- 1Department of Anesthesiology & Perioperative Medicine, The University of Texas MD Anderson Cancer Center, Houston, TX, United States
- 2Neuroscience Program, Graduate School of Biomedical Sciences, UT Health, The University of Texas MD Anderson Cancer Center, Houston, TX, United States
- 3Biochemistry and Cell Biology Program, Graduate School of Biomedical Sciences, UT Health, The University of Texas MD Anderson Cancer Center, Houston, TX, United States
Biochemical and functional studies of ion channels have shown that many of these integral membrane proteins form macromolecular signaling complexes by physically associating with many other proteins. These macromolecular signaling complexes ensure specificity and proper rates of signal transduction. The large-conductance, Ca2+-activated K+ (BK) channel is dually activated by membrane depolarization and increases in intracellular free Ca2+ ([Ca2+]i). The activation of BK channels results in a large K+ efflux and, consequently, rapid membrane repolarization and closing of the voltage-dependent Ca2+-permeable channels to limit further increases in [Ca2+]i. Therefore, BK channel-mediated K+ signaling is a negative feedback regulator of both membrane potential and [Ca2+]i and plays important roles in many physiological processes and diseases. However, the BK channel formed by the pore-forming and voltage- and Ca2+-sensing α subunit alone requires high [Ca2+]i levels for channel activation under physiological voltage conditions. Thus, most native BK channels are believed to co-localize with Ca2+-permeable channels within nanodomains (a few tens of nanometers in distance) to detect high levels of [Ca2+]i around the open pores of Ca2+-permeable channels. Over the last two decades, advancement in research on the BK channel’s coupling with Ca2+-permeable channels including recent reports involving NMDA receptors demonstrate exemplary models of nanodomain structural and functional coupling among ion channels for efficient signal transduction and negative feedback regulation. We hereby review our current understanding regarding the structural and functional coupling of BK channels with different Ca2+-permeable channels.
Introduction
Ca2+ and K+ Signaling via Ion Channels
Cells need to sense and respond to changes in the extracellular environment and communicate with adjoining and distant cells. Cells use different signaling molecules to carry out these tasks. Ca2+ and K+ cations are two signaling molecules that are abundant and used for cell signaling in all living systems. Ca2+ is a prominent second messenger molecule that is involved in nearly all biochemical, cellular, and physiological processes. It is essential for proper cardiac function, the structural integrity of bone, and muscular contraction, and it acts as a substrate for enzymatic signal in biochemical pathways. Cells invest vast amounts of energy into the regulation of intracellular concentrations of free Ca2+. The speed and effectiveness of Ca2+ signaling builds upon the greater than 10,000-fold gradient between the Ca2+ concentrations inside (∼100 nM) and outside (∼2 mM) the cells. Rapid global (10-fold) and local (100-fold) increases in intracellular free Ca2+ ([Ca2+]i) concentrations can be achieved by Ca2+ influx from extracellular mediums or Ca2+ release from intracellular Ca2+ stores via the activation of Ca2+-permeable channels on the plasma membrane and intracellular organelle membranes, respectively.
Ca2+-permeable channels passively diffuse Ca2+ ions down their electrochemical gradient. There is a wide variety of Ca2+-permeable channels that differ in Ca2+ selectivity and activation mechanisms. Ca2+-selective channels include voltage-gated Ca2+ (CaV) channels, including CaV1.1-4, CaV2.1-3, and CaV3.1-3; ligand-gated Ca2+ channels, including the store-operated Ca2+ channels (SOCCs or ORAI1-3) on the plasma membrane; and inositol 1,4,5-trisphosphate (IP3) and ryanodine receptors (IP3Rs and RyRs) on the endoplasmic reticulum (ER) membrane. Non-selective cation channels include mechanosensitive piezo channels, transient receptor potential (TRP) channels, cyclic nucleotide–gated ion channels (CNGs), acid-sensing ion channels (ASICs), and ionotropic receptors, including N-methyl-D-aspartate (NMDA) receptors, serotonin 5-hydroxytryptamine (HT3) receptors, and adenosine triphosphate (ATP)–activated P2X receptors.
K+ is the most abundant cation in the intracellular fluid. The concentration of K+ ions is usually about 25-fold higher on the cytoplasmic than on the extracellular side of the plasma membrane. K+ channels selectively pass K+ ions across membrane according to electrochemical driving forces. Channel-mediating K+ signaling dynamically controls K+ distribution across the cell membrane and is critical for normal cell function. The K+ channel current dominates the ionic flow in a cell’s resting state and thus is critical for setting a cell’s resting membrane potential. K+ channels are also involved in fluid secretion and cell volume regulation. In excitable cells, the K+ channel’s activities affect the action potential firing threshold, which is determined by the balance between inward Na+ and outward K+ currents and also underlies the repolarization, hyperpolarization, and after-hyperpolarization phases of the action potential. K+ channels play a crucial role in all aspects of life by regulating the excitability of neurons and the heart, contracting muscles, secreting hormones, water homeostasis, and activating immune cells.
K+ channels are the most diverse and abundantly expressed ion channels in living organisms. These channels are expressed in most excitable and non-excitable cells and perform numerous important functions, as is evident from the large number of genes (∼80 in mammals) encoding for the K+ channels’ pore-forming subunits. K+ channels can be classified into four main groups: Ca2+–activated K+ (KCa) channels, tandem pore domain K+ (K2P) channels, voltage-gated K+ (Kv) channels, and inwardly rectifying K+ (Kir) channels. Each type of K+ channel possesses unique electrophysiological and pharmacological properties. KCa channels activate in response to increases in [Ca2+]i and thus cause changes in cell membrane potential toward the negative voltage direction via K+ efflux. This common functionality enables KCa channels to play an important role in bridging cell excitability and the intracellular calcium concentration. KCa channels are a diversified group of channels with various biophysical and pharmacological properties. KCa channels are divided into 3 classes based on their single channel conductance: big conductance (BK, 200–300 pS), intermediate conductance (IK, 32–39 pS), and small conductance (SK, 4–14 pS).
BK Channels: General Properties and Function
The BK channel (also known as KCa1.1, MaxiK, and slo1) is a homotetrameric channel consisting of four identical subunits of the pore-forming, Ca2+- and voltage-sensing α-subunit (BKα, encoded by a single gene KCNMA1) either alone or in association with regulatory β or γ subunits. The BKα channel (∼130 kDa) contains 7 transmembrane (TM) segments (S0–S6), a short extracellular N-terminus, and a large cytosolic C-terminus composed of two regulating conductance of K+ (RCK) domains for Ca2+ sensing (Tao and MacKinnon, 2019) (Figure 1). The four auxiliary β subunits (β1–β4) and the four γ subunits (γ1–γ4) are double- and single-spanning membrane proteins, respectively, with tissue-specific expression patterns. For example, β1 is mainly in smooth muscles, γ1 is in secretory, non-excitable cells, and γ3 and β4 are in the brain (Solaro and Lingle, 1992; Behrens et al., 2000; Brenner et al., 2000; Cox and Aldrich, 2000; Yan and Aldrich, 2010, 2012; Zhang and Yan, 2014).
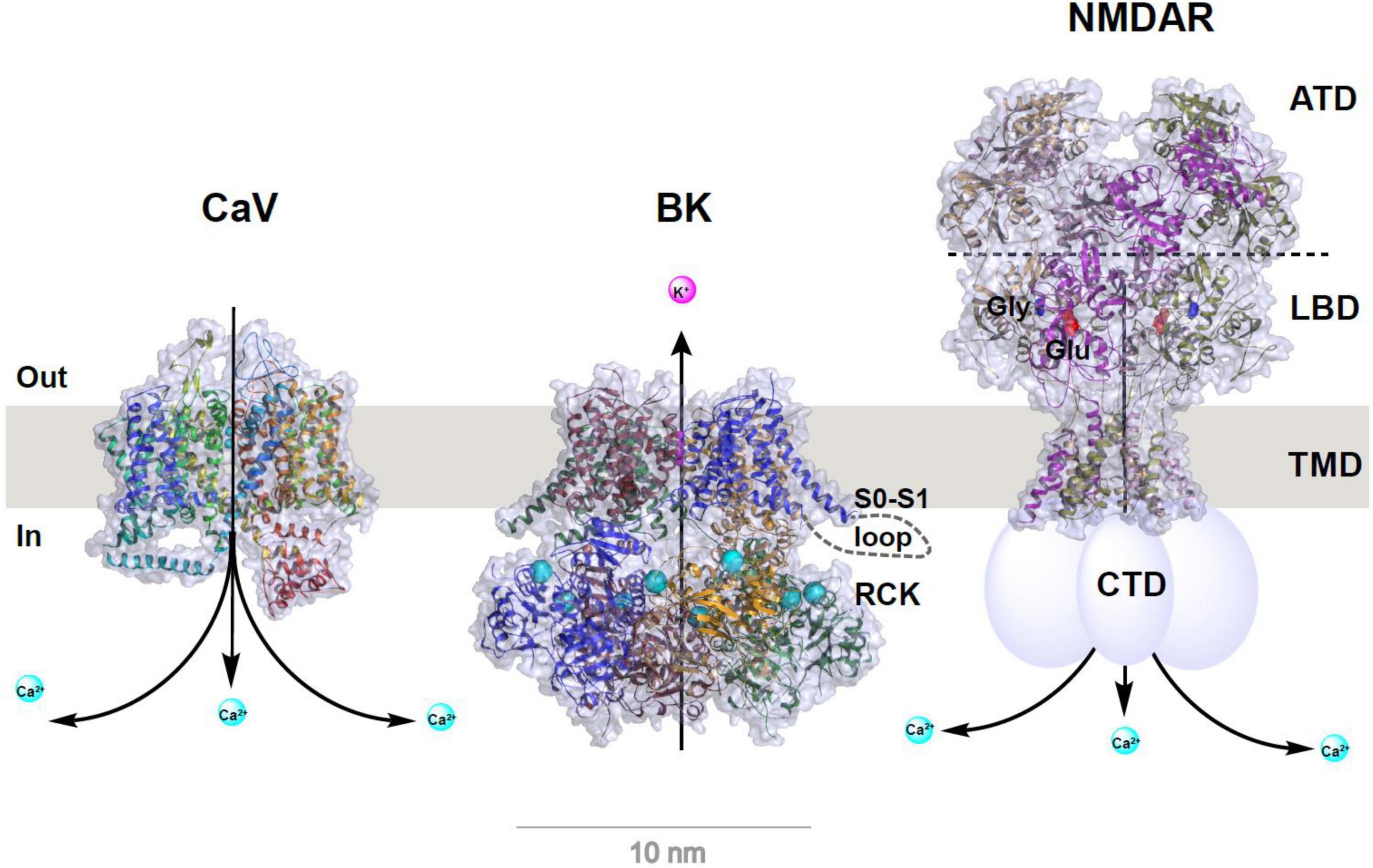
Figure 1. Structural models of CaV and BK channels and NMDA receptor. Structures were redrawn from published structures of rabbit CaV1.1 (PDB ID: 5GJV) (Wu et al., 2016), human BK (PDB ID: 6V22) (Tao and MacKinnon, 2019), and rat GluN1a/GluN2B NMDA receptor (PDB ID: 4PE5) (Karakas and Furukawa, 2014). For clarity, auxiliary subunits of CaV and BK channels are omitted and the bound Ca2+ ions in BK channel’s RCK domains are shown as enlarged cyan balls. Depiction in cartoon was added manually for the S0–S1 loop (only on one subunit) of BK channel and C-terminal domain (CTD) of NMDA receptor whose structures were unresolved. For NMDA receptor, the amino terminal domain (ATD), ligand binding domain (LBD), transmembrane domain (TMD), the bound glutamate (Glu) and glycine (Gly) are indicated on or near the corresponding structural parts.
BK channels have an exceptionally large single-channel conductance, which is 10–20 times larger than that of most other K+ channels. BK channel activation is regulated by membrane voltage and a wide range of [Ca2+]i (from sub-micromole to hundreds of micromoles) via Ca2+ bindings at the RCK domains. When activated by Ca2+ channel–mediated [Ca2+]i elevation, BK channels generate large K+ efflux and, consequently, rapid membrane repolarization that can limit further Ca2+ flux through membrane repolarization–induced deactivation of Ca2+ channels. Via this negative feedback mechanism, BK channel–mediated K+ signaling plays a powerful, integrative role in regulating cellular excitability and calcium signaling in electrically excitable cells (Ghatta et al., 2006; Salkoff et al., 2006).
The BK channels are critically involved in various cellular and physiological processes. In central neurons, BK channels mediate the repolarization and fast after hyperpolarization (fAHP) of action potentials (Shao et al., 1999; Womack and Khodakhah, 2002), shape dendritic Ca2+ spikes (Golding et al., 1999), and regulate neurotransmitter release at presynaptic terminals (Hu et al., 2001; Raffaelli et al., 2004; Xu and Slaughter, 2005; Samengo et al., 2014). In addition, they are involved in motor coordination (Sausbier et al., 2004), learning and memory (Matthews and Disterhoft, 2009; Ye et al., 2010; Typlt et al., 2013; Springer et al., 2014), the brain’s intrinsic rhythmicity of the circadian clock (Meredith et al., 2006; Pitts et al., 2006; Montgomery et al., 2013; Farajnia et al., 2015) and respiration (Onimaru et al., 2003; Zhao et al., 2006; Zavala-Tecuapetla et al., 2008), frequency tuning of cochlear hair cells (Fettiplace and Fuchs, 1999), pain modulation (Chen et al., 2009; Cao et al., 2012; Zhang et al., 2012; Waxman and Zamponi, 2014), and neuroprotection under pathological conditions (Runden-Pran et al., 2002; Shen et al., 2007; Zhang et al., 2009; Mancini et al., 2014). Defects in or dysregulation of human neuronal BK channels can cause epilepsy and paroxysmal dyskinesia (Brenner et al., 2005; Du et al., 2005) and are implicated in intellectual disability (Higgins et al., 2008; Deng et al., 2013), autism (Laumonnier et al., 2006), and schizophrenia (Zhang et al., 2006).
The Necessity and Properties of Nanodomain Coupling of BK Channels With Ca2+-Permeable Channels
In spite of multimode activation by cell membrane depolarization, a rise in [Ca2+]i, or both synergistically, BK channels are generally considered high-threshold channels. For the BK channels formed by the α subunit alone, a very high voltage of more than 100 mV, which is out of the physiological range, is needed for measurable BK channel activation under the cell’s resting Ca2+ conditions (≤∼0.1 μM) (Figure 2). Under resting membrane voltage conditions (e.g., −60 to −80 mV), an extremely high (≥100 μM) concentration of [Ca2+]i is needed to produce channel activation (Figure 2). Under membrane depolarization conditions in excitable neuronal (Vm ≤ +40 mV) or smooth muscle cells (Vm ≤ +20 mV) during action potential, the required [Ca2+]i concentration for significant BK channel activation is also approximately a few μM, which is generally higher than the cellular global [Ca2+]i concentration in resting (≤∼0.1 μM) and excited (≤∼1 μM) states (Figure 2). In contrast, the other two types of KCa channels, IK and SK, have a much higher affinity for Ca2+ (EC50 ≈ 0.3–0.5 μM) owing to their constitutively bound Ca2+-binding messenger protein, calmodulin (CaM). Therefore, BK channels had been considered to function mainly as a brake operating only under extreme conditions, e.g., a pathological [Ca2+]i overload repolarizing the membrane to limit further Ca2+ influx. Research progress in the last 2 decades has revealed that cells employ at least two effective biochemical strategies to allow BK channels to be activated under normal cellular or physiological conditions.
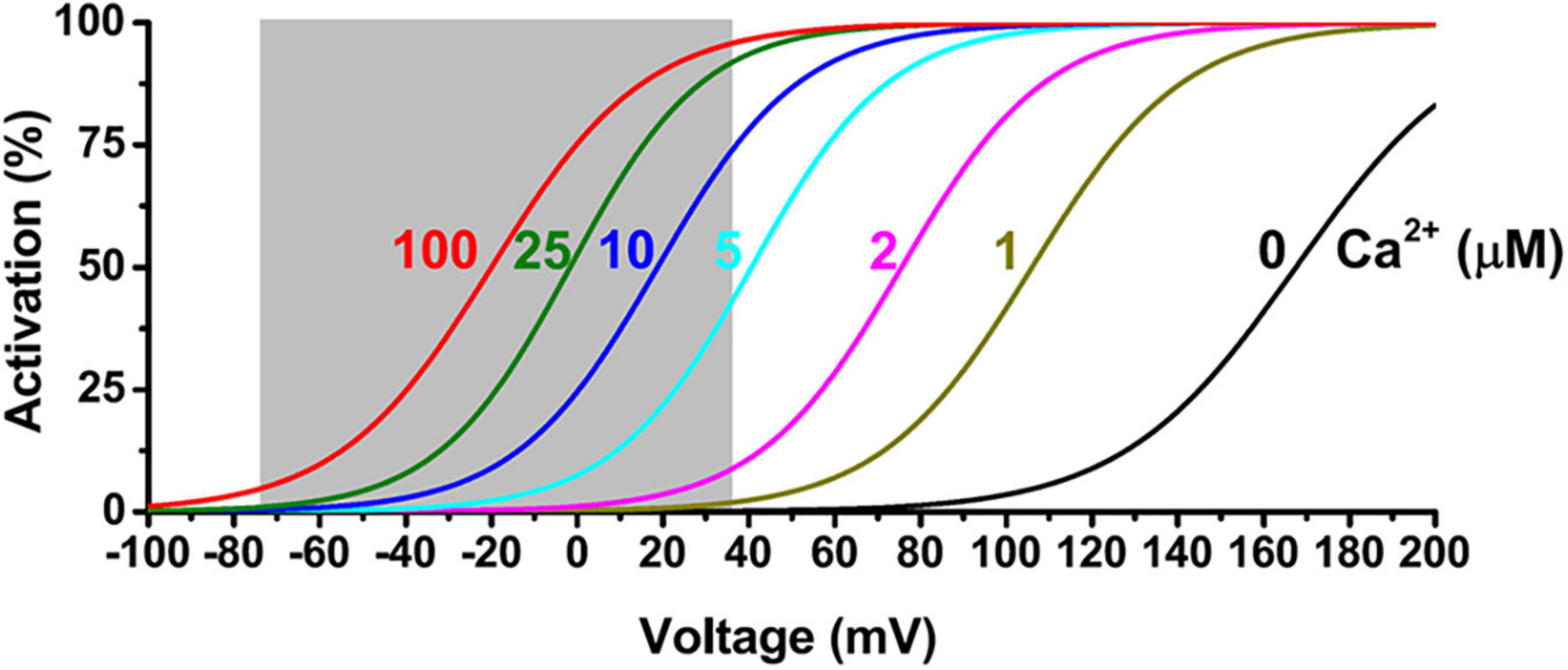
Figure 2. Voltage and Ca2+ dependence of BKα channel activation. The shaded area indicates a physiological range of membrane voltages.
The first strategy is to modulate the voltage dependence of the channel activation via auxiliary proteins. The γ1 (LRRC26) subunit in particular gives BK channels an unusual capability to be constitutively active at physiological voltages and [Ca2+]i levels in non-excitable cells by causing a large negative shift (∼−140 mV) in the voltage dependence of the channel activation (Yan and Aldrich, 2010, 2012). The γ1 subunit is highly expressed in secretory epithelia cells in different organs and plays an important role in the resting K+ efflux and fluid secretion in these cells (Yang et al., 2017; Gonzalez-Perez et al., 2021). The γ2 subunit also results in a great shift (∼−100 mV) in BK channel voltage gating (Yan and Aldrich, 2012). The γ2 subunit is highly expressed in the testis and is a potent regulator of BK channel function in the cochlea’s inner hair cells (Lingle, 2019). The β subunits overall do not strongly affect the thresholds of BK channel activation, despite their complex effects on BK channel voltage and Ca2+ gating and current kinetics. A detailed review of the BK channel β and γ subunits is beyond the scope of this work and can be found in previous articles (Zhang and Yan, 2014; Li and Yan, 2016; Latorre et al., 2017; Gonzalez-Perez and Lingle, 2019). The second strategy that cells use to activate BK channels is to position BK channels in proximity to some Ca2+-permeable channels to gain immediate access to the local high concentrations of [Ca2+]i during extracellular Ca2+ influx or Ca2+ release from intracellular Ca2+ store organelles.
Given the presence of endogenous Ca2+ buffers and the quick diffusion of Ca2+ ions, the distance to the Ca2+-originating site determines the local [Ca2+]i concentration. Thus, rapid and precise spatiotemporal control of the [Ca2+]i concentration is found in local Ca2+-signaling domains, in which Ca2+ channels and Ca2+-effector sensors are within proximity, i.e., within the nanodomain or microdomain (Augustine et al., 2003; Eggermann et al., 2011) (Figure 3). In Ca2+ signaling, a nanodomain is a conceptual region of highly localized Ca2+ signals extending over a few tens of nm from the cytoplasmic mouths of the Ca2+ channels (Eggermann et al., 2011; Tay et al., 2012). Coupling between Ca2+ sensors and Ca2+ channels within nanodomains can achieve high efficacy, speed, and energy efficiency for Ca2+ signaling (Eggermann et al., 2011). Experimentally, the colocalization of Ca2+ channels and Ca2+-effector sensors can be functionally probed with Ca2+ chelators, ethylene glycol tetraacetic acid (EGTA), and 1,2-bis(o-aminophenoxy)ethane-N,N,N′,N′-tetraacetic acid (BAPTA) (Figure 4). Both EGTA and BAPTA compete with Ca2+-sensing proteins for [Ca2+]i and have similar steady-state binding affinities for Ca2+. However, they differ greatly in their rates of Ca2+ binding (Naraghi and Neher, 1997). BAPTA has a binding rate constant 150 times higher than that of EGTA. At millimolar levels, BAPTA can efficiently prevent the spreading of free Ca2+ from the entry site. The slower chelator, EGTA, is relatively ineffective in sequestering Ca2+ within a short distance of the Ca2+ exit site but can intercept Ca2+ during Ca2+ diffusion over a longer distance. For convenience, the local Ca2+ signaling domain is classified as a Ca2+ nanodomain or microdomain depending on the sensitivity of the Ca2+-sensing effector to EGTA and BAPTA. The Ca2+ nanodomain signaling process is effectively disrupted by millimolar levels (e.g., 2–10 mM) of BAPTA but not of EGTA, whereas in Ca2+ microdomains (e.g., from a few tens to a few hundred nm), EGTA also dissipates the signaling process effectively. Biochemically, the nanodomain or microdomain coupling of Ca2+-sensing proteins to Ca2+ channels can be achieved by direct protein-protein physical interactions for nanodomain colocalization; indirect interactions mediated by other proteins, e.g., scaffold proteins (Sclip et al., 2018), for nano- or microdomain colocalization; and special membrane domains, e.g., lipid rafts (Ma et al., 2015), that concentrate and restrict their distribution for nano- or microdomain colocalization.
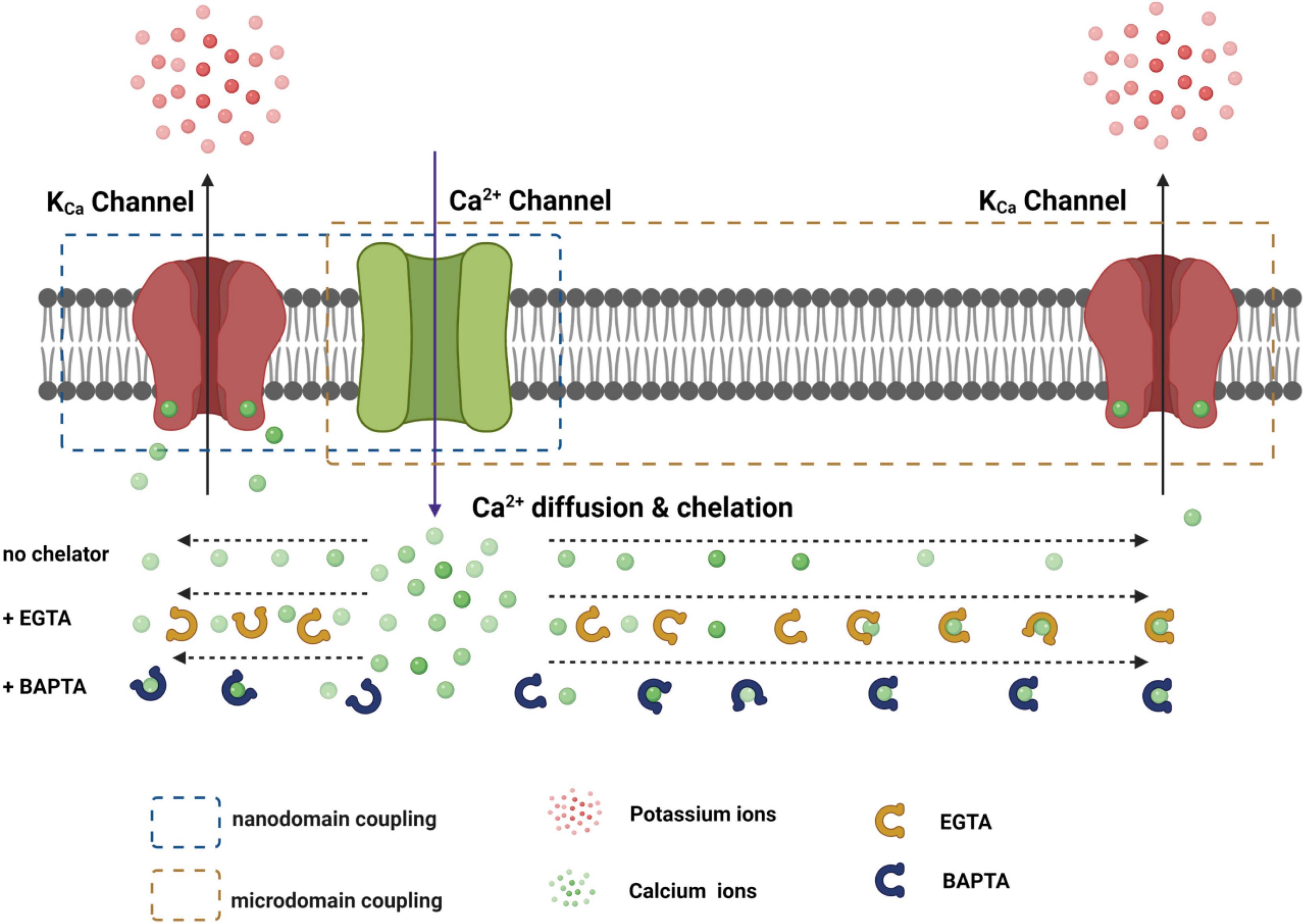
Figure 3. Functional coupling of the KCa channel with the Ca2+ channel within nanodomain and microdomain. The distance-dependent effects of EGTA and BATPA on the availability of free Ca2+ ions during their diffusion from the Ca2+ channel pore are roughly depicted.
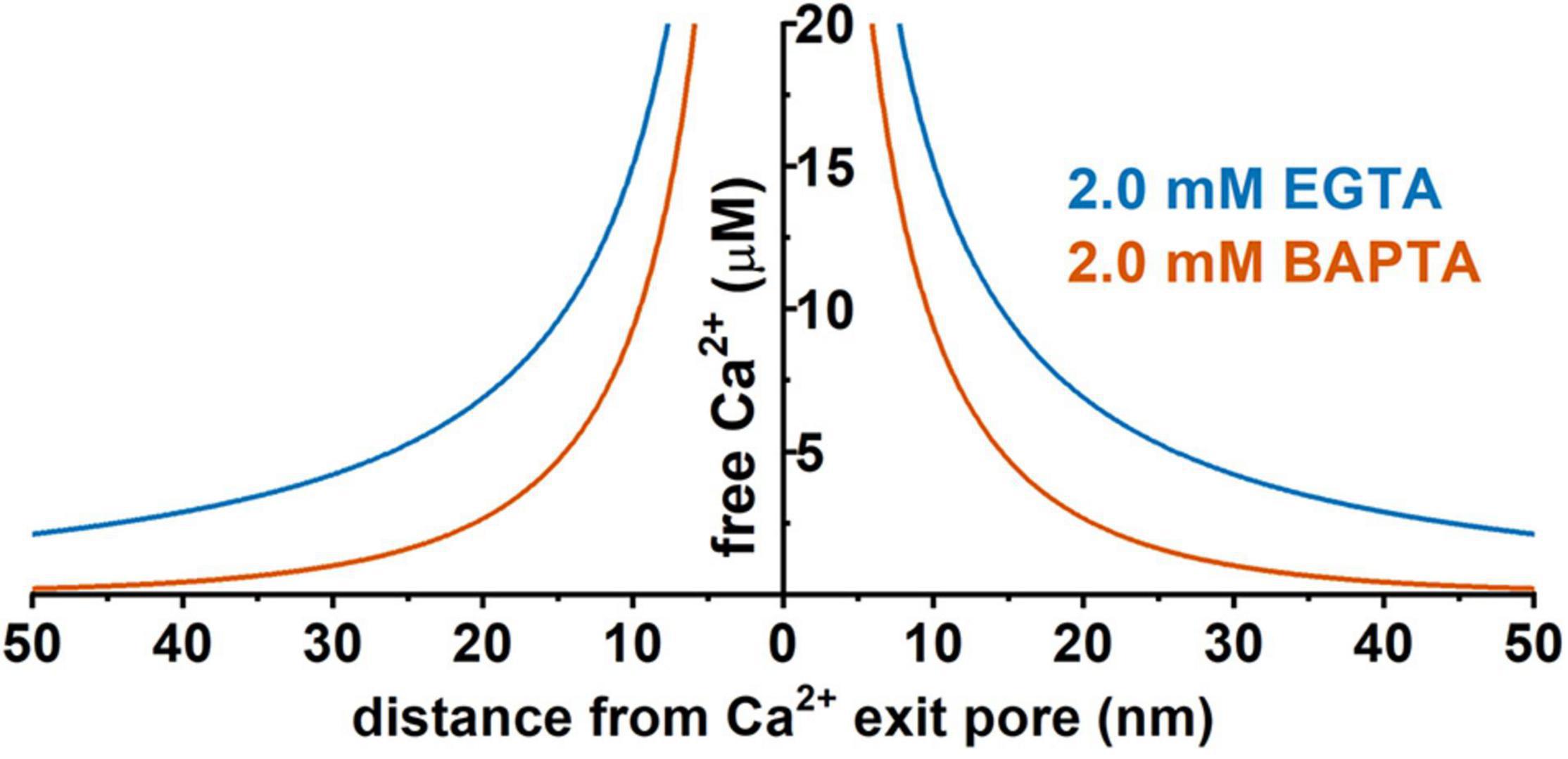
Figure 4. Predicted distance dependence of the local free Ca2+ concentration originating from a hypothetic Ca2+ channel pore in the presence of 2 mM EGTA or 2 mM BAPTA. Prediction was performed using the CalC software (version 6.8.0) (Matveev et al., 2004) at 0.1 pA for the single-channel Ca2+ current. Chelator and Ca2+ parameters were taken from previous reports (Naraghi and Neher, 1997; Fakler and Adelman, 2008; Eggermann et al., 2011).
The distance to the Ca2+ source is a key factor determining BK channel activation and function. Given the presence of endogenous cytosolic Ca2+ chelating buffer that can be comparable to EGTA in capacity (Fakler and Adelman, 2008; Schwaller, 2010), the microdomain coupling is expected to be largely ineffective in BK channel activation. For example, in the presence of 2 mM EGTA, a BK channel must be within 50 nm of a Ca2+ source to sense an effective local [Ca2+]i concentration of at least 2 μM (Figure 4) in order to achieve significant activation, e.g., an open probability (Po) of 0.1 or greater, even at a maximally depolarized membrane voltage of +40 mV (Figure 2). Thus, under physiological conditions of limited membrane depolarization, native BK channels, particularly those in excitable cells that lack γ1 and γ2 expression, must colocalize with Ca2+ sources within nanodomains to be physiologically active and functional. Compared to other KCa channels, the requirement of nanodomain colocalization with Ca2+ source is specific to BK channels because the IK and SK channels’ exquisitely high affinity for Ca2+ theoretically relieves them from the requirement of close nanodomain interaction with Ca2+ sources for functional coupling, even in the presence of EGTA (Fakler and Adelman, 2008).
From the above discussion, BK channels coupled with Ca2+ channels within the nanodomain are expected to demonstrate: (1) the Ca2+ channel–mediated BK channel currents that are largely resistant to Ca2+ chelating by millimolar levels of EGTA but could be fully or partially sensitive to millimolar levels of BAPTA; (2) physical formation of protein complex by the 2 channels that can be copurified or coimmunoprecipitated in the forms of channel-channel complexes or supercomplexes via direct or indirect physical interactions, or membrane domains with special lipid compositions and cytoskeletal support resistant to detergent disruption; and (3) physical colocalization in situ within a very short distance, which can be probed either directly with super resolution (under 100 nm) microscopy or more conveniently and indirectly via a nanometer distance-sensitive imaging analysis method such as förster resonance energy transfer (FRET) or proximity ligation assay (PLA) (see section “BK-NMDAR Colocalization and Functional Coupling Within the Nanodomain”). We review the evidence and findings from these 3 directions in support of nanodomain coupling of BK channels with a specific Ca2+ channel.
BK-CaV Coupling
CaV Channels
Of the different types of Ca2+ channels, voltage-gated calcium (CaV) channels play a vital role in providing intracellular Ca2+ ions to KCa channels. CaV channels are formed by central pore-forming α1 subunits and regulatory auxiliary subunits and are responsible for membrane depolarization–induced Ca2+ entry into excitable cells (Zamponi et al., 2015; Nanou and Catterall, 2018). The auxiliary subunits, which contribute to calcium channel diversity, are encoded by 4 α2δ genes, 4 β-subunit genes, and 9 γ-subunit genes. The α1 subunit forms the Ca2+ selective pore and consists of 24 TM α helices divided into 4 homologous domains, each containing 6 TM α helices (Wu et al., 2016) (Figure 1). Based on the electrophysiological and pharmacological properties of their ionic currents, CaV channels are classified into L-, N-, P/Q-, R-, and T-types. Based on the amino acid sequence similarities of the pore-forming α1 subunits, they are grouped into CaV1, CaV2, and CaV3 types. L-type (CaV1.1, CaV1.2, CaV1.3, and CaV1.4) channels are activated by high voltages and distinguished by long-lasting (L) activation, i.e., slow voltage-dependent inactivation and blocked by calcium antagonist drugs such as dihydropyridines and phenylalkylamines. L-type channels have diverse functions, including the initiation of contraction in muscle cells, hormone secretion in endocrine cells, and local calcium signaling for gene transcription. P/Q- (CaV2.1), N- (CaV2.2), and R- (CaV2.3) type channels are also activated by high voltages but have faster voltage-dependent inactivation. They are found primarily in neurons and are blocked by peptide toxins from spiders and snails. T-type (CaV3.1, CaV3.2, and CaV3.3) channels are activated by low voltages (negative membrane potentials) and have fast deactivation upon repolarization and fast voltage-dependent inactivation. They are predominantly found in cardiac myocytes, sinoatrial nodes, and thalamic neurons.
BK-CaV Functional Coupling
Researchers studying BK channels from different types of neurons have found that channel activity depends on Ca2+-influx through CaV channels and that blocking of the L-type (Storm, 1987; Prakriya and Lingle, 1999; Vivas et al., 2017), P/Q type (Edgerton and Reinhart, 2003; Womack et al., 2004), and N-type CaV channels (Marrion and Tavalin, 1998; Loane et al., 2007) with subunit-specific toxins suppresses neuronal BK channel currents. In addition, they discovered that BK-CaV coupling must occur within a nanodomain because the CaV-dependent BK channel activation was rapid (e.g., ∼1 ms) and not disrupted by the Ca2+ chelator EGTA when patch-clamp recordings were performed on channels heterologously expressed in Xenopus oocytes, Chinese hamster ovaries (CHO), and tsA-201 cells (Berkefeld et al., 2006; Berkefeld and Fakler, 2008; Vivas et al., 2017), native channels in chromaffin cells (Prakriya and Lingle, 2000; Berkefeld et al., 2006), or neurons (Gola and Crest, 1993; Robitaille et al., 1993; Edgerton and Reinhart, 2003; Sun et al., 2003; Muller et al., 2007; Vivas et al., 2017). During functional coupling, the apparent voltage dependence, current kinetics, and amplitude of BK channel activation are affected by the voltage dependence, conductance, and coupling strength of the CaV channels. Thus, different CaV channels confer different apparent gating properties for BK channel activation (Berkefeld et al., 2006; Vivas et al., 2017). The lower-voltage–activated CaV1.3 caused a much greater shift toward negative voltage in the activation of the BK channel than in that of the CaV2.2 channel (Vivas et al., 2017). Based on EGTA’s and BAPTA’s effects on the CaV2.1-induced BK channel’s current amplitude and time course recorded in the heterologous expression system, the distance between these 2 channels was estimated to be approximately 10–15 nm (Berkefeld et al., 2006). Similarly, the diffusional distance for Ca2+ ions from the CaV channels to the BK channels in hippocampal granule cells was estimated to be 13 nm per linear approximation of buffer Ca2+ diffusion (Muller et al., 2007). The T-type CaV3.2 channel also provided Ca2+ for BK channel activation in a heterologous expression system of tsA-201 cells and in rat medial vestibular neurons (Rehak et al., 2013). However, the CaV3-evoked BK channel currents were sensitive to EGTA, suggesting weaker microdomain coupling than that observed for nanodomain coupling with CaV1.2, 1.3, 2.1, and 2.2 channels.
Molecular Organization of BK-CaV Nanodomain Coupling
Affinity purification of BK channels in rat brains with anti-BKα antibodies and subsequent mass spectrometry analyses identified the formation of macromolecular protein complexes between BK channels and CaV1.2 (L-type), CaV2.1 (P/Q-type), and CaV2.2 (N-type) channels (Berkefeld et al., 2006). Of the CaV channel proteins, CaV2.1 was the most abundantly copurified with BKα. The auxiliary subunits of BKβ2, BKβ4, CaVβ1b, CaVβ2, CaVβ3, and CaVβ4 were also identified in the complexes. By coimmunoprecipitation, BKα was found to form complexes with CaV1.2 and CaV2.1 as well in the heterologous expression system of Xenopus oocytes (Berkefeld et al., 2006). The coimmunoprecipitation of BK channels and CaV1.3 was also reported for native channels from rat brains (Grunnet and Kaufmann, 2004) and for heterologously expressed channels in tsA-201 cells (Vivas et al., 2017). These studies showed that BK and CaV channels formed complexes in both brain and heterologous expression systems, which rules out the requirement for neuron-specific protein to be present in order for the physical association to occur. Interestingly, the S0 TM segment of the BK channels was necessary for coimmunoprecipitation with CaV3.2 in spite of the likely microdomain coupling of these two channels (Rehak et al., 2013).
Given the predicted close distance (10–15 nm) in functional coupling (Berkefeld et al., 2006; Muller et al., 2007) and the large sizes of BK and CaV channels, which are ∼13 and ∼10 nm wide (parallel to the membrane), respectively (Wu et al., 2015; Tao and MacKinnon, 2019) (Figure 1), they could be positioned in close contact via direct physical interactions. However, no protein domains, regions, or residues are known to be involved in BK-CaV interactions. Some reports support the possibility of indirect interactions between the BK and CaV channels. The colocalization of CaV and BK channels at the active zone of presynaptic nerve terminals was proposed to be mediated by the scaffold proteins, RIMs and RIMs binding proteins, which interact with CaV and BK channels, respectively (Sclip et al., 2018). Co-expression of the channels with a G protein-coupled receptor, β protein-co receptor, was reported to be needed for coimmunoprecipitation of BK CaV1.2 channels in HEK293 cells (Liu et al., 2004), which is in contrast with the reported BK-CaV1.2 complex formation without expression of any other exogenous protein in another heterologous system of Xenopus oocytes (Berkefeld et al., 2006). It is also unclear whether BK and CaV channels can directly interact with each other and form complexes in 1:1 stoichiometric relationships, as has been previously speculated (Berkefeld et al., 2006; Fakler and Adelman, 2008). Computational modeling of BK-CaV coupling at 1:1 (Cox, 2014) and other stoichiometric relationships (Montefusco et al., 2017) could reproduce the coupled electric activity observed in cells. A super-resolution microscopic study of BK and CaV1.3 in a heterologous system and in rat hippocampal and sympathetic neurons revealed the formation of homotypic multichannel clusters of both CaV1.3 and BK channels and a skewed, concentrated distribution of CaV1.3 clusters occupying areas adjacent to BK clusters (Vivas et al., 2017). The BK channel clusters had a median area of 1,600 nm2 in tsA-201 cells, 2,000 nm2 in hippocampal neurons, and 2,800 nm2 in superior cervical ganglion neurons, containing roughly an average of 10–15 BK channels in a cluster. Most of the BK channel clusters were surrounded (within a radius of 200 nm) by a variable number (mean, ∼4) of CaV1.3 clusters (median area, 1,600 nm2). Thus, surprisingly, the association between BK and CaV1.3 channels occurred mainly in multichannel clusters and was not fixed in stoichiometric or geometric relationships, which was contradictory to the concept of 1:1 stoichiometry in physical interactions. In hippocampal and superior cervical ganglion neurons, small portions (3 and 10%, respectively) of the BK channel clusters contained CaV1.3 channels, indicating the presence of some intimate BK-CaV coupling and interactions within a cluster. For BK-CaV1.3 coupling via the observed heterogeneous, multichannel clustering, the local Ca2+ source for BK channel activation varies greatly according to the number and locations of the surrounding activating CaV channels. Interestingly, clustering facilitates the cooperative opening of the CaV1.3 channels within a given cluster (Moreno et al., 2016), which could enhance BK-CaV functional coupling.
Localization and Roles of BK-CaV Coupling in the Nervous System
BK-CaV coupling has been recorded in a variety of different neurons and plays fundamental roles in the regulation of neural firing and transmission. The exceptionally large, single-channel K+ currents and tight BK-CaV coupling allow BK channels to function as potent negative-feedback regulators of both membrane potentials and Ca2+-influx. During the membrane depolarization–induced sequential activation of CaV and BK channels, BK channel-mediated large K+ currents rapidly counterbalance membrane depolarization and Ca2+ influx by repolarizing the membrane potential, which also limits further Ca2+ influx via the repolarization-induced closure of CaV channels. For BK-CaV nanodomain coupling, the voltage threshold of BK channel activation tends to reflect the voltage threshold of the coupled CaV channels (Vivas et al., 2017). If BK channels are activated by CaV channels before the membrane potential reaches the action potential firing threshold, BK-CaV coupling can prevent neuronal firing, as that implicated for BK-CaV1.3 coupling in sympathetic neurons (Vivas et al., 2014, 2017). In most studies, the BK-CaV coupling was recorded on somatic membranes (Figure 5) and regulated neuronal firing by repolarizing action potentials and generating fAHP, such as those observed in Helix neurons (Gola and Crest, 1993), hippocampal neurons (Marrion and Tavalin, 1998), guinea pig sympathetic neurons (Davies et al., 1999), cerebellum Purkinje neurons (Edgerton and Reinhart, 2003), neocortical pyramidal neurons (Sun et al., 2003), hippocampal granule cells (Sun et al., 2003), and striatal cholinergic interneurons (Goldberg and Wilson, 2005). BK and CaV channels were also expressed on presynaptic terminals on which neurotransmitter release is tightly controlled by Ca2+ entry via CaV channels. The BK-CaV coupling at presynaptic terminals (Figure 5) negatively modulated the neurotransmitter release by negative feedback regulation of CaV-mediated Ca2+ influx. Such a role for BK-CaV coupling as a controller of neurotransmitter release at presynaptic terminals has been demonstrated in frog neuromuscular junctions (Robitaille et al., 1993), Xenopus nerve–muscle synapses (Yazejian et al., 1997), and mouse motor nerve terminals (Protti and Uchitel, 1997). For the CaV2.3 channel, it was previously reported to be absent in the isolated rat brain BK-CaV complexes and also failed in activating BK channels in the presence of EGTA in the heterologous expression system (Berkefeld et al., 2006). A later study showed co-immunoprecipitation of BK channels and CaV2.3 from mouse hippocampus and deletion of CaV2.3 in CA1 pyramidal cells reduced the BK channel function resulting in altered action potential waveforms which strengthens the synaptic transmission between CA1 and subiculum and increased the short-term plasticity (Gutzmann et al., 2019).
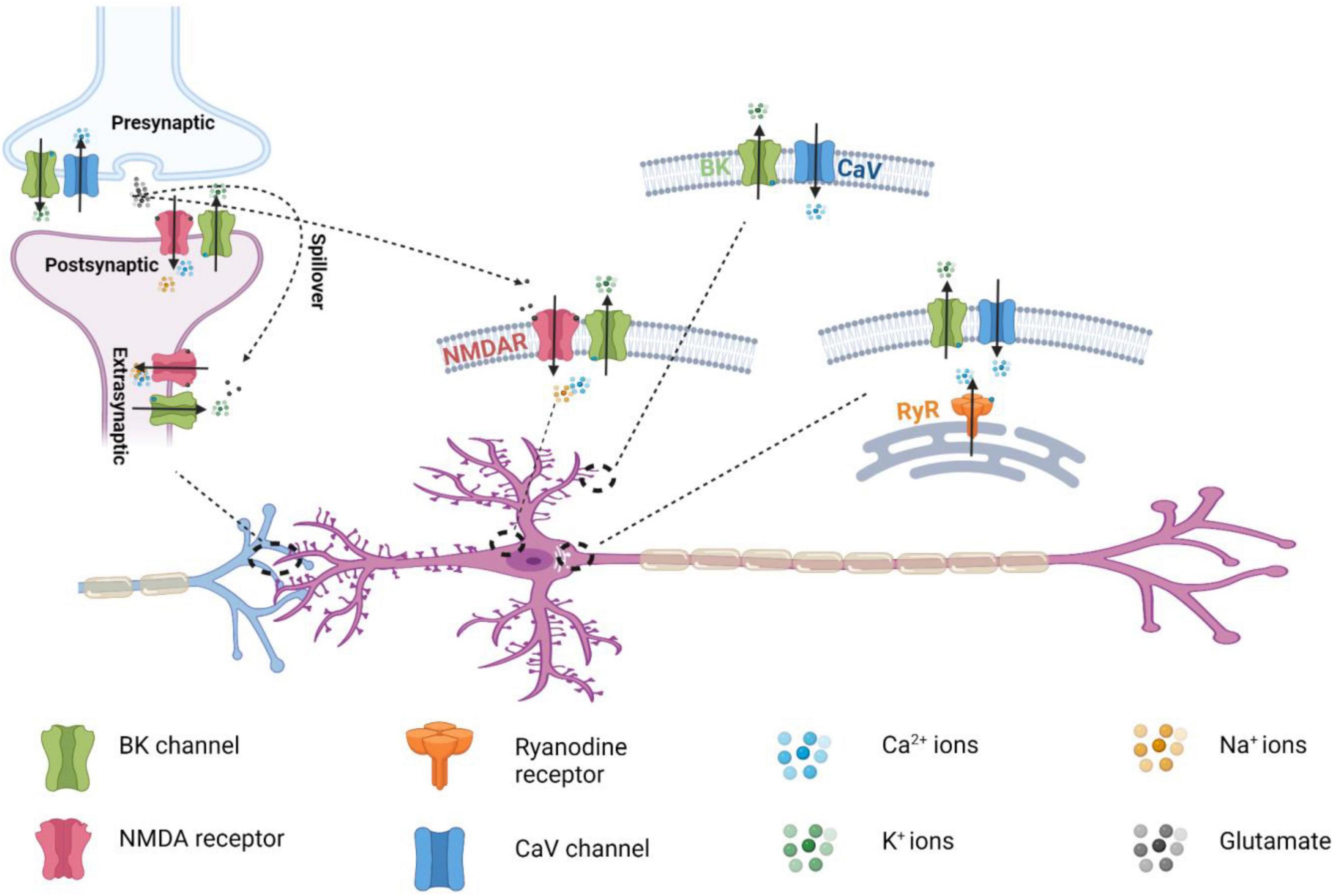
Figure 5. Distribution of the nanodomain coupling of BK channels with different Ca2+ permeable channels in neurons.
BK-NMDAR Coupling
NMDARs
NMDARs are ligand- and voltage-gated, Ca2+-permeable cation channels that function as coincidence detectors of elevated extracellular glutamate levels and membrane depolarization. Channel opening in NMDARs requires coincident glutamate binding–evoked channel activation and the membrane depolarization–induced release of pore blockades caused by extracellular Mg2+. NMDAR activation results in prolonged Na+ and Ca2+ influx that is critical to excitatory synaptic transmission and synaptic plasticity. Unlike the two other classes of ionotropic glutamate receptors, AMPA receptors (AMPARs) and kainate receptors (KARs), NMDARs are characterized by high Ca2+ permeability, slow deactivation, high affinity for glutamate, high unitary conductance (30–50 pS), and voltage-dependent blockading by extracellular Mg2+ (Zito and Scheuss, 2009; Paoletti, 2011; Paoletti et al., 2013). NMDARs are heterotetrameric channels composed of two obligatory GluN1 subunits and 2 modulatory GluN2 (A-D) or GluN3 (A–B) subunits (Figure 1). Each NMDAR subunit consists of 4 structural domains: 2 extracellular, tandem, globular, clamshell-like domains [the amino-terminal domain (ATD) for subunit assembly and the ligand-binding domain (LBD)] for the binding of glycine and glutamate; an ion channel pore TM domain made of 3 TM segments; and a highly variable, intracellular carboxyl-terminal domain (CTD) (Karakas and Furukawa, 2014) (Figure 1). NMDARs are involved in many aspects of synaptic transmission, dendritic integration, synaptic and neuronal maturation, and synaptic plasticity throughout the brain (Zito and Scheuss, 2009). They are also involved in various neurological and psychiatric diseases, including NMDAR hyperactivity–induced acute excitotoxicity (e.g., epilepsy and ischemic stroke) and chronic neurodegeneration (e.g., Alzheimer, Parkinson, and Huntington diseases and amyotrophic lateral sclerosis), NMDAR hypofunction–related neurodevelopmental disorders (e.g., schizophrenia), and many others (e.g., pain, depression, autism, white matter injury, and anti-NMDAR encephalitis) (Lakhan et al., 2013; Paoletti et al., 2013; Zhou and Sheng, 2013; Bourinet et al., 2014). In spite of their ubiquitous expression in the mammalian central and peripheral nervous systems, BK channels and NMDARs were traditionally considered to be localized in different compartments as presynaptic and postsynaptic channels, respectively. However, in an early 2001 study, BK channels were activated by NMDAR-mediated Ca2+ influx at extrasynaptic sites in rat olfactory bulb granule cells; this was a previously unknown neuronal activity-inhibitory role for NMDARs (Isaacson and Murphy, 2001).
Compared to the intensive research on BK-CaV coupling, the attention to BK-NMDAR coupling had been lacking. Questions regarding whether BK channels and NMDARs can directly associate to form protein complexes, whether such extrasynaptic BK-NMDAR coupling is also present in other neurons or brain regions, and whether this coupling occurs in other subcellular location were not addressed until more recently.
Biochemical Basis of BK-NMDAR Interactions
Affinity purification and mass spectrometry analyses of immunopurified BK channels and NMDA receptors from rat brains have found that they were mutually identified together (Zhang et al., 2018). Tandem immunopurification with anti-BKα and ant-GluN1 antibodies in the first and second rounds of purification, respectively, was able to purify the protein complexes containing both BKα and GluN1. The copurification of GluN2A and GluN2B with the BKα-GluN1 complex indicates the association of BK channels with functional NMDARs. In the heterologous expression system of HEK293 cells, it was found that BKα specifically interacts with the NMDARs’ obligatory GluN1 subunit, whereas there were no interactions observed between BKα and the NMDARs’ GluN2A and GluN2B, the kainate receptors’ GluK1 and GluK2, or the AMPA receptors’ GluR1 and GluR2. With truncation constructs of BKα and GluN1 and chimeric constructs of GluN1/GluK2, the BKα-GluN1 interactions involved the former’s S0–S1 long loop and the latter’s TM regions, including the loops and cytosolic C-terminus. An engineered protein containing the GluN1’s cytosolic regions (residues 563–587 for the M1–M2 loop region and residues 813–920 for the C-terminus), which was expressed in and purified from Escherichia coli, was able to interact directly with a synthesized peptide of the BKα S0–S1 loop region (residues 46–93) in vitro. Furthermore, the synthesized peptide of the S0–S1 loop region competitively disrupted the association between BKα and GluN1. These findings indicate that BKα and GluN1 directly interact with each other via their cytosolic regions, including the BKα S0–S1 loop region (Figure 1), which seems to be flexible in structure as its structure was undefined in the reported cryo-EM structures of the BK channels (Tao and MacKinnon, 2019).
BK-NMDAR Colocalization and Functional Coupling Within the Nanodomain
The colocalization of BK and NMDARs was probed with an in situ PLA (Zhang et al., 2018; Gomez et al., 2021). PLA displays point-like staining signals only when the 2 epitopes on the interacting proteins for primary antibodies are in proximity (<40 nm) (Soderberg et al., 2006), making PLA a suitable assay for the detection of protein colocalization within the nanodomain. With in situ PLA, BKα and GluN1 were found to be colocalized in both the heterologous expression system and, broadly, in different regions of mouse hippocampus (Zhang et al., 2018). Consistent with the sole requirement of GluN1 for protein complex formation with BK channels (Zhang et al., 2018), PLA with HEK293 cells co-expressing BK channels with GluN1/GluN2A or GluN1/GluN2B showed no preference in BK channels’ proximity to these 2 types of NMDARs (Gomez et al., 2021). In the dentate gyrus, PLA signals of the BKα-GluN1 complexes were most abundant in the molecular layer region (Zhang et al., 2018), which is consistent with the predominant dendritic distribution of NMDARs. Glutamate-induced BK channel outward currents were observed when whole-cell voltage clamp recording was performed on mature dentate gyrus granule cells in mouse hippocampal slices upon application of glutamate toward soma (Zhang et al., 2018). The currents were blocked by the NMDAR antagonist (2R)-amino-5-phosphonovaleric acid (AP5) and pore-blocker MK-801, indicating involvement of the receptor’s ion-conducting function. The glutamate-induced BK channel currents were insensitive to intracellularly applied EGTA but became significantly smaller in the presence of BAPTA, confirming nanodomain functional coupling of BK channels with NMDARs. Intracellular application of the synthesized S0–S1 loop peptide reduced the glutamate-induced BK channel outward currents, indicating that the physical interactions between these 2 channels play a role in their functional coupling. EGTA-insensitive coupling of BK channels with NMDARs was also recently observed in a subset of barrel cortex layer 5 pyramidal neurons (BC-L5PNs) but when NMDA was applied toward basal dendrites instead of soma (Gomez et al., 2021). In a heterologous expression system of HEK293 cells, similar results of BK-NMDAR (GluN1/GluN2A) functional coupling were revealed by whole cell voltage-clamp recording (Zhang et al., 2018). In excised inside-out membrane patches of HEK293 cells, BK channel openings at the single channel level were observed upon NMDAR activation using flash photolysis of caged NMDA (Zhang et al., 2018). In HEK293 cells, activation of NMDARs formed by GluN1/GluN2A or GluN1/GluN2B both resulted in large shifts in the apparent voltage dependence of BK channel activation (Gomez et al., 2021).
Localization and Roles of BK-NMDAR Coupling in the Nervous System
Both BK channels and NMDARs are widely expressed in different regions of the central and peripheral nervous system. Using a pull-down assay, Zhang et al. (2018) showed that BK-NMDAR complexes are ubiquitously present in the brain, as BKα and GluN1 mutually pulled down each other in all examined brain regions, including the hippocampus, cerebellum, cortex, thalamus, striatum, and olfactory bulb. The subcellular distribution of NMDARs is affected by their subunit composition. In the adult central nervous system, GluN2B is mainly expressed at extrasynaptic sites, whereas GluN2A-containting receptors are enriched at postsynaptic sites at synapses (Paoletti et al., 2013). Given that both GluN2A and GluN2B are present in neuronal BK-NMDAR complexes, these complexes are expected to exist both extrasynaptically and postsynaptically. NMDAR-induced BK channel activation has been observed at the extrasynaptic sites of olfactory bulb granule cells (Isaacson and Murphy, 2001) and dentate gyrus granule cells (Zhang et al., 2018). At extrasynaptic sites, functional BK-NMDAR coupling can be induced by accumulated glutamate spillover during repetitive synaptic activities (Figure 4), such as those observed in olfactory bulb granule cells (Isaacson and Murphy, 2001). At postsynaptic sites, NMDAR-coupled BK channels can be immediately activated by presynaptically released glutamate during synaptic transmission (Figure 5). BK channels are generally considered to be axonal channels (Misonou et al., 2006), but a significant portion has also been observed at postsynaptic terminals in the stratum radiatum and oriens of the rat hippocampus (Sailer et al., 2006). However, the mechanism of channel activation and the function of BK channels at postsynaptic sites were largely unknown until recently. Interestingly, with BKα knockout mice and pharmacological tools, BK channels were found to negatively regulate the excitatory postsynaptic potentials (EPSPs) of dentate gyrus granule cells evoked by single-pulse stimulation of presynaptic fibers from the in the middle of the molecular layer (Zhang et al., 2018). Such a regulatory effect of BK channels on synaptic transmission at perforant path–dentate granule cell synapses was found to be insensitive to EGTA and strictly dependent on NMDAR activity. Given that the BK-NMDAR coupling was induced by a single pulse stimulation, the coupling must occur within the immediate reach of newly released glutamate in the synaptic cleft, i.e., at the pre- or postsynaptic terminal. A presynaptic effect of the BK channels was largely ruled out because of the lack of effect of paxilline and BKα knockout on presynaptic transmitter release. The postsynaptic dendritic location of the BK-NMDAR coupling was confirmed by the blockade effects of postsynaptically loaded paxilline and MK-801. When loaded intracellularly, the synthetic S0–S1 peptide also abolished the BK channels’ effect on synaptic transmission, suggesting that physical interactions are necessary for functional coupling at postsynaptic terminals (Zhang et al., 2018). The postsynaptic BK-NMDAR interactions and coupling (Figure 5) provide a novel mechanism for the negative feedback regulation of synaptic transmission via NMDAR-mediated activation of postsynaptic BK channels by the presynaptically released neurotransmitter glutamate in the synaptic cleft. Dentate gyrus granule cells are the first checkpoints for cortical information entering the hippocampus, where learning and memory take place. The findings on NMDAR-mediated BK channel activation and the modulatory effect of postsynaptic BK-NMDAR coupling on synaptic transmission provide a new molecular basis for understanding the role of BK channels in hippocampal learning and memory (Matthews and Disterhoft, 2009; Ye et al., 2010; Typlt et al., 2013; Springer et al., 2014).
Postsynaptic BK-NMDAR coupling was also recently reported to occur in the basal dendrites of approximately 40% of BC-L5PNs (Gomez et al., 2021). The application of NMDA toward basal dendrites led to large NMDAR inward and BK channel outward currents recorded in soma, supporting dendritic locations of BK-NMDAR coupling (Gomez et al., 2021). Such large inward currents of NMDARs and outward currents of BK channels were typically observed when BK-NMDAR coupling occurred locally at the soma (Isaacson and Murphy, 2001; Zhang et al., 2018). Similar to that observed in dentate gyrus granule cells (Zhang et al., 2018), the NMDAR-mediated BK channel activity in the basal dendrites reduced the postsynaptic membrane potentials (Gomez et al., 2021). Interestingly, as compared to the BC-L5PNs lacking BK-NMDAR coupling, the basal dendrites displaying NMDAR-mediated BK activation had no Ca2+ spike and had shortened durations and reduced amplitudes of after hyperpolarization in current injection–elicited action potentials. Furthermore, these BC-L5PNs also had reductions in spike timing–dependent LTP (t-LTP). t-LTP levels were restored by blockading the BK channel with paxilline, and reductions in these levels were prevented when the frequency and number of pre- and postsynaptic stimulation pairings were increased (Gomez et al., 2021). It was proposed that the BK-NMDAR coupling increases threshold for induction of t-LTP by functioning as high-pass filters for incoming synaptic input (Gomez et al., 2021). These putative roles for postsynaptic BK-NMDAR coupling on the regulation of action potential and LTP are novel. However, it remains to be determined whether NMDARs and their nanodomain coupling to BK channels are the major contributors to the BK channel activities underlying the observed differences between these 2 types of BC-L5PNs. Application of the synthetic S0–S1 peptide to decouple BK-NMDAR interactions (Zhang et al., 2018) could potentially answer this question.
The recent discovery of BK-NMDAR complex formation and functional coupling helps explain previous reports of NMDAR-mediated, apamin-insensitive KCa currents in cultured, postnatal rat hippocampal neurons (Zorumski et al., 1989); the need for both NMDAR and BK channel activities in the inhibition of opioid release in the spinal dorsal horn (Song and Marvizon, 2005); and BK channel–mediated negative feedback on NMDAR-mediated dendritic spine Ca2+ transients in the cartwheel cells of the dorsal cochlear nucleus (He et al., 2014).
Comparison of BK-CaV and BK-NMDAR Coupling
Compared with BK-CaV coupling, BK-NMDAR coupling has distinct kinetic and functional properties. It produces unique neurotransmitter release–dependent K+ signaling. NMDARs provide more sustained Ca2+ sources for BK channel activation than do CaV channels because of the slow rate of NMDAR deactivation (τ = ∼40 ms–2 s). Functional coupling of BK channels with NMDARs at extrasynaptic sites results in glutamate spillover–induced, extrasynaptic BK channel activation and neuronal activity inhibition. Postsynaptic NMDARs coincidently detect presynaptic glutamate release and postsynaptic membrane depolarization and can thus facilitate BK channel activation in a spike timing–dependent manner owing to the intrinsic properties of NMDAR-mediated Ca2+ influx. The resultant BK channel K+ currents reduce the amplitude of EPSPs and promote Mg2+ blockade of NMDARs by repolarizing postsynaptic membranes. Given the involvement of BK channels in learning and memory (Matthews and Disterhoft, 2009; Ye et al., 2010; Typlt et al., 2013; Springer et al., 2014), BK channels may regulate long-term potentiation or depression via postsynaptic BK-NMDAR coupling. It is of note that the other types of KCa channels, e.g., SK channels, are also reported to be functionally coupled with CaV channels and NMDA receptors (NMDARs) (Marrion and Tavalin, 1998; Ngo-Anh et al., 2005; Wang et al., 2014). However, there is scarce evidence on the physical complex formation between Ca2+ channels and SK or IK channels, which is consistent with the lack of the requirement for strict, close colocalization for functional coupling. In spite of the physiological significance, the coupling of IK and SK channels with Ca2+-permeable channels is beyond the focus of this review. Previous reviews of this topic can be found elsewhere (Fakler and Adelman, 2008; Gueguinou et al., 2014).
Coupling of BK Channels With Other Channels
Coupling of BK Channels With Intracellular Ca2+-Release Channels
Ca2+-release channels are intracellular Ca2+ channels responsible for the release of Ca2+ from endoplasmic and sarcoplasmic reticulum (ER and SR) which form the intracellular Ca2+ stores (Woll and Van Petegem, 2022). They are giant membrane proteins consisting of two evolutionarily related gene families, ryanodine receptors (RyRs) and inositol-1,4,5-trisphosphate receptors (IP3Rs). The three RyR isoforms (RyR1, RyR2, and RyR3) are each ∼ 5,000 amino acid residue polypeptides that assemble into ∼2.2 MDa homotetrameric channels. They are expressed in different tissues including brains. As they are mostly studied in the context of muscle contraction, RyR1 is known as the skeletal muscle isoform and RyR2 as the cardiac isoform. RyR3 is ubiquitously expressed. The three IP3R isoforms (IP3R1, IP3R2, and IP3R3) are each ∼2,700 amino acid residue polypeptides that assemble into ∼1.2 MDa homotetrameric or heterotetrameric channels. IP3Rs have a broad tissue distribution with a high abundance in the cerebellum. Both RyRs and IP3Rs are sensitive to changes in cytosolic Ca2+ concentrations in that the channels are stimulated by rises in the cytosolic Ca2+ concentration but inhibited by high cytosolic Ca2+ concentration. RyRs are activated by Ca2+-influx mediated by plasma membrane CaV channels via a mechanism of Ca2+-induced Ca2+ release (CICR). IP3Rs are activated by the cytosolic IP3 molecule formed by protein lipase C (PLC) through hydrolysis of phosphatidylinositol 4,5-bisphosphate (PIP2) into IP3 and diacylglycerol (DAG). PLC is activated through a G-protein-coupled receptor (GPCR) or receptor tyrosine kinase (RTK) signaling pathway.
BK and CaV channels on somatic plasma membranes (PM) couple to RyRs on ER to form double (CaV-RyR and RyR-BK) PM-ER nanodomains in Ca2+ signaling in the cartwheel inhibitory interneurons of the dorsal cochlear nucleus (Irie and Trussell, 2017). The triad CaV/RyR/BK channel coupling (Figure 5) was reported to be EGTA-resistant and rapid (within the time of a single spike), and thus provided a mechanism for the rapid control of action potentials on a millisecond timescale (Irie and Trussell, 2017). Immunofluorescence analysis showed partial overlap of puncta of BK and RyR labeling in mouse cartwheel cells (Irie and Trussell, 2017). In mouse dentate gyrus granule neurons, knockout of the BK channel β4 subunit caused increased functional coupling between RyR and BK channels, resulted in an increase in the fAHP amplitude (Wang et al., 2016). A similar effect was observed by knockin of the seizure-prone gain-of-function (R2474S) RyR mutant channels. This study revealed different roles of the BK-CaV and BK-RyR coupling during action potential in that BK channel activation is dependent on L-type CaV channels in repolarization phase but RyRs during fAHP (Wang et al., 2016). In cerebral smooth muscle cells, another type of triad channel coupling, TRPV4/RyR/BK, has been reported for RyR-induced BK channel activation (Earley et al., 2005). Activation of TRPV4 by 11,12 EET in freshly isolated cerebral myocytes led to elevated Ca2+ spark from RyRs and transient BK activity which was unaffected by inhibition of CaV channels. The TRPV4-RyR-BK channel coupling accounts for smooth muscle hyperpolarization and arterial dilation via Ca2+-induced Ca2+ release in response to TRPV4 activation by an endothelial-derived factor (Earley et al., 2005). However, whether the coupling also occurs within nanodomains is unclear, as no Ca2+ chelators were used to probe the strengths of the Ca2+ coupling.
Potential functional coupling of BK channels with IP3Rs was reported in glioma cells via colocalization within lipid rafts (Weaver et al., 2007). With whole cell patch-clamp recording, it was observed that the voltage-induced BK channel activity was reduced upon lipid raft disruption with methyl-β-cyclodextrin. Pretreatment of glioma cells with thapsigargin to deplete the intracellular Ca2+ store or 2-aminoethoxydiphenyl borate to inhibit IP3Rs negated the effect of methyl-β-cyclodextrin. Stimulation of muscarinic acetylcholine receptors (mAChRs) with muscarine or acetylcholine (ACh), which was known to promote IP3 formation, elicited an increase in [Ca2+]i that subsequently activated BK channels and caused cell hyperpolarization. Disruption of lipid rafts or inhibition of IP3Rs prevented the ACh-induced rise in [Ca2+]i and the BK channel-induced hyperpolarization of the membrane. Both BK channels and IP3Rs were found to associate or localize with lipid rafts as detected by immunoblot in the isolated lipid raft fractions or by immunofluorescence on cells. Given the presence of 10 mM EGTA in the pipette solution of the whole cell recording and the sensitivity to Ca2+ store depletion, it is likely that the BK-IP3R coupling involves Ca2+ and the coupling exists within nanodomain. However, the lack of data for comparison with/without EGTA or BAPTA prevents drawing a solid conclusion. Another instance of potential BK-IP3Rs interactions was observed in rat and mouse cerebral artery smooth muscle cells (Zhao et al., 2010). Activation of BK channels by IP3 or its membrane-permeable analog occurred in both intact cells and excised membrane patches via cell-attached and inside-out patch-clamp recording configurations, respectively. Inhibition of IP3Rs, knockout of IP3R1, or application of an IP3R1 antibody suppressed the IP3-induced BK channel activation in inside-out recording of excised membrane patches. Immuno-FRET imaging analysis indicated BK-RyR1 colocalization. The BK channel α and β1 subunit were detected to be coimmunoprecipitated with RyR1. However, the IP3-induced BK channel activation in inside-out recording was time-independent after membrane patch excision and was insensitive to both BAPTA and EGTA, indicating some SR Ca2+-release independent mechanism. In another conflicting report, the IP3 was found to activate BK channel in pig coronary artery smooth muscle cells via an IP3R-indepenent mechanism (Yang et al., 2013). Therefore, uncertainty exists whether there is Ca2+-mediated BK-IP3R coupling and caution is needed in interpretation of the data on IP3-related BK channel activation.
Coupling of BK Channels With Transient Receptor Potential Channels
BK channels have also been reported to functionally couple with TRP channels. TRP channels are a superfamily of cation channels whose activation mechanisms are more diverse than those of any other group of ion channels. TRP channels play critical roles in sensory physiology, including vision, taste, olfaction, hearing, touch, and thermo- and osmosensation. The TRP superfamily is divided into 7 subfamilies: 5 group 1 TRPs (TRPC, TRPV, TRPM, TRPN, and TRPA) and 2 group 2 subfamilies (TRPP and TRPML). The transient receptor potential vanilloid receptor 1(TRPV1) channel is a non-selective cation channel activated by a variety of exogenous and endogenous physical and chemical stimuli, such as temperature and capsaicin. In the heterologous expression system of HEK293 cells, BK channels were found to be activated by Ca2+ influx through TRPV1 channels in a largely EGTA-insensitive manner (Wu et al., 2013). The TRPV1-induced BK currents were also observed in dorsal root ganglion (DRG) cells (Wu et al., 2013). Coimmunoprecipitation showed formation of the BK-TRPV1 complex in both HEK293 and DRG cells. There have also been reports of the functional coupling of BK channels with TRPV4 in human bronchial epithelial cell lines (Fernandez-Fernandez et al., 2008) and with TRPC1 in vascular smooth muscle cells (Kwan et al., 2009). Activation of TRPV4 by activator, osmotic and mechanical stimulation led to BK channel activities, which was lost upon of TRPV4 knockdown (Fernandez-Fernandez et al., 2008). Coimmunoprecipitation and immunofluorescence showed complex formation between BK and TRPC1 channels in HEK293 cells and their colocalization in vascular smooth muscle cells (Kwan et al., 2009). The BK-TRPC1 coupling was found to play a role in agonist-induced membrane depolarization and vascular contraction in isolated rat mesenteric arteries (Kwan et al., 2009). Whether the BK-TRPV4 and BK-TRPC1 couplings occurred within nanodomain is unknown, as no data on EGTA-sensitivity were presented in both studies (Fernandez-Fernandez et al., 2008; Kwan et al., 2009) and no protein complex formation and colocalization was demonstrated in the BK-TRPV4 coupling case (Kwan et al., 2009). Coimmunoprecipitation of BK with TRPC3 and TRPC6 channels was observed in differentiated podocyte cell line and heterologous expression system of HEK293 cells (Kim et al., 2009). TRPC3 but not TRPC6 was found to increase the surface expression of BKα subunit splice variant (Slo1VEDEC) (Kim et al., 2009). However, the possibility of Ca2+-mediated functional coupling of the BK with TRPC3 or TRPC6 channels was not addressed in this report (Kim et al., 2009).
Discussion
Reported studies have clearly demonstrated the nanodomain functional couplings of BK channels with Ca2+-permeable channels, particularly the CaV and NMDAR channels, in a variety of different cells. These mechanisms of nanodomain couplings allow BK channels to play diverse cellular and physiological roles. Given the BK channel’s widespread expression and critical physiological roles, directly targeting it can have unavoidable adverse side effects. Selective enhancement or disruption of the interactions between BK channels and Ca2+ permeable channels using small chemicals, peptidomimetic molecules, or genetic methods can be an effective way to modify BK channel activity or to indirectly intervene in CaV or NMDAR function by limiting or enhancing the negative feedback from BK channels. It will be important to know how they organize and interact on membrane within nanodomains for effective functional coupling. However, the biochemical bases of the interactions of BK channels with Ca2+ permeable channels, either direct or indirect, remain mostly unknown. The recent advances in the cryo-EM–based structural determination of ion channels have given us a better understanding of the structure-function relationship of ion channels. Future determination of the structures of whole coupling complexes should enable researchers to develop potent and specific inhibitors or activators of these complexes and thus design novel therapeutic interventions. The initial investigation into the biochemical basis of BK-NMDAR coupling found direct physical interactions between the BK and NMDAR intracellular regions and domains (Zhang et al., 2018). Encouragingly, the synthetic S0–S1 peptide of BK channels was shown to be effective in disrupting the BK-NMDAR interactions and couplings (Zhang et al., 2018).
BK-CaV nanodomain coupling intertwines the functions of these two types of channels in the regulation of neuronal excitability. Compared to the cellular and physiological roles of BK-CaV coupling, those of BK-NMDAR coupling in different cells are less studied and understood. Postsynaptic and extrasynaptic BK-NMDAR couplings (Figure 5) provide unique neurotransmitter-dependent Ca2+ sources for BK channel activation and function, which could be important for synaptic plasticity, as was noted in the recent study (Gomez et al., 2021). More studies in different brain regions or neurons under normal or pathological conditions could expand our understanding of the physiological and pathological roles of BK-NMDAR coupling. NMDARs play numerous physiological and pathological roles. The extent to which BK-NMDAR coupling contributes to NMDAR-mediated Ca2+ signaling remains to be determined. The development of pharmacological tools that specifically interrupt BK-NMDAR interactions, such as the synthetic S0–S1 peptide (Zhang et al., 2018), will be helpful in determining the physiological and pathological roles of BK-NMDAR coupling. For the structural and functional coupling of BK channels with other Ca2+ permeable channels within nanodomain, the reported studies remain sparse or very limited in evidence. More studies will be needed to establish the structural and functional coupling, determine the underlying biochemical mechanisms, and understand the physiological roles.
Author Contributions
All authors listed have made a substantial, direct, and intellectual contribution to the work, and approved it for publication.
Funding
This work was supported by National Institutes of Health grants NS078152 (JY).
Conflict of Interest
The authors declare that the research was conducted in the absence of any commercial or financial relationships that could be construed as a potential conflict of interest.
Publisher’s Note
All claims expressed in this article are solely those of the authors and do not necessarily represent those of their affiliated organizations, or those of the publisher, the editors and the reviewers. Any product that may be evaluated in this article, or claim that may be made by its manufacturer, is not guaranteed or endorsed by the publisher.
Acknowledgments
We thank Laura L. Russell, scientific editor, Research Medical Library, for editing this article.
References
Augustine, G. J., Santamaria, F., and Tanaka, K. (2003). Local calcium signaling in neurons. Neuron 40, 331–346. doi: 10.1016/S0896-6273(03)00639-1
Behrens, R., Nolting, A., Reimann, F., Schwarz, M., Waldschutz, R., and Pongs, O. (2000). hKCNMB3 and hKCNMB4, cloning and characterization of two members of the large-conductance calcium-activated potassium channel beta subunit family. FEBS Lett. 474, 99–106. doi: 10.1016/S0014-5793(00)01584-2
Berkefeld, H., and Fakler, B. (2008). Repolarizing responses of BKCa-CaV complexes are distinctly shaped by their Cav subunits. J. Neurosci. 28, 8238–8245. doi: 10.1523/JNEUROSCI.2274-08.2008
Berkefeld, H., Sailer, C. A., Bildl, W., Rohde, V., Thumfart, J. O., Eble, S., et al. (2006). BKCa-CaV channel complexes mediate rapid and localized Ca2+-activated K+ signaling. Science 314, 615–620. doi: 10.1126/science.1132915
Bourinet, E., Altier, C., Hildebrand, M. E., Trang, T., Salter, M. W., and Zamponi, G. W. (2014). Calcium-permeable ion channels in pain signaling. Physiol. Rev. 94, 81–140. doi: 10.1152/physrev.00023.2013
Brenner, R., Chen, Q. H., Vilaythong, A., Toney, G. M., Noebels, J. L., and Aldrich, R. W. (2005). BK channel beta4 subunit reduces dentate gyrus excitability and protects against temporal lobe seizures. Nat. Neurosci. 8, 1752–1759. doi: 10.1038/nn1573
Brenner, R., Jegla, T. J., Wickenden, A., Liu, Y., and Aldrich, R. W. (2000). Cloning and functional characterization of novel large conductance calcium-activated potassium channel beta subunits, hKCNMB3 and hKCNMB4. J. Biol. Chem. 275, 6453–6461. doi: 10.1074/jbc.275.9.6453
Cao, X. H., Chen, S. R., Li, L., and Pan, H. L. (2012). Nerve injury increases brain-derived neurotrophic factor levels to suppress BK channel activity in primary sensory neurons. J. Neurochem. 121, 944–953. doi: 10.1111/j.1471-4159.2012.07736.x
Chen, S. R., Cai, Y. Q., and Pan, H. L. (2009). Plasticity and emerging role of BKCa channels in nociceptive control in neuropathic pain. J. Neurochem. 110, 352–362. doi: 10.1111/j.1471-4159.2009.06138.x
Cox, D. H. (2014). Modeling a Ca2+ channel/BKCa channel complex at the single-complex level. Biophys. J. 107, 2797–2814. doi: 10.1016/j.bpj.2014.10.069
Cox, D. H., and Aldrich, R. W. (2000). Role of the beta1 subunit in large-conductance Ca2+-activated K+ channel gating energetics. Mechanisms of enhanced Ca2+ sensitivity. J. Gen. Physiol. 116, 411–432. doi: 10.1085/jgp.116.3.411
Davies, P. J., Ireland, D. R., Martinez-Pinna, J., and Mclachlan, E. M. (1999). Electrophysiological roles of L-type channels in different classes of guinea pig sympathetic neuron. J. Neurophysiol. 82, 818–828. doi: 10.1152/jn.1999.82.2.818
Deng, P. Y., Rotman, Z., Blundon, J. A., Cho, Y., Cui, J., Cavalli, V., et al. (2013). FMRP regulates neurotransmitter release and synaptic information transmission by modulating action potential duration via BK channels. Neuron 77, 696–711. doi: 10.1016/j.neuron.2012.12.018
Du, W., Bautista, J. F., Yang, H., Diez-Sampedro, A., You, S. A., Wang, L., et al. (2005). Calcium-sensitive potassium channelopathy in human epilepsy and paroxysmal movement disorder. Nat. Genet. 37, 733–738. doi: 10.1038/ng1585
Earley, S., Heppner, T. J., Nelson, M. T., and Brayden, J. E. (2005). TRPV4 forms a novel Ca2+ signaling complex with ryanodine receptors and BKCa channels. Circ. Res. 97, 1270–1279. doi: 10.1161/01.RES.0000194321.60300.d6
Edgerton, J. R., and Reinhart, P. H. (2003). Distinct contributions of small and large conductance Ca2+-activated K+ channels to rat Purkinje neuron function. J. Physiol. 548, 53–69. doi: 10.1113/jphysiol.2002.027854
Eggermann, E., Bucurenciu, I., Goswami, S. P., and Jonas, P. (2011). Nanodomain coupling between Ca2+ channels and sensors of exocytosis at fast mammalian synapses. Nat. Rev. Neurosci. 13, 7–21. doi: 10.1038/nrn3125
Fakler, B., and Adelman, J. P. (2008). Control of K(Ca) channels by calcium nano/microdomains. Neuron 59, 873–881. doi: 10.1016/j.neuron.2008.09.001
Farajnia, S., Meijer, J. H., and Michel, S. (2015). Age-related changes in large-conductance calcium-activated potassium channels in mammalian circadian clock neurons. Neurobiol. Aging 36, 2176–2183. doi: 10.1016/j.neurobiolaging.2014.12.040
Fernandez-Fernandez, J. M., Andrade, Y. N., Arniges, M., Fernandes, J., Plata, C., Rubio-Moscardo, F., et al. (2008). Functional coupling of TRPV4 cationic channel and large conductance, calcium-dependent potassium channel in human bronchial epithelial cell lines. Pflugers Arch. 457, 149–159. doi: 10.1007/s00424-008-0516-3
Fettiplace, R., and Fuchs, P. A. (1999). Mechanisms of hair cell tuning. Annu. Rev. Physiol. 61, 809–834. doi: 10.1146/annurev.physiol.61.1.809
Ghatta, S., Nimmagadda, D., Xu, X., and O’rourke, S. T. (2006). Large-conductance, calcium-activated potassium channels: structural and functional implications. Pharmacol. Ther. 110, 103–116. doi: 10.1016/j.pharmthera.2005.10.007
Gola, M., and Crest, M. (1993). Colocalization of active KCa channels and Ca2+ channels within Ca2+ domains in helix neurons. Neuron 10, 689–699. doi: 10.1016/0896-6273(93)90170-V
Goldberg, J. A., and Wilson, C. J. (2005). Control of spontaneous firing patterns by the selective coupling of calcium currents to calcium-activated potassium currents in striatal cholinergic interneurons. J. Neurosci. 25, 10230–10238. doi: 10.1523/JNEUROSCI.2734-05.2005
Golding, N. L., Jung, H. Y., Mickus, T., and Spruston, N. (1999). Dendritic calcium spike initiation and repolarization are controlled by distinct potassium channel subtypes in CA1 pyramidal neurons. J. Neurosci. 19, 8789–8798. doi: 10.1523/JNEUROSCI.19-20-08789.1999
Gomez, R., Maglio, L. E., Gonzalez-Hernandez, A. J., Rivero-Perez, B., Bartolome-Martin, D., and Giraldez, T. (2021). NMDA receptor-BK channel coupling regulates synaptic plasticity in the barrel cortex. Proc. Natl. Acad. Sci. USA 118: e2107026118. doi: 10.1073/pnas.2107026118
Gonzalez-Perez, V., and Lingle, C. J. (2019). Regulation of BK channels by beta and gamma subunits. Annu. Rev. Physiol. 81, 113–137. doi: 10.1146/annurev-physiol-022516-034038
Gonzalez-Perez, V., Martinez-Espinosa, P. L., Sala-Rabanal, M., Bharadwaj, N., Xia, X. M., Chen, A. C., et al. (2021). Goblet cell LRRC26 regulates BK channel activation and protects against colitis in mice. Proc. Natl. Acad. Sci. USA 118: e2019149118. doi: 10.1073/pnas.2019149118
Grunnet, M., and Kaufmann, W. A. (2004). Coassembly of big conductance Ca2+-activated K+ channels and L-type voltage-gated Ca2+ channels in rat brain. J. Biol. Chem. 279, 36445–36453. doi: 10.1074/jbc.M402254200
Gueguinou, M., Chantome, A., Fromont, G., Bougnoux, P., Vandier, C., and Potier-Cartereau, M. (2014). KCa and Ca2+ channels: the complex thought. Biochim. Biophys. Acta 1843, 2322–2333. doi: 10.1016/j.bbamcr.2014.02.019
Gutzmann, J. J., Lin, L., and Hoffman, D. A. (2019). Functional Coupling of Cav2.3 and BK potassium channels regulates action potential repolarization and short-term plasticity in the mouse hippocampus. Front. Cell Neurosci. 13:27. doi: 10.3389/fncel.2019.00027
He, S., Wang, Y. X., Petralia, R. S., and Brenowitz, S. D. (2014). Cholinergic modulation of large-conductance calcium-activated potassium channels regulates synaptic strength and spine calcium in cartwheel cells of the dorsal cochlear nucleus. J. Neurosci. 34, 5261–5272. doi: 10.1523/JNEUROSCI.3728-13.2014
Higgins, J. J., Hao, J., Kosofsky, B. E., and Rajadhyaksha, A. M. (2008). Dysregulation of large-conductance Ca2+-activated K+ channel expression in nonsyndromal mental retardation due to a cereblon p.R419X mutation. Neurogenetics 9, 219–223. doi: 10.1007/s10048-008-0128-2
Hu, H., Shao, L. R., Chavoshy, S., Gu, N., Trieb, M., Behrens, R., et al. (2001). Presynaptic Ca2+-activated K+ channels in glutamatergic hippocampal terminals and their role in spike repolarization and regulation of transmitter release. J. Neurosci. 21, 9585–9597. doi: 10.1523/JNEUROSCI.21-24-09585.2001
Irie, T., and Trussell, L. O. (2017). Double-nanodomain coupling of calcium channels, ryanodine receptors, and BK channels controls the generation of burst firing. Neuron 96:e854. doi: 10.1016/j.neuron.2017.10.014
Isaacson, J. S., and Murphy, G. J. (2001). Glutamate-mediated extrasynaptic inhibition: direct coupling of NMDA receptors to Ca2+-activated K+ channels. Neuron 31, 1027–1034. doi: 10.1016/S0896-6273(01)00428-7
Karakas, E., and Furukawa, H. (2014). Crystal structure of a heterotetrameric NMDA receptor ion channel. Science 344, 992–997. doi: 10.1126/science.1251915
Kim, E. Y., Alvarez-Baron, C. P., and Dryer, S. E. (2009). Canonical transient receptor potential channel (TRPC)3 and TRPC6 associate with large-conductance Ca2+-activated K+ (BKCa) channels: role in BKCa trafficking to the surface of cultured podocytes. Mol. Pharmacol. 75, 466–477. doi: 10.1124/mol.108.051912
Kwan, H. Y., Shen, B., Ma, X., Kwok, Y. C., Huang, Y., Man, Y. B., et al. (2009). TRPC1 associates with BKCa channel to form a signal complex in vascular smooth muscle cells. Circ. Res. 104, 670–678. doi: 10.1161/CIRCRESAHA.108.188748
Lakhan, S. E., Caro, M., and Hadzimichalis, N. (2013). NMDA receptor activity in neuropsychiatric disorders. Front. Psychiatry 4:52. doi: 10.3389/fpsyt.2013.00052
Latorre, R., Castillo, K., Carrasquel-Ursulaez, W., Sepulveda, R. V., Gonzalez-Nilo, F., Gonzalez, C., et al. (2017). Molecular determinants of BK channel functional diversity and functioning. Physiol. Rev. 97, 39–87. doi: 10.1152/physrev.00001.2016
Laumonnier, F., Roger, S., Guerin, P., Molinari, F., Cahard, D., Belhadj, A., et al. (2006). Association of a functional deficit of the BKCa channel, a synaptic regulator of neuronal excitability, with autism and mental retardation. Am. J. Psychiatry 163, 1622–1629. doi: 10.1176/ajp.2006.163.9.1622
Li, Q., and Yan, J. (2016). Modulation of BK Channel Function by Auxiliary Beta and Gamma Subunits. Int. Rev. Neurobiol. 128, 51–90. doi: 10.1016/bs.irn.2016.03.015
Lingle, C. J. (2019). LRRC52 regulates BK channel function and localization in mouse cochlear inner hair cells. Proc. Natl. Acad. Sci. USA 116, 18397–18403. doi: 10.1073/pnas.1907065116
Liu, G., Shi, J., Yang, L., Cao, L., Park, S. M., Cui, J., et al. (2004). Assembly of a Ca2+-dependent BK channel signaling complex by binding to beta2 adrenergic receptor. EMBO J. 23, 2196–2205. doi: 10.1038/sj.emboj.7600228
Loane, D. J., Lima, P. A., and Marrion, N. V. (2007). Co-assembly of N-type Ca2+ and BK channels underlies functional coupling in rat brain. J. Cell Sci. 120, 985–995. doi: 10.1242/jcs.03399
Ma, Y., Hinde, E., and Gaus, K. (2015). Nanodomains in biological membranes. Essays Biochem. 57, 93–107. doi: 10.1042/bse0570093
Mancini, M., Soldovieri, M. V., Gessner, G., Wissuwa, B., Barrese, V., Boscia, F., et al. (2014). Critical role of large-conductance calcium- and voltage-activated potassium channels in leptin-induced neuroprotection of N-methyl-d-aspartate-exposed cortical neurons. Pharmacol. Res. 87, 80–86. doi: 10.1016/j.phrs.2014.06.010
Marrion, N. V., and Tavalin, S. J. (1998). Selective activation of Ca2+-activated K+ channels by co-localized Ca2+ channels in hippocampal neurons. Nature 395, 900–905. doi: 10.1038/27674
Matthews, E. A., and Disterhoft, J. F. (2009). Blocking the BK channel impedes acquisition of trace eyeblink conditioning. Learn Mem. 16, 106–109. doi: 10.1101/lm.1289809
Matveev, V., Zucker, R. S., and Sherman, A. (2004). Facilitation through buffer saturation: constraints on endogenous buffering properties. Biophys. J. 86, 2691–2709. doi: 10.1016/S0006-3495(04)74324-6
Meredith, A. L., Wiler, S. W., Miller, B. H., Takahashi, J. S., Fodor, A. A., Ruby, N. F., et al. (2006). BK calcium-activated potassium channels regulate circadian behavioral rhythms and pacemaker output. Nat. Neurosci. 9, 1041–1049. doi: 10.1038/nn1740
Misonou, H., Menegola, M., Buchwalder, L., Park, E. W., Meredith, A., Rhodes, K. J., et al. (2006). Immunolocalization of the Ca2+-activated K+ channel Slo1 in axons and nerve terminals of mammalian brain and cultured neurons. J. Comp. Neurol. 496, 289–302. doi: 10.1002/cne.20931
Montefusco, F., Tagliavini, A., Ferrante, M., and Pedersen, M. G. (2017). Concise whole-cell modeling of BKCa-CaV activity controlled by local coupling and stoichiometry. Biophys. J. 112, 2387–2396. doi: 10.1016/j.bpj.2017.04.035
Montgomery, J. R., Whitt, J. P., Wright, B. N., Lai, M. H., and Meredith, A. L. (2013). Mis-expression of the BK K+ channel disrupts suprachiasmatic nucleus circuit rhythmicity and alters clock-controlled behavior. Am. J. Physiol. Cell Physiol. 304, C299–C311. doi: 10.1152/ajpcell.00302.2012
Moreno, C. M., Dixon, R. E., Tajada, S., Yuan, C., Opitz-Araya, X., Binder, M. D., et al. (2016). Ca2+ entry into neurons is facilitated by cooperative gating of clustered CaV1.3 channels. Elife 5: e15744. doi: 10.7554/eLife.15744
Muller, A., Kukley, M., Uebachs, M., Beck, H., and Dietrich, D. (2007). Nanodomains of single Ca2+ channels contribute to action potential repolarization in cortical neurons. J. Neurosci. 27, 483–495. doi: 10.1523/JNEUROSCI.3816-06.2007
Nanou, E., and Catterall, W. A. (2018). Calcium channels, synaptic plasticity, and neuropsychiatric disease. Neuron 98, 466–481. doi: 10.1016/j.neuron.2018.03.017
Naraghi, M., and Neher, E. (1997). Linearized buffered Ca2+ diffusion in microdomains and its implications for calculation of [Ca2+] at the mouth of a calcium channel. J. Neurosci. 17, 6961–6973. doi: 10.1523/JNEUROSCI.17-18-06961.1997
Ngo-Anh, T. J., Bloodgood, B. L., Lin, M., Sabatini, B. L., Maylie, J., and Adelman, J. P. (2005). SK channels and NMDA receptors form a Ca2+-mediated feedback loop in dendritic spines. Nat. Neurosci. 8, 642–649. doi: 10.1038/nn1449
Onimaru, H., Ballanyi, K., and Homma, I. (2003). Contribution of Ca2+-dependent conductances to membrane potential fluctuations of medullary respiratory neurons of newborn rats in vitro. J. Physiol. 552, 727–741. doi: 10.1113/jphysiol.2003.049312
Paoletti, P. (2011). Molecular basis of NMDA receptor functional diversity. Eur. J. Neurosci. 33, 1351–1365. doi: 10.1111/j.1460-9568.2011.07628.x
Paoletti, P., Bellone, C., and Zhou, Q. (2013). NMDA receptor subunit diversity: impact on receptor properties, synaptic plasticity and disease. Nat. Rev. Neurosci. 14, 383–400. doi: 10.1038/nrn3504
Pitts, G. R., Ohta, H., and Mcmahon, D. G. (2006). Daily rhythmicity of large-conductance Ca2+ -activated K+ currents in suprachiasmatic nucleus neurons. Brain Res. 1071, 54–62. doi: 10.1016/j.brainres.2005.11.078
Prakriya, M., and Lingle, C. J. (1999). BK channel activation by brief depolarizations requires Ca2+ influx through L- and Q-type Ca2+ channels in rat chromaffin cells. J. Neurophysiol. 81, 2267–2278. doi: 10.1152/jn.1999.81.5.2267
Prakriya, M., and Lingle, C. J. (2000). Activation of BK channels in rat chromaffin cells requires summation of Ca2+ influx from multiple Ca2+ channels. J. Neurophysiol. 84, 1123–1135. doi: 10.1152/jn.2000.84.3.1123
Protti, D. A., and Uchitel, O. D. (1997). P/Q-type calcium channels activate neighboring calcium-dependent potassium channels in mouse motor nerve terminals. Pflugers Arch. 434, 406–412. doi: 10.1007/s004240050414
Raffaelli, G., Saviane, C., Mohajerani, M. H., Pedarzani, P., and Cherubini, E. (2004). BK potassium channels control transmitter release at CA3-CA3 synapses in the rat hippocampus. J. Physiol. 557, 147–157. doi: 10.1113/jphysiol.2004.062661
Rehak, R., Bartoletti, T. M., Engbers, J. D., Berecki, G., Turner, R. W., and Zamponi, G. W. (2013). Low voltage activation of KCa1.1 current by Cav3-KCa1.1 complexes. PLoS One 8:e61844. doi: 10.1371/journal.pone.0061844
Robitaille, R., Garcia, M. L., Kaczorowski, G. J., and Charlton, M. P. (1993). Functional colocalization of calcium and calcium-gated potassium channels in control of transmitter release. Neuron 11, 645–655. doi: 10.1016/0896-6273(93)90076-4
Runden-Pran, E., Haug, F. M., Storm, J. F., and Ottersen, O. P. (2002). BK channel activity determines the extent of cell degeneration after oxygen and glucose deprivation: a study in organotypical hippocampal slice cultures. Neuroscience 112, 277–288. doi: 10.1016/S0306-4522(02)00092-1
Sailer, C. A., Kaufmann, W. A., Kogler, M., Chen, L., Sausbier, U., Ottersen, O. P., et al. (2006). Immunolocalization of BK channels in hippocampal pyramidal neurons. Eur. J. Neurosci. 24, 442–454. doi: 10.1111/j.1460-9568.2006.04936.x
Salkoff, L., Butler, A., Ferreira, G., Santi, C., and Wei, A. (2006). High-conductance potassium channels of the SLO family. Nat. Rev. Neurosci. 7, 921–931. doi: 10.1038/nrn1992
Samengo, I., Curro, D., Barrese, V., Taglialatela, M., and Martire, M. (2014). Large conductance calcium-activated potassium channels: their expression and modulation of glutamate release from nerve terminals isolated from rat trigeminal caudal nucleus and cerebral cortex. Neurochem. Res. 39, 901–910. doi: 10.1007/s11064-014-1287-1
Sausbier, M., Hu, H., Arntz, C., Feil, S., Kamm, S., Adelsberger, H., et al. (2004). Cerebellar ataxia and Purkinje cell dysfunction caused by Ca2+-activated K+ channel deficiency. Proc. Natl. Acad. Sci. USA 101, 9474–9478. doi: 10.1073/pnas.0401702101
Schwaller, B. (2010). Cytosolic Ca2+ buffers. Cold Spring Harb. Persp. Biol. 2:a004051. doi: 10.1101/cshperspect.a004051
Sclip, A., Acuna, C., Luo, F., and Sudhof, T. C. (2018). RIM-binding proteins recruit BK-channels to presynaptic release sites adjacent to voltage-gated Ca2+-channels. EMBO J. 37: e98637. doi: 10.15252/embj.201798637
Shao, L. R., Halvorsrud, R., Borg-Graham, L., and Storm, J. F. (1999). The role of BK-type Ca2+-dependent K+ channels in spike broadening during repetitive firing in rat hippocampal pyramidal cells. J. Physiol. 521, 135–146. doi: 10.1111/j.1469-7793.1999.00135.x
Shen, Y., Kishimoto, K., Linden, D. J., and Sapirstein, A. (2007). Cytosolic phospholipase A(2) alpha mediates electrophysiologic responses of hippocampal pyramidal neurons to neurotoxic NMDA treatment. Proc. Natl. Acad. Sci. USA 104, 6078–6083. doi: 10.1073/pnas.0605427104
Soderberg, O., Gullberg, M., Jarvius, M., Ridderstrale, K., Leuchowius, K. J., Jarvius, J., et al. (2006). Direct observation of individual endogenous protein complexes in situ by proximity ligation. Nat. Methods 3, 995–1000. doi: 10.1038/nmeth947
Solaro, C. R., and Lingle, C. J. (1992). Trypsin-sensitive, rapid inactivation of a calcium-activated potassium channel. Science 257, 1694–1698. doi: 10.1126/science.1529355
Song, B., and Marvizon, J. C. (2005). N-methyl-D-aspartate receptors and large conductance calcium-sensitive potassium channels inhibit the release of opioid peptides that induce mu-opioid receptor internalization in the rat spinal cord. Neuroscience 136, 549–562. doi: 10.1016/j.neuroscience.2005.08.032
Springer, S. J., Burkett, B. J., and Schrader, L. A. (2014). Modulation of BK channels contributes to activity-dependent increase of excitability through MTORC1 activity in CA1 pyramidal cells of mouse hippocampus. Front. Cell Neurosci. 8:451. doi: 10.3389/fncel.2014.00451
Storm, J. F. (1987). Action potential repolarization and a fast after-hyperpolarization in rat hippocampal pyramidal cells. J. Physiol. 385, 733–759. doi: 10.1113/jphysiol.1987.sp016517
Sun, X., Gu, X. Q., and Haddad, G. G. (2003). Calcium influx via L- and N-type calcium channels activates a transient large-conductance Ca2+-activated K+ current in mouse neocortical pyramidal neurons. J. Neurosci. 23, 3639–3648. doi: 10.1523/JNEUROSCI.23-09-03639.2003
Tao, X., and MacKinnon, R. (2019). Molecular structures of the human Slo1 K+ channel in complex with β4. Elife 8:e51409. doi: 10.7554/eLife.51409
Tay, L. H., Dick, I. E., Yang, W., Mank, M., Griesbeck, O., and Yue, D. T. (2012). Nanodomain Ca2+ of Ca2+ channels detected by a tethered genetically encoded Ca2+ sensor. Nat. Commun. 3:778. doi: 10.1038/ncomms1777
Typlt, M., Mirkowski, M., Azzopardi, E., Ruettiger, L., Ruth, P., and Schmid, S. (2013). Mice with deficient BK channel function show impaired prepulse inhibition and spatial learning, but normal working and spatial reference memory. PLoS One 8:e81270. doi: 10.1371/journal.pone.0081270
Vivas, O., Kruse, M., and Hille, B. (2014). Nerve growth factor sensitizes adult sympathetic neurons to the proinflammatory peptide bradykinin. J. Neurosci. 34, 11959–11971. doi: 10.1523/JNEUROSCI.1536-14.2014
Vivas, O., Moreno, C. M., Santana, L. F., and Hille, B. (2017). Proximal clustering between BK and CaV1.3 channels promotes functional coupling and BK channel activation at low voltage. Elife 6:e28029. doi: 10.7554/eLife.28029
Wang, B., Bugay, V., Ling, L., Chuang, H. H., Jaffe, D. B., and Brenner, R. (2016). Knockout of the BK beta4-subunit promotes a functional coupling of BK channels and ryanodine receptors that mediate a fAHP-induced increase in excitability. J. Neurophysiol. 116, 456–465. doi: 10.1152/jn.00857.2015
Wang, K., Lin, M. T., Adelman, J. P., and Maylie, J. (2014). Distinct Ca2+ sources in dendritic spines of hippocampal CA1 neurons couple to SK and Kv4 channels. Neuron 81, 379–387. doi: 10.1016/j.neuron.2013.11.004
Waxman, S. G., and Zamponi, G. W. (2014). Regulating excitability of peripheral afferents: emerging ion channel targets. Nat. Neurosci. 17, 153–163. doi: 10.1038/nn.3602
Weaver, A. K., Olsen, M. L., Mcferrin, M. B., and Sontheimer, H. (2007). BK channels are linked to inositol 1,4,5-triphosphate receptors via lipid rafts: a novel mechanism for coupling [Ca2+](i) to ion channel activation. J. Biol. Chem. 282, 31558–31568. doi: 10.1074/jbc.M702866200
Woll, K. A., and Van Petegem, F. (2022). Calcium-release channels: structure and function of IP3 receptors and ryanodine receptors. Physiol. Rev. 102, 209–268. doi: 10.1152/physrev.00033.2020
Womack, M. D., Chevez, C., and Khodakhah, K. (2004). Calcium-activated potassium channels are selectively coupled to P/Q-type calcium channels in cerebellar Purkinje neurons. J. Neurosci. 24, 8818–8822. doi: 10.1523/JNEUROSCI.2915-04.2004
Womack, M. D., and Khodakhah, K. (2002). Characterization of large conductance Ca2+-activated K+ channels in cerebellar Purkinje neurons. Eur. J. Neurosci. 16, 1214–1222. doi: 10.1046/j.1460-9568.2002.02171.x
Wu, J., Yan, Z., Li, Z., Qian, X., Lu, S., Dong, M., et al. (2016). Structure of the voltage-gated calcium channel Ca(v)1.1 at 3.6 A resolution. Nature 537, 191–196. doi: 10.1038/nature19321
Wu, J., Yan, Z., Li, Z., Yan, C., Lu, S., Dong, M., et al. (2015). Structure of the voltage-gated calcium channel Cav1.1 complex. Science 350:aad2395. doi: 10.1126/science.aad2395
Wu, Y., Liu, Y., Hou, P., Yan, Z., Kong, W., Liu, B., et al. (2013). TRPV1 channels are functionally coupled with BK(mSlo1) channels in rat dorsal root ganglion (DRG) neurons. PLoS One 8:e78203. doi: 10.1371/journal.pone.0078203
Xu, J. W., and Slaughter, M. M. (2005). Large-conductance calcium-activated potassium channels facilitate transmitter release in salamander rod synapse. J. Neurosci. 25, 7660–7668. doi: 10.1523/JNEUROSCI.1572-05.2005
Yan, J., and Aldrich, R. W. (2010). LRRC26 auxiliary protein allows BK channel activation at resting voltage without calcium. Nature 466, 513–516. doi: 10.1038/nature09162
Yan, J., and Aldrich, R. W. (2012). BK potassium channel modulation by leucine-rich repeat-containing proteins. Proc. Natl. Acad. Sci. USA 109, 7917–7922. doi: 10.1073/pnas.1205435109
Yang, C., Gonzalez-Perez, V., Mukaibo, T., Melvin, J. E., Xia, X. M., and Lingle, C. J. (2017). Knockout of the LRRC26 subunit reveals a primary role of LRRC26-containing BK channels in secretory epithelial cells. Proc. Natl. Acad. Sci. USA 114, E3739–E3747. doi: 10.1073/pnas.1703081114
Yang, Y., Li, P. Y., Cheng, J., Cai, F., Lei, M., Tan, X. Q., et al. (2013). IP3 decreases coronary artery tone via activating the BKCa channel of coronary artery smooth muscle cells in pigs. Biochem. Biophys. Res. Commun. 439, 363–368. doi: 10.1016/j.bbrc.2013.08.079
Yazejian, B., Digregorio, D. A., Vergara, J. L., Poage, R. E., Meriney, S. D., and Grinnell, A. D. (1997). Direct measurements of presynaptic calcium and calcium-activated potassium currents regulating neurotransmitter release at cultured Xenopus nerve-muscle synapses. J. Neurosci. 17, 2990–3001. doi: 10.1523/JNEUROSCI.17-09-02990.1997
Ye, H., Jalini, S., Mylvaganam, S., and Carlen, P. (2010). Activation of large-conductance Ca2+-activated K+ channels depresses basal synaptic transmission in the hippocampal CA1 area in APP (swe/ind) TgCRND8 mice. Neurobiol. Aging 31, 591–604. doi: 10.1016/j.neurobiolaging.2008.05.012
Zamponi, G. W., Striessnig, J., Koschak, A., and Dolphin, A. C. (2015). The physiology, pathology, and pharmacology of voltage-gated calcium channels and their future therapeutic potential. Pharmacol. Rev. 67, 821–870. doi: 10.1124/pr.114.009654
Zavala-Tecuapetla, C., Aguileta, M. A., Lopez-Guerrero, J. J., Gonzalez-Marin, M. C., and Pena, F. (2008). Calcium-activated potassium currents differentially modulate respiratory rhythm generation. Eur. J. Neurosci. 27, 2871–2884. doi: 10.1111/j.1460-9568.2008.06214.x
Zhang, H., Xie, M., Schools, G. P., Feustel, P. F., Wang, W., Lei, T., et al. (2009). Tamoxifen mediated estrogen receptor activation protects against early impairment of hippocampal neuron excitability in an oxygen/glucose deprivation brain slice ischemia model. Brain Res. 1247, 196–211. doi: 10.1016/j.brainres.2008.10.015
Zhang, J., Li, Q., Meredith, A. L., Pan, H., and Yan, J. (2018). Glutamate-activated BK channel complexes formed with NMDA receptors. Proc. Natl. Acad. Sci. USA 115, E9006–E9014. doi: 10.1073/pnas.1802567115
Zhang, J., and Yan, J. (2014). Regulation of BK channels by auxiliary gamma subunits. Front. Physiol. 5:401. doi: 10.3389/fphys.2014.00401
Zhang, L., Li, X., Zhou, R., and Xing, G. (2006). Possible role of potassium channel, big K in etiology of schizophrenia. Med. Hypo. 67, 41–43. doi: 10.1016/j.mehy.2005.09.055
Zhang, X. L., Mok, L. P., Lee, K. Y., Charbonnet, M., and Gold, M. S. (2012). Inflammation-induced changes in BK(Ca) currents in cutaneous dorsal root ganglion neurons from the adult rat. Mol. Pain 8:37. doi: 10.1186/1744-8069-8-37
Zhao, G., Neeb, Z. P., Leo, M. D., Pachuau, J., Adebiyi, A., Ouyang, K., et al. (2010). Type 1 IP3 receptors activate BKCa channels via local molecular coupling in arterial smooth muscle cells. J. Gen. Physiol. 136, 283–291. doi: 10.1085/jgp.201010453
Zhao, M. G., Hulsmann, S., Winter, S. M., Dutschmann, M., and Richter, D. W. (2006). Calcium-regulated potassium currents secure respiratory rhythm generation after loss of glycinergic inhibition. Eur. J. Neurosci. 24, 145–154. doi: 10.1111/j.1460-9568.2006.04877.x
Zhou, Q., and Sheng, M. (2013). NMDA receptors in nervous system diseases. Neuropharmacology 74, 69–75. doi: 10.1016/j.neuropharm.2013.03.030
Zito, K., and Scheuss, V. (2009). “NMDA receptor function and physiological modulation,” in Encyclopedia of Neuroscience, ed. L. R. Squire (Oxford: Academic Press), 1157–1164. doi: 10.1016/B978-008045046-9.01225-0
Keywords: BK channels, calcium-activated channels, calcium channels, NMDA receptors, nanodomain, coupling, calcium signaling
Citation: Shah KR, Guan X and Yan J (2022) Structural and Functional Coupling of Calcium-Activated BK Channels and Calcium-Permeable Channels Within Nanodomain Signaling Complexes. Front. Physiol. 12:796540. doi: 10.3389/fphys.2021.796540
Received: 17 October 2021; Accepted: 28 December 2021;
Published: 14 January 2022.
Edited by:
Yoshiaki Suzuki, Nagoya City University, JapanReviewed by:
Klaus Schicker, Medical University of Vienna, AustriaKazuharu Furutani, Tokushima Bunri University, Japan
Copyright © 2022 Shah, Guan and Yan. This is an open-access article distributed under the terms of the Creative Commons Attribution License (CC BY). The use, distribution or reproduction in other forums is permitted, provided the original author(s) and the copyright owner(s) are credited and that the original publication in this journal is cited, in accordance with accepted academic practice. No use, distribution or reproduction is permitted which does not comply with these terms.
*Correspondence: Jiusheng Yan, jyan1@mdanderson.org