- 1Department of Chemistry, University of Akron, Akron, OH, United States
- 2Department of Physiology and Pharmacology, University of Toledo College of Medicine and Life Sciences, Toledo, OH, United States
- 3Department of Integrative Medical Sciences, Northeast Ohio Medical University, Rootstown, OH, United States
Endothelial permeability, leukocyte attachment, and unregulated oxidized LDL (oxLDL) uptake by macrophages leading to the formation of foam cells are all vital in the initiation and progression of atherosclerosis. During inflammation, several inflammatory mediators regulate this process through the expression of distinct oxLDL binding cell surface receptors on macrophages. We have previously shown that Leukotriene D4 (LTD4) promotes endothelial dysfunction, increasing endothelial permeability and enhancing TNFα-mediated attachment of monocytes to endothelium, which hints at its possible role in atherosclerosis. Here we analyzed the effect of LTD4 on macrophage function. Macrophages mainly express CysLT1R and flux calcium in response to LTD4. Further, LTD4 potentiates phagocytosis in macrophages as revealed by the uptake of zymosan particles. Notably, LTD4 augmented macrophage phagocytosis and oxLDL uptake which is sensitive to MK-571 [Montelukast (MK)], a CysLT1R-specific antagonist. Mechanistically, LTD4 upregulated two receptors central to foam cell formation, oxidized low-density lipoprotein receptor-1 (OLR1/LOX-1), and CD36 in a time and dose-dependent manner. Finally, LTD4 enhanced the secretion of chemokines MCP-1 and MIP1β. Our results suggest that LTD4 contributes to atherosclerosis either through driving foam cell formation or recruitment of immune cells or both. CysLT1R antagonists are safely being used in the treatment of asthma, and the findings from the current study suggest that these can be re-purposed for the treatment of atherosclerosis.
Introduction
Macrophages are innate immune cells present ubiquitously in the body, and they are involved in the phagocytosis of foreign materials and pathogens (Han et al., 2016). The role of macrophages is not only limited to engulfing foreign allergens, but also extends to ingesting self-antigens like extracellular debris and modified lipids (Patten and Shetty, 2018). Macrophages encounter diverse antigens, and they need distinct receptors to recognize them and initiate phagocytosis (Kelley et al., 2014). Phagocytosis is mediated through scavenger receptors classified into different groups ranging from A–J (Aderem and Underhill, 1999). Scavenger receptors not only function in scavenging self-antigens expressing damage associated molecular patterns (DAMPS) (Patten and Shetty, 2018), they also facilitate phagocytosis of particles like oxLDL that are the products of oxidative stress (Woo et al., 2016). Receptors like class B scavenger receptor CD36, Scavenger Receptor A (SR-A), CD204, and lectin like oxidized low density lipoprotein receptor (OLR1) in macrophages facilitate the internalization and degradation of modified lipids (Woo et al., 2016; Arslan et al., 2017), which initiates the buildup of foam cells, an event that is crucial in the initiation and progression of atherosclerosis. Atherosclerosis is an inflammatory disease involving endothelial dysfunction and the dysregulated uptake of lipid molecules into the blood vessels (Hansson and Hermansson, 2011). The accumulation of foam cells results in the formation of atherosclerotic plaques that further release their lipid contents into the vasculature. Plaque instability and its ultimate rupture results in the formation of a pro-thrombotic necrotic core during atherogenesis (Tabas and Bornfeldt, 2016). Macrophages are the key effector cells, and they have been extensively studied with respect to the disease (Moore et al., 2013). Attenuation of atherosclerotic complications in mice was observed when macrophages were egressed from the lesion microenvironment or when their phenotype was switched to resolution (M2) subset from their inflammatory (M1) counterparts (Feig et al., 2011a, b). Therefore, it is important to understand how soluble factors secreted during inflammation affect macrophage behavior, impacting atherosclerosis progression. From the time a link between inflammation and atherosclerosis was proposed, a range of inflammatory mediators were investigated for their possible role in this disorder (Nguyen et al., 2019). Increased expression of 5-lipoxygenase (5-LO) products, including leukotrienes and their receptors, were reported in atherosclerotic lesions, identifying these molecules as potential therapeutic targets for the disease (Back, 2009). Cysteinyl leukotrienes (cys-LTs) comprising of LTC4, LTD4, and LTE4 are derivatives of arachidonic acid generated by mast cells, macrophages, eosinophils, and basophils (Kanaoka and Boyce, 2004). Cys-LTs are the most potent bronchoconstrictors (Davidson et al., 1987; Drazen and Austen, 1987), and they are involved in the pathophysiology of various inflammatory diseases like asthma, rheumatoid arthritis, and cardiovascular diseases (Chung, 1995; Busse, 1996; Liu and Yokomizo, 2015). Cys-LTs mediate their biologic functions mainly through two known G protein-coupled receptors (GPCRs), CysLT1R, and CysLT2R (Lynch et al., 1999; Heise et al., 2000). Apart from these two main receptors, GPR17 is activated by LTD4 and acts as a negative regulator for CysLT1R (Maekawa et al., 2009). Further, LTE4, the most abundant and stable of the cys-LTs, is a weak, partial agonist for the CysLT1R and CysLT2R (Evans, 2002). In contrast to LTD4, LTE4 relays signals through both peroxisome proliferator activating receptor (PPAR)-γ, a ligand-activated transcription factor (Paruchuri et al., 2008), and P2Y12 receptor (P2Y12R), a GPCR that recognizes adenosine diphosphate (ADP) (Paruchuri et al., 2009). Recently, GPR99 was identified as another CysLTR with a preference for LTE4 (Kanaoka et al., 2013). Pro-inflammatory mediators generated during inflammation activate endothelial cells (EC) and leukocyte extravasation. Injection of each of the three cys-LTs has been shown to enhance dermal vascular permeability in mice and humans (Soter et al., 1983; Maekawa et al., 2008; Kondeti et al., 2013). We recently demonstrated that EC CysLT2R mediates calcium influx, EC contraction in vitro, permeability of blood vessels, as well as angiogenesis in vivo (Duah et al., 2013, 2019). In addition, we also demonstrated that cys-LTs enhance TNFα-mediated up-regulation of vascular cell adhesion molecule (VCAM-1) and also enhance the attachment of monocytes to the endothelium (Duah et al., 2013). Since CysLTR signaling causes endothelial dysfunction, leading to enhanced vessel contraction and permeability facilitating monocyte attachment to endothelium, we explored their role in regulating macrophage function in the current study. While there have been many studies on macrophages, foam cell formation, and atherosclerosis, the involvement of cys-LTs or associated molecular mechanisms in macrophage function impacting atherosclerosis progression is elusive. Therefore in this study, we analyzed the role of cys-LTs in the uptake of oxidized LDL by macrophages, an initial step in the formation of foam cells, and the mechanism involved.
Materials and Methods
Animals
Bone marrow-derived macrophages (BMDM) were cultured from wild type C57BL/6 (WT) mice (6–8-weeks old), purchased from the Jackson Laboratory and maintained at the University of Akron Research vivarium (UARV). Animals were euthanized in accordance with standard guidelines, as approved by the Animal Care and Use Committee of UA.
Materials
Murine recombinant colony stimulating factor (m-CSF) was purchased from Peprotech (Cranbury, NJ). LTD4 and MK571 (MK) were from Cayman Chemicals (Ann Arbor, MI). Fura-2 AM was purchased from Molecular Probes (Eugene, OR). Texas-red conjugated zymosan bioparticles and DiI conjugated oxLDL were purchased from fisher scientific (Waltham, MA).
Cell Culture
Raw 264.7 (raw) cells were cultured in Dulbecco’s Modified Eagle’s high glucose medium (DMEM; Corning, NY) supplemented with 10% FBS and 1% pen-strep. THP-1 monocytes were cultured in RPMI-1640 medium supplemented with 10% FBS and 1% pen-strep. These cells were differentiated into macrophages for 48 h in the presence of 50 ng/ml phorbol-12-myristate-13-acetate (PMA). For BMDM, bones (tibia and femur) were collected from 6 to 8 weeks old WT, Cysltr1–/–, and Cysltr2–/– mice on C57BL/6 background, and bone marrow cells (BMCs) were isolated by flushing bones. Cells were suspended in R10 media (RPMI-1640 supplemented with 10% FBS, 5% non-essential amino acids, 1% pen-strep, and 50 μM β-mercaptoethanol) and maintained at 37°C. BMCs were differentiated into BMDMs using 10 ng/ml macrophage colony stimulating factor (M-CSF). On third day, the culture plate was replenished with fresh R10 medium containing 10 ng/ml M-CSF, and incubated for 3 more days. We confirmed the purity of the culture by F4/80 staining.
Immunofluorescence
Raw macrophages were fixed with 4% paraformaldehyde solution, and permeabilised with 0.25% Triton X-100 for 15 min. Cells were washed twice with PBS, blocked with 10% FBS containing medium for 30 min and were stained with CysLT1R antibody for 1 h. Thereafter, the cells were washed twice in PBS and incubated with Alexa Fluor 488 goat anti-rabbit secondary antibody for 45 min. Images were obtained using EVOS fluorescence microscope.
Ca2+ Flux Assay
Raw cells, THP-1-derived macrophages, and BMDMs were loaded with Fura-2 AM for 30 min and washed in calcium buffer. Cells were stimulated with LTD4 (0.5 μM) in the presence or absence of CysLT1R antagonist MK (1 μM, 30 min pre-incubation). Changes in the intracellular calcium levels were measured using the ratio of excitation wavelengths (340/380 nm) in a fluorescence spectrophotometer (Hitachi F-4500).
The relative ratios of fluorescence emitted at 510 nm were recorded and displayed as a reflection of intracellular calcium concentration (Paruchuri et al., 2008).
Zymosan Phagocytosis Assay
Macrophages were cultured as mentioned earlier, and 50,000 cells were plated in each well of an 8-well chamber slide in 200 μl DMEM high glucose, supplemented with 10% FBS, and stimulated with LTD4 (0.5 μM) for 24 h. Texas-red conjugated zymosan bioparticles were reconstituted to obtain uniform suspension according to the manufacturer’s protocol, and 500,000 zymosan bioparticles (1:10) were added to each well and incubated for 1 h. Excess zymosan particles were removed and washed with PBS, and imaged using a fluorescence microscope. The images were quantified by ImageJ and the percentage phagocytosis was calculated based on the percentage of number of cells with zymosan particles compared to total number of cells (DAPI staining).
Oxidized LDL Uptake Assay
Macrophages were stimulated with 0.5 μM LTD4 for 24 h in the presence or absence of CysLT1R antagonist MK (1 μM) pre-incubated for 30 min. After 24 h, macrophages were incubated with oxLDL (10 μg/ml) for 1 h at 37°C in a humidified incubator with 5% CO2 environment and stained with oil red O (only stains the lipid particles). Excess stain was washed with PBS, and the slides were observed under the microscope. Quantification of phagocytosis was done using ImageJ (NIH) as described above.
Real-Time Quantitative PCR
The expressions of mOLR1, mCD36, and mMCP-1 were determined with qPCR performed on Light cycler 480 (Roche) (Kondeti et al., 2016). Total RNA was isolated from Raw cells, THP-1-derived macrophages, and BMDMs after respective treatments with an E.Z.N.A. Total RNA kit 1 (Omega Bio-Tek, Norcross, Georgia). DNAse contamination was removed using a DNA-free DNA Removal Kit (Invitrogen, Waltham, MA) based on the manufacturer’s instructions. cDNA was synthesized using a cDNA synthesis kit (Roche, Indianapolis, IN). qPCR was performed using the primers mentioned below. The levels of respective genes relative to the GAPDH were analyzed, and the ΔΔCT values were calculated and expressed as relative expression or fold change compared to control (no template). The quality of the RNA, primers, and qPCR reaction was validated using proper controls, like no RT control or no template control. Real time PCR for each sample was performed in at least triplicates and then repeated in three different experiments.
Primers
mOLR1
F: 5′-ACAATACCAAGCGAACCTTACT-3′; R: 5′-TGGGT GAGGGTGTCTATCTT-3′
mCD36
F: 5′-CCAGTCGGAGACATGCTTATT-3′; R: 5′-GTACAC AGTGGTGCCTGTT-3′
mMCP-1
F: 5′-AGTAGGCTGGAGAGCTACAA-3; R: 5′-GTATGT CTGGACCCATTCCTTC-3′
mGAPDH
F: 5′-CTCCCACTCTTCCACCTTCG-3′; R: 5′-CCACCA CCCTGTTGCTGTAG-3′
ELISA
The concentrations of MCP-1 and MIP1β secreted into the medium by macrophages after respective treatments were analyzed by MCP-1 ELISA kit (Invitrogen, Waltham, MA) and MIP1β ELISA kit (R & D Systems, Minneapolis, MN), respectively, according to the manufacturer’s protocol (Kondeti et al., 2016).
Statistical Analysis
Data are expressed as means ± SEM from at least three experiments except where otherwise indicated. Data were converted to a percentage of control for each experiment where indicated. Significance was determined using one-way ANOVA, and comparisons between the groups were determined by Tukey’s multiple comparisons test (GraphPad Prism 7.01; GraphPad Software, La Jolla, CA, United States). *P < 0.05, **P < 0.01, ***P < 0.001.
Results
Leukotriene D4 Mediated Calcium Flux in Macrophages
To understand the role of CysLTR signaling in regulating macrophage function, first we studied the expression of CysLT1R and CysLT2R in three different macrophage cell types- raw macrophages, THP-1-derived macrophages, and BMDMs by qPCR. Our results revealed that all macrophages mainly express CysLT1R compared to CysLT2R (Figures 1A–C). We observed a modest expression of CysLT2R in BMDMs. None of the macrophages revealed expression of GPR99 transcript (not shown). Immune-staining of raw macrophages revealed significant CysLT1R expression at protein level (Figure 1D). Further, in Fura-2 loaded macrophages, LTD4 induced robust calcium flux, which is completely blocked by pretreatment of the cells with MK (Figures 1E–J), which competitively antagonizes CysLT1R, but not CysLT2R (Paruchuri et al., 2008; Duah et al., 2019). Thus, macrophages flux calcium mainly via CysLT1R.
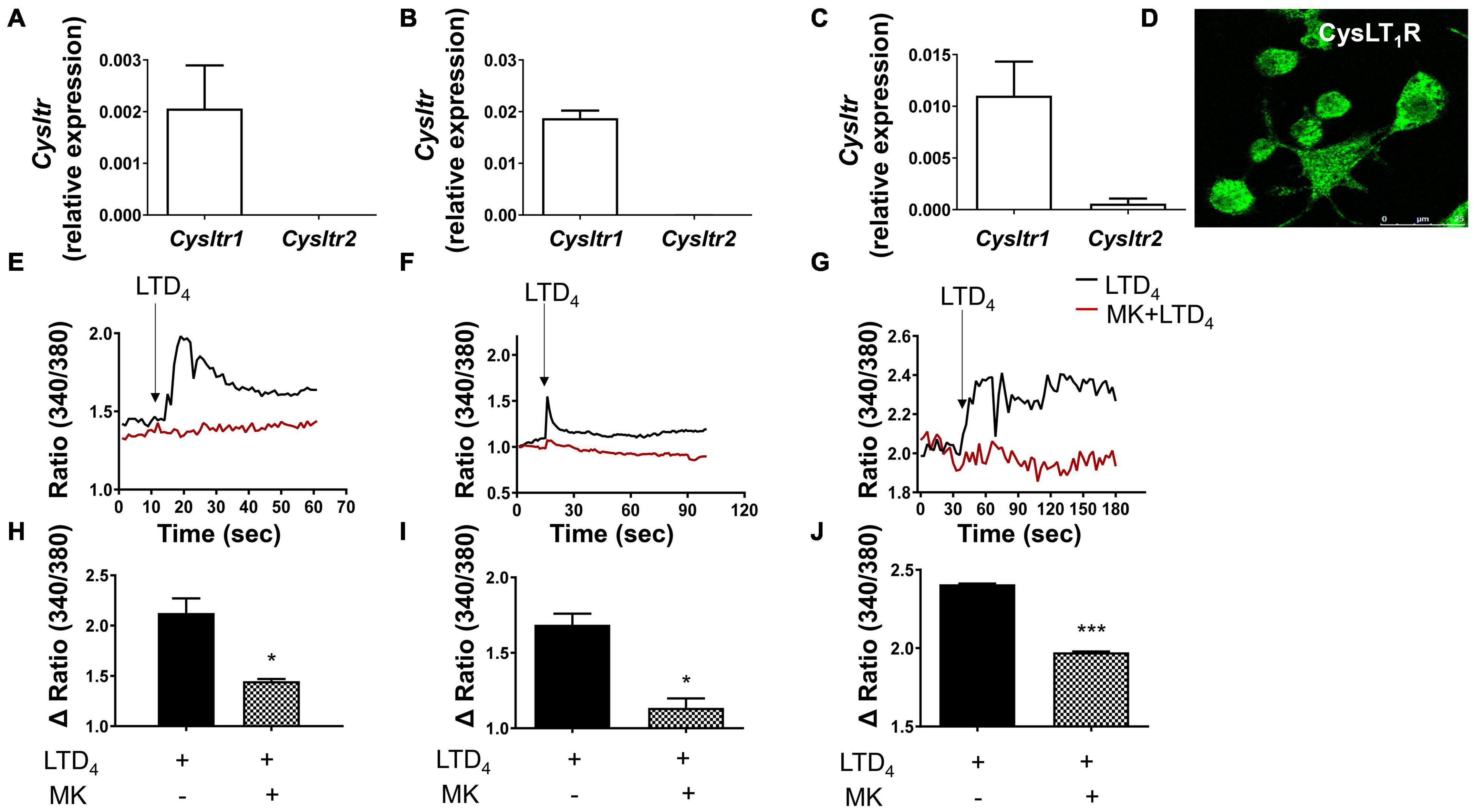
Figure 1. Cys-LTs induce calcium flux in macrophages through CysLT1R. The expression of CysLT1R and CysLT2R transcript was analyzed in (A) raw macrophages, (B) THP-1-derived macrophages, and (C) BMDMs by qPCR. (D) Immune-staining of raw macrophages for CysLT1R expression. Macrophages were loaded with Fura-2-AM, stimulated with LTD4 (0.5 μM), and then calcium flux was measured in (E) raw macrophages, (F) THP-1-derived macrophages, and (G) BMDM in the presence or absence of CysLT1R antagonist MK. Panels (H–J) represent quantification of data from panels (E–G), respectively. The results shown are mean ± SEM from three independent experiments (Student’s t-test, *p ≤ 0.05 and ***p ≤ 0.001).
Phagocytosis in Response to Leukotriene D4 in Macrophages
To explore the phagocytic ability of macrophages in response to LTD4, we treated raw macrophages and BMDMs with 0.5 μM LTD4 for 24 h, and then performed phagocytosis assay using Texas red conjugated zymosan particles. LTD4 increased the phagocytosis of zymosan particles in raw macrophages (Figures 2A,B). Although BMDM exhibited higher basal phagocytosis compared to raw macrophages, LTD4 significantly potentiated phagocytosis in these macrophages (Figures 2C,D).
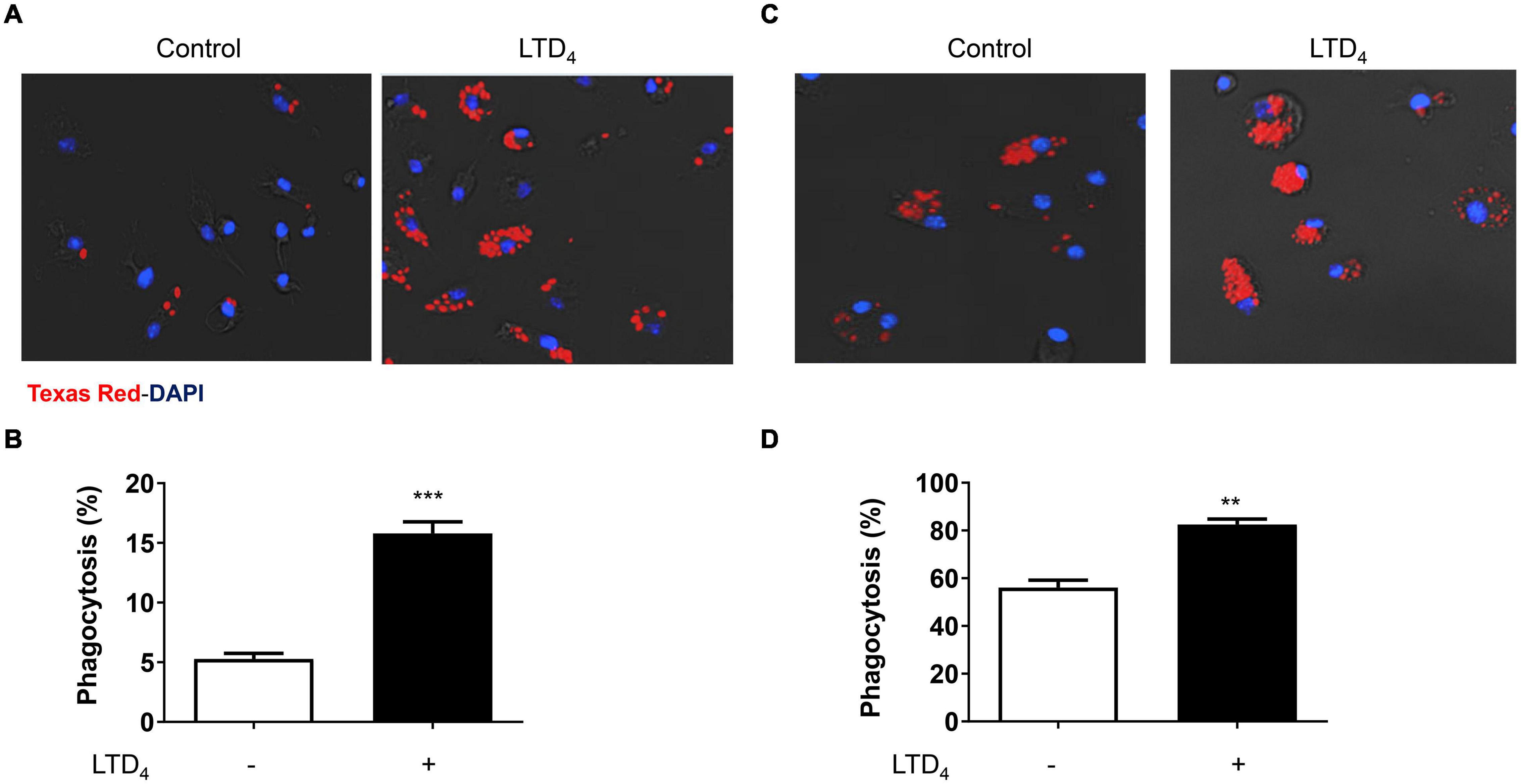
Figure 2. Cys-LTs enhance phagocytosis in macrophages. Fluorescence micrographs showing zymosan particle phagocytosis in (A) raw macrophages and (C) BMDMs. Macrophages were treated with 0.5 μM LTD4 for 24 h and incubated with zymosan particles (1:10) for 1 h. Images were quantified using ImageJ. Panels (B,D) represent the quantification of raw macrophages and BMDMs, respectively. The results shown are mean ± SEM from three experiments performed (Student’s t-test, **p ≤ 0.01 ***p ≤ 0.001).
Effect of Leukotriene D4 on Oxidized LDL Uptake in Macrophages
To determine whether LTD4 can modulate the uptake of oxLDL, macrophages were subjected to LTD4 for 24 h followed by incubation with oxLDL for another hour. The uptake of oxLDL was determined by staining with oil red O. We observed enhanced uptake of oxLDL when macrophages were treated with LTD4, as visualized by oil red O staining (Figures 3A,B). Notably, CysLT1R antagonist MK abrogated this response, suggesting that LTD4 potentiates oxLDL uptake via CysLT1R. In agreement, BMDM lacking CysLT1R exhibited an attenuated oxLDL uptake compared to WT and CysLT2R-deficient BMDMs (Figure 3C).
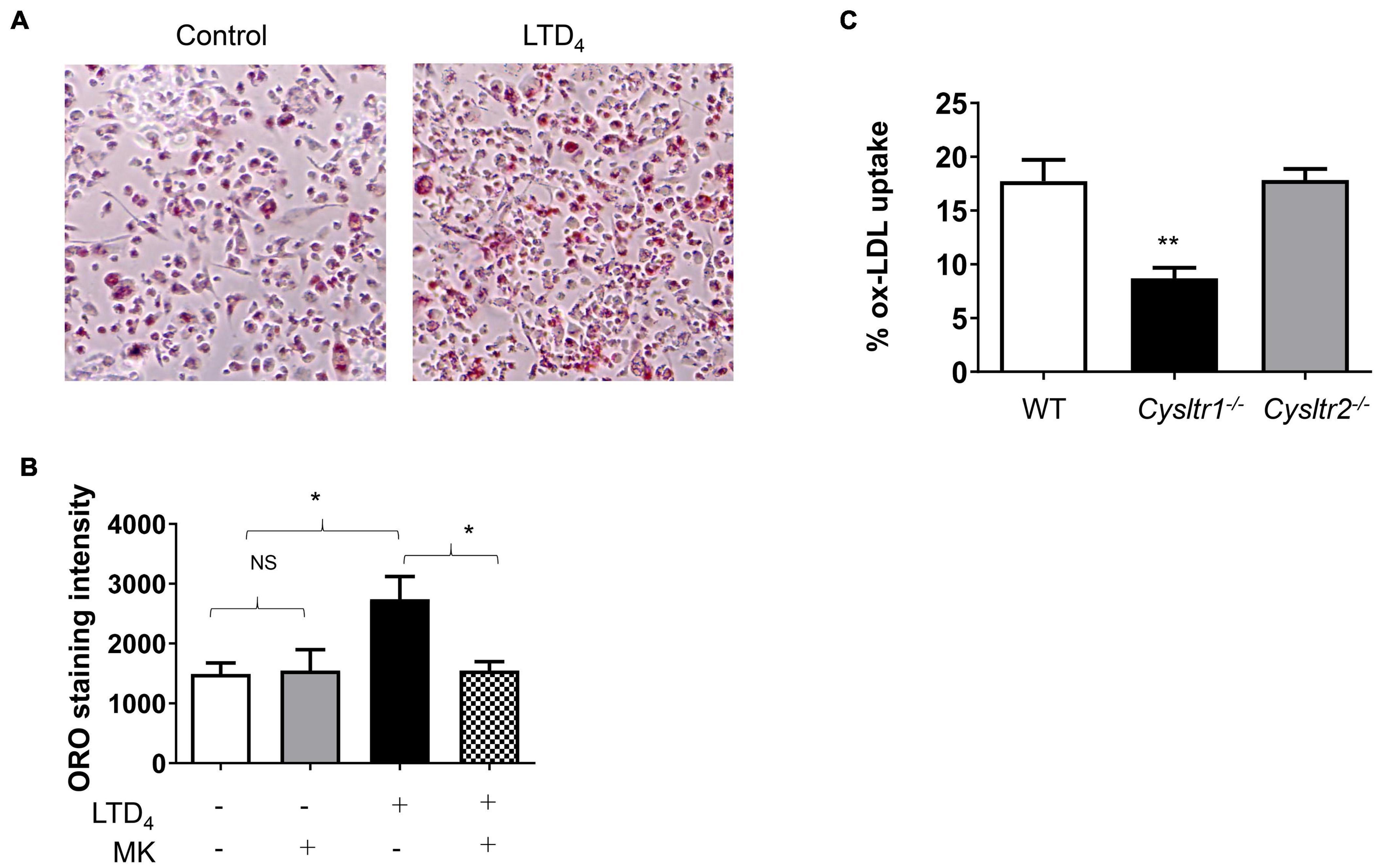
Figure 3. Cys-LTs induce oxLDL uptake in macrophages. Representative images showing oxLDL uptake in macrophages. Cells were treated with 0.5 μM LTD4 for 24 h in the presence or absence of CysLT1R antagonist MK (1 μM) and then incubated with oxLDL for 1 h. Thereafter, cells were stained with oil red-O (ORO), imaged and quantified using ImageJ. (A) Images of control and LTD4-treated cells depicting positive ORO staining, (B) quantification of ORO staining in macrophages pre-treated with or without MK and treated with or without LTD4. (C) Bone marrow cells were isolated from WT, Cysltr1– /– , and Cysltr2– /– mice and then cultured for 6 days with M-CSF to differentiate them into macrophages. BMDMs were incubated with fluorescently labeled oxLDL for 1 h and the oxLDL uptake was measured by analyzing the fluorescence incorporated into the cells using BioTek microplate reader. Results shown are mean ± SEM from three separate experiments (one-way ANOVA followed by post hoc Tukey multiple comparison test, *p ≤ 0.05, **p ≤ 0.01, ns = not significant).
Leukotriene D4-Induced Changes in Oxidized LDL Receptors
Macrophages are known for their receptor-mediated phagocytosis to ingest extracellular particles (Guest et al., 2007). Because LTD4 enhances phagocytosis and oxLDL uptake, we examined if LTD4 promotes the expression of scavenger receptors. We treated macrophages with LTD4 and analyzed the mRNA expression of receptors known to be involved in phagocytosis by qPCR. OLR1 transcript was upregulated with LTD4 in a dose-dependent manner (Figure 4A). Similarly, LTD4 caused up-regulation of CD36 transcript (Figure 5A), starting from 0.1 μM and sustained with increasing doses. Temporally, OLR1 mRNA upregulation by LTD4 was relatively early, peaking at 6 h and declined later (Figure 4B). In contrast, CD36 transcript was enhanced starting 6 h and sustained till 24 h (Figure 5B). Reflecting our transcript data, we observed increase in OLR1 protein at 6 and 12 h of LTD4 treatment and declined by 24 h (Figures 4C,D). Similarly, CD36 protein expression is augmented by LTD4 treatment starting at 6 h with a significant increase at 12 and 24 h (Figures 5C,D).
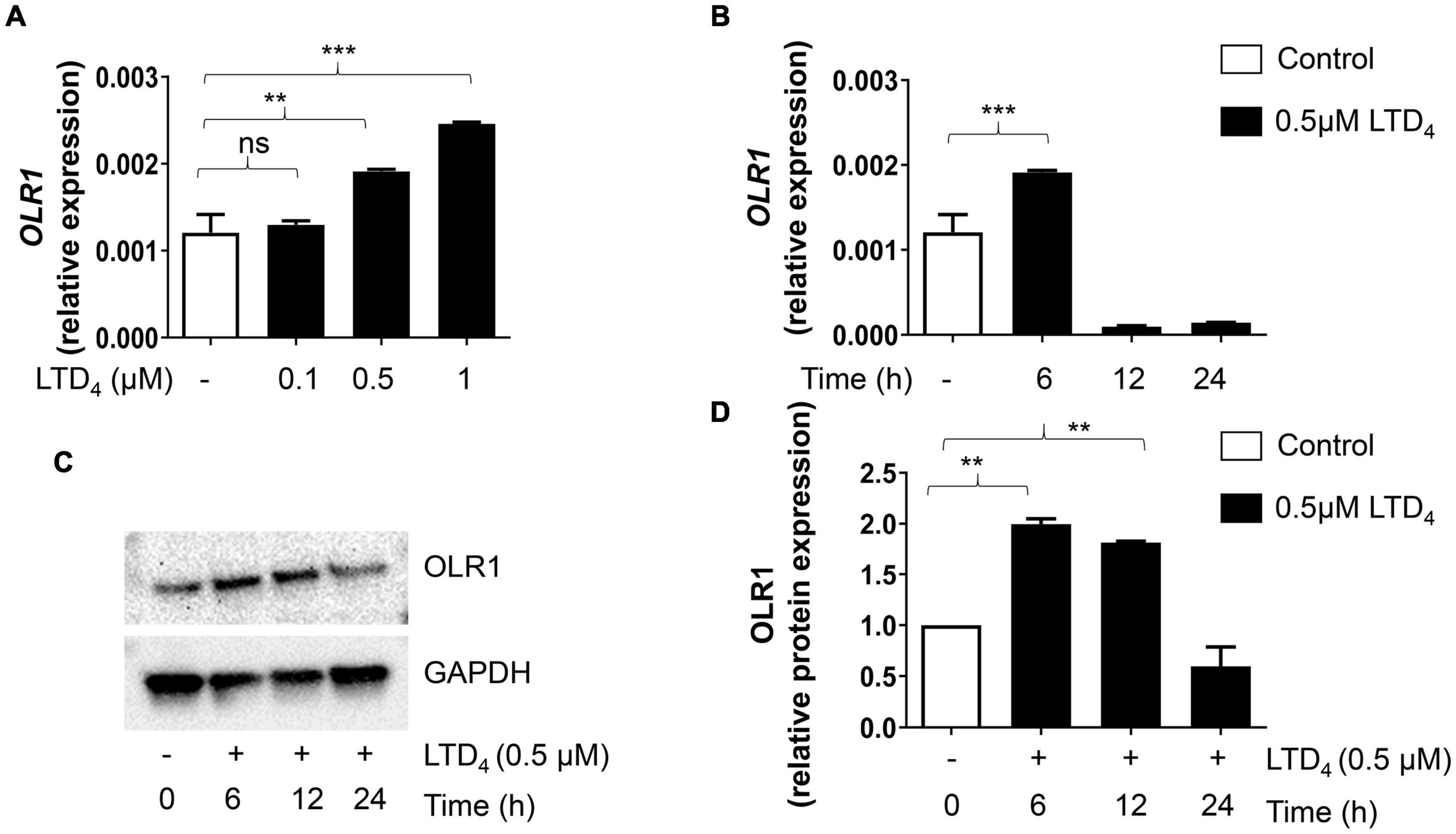
Figure 4. Cys-LTs induce upregulation of OLR1 transcript and protein. Raw macrophages were treated with increasing concentrations of LTD4 for 6, 12, and 24 h, and the expressions of OLR1 transcript (A,B) was analyzed using qPCR and protein expression was analyzed by Western blotting (C,D). The results shown are mean ± SEM from three separate experiments (one-way ANOVA followed by post hoc Tukey multiple comparison test, **p ≤ 0.01, ***p ≤ 0.001, ns = not significant).
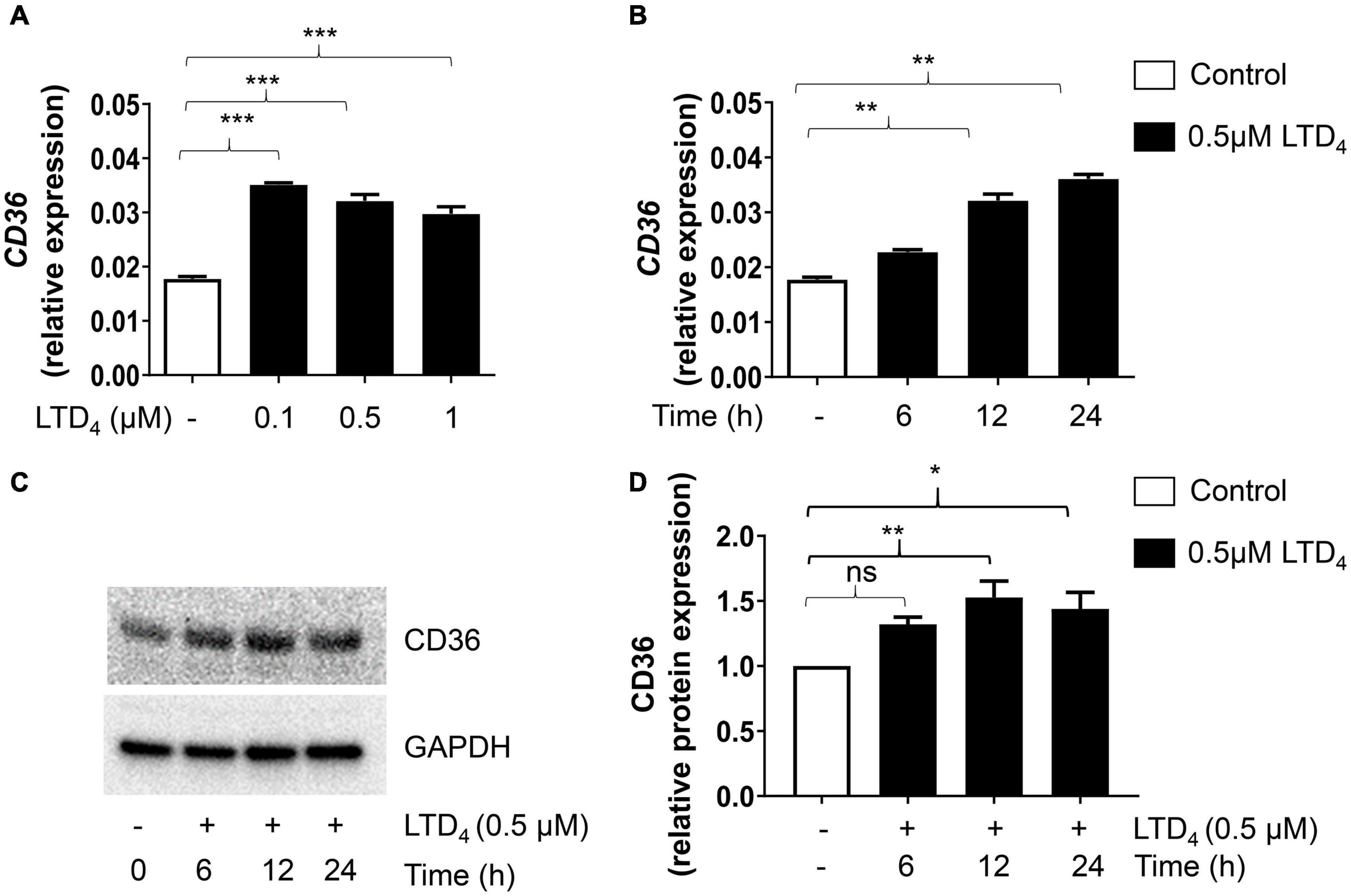
Figure 5. Cys-LTs induce upregulation of CD36 transcript and protein. Raw macrophages were treated with increasing concentrations of LTD4 for 6, 12, and 24 h, and the expressions of CD36 transcript (A,B) was analyzed using qPCR and protein expression was analyzed by Western blotting (C,D). The results shown are mean ± SEM from three separate experiments (one-way ANOVA followed by post hoc Tukey multiple comparison test, *p ≤ 0.05, **p ≤ 0.01, ***p ≤ 0.001, ns = not significant).
Induction of Monocyte Chemoattractant Protein-1 by Leukotriene D4
MCP-1 (CCL-2) has been associated with atherosclerosis via increasing foam cell load in the intima of the blood vessels (Lin et al., 2014). We asked whether LTD4 induces MCP-1 expression by macrophages. Real-time PCR analysis showed that LTD4 stimulation of raw macrophages induced the expression of MCP-1 transcripts at all doses tested (Figure 6A). Further. LTD4-potentiated MCP-1 transcript peaked at 12 h and sustained till 24 h (Figure 6B). Consistent with mRNA data, LTD4 induced MCP-1 expression at the protein level as determined by ELISA, sensitive to MK571 (Figure 6C). Notably, we found similar potentiation of MCP-1 and MIP1β in BMDMs (Figures 6D,E).
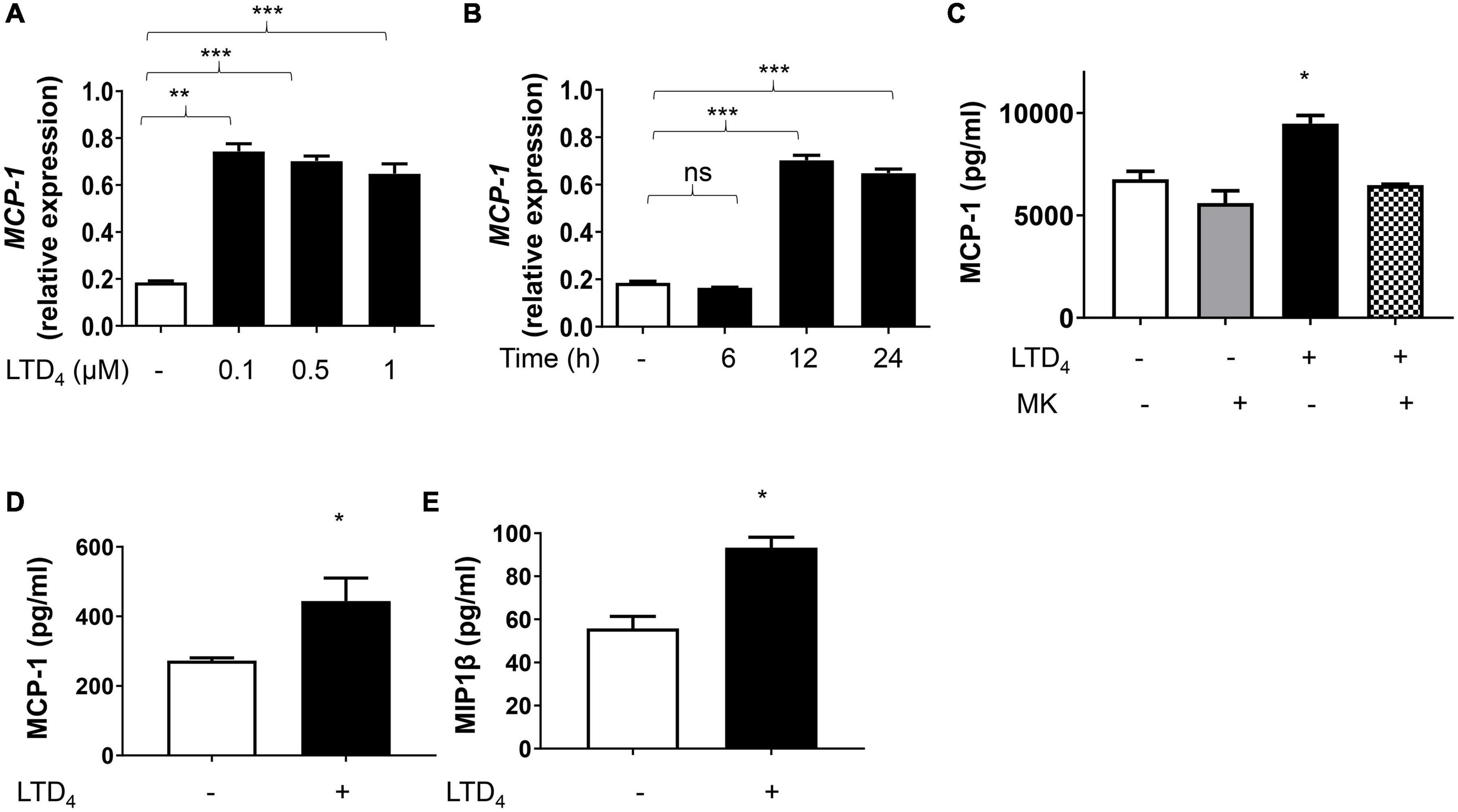
Figure 6. CysLTs enhance MCP-1 production in macrophages. Raw macrophages were treated with increasing concentrations of LTD4 (A), for 6, 12, and 24 h (B), and MCP-1 transcript was analyzed using qPCR. In panel (C), raw macrophages were pre-incubated for 30 min in the presence or absence of MK571, treated with 0.5 μM LTD4 for 6 h and supernatants were collected and analyzed for MCP-1. BMDMs (D,E) were treated with 0.5 μM LTD4 for 6 h, supernatants were collected and (D) MCP-1 protein and (E) MIP1β protein in the supernatants were analyzed by ELISA according to the manufacturer’s instructions. The results shown are mean ± SEM from three experiments (one-way ANOVA followed by post hoc Tukey multiple comparison test, *p ≤ 0.05, **p ≤ 0.01 ***p ≤ 0.001, ns = not significant).
Discussion
5-Lipoxygenase metabolites have been implicated to play an important role in phagocytosis of macrophages (Serezani et al., 2011), and they are associated with inflammatory diseases like atherosclerosis (Back and Hansson, 2006). The 5-LO pathway has been demonstrated to be abundantly expressed in the arterial walls of patients suffering from various lesion stages of atherosclerosis of the aorta, with an increased number of 5-LO expressing cells (macrophages, dendritic cells, foam cells, mast cells, and neutrophilic granulocytes) in advanced lesions (Spanbroek et al., 2003). Notably, mice deficient in 5-LO were reported to exhibit reduced lesions in LDLR–/– background, suggesting that leukotrienes may play a dominant role in atherogenesis (Mehrabian et al., 2002). LTB4, also a 5-LO metabolite, was shown to play vital roles during atherogenesis via its receptors, BLT-1 and BLT-2 (Subbarao et al., 2004). Although the involvement of the 5-LO pathway in mediating atherosclerosis is convincing, the role of CysLTR and associated signaling in modulating macrophage function and atherosclerosis still remains elusive. Macrophages are not only equipped with all the essential enzymes to synthesize cys-LTs in response to various agonists, but also possess the relevant receptors to facilitate autocrine signaling. Therefore, it is vital to understand how cys-LTs modulate macrophage function. Previous studies from our lab suggest that CysLTR signaling causes endothelial dysfunction and potentiates the attachment of monocytes to EC in response to TNFα (Duah et al., 2013). Based on these findings, we speculated that cys-LTs generated at the site of inflammation may also trigger macrophage dysfunction and contribute to atherosclerosis. To address this, we first confirmed the CysLTR expression in three different macrophage populations. We found that macrophages mainly express CysLT1R compared to CysLT2R, in agreement with the literature (Lotzer et al., 2003). Since CysLT1R couples to Gαq in many systems, generating calcium flux upon activation (Lynch et al., 1999), we measured intracellular calcium in macrophages in response to LTD4 and confirmed that macrophages mainly flux calcium in response to LTD4 via CysLT1R, employing CysLT1R antagonist MK. We next asked what effect this receptor has in modulating macrophage phagocytosis. Macrophages play a vital role in the phagocytosis of infectious agents, pathogens, and debris during inflammation, which is crucial for maintaining cellular homeostasis (Han et al., 2016). We observed that LTD4 significantly promoted phagocytosis of zymosan bioparticles in both raw macrophages and BMDMs, although BMDMs exhibited enhanced basal phagocytosis compared to raw macrophages. Endothelial dysfunction leading to lipid modification is perceived as a danger signal by the macrophages, and they function by engulfing these cholesterol-rich lipid molecules, leading to the formation of lipid-laden foam cells (Tabas and Bornfeldt, 2016). Our previous study demonstrated that cys-LTs cause endothelial cell (EC) dysfunction such as EC contraction, gap formation, and attachment of monocytes to the endothelium (Duah et al., 2013). Notably, LTB4 (Zhang et al., 2017) and cys-LTs (Yu et al., 2014) have been shown to be involved in the recruitment of immune cells to the site of inflammation, enhancing phagocytosis. Further, the enhanced expression of 5-LO and cys-LTs have been shown in atherosclerotic lesions, suggesting their potential role in plaque instability and atherosclerosis progression (Qiu et al., 2006). Based on these studies, we wondered about the role of the LTD4/CysLT1R axis on oxLDL uptake in macrophages. Our results demonstrate that LTD4 via CysLT1R enhanced the uptake of oxLDL in macrophages. BMDMs lacking CysLT1R exhibited an attenuated uptake compared to WT and CysLT2R null BMDMs, further suggesting an important role of cys-LTs in engulfing oxidized lipids. We further explored the mechanism and relevant cell surface oxLDL receptors activated by LTD4, which are responsible for lipid accumulation and foam cells in macrophages. OxLDL acts via binding to several receptors, including CD36, and OLR1, Peroxisome proliferator-activated receptor-gamma coactivator 1 α (PGC-1α), and SRA mediating lipid accumulation (Febbraio et al., 2000; Guest et al., 2007; Patten and Shetty, 2018). We observed the upregulation of OLR1 and CD36 in response to LTD4. Notably, we could not detect the upregulation of other scavenger receptors like PGC1α and SRA1 by LTD4 (not shown), suggesting that LTD4 signaling is relayed mainly via CD36 and OLR1, contributing to enhanced uptake of lipid molecules. OLR1 is a membrane glycoprotein that can selectively bind and internalize oxLDL (Ogura et al., 2009). Several inflammatory and atherosclerosis-related stimuli have been shown to induce OLR1 expression, including lipopolysaccharide (LPS), TNFα, interleukin-1 (IL-1), interferon gamma (IFNγ), oxLDL, and angiotensin II (Xu et al., 2013). CD36 belongs to the class B scavenger receptor family, and it is expressed on various cell types, including macrophages, platelets, and microvascular EC (Park, 2014). CD36-null mice were shown to exhibit increased cholesterol, triacylglycerol, and fatty acids in the plasma level, suggesting a major role of CD36 in fatty acid uptake and lipid metabolism in vivo (Febbraio et al., 1999).
Apart from the above mentioned receptors, chemokine CCL2/MCP-1 is a critical mediator of atherosclerosis, and the absence of MCP-1 has been shown to reduce atherosclerosis in low-density lipoprotein receptor-deficient mice (Gu et al., 1998). In support, MCP-1 expression was observed in human and rabbit atherosclerotic plaques (Yla-Herttuala et al., 1991), and a reduction in arterial lipid deposition was observed in CCL2 deficient mice (Boring et al., 1998). MCP-1 null mice were shown to have severe defects in monocyte recruitment to inflammatory sites (Gu et al., 1998), suggesting that MCP-1 plays an essential role in monocyte/macrophage populations. Interestingly, LTD4 was shown to up-regulate MCP-1 in human monocytes and macrophages (Ichiyama et al., 2005). This prompted us to analyze if LTD4 signaling to lipid uptake required MCP-1. We observed an enhanced MCP-1 expression in response to LTD4, both at the transcript and protein level.
Conclusion
In conclusion, our study demonstrated a role for cys-LT/CysLT1R in upregulating CD36 and OLR1 receptors and MCP-1, and subsequent uptake of oxidized lipid molecules (Figure 7). All these events are crucial for foam cell formation during atherosclerosis. CysLT1R antagonists are FDA-approved and have been widely used in the therapy of asthma for the past few decades, with minimal side effects. Our study further suggests that these drugs may be repurposed for the treatment of atherosclerosis.
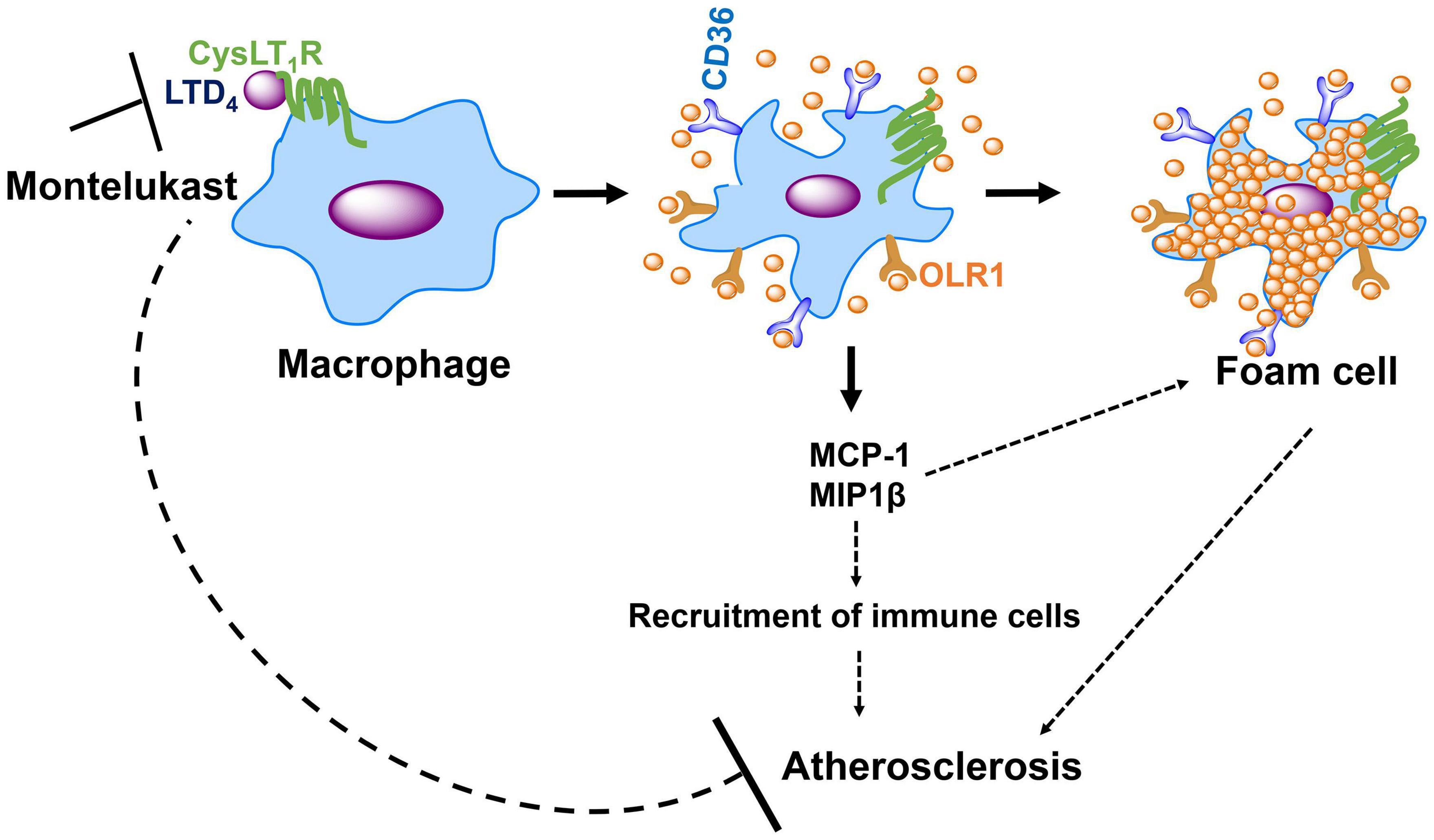
Figure 7. Schematic, suggests the role for CysLT1R in macrophage activation and atherosclerosis. LTD4 stimulation induces the upregulation of oxLDL receptors, OLR1, and CD36 via CysLT1R, which in turn facilitates oxLDL uptake in macrophages, resulting in foam cell formation. LTD4 also induces the secretion of MCP-1 and MIP1β in macrophages, which further recruits immune cells, amplifying inflammation. These events can lead to atherosclerosis, and our study suggests that CysLT1R antagonist, MK (Montelukast) can be used as a novel therapeutic target for the treatment of atherosclerosis.
Data Availability Statement
The raw data supporting the conclusions of this article will be made available by the authors, without undue reservation.
Ethics Statement
The animal study was reviewed and approved by the Animal Care and Use Committee of University of Akron.
Author Contributions
SPo, RG, LT, and ED performed the experiments, analyzed the data, and edited the manuscript. CT designed the experiments and edited the manuscript. SPa designed the experiments, performed the research, analyzed and interpreted the data, and wrote the manuscript. All authors contributed to the article and approved the submitted version.
Funding
This work was supported by James Foght Professor support (University of Akron), NIH R01AI144115 (SPa), and NIH R01HL148585 (CT).
Conflict of Interest
The authors declare that the research was conducted in the absence of any commercial or financial relationships that could be construed as a potential conflict of interest.
Publisher’s Note
All claims expressed in this article are solely those of the authors and do not necessarily represent those of their affiliated organizations, or those of the publisher, the editors and the reviewers. Any product that may be evaluated in this article, or claim that may be made by its manufacturer, is not guaranteed or endorsed by the publisher.
Abbreviations
CysLT1R, Cysteinyl Leukotriene 1 Receptor; CysLT2R, Cysteinyl Leukotriene 2 Receptor; BMDM, Bone marrow-derived macrophages; LTD4, Leukotriene D4; OLR1, Oxidized low-density lipoprotein receptor 1; LDL, Low density lipoprotein; MCP-1, Monocyte chemoattractant protein-1; MIP1 β, Macrophage Inflammatory protein-1 β.
References
Aderem, A., and Underhill, D. M. (1999). Mechanisms of phagocytosis in macrophages. Annu. Rev. Immunol. 17, 593–623. doi: 10.1146/annurev.immunol.17.1.593
Arslan, C., Bayoglu, B., Tel, C., Cengiz, M., Dirican, A., and Besirli, K. (2017). Upregulation of OLR1 and IL17A genes and their association with blood glucose and lipid levels in femoropopliteal artery disease. Exp. Ther. Med. 13, 1160–1168. doi: 10.3892/etm.2017.4081
Back, M. (2009). Leukotriene signaling in atherosclerosis and ischemia. Cardiovasc. Drugs Ther. 23, 41–48. doi: 10.1007/s10557-008-6140-9
Back, M., and Hansson, G. K. (2006). Leukotriene receptors in atherosclerosis. Ann. Med. 38, 493–502.
Boring, L., Gosling, J., Cleary, M., and Charo, I. F. (1998). Decreased lesion formation in CCR2-/- mice reveals a role for chemokines in the initiation of atherosclerosis. Nature 394, 894–897. doi: 10.1038/29788
Busse, W. W. (1996). The role of leukotrienes in asthma and allergic rhinitis. Clin. Exp. Allergy 26, 868–879.
Chung, K. F. (1995). Leukotriene receptor antagonists and biosynthesis inhibitors: potential breakthrough in asthma therapy. Eur. Respir. J. 8, 1203–1213. doi: 10.1183/09031936.95.08071203
Davidson, A. B., Lee, T. H., Scanlon, P. D., Solway, J., McFadden, E. R. Jr., and Ingram, R. H. Jr., et al. (1987). Bronchoconstrictor effects of leukotriene E4 in normal and asthmatic subjects. Am. Rev. Respir. Dis. 135, 333–337.
Drazen, J. M., and Austen, K. F. (1987). Leukotrienes and airway responses. Am. Rev. Respir. Dis. 136, 985–998. doi: 10.1164/ajrccm/136.4.985
Duah, E., Adapala, R. K., Al-Azzam, N., Kondeti, V., Gombedza, F., Thodeti, C. K., et al. (2013). Cysteinyl leukotrienes regulate endothelial cell inflammatory and proliferative signals through CysLT(2) and CysLT(1) receptors. Sci. Rep. 3:3274. doi: 10.1038/srep03274
Duah, E., Teegala, L. R., Kondeti, V., Adapala, R. K., Keshamouni, V. G., Kanaoka, Y., et al. (2019). Cysteinyl leukotriene 2 receptor promotes endothelial permeability, tumor angiogenesis, and metastasis. Proc. Natl. Acad. Sci. U. S. A. 116, 199–204. doi: 10.1073/pnas.1817325115
Evans, J. F. (2002). Cysteinyl leukotriene receptors. Prostaglandins Other Lipid Mediat. 68-69, 587–597.
Febbraio, M., Abumrad, N. A., Hajjar, D. P., Sharma, K., Cheng, W., Pearce, S. F., et al. (1999). A null mutation in murine CD36 reveals an important role in fatty acid and lipoprotein metabolism. J. Biol. Chem. 274, 19055–19062. doi: 10.1074/jbc.274.27.19055
Febbraio, M., Podrez, E. A., Smith, J. D., Hajjar, D. P., Hazen, S. L., Hoff, H. F., et al. (2000). Targeted disruption of the class B scavenger receptor CD36 protects against atherosclerotic lesion development in mice. J. Clin. Invest. 105, 1049–1056. doi: 10.1172/JCI9259
Feig, J. E., Parathath, S., Rong, J. X., Mick, S. L., Vengrenyuk, Y., Grauer, L., et al. (2011a). Reversal of hyperlipidemia with a genetic switch favorably affects the content and inflammatory state of macrophages in atherosclerotic plaques. Circulation 123, 989–998. doi: 10.1161/CIRCULATIONAHA.110.984146
Feig, J. E., Rong, J. X., Shamir, R., Sanson, M., Vengrenyuk, Y., Liu, J., et al. (2011b). HDL promotes rapid atherosclerosis regression in mice and alters inflammatory properties of plaque monocyte-derived cells. Proc. Natl. Acad. Sci. U. S. A. 108, 7166–7171. doi: 10.1073/pnas.1016086108
Gu, L., Okada, Y., Clinton, S. K., Gerard, C., Sukhova, G. K., Libby, P., et al. (1998). Absence of monocyte chemoattractant protein-1 reduces atherosclerosis in low density lipoprotein receptor-deficient mice. Mol. cell 2, 275–281.
Guest, C. B., Hartman, M. E., O’Connor, J. C., Chakour, K. S., Sovari, A. A., and Freund, G. G. (2007). Phagocytosis of cholesteryl ester is amplified in diabetic mouse macrophages and is largely mediated by CD36 and SR-A. PLoS One 2:e511. doi: 10.1371/journal.pone.0000511
Han, C. Z., Juncadella, J. I., Kinchen, J. M., Buckley, M. W., Klibanov, A. L., Dryden, K., et al. (2016). Macrophages redirect phagocytosis by non-professional phagocytes and influence inflammation. Nature 539, 570–574. doi: 10.1038/nature20141
Hansson, G. K., and Hermansson, A. (2011). The immune system in atherosclerosis. Nat. Immunol. 12, 204–212.
Heise, C. E., O’Dowd, B. F., Figueroa, D. J., Sawyer, N., Nguyen, T., Im, D. S., et al. (2000). Characterization of the human cysteinyl leukotriene 2 receptor. J. Biol. Chem. 275, 30531–30536.
Ichiyama, T., Hasegawa, M., Ueno, Y., Makata, H., Matsubara, T., and Furukawa, S. (2005). Cysteinyl leukotrienes induce monocyte chemoattractant protein 1 in human monocytes/macrophages. Clin. Exp. Allergy 35, 1214–1219. doi: 10.1111/j.1365-2222.2005.02323.x
Kanaoka, Y., and Boyce, J. A. (2004). Cysteinyl leukotrienes and their receptors: cellular distribution and function in immune and inflammatory responses. J. Immunol. 173, 1503–1510. doi: 10.4049/jimmunol.173.3.1503
Kanaoka, Y., Maekawa, A., and Austen, K. F. (2013). Identification of GPR99 protein as a potential third cysteinyl leukotriene receptor with a preference for leukotriene E4 ligand. J. Biol. Chem. 288, 10967–10972. doi: 10.1074/jbc.C113.453704
Kelley, J. L., Ozment, T. R., Li, C., Schweitzer, J. B., and Williams, D. L. (2014). Scavenger receptor-A (CD204): a two-edged sword in health and disease. Crit. Rev. Immunol. 34, 241–261. doi: 10.1615/critrevimmunol.2014010267
Kondeti, V., Al-Azzam, N., Duah, E., Thodeti, C. K., Boyce, J. A., and Paruchuri, S. (2016). Leukotriene D4 and prostaglandin E2 signals synergize and potentiate vascular inflammation in a mast cell-dependent manner through cysteinyl leukotriene receptor 1 and E-prostanoid receptor 3. J. Allergy Clin. Immunol. 137, 289–298. doi: 10.1016/j.jaci.2015.06.030
Kondeti, V., Duah, E., Al-Azzam, N., Thodeti, C. K., Boyce, J. A., and Paruchuri, S. (2013). Differential regulation of cysteinyl leukotriene receptor signaling by protein kinase C in human mast cells. PLoS One 8:e71536. doi: 10.1371/journal.pone.0071536
Lin, J., Kakkar, V., and Lu, X. (2014). Impact of MCP-1 in atherosclerosis. Current Pharm. Des. 20, 4580–4588. doi: 10.2174/1381612820666140522115801
Liu, M., and Yokomizo, T. (2015). The role of leukotrienes in allergic diseases. Allergol. Int. 64, 17–26. doi: 10.1016/j.alit.2014.09.001
Lotzer, K., Spanbroek, R., Hildner, M., Urbach, A., Heller, R., Bretschneider, E., et al. (2003). Differential leukotriene receptor expression and calcium responses in endothelial cells and macrophages indicate 5-lipoxygenase-dependent circuits of inflammation and atherogenesis. Arterioscler. Thromb. Vasc. Biol. 23, e32–6. doi: 10.1161/01.ATV.0000082690.23131.CB
Lynch, K. R., O’Neill, G. P., Liu, Q., Im, D. S., Sawyer, N., Metters, K. M., et al. (1999). Characterization of the human cysteinyl leukotriene CysLT1 receptor. Nature 399, 789–793.
Maekawa, A., Balestrieri, B., Austen, K. F., and Kanaoka, Y. (2009). GPR17 is a negative regulator of the cysteinyl leukotriene 1 receptor response to leukotriene D4. Proc. Natl. Acad. Sci. U. S. A. 106, 11685–11690.
Maekawa, A., Kanaoka, Y., Xing, W., and Austen, K. F. (2008). Functional recognition of a distinct receptor preferential for leukotriene E4 in mice lacking the cysteinyl leukotriene 1 and 2 receptors. Proc. Natl. Acad. Sci. U. S. A. 105, 16695–16700. doi: 10.1073/pnas.0808993105
Mehrabian, M., Allayee, H., Wong, J., Shi, W., Wang, X. P., Shaposhnik, Z., et al. (2002). Identification of 5-lipoxygenase as a major gene contributing to atherosclerosis susceptibility in mice. Circ. Res. 91, 120–126. doi: 10.1161/01.res.0000028008.99774.7f
Moore, K. J., Sheedy, F. J., and Fisher, E. A. (2013). Macrophages in atherosclerosis: a dynamic balance. Nat. Rev. Immunol. 13, 709–721. doi: 10.1038/nri3520
Nguyen, M. T., Fernando, S., Schwarz, N., Tan, J. T., Bursill, C. A., and Psaltis, P. J. (2019). Inflammation as a therapeutic target in atherosclerosis. J. Clin. Med. 8:1109.
Ogura, S., Kakino, A., Sato, Y., Fujita, Y., Iwamoto, S., Otsui, K., et al. (2009). Lox-1: the multifunctional receptor underlying cardiovascular dysfunction. Circ. J. 73, 1993–1999. doi: 10.1253/circj.cj-09-0587
Park, Y. M. (2014). CD36, a scavenger receptor implicated in atherosclerosis. Exp. Mol. Med. 46:e99. doi: 10.1038/emm.2014.38
Paruchuri, S., Jiang, Y., Feng, C., Francis, S. A., Plutzky, J., and Boyce, J. A. (2008). Leukotriene E4 activates peroxisome proliferator-activated receptor gamma and induces prostaglandin D2 generation by human mast cells. J. Biol. Chem. 283, 16477–16487. doi: 10.1074/jbc.M705822200
Paruchuri, S., Tashimo, H., Feng, C., Maekawa, A., Xing, W., Jiang, Y., et al. (2009). Leukotriene E4-induced pulmonary inflammation is mediated by the P2Y12 receptor. J. Exp. Med. 206, 2543–2555. doi: 10.1084/jem.20091240
Patten, D. A., and Shetty, S. (2018). More than just a removal service: scavenger receptors in leukocyte trafficking. Front. Immunol. 9:2904. doi: 10.3389/fimmu.2018.02904
Qiu, H., Gabrielsen, A., Agardh, H. E., Wan, M., Wetterholm, A., Wong, C. H., et al. (2006). Expression of 5-lipoxygenase and leukotriene A4 hydrolase in human atherosclerotic lesions correlates with symptoms of plaque instability. Proc. Natl. Acad. Sci. U. S. A. 103, 8161–8166. doi: 10.1073/pnas.0602414103
Serezani, C. H., Lewis, C., Jancar, S., and Peters-Golden, M. (2011). Leukotriene B4 amplifies NF-kappaB activation in mouse macrophages by reducing SOCS1 inhibition of MyD88 expression. J. Clin. Invest. 121, 671–682. doi: 10.1172/JCI43302
Soter, N. A., Lewis, R. A., Corey, E. J., and Austen, K. F. (1983). Local effects of synthetic leukotrienes (LTC4, LTD4, LTE4, and LTB4) in human skin. J. Invest. Dermatol. 80, 115–119.
Spanbroek, R., Grabner, R., Lotzer, K., Hildner, M., Urbach, A., Ruhling, K., et al. (2003). Expanding expression of the 5-lipoxygenase pathway within the arterial wall during human atherogenesis. Proc. Natl. Acad. Sci. U. S. A. 100, 1238–1243. doi: 10.1073/pnas.242716099
Subbarao, K., Jala, V. R., Mathis, S., Suttles, J., Zacharias, W., Ahamed, J., et al. (2004). Role of leukotriene B4 receptors in the development of atherosclerosis: potential mechanisms. Arterioscler. Thromb. Vasc. Biol. 24, 369–375. doi: 10.1161/01.ATV.0000110503.16605.15
Tabas, I., and Bornfeldt, K. E. (2016). Macrophage Phenotype and Function in Different Stages of Atherosclerosis. Circ. Res. 118, 653–667. doi: 10.1161/circresaha.115.306256
Woo, M. S., Yang, J., Beltran, C., and Cho, S. (2016). Cell Surface CD36 Protein in Monocyte/Macrophage Contributes to Phagocytosis during the Resolution Phase of Ischemic Stroke in Mice. J. Biol. Chem. 291, 23654–23661. doi: 10.1074/jbc.M116.750018
Xu, S., Ogura, S., Chen, J., Little, P. J., Moss, J., and Liu, P. (2013). LOX-1 in atherosclerosis: biological functions and pharmacological modifiers. Cel. Mol. Life Sci. 70, 2859–2872. doi: 10.1007/s00018-012-1194-z
Yla-Herttuala, S., Lipton, B. A., Rosenfeld, M. E., Sarkioja, T., Yoshimura, T., Leonard, E. J., et al. (1991). Expression of monocyte chemoattractant protein 1 in macrophage-rich areas of human and rabbit atherosclerotic lesions. Proc. Natl. Acad. Sci. U. S. A. 88, 5252–5256. doi: 10.1073/pnas.88.12.5252
Yu, S. Y., Zhang, X. Y., Wang, X. R., Xu, D. M., Chen, L., Zhang, L. H., et al. (2014). Cysteinyl leukotriene receptor 1 mediates LTD4-induced activation of mouse microglial cells in vitro. Acta Pharmacol. Sin. 35, 33–40. doi: 10.1038/aps.2013.130
Zhang, M., Ahn, W., Kim, S., Hong, H. S., Quan, C., and Son, Y. (2017). Endothelial precursor cells stimulate pericyte-like coverage of bone marrow-derived mesenchymal stem cells through platelet-derived growth factor-BB induction, which is enhanced by substance P. Microcirculation 24:e12394. doi: 10.1111/micc.12394
Keywords: LTD4, CysLT1R, phagocytosis, oxLDL, CD36, OLR1, MCP-1, atherosclerosis
Citation: Pokhrel S, Gudneppanavar R, Teegala LR, Duah E, Thodeti CK and Paruchuri S (2021) Leukotriene D4 Upregulates Oxidized Low-Density Lipoprotein Receptor 1 and CD36 to Enhance Oxidized LDL Uptake and Phagocytosis in Macrophages Through Cysteinyl Leukotriene Receptor 1. Front. Physiol. 12:756450. doi: 10.3389/fphys.2021.756450
Received: 10 August 2021; Accepted: 14 October 2021;
Published: 18 November 2021.
Edited by:
Anna Maria Giudetti, University of Salento, ItalyReviewed by:
Gang Shu, South China Agricultural University, ChinaKameswara Rao Badri, Morehouse School of Medicine, United States
Mathumai Kanapathipillai, University of Michigan-Dearborn, United States
Copyright © 2021 Pokhrel, Gudneppanavar, Teegala, Duah, Thodeti and Paruchuri. This is an open-access article distributed under the terms of the Creative Commons Attribution License (CC BY). The use, distribution or reproduction in other forums is permitted, provided the original author(s) and the copyright owner(s) are credited and that the original publication in this journal is cited, in accordance with accepted academic practice. No use, distribution or reproduction is permitted which does not comply with these terms.
*Correspondence: Sailaja Paruchuri, c2FpbGFqYS5wYXJ1Y2h1cmlAVVRvbGVkby5lZHU=