- 1Department of Biomedical Engineering, Toyo University, Kawagoe, Japan
- 2Research Fellow of Japan Society for the Promotion of Science, Tokyo, Japan
- 3Neurovascular Research Laboratory, University of South Wales, Pontypridd, United Kingdom
Cerebrovascular reactivity (CVR) to changes in the partial pressure of arterial carbon dioxide (PaCO2) is an important mechanism that maintains CO2 or pH homeostasis in the brain. To what extent this is influenced by gravitational stress and corresponding implications for the regulation of cerebral blood flow (CBF) remain unclear. The present study examined the onset responses of pulmonary ventilation (V̇E) and anterior middle (MCA) and posterior (PCA) cerebral artery mean blood velocity (Vmean) responses to acute hypercapnia (5% CO2) to infer dynamic changes in the central respiratory chemoreflex and cerebrovascular reactivity (CVR), in supine and 50° head-up tilt (HUT) positions. Each onset response was evaluated using a single-exponential regression model consisting of the response time latency [CO2-response delay (t0)] and time constant (τ). Onset response of V̇E and PCA Vmean to changes in CO2 was unchanged during 50° HUT compared with supine (τ: V̇E, p = 0.707; PCA Vmean, p = 0.071 vs. supine) but the MCA Vmean onset response was faster during supine than during 50° HUT (τ: p = 0.003 vs. supine). These data indicate that gravitational stress selectively impaired dynamic CVR in the anterior cerebral circulation, whereas the posterior circulation was preserved, independent of any changes to the central respiratory chemoreflex. Collectively, our findings highlight the regional heterogeneity underlying CBF regulation that may have translational implications for the microgravity (and hypercapnia) associated with deep-space flight notwithstanding terrestrial orthostatic diseases that have been linked to accelerated cognitive decline and neurodegeneration.
Introduction
Numerous enzymatic and ion channels that influence neural activity are modulated by changes in pH (Chesler, 2003). Since the regulation of carbon dioxide (CO2) helps maintain constant pH (Ogoh, 2019), cerebrovascular CO2 reactivity (CVR), an indicator of the compensatory dilatory capacity of blood flow in the brain in response to vasoactive stimuli, plays an important role in cerebral CO2 regulation in order to preserve and maintain stable brain pH. Importantly, a reduction in CVR is associated with impaired cognition in patients with Alzheimer’s disease (Silvestrini et al., 2006; Richiardi et al., 2015), dementia (Silvestrini et al., 2006; Lee et al., 2007), and cerebrovascular endothelial dysfunction (Lavi et al., 2006; Kim et al., 2021), indirectly supporting a “pH-sensitive” regulatory role. Equally, the cerebrovascular responses to altered brain pH also impact central chemoreflex sensitivity (Siesjo, 1972). Since intravascular CO2 concentration influences the CO2 concentration gradient from the brain, the central respiratory chemoreflex, as well as CVR, collectively contributes to maintaining stable brain pH (Ainslie and Duffin, 2009; Ogoh, 2019). Indeed, our previous studies (Ogoh et al., 2008, 2009, 2013) demonstrated that CVR interacts with the central respiratory chemoreflex to maintain cerebral CO2 homeostasis. These data collectively justify the need to better phenotype the functional interaction between the central respiratory chemoreflex and CVR modulation.
This pathway is especially relevant during the microgravity of space since astronauts need to adapt to multiple environmental stressors including hypercapnia, hypoxia, and physical deconditioning, notwithstanding the endogenous challenges posed by pronounced cephalad fluid shifts (Bailey et al., 2021). Our previous study (Ogoh et al., 2013) demonstrated that compared to supine, orthostatic stress-induced reduction in cerebral blood flow (CBF) attenuated the “washout” of CO2 from the brain causing hyperpnea subsequent to autochemoactivation of the central chemoreflex. This finding indicates that gravitational stress (microgravity) modified CO2 regulation via the central respiratory chemoreflex and CVR, and that both CO2 regulatory mechanisms are functionally interactive. Importantly, these findings provide the possibility that CO2 homeostasis in the brain may be altered via modified CO2 regulatory mechanisms in space. Indeed, it has been reported that long-term microgravity decreased cognitive function (Salazar et al., 2020, 2021) and that this is associated with impaired CVR (Kim et al., 2021).
In contrast, the CVR and central respiratory chemoreflex were unchanged during orthostatic stress-induced via lower negative pressure (LBNP) and head-up tilt (HUT; Ogoh et al., 2013; Tymko et al., 2015). However, these previous studies (Ogoh et al., 2013; Tymko et al., 2015) have been constrained to the steady-state characteristics of CVR and central respiratory chemoreflex and have not considered the dynamic responses. Early reports (Shapiro et al., 1965) indicated that the elevation in CBF proceeds within 30 s of CO2 inhalation and that less than 2 min were required to achieve peak perfusion (Ellingsen et al., 1987). Since the cerebrovascular and respiration regulatory systems interact via the same mediator (i.e., CO2), whereas the respiratory response to CO2 is slower than that of the cerebrovasculature (Ogoh, 2019), the onset of the cerebrovascular response may be isolated from the respiratory response. This highlights the potential mechanistic importance of the CBF “onset” that is different from that of the traditional steady-state CVR (Rasmussen et al., 2006; Ogoh et al., 2008, 2009, 2020). Furthermore, to what extent altered gravitational stress impacts the corresponding kinetics underlying the dynamic cerebrovascular responses to hypercapnia remains to be investigated.
Cerebrovascular regulation is also subject to considerable regional heterogeneity. Traditionally, studies have focused on changes in perfusion to the anterior circulation employing middle cerebral artery blood velocity (MCA V) as a surrogate for CBF (Ogoh and Ainslie, 2009a,b; Willie et al., 2011). However, it is noteworthy that MCA V response to orthostatic stress differs compared to the posterior circulation (Sato et al., 2012a; Ogoh et al., 2015; Washio et al., 2018). In the anterior cerebral circulation, neural activity and metabolism are closely related to regional CBF, termed neurovascular coupling, the vascular beds supplying more metabolically active brain regions are likely to be dilated to maintain perfusion (Nakagawa et al., 2009). On the other hand, the territories supplied by the posterior circulation (i.e., brain stem, medulla oblongata, visual cortex, cerebellum, and vestibular regions) are robustly and constantly activated during orthostatic stress due to sympathoexcitation, visual stimulation, postural control, and gravitational stress. This would place the posterior territories in a state of continuous vasodilatation relative to the internal carotid territories (Haubrich et al., 2004; Nakagawa et al., 2009) highlighting site-specific regulation. These observations are consistent with previous studies (Sato et al., 2012b; Skow et al., 2013) demonstrating that CVR in the anterior circulation is higher relative to the posterior.
Given this knowledge and in contrast to what would be expected during steady-state CVR, we hypothesized that the onset of CBF response to changes in CO2 (dynamic CVR) would be altered by gravitational stress subsequent to changes in the central respiratory chemoreflex (Ogoh et al., 2009). Also, given the preferential defense of cerebral substrate delivery to the phylogenetically older, evolutionary conserved hindbrain (supplied by the posterior circulation; Bailey et al., 2020; Calverley et al., 2020), we further hypothesized that these interactive changes would be more pronounced in the anterior circulation subserved by the MCA. To test these hypotheses, the present study sought to characterize the onset responses of the respiratory chemoreflex and middle cerebral artery (MCA) and posterior cerebral artery (PCA) mean blood velocity (Vmean) to hypercapnia incorporating HUT-induced orthostasis as a terrestrial spaceflight analogue of gravitational stress.
Materials and Methods
Participants
Thirteen healthy participants participated in this study (10 men and 3 women; mean age, 24 ± 4 years; stature, 167.4 ± 6.9 cm; body mass, 62.0 ± 12.1 kg). All participants were non-smokers, free of any cerebrovascular or cardiovascular disease and were not taking any over-the-counter or prescribed medication. Before the experiment, participants were required to abstain from caffeinated beverages, strenuous exercise, and alcohol for 24 h. Furthermore, the participants were instructed to consume a light meal 4 h prior to the start of the experiment in order to minimize the potential effect of individual meals on cardiorespiratory and cerebrovascular responses.
Design
All measurements were performed on the same day for each participant. This study was conducted using the following two body position: supine and 50° HUT conditions. Participants did not move their head in an attempt to prevent any potential confounds associated with vestibular activation (Hume and Ray, 1999; Ogoh et al., 2018). After instrumentation, participants were placed on the tilt table. To characterize cerebral blood velocities and respiratory responses to hypercapnia, the participants breathed through a face mask and inspired a selected gas mixture from a Douglas bag containing 5.0% CO2, 21.0% O2 balanced with N2 [inspired CO2 (FICO2) = 5%] during supine and 50° HUT. After 20 min rest at either position, 8 min of baseline data were recorded while breathing room air. After baseline recording, the hypercapnia trial was induced by a rapid change in the FICO2 and lasted for 9 min. It takes a few minutes for fluid shifts to reach equilibrium following a change in body position (Ogoh et al., 2003). Equally, positional changes alter pulmonary ventilation (Ogoh et al., 2013) taking circa 7–8 min to reach steady-state subsequent to chemoreflex activation (Poon and Greene, 1985). Thus, we allowed a 20 min period that we considered adequate for steady-state equilibration. After each trial, body position was changed, and the participants rested for at least 20 min while inspiring room air. Following that, the other trial was conducted in the same manner. The order of the supine and 50° HUT trials was randomized for each participant. The room temperature was set at 24–25°C.
Measurements
Heart rate (HR) was measured using a lead II electrocardiogram (bedside monitor, BMS-3400; Nihon Kohden, Tokyo, Japan). Beat-to-beat arterial blood pressure (ABP) was monitored continuously using a finger photoplethysmography (Finapres Medical Systems, Amsterdam, Netherlands) with a cuff placed on the middle finger of the left hand. Stroke volume (SV) and cardiac output (Q) were determined from the ABP waveform using a Modelflow software program, which incorporates the sex, age, stature, and body mass of the participants (Beat Scope1.1; Finapres Medical Systems). MCA V and PCA V were measured as surrogate metrics for regional CBF through the right and left temporal windows, respectively, using transcranial Doppler ultrasonography (TCD) system (DWL Doppler Box-X; Compumedics, Singen, Germany). The TCD probe was fixed and held in a measurement position using a dedicated headband (Elastic headband, DWL) to maintain a constant insonation angle throughout the experiment. For characterization of respiratory responses to hypercapnia, participants breathed through a leak-free respiratory mask attached to a flowmeter and two-way valve. The valve mechanism allowed participants to inspire room air or a gas mixture from a 300-liter Douglas bag. Pulmonary ventilation (V̇E), tidal volume (Vt), and end-tidal partial pressure of CO2 (PETCO2) were measured breath-by-breath using an automated gas analyzer (AE-310S, Minato Medical Science Co., Osaka, Japan).
Data Analysis
All data were sampled continuously at 1 kHz using an analog-to-digital converter (Power Lab 16 s; AD Instruments, Sydney, Australia) and stored on a laboratory computer for offline analysis. Mean arterial pressure (MAP), mean MCA V (MCA Vmean), and mean PCA V (PCA Vmean) were obtained from each waveform and resampled at 1 Hz. The predicted partial pressure of arterial CO2 (PaCO2) was derived from PETCO2 to Vt using the following equation (Jones et al., 1979).
Importantly, a previous study (Miyamoto et al., 2014) demonstrated that the relationship between PETCO2 and PaCO2 was unchanged by differential changes in central blood volume shifts. During supine and 50°. HUT, all variables were averaged over 60 s immediately before CO2 administration and end of hypercapnia trial for baseline and steady-state measurements.
Dynamic responses of V̇E, predicted PaCO2, MCA Vmean, and PCA Vmean were evaluated using a one-compartment nonlinear least-squares optimization method. The remaining data of onset responses of predicted PaCO2, MCA Vmean, PCA Vmean, and V̇E were fitted to the following single-exponential regression equation consisting of the response time latency [CO2-response delay (t0)], baseline value, gain term (G), and time constant (τ) fitted to the CO2 administration protocol:
where y is the response, t is time, and y0 is a baseline value. Time 0 reflects start of CO2 administration (Figure 1).
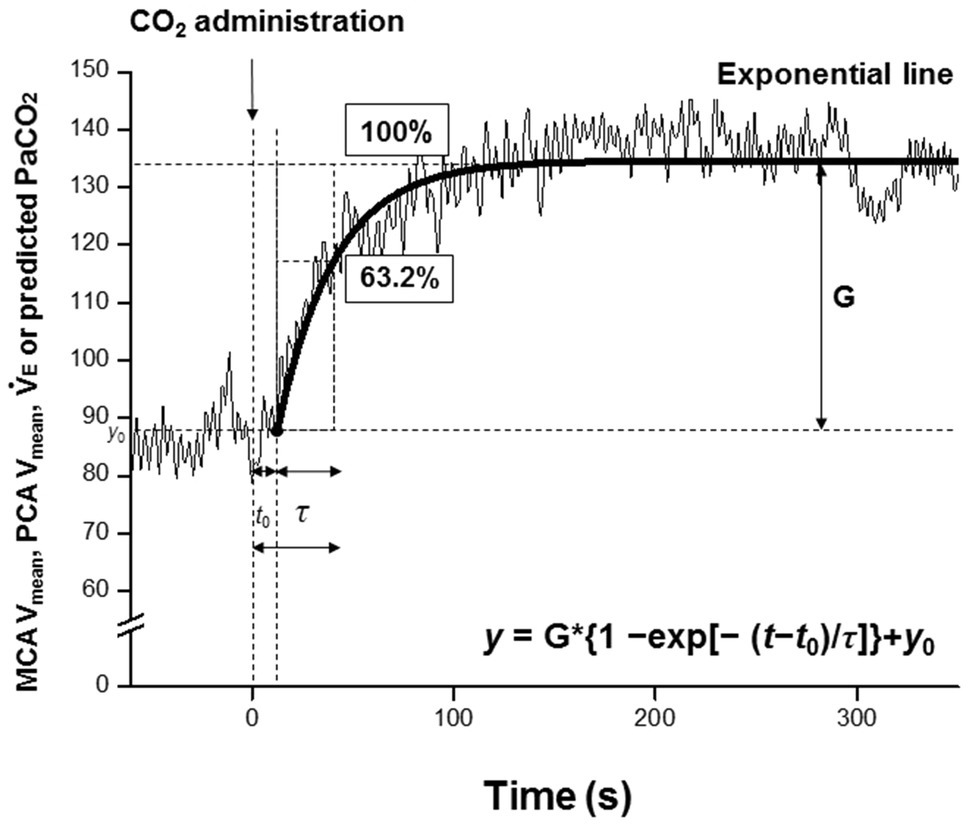
Figure 1. Single-exponential regression model, t0; the response time latency from induction of carbon dioxide administration to change [CO2-response delay], y0, baseline value; and G, gain term, and τ; time constant of the fitted curve of exponential regression during CO2 administration. Time 0 (t0 = 0) refers to the start of CO2 administration. The τ is the time (unit: second) from t0 to reach 63.2% of the steady-state value.
Statistical Analysis
Data from our pilot study (n = 5) were used to perform prospectively power analysis in this study with an assumed type 1 error of 0.05 and statistical power of 80% to detect differences in τ assessed by MCA Vmean between supine and 50° HUT conditions. This power analysis indicated that the critical sample size was estimated to be 10 participants.
All data were analyzed using SPSS (IBM SPSS Statistics Version 27.0) and expressed as mean ± standard deviation (SD). A linear mixed model with fixed effects for Condition (supine vs. 50° HUT) or Time (baseline vs. hypercapnia) was used to compare steady-state data. Before the analysis for dynamic responses of the respiratory chemoreflex and CVR to hypercapnia during supine and 50° HUT, the Shapiro–Wilk’s test was applied to verify the normal distribution for each variable. The distribution normality was confirmed in variables (W ≥ 0.870, p ≥ 0.081), excluding t0 of V̇E, MCA Vmean and PCA Vmean, τ of MCA Vmean and PCA Vmean, τ + t0 of PaCO2, and G of predicted MCA Vmean, PCA Vmean, and PaCO2 (W ≥ 0.713, p ≤ 0.045). To compare normally distributed outcomes between conditions, we incorporated paired samples t-tests. Wilcoxon matched-pairs signed ranks tests were employed where appropriate as a non-parametric equivalent. Statistical significance was set at p < 0.05.
Results
Loss to Follow-Up
Steady-state V̇E and PCA V signals were not acquired in one participant. Thus, overall data analyzes included a sample size of 12 participants.
Steady-State Responses to CO2 at Supine and 50° HUT
Table 1 outlines the hemodynamic and respiratory responses during supine and 50° HUT. During 50° HUT, SV and MCA Vmean decreased (p < 0.006), whereas HR and MAP increased compared to supine (p < 0.049). During hypercapnia, MAP, Q, MCA Vmean, PCA Vmean, V̇E, Vt, PETCO2, and predicted PaCO2 were elevated throughout both supine and 50° HUT conditions (p < 0.010).
Dynamic Response to CO2 Administration at Supine and 50° HUT
The onset of response of predicted PaCO2 to CO2 administration was faster than that of other variables (i.e., MCA Vmean, PCA Vmean, and V̇E), but this response did not differ between supine and 50° HUT (G: predicted PaCO2, p = 0.754 and t0 + τ: predicted PaCO2, p = 0.489, Figure 2).
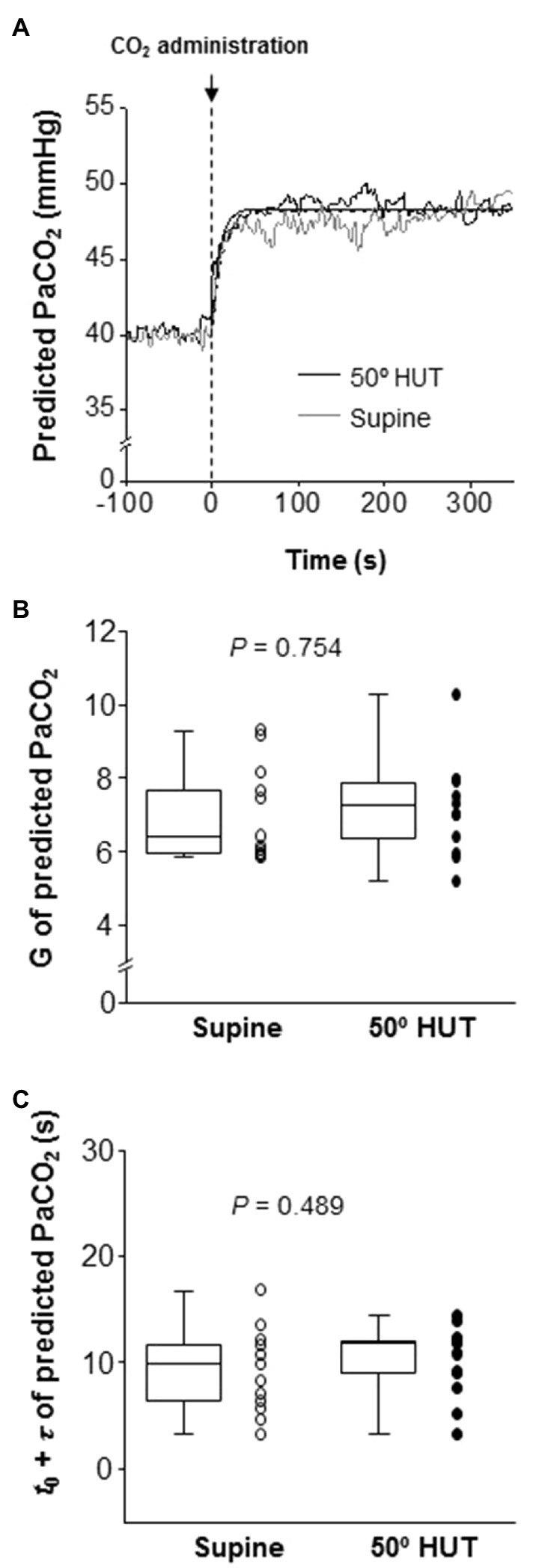
Figure 2. Panel A: Continuous recording of predicted partial pressure of arterial CO2 (PaCO2) responses to CO2 administration (5% CO2) during supine (gray line) and 50° head-up tilt (HUT; black line) in one representative participant. The dash-dotted and smooth curve represent the exponential lines at supine and 50° HUT, respectively. Panel B: Group-averaged gain (G) of predicted PaCO2 exponential fitting curves during supine and 50° HUT. Panel C: Grouped sum of CO2-response delay (t0) and time constant (τ) of predicted PaCO2 exponential fitting curves during supine and 50° HUT. The predicted PaCO2 was derived from PETCO2 and Vt using the following equation (Jones et al., 1979); Predicted PaCO2 = 5.5 + 0.9*PETCO2–0.0021*Vt. Grouped data are shown as median and interquartile range with individual data points.
Following the change in predicted PaCO2, change in MCA Vmean, PCA Vmean, and V̇E also fitted to the similar exponential onset curve during hypercapnia (Figure 3). Despite different steady-state MCA Vmean, PCA Vmean and V̇E during hypercapnia between conditions, G and t0, the fitting curve variable of MCA Vmean, PCA Vmean, and V̇E did not differ between supine and 50° HUT (G: MCA Vmean, p = 0.182; PCA Vmean, p = 0.530 and V̇E, p = 0.838; t0: MCA Vmean, p = 0.413; PCA Vmean, p = 0.350 and V̇E, p = 0.139). In contrast, the average of τ of MCA Vmean was elevated compared to supine, indicating that the onset of MCA Vmean response was slower during 50° HUT (p = 0.003) while that of PCA Vmean, V̇E did not differ between conditions (PCA Vmean, p = 0.071; V̇E, p = 0.707).
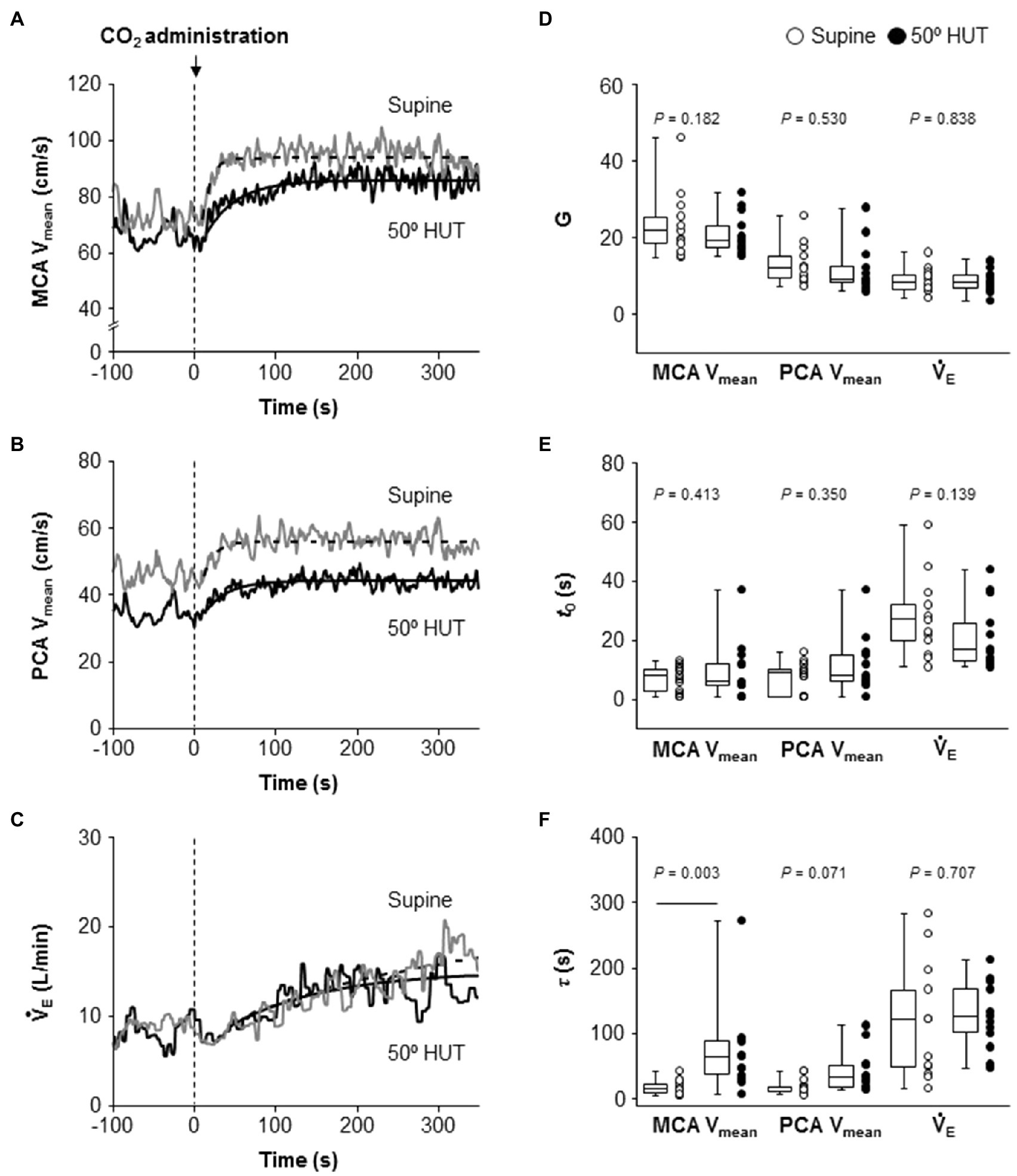
Figure 3. Dynamic cerebrovascular carbon dioxide (CO2) reactivity and central respiratory chemoreflex were characterized using a single-exponential regression model. Panels A–C: Continuous recordings of middle and posterior cerebral artery mean blood velocities (MCA Vmean, A and PCA Vmean, B) and pulmonary ventilation (V̇E, C) response to CO2 administration (5% CO2) during hypercapnia during supine (gray line) and 50° head-up tilt (HUT; black line) in one representative participant. The dash-dotted and smooth curve represent the exponential lines at supine and 50° HUT, respectively. Panels D–F: Grouped gain (G, D), CO2-response delay (t0, E), and time constant (τ, F) of MCA Vmean, PCA Vmean, and V̇E exponential fitting curves during supine and 50° HUT. Grouped data are shown as median and interquartile range with individual data points.
Discussion
The present study has identified two novel findings. First and consistent with our original hypothesis, acute gravitational stress selectively impaired dynamic CVR in the anterior cerebral circulation, whereas the posterior circulation was preserved. Second, albeit contrary to original expectations, this impairment was independent of any changes to the central respiratory chemoreflex. Collectively, these findings further highlight the regional heterogeneity underlying CBF autochemoregulation that may have translational implications for the microgravity (and hypercapnia) associated with deep-space flight notwithstanding terrestrial orthostatic diseases that have been linked to accelerated cognitive decline and neurodegeneration.
Prior studies have identified that changes in central blood volume (CBV) modify ventilation (Miyamoto et al., 2014). For example, increased CBV through water immersion causes hypoventilation, in contrast, a lower body negative pressure-induced decrease in CBV causes hyperventilation. This modification may be linked to a CBV-induced change in CBF (Ogoh et al., 2013). In support, Ogoh et al. (2013) demonstrated that an orthostatic stress-mediated reduction in CBF induced a leftward shift of the central respiratory chemoreflex (V̇E-PETCO2 relationship) without altering its sensitivity (Miyamoto et al., 2014), indicating an elevated V̇E for any given PETCO2. This finding indicates that the gravitational stress-induced reduction in CBF likely attenuated cerebral CO2 (elimination) “washout” causing hyperpnea following autochemoactivation of the central respiratory chemoreflex. Given such conflict, we herein speculated that the onset (dynamic) response of ventilation to hypercapnia would be altered via a gravitational stress-induced change in CBV since orthostatic stress causes hyperventilation (Ogoh et al., 2013) via an interaction between CBF regulation and respiratory response (Ogoh et al., 2008, 2009, 2019). However, contrary to our original expectations, HUT failed to alter the onset response of ventilation to hypercapnia. Importantly, these findings indicate that alteration in CBF regulation was independent of the central chemoreflex.
In contrast to published data indicating that orthostatic stress failed to alter steady-state CVR (Tymko et al., 2015), our findings indicate that the (dynamic) onset of CVR was attenuated by gravitational stress and selectively constrained to the anterior circulation. This apparent contradiction clearly highlights the importance of the “on-kinetic” when exploring the physiological response to hypercapnia. In addition, a steady-state data determined CVR includes central respiratory chemoreflex, indicating that steady-state CVR may not reflect a purely cerebrovascular response (Ogoh et al., 2009). Indeed, it has been reported the different response between steady-state and onset dynamic response of CVR, for example, exercise enhanced the steady-state CVR (Rasmussen et al., 2006), in contrast, the onset response of CVR unchanged during exercise (Ogoh et al., 2009). Similarly to the previous study, the finding of the present study indicates that the onset (dynamic) cerebrovascular response to CO2 is different from the traditional steady-state CVR against gravitational stress. However, further research is warranted to identify the underlying mechanisms.
In contrast, the posterior cerebrovascular response to CO2 was unchanged during HUT, replicating traditional steady-state CVR data of MCA Vmean and PCA Vmean responses (Tymko et al., 2015). Several observations may indirectly explain these differential findings. The posterior circulation is characterized by lower dynamic cerebral autoregulation compared to the anterior circulation (Sato et al., 2012a) notwithstanding other factors including some reports of comparatively lower sympathetic innervation (Edvinsson, 1975) and CO2 vasoreactivity (Sato et al., 2012b) and thus better equipped to “defend” CBF against acute changes in CBV. This makes teleological sense given that the territories the vertebral-basilar system feeds, notably the medulla oblongata, cerebellum, hypothalamus, thalamus, and brainstem, are phylogenetically older with priority over other (younger, more anterior) regions for O2 and glucose supply given their arguably more critical roles in maintaining homeostasis (Bailey, 2019). However, the mechanism of the effect of gravitational stress on regional differences in dynamic cerebrovascular response to CO2 between anterior and posterior cerebral arteries remains unknown and warrants further consideration in follow-up studies. One possible mechanism is the different CBF responses between anterior and posterior cerebral arteries to HUT. It has been reported that the decrease in posterior CBF during gravitational stress is lower than that of anterior CBF (Ogoh et al., 2015). It is possible that gravitational stress-induced CBF reduction in the anterior cerebral artery may be associated with an attenuation in the cerebrovascular response to CO2.
Limitations
Potential limitations of the present study warrant careful consideration. First, the TCD-determined MCA Vmean and PCA Vmean are widely used as an index of anterior and posterior (intracranial) CBF, respectively (Tymko et al., 2015; Washio et al., 2020). While this approach provides excellent continuous high-resolution sampling of CBF kinetics, it would have been interesting to “map” perfusion through other intra/extracranial vessels given the aforementioned perfusion heterogeneity and site-specific differences in autochemoregulation, e.g., MCA vs. anterior cerebral artery (Jorgensen et al., 1992; Linkis et al., 1995), and PCA vs. vertebral artery (Washio et al., 2017). In addition, this methodological technique can identify a transient change in CBF, albeit limited by the misplaced assumption that artery diameter remains constant. Previous work (Willie et al., 2011) clearly demonstrates that there is likely to be some degree of vasodilation induced by the increases in PETCO2 stimulated in the present study. Indeed, Al-Khazra et al. (Al-Khazraji et al., 2021) demonstrated that step changes in CO2 altered the MCA diameter despite no change in MCA diameter during the ramp CO2 stimulation, indicating that the protocol of the present study may have potentially underestimated CVR but it is unclear whether orthostatic stress modifies this limitation. The human brain has evolved heightened sensitivity to PaCO2/H+ (more so than PaO2) that extends throughout the cerebrovasculature, from the large extracranial and intracranial conduit and middle cerebral arteries through to the smallest pial arterioles and parenchymal vessels, prioritizing the buffering of brain tissue pH for stabilization of chemosensory and autonomic control at the level of the brainstem (Bailey et al., 2017). In addition, orthostatic stress causes hypocapnia subsequent to hyperventilation effecting a reduction in CBF (Ogoh et al., 2013, 2019). However, in the present study, VE was unchanged during orthostatic stress (p = 0.871). Furthermore, the definitely addressing how varying degrees of gravity notwithstanding differences in age, sex, race, medication, altitude acclimatization, atmospheric pressure, physical training, etc. on CVR may be important for the space physiology but it remains unclear in the present study. Finally, we observed differences in MAP between the two trials highlighting two distinct mechanisms that could potentially influence CBF; mechanical (pressure-induced) and chemo (CO2-induced) autoregulation, with ongoing controversy as to which mechanism “dominates.” Evidence suggests that nitric oxide (NO) is more important for chemo as opposed to the mechanoregulation of CBF (Weiss et al., 1979; Buchanan and Phillis, 1993; Thompson et al., 1996). Indeed, low doses of a NO donor, without causing major systemic hemodynamic perturbations, have been shown to blunt hyperventilation-mediated cerebral vasoconstriction and enhance the vasodilatory effect of hypercapnia, shifting the vasomotor CO2-reactivity slope to the left. Furthermore, rapid changes in pH that occur during hypercapnia serve as an important modulator of NO synthase. Equally, pharmacological manipulation of opiate receptors, prostaglandins, ATP-dependent K+ channel activation, and free radicals modulates the CO2–NO axis and underlying cerebral vasomotor reactivity (Lavi et al., 2003). To what extent redox-sensitive mechanisms activated by the shear stress imparted by HUT-induced CBV shifts contribute to the observed findings cannot be ignored and warrants further consideration in follow-up research.
Conclusion
In contrast to steady-state CVR, the onset of cerebrovascular to CO2 during gravitational stress was selectively impaired in the anterior but not posterior cerebral circulation. These findings indicate that dynamic CBF regulation may contribute to microgravity-induced cognitive dysfunction.
Data Availability Statement
The raw data supporting the conclusions of this article will be made available by the authors, without undue reservation.
Ethics Statement
The studies involving human participants were reviewed and approved by the Institutional Review Board at Toyo University. The patients/participants provided their written informed consent to participate in this study.
Author Contributions
HW and SO conceptualized and designed the research. HW, SS, TW, and SO performed the experiments. HW and SS analyzed the data. HW, DB, and SS interpreted the results of experiments. HW prepared the figures. HW, SO, and DB drafted the manuscript. All authors edited, revised, and approved final version of manuscript.
Funding
SO is supported by a Grant-in-Aid for Scientific Research [Grant Number 15H003098] from the Japanese Ministry of Education, Culture, Sports, Science and Technology. DB was supported by a Royal Society Wolfson Research Fellowship (#WM170007) and Japan Society for the Promotion of Science (#JSPS/OF317).
Conflict of Interest
DB is Chair of the Life Sciences Working Group and ex-officio member of the Human Spaceflight and Exploration Science Advisory Committee to the European Space Agency and Member of the Space Exploration Advisory Committee to the UK Space Agency.
The remaining authors declare that the research was conducted in the absence of any commercial or financial relationships that could be construed as a potential conflict of interest.
Publisher’s Note
All claims expressed in this article are solely those of the authors and do not necessarily represent those of their affiliated organizations, or those of the publisher, the editors and the reviewers. Any product that may be evaluated in this article, or claim that may be made by its manufacturer, is not guaranteed or endorsed by the publisher.
Acknowledgments
We appreciate the commitment of all participants.
References
Ainslie, P. N., and Duffin, J. (2009). Integration of cerebrovascular CO2 reactivity and chemoreflex control of breathing: mechanisms of regulation, measurement, and interpretation. Am. J. Physiol. Regul. Integr. Comp. Physiol. 296, R1473–R1495. doi: 10.1152/ajpregu.91008.2008
Al-Khazraji, B. K., Buch, S., Kadem, M., Matushewski, B. J., Norozi, K., Menon, R. S., et al. (2021). Protocol-dependence of middle cerebral artery dilation to modest hypercapnia. Appl. Physiol. Nutr. Metab. 46, 1038–1046. doi: 10.1139/apnm-2021-0220
Bailey, D. M. (2019). Oxygen, evolution and redox signalling in the human brain; quantum in the quotidian. J. Physiol. 597, 15–28. doi: 10.1113/JP276814
Bailey, D. M., Ainslie, P. N., Petersen, L., and Zu Eulenburg, P. (2021). Jumping at a chance to control cerebral blood flow in astronauts. Exp. Physiol. 106, 1407–1409. doi: 10.1113/EP089648
Bailey, D. M., Laneelle, D., Trihan, J. E., Marchi, N., Stacey, B. S., Tamiya, K., et al. (2020). Gravitational transitions increase posterior cerebral perfusion and systemic oxidative-nitrosative stress: implications for neurovascular unit integrity. Neuroscience 441, 142–160. doi: 10.1016/j.neuroscience.2020.05.048
Bailey, D. M., Willie, C. K., Hoiland, R. L., Bain, A. R., Macleod, D. B., Santoro, M. A., et al. (2017). Surviving without oxygen: how low can the human brain go? High Alt. Med. Biol. 18, 73–79. doi: 10.1089/ham.2016.0081
Buchanan, J. E., and Phillis, J. W. (1993). The role of nitric oxide in the regulation of cerebral blood flow. Brain Res. 610, 248–255. doi: 10.1016/0006-8993(93)91408-K
Calverley, T. A., Ogoh, S., Marley, C. J., Steggall, M., Marchi, N., Brassard, P., et al. (2020). HIITing the brain with exercise: mechanisms, consequences and practical recommendations. J. Physiol. 598, 2513–2530. doi: 10.1113/JP275021
Chesler, M. (2003). Regulation and modulation of pH in the brain. Physiol. Rev. 83, 1183–1221. doi: 10.1152/physrev.00010.2003
Edvinsson, L. (1975). Neurogenic mechanisms in the cerebrovascular bed. Autonomic nerves, amine receptors and their effects on cerebral blood flow. Acta Physiol. Scand. Suppl. 427, 1–35.
Ellingsen, I., Hauge, A., Nicolaysen, G., Thoresen, M., and Walloe, L. (1987). Changes in human cerebral blood flow due to step changes in PaO2 and PaCO2. Acta Physiol. Scand. 129, 157–163. doi: 10.1111/j.1748-1716.1987.tb08054.x
Haubrich, C., Wendt, A., Diehl, R. R., and Klotzsch, C. (2004). Dynamic autoregulation testing in the posterior cerebral artery. Stroke 35, 848–852. doi: 10.1161/01.STR.0000120729.99039.B6
Hume, K. M., and Ray, C. A. (1999). Sympathetic responses to head-down rotations in humans. J. Appl. Physiol. 86, 1971–1976. doi: 10.1152/jappl.1999.86.6.1971
Jones, N. L., Robertson, D. G., and Kane, J. W. (1979). Difference between end-tidal and arterial PCO2 in exercise. J. Appl. Physiol. Respir. Environ. Exerc. Physiol. 47, 954–960. doi: 10.1152/jappl.1979.47.5.954
Jorgensen, L. G., Perko, G., and Secher, N. H. (1992). Regional cerebral artery mean flow velocity and blood flow during dynamic exercise in humans. J. Appl. Physiol. 73, 1825–1830. doi: 10.1152/jappl.1992.73.5.1825
Kim, D., Hughes, T. M., Lipford, M. E., Craft, S., Baker, L. D., Lockhart, S. N., et al. (2021). Relationship between cerebrovascular reactivity and cognition among people with risk of cognitive decline. Front. Physiol. 12:645342. doi: 10.3389/fphys.2021.645342
Lavi, S., Egbarya, R., Lavi, R., and Jacob, G. (2003). Role of nitric oxide in the regulation of cerebral blood flow in humans: chemoregulation versus mechanoregulation. Circulation 107, 1901–1905. doi: 10.1161/01.CIR.0000057973.99140.5A
Lavi, S., Gaitini, D., Milloul, V., and Jacob, G. (2006). Impaired cerebral CO2 vasoreactivity: association with endothelial dysfunction. Am. J. Physiol. Heart Circ. Physiol. 291, H1856–H1861. doi: 10.1152/ajpheart.00014.2006
Lee, S. T., Jung, K. H., and Lee, Y. S. (2007). Decreased vasomotor reactivity in Alzheimer’s disease. J. Clin. Neurol. 3, 18–23. doi: 10.3988/jcn.2007.3.1.18
Linkis, P., Jorgensen, L. G., Olesen, H. L., Madsen, P. L., Lassen, N. A., and Secher, N. H. (1995). Dynamic exercise enhances regional cerebral artery mean flow velocity. J. Appl. Physiol. 78, 12–16. doi: 10.1152/jappl.1995.78.1.12
Miyamoto, T., Bailey, D. M., Nakahara, H., Ueda, S., Inagaki, M., and Ogoh, S. (2014). Manipulation of central blood volume and implications for respiratory control function. Am. J. Physiol. Heart Circ. Physiol. 306, H1669–H1678. doi: 10.1152/ajpheart.00987.2013
Nakagawa, K., Serrador, J. M., Larose, S. L., Moslehi, F., Lipsitz, L. A., and Sorond, F. A. (2009). Autoregulation in the posterior circulation is altered by the metabolic state of the visual cortex. Stroke 40, 2062–2067. doi: 10.1161/STROKEAHA.108.545285
Ogoh, S. (2019). Interaction between the respiratory system and cerebral blood flow regulation. J. Appl. Physiol. 127, 1197–1205. doi: 10.1152/japplphysiol.00057.2019
Ogoh, S., and Ainslie, P. N. (2009a). Cerebral blood flow during exercise: mechanisms of regulation. J. Appl. Physiol. 107, 1370–1380. doi: 10.1152/japplphysiol.00573.2009
Ogoh, S., and Ainslie, P. N. (2009b). Regulatory mechanisms of cerebral blood flow during exercise: new concepts. Exerc. Sport Sci. Rev. 37, 123–129. doi: 10.1097/JES.0b013e3181aa64d7
Ogoh, S., Ainslie, P. N., and Miyamoto, T. (2009). Onset responses of ventilation and cerebral blood flow to hypercapnia in humans: rest and exercise. J. Appl. Physiol. 106(3), 880–886. doi: 10.1152/japplphysiol.91292.2008
Ogoh, S., Hayashi, N., Inagaki, M., Ainslie, P. N., and Miyamoto, T. (2008). Interaction between the ventilatory and cerebrovascular responses to hypo- and hypercapnia at rest and during exercise. J. Physiol. 586, 4327–4338. doi: 10.1113/jphysiol.2008.157073
Ogoh, S., Marais, M., Lericollais, R., Denise, P., Raven, P. B., and Normand, H. (2018). Interaction between graviception and carotid baroreflex function in humans during parabolic flight-induced microgravity. J. Appl. Physiol. 125(2), 634–641. doi: 10.1152/japplphysiol.00198.2018
Ogoh, S., Nakahara, H., Okazaki, K., Bailey, D. M., and Miyamoto, T. (2013). Cerebral hypoperfusion modifies the respiratory chemoreflex during orthostatic stress. Clin. Sci. (Lond.) 125, 37–44. doi: 10.1042/CS201200335
Ogoh, S., Sato, K., Okazaki, K., Miyamoto, T., Hirasawa, A., Sadamoto, T., et al. (2015). Blood flow in internal carotid and vertebral arteries during graded lower body negative pressure in humans. Exp. Physiol. 100, 259–266. doi: 10.1113/expphysiol.2014.083964
Ogoh, S., Shibata, S., Ito, G., and Miyamoto, T. (2020). Dynamic characteristics of cerebrovascular reactivity or ventilatory response to change in carbon dioxide. Exp. Physiol. 105, 1515–1523. doi: 10.1113/EP088800
Ogoh, S., Volianitis, S., Nissen, P., Wray, D. W., Secher, N. H., and Raven, P. B. (2003). Carotid baroreflex responsiveness to head-up tilt-induced central hypovolaemia: effect of aerobic fitness. J. Physiol. 551, 601–608. doi: 10.1113/jphysiol.2003.046029
Poon, C. S., and Greene, J. G. (1985). Control of exercise hyperpnea during hypercapnia in humans. J. Appl. Physiol. 59, 792–797. doi: 10.1152/jappl.1985.59.3.792
Rasmussen, P., Stie, H., Nielsen, B., and Nybo, L. (2006). Enhanced cerebral CO2 reactivity during strenuous exercise in man. Eur. J. Appl. Physiol. 96, 299–304. doi: 10.1007/s00421-005-0079-3
Richiardi, J., Monsch, A. U., Haas, T., Barkhof, F., Van De Ville, D., Radu, E. W., et al. (2015). Altered cerebrovascular reactivity velocity in mild cognitive impairment and Alzheimer’s disease. Neurobiol. Aging 36, 33–41. doi: 10.1016/j.neurobiolaging.2014.07.020
Salazar, A. P., Hupfeld, K. E., Lee, J. K., Banker, L. A., Tays, G. D., Beltran, N. E., et al. (2021). Visuomotor adaptation brain changes during a spaceflight analog with elevated carbon dioxide (CO2): A pilot study. Front. Neural Circuits 15:659557. doi: 10.3389/fncir.2021.659557
Salazar, A. P., Hupfeld, K. E., Lee, J. K., Beltran, N. E., Kofman, I. S., De Dios, Y. E., et al. (2020). Neural working memory changes during a spaceflight analog With elevated carbon dioxide: A pilot study. Front. Syst. Neurosci. 14:48. doi: 10.3389/fnsys.2020.00048
Sato, K., Fisher, J. P., Seifert, T., Overgaard, M., Secher, N. H., and Ogoh, S. (2012a). Blood flow in internal carotid and vertebral arteries during orthostatic stress. Exp. Physiol. 97, 1272–1280. doi: 10.1113/expphysiol.2012.064774
Sato, K., Sadamoto, T., Hirasawa, A., Oue, A., Subudhi, A. W., Miyazawa, T., et al. (2012b). Differential blood flow responses to CO2 in human internal and external carotid and vertebral arteries. J. Physiol. 590, 3277–3290. doi: 10.1113/jphysiol.2012.230425
Shapiro, E., Wasserman, A. J., and Patterson, J. L. Jr. (1965). Human cerebrovascular response time to elevation of arterial carbon dioxide tension. Arch. Neurol. 13, 130–138. doi: 10.1001/archneur.1965.00470020020003
Siesjo, B. K. (1972). Symposium on acid-base homeostasis. The regulation of cerebrospinal fluid pH. Kidney Int. 1, 360–374. doi: 10.1038/ki.1972.47
Silvestrini, M., Pasqualetti, P., Baruffaldi, R., Bartolini, M., Handouk, Y., Matteis, M., et al. (2006). Cerebrovascular reactivity and cognitive decline in patients with Alzheimer disease. Stroke 37, 1010–1015. doi: 10.1161/01.STR.0000206439.62025.97
Skow, R. J., Mackay, C. M., Tymko, M. M., Willie, C. K., Smith, K. J., Ainslie, P. N., et al. (2013). Differential cerebrovascular CO2 reactivity in anterior and posterior cerebral circulations. Respir. Physiol. Neurobiol. 189, 76–86. doi: 10.1016/j.resp.2013.05.036
Thompson, B. G., Pluta, R. M., Girton, M. E., and Oldfield, E. H. (1996). Nitric oxide mediation of chemoregulation but not autoregulation of cerebral blood flow in primates. J. Neurosurg. 84, 71–78. doi: 10.3171/jns.1996.84.1.0071
Tymko, M. M., Skow, R. J., Mackay, C. M., and Day, T. A. (2015). Steady-state tilt has no effect on cerebrovascular CO2 reactivity in anterior and posterior cerebral circulations. Exp. Physiol. 100, 839–851. doi: 10.1113/EP085084
Washio, T., Sasaki, H., and Ogoh, S. (2017). Transcranial Doppler-determined change in posterior cerebral artery blood flow velocity does not reflect vertebral artery blood flow during exercise. Am. J. Physiol. Heart Circ. Physiol. 312, H827–H831. doi: 10.1152/ajpheart.00676.2016
Washio, T., Vranish, J. R., Kaur, J., Young, B. E., Katayama, K., Fadel, P. J., et al. (2018). Acute reduction in posterior cerebral blood flow following isometric handgrip exercise is augmented by lower body negative pressure. Physiol. Rep. 6:e13886. doi: 10.14814/phy2.13886
Washio, T., Watanabe, H., and Ogoh, S. (2020). Dynamic cerebral autoregulation in anterior and posterior cerebral circulation during cold pressor test. J. Physiol. Sci. 70:1. doi: 10.1186/s12576-020-00732-7
Weiss, M. H., Spence, J., Apuzzo, M. L., Heiden, J. S., Mccomb, J. G., and Kurze, T. (1979). Influence of nitroprusside on cerebral pressure autoregulation. Neurosurgery 4, 56–59. doi: 10.1227/00006123-197901000-00011
Keywords: anterior cerebral blood flow, posterior cerebral blood flow, respiratory chemoreflex, head-up tilt, hypercapnia
Citation: Watanabe H, Saito S, Washio T, Bailey DM and Ogoh S (2022) Acute Gravitational Stress Selectively Impairs Dynamic Cerebrovascular Reactivity in the Anterior Circulation Independent of Changes to the Central Respiratory Chemoreflex. Front. Physiol. 12:749255. doi: 10.3389/fphys.2021.749255
Edited by:
Hanns-Christian Gunga, Charité University Medicine Berlin, GermanyReviewed by:
Olga Vinogradova, Institute of Biomedical Problems, Russian Academy of Sciences (RAS), RussiaJulien Vincent Brugniaux, Université Grenoble Alpes, France
Copyright © 2022 Watanabe, Saito, Washio, Bailey and Ogoh. This is an open-access article distributed under the terms of the Creative Commons Attribution License (CC BY). The use, distribution or reproduction in other forums is permitted, provided the original author(s) and the copyright owner(s) are credited and that the original publication in this journal is cited, in accordance with accepted academic practice. No use, distribution or reproduction is permitted which does not comply with these terms.
*Correspondence: Shigehiko Ogoh, b2dvaEB0b3lvLmpw