- 1Division of Sport and Exercise Sciences, Abertay University, Dundee, United Kingdom
- 2Department of Physiology, School of Medicine, Trinity College Dublin, The University of Dublin, Dublin, Ireland
- 3Endocrinology, St Columcille’s and St Vincent’s Hospitals, Dublin, Ireland
- 4School of Science and Health, Western Sydney University, Sydney, AU-NSW, Australia
Background: The primary phase time constant of pulmonary oxygen uptake kinetics (
Methods: Eleven (4 women) middle-aged individuals with type 2 diabetes and 11 (4 women) non-diabetic controls completed four separate cycling bouts each starting at an ‘unloaded’ baseline of 10 W and transitioning to a high-intensity constant-load. Two of the four cycling bouts were preceded by priming exercise. The dynamics of pulmonary
Results: At baseline
Conclusion: These results suggest that in middle-aged men and women with T2D, PE speeds
1 Introduction
Type 2 diabetes (T2D) is a major chronic condition with a concerning rapidly increasing global prevalence. Importantly, men and women with T2D demonstrate a consistent impairment in cardiorespiratory capacity reflected by a decreased peak oxygen uptake (
The impediments in
Importantly, abrupt or sudden transitions to high-intensity activity (i.e. running, cycling or stair climbing) from rest or very light activity are akin to those in daily life (such as commuting to work), so, there is a need to examine interventions that may enhance the
Thus, considering that O2 supply to the muscle seems to be constrained in individuals with T2D, and high-intensity priming exercise has been proposed as an intervention that can augment the delivery of O2 to the muscle, we tested the hypothesis that PE would reduce
2 Methods
2.1 Participants and recruitment
A total of 22 individuals, 11 with T2D (7 men/4 women) and 11 healthy controls (7 men/4 women) volunteered and provided written informed consent to take part in this study (Table 1). Recruitment for the control group was undertaken from the general population, whilst individuals with T2D were recruited from outpatient diabetes clinics of two hospitals in Dublin (i.e. St. Vincent’s University Hospital and St. Columcille’s Hospital). Four of the women participating in this study were premenopausal (2 T2D and 2 Control) and four were postmenopausal (2 T2D and 2 Control) not undergoing hormone replacement therapy. All participants were non-smokers (not smoking during the previous 12 months) and physically inactive [(≤150 min week−1 of moderate-intensity (<ventilatory threshold, VT) exercise in the preceding 6 months] (McKay et al., 2022). The latter status was confirmed by participants wearing RT3 triaxial accelerometers (Stayhealthy Inc., CA) over a 5-day period (Table 1) (Rowlands et al., 2004). Participants’ time since diagnosis of T2D was between 2 and 10 years (mean ± SD = 4.5 ± 3.2 yrs) and had HbA1c levels below 10%. In addition, exclusion criteria included the use of β-blockers or insulin, clinical evidence of liver or renal disease, if they suffered from persistent proteinuria (urine protein >200 mg/dl) or had high creatinine levels (suggestive of renal disease, which can alter exercise performance); autonomic insufficiency/dysfunction, symmetrical neuropathy, abnormal cardiac function, or evidence of ischaemic heart disease. Regarding medication, two individuals in the control group were on statins whilst participants with T2D were treated with either oral (n = 9) and/or subcutaneous (n = 2) hypoglycaemic agents (only metformin, n = 5; metformin and sulphonylurea, n = 1; SGLT-2 inhibitors, n = 1; GLP-1, n = 1), as well as antihypertensive medication (ACE inhibitor, n = 3; ARBs, n = 2; CCBs, n = 3) and statins (n = 6). The study received ethical approval from both Trinity College Dublin (TCD), and St Vincent’s Healthcare research ethics committees.
2.2 Experimental procedures
2.2.1 Overview
Upon successful completion of a treadmill stress test (Bruce protocol) at St Columcille’s Hospital, participants attended the testing laboratories on two separate occasions. The participants in the control group completed all tests in the University’s human performance laboratory whilst participants with T2D did so in the exercise testing facility at St Columcille's Hospital. Visit one consisted of participants completing a maximal cycling test to exhaustion to measure peak oxygen uptake (
2.2.2 Visit 1: Maximal cycling test to exhaustion
All participants completed a ramp incremental cycling test to volitional exhaustion with an initial work rate of 10 W for 2 min (i.e., ‘unloaded’ cycling) followed by a progressive increase in power output of 10–25 W/min (based on each individual’s physical activity level). Participants were required to maintain a constant cadence throughout the test, self-selecting a pedalling rate between 60 and 75 rpm. Test termination occurred when participants had a cadence reduction of 10 rpm for more than 5s. At the end of the test peak work rate was determined as the highest power output achieved, whilst
2.2.3 Visit 2: Four cycling exercise transitions
All participants completed four identical 9-min cycling exercise bouts transitioning from an ‘unloaded’ power output of 10 W (3 min) to a constant-load of 50% delta (50%Δ) (6 min). The latter intensity was determined from the results of the maximal cycling test by adding the 50% difference between the power outputs at
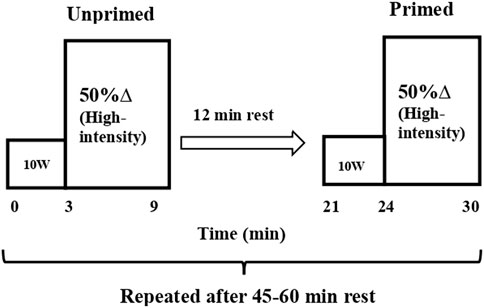
FIGURE 1. Schematic representation of the protocol. Unprimed and primed cycling step transitions performed at high-intensity cycling exercise (Δ50%; the sum of the power output at VT and 50% of the difference between the power output at VT and
2.3 Measures
2.3.1 Pulmonary gas exchange and heart rate
Breath-by-breath data was continuously obtained during exercise by participants wearing a facemask that was connected to a metabolic gas analysis system (Innocor, Innovision A/S, Odense, Denmark). Parameters analysed were oxygen consumption, carbon dioxide production, respiratory exchange ratio and minute ventilation. Calibration of equipment was undertaken before each use according to the recommendations of the manufacturer. In addition, calibration of the system’s oxygen and photoacoustic sensors is undertaken periodically (every 6–12 months) by the manufacturer. A heart rate monitor was used to measure HR at 5 s intervals (Polar S610i, Polar Ltd., Finland).
2.3.2 Muscle deoxygenation and tissue oxygenation index
Muscle oxygenation (O2Hb + Mb), deoxygenation (HHb + Mb) and tissue oxygenation index (TOI) data were acquired using a continuous wave NIRS system (Niro 200NX; Hamamatsu, Japan). This device uses the spatially resolved spectroscopy (SRS) technique and modified Beer-Lambert (MBL) principle. Detailed information about this technique and its application during exercise is available elsewhere (Ferrari et al., 2011). In the present study this measurement was undertaken in the vastus lateralis (VL) muscle of the participant’s right quadriceps given the VL is the primary locomotor muscle during leg cycling (Laplaud et al., 2006; Okushima et al., 2016). In order to ensure good quality signals, necessary skin preparation was undertaken involving shaving any hair present and cleaning the area with alcohol. After the skin was dried, the probes in their rubber holder were securely positioned on the muscle, between 10 and 16 cm above the femoral condyle using transparent adhesive tape. A dark elastic bandage was also used to further protect the probes from external light and movement. The depth of the area being measured is approximately one-half the distance between the emitter and the receiver probes (∼1.5 cm). Therefore, ultrasound measurements of the skin and adipose tissue at the probe location were taken in all participants using the B-mode of a 2D ultrasound (Zonare Ultra Smart Cart, Software version 4.7, United States) to ensure that data collected was representative of the muscle tissue. This was confirmed with all participants having less than 1.5 cm of adipose tissue thickness at the probe location.
2.4 Data analysis
2.4.1 Oxygen uptake kinetics
The linear interpolation method was used to estimate second by second values from the breath-by-breath
In the above function
2.4.2 [HHb + Mb] kinetics and tissue oxygenation index
The muscle deoxygenation (i.e. Δ[HHb + Mb]) response during high-intensity cycling was collected at a frequency of 1 Hz, was ensemble-averaged and time averaged into 5 s bins for each participant and was fitted using the same biexponential function (Eq. 1). TD was determined by visually inspecting a consistent rise from the pre-transition level since the Δ[HHb + Mb] is known to present a TD at the start of exercise before increasing in an exponential manner. This phenomenon has been suggested to reflect a close association between local oxygen delivery and muscular oxygen consumption (DeLorey et al., 2003; Grassi et al., 2003). Muscle deoxygenation data was therefore fitted from the TD onwards. The sum of TD and t was used to determine the effective response time (τ′Δ[HHb + Mb]) representing the time course for the primary phase of the Δ [HHb + Mb] response. Baseline TOI was calculated as the 30 s preceding each transition, and the End TOI as the final 30 s of exercise. ΔTOI was calculated by subtracting baseline TOI from End TOI.
2.4.3 Heart rate kinetics
The heart rate data was fitted using a monoexponential function (Eq. 2) with the fitting window constrained to
In the above function HR baseline represents the mean HR in the last 30 s of the “unloaded” cycling.
2.5 Statistical analysis
The Shapiro-Wilk’s test was used to assess the normal distribution of the data. Between group comparisons of participants’ characteristics and peak performance data were undertaken using an unpaired Student’s t-test (for parametric data) or a Mann-Whitney U test (for non-parametric data). A two-way mixed model ANOVA [condition (unprimed, primed) x diabetes status (T2D, Control)] and the post hoc Tukey test were used to analyse all the dynamic response data for oxygen uptake, heart rate and muscle deoxygenation as well as TOI responses. p < 0.05 was used to determine statistical significance. Results from parametric analyses are presented as mean ± SD whereas non-parametric results are presented using median and interquartile ranges.
3 Results
3.1 Participant characteristics
Unsurprisingly, individuals with T2D had significantly higher HbA1c and fasting plasma glucose levels than healthy individuals (Table 1). Importantly, the T2D and control groups were matched according to sex distribution, age, BMI, body mass and activity levels.
3.2 Peak exercise responses
Individuals with T2D had significantly lower absolute
3.3 Effect of priming exercise on oxygen uptake kinetics
The primed and unprimed dynamic response characteristics of
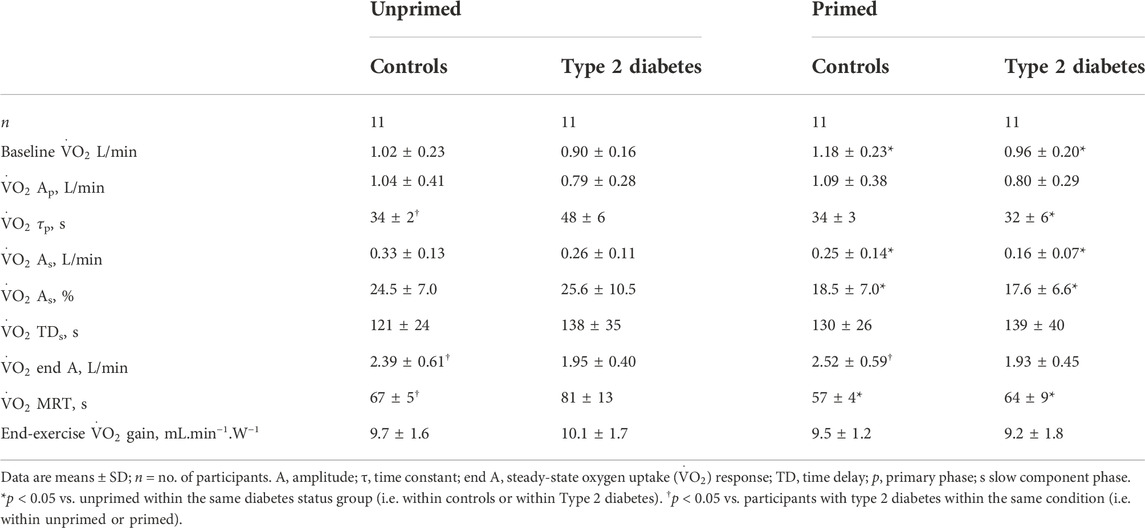
TABLE 2. Dynamic response characteristics of oxygen uptake (
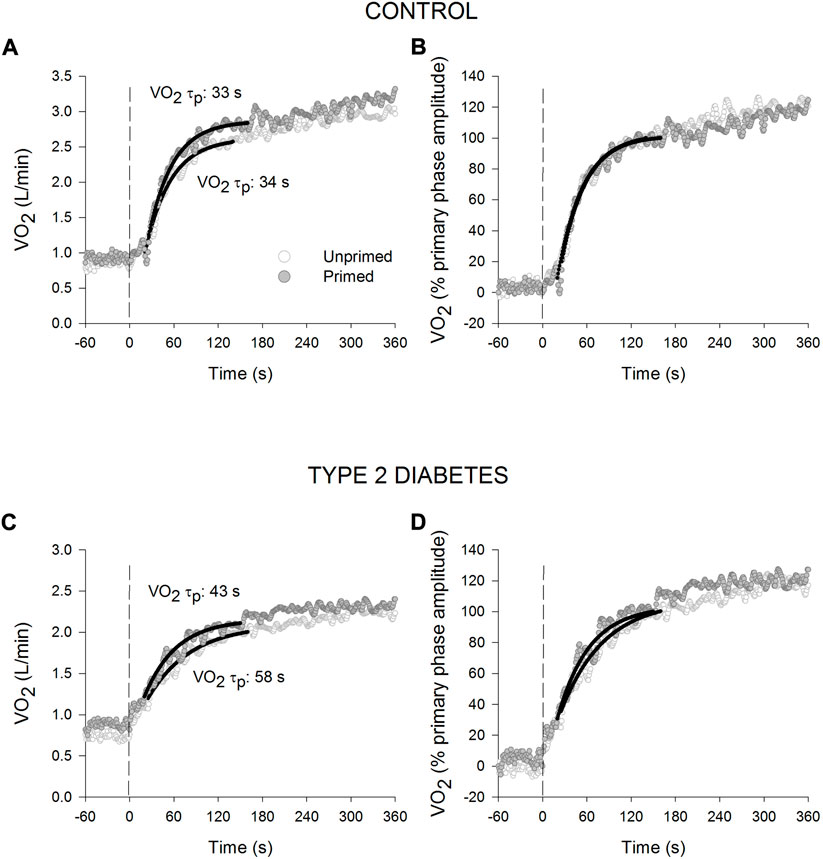
FIGURE 2. Oxygen uptake (
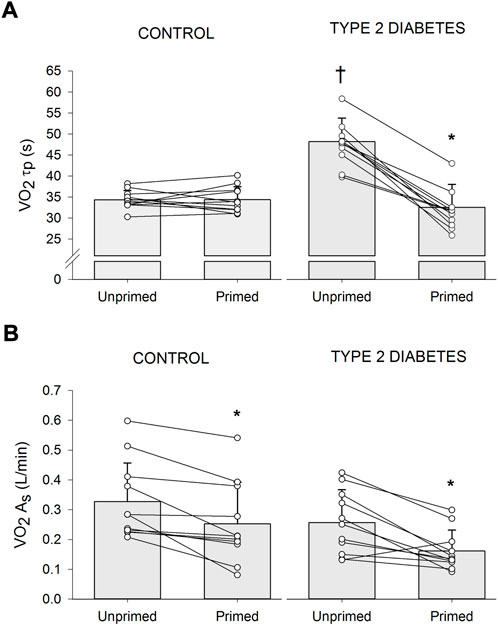
FIGURE 3. Individual and mean ± SD (bar graph) changes in time constant of the primary phase of oxygen uptake (
3.4 Effect of priming exercise on Δ [HHb + Mb] kinetics and tissue oxygenation index responses
Table 3 presents the parameter estimates for Δ[HHb + Mb] kinetics and TOI responses whereas Figure 4 shows the Δ[HHb + Mb] responses for representative individuals. No statistical group difference was observed in the unprimed parameter estimates. PE resulted in significantly elevated levels of baseline Δ [HHb + Mb] in T2D (p < 0.001) and a tendency for higher levels in controls (p = 0.08) (group × condition interaction, p = 0.04). Participants with T2D showed a larger ratio of the modelled amplitudes of Δ [HHb + Mb]/Δ
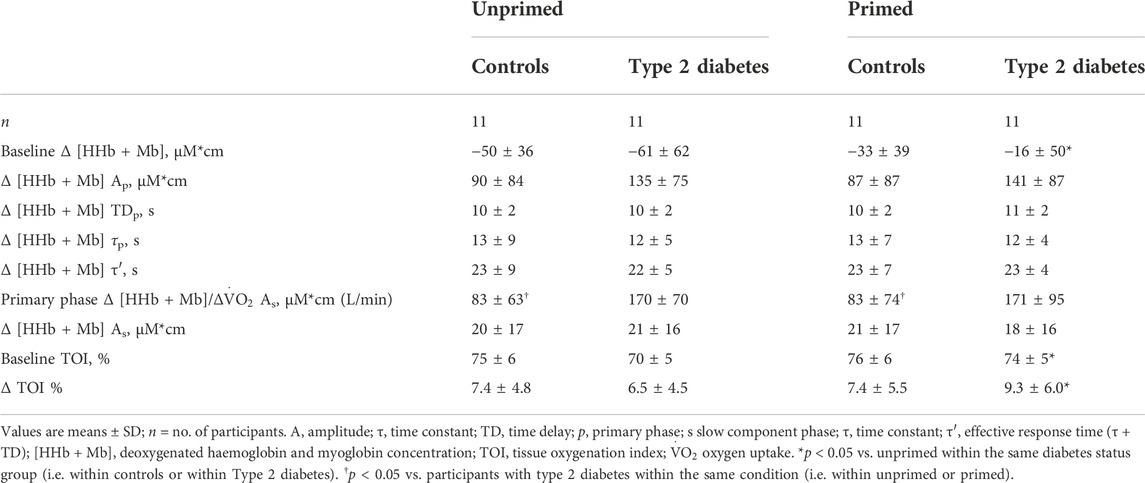
TABLE 3. Dynamic response characteristics of Δ [HHb + Mb] and TOI during high-intensity cycling exercise transitions.
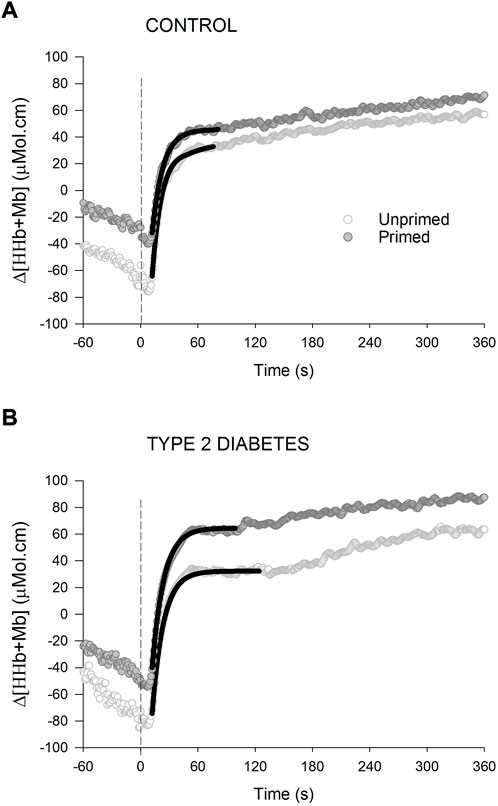
FIGURE 4. Changes in deoxygenated hemoglobin and myoglobin concentration [Δ (HHb + Mb)] for a representative healthy control (A) and an individual with type 2 diabetes (B) during high-intensity cycling transitions without priming exercise (open circles) and with priming exercise (solid circles). The vertical line illustrates the abrupt transition to the higher work rate. The continuous black lines of best fit illustrate the primary phase of the Δ (HHb + Mb) response. Note the time constant of the primary phase of the Δ [HHb + Mb] response is not affected by prior priming exercise in any of the 2 groups.
3.5 Effect of priming exercise on heart rate kinetics
The HR τ was significantly longer in individuals with T2D compared with healthy controls (main effect of group, p < 0.01), but PE did not affect HR τ in any of the groups (T2D unprimed: 56 ± 3 s, T2D primed: 55 ± 2 s; controls unprimed: 51 ± 3 s, controls primed: 50 ± 5 s). Baseline HR was higher in T2D (main effect of group, p = 0.04) and subsequent to PE it increased (main effect of condition, p < 0.001) in both groups (T2D unprimed: 109 ± 14 beats. min−1, T2D primed: 118 ± 13 beats. min−1; controls unprimed: 98 ± 9 beats. min−1, controls primed: 106 ± 10 beats. min−1). End-exercise HR was not different among groups, but it increased following PE (main effect of condition, p = 0.017) in both groups (T2D unprimed: 157 ± 12 beats. min−1, T2D primed: 159 ± 13 beats. min−1; controls unprimed: 156 ± 16 beats. min−1, controls primed: 162 ± 15 beats. min−1).
4 Discussion
In agreement with our primary hypothesis, this study presents for the first-time evidence that in middle-aged individuals with T2D PE reduces
4.1 Effect of priming exercise on oxygen uptake τp
In the present study,
The performance of a PE bout herein resulted in a subsequent significant reduction in this
The notion that priming exercise enhanced O2 supply in the subsequent exercise transition in T2D is evidenced by an increased TOI which suggests an increase in O2 availability, likely mediated by a PE-induced increased vasodilation and muscle blood flow at the beginning of the subsequent exercise (Gerbino et al., 1996). However, given that the NIRS-derived overall muscle deoxygenation kinetics and/or amplitude were not affected by PE herein, there is the possibility that the priming-induced reduction in
4.2 Effect of priming exercise on oxygen uptake slow component
In the present study, PE significantly reduced both
The priming-induced reduction in the slow component of the present study can likely be attributed to alterations in motor unit recruitment patterns. For instance, our group has recently shown (Gildea et al., 2021b) a priming-induced reduction in iEMG between the end of exercise and the time of the onset of
The observed PE-induced reduction in the
With this new physiological insight of impaired
5 Limitations
Our results are limited to middle-aged mixed groups of men and women, hence, further studies should explore sex- and/or age-related differences in these outcomes. Even if our protocol did not allow block randomization, the sequence of the unprimed and primed exercise transitions was the same for all participants; hence, this likely has a minor impact on the interpretation of the current findings.
6 Conclusion
The present study showed that a preceding high-intensity exercise (i.e. warm-up) or priming exercise accelerated the overall MRT of the
Data availability statement
The raw data supporting the conclusions of this article will be made available by the authors, without undue reservation.
Ethics statement
The studies involving human participants were reviewed and approved by Faculty of Health Science Research Ethics Committee, Trinity College Dublin and St Vincent’s Healthcare Ethics and Medical Research Committee. The patients/participants provided their written informed consent to participate in this study.
Author contributions
JR, NG, DO’S, SG and ME conceived and designed the research and analyzed data. JR and NG conducted experiments and collected all participant data. NG and ME drafted the paper. All authors interpreted data and contributed to the writing of the final paper. All authors contributed to the article and approved the submitted version.
Funding
This publication has emanated from research conducted with the financial support of the Health Research Board (Grant No HRA_POR/2073/274).
Conflict of interest
The authors declare that the research was conducted in the absence of any commercial or financial relationships that could be construed as a potential conflict of interest.
Publisher’s note
All claims expressed in this article are solely those of the authors and do not necessarily represent those of their affiliated organizations, or those of the publisher, the editors and the reviewers. Any product that may be evaluated in this article, or claim that may be made by its manufacturer, is not guaranteed or endorsed by the publisher.
References
Barstow T. J., Jones A. M., Nguyen P. H., Casaburi R. (1996). Influence of muscle fiber type and pedal frequency on oxygen uptake kinetics of heavy exercise. J. Appl. Physiol. 81, 1642–1650. doi:10.1152/jappl.1996.81.4.1642
Bauer T. A., Reusch J. E., Levi M., Regensteiner J. G. (2007). Skeletal muscle deoxygenation after the onset of moderate exercise suggests slowed microvascular blood flow kinetics in type 2 diabetes. Diabetes Care 30, 2880–2885. doi:10.2337/dc07-0843
Beaver W. L., Wasserman K., Whipp B. J. (1986). A new method for detecting anaerobic threshold by gas exchange. J. Appl. Physiol. 60, 2020–2027. doi:10.1152/jappl.1986.60.6.2020
Brandenburg S. L., Reusch J. E., Bauer T. A., Jeffers B. W., Hiatt W. R., Regensteiner J. G. (1999). Effects of exercise training on oxygen uptake kinetic responses in women with type 2 diabetes. Diabetes Care 22, 1640–1646. doi:10.2337/diacare.22.10.1640
Burnley M., Doust J. H., Ball D., Jones A. M. (2002a1985). Effects of prior heavy exercise on VO(2) kinetics during heavy exercise are related to changes in muscle activity. J. Appl. Physiol. 93, 167–174. doi:10.1152/japplphysiol.01217.2001
Burnley M., Doust J. H., Carter H., Jones A. M. (2001). Effects of prior exercise and recovery duration on oxygen uptake kinetics during heavy exercise in humans. Exp. Physiol. 86, 417–425. doi:10.1113/eph8602122
Burnley M., Doust J. H., Jones A. M. (2002b). Effects of prior heavy exercise, prior sprint exercise and passive warming on oxygen uptake kinetics during heavy exercise in humans. Eur. J. Appl. Physiol. 87, 424–432. doi:10.1007/s00421-002-0647-8
Burnley M., Doust J. H., Jones A. M. (2006). Time required for the restoration of normal heavy exercise VO2 kinetics following prior heavy exercise. J. Appl. Physiol. 101, 1320–1327. doi:10.1152/japplphysiol.00475.2006
Burnley M., Jones A. M., Carter H., Doust J. H. (2000). Effects of prior heavy exercise on phase II pulmonary oxygen uptake kinetics during heavy exercise. J. Appl. Physiol. 89, 1387–1396. doi:10.1152/jappl.2000.89.4.1387
Cannon D. T., Kolkhorst F. W., Cipriani D. J. (2007). Electromyographic data do not support a progressive recruitment of muscle fibers during exercise exhibiting a VO2 slow component. J. Physiol. Anthropol. 26, 541–546. doi:10.2114/jpa2.26.541
Delorey D. S., Kowalchuk J. M., Paterson D. H. (2003). Relationship between pulmonary O2 uptake kinetics and muscle deoxygenation during moderate-intensity exercise. J. Appl. Physiol. 95, 113–120. doi:10.1152/japplphysiol.00956.2002
Dimenna F. J., Wilkerson D. P., Burnley M., Bailey S. J., Jones A. M. (2010). Priming exercise speeds pulmonary O2 uptake kinetics during supine "work-to-work" high-intensity cycle exercise. J. Appl. Physiol. 108, 283–292. doi:10.1152/japplphysiol.01047.2009
Dimenna F. J., Wilkerson D. P., Burnley M., Jones A. M. (2008). Influence of priming exercise on pulmonary O2 uptake kinetics during transitions to high-intensity exercise from an elevated baseline. J. Appl. Physiol. 105, 538–546. doi:10.1152/japplphysiol.90357.2008
Drouin P. J., Forbes S. P. A., Liu T., Lew L. A., Mcgarity-Shipley E., Tschakovsky M. E. (2022). Muscle contraction force conforms to muscle oxygenation during constant-activation voluntary forearm exercise. Exp. Physiol. 107 (11), 1360–1374. doi:10.1113/ep090576
Egaña M., Columb D., O'donnell S. (2013). Effect of low recumbent angle on cycling performance, fatigue, and V O(2) kinetics. Med. Sci. Sports Exerc. 45, 663–673. doi:10.1249/MSS.0b013e318279a9f2
Egaña M., Ryan K., Warmington S. A., Green S. (2010). Effect of body tilt angle on fatigue and EMG activities in lower limbs during cycling. Eur. J. Appl. Physiol. 108, 649–656. doi:10.1007/s00421-009-1254-8
Ferrari M., Muthalib M., Quaresima V. (2011). The use of near-infrared spectroscopy in understanding skeletal muscle physiology: Recent developments. Philos. Trans. A Math. Phys. Eng. Sci. 369, 4577–4590. doi:10.1098/rsta.2011.0230
Fitzpatrick R., Taylor J. L., Mccloskey D. I. (1996). Effects of arterial perfusion pressure on force production in working human hand muscles. J. Physiol. 495 (3), 885–891. doi:10.1113/jphysiol.1996.sp021640
Fukuba Y., Hayashi N., Koga S., Yoshida T. (2002). VO(2) kinetics in heavy exercise is not altered by prior exercise with a different muscle group. J. Appl. Physiol. 92, 2467–2474. doi:10.1152/japplphysiol.00207.2001
Garland S. W., Wang W., Ward S. A. (2006). Indices of electromyographic activity and the "slow" component of oxygen uptake kinetics during high-intensity knee-extension exercise in humans. Eur. J. Appl. Physiol. 97, 413–423. doi:10.1007/s00421-006-0185-x
Gerbino A., Ward S. A., Whipp B. J. (1996). Effects of prior exercise on pulmonary gas-exchange kinetics during high-intensity exercise in humans. J. Appl. Physiol. 80, 99–107. doi:10.1152/jappl.1996.80.1.99
Gildea N., Mcdermott A., Rocha J., Crognale D., Nevin A., O'shea D., et al. (2022). Low-volume HIIT and MICT speed VO(2) kinetics during high-intensity "work-to-work" cycling with a similar time-course in type 2 diabetes. J. Appl. Physiol. 133, 273–287. doi:10.1152/japplphysiol.00148.2022
Gildea N., Mcdermott A., Rocha J., O'shea D., Green S., Egaña M. (2021a). Time-course of Vo(2) kinetics responses during moderate-intensity exercise subsequent to HIIT versus moderate-intensity continuous training in type 2 diabetes. J. Appl. Physiol. 130, 1646–1659. doi:10.1152/japplphysiol.00952.2020
Gildea N., Rocha J., Mcdermott A., O'shea D., Green S., Egaña M. (2019). Influence of type 2 diabetes on muscle deoxygenation during ramp incremental cycle exercise. Respir. Physiol. Neurobiol. 269, 103258. doi:10.1016/j.resp.2019.103258
Gildea N., Rocha J., O'shea D., Green S., Egaña M. (2021b). Priming exercise accelerates pulmonary oxygen uptake kinetics during "work-to-work" cycle exercise in middle-aged individuals with type 2 diabetes. Eur. J. Appl. Physiol. 121, 409–423. doi:10.1007/s00421-020-04518-y
Goulding R. P., Roche D. M., Marwood S. (2017). Prior exercise speeds pulmonary oxygen uptake kinetics and increases critical power during supine but not upright cycling. Exp. Physiol. 102, 1158–1176. doi:10.1113/ep086304
Goulding R. P., Rossiter H. B., Marwood S., Ferguson C. (2021). Bioenergetic mechanisms linking V˙O2 kinetics and exercise tolerance. Exerc. Sport Sci. Rev. 49, 274–283. doi:10.1249/jes.0000000000000267
Grassi B., Pogliaghi S., Rampichini S., Quaresima V., Ferrari M., Marconi C., et al. (2003). Muscle oxygenation and pulmonary gas exchange kinetics during cycling exercise on-transitions in humans. J. Appl. Physiol. 95, 149–158. doi:10.1152/japplphysiol.00695.2002
Green S., Egaña M., Baldi J. C., Lamberts R., Regensteiner J. G. (2015). Cardiovascular control during exercise in type 2 diabetes mellitus. J. Diabetes Res. 2015, 654204. doi:10.1155/2015/654204
Green S., Kiely C., O'connor E., Gildea N., O'shea D., Egaña M. (2020). Effects of exercise training and sex on dynamic responses of O(2) uptake in type 2 diabetes. Appl. Physiol. Nutr. Metab. 45, 865–874. doi:10.1139/apnm-2019-0636
Gurd B. J., Peters S. J., Heigenhauser G. J., Leblanc P. J., Doherty T. J., Paterson D. H., et al. (2009). Prior heavy exercise elevates pyruvate dehydrogenase activity and muscle oxygenation and speeds O2 uptake kinetics during moderate exercise in older adults. Am. J. Physiol. Regul. Integr. Comp. Physiol. 297, R877–R884. doi:10.1152/ajpregu.90848.2008
Gurd B. J., Peters S. J., Heigenhauser G. J., Leblanc P. J., Doherty T. J., Paterson D. H., et al. (2006). Prior heavy exercise elevates pyruvate dehydrogenase activity and speeds O2 uptake kinetics during subsequent moderate-intensity exercise in healthy young adults. J. Physiol. 577, 985–996. doi:10.1113/jphysiol.2006.112706
Jones A. M., Berger N. J., Wilkerson D. P., Roberts C. L. (2006). Effects of "priming" exercise on pulmonary O2 uptake and muscle deoxygenation kinetics during heavy-intensity cycle exercise in the supine and upright positions. J. Appl. Physiol. 101, 1432–1441. doi:10.1152/japplphysiol.00436.2006
Jones A. M., Poole D. C. (2005). Oxygen uptake dynamics: From muscle to mouth--an introduction to the symposium. Med. Sci. Sports Exerc. 37, 1542–1550. doi:10.1249/01.mss.0000177466.01232.7e
Kiely C., O'connor E., O'shea D., Green S., Egaña M. (2014). Hemodynamic responses during graded and constant-load plantar flexion exercise in middle-aged men and women with type 2 diabetes. J. Appl. Physiol. 117, 755–764. doi:10.1152/japplphysiol.00555.2014
Kiely C., Rocha J., O'connor E., O'shea D., Green S., Egaña M. (2015). Influence of menopause and Type 2 diabetes on pulmonary oxygen uptake kinetics and peak exercise performance during cycling. Am. J. Physiol. Regul. Integr. Comp. Physiol. 309, R875–R883. doi:10.1152/ajpregu.00258.2015
Koppo K., Bouckaert J. (2002). The decrease in VO(2) slow component induced by prior exercise does not affect the time to exhaustion. Int. J. Sports Med. 23, 262–267. doi:10.1055/s-2002-29080
Krustrup P., Soderlund K., Mohr M., Bangsbo J. (2004). The slow component of oxygen uptake during intense, sub-maximal exercise in man is associated with additional fibre recruitment. Pflugers Arch. 447, 855–866. doi:10.1007/s00424-003-1203-z
Laplaud D., Hug F., Grelot L. (2006). Reproducibility of eight lower limb muscles activity level in the course of an incremental pedaling exercise. J. Electromyogr. Kinesiol. 16, 158–166. doi:10.1016/j.jelekin.2005.04.002
Mac Ananey O., Malone J., Warmington S., O'shea D., Green S., Egaña M. (2011). Cardiac output is not related to the slowed o2 uptake kinetics in type 2 diabetes. Med. Sci. Sports Exerc. 43, 935–942. doi:10.1249/MSS.0b013e3182061cdb
Macananey O., Reilly H., O'shea D., Egaña M., Green S. (2011). Effect of type 2 diabetes on the dynamic response characteristics of leg vascular conductance during exercise. Diab. Vasc. Dis. Res. 8, 12–21. doi:10.1177/1479164110389625
Marin P., Andersson B., Krotkiewski M., Bjorntorp P. (1994). Muscle fiber composition and capillary density in women and men with NIDDM. Diabetes Care 17, 382–386. doi:10.2337/diacare.17.5.382
Mckay A. K. A., Stellingwerff T., Smith E. S., Martin D. T., Mujika I., Goosey-Tolfrey V. L., et al. (2022). Defining training and performance caliber: A participant classification framework. Int. J. Sports Physiol. Perform. 1, 317–331. doi:10.1123/ijspp.2021-0451
Miyamoto T., Watanabe K., Fukuda K., Moritani T. (2020). Near-infrared spectroscopy of vastus lateralis muscle during incremental cycling exercise in patients with type 2 diabetes. Phys. Ther. Res. 23, 23–30. doi:10.1298/ptr.E9984
Mogensen M., Sahlin K., Fernstrom M., Glintborg D., Vind B. F., Beck-Nielsen H., et al. (2007). Mitochondrial respiration is decreased in skeletal muscle of patients with type 2 diabetes. Diabetes 56, 1592–1599. doi:10.2337/db06-0981
Murias J. M., Spencer M. D., Kowalchuk J. M., Paterson D. H. (2011). Influence of phase I duration on phase II VO2 kinetics parameter estimates in older and young adults. Am. J. Physiol. Regul. Integr. Comp. Physiol. 301, R218–R224. doi:10.1152/ajpregu.00060.2011
O'connor E., Green S., Kiely C., O'shea D., Egaña M. (2015). Differential effects of age and type 2 diabetes on dynamic vs. peak response of pulmonary oxygen uptake during exercise. J. Appl. Physiol. 118, 1031–1039. doi:10.1152/japplphysiol.01040.2014
O'connor E., Kiely C., O'shea D., Green S., Egaña M. (2012). Similar level of impairment in exercise performance and oxygen uptake kinetics in middle-aged men and women with type 2 diabetes. Am. J. Physiol. Regul. Integr. Comp. Physiol. 303, R70–R76. doi:10.1152/ajpregu.00012.2012
Okushima D., Poole D. C., Barstow T. J., Rossiter H. B., Kondo N., Bowen T. S., et al. (2016). Greater V O2peak is correlated with greater skeletal muscle deoxygenation amplitude and hemoglobin concentration within individual muscles during ramp-incremental cycle exercise. Physiol. Rep. 4, e13065. doi:10.14814/phy2.13065
Padilla D. J., Mcdonough P., Behnke B. J., Kano Y., Hageman K. S., Musch T. I., et al. (2006). Effects of Type II diabetes on capillary hemodynamics in skeletal muscle. Am. J. Physiol. Heart Circ. Physiol. 291, H2439–H2444. doi:10.1152/ajpheart.00290.2006
Poole D. C., Schaffartzik W., Knight D. R., Derion T., Kennedy B., Guy H. J., et al. (1991). Contribution of exercising legs to the slow component of oxygen uptake kinetics in humans. J. Appl. Physiol. 71, 1245–1260. doi:10.1152/jappl.1991.71.4.1245
Poole D. C., Ward S. A., Gardner G. W., Whipp B. J. (1988). Metabolic and respiratory profile of the upper limit for prolonged exercise in man. Ergonomics 31, 1265–1279. doi:10.1080/00140138808966766
Rocha J., Gildea N., O'shea D., Green S., Egaña M. (2019). Influence of priming exercise on oxygen uptake and muscle deoxygenation kinetics during moderate-intensity cycling in type 2 diabetes. J. Appl. Physiol. 127, 1140–1149. doi:10.1152/japplphysiol.00344.2019
Rocha J., Gildea N., O'shea D., Green S., Egaña M. (2020). Reply to Senefeld et al. J. Appl. Physiol. 128, 227. doi:10.1152/japplphysiol.00804.2019
Rossiter H. B., Ward S. A., Kowalchuk J. M., Howe F. A., Griffiths J. R., Whipp B. J. (2002). Dynamic asymmetry of phosphocreatine concentration and O(2) uptake between the on- and off-transients of moderate- and high-intensity exercise in humans. J. Physiol. 541, 991–1002. doi:10.1113/jphysiol.2001.012910
Rossiter H. B., Ward S. A., Kowalchuk J. M., Howe F. A., Griffiths J. R., Whipp B. J. (2001). Effects of prior exercise on oxygen uptake and phosphocreatine kinetics during high-intensity knee-extension exercise in humans. J. Physiol. 537, 291–303. doi:10.1111/j.1469-7793.2001.0291k.x
Rowlands A. V., Thomas P. W., Eston R. G., Topping R. (2004). Validation of the RT3 triaxial accelerometer for the assessment of physical activity. Med. Sci. Sports Exerc. 36, 518–524. doi:10.1249/01.mss.0000117158.14542.e7
Sahlin K., Sorensen J. B., Gladden L. B., Rossiter H. B., Pedersen P. K. (2005). Prior heavy exercise eliminates VO2 slow component and reduces efficiency during submaximal exercise in humans. J. Physiol. 564, 765–773. doi:10.1113/jphysiol.2005.083840
Scheuermann B. W., Hoelting B. D., Noble M. L., Barstow T. J. (2001). The slow component of O(2) uptake is not accompanied by changes in muscle EMG during repeated bouts of heavy exercise in humans. J. Physiol. 531, 245–256. doi:10.1111/j.1469-7793.2001.0245j.x
Watanabe K., Gazzoni M., Holobar A., Miyamoto T., Fukuda K., Merletti R., et al. (2013). Motor unit firing pattern of vastus lateralis muscle in type 2 diabetes mellitus patients. Muscle Nerve 48, 806–813. doi:10.1002/mus.23828
Watanabe K., Miyamoto T., Tanaka Y., Fukuda K., Moritani T. (2012). Type 2 diabetes mellitus patients manifest characteristic spatial EMG potential distribution pattern during sustained isometric contraction. Diabetes Res. Clin. Pract. 97, 468–473. doi:10.1016/j.diabres.2012.03.004
Wei M., Gibbons L. W., Kampert J. B., Nichaman M. Z., Blair S. N. (2000). Low cardiorespiratory fitness and physical inactivity as predictors of mortality in men with type 2 diabetes. Ann. Intern. Med. 132, 605–611. doi:10.7326/0003-4819-132-8-200004180-00002
Whipp B. J., Ward S. A., Lamarra N., Davis J. A., Wasserman K. (1982). Parameters of ventilatory and gas exchange dynamics during exercise. J. Appl. Physiol. Respir. Environ. Exerc. Physiol. 52, 1506–1513. doi:10.1152/jappl.1982.52.6.1506
Wilkerson D. P., Poole D. C., Jones A. M., Fulford J., Mawson D. M., Ball C. I., et al. (2011). Older type 2 diabetic males do not exhibit abnormal pulmonary oxygen uptake and muscle oxygen utilization dynamics during submaximal cycling exercise. Am. J. Physiol. Regul. Integr. Comp. Physiol. 300, R685–R692. doi:10.1152/ajpregu.00479.2010
Keywords: near-infrared spectroscopy, oxygen extraction, cycling, exercise tolerance, oxygen uptake slow component
Citation: Rocha J, Gildea N, O’Shea D, Green S and Egaña M (2022) Priming exercise accelerates oxygen uptake kinetics during high-intensity cycle exercise in middle-aged individuals with type 2 diabetes. Front. Physiol. 13:1006993. doi: 10.3389/fphys.2022.1006993
Received: 29 July 2022; Accepted: 31 October 2022;
Published: 18 November 2022.
Edited by:
Michael E Tschakovsky, Queen’s University, CanadaReviewed by:
Christopher Hearon, University of Texas Southwestern Medical Center, United StatesToshiaki Miyamoto, Hyogo University of Health Sciences, Japan
Copyright © 2022 Rocha, Gildea, O’Shea, Green and Egaña. This is an open-access article distributed under the terms of the Creative Commons Attribution License (CC BY). The use, distribution or reproduction in other forums is permitted, provided the original author(s) and the copyright owner(s) are credited and that the original publication in this journal is cited, in accordance with accepted academic practice. No use, distribution or reproduction is permitted which does not comply with these terms.
*Correspondence: Mikel Egaña, bWVnYW5hQHRjZC5pZQ==
†These authors have contributed equally to this work and share first authorship