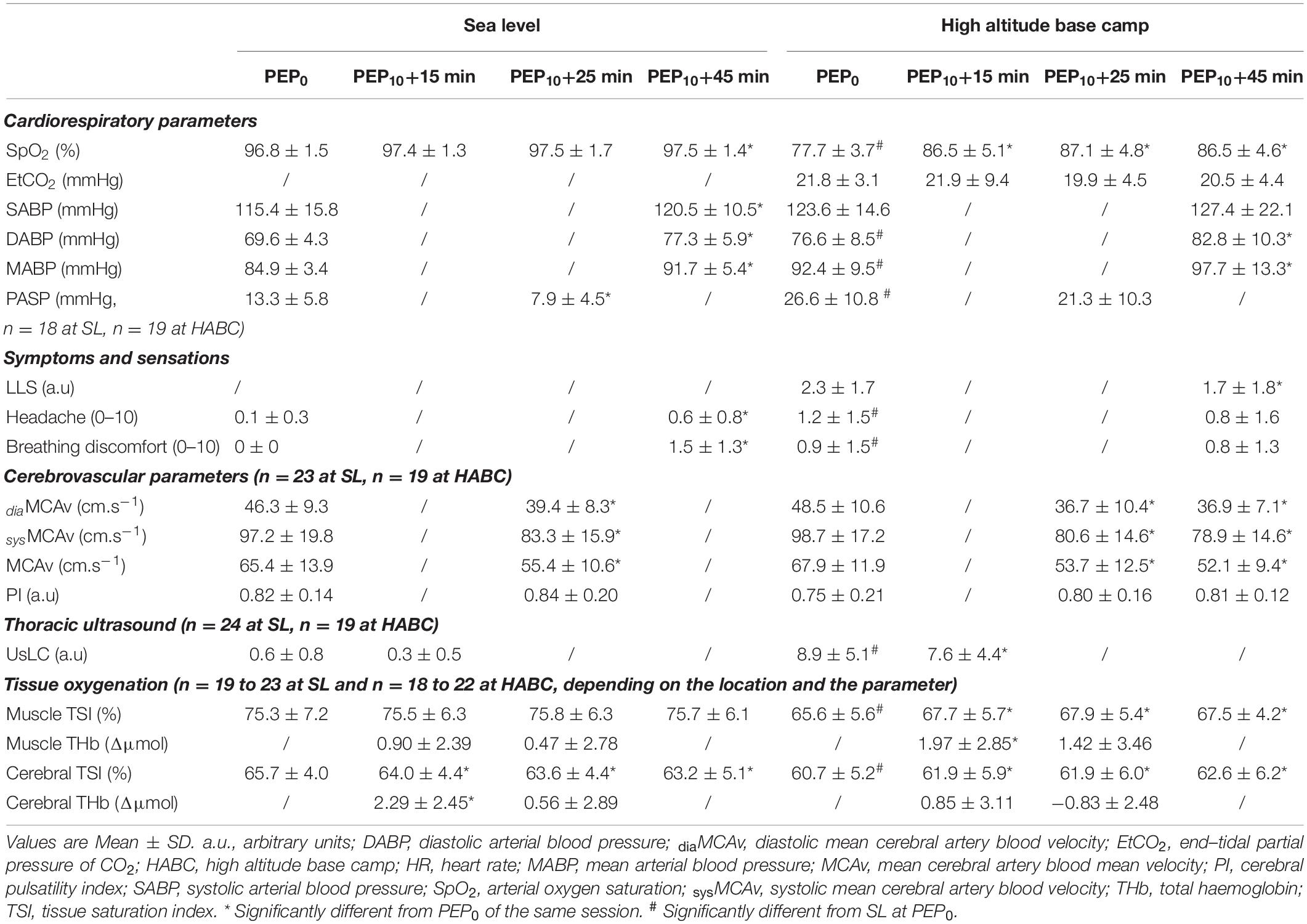
Table 1. Changes in cardiorespiratory data, subjective feelings and cerebrovascular and tissue oxygenation parameters while breathing with PEP0 and PEP10 at sea level and at high altitude base camp.
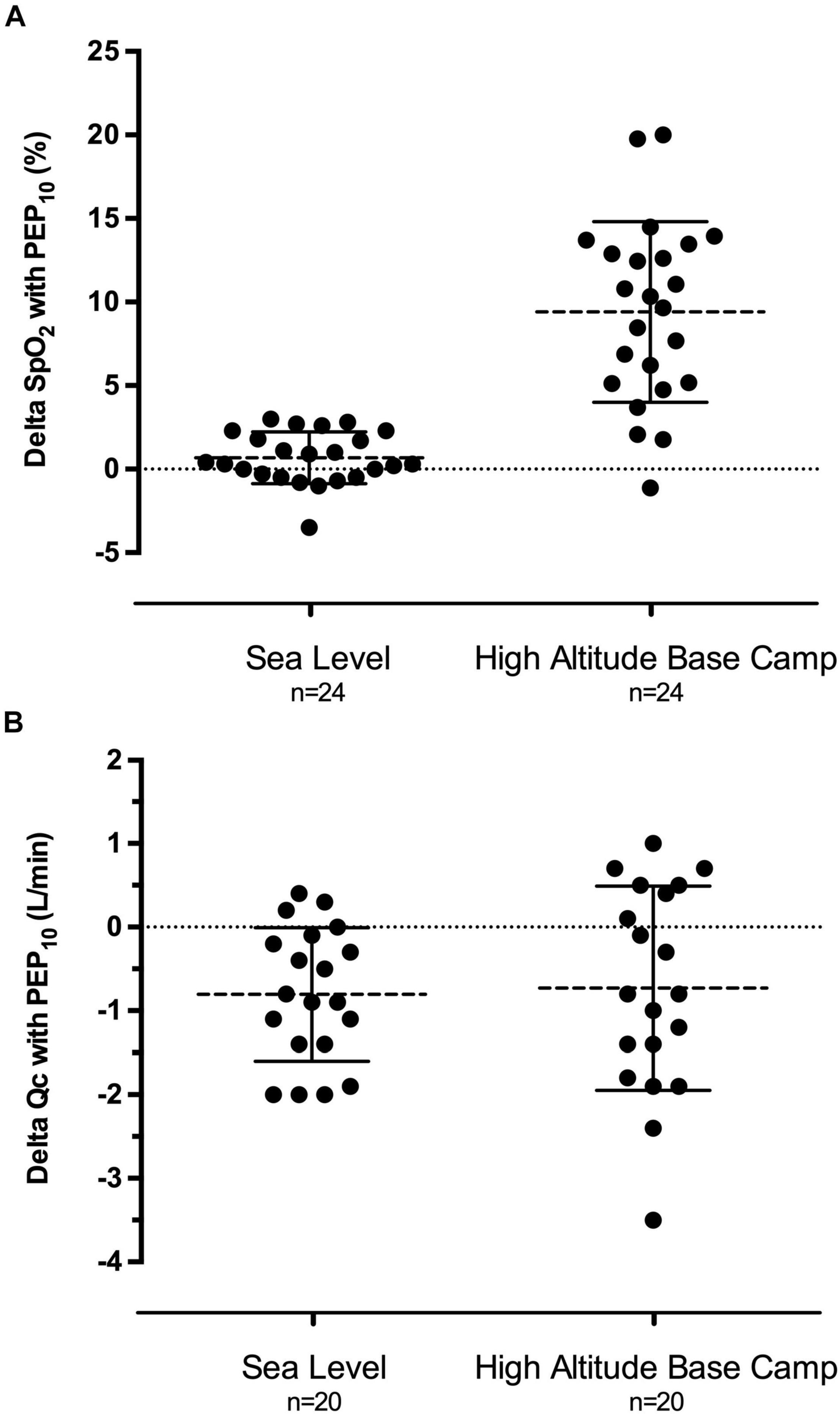
Figure 2. Individual modifications of arterial pulse oxygen saturation (A, delta SpO2) and cardiac output (B, delta Qc) induced by 25 min of PEP10 breathing compared to PEP0 at sea level in normoxia and the day after reaching high altitude base camp at 5,085 m. Mean SpO2 increase was statistically significant at high altitude (P < 0.001) and mean Qc decrease was statistically significant both at seal level (P < 0.001) and at high altitude (P = 0.015).
Headache and breathing discomfort were slightly increased with altitude (P = 0.018 and P = 0.008, respectively). At HABC, PEP10 significantly reduced LLS (from 2.3 ± 1.7 to 1.7 ± 1.8, P = 0.044) and AMS incidence (from 45 to 24% of the participants, P = 0.043) with no deleterious effect on headache (P = 0.25) and breathing comfort (P = 0.91) compared to PEP0, unlike what was observed at SL (Table 1).
Echocardiographic Data
Altitude induced significant changes in several morphological and functional cardiac parameters with PEP0 (Table 2). HR was increased at HABC compared to SL (P < 0.001) and was reduced by PEP10 compared to PEP0 only at SL (P = 0.004). PEP10 breathing at HABC induced modest but significant morphological changes, slightly decreasing RA end diastolic area (P = 0.001) and increasing LV end-diastolic diameter (P = 0.019) compared to PEP0. Transmitral filling was reduced with PEP10 both at SL and HABC (peak E, P = 0.002 and P = 0.016, respectively). LV stroke volume also decreased with PEP10 at HABC (P = 0.016), inducing a ∼9% decrease in cardiac output (P = 0.015, Figure 2B). LV tissue Doppler imaging at HABC provided evidence of a reduction in both systolic (e.g., S’, P < 0.001) and diastolic (e.g., E’, P < 0.001) function indices with PEP10, as also observed at SL. From a functional point of view, RV systolic function also appeared diminished with PEP10 compared to PEP0 at SL and HABC (e.g., decrease in Peak S’RV, P = 0.011 and P = 0.001, respectively). Multiple myocardial indices of contractility were enhanced at HABC with PEP0 compared to SL and almost all indicators showed diminished contractility with PEP10 compared to PEP0 at HABC (e.g., LV longitudinal strain and strain rate, P = 0.020 and P = 0.026, respectively).
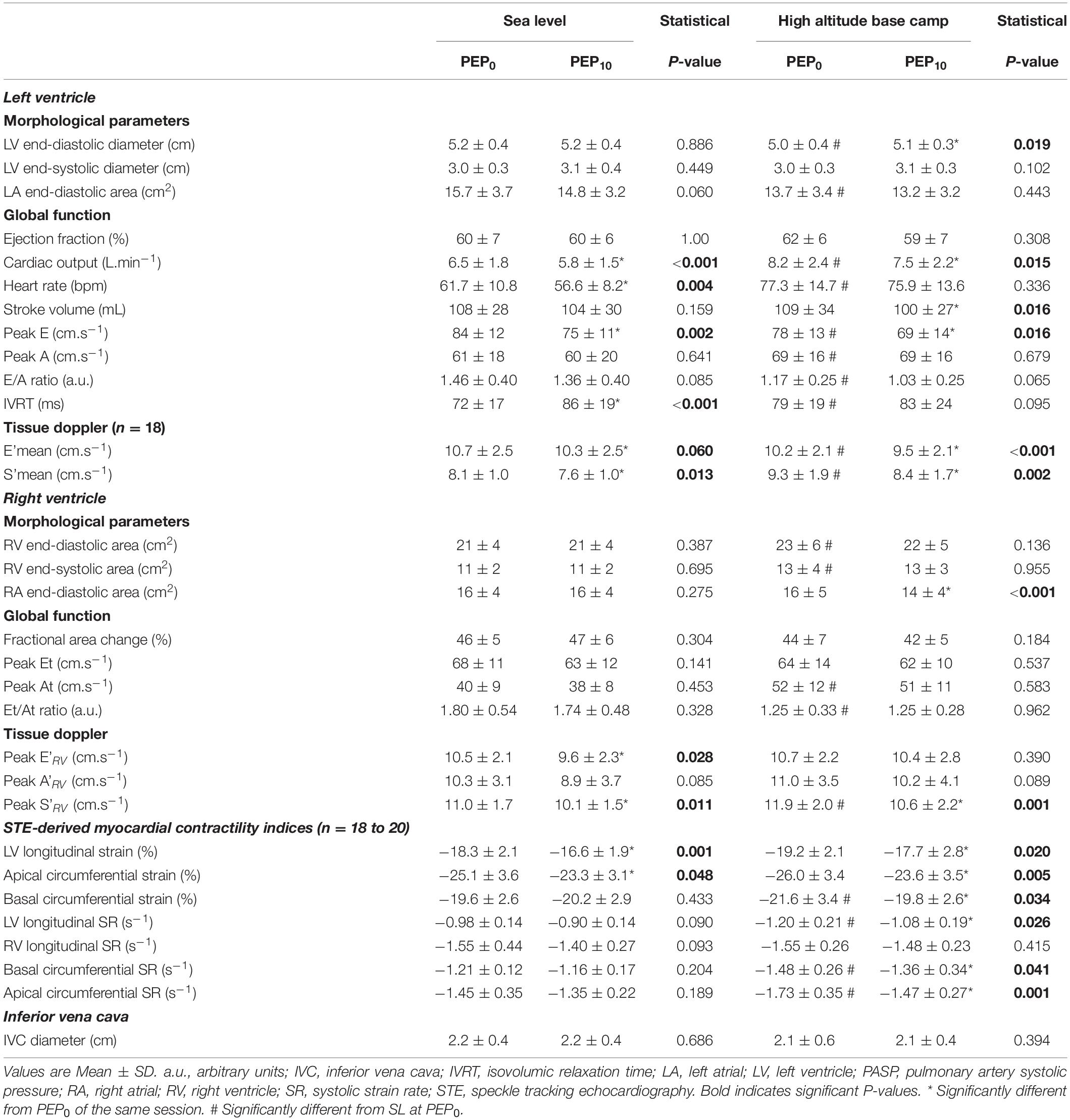
Table 2. Morphological and functional cardiac parameters while breathing with PEP0 and PEP10 at sea level and at high altitude base camp.
Thoracic Ultrasonography
The number of UsLC was significantly increased at HABC compared to SL (P < 0.001, Table 1). PEP10 breathing significantly reduced the amount of UsLC in participants presenting ≥ 4 UsLC with PEP0 at HABC (n = 19, P = 0.038).
Cerebral Perfusion
Middle cerebral artery blood flow velocity was not different with PEP0 at SL and HABC (P = 0.30, Table 1). PEP10 breathing decreased MCAv at SL and at HABC (∼−15% and −20%, respectively after 25 min, both P < 0.001; Figure 3A) compared to PEP0. PI was not affected by altitude (P = 0.18) or PEP10 breathing (P = 0.15).
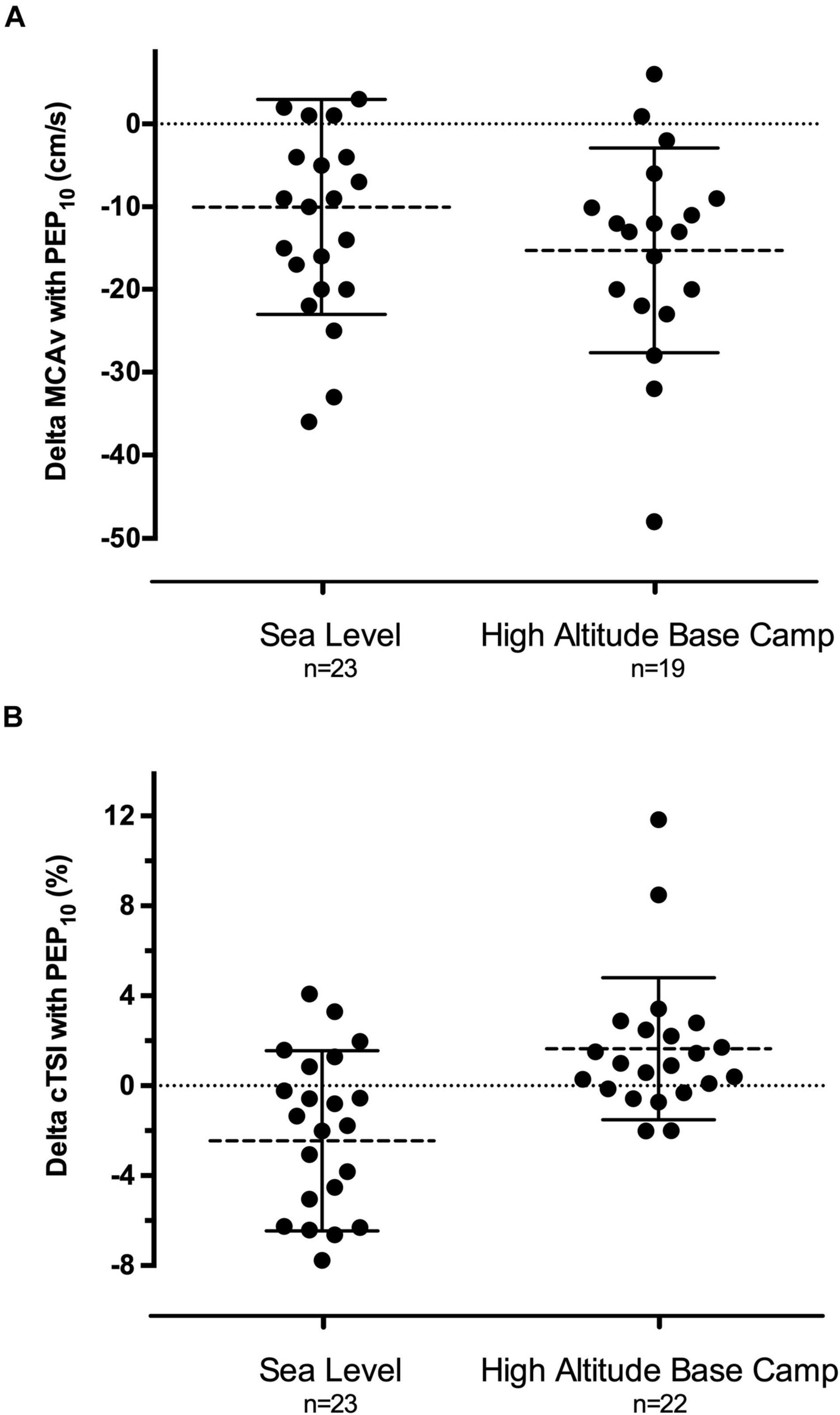
Figure 3. Individual modifications of mean cerebral artery blood velocity (A, delta MCAv) and prefrontal cortex oxygenation (B, delta cTSI) induced by 45 min of PEP10 breathing compared to PEP0 at sea level in normoxia and the day after reaching high altitude base camp at 5,085 m. Mean MCAv decrease was statistically significant both at sea level and at high altitude (both P < 0.001). Mean cTSI decrease at sea level was statistically significant (P < 0.001) and so did mean cTSI increase at high altitude (P < 0.022).
Tissue Oxygenation
Muscle TSI was lower at HABC compared to SL (PEP0: ∼−10%, P < 0.001, Table 1). PEP10 did not affect muscle TSI at SL but significantly increased it compared to PEP0 at HABC (+1.9 to 2.3% on average depending on the time point throughout the 45 min, P = 0.003). Muscle THb was slightly enhanced with PEP10 at HABC (P = 0.008) but not at SL.
Cerebral TSI was lower at HABC compared to SL (PEP0: ∼−5%, P = 0.001). PEP10 decreased cerebral TSI at SL (−1.7 to −2.5% on average depending on the time point throughout the 45 min, P < 0.001) while it increased it at HABC (+1.2 to +1.9%, P = 0.022, Figure 3B). Cerebral THb was slightly enhanced after 15 min of PEP10 compared to PEP0 at SL (P = 0.001) but not at HABC.
Discussion
The main findings of this study were that PEP10 breathing in trekkers reaching very high altitude (5,085 m) after 18 days of ascent substantially increased SpO2 (∼+9%) as well as muscle and cerebral oxygenation (both ∼+2%). These positive effects on arterial and tissue oxygenation were concomitant with a significant decrease in interstitial lung fluid accumulation and AMS symptoms and a reduction in cardiac output and cerebral blood flow.
Arterial Oxygenation With PEP10 Breathing
To our knowledge only two studies explored the effect of positive airway pressure at very high altitude (>4,500 m) (Launay et al., 2004; Agostoni et al., 2010) and only one was designed to explore its effectiveness in trekkers after prolonged altitude exposure. In this context, Agostoni et al. (2010) did not show any change in SpO2 after 30 min of CPAP at 7 cmH2O (administered with a standard electrical ventilatory device used for sleep apnoea treatment) after 10 days of acclimatization at 5,400 m. The authors concluded that CPAP is not useful at altitude after acclimatization (i.e., disappearance of AMS symptoms together with SpO2 improvement) but did not provide any information about the effectiveness of the intervention within 24–72 h after arrival at high altitude, despite this time frame being critical in terms of AMS and HAPE occurrence (Hackett and Roach, 2001; Bärtsch et al., 2005).
In the present study, the day after reaching >5,000 m, the mean increase of ∼9% in SpO2 with PEP10 breathing was highly significant and exceeds the effect we have previously reported at lower altitude (+7% SpO2 with PEP10 breathing after 2–3 days at 4,350 m) (Nespoulet et al., 2013). Loeppky et al. (2008) demonstrated that participants developing AMS exhibit larger arterial desaturation at altitude, i.e., 4–5% lower SpO2 at 4,880 m compared to participants without symptoms. Other authors confirmed that this range of significant difference is also seen for altitude as low as 2,300 m (Burtscher et al., 2004). Therefore, the large effect of PEP10 breathing on SpO2 observed throughout the 45 min of application in the present study is likely to be clinically relevant. In addition, compared to electronic medical device such as CPAP ventilators, PEP breathing appears to be of special interest as a well-tolerated lightweight, non-electronic and non-pharmacologic solution to improve oxygenation in such hypoxically challenging environment.
One may wonder whether part of the increase in SpO2 could result from slightly higher minute ventilation during PEP10 breathing, as we did not assess minute ventilation in this study. However, previous studies demonstrated that mechanisms other than changes in ventilation are responsible for the PEP-induced improvement in arterial oxygenation in healthy hypoxic (Nespoulet et al., 2013) or HAPE participants (Schoene et al., 1985). Furthermore the similar EtCO2 with PEP0 and PEP10 at HABC indicated the absence of significant hyperventilation with PEP10. Improved SpO2 during PEP10 breathing at altitude may result at least in part from an increased alveoli-capillary gradient and improved ventilation in lung regions with low ventilation/perfusion ratio (e.g., improved ventilation of hitherto collapsed, fluid-filled or poorly ventilated alveoli enlarging gas exchange surface), although this remains to be demonstrated. Alveolar-to-arterial PO2 gradients alterations with the use of PEP breathing at altitude clearly deserve to be further investigated.
High altitude pulmonary oedema is a hypoxemia-dependent altitude sickness that may affect 0.2% to >15% of individuals reaching 2,500 to 5,500 m depending on the ascent profile (Luks et al., 2017). Silent high altitude interstitial pulmonary oedema is likely even more frequent in climbers reaching high altitude (Pratali et al., 2010; Bouzat et al., 2013) and, although debated, has been proposed to be a clinically relevant marker of individual vulnerability to HAPE (Wimalasena et al., 2013; Pratali, 2018). In the present study, we confirmed that the majority of the participants presented clear ultrasonographic signs of extravascular lung water accumulation the day after arrival at HABC (cf. Table 1). Moreover, we showed for the first time that among participants with abnormal lung patterns (i.e., UsLC ≥ 4), PEP10 breathing for only 15 min already significantly reduces the amount of UsLC and may therefore resorb at least part of the pulmonary extravascular fluid accumulation. Whether this may result in improved alveolar-arterial oxygen diffusion and therefore contribute to a virtuous circle promoting increased SpO2 remains to be determined. The effect of PEP10 breathing needs also to be evaluated in climbers exhibiting larger amount of UsLC and more severe pulmonary extravascular fluid accumulation, as for example in case of more rapid ascent to high altitude.
Muscle Oxygenation and Cardiac Adaptations With PEP10 Breathing
In accordance with our previous observation at lower simulated (Nespoulet et al., 2013; Rupp et al., 2019) or real (Rupp et al., 2019) altitude, muscle oxygenation (i.e., TSI, Table 1) was improved by PEP10 breathing at HABC. Although modest, the concomitant increase in muscle THb suggests this tissue oxygenation improvement may be at least in part associated with an increased muscle blood volume. A weakened venous return due to the increased intrathoracic pressure during PEP breathing (Luecke and Pelosi, 2005) could be responsible for a slight increase in blood volume in the lower limbs. Although such slight peripheral venous stasis is in accordance with the literature underlining macrohemodynamic side effects of PEEP (Luecke and Pelosi, 2005), our echocardiographic data do not suggest that venous return was deleteriously affected with PEP10 at HABC. Sonographic measurement of inferior vena cava diameter is a valid method of estimating central venous pressure and RA pressure (Ciozda et al., 2016), but did not reveal changes with PEP10 breathing in our study. Also, despite a slight decrease in RA end-diastolic area with PEP10 breathing at HABC, RV filling (cf. Peak Et and Peak At, Table 2) and end-diastolic area were preserved, supporting that RV preload was indeed not affected in the context of our setup. One may speculate that together with a reduced number of UsLC, PEP10 at HABC could have been prone to reduce pulmonary vascular resistance; this is supported by the trend toward facilitated RV afterload (i.e., lower PASP, increased LV EDD) but needs to be confirmed in the future. As often shown in the context of CPAP or PEEP, cardiac output was depressed with PEP10 at HABC, but with no concomitant hypotension here. We discussed above that this reduction is not triggered by a reduced preload to the heart and Table 2 provides evidence that the diminished stroke volume is rather consecutive to an increase in arterial peripheral resistance, as suggested by the enhanced mean arterial pressure with PEP10 at HABC. Interestingly, speckle tracking echocardiography-derived parameters also suggest that part of this depressed stroke volume might be the consequence of a decrease in myocardial contractility, the latter remaining, however, in the range of normal sea level values (Table 2). An explanation may come from the particular way of breathing with a PEP (i.e., slow pace and longer expiration phase) and the fact that during prolonged expiratory breathing, parasympathetic nervous function is known to be activated (Komori, 2018). An activation of parasympathetic tone induces a negative inotropic effect (Lewis et al., 2001) so that the modulation of autonomic nervous system with PEP10 could explain the decrease in SR observed both at sea level (although not significant) and at base camp. The absence of major cardiovascular impairment (according to arterial blood pressure and biventricular evaluation) during PEP10 breathing at high altitude suggests that PEP may have advantages over CPAP and PEEP in this context.
Cerebral Hemodynamics and Oxygenation With PEP10 Breathing
Ventilation strategies involving positive airway pressure, as commonly used in patients with acute respiratory distress syndrome, are concomitantly potentially increasing the risk of intracranial hypertension and undesirable effects on cerebral function. These are likely caused by impeded cerebral venous return, decreased mean arterial pressure, cerebral perfusion pressure and CBF (Scala et al., 2003). Therefore we aimed to assess the effect of PEP breathing on cerebral hemodynamics and oxygenation, which can already be significantly impaired by altitude exposure and contribute to functional impairment and increased risk of cerebral (sub)oedema (Wilson et al., 2009; Verges et al., 2012; Luks et al., 2017). Our results show for the first time a significant decrease in MCAv with PEP10 breathing at very high altitude. This effect was also observed at SL (Table 1) and confirms what we recently reported in acute moderate hypoxia (simulated and real altitude of 3450 m) (Rupp et al., 2019). Since EtCO2 did not change during PEP10 breathing at HABC, this decrease was unlikely the consequence of hypocapnia and subsequent cerebral arteriole constriction (Markwalder et al., 1984). Because MCAv was reduced by PEP10 breathing both at SL and at HABC, whilst arterial oxygenation was enhanced at altitude only, other mechanisms than the change in arterial oxygenation are probably responsible for the reduction in MCAv. At HABC, however, the substantial increase in arterial oxygenation with PEP10 may allow CBF (as well as cardiac output) to decrease while tissue oxygen delivery would be maintained or even enhanced as shown by muscle and cerebral TSI (e.g., ∼40% of the altitude-induced cerebral deoxygenation is reversed with PEP10 at HABC, Table 1). It should be emphasized that MCAv is a measure of blood velocity, not flow, so it is a reliable index of CBF when assuming a constant MCA diameter. The literature still questions the assumption of a constant MCA diameter within a wide range of EtCO2 and hypoxic conditions (Poulin and Robbins, 1996). This might be the case at low to high altitude but not at very high altitude (>5,000 m) (Willie et al., 2014) or when oxygen is administered (Wilson et al., 2011). Whether MCA diameter changes with PEP10 breathing at high altitude was not assessed in the current study and needs further investigation.
Theoretically, high levels of intrathoracic pressure (e.g., with CPAP or PEEP > 15 cmH2O) can increase the ICP through a reduced cerebral venous outflow (i.e., increased central venous pressure and cerebral blood volume). The TCD-derived pulsatility index (PI) is an indirect index of vascular resistance and is believed to be positively influenced by ICP. Even if the strength of this relationship remains contentious and varies between studies (Wakerley et al., 2015), a PI value between 0.8 and 1 is widely considered as “normal” and likely to exclude the presence of exaggerated ICP. Hence, PI values in the present study do not suggest a clinically relevant increase in ICP due to PEP10 breathing as previously hypothesized (Oelz, 1983). The lack of increase in cerebral THb during PEP10 breathing at HABC reinforces the idea that PEP is unlikely to promote an increase in cerebral blood volume due to impaired venous return in the context of our study.
Acute Mountain Sickness and PEP10 Breathing
Interestingly, symptoms of AMS were significantly decreased after 45 min of PEP10 breathing at HABC. The amount of participants having a LLS ≥ 3 (i.e., a positive AMS diagnosis) was also significantly reduced after PEP10 breathing. This reduction in AMS symptoms is in accordance with the significant improvement in arterial oxygenation associated with PEP10. Additionally, the decrease in symptoms may relate to the reduction in MCAv. A widespread traditional paradigm indeed considers AMS symptoms are primarily driven by ICP (Hackett and Roach, 2001; Wilson et al., 2009) and one would expect the substantial decrease in MCA flow observed herein to reduce pulsatile ICP, although only a «gold standard» measure of ICP could confirm this assumption.
It should be acknowledged, however, that the mean LLS score was relatively low in this group of trekkers having reached 5,085 m within 18 days allowing progressive acclimatization. Future studies should evaluate the effect of PEP10 breathing on more symptomatic participants in order to establish whether PEP breathing could be considered as a useful tool also for moderate to severe AMS management. Despite PEP breathing with 10 cmH2O requiring some respiratory effort which might be unpleasant at high altitude, especially for trekkers presenting symptoms such as headache or dizziness, PEP10 breathing was well tolerated in the present study, as shown for example by the lack of increase in breathing discomfort compared to PEP0 at HABC.
Conclusion
This study demonstrates in the context of a trek to very high altitude (as commonly performed by an increasing number of people worldwide) that breathing with PEP10 significantly increases arterial, muscle and cerebral oxygenation and decreases interstitial lung fluid accumulation and AMS symptoms. PEP10 breathing is well tolerated by trekkers and does not induce deleterious cardiac or cerebral consequences at least when applied for 45 min. Hence, PEP breathing appears to be a useful tool able to improve oxygenation and symptoms at rest at high altitude. As a perspective, development of individualized devices based on this principle may contribute to widespread alternative/complementary clinical practice regarding mild altitude illness, and future research should assess whether PEP breathing may also be relevant during walking/trekking on the one hand and in participants experiencing more severe AMS on the other hand.
Physiological Relevance
Ascent to very high altitude induces a critical reduction in human arterial saturation that put individuals at risk of developing acute mountain sickness (AMS), high-altitude pulmonary or cerebral oedema. As a non-pharmacological countermeasure to hypoxemia, breathing with positive expiratory resistance (PEP) has been shown to quickly increase arterial oxygenation during acute hypoxic exposure, due to an increased intrathoracic pressure facilitating pulmonary gas exchanges. Cardiovascular and cerebral side effects of PEP breathing have, however, been reported at sea level and remain unknown in individuals trekking to high altitude (as commonly performed by an increasing number of people worldwide). Following a 18-day trek to 5,085 m, we found that PEP breathing increased participants’ arterial, muscle and cerebral oxygenation and decreased symptoms of AMS and subclinical signs of pulmonary oedema, with no deleterious cardiac or cerebral consequences. PEP breathing may be a relevant and safe alternative/complementary clinical practice to manage AMS in this context of extreme environment.
Data Availability Statement
The raw data supporting the conclusions of this article will be made available by the authors, without undue reservation.
Ethics Statement
The studies involving human participants were reviewed and approved by the National Institute for Social Care and Health Research Wales Research Ethics Service. The patients/participants provided their written informed consent to participate in this study.
Author Contributions
TR: study design, data collection, data analysis, intellectual contribution, first draft of manuscript, and manuscript editing. CM and GW: study design, data collection, data analysis, intellectual contribution, and manuscript editing. JM: expedition organizer, ethical clearance, intellectual contribution, and manuscript editing. PB: data collection, data analysis, and manuscript editing. FE: data collection and manuscript editing. SV: study design, research coordinator, data collection, intellectual contribution, and manuscript editing. All authors approved the final version of the manuscript, agreed to be accountable for all aspects of the work in ensuring that questions related to the accuracy or integrity of any part of the work are appropriately investigated and resolved, all persons designated as authors qualify for authorship, and all those who qualify for authorship are listed.
Funding
This study was supported by (a) University Savoie Mont Blanc, France (TR), (b) Grenoble Alpes University and the French National Institute for Health and Medical Research (Inserm; SV), (c) the Petzl Foundation.
Conflict of Interest
The authors declare that the research was conducted in the absence of any commercial or financial relationships that could be construed as a potential conflict of interest.
Publisher’s Note
All claims expressed in this article are solely those of the authors and do not necessarily represent those of their affiliated organizations, or those of the publisher, the editors and the reviewers. Any product that may be evaluated in this article, or claim that may be made by its manufacturer, is not guaranteed or endorsed by the publisher.
Acknowledgments
MEDEX Manaslu 2015 research expedition comprised of many persons who without their help the study would not have been possible. In particular, the authors would like to thank the expedition organizers and volunteers who participated in this research, and also Denzil Broadhurst and Nepalese mountain guides for their technical assistance.
References
Agostoni, P., Caldara, G., Bussotti, M., Revera, M., Valentini, M., Gregorini, F., et al. (2010). Continuous positive airway pressure increases haemoglobin O2 saturation after acute but not prolonged altitude exposure. Eur. Heart J. 31, 457–463. doi: 10.1093/eurheartj/ehp472
Agostoni, P., Swenson, E. R., Fumagalli, R., Salvioni, E., Cattadori, G., Farina, S., et al. (2013). Acute high-altitude exposure reduces lung diffusion: data from the HIGHCARE Alps project. Respir. Physiol. Neurobiol. 188, 223–228. doi: 10.1016/j.resp.2013.04.005
Agricola, E., Bove, T., Oppizzi, M., Marino, G., Zangrillo, A., Margonato, A., et al. (2005). “Ultrasound comet-tail images”: a marker of pulmonary edema–a comparative study with wedge pressure and extravascular lung water. Chest 127, 1690–1695. doi: 10.1378/chest.127.5.1690
Bailey, D. M., Bärtsch, P., Knauth, M., and Baumgartner, R. W. (2009). Emerging concepts in acute mountain sickness and high-altitude cerebral edema: from the molecular to the morphological. Cell. Mol. Life Sci. CMLS 66, 3583–3594. doi: 10.1007/s00018-009-0145-9
Bärtsch, P., Mairbäurl, H., Maggiorini, M., and Swenson, E. R. (2005). Physiological aspects of high-altitude pulmonary edema. J. Appl. Physiol.(1985) 98, 1101–1110. doi: 10.1152/japplphysiol.01167.2004
Bouzat, P., Walther, G., Rupp, T., Doucende, G., Payen, J.-F., Levy, P., et al. (2013). Time course of asymptomatic interstitial pulmonary oedema at high altitude. Respir. Physiol. Neurobiol. 186, 16–21. doi: 10.1016/j.resp.2012.12.009
Burtscher, M., Flatz, M., and Faulhaber, M. (2004). Prediction of susceptibility to acute mountain sickness by SaO2 values during short-term exposure to hypoxia. High Alt. Med. Biol. 5, 335–340.
Ciozda, W., Kedan, I., Kehl, D. W., Zimmer, R., Khandwalla, R., and Kimchi, A. (2016). The efficacy of sonographic measurement of inferior vena cava diameter as an estimate of central venous pressure. Cardiovasc. Ultrasound 14:13. doi: 10.1186/s12947-016-0076-1
Di Marco, F., Devaquet, J., Lyazidi, A., Galia, F., da Costa, N. P., Fumagalli, R., et al. (2010). Positive end-expiratory pressure-induced functional recruitment in patients with acute respiratory distress syndrome. Crit. Care Med. 38, 127–132. doi: 10.1097/CCM.0b013e3181b4a7e7
Duncan, A. W., Oh, T. E., and Hillman, D. R. (1986). PEEP and CPAP. Anaesth. Intensive Care 14, 236–250.
Fagenholz, P. J., Gutman, J. A., Murray, A. F., Noble, V. E., Thomas, S. H., and Harris, N. S. (2007). Chest ultrasonography for the diagnosis and monitoring of high-altitude pulmonary edema. Chest 131, 1013–1018. doi: 10.1378/chest.06-1864
Hackett, P. H., and Roach, R. C. (2001). High-altitude illness. N. Engl. J. Med. 345, 107–114. doi: 10.1056/NEJM200107123450206
Hoshi, Y., Kobayashi, N., and Tamura, M. (2001). Interpretation of near-infrared spectroscopy signals: a study with a newly developed perfused rat brain model. J. Appl. Physiol. 90, 1657–1662.
Korzeniewski, K., Nitsch-Osuch, A., Guzek, A., and Juszczak, D. (2015). High altitude pulmonary edema in mountain climbers. Respir. Physiol. Neurobiol. 209, 33–38. doi: 10.1016/j.resp.2014.09.023
Launay, J. C., Nespoulos, O., Guinet-Lebreton, A., Besnard, Y., and Savourey, G. (2004). Prevention of acute mountain sickness by low positive end-expiratory pressure in field conditions. Scand. J. Work Environ. Health 30, 322–326.
Lewis, M., Al-Khalidi, A., Bonser, R., Clutton-Brock, T., Morton, D., Paterson, D., et al. (2001). Vagus nerve stimulation decreases left ventricular contractility in vivo in the human and pig heart. J. Physiol. 534(Pt 2) 547–552.
Lipman, G. S., Kanaan, N. C., Phillips, C., Pomeranz, D., Cain, P., Fontes, K., et al. (2015). Study looking at end expiratory pressure for altitude illness decrease (SLEEP-AID). High Alt. Med. Biol. 16, 154–161. doi: 10.1089/ham.2014.1110
Loeppky, J. A., Icenogle, M. V., Charlton, G. A., Conn, C. A., Maes, D., Riboni, K., et al. (2008). Hypoxemia and acute mountain sickness: which comes first? High Alt. Med. Biol. 9, 271–279. doi: 10.1089/ham.2008.1035
Luecke, T., and Pelosi, P. (2005). Clinical review: positive end-expiratory pressure and cardiac output. Crit. Care 9, 607–621. doi: 10.1186/cc3877
Luks, A. M., Swenson, E. R., and Bärtsch, P. (2017). Acute high-altitude sickness. Eur. Respir. Rev. 26:160096. doi: 10.1183/16000617.0096-2016
Markwalder, T. M., Grolimund, P., Seiler, R. W., Roth, F., and Aaslid, R. (1984). Dependency of blood flow velocity in the middle cerebral artery on end-tidal carbon dioxide partial pressure–a transcranial ultrasound Doppler study. J. Cereb. Blood Flow Metab. 4, 368–372. doi: 10.1038/jcbfm.1984.54
Maufrais, C., Rupp, T., Bouzat, P., Doucende, G., Verges, S., Nottin, S., et al. (2017). Heart mechanics at high altitude: 6 days on the top of Europe. Eur. Heart J. Cardiovasc. Imaging 18, 1369–1377. doi: 10.1093/ehjci/jew286
Muench, E., Bauhuf, C., Roth, H., Horn, P., Phillips, M., Marquetant, N., et al. (2005). Effects of positive end-expiratory pressure on regional cerebral blood flow, intracranial pressure, and brain tissue oxygenation. Crit. Care Med. 33, 2367–2372. doi: 10.1097/01.CCM.0000181732.37319.DF
Nespoulet, H., Rupp, T., Bachasson, D., Tamisier, R., Wuyam, B., Lev́y, P., et al. (2013). Positive expiratory pressure improves oxygenation in healthy subjects exposed to hypoxia. PLoS One 8:e85219. doi: 10.1371/journal.pone.0085219
Oelz, O. (1983). High altitude cerebral oedema after positive airway pressure breathing at high altitude. Lancet 2:1148.
Perrey, S. (2008). Non-invasive NIR spectroscopy of human brain function during exercise. Methods 45, 289–299. doi: 10.1016/j.ymeth.2008.04.005
Picano, E., Frassi, F., Agricola, E., Gligorova, S., Gargani, L., and Mottola, G. (2006). Ultrasound lung comets: a clinically useful sign of extravascular lung water. J. Am. Soc. Echocardiogr. 19, 356–363. doi: 10.1016/j.echo.2005.05.019
Poulin, M. J., and Robbins, P. A. (1996). Indexes of flow and cross-sectional area of the middle cerebral artery using doppler ultrasound during hypoxia and hypercapnia in humans. Stroke 27, 2244–2250.
Pratali, L. (2018). Right heart-pulmonary circulation at high altitude and the development of subclinical pulmonary interstitial edema. Heart Fail. Clin. 14, 333–337. doi: 10.1016/j.hfc.2018.02.008
Pratali, L., Cavana, M., Sicari, R., and Picano, E. (2010). Frequent subclinical high-altitude pulmonary edema detected by chest sonography as ultrasound lung comets in recreational climbers. Crit. Care Med. 38, 1818–1823. doi: 10.1097/CCM.0b013e3181e8ae0e
Rupp, T., Saugy, J. J., Bourdillon, N., Verges, S., and Millet, G. P. (2019). Positive expiratory pressure improves arterial and cerebral oxygenation in acute normobaric and hypobaric hypoxia. Am. J. Physiol. Regul. Integr. Comp. Physiol. 317, R754–R762. doi: 10.1152/ajpregu.00025.2019
Savourey, G., Caterini, R., Launay, J. C., Guinet, A., Besnard, Y., Hanniquet, A. M., et al. (1998). Positive end expiratory pressure as a method for preventing acute mountain sickness. Eur. J. Appl. Physiol. Occup. Physiol. 77, 32–36.
Scala, R., Turkington, P. M., Wanklyn, P., Bamford, J., and Elliott, M. W. (2003). Effects of incremental levels of continuous positive airway pressure on cerebral blood flow velocity in healthy adult humans. Clin. Sci. 104, 633–639. doi: 10.1042/CS20020305
Schoene, R. B., Roach, R. C., Hackett, P. H., Harrison, G., and Mills, W. J. (1985). High altitude pulmonary edema and exercise at 4,400 meters on Mount McKinley. Effect of expiratory positive airway pressure. Chest 87, 330–333. doi: 10.1378/chest.87.3.330
Sutherland, A., Freer, J., Evans, L., Dolci, A., Crotti, M., and Macdonald, J. H. (2017). MEDEX 2015: heart rate variability predicts development of acute mountain sickness. High Alt. Med. Biol. 18, 199–208. doi: 10.1089/ham.2016.0145
Tannheimer, M., Tannheimer, S., Thomas, A., Engelhardt, M., and Schmidt, R. (2009). Auto-PEEP in the therapy of AMS in one person at 4,330 m. Sleep Breath. 13, 195–199. doi: 10.1007/s11325-008-0237-z
Verges, S., Rupp, T., Jubeau, M., Wuyam, B., Esteve, F., Levy, P., et al. (2012). Invited review: cerebral perturbations during exercise in hypoxia. Am. J. Physiol. Regul. Integr. Comp. Physiol. 302, R903–R916. doi: 10.1152/ajpregu.00555.2011
Wakerley, B. R., Kusuma, Y., Yeo, L. L. L., Liang, S., Kumar, K., Sharma, A. K., et al. (2015). Usefulness of transcranial doppler-derived cerebral hemodynamic parameters in the noninvasive assessment of intracranial pressure. J. Neuroimaging 25, 111–116. doi: 10.1111/jon.12100
Wang, C., Wang, X., Chi, C., Guo, L., Guo, L., Zhao, N., et al. (2016). Lung ventilation strategies for acute respiratory distress syndrome: a systematic review and network meta-analysis. Sci. Rep. 6:22855. doi: 10.1038/srep22855
Willie, C. K., Smith, K. J., Day, T. A., Ray, L. A., Lewis, N. C. S., Bakker, A., et al. (2014). Regional cerebral blood flow in humans at high altitude: gradual ascent and 2 wk at 5,050 m. J. Appl. Physiol. 116, 905–910. doi: 10.1152/japplphysiol.00594.2013
Wilson, M. H., Edsell, M. E. G., Davagnanam, I., Hirani, S. P., Martin, D. S., Levett, D. Z. H., et al. (2011). Cerebral artery dilatation maintains cerebral oxygenation at extreme altitude and in acute hypoxia: an ultrasound and MRI study. J. Cereb. Blood Flow Metab. 31, 2019–2029. doi: 10.1038/jcbfm.2011.81
Wilson, M. H., Newman, S., and Imray, C. H. (2009). The cerebral effects of ascent to high altitudes. Lancet Neurol. 8, 175–191. doi: 10.1016/S1474-4422(09)70014-6
Keywords: altitude illness, cardiac function, cerebral perfusion, PEP breathing, tissue oxygenation, extreme environment, medical expedition, hypoxia
Citation: Rupp T, Maufrais C, Walther G, Esteve F, Macdonald JH, Bouzat P and Verges S (2021) MEDEX 2015: Prophylactic Effects of Positive Expiratory Pressure in Trekkers at Very High Altitude. Front. Physiol. 12:710622. doi: 10.3389/fphys.2021.710622
Received: 16 May 2021; Accepted: 31 August 2021;
Published: 21 September 2021.
Edited by:
David Cristóbal Andrade, University of Antofagasta, ChileReviewed by:
Carlo Capelli, University of Verona, ItalyRodrigo L. Castillo, University of Chile, Chile
Copyright © 2021 Rupp, Maufrais, Walther, Esteve, Macdonald, Bouzat and Verges. This is an open-access article distributed under the terms of the Creative Commons Attribution License (CC BY). The use, distribution or reproduction in other forums is permitted, provided the original author(s) and the copyright owner(s) are credited and that the original publication in this journal is cited, in accordance with accepted academic practice. No use, distribution or reproduction is permitted which does not comply with these terms.
*Correspondence: Thomas Rupp, thomas.rupp@univ-smb.fr