- 1Nemours Children’s Health, Wilmington, DE, United States
- 2Sidney Kimmel School of Medicine, Thomas Jefferson University, Philadelphia, PA, United States
- 3Lewis Katz School of Medicine, Temple University, Philadelphia, PA, United States
The present review is a historical perspective of methodology and applications using inert liquids for respiratory support and as a vehicle to deliver biological agents to the respiratory system. As such, the background of using oxygenated inert liquids (considered a drug when used in the lungs) opposed to an oxygen-nitrogen gas mixture for respiratory support is presented. The properties of these inert liquids and the mechanisms of gas exchange and lung function alterations using this technology are described. In addition, published preclinical and clinical trial results are discussed with respect to treatment modalities for respiratory diseases. Finally, this forward-looking review provides a comprehensive overview of potential methods for administration of drugs/gene products to the respiratory system and potential biomedical applications.
Introduction
Aerosol drugs have been delivered to the lungs for several thousand years (Stein and Thiel, 2017). The use of aerosol delivery is complex, and deposition of drugs in the respiratory system is influenced by several specific factors: physics of the aerosol (inertia of the aerosol), gravitational factors, diffusion (airflow patterns in the lungs), and pulmonary defense mechanisms. Pulmonary drug delivery has been only partly explored in recent decades even though it could represent an alternative route of administration of drug-based therapies. Pulmonary drug delivery is an attractive route of administration of drugs, since the lungs are an ideal entry point for drugs to the bloodstream because of the large surface area, the very short diffusion distances in the alveolar spaces, and exposure to the entire cardiac output. Today there is an increased need for topical delivery of lung cancer therapy drugs, anti-inflammatory drugs to treat acute respiratory distress (i.e., COVID-19, H1N1 influenza), and gene-targeted lung agents for several relatively uncommon (orphan) diseases and pulmonary arterial hypertension (PAH) (Ali et al., 2015; Muralidharan et al., 2015; Alapati et al., 2019; Keshavarz et al., 2020; Kumar et al., 2020).
During the last 20 years, the combination of nanocrystal technology combined with an inert perfluorochemical vehicle has demonstrated the efficacy of large volume drug delivery to the entire lung because of the vehicle physical-chemical properties (inert properties, low surface tension, and high respiratory gas solubility) (Cullen et al., 1999). Furthermore, based on this combination delivery approach, it has been possible to demonstrate increased lung targeted drug delivery as opposed to systemic delivery. Nanocarriers have been found to be most promising because of their significant advantages (i.e., cell-specific targeted drug delivery and prolonged drug release). Thus, in combination with inert perfluorochemical vehicles, nanocarriers may provide effective delivery to the entire lung. The advantages offered by pulmonary drug delivery indicate that the challenges of such a delivery approach are worth addressing; if successfully addressed, there are great opportunities to treat unmet clinical needs. The present review focuses on providing a comprehensive historical perspective of the use of inert liquids for respiratory applications.
Respiratory Support With Inert Liquids
The use of liquids for respiratory support is reviewed in this section, as well as the physical properties of fluid used and the rationale for using specific liquids.
The first liquid used as a respiratory medium for lung lavage was saline (Winternitz and Smith, 1920). It debrides the lung and eliminates the gas-liquid interface within it. Early saline studies clarified factors influencing distensibility, alveolar structure, stability, pulmonary blood flow, and ventilation (Neergard, 1929; Mead et al., 1957; Avery and Mead, 1959; Leith and Mead, 1966; Hamosh and Luchsinger, 1968; Davidson et al., 1995; Fournier et al., 1995). Low respiratory gas solubility (O2; CO2) and diffusion gradients at atmospheric conditions limited the functional use of saline solution to provide adequate gas exchange (Kylstra et al., 1966; Kylstra, 1967; Kylstra et al., 1973; Wessler et al., 1977; Lynch et al., 1983). The hypothesis that O2-saturated saline solution dissolved under pressure could possibly sustain submersed mammals was formulated (Stein and Sonnenschein, 1950), and subsequent research revealed that adequately oxygenated liquid could be breathed by and support mammals submerged in hyperbaric oxygenated saline solution (Goodlin, 1962; Kylstra et al., 1962; Pegg et al., 1963; Kylstra et al., 1966). However, CO2 retention and profound acidosis occurred because of the small gradient between arterial and alveolar CO2 gradients, thus eliminating saline ventilation for either normobaric or hyperbaric conditions. In addition, it should be noted that although saline has been used to lavage debris and inflammatory mediators from the lungs as noted above, it has also been shown to inactivate pulmonary surfactant and impair lung function (Shaffer and Wolfson, 2011).
Inert Perfluorochemical Liquid Physicochemical Properties
As an alternative to saline as a respiratory medium, the utility of other liquids (silicone, vegetable oils, and animal oils) was investigated as respiratory media; however, these oils, although having high gas solubility, also demonstrated toxic effects (Clark, 1970; Sargent and Seffl, 1970). Perfluorochemicals (PFCs) were initially produced as part of the Manhattan Project during World War II. In 1966, they were used to support normobaric respiration on the basis of their high solubility for respiratory gases (Table 1) (Clark and Gollan, 1966), which delineated their use as alternative respiratory mediums. True PFCs are formulated from common organic compounds (e.g., benzene) by substituting carbon-bound hydrogen atoms with fluorine atoms. They provide the advantage of easy storage (indefinitely at room temperature) and can be used under antiseptic conditions without modification (i.e., autoclave, small-pore filtering). They are clear, in most cases, not soluble in aqueous media or nonlipid biologic fluids and are odorless, inert, and transparent—very inoffensive in their use (Shaffer and Wolfson, 2011).
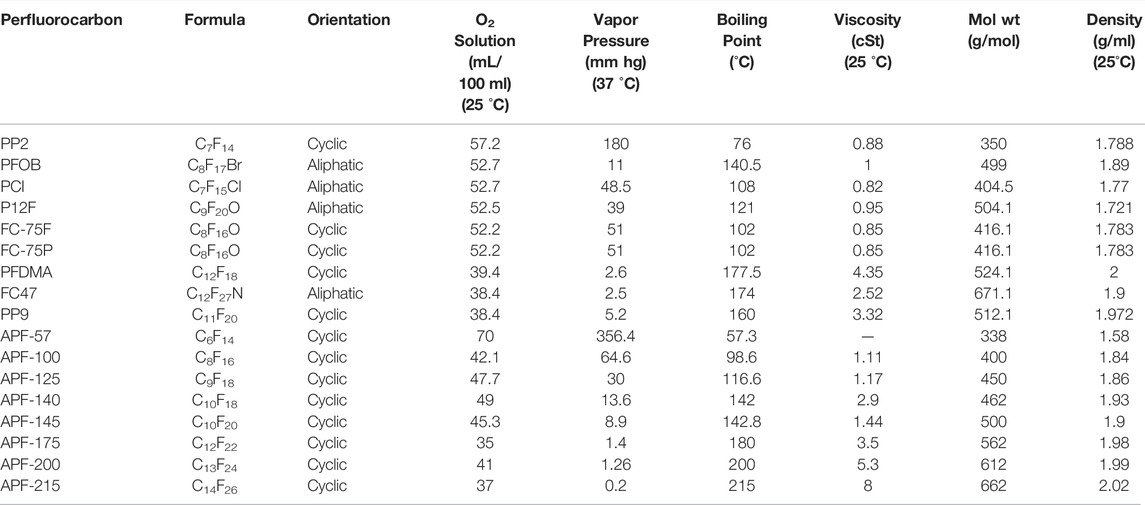
TABLE 1. Physiochemical profile of various perfluorocarbons. Reprinted from Shaffer, T.H., and Wolfson, M.R. (2011). “Liquid Ventilation,” in Fetal and Neonatal Physiology, 4th Edition, eds: R. Polin, W.W. Fox, and S. Abman (Philadelphia, PA: WB Saunders), 1063–1081, with permission from Elsevier.
Please refer to Table 1 (Shaffer and Wolfson, 2011) for details regarding the physicochemical profile and structure of various PFC liquids for PFC ventilation. O2 and CO2 are specific to respiratory gas exchange and carried only as dissolved gases with solubilities ranging as much as 16 and three times greater, respectively, in PFC than in saline. Oxygen solubilities range from 35 to 70 ml gas per deciliter at 25°C (Riess, 1992). The carrying capacity for CO2 is known for only a few PFC compounds, but reported values of CO2 solubility are approximately four times greater than those for O2 (122–225 ml/dl [i.e., PFOB; perfluorooctylbromide [PFOB] = 225 ml/dl]). It is noteworthy that perflubron (PFOB; perfluorooctylbromide) is the only medical grade perfluorochemical approved by the FDA for emergency medical use. While many properties of PFC liquids vary, they do provide relatively low surface tension and viscosity, and are more dense than both water and soft tissue.
Variations in specific physicochemical properties of the PFC liquids are significant to their use as respiratory media and as vehicles for the administration of biological agents. Fluids of higher vapor pressure may volatilize from the lung more rapidly than liquids having lower vapor pressure. Fluids with greater spreading coefficients (dependent on surface tension) may distribute more easily in the lung than fluids whose spreading coefficients are lower (i.e., FC-75 > PFOB > APF-140) (Weers and Johnson, 1991; Sekins, 1995). Fluids of higher viscosity or kinematic viscosity may balk at redistribution in the lung, thus remaining in contact with a greater area of the alveolar surface for more time than those stratifying with increased rapidity (Miller et al., 1999; Miller et al., 2001) resulting in greater flow resistance.
Non-Clinical and Clinical Studies With Inert Liquids
The initial preclinical studies in liquid spontaneous breathing and ventilation support were directed at breathing in unusual environments such as deep sea diving, zero gravity, and space travel (Clark and Gollan, 1966; Modell et al., 1973; Moskowitz et al., 1975; Lynch et al., 1983). It was not until the studies with premature lambs (Shaffer et al., 1976; Shaffer et al., 1983a; Shaffer et al., 1983b; Shaffer et al., 1984b; Wolfson et al., 1988) that the application to respiratory distress became evident because of the advantages of low surface tension, improved lung compliance, and gas exchange. As a result of these investigations, the first in extremis FDA-approved total liquid ventilation study in a near-death premature infant with severe respiratory distress was performed (Greenspan et al., 1989). This study and a subsequent study in several critically ill infants (Greenspan et al., 1990) demonstrated that PFC liquid ventilation could support gas exchange and residual improvement in pulmonary function following the return to conventional gas ventilation. The need for a medically approved combination liquid ventilator and medical grade PFC breathing fluid restricted further clinical trials. It is noteworthy, however, that a corporate-sponsored multicenter trial resulted from the success of the neonatal and adult animal trials with PFC liquid-assisted gas ventilation and the initial clinical trials with human subjects.
Subsequently, several separate investigational new drug applications were approved by the FDA to investigate the safety and efficacy of PFCs, mainly PFOB, as a liquid breathing media in neonates. While animal studies over the years showed significant efficacy and safety of liquid breathing, clinical studies using several techniques in humans (infants, children, and adults) had mixed outcomes. The findings from non-clinical and clinical studies are summarized below.
Non-Clinical Studies With Inert Liquids
Over the course of the last 50 years, many animal studies demonstrated liquid ventilation to be an effective approach/treatment for deep sea diving, zero gravity, severe lung injury, and congenital diaphragmatic hernia (CDH). These studies supported the use of liquid ventilation as a superior source of respiratory support when compared with gas media with spontaneous breathing or conventional mechanical ventilation (CMV). Various studies also demonstrated short-term beneficial physiologic responses in lung function because of improved alveolar recruitment and significant preservation of normal histological structure of the lung (Moskowitz et al., 1975; Shaffer et al., 1983a; Shaffer et al., 1983b; Shaffer et al., 1984a; Shaffer et al., 1984b; Wolfson et al., 1992; Leach et al., 1993; Richman et al., 1993; Sekins et al., 1994; Major et al., 1995; Al-Rahmani et al., 2000; Cox et al., 2003). Non-clinical studies in newborn animal models of respiratory distress syndrome (RDS) showed that PFOB enhances uniformity of the lung inflation consistent with PFOB working as an artificial surfactant (Weis et al., 1997; Wolfson et al., 1998; Kandler et al., 2001; Hübler et al., 2002; Merz et al., 2002). Animal studies also showed that PFOB minimizes functional lung impairment because of the high airway pressures and sustained FiO2 requirements that are characteristics of ventilator-induced lung injury (Greenspan et al., 1990; Wolfson et al., 1992; Bateman et al., 2001; Davies et al., 2002).
Recent studies continue to show PFOB improves oxygenation (Hartog et al., 1997; Bleyl et al., 1999; Al-Rahmani et al., 2000; Bateman et al., 2001; Davies et al., 2002) in animal models of lung injury consistent with earlier findings. Additionally, recent studies report that PFOB increases lung compliance (Bleyl et al., 1999; Al-Rahmani et al., 2000; Kandler et al., 2001; Davies et al., 2002). Findings from earlier studies indicated that PFOB may have potential anti-inflammatory properties. Animal studies showed that administration of PFOB decreased the expression of known inflammatory markers (Kawamae et al., 2000; Haeberle et al., 2002; Merz et al., 2002). Two additional research studies reported PFOB not interfering with cerebral blood flow (Davies et al., 2010; Davies et al., 2013), suggesting partial liquid ventilation (PLV) with PFOB will have limited impact on cardiac output and circulation.
Animal studies consistently support the safety of PFOB, as few negative effects have been reported. Studies show PFOB is not absorbed systemically and causes no long-term harm (Holaday et al., 1972; Shaffer et al., 1996; Cox et al., 2002). Several animal studies reported final concentrations of PFC measured within the blood and tissue after the end of treatment were considered minimal compared with their baseline control measurements (Holaday et al., 1972; Modell et al., 1973; Shaffer et al., 1996; Cox et al., 2002). Additionally, studies revealed initiation of PLV and removal of PFOB does not produce significant adverse effects. Pneumothorax, a documented adverse event in adult PFOB clinical studies, was not reported with any significance in animal models of severe lung injury employing PFOB.
Clinical Studies in Infants With Inert Liquids
Early clinical studies demonstrated that infants with severe RDS, meconium aspiration, and CDH tolerated liquid PFC in their lungs and were able to effectively exchange gas and maintain cardiovascular stability (Greenspan et al., 1989; Greenspan et al., 1990; Gross et al., 1995; Pranikoff et al., 1996; Henrichsen et al., 2012).
Consistent with those studies and animal studies, additional published reports indicated PFOB increased gas exchange (Hirschl et al., 1995; Gauger et al., 1996; Hirschl et al., 1996; Hirschl et al., 2002) in adults and children. Subsequent studies using PFOB on preterm neonates also reported increases in lung compliance (Greenspan et al., 1990; Hirschl et al., 1995; Hirschl et al., 1996). PFOB rapidly improved lung function and increased survival in a population of neonates with high mortality (Leach et al., 1996).
Most importantly, the utility of PFOB was demonstrated in a multicenter study of premature infants with severe RDS refractory to other available treatments (surfactant therapy, high frequency, too young for extracorporeal membrane oxygenation) (Leach et al., 1996). Thirteen (13) infants were treated with PFOB. Within an hour following the instillation of PFOB, there was an increase in arterial oxygen tension of 138%. Dynamic compliance increased by 61% and continued to climb through the first 24 h (Leach et al., 1996). Furthermore, the mean oxygenation index, markedly elevated at baseline (49 ± 60), fell to 17 ± 16 within the first hour and continued to fall to 9 ± 7 at 24 h. Arterial carbon dioxide tension normalized within 4 h after PFOB treatment. Mean airway pressure decreased from 17 ± 3 to 12 ± 2 cm of water (29%) in the first 24 h despite an increase in tidal volume (5.0 ± 3.4 ml/kg during gas ventilation to 7.8 ± 3.4 ml/kg during PFOB ventilation). It should be noted that no serious adverse events were reported during PFOB-assisted ventilation. It was determined that PFOB-assisted ventilation could be utilized for critically ill infants for several days without serious adverse events. A number of the surviving participants are currently well and in their twenties.
More recently, lavage with PFC has been shown to be safe for treatment of persistent and difficult-to-treat lung atelectasis (Henrichsen et al., 2012). Bronchoalveolar lavage utilizing PFC liquid was performed without incident in infants with severe alveolar proteinosis during conventional mechanical ventilation without necessitating the additional support of extracorporeal membrane oxygenation. Furthermore, recent PFC liquid studies have reported safe imaging studies in bronchopulmonary patients (Degnan et al., 2019).
Follow-up imaging studies up to 20 years after treatment with PFOB in humans demonstrated no negative effects from this treatment (Tiruvoipati et al., 2007; Hagerty et al., 2008; Servaes and Epelman, 2009). The studies demonstrated evidence of residual PFC specs in the lung, thorax, mediastinum, and retroperitoneum. These are also cautionary when interpreting the high-density opacifications associated with Hounsfield unit densities of some PFCs used with intrapulmonary applications such as pulmonary calcification (Tiruvoipati et al., 2007) and stress the necessity of obtaining precise clinical histories in the light of unusual radiographic findings (Hagerty et al., 2008).
Clinical Studies in Adults With Inert Liquids
In early liquid ventilation studies, it was reported that PFOB usage in adults increased lung compliance (Hirschl et al., 1996), which is consistent with preclinical animal studies. However, a randomized clinical trial in adults with acute respiratory distress syndrome (ARDS) randomized to protective conventional mechanical ventilation of the lung, low-dose PFC, or high-dose PFC and partial liquid ventilation did not result in improved mortality. Additional ventilator-free days were realized in the conventional mechanical ventilation group when compared with the low-dose and high-dose PFC groups (Hirschl et al., 2002; Degraeuwe and Zimmermann, 2006; Kacmarek et al., 2006). Improved mortality or ventilator-free days did not result in another randomized clinical trial in spite of decreased progression in respiratory insufficiency to ARDS in patients treated with partial liquid ventilation with PFC.
Drug/Gene Product Administration
Systemic administration of therapeutics to target the lung is faced with numerous challenges secondary to potential degradation by serum and hepatic enzymes and rapid renal clearance. Compromised pulmonary blood flow in the injured lung may further limit passive diffusion of the drug from the blood into the lung parenchyma. Retention of the therapeutics in the lung is also often suboptimal. These challenges can be mitigated by local administration of therapeutics through inhalation or airway instillation (Bennett et al., 2002). However, many acute and chronic lung diseases affect distally located alveoli and thus require delivery of biological agents to the distal lung parenchyma for optimal therapeutic effect. Distal delivery of therapeutic agents requires obtaining the correct particle size, which has been challenging. Furthermore, ventilation abnormalities in the impaired lung regions also minimize drug delivery to these target areas.
Drug Delivery With Inert Liquids
Some studies have successfully demonstrated that lungs filled with PFC liquid have the ability to deliver active and inactive agents for the diagnosis and treatment of respiratory disorders.
Respiratory infections affect distally located alveoli with bacteria and viruses multiplying in the alveolar cells. Distal lung distribution of intra-tracheally delivered anti-infective agents is essential to halt disease progression. In a newborn lamb model of acid lung injury, gentamicin administration during tidal liquid ventilation using PFC resulted in significantly higher lung gentamicin levels compared with intravenous administration (Fox et al., 1997; Zelinka et al., 1997; Cullen et al., 1999; Cox et al., 2001). This technique also resulted in greater pulmonary gentamicin levels and lower serum levels, which are required to achieve therapeutic levels in the lung while mitigating systemic adverse effects of gentamicin. Similarly, when utilizing a piglet model of meconium aspiration, greater pulmonary levels and lower serum levels of vancomycin were achieved after intrapulmonary instillation of vancomycin and PFC followed by partial liquid ventilation, compared with intravenous injection of vancomycin followed by conventional gas ventilation (Jeng et al., 2007).
In an animal model of meconium aspiration syndrome, partial liquid ventilation improved regional distribution of intratracheally administered radio-labelled surfactant compared with conventional mechanical ventilation. There was more uniform distribution of surfactant between the lungs as well as between both ventral and dorsal regions of the lungs. Such uniform distribution was associated with improved systemic oxygenation (Chappell et al., 2001). PFC was also an effective delivery vehicle for pulmonary administration of vasoactive agents (Wolfson et al., 1996).
Inhalational smoke-induced acute lung injury is characterized by airway epithelial injury leading to excess leakage of plasma substrates into large airways and the formation of fibrin casts. Interventions to prevent or treat airway casts are limited. In this regard, PFC has been used for intratracheal administration of plasminogen activators (tissue plasminogen activator [tPA] and single-chain urokinase plasminogen activator [scuPA]) for management of airway clot and fibrinous cast formation associated with smoke-induced acute lung injury. Enzymatic activities of the plasminogen activator following dispersion and storage in PFC were preserved, and PFC administration alone did not impact physiologic or histological differences. In contrast, PFC-facilitated plasminogen activator delivery resulted in significantly better physiologic and histologic outcomes. PFC-facilitated delivery of plasminogen activator demonstrated improved outcomes than achieved by nebulization of plasminogen activators alone (Wolfson et al., 2020).
Drug delivery during PFC PLV respiratory support has been demonstrated with other soluble gases in PFOB such as inspired nitric oxide (NO). NO administration with PLV in surfactant-depleted adult pigs resulted in a significant improvement in gas exchange and decrease in pulmonary artery pressure, most notably without deleterious effects on systemic hemodynamic conditions (Houmes et al., 1997). In a congenital diaphragmatic hernia lamb preparation treated prophylactically with PLV, it was demonstrated that NO improved oxygenation and reduced pulmonary hypertension (Wilcox et al., 1994). As such, the ability to deliver NO during PLV is probably related to distribution of NO in the gas-ventilated regions of the lung, the solubility and diffusion of this gas in the PFC, and recruitment of lung volume. Results on the effective delivery of NO in PFC liquid are consistent with earlier studies showing the use of PFC liquid as a vehicle to deliver biologic agents. Based on transport principles, it appears that the amount of NO delivered to pulmonary structures is dependent on NO concentration in PFC liquid, stratification pattern of gas and PFC liquid in the lung, distribution of pulmonary blood flow, and ventilation-perfusion matching. Finally, the clearance of NO from the partially filled PFC lung and potential formation of NO2 during liquid ventilation potentially could be different compared with the gas-filled lung.
Gene Delivery to the Respiratory System
Twenty-two percent of all pediatric hospital admissions are due to respiratory illness. Genetic lung diseases account for increased morbidity and mortality (Nogee, 2010; Tanash et al., 2010; Witt et al., 2012). Genetic diseases such as surfactant protein disease, cystic fibrosis, Hermansky-Pudlak syndrome, and neuroendocrine cell hyperplasia of infancy (NEHI) cause severe lung disease and are associated with high mortality and morbidity. No cure currently exists. Pulmonary epithelial cell-specific genetic mutations and abnormal gene regulation play a causal role in many genetic lung diseases and are attractive targets for airway delivery of therapeutic agents. Effective airway-based delivery of gene therapy vectors is a substantial hurdle to successful gene therapy for lung diseases.
CRISPR-Cas9 gene editing provides an unprecedented opportunity to manipulate genes in somatic cells. Editing technologies have demonstrated clear therapeutic promise in non-human primates and early human clinical trials (Komor et al., 2016; Maeder et al., 2019; Frangoul et al., 2021; Musunuru et al., 2021; Rothgangl et al., 2021). New approaches in base editor design enable installation of targeted, single-nucleotide mutations without double strand breaks or the need for donor DNA templates, an exciting advance that paves the way for correction of single nucleotide polymorphisms that comprise the largest class of known pathogenic genetic variants in humans (Landrum et al., 2016; Stenson et al., 2017). Genetic surfactant protein diseases are a particularly attractive target given that the lung is a barrier organ amenable to intratracheal or nasal treatment applications to selectively reach pulmonary cell lineages (Alton et al., 2015; Alapati et al., 2019; Kang et al., 2020).
The postnatal lung presents important limitations to airway delivery because of the substantial mucus and surfactant barrier at the air-epithelial interface, repulsive charge interactions at the cell membrane, and unequal reagent distribution due to the heterogeneity of lung disease with some areas being overinflated and others collapsed (Kim et al., 2016; Alapati and Morrisey, 2017; Roesch and Drumm, 2017). Furthermore, proteinaceous debris and inflammatory fluids contribute to additional physical barriers in diseased lungs. These barriers limit adequate ventilation, particularly to diseased tissues, resulting in limited delivery of inhaled therapies to needed locations. Systemic drug delivery to diseased lung may be affected by the displacement of blood flow away from the injury site. In contrast, any related absence of such immune and physical barriers in the fluid-filled fetal lungs has resulted in systematic gene transfer to the pulmonary epithelial cells following prenatal viral vector delivery by intra-amniotic injection, taking advantage of fetal breathing movements for lung targeting (Buckley et al., 2005; Endo et al., 2010; Joyeux et al., 2014; Alapati et al., 2019). Additionally, the fluid-filled fetal lung supports relatively uniform targeting of most major pulmonary epithelial cell types, including distal and proximal lineages (Alapati et al., 2019).
Gene Product Delivery With Inert Liquids
The fluid-filled fetal lung physiology could be partially mimicked in postnatal lungs with PFC liquids because of their high spreading coefficients and intriguing properties for pulmonary distribution of biological agents. The high O2 and CO2 solubility of PFC liquids allows effective gas exchange, even in lungs filled with fluid. PFCs also reduce surface tension, which assists lung volume recruitment at reduced inspiratory pressures by eliminating the air-liquid interface. Importantly, PFC liquids effectively penetrate collapsed regions of the lung to facilitate access to under-ventilated regions. This is particularly important in non-homogenous lung diseases such as surfactant protein diseases, in which PFC liquids could simultaneously facilitate delivery of therapies, increase gas exchange, and improve pulmonary function. Several groups have exhibited the superior effectiveness of PFC liquids as vehicles for pulmonary distribution of genetic cargo (Lisby et al., 1997; Weiss et al., 1999a; Weiss et al., 1999b; Weiss et al., 2000; Kazzaz et al., 2011). PFC liquids instilled during the intratracheal administration of recombinant viral vector propelled the vector more effectively into the lung. As a result, PFC enhanced airway and alveolar epithelial gene expression in both normal and injured rodent lungs (Weiss et al., 2001). Use of PFC as vehicle for delivery of genetic cargo also resulted in earlier detection of gene expression and need for lesser amounts of vector. In all of these studies, PFC was administered immediately following administration of aqueous vector because of immiscibility of PFC with aqueous solutions. The propulsive effect of PFC resulted in improved delivery and distribution of the vectors. PFC liquids also transiently decreased transepithelial resistance and increased tight junction permeability. This transient increase in epithelial permeability enhanced access to viral vectors and gene expression. The peak effect was observed from 6 h to 1 day following instillation. Notably, alveolar-capillary permeability was not affected (Weiss et al., 2003). Many studies have also demonstrated improvement in lung mechanics and oxygenation in research models of lung injury following administration of PFC liquids in nebulized or aerosolized forms (Bleyl et al., 1999; Ragaller et al., 2001; Kandler et al., 2004; von der Hardt et al., 2004). As such, administration of nebulized perflubron improved resulting recombinant viral vector mediated gene expression (Beckett et al., 2012). By adapting and optimizing PFC liquid strategies demonstrated to be beneficial for viral vector gene delivery, PFC liquids hold promise for enhanced airway delivery of CRISPR systems as therapeutic strategy for a myriad of respiratory disorders.
Considerations for Improving Drug/Gene Delivery With Inert Liquids
The fact that aqueous solutions are not readily soluble in PFCs is an important consideration for PFC drug delivery. Some research projects have circumvented this barrier by relying on bulk flow turbulent mixing (Wolfson et al., 1996; Lisby et al., 1997). However, techniques that improve solubility of the drug or biologic agent in PFC are advantageous to provide stability and equivalent disbursement of the drug within the lung and for more controlled dosing procedures. One such method is generation of nanocrystals that can then be administered during partial liquid ventilation. This approach was successfully utilized by developing gentamicin/perfluorochemical nanocrystal suspension that was delivered using two techniques (Cullen et al., 1999). In the first technique, called the top-fill technique, gentamicin/PFC nanocrystal suspension was instilled through the sideport of an endotracheal tube 29 ± 8 min after initiation of partial liquid ventilation with a bolus of oxygenated perflubron. In the second technique, called the slow-fill technique, the gentamicin/PFC nanocrystal suspension was combined with perflubron, vortexed, and delivered through the sideport of an endotracheal tube. Thus, in the second technique, partial liquid ventilation and gentamicin treatment were initiated simultaneously. Both the techniques resulted in effective distribution of gentamicin into the lung and greater gentamicin levels per gram of dry lung tissue compared with intravenous administration of aqueous gentamicin. The amount of original gentamicin dose left in the lobes of the lungs adjusted for dry weight after 4 h and was greater in the slow-fill technique compared with the top-fill technique.
Summary
When lung parenchymal disease and/or injury are present in the lung, pulmonary ventilation and perfusion are compromised. Ventilation can be irregular and perfusion may be inhibited by ventilation-perfusion mismatch. The route of therapy administration is hindered by these abnormalities, rendering standard intravenous and aerosol/endotracheal tube delivery ineffective in delivering therapeutic agents to the affected area.
Liquid ventilation with an inert respiratory gas solubility is a revolutionary mode for respiratory support, as well as delivery of drug/gene product to the respiratory system. As noted, inert perfluorochemical liquids have low viscosity and high oxygen and carbon dioxide capabilities (Grotberg, 2001). The physical properties of PFC liquids improve lung mechanics and gas exchange and condition the lung parenchymal surface for optimal administration of drug/gene product.
The use of PFC liquid in the respiratory system enhances ventilation and perfusion matching, boosting exposure of the drug/gene product to the circulation, successfully reaching required therapeutic serum drug levels (Fox et al., 1997). Studies have demonstrated the utilization of PFCs as adjuncts for intrapulmonary biological agent delivery both preclinically and clinically as reported herein.
Author Contributions
DA and TS conceptualized the idea and prepared the first draft and final manuscript. Both authors approve the final version of the manuscript and agree to be accountable for the content of the work.
Funding
This study was supported by NIH K08 HL151760 01 (DA), American Thoracic Society Foundation (DA), Institutional Development Award (IDeA) from the NIH/NGMS COBRE P30GM114736 (TS), and The Nemours Foundation.
Conflict of Interest
The authors declare that the research was conducted in the absence of any commercial or financial relationships that could be construed as a potential conflict of interest.
Publisher’s Note
All claims expressed in this article are solely those of the authors and do not necessarily represent those of their affiliated organizations, or those of the publisher, the editors and the reviewers. Any product that may be evaluated in this article, or claim that may be made by its manufacturer, is not guaranteed or endorsed by the publisher.
References
Al-Rahmani A., Awad K., Miller T. F., Wolfson M. R., Shaffer T. H. (2000). Effects of Partial Liquid Ventilation with Perfluorodecalin in the Juvenile Rabbit Lung after Saline Injury. Crit. Care Med. 28, 1459–1464. doi:10.1097/00003246-200005000-00034
Alapati D., Zacharias W. J., Hartman H. A., Rossidis A. C., Stratigis J. D., Ahn N. J., et al. (2019). In Utero Gene Editing for Monogenic Lung Disease. Sci. Transl. Med. 11, eaav8375. doi:10.1126/scitranslmed.aav8375
Alapati D., Morrisey E. E. (2017). Gene Editing and Genetic Lung Disease. Basic Research Meets Therapeutic Application. Am. J. Respir. Cel Mol. Biol. 56, 283–290. doi:10.1165/rcmb.2016-0301ps
Ali M. E., McConville J. T., Lamprecht A. (2015). Pulmonary Delivery of Anti-Inflammatory Agents. Expert Opin. Drug Deliv. 12, 929–945. doi:10.1517/17425247.2015.993968
Alton E. W. F. W., Armstrong D. K., Ashby D., Bayfield K. J., Bilton D., Bloomfield E. V., et al. (2015). Repeated Nebulisation of Non-Viral CFTR Gene Therapy in Patients with Cystic Fibrosis: A Randomised, Double-Blind, Placebo-Controlled, Phase 2b Trial. Lancet Respir. Med. 3, 684–691. doi:10.1016/S2213-2600(15)00245-3
Avery M. E., Mead J. (1959). Surface Properties in Relation to Atelectasis and Hyaline Membrane Disease. Arch. Pediatr. Adolesc. Med. 97, 517. doi:10.1001/archpedi.1959.02070010519001
Bateman S. T., Doctor A., Price B., Murphy M. A., Thompson J. E., Zurakowski D., et al. (2001). Optimizing Intrapulmonary Perfluorocarbon Distribution: Fluoroscopic Comparison of Mode of Ventilation and Body Position. Crit. Care Med. 29, 601–608. doi:10.1097/00003246-200103000-00024
Beckett T., Bonneau L., Howard A., Blanchard J., Borda J., Weiner D. J., et al. (2012). Inhalation of Nebulized Perfluorochemical Enhances Recombinant Adenovirus and Adeno-Associated Virus-Mediated Gene Expression in Lung Epithelium. Hum. Gene Ther. Methods 23, 98–110. doi:10.1089/hgtb.2012.014
Bennett W. D., Brown J. S., Zeman K. L., Hu S.-C., Scheuch G., Sommerer K. (2002). Targeting Delivery of Aerosols to Different Lung Regions. J. Aerosol Med. 15, 179–188. doi:10.1089/089426802320282301
Bleyl J. U., Ragaller M., Tscho U., Regner M., Kanzow M., Hubler M., et al. (1999). Vaporized Perfluorocarbon Improves Oxygenation and Pulmonary Function in an Ovine Model of Acute Respiratory Distress Syndrome. Anesthesiology 91, 461–469. doi:10.1097/00000542-199908000-00021
Buckley S. M. K., Waddington S. N., Jezzard S., Lawrence L., Schneider H., Holder M. V., et al. (2005). Factors Influencing Adenovirus-Mediated Airway Transduction in Fetal Mice. Mol. Ther. 12, 484–492. doi:10.1016/j.ymthe.2005.02.020
Chappell S. E., Wolfson M. R., Shaffer T. H. (2001). A Comparison of Surfactant Delivery with Conventional Mechanical Ventilation and Partial Liquid Ventilation in Meconium Aspiration Injury. Respir. Med. 95, 612–617. doi:10.1053/rmed.2001.1114
Clark L. C., Gollan F. (1966). Survival of Mammals Breathing Organic Liquids Equilibrated with Oxygen at Atmospheric Pressure. Science 152, 1755–1756. doi:10.1126/science.152.3730.1755
Cox C. A., Cullen A. B., Wolfson M. R., Shaffer T. H. (2001). Intratracheal Administration of Perfluorochemical-Gentamicin Suspension: A Comparison to Intravenous Administration in Normal and Injured Lungs. Pediatr. Pulmonol. 32, 142–151. doi:10.1002/ppul.1100
Cox C. A., Fox W. W., Weiss C. M., Wolfson M. R., Shaffer T. H. (2002). Liquid Ventilation: Gas Exchange, Perfluorochemical Uptake and Biodistribution in an Acute Lung Injury Model. Pediatr. Crit. Care Med. 3, 288–296. doi:10.1097/00130478-200207000-00017
Cox C., Stavis R. L., Wolfson M. R., Shaffer T. H. (2003). Long-Term Tidal Liquid Ventilation in Premature Lambs: Physiologic, Biochemical and Histological Correlates. Biol. Neonate. 84, 232–242. doi:10.1159/000072307
Cullen A. B., Cox C. A., Hipp S. J., Wolfson M. R., Shaffer T. H. (1999). Intra-Tracheal Delivery Strategy of Gentamicin with Partial Liquid Ventilation. Respir. Med. 93, 770–778. doi:10.1016/s0954-6111(99)90261-5
Davidson A., Donner R., Heckman J. L., Miller T. F., Shaffer T. H., Wolfson M. R. (1995). Partial Liquid Ventilation Reduces Left Ventricular Wall Stress in Rescue of Surfactant-Treated Preterm Lambs. Chest 3, 108.
Davies M., Stewart M., Chavasse R., Bayley G., Butt W. (2002). Partial Liquid Ventilation and Nitric Oxide in Experimental Acute Lung Injury. J. Paediatr. Child. Health 38, 492–496. doi:10.1046/j.1440-1754.2002.00036.x
Davies M. W., Dunster K. R., Fraser J. F., Colditz P. B. (2013). Cerebral Blood Flow Is Not Affected during Perfluorocarbon Dosing with Volume-Controlled Ventilation. J. Paediatr. Child. Health 49, 1010–1018. doi:10.1111/jpc.12297
Davies M. W., Dunster K. R., Wilson K., Colditz P. B. (2010). Perfluorocarbon Dosing when Starting Partial Liquid Ventilation: Haemodynamics and Cerebral Blood Flow in Preterm Lambs. Neonatology 97, 144–153. doi:10.1159/000239768
Degnan A. J., Fox W. W., Zhang H., Saul D. (2019). Partial Liquid Ventilation for Bronchopulmonary Dysplasia: Visualizing Ventilation Patterns on Chest Radiographs. Pediatr. Neonatal. 60, 587–588. doi:10.1016/j.pedneo.2018.10.004
Degraeuwe P. L. J., Zimmermann L. J. I. (2006). Why Partial Liquid Ventilation Did Not Fulfill its Promise. Am. J. Respir. Crit. Care Med. 174, 615. doi:10.1164/ajrccm.174.5.615
Endo M., Henriques-Coelho T., Zoltick P. W., Stitelman D. H., Peranteau W. H., Radu A., et al. (2010). The Developmental Stage Determines the Distribution and Duration of Gene Expression after Early Intra-Amniotic Gene Transfer Using Lentiviral Vectors. Gene Ther. 17, 61–71. doi:10.1038/gt.2009.115
Fournier L., Major D., Cadenas M., Leclerc S., Cloutler R., Wolfson M. R., et al. (1995). Morphometric and Physiological Differences between Gas and Liquid Ventilated Lung. Can. J. Anaesth. 42, A29B.
Fox W. W., Weis C. M., Cox C., Farina C., Drott H., Wolfson M. R., et al. (1997). Pulmonary Administration of Gentamicin during Liquid Ventilation in a Newborn Lamb Lung Injury Model. Pediatrics 100, E5. doi:10.1542/peds.100.5.e5
Frangoul H., Altshuler D., Cappellini M. D., Chen Y.-S., Domm J., Eustace B. K., et al. (2021). CRISPR-Cas9 Gene Editing for Sickle Cell Disease and β-Thalassemia. N. Engl. J. Med. 384, 252–260. doi:10.1056/nejmoa2031054
Gauger P. G., Pranikoff T., Schreiner R. J., Moler F. W., Hirschl R. B. (1996). Initial Experience with Partial Liquid Ventilation in Pediatric Patients with the Acute Respiratory Distress Syndrome. Crit. Care Med. 24, 16–22. doi:10.1097/00003246-199601000-00006
Greenspan J. S., Wolfson M. R., Rubenstein S. D., Shaffer T. H. (1990). Liquid Ventilation of Human Preterm Neonates. J. Pediatr. 117, 106–111. doi:10.1016/s0022-3476(05)82457-6
Greenspan J., Wolfson M., David Rubenstein S., Shaffer T. (1989). Liquid Ventilation of Preterm Baby. Lancet 334, 1095. doi:10.1016/s0140-6736(89)91101-x
Gross G. W., Greenspan J. S., Fox W. W., Rubenstein S. D., Wolfson M. R., Shaffer T. H. (1995). Use of Liquid Ventilation with Perflubron during Extracorporeal Membrane Oxygenation: Chest Radiographic Appearances. Radiology 194, 717–720. doi:10.1148/radiology.194.3.7862968
Grotberg J. B. (2001). Respiratory Fluid Mechanics and Transport Processes. Annu. Rev. Biomed. Eng. 3, 421–457. doi:10.1146/annurev.bioeng.3.1.421
Haeberle H. A., Nesti F., Dieterich H.-J., Gatalica Z., Garofalo R. P. (2002). Perflubron Reduces Lung Inflammation in Respiratory Syncytial Virus Infection by Inhibiting Chemokine Expression and Nuclear Factor-κB Activation. Am. J. Respir. Crit. Care Med. 165, 1433–1438. doi:10.1164/rccm.2109077
Hagerty R. D., Phelan M. P., Morrison S. C., Hatem S. F. (2008). Radiographic Detection of Perflubron Fluoromediastinum and Fluororetroperitoneum 9 Years after Partial Liquid Ventilation. Emerg. Radiol. 15, 71–75. doi:10.1007/s10140-007-0673-2
Hamosh P., Luchsinger P. C. (1968). Maximum Expiratory Flow in Isolated Liquid-Filled Lungs. J. Appl. Physiol. 25, 485–488. doi:10.1152/jappl.1968.25.5.485
Hartog A., Houmes R. J. M., Verbrugge S. J. C., Erdmann W., Lachmann B. (1997). Partial Liquid Ventilation and Inhaled Nitric Oxide Have a Cumulative Effect in Improving Arterial Oxygenation in Experimental ARDS. Adv. Exp. Med. Biol. 428, 281–283. doi:10.1007/978-1-4615-5399-1_39
Henrichsen T., Lindenskov P. H., Shaffer T. H., Loekke R. J., Fugelseth D., Lindemann R. (2012). Perfluorodecalin Lavage of a Longstanding Lung Atelectasis in a Child with Spinal Muscle Atrophy. Pediatr. Pulmonol. 47, 415–419. doi:10.1002/ppul.21565
Hirschl R. B., Croce M., Gore D., Wiedemann H., Davis K., Zwischenberger J., et al. (2002). Prospective, Randomized, Controlled Pilot Study of Partial Liquid Ventilation in Adult Acute Respiratory Distress Syndrome. Am. J. Respir. Crit. Care Med. 165, 781–787. doi:10.1164/ajrccm.165.6.2003052
Hirschl R. B., Pranikoff T., Gauger P., Schreiner R. J., Dechert R., Bartlett R. H. (1995). Liquid Ventilation in Adults, Children and Full-Term Neonates. Lancet 346, 1201–1202. doi:10.1016/s0140-6736(95)92903-7
Hirschl R. B., Pranikoff T., Wise C., Overbeck M. C., Gauger P., Schreiner R. J., et al. (1996). Initial Experience with Partial Liquid Ventilation in Adult Patients with the Acute Respiratory Distress Syndrome. JAMA 275, 383–389. doi:10.1001/jama.275.5.383
Holaday D. A., Fiserova-Bergerova V., Modell J. H. (1972). Uptake, Distribution, and Excretion of Fluorocarbon FX-80 (Perfluorobutyl Perfluorotetrahydrofuran) during Liquid Breathing in the Dog. Anesthesiolog 37, 387–394. doi:10.1097/00000542-197210000-00005
Houmes R. J., Hartog A., Verbrugge S. J., Böhm S., Lachmann B. (1997). Combining Partial Liquid Ventilation with Nitric Oxide to Improve Gas Exchange in Acute Lung Injury. Intensive Care Med. 23, 163–169.
Hübler M., Souders J. E., Shade E. D., Polissar N. L., Bleyl J. U., Hlastala M. P. (2002). Effects of Perfluorohexane Vapor on Relative Blood Flow Distribution in an Animal Model of Surfactant-Depleted Lung Injury. Crit. Care Med. 30, 422–427. doi:10.1097/00003246-200202000-00026
Jeng M. J., Lee Y. S., Soong W. J. (2007). Effects of Perfluorochemicals for Intrapulmonary Vancomycin Administration and Partial Liquid Ventilation in an Animal Model of Meconium-Injured Lungs. Acta Paediatr. Taiwan. 48, 309–316.
Joyeux L., Danzer E., Limberis M. P., Zoltick P. W., Radu A., Flake A. W., et al. (2014). In Utero lung Gene Transfer Using Adeno-Associated Viral and Lentiviral Vectors in Mice. Hum. Gene Ther. Methods 25, 197–205. doi:10.1089/hgtb.2013.143
Kacmarek R. M., Wiedemann H. P., Lavin P. T., Wedel M. K., Tütüncü A. S., Slutsky A. S. (2006). Partial Liquid Ventilation in Adult Patients with Acute Respiratory Distress Syndrome. Am. J. Respir. Crit. Care Med. 173, 882–889. doi:10.1164/rccm.200508-1196oc
Kandler M. A., von der Hardt K., Gericke N., Chada M., Dötsch J., Rascher W. (2004). Dose Response to Aerosolized Perflubron in a Neonatal Swine Model of Lung Injury. Pediatr. Res. 56, 191–197. doi:10.1203/01.pdr.0000132667.47744.f4
Kandler M. A., von der Hardt K., Schoof E., Dötsch J., Rascher W. (2001). Persistent Improvement of Gas Exchange and Lung Mechanics by Aerosolized Perfluorocarbon. Am. J. Respir. Crit. Care Med. 164, 31–35. doi:10.1164/ajrccm.164.1.2010049
Kang M. H., van Lieshout L. P., Xu L., Domm J. M., Vadivel A., Renesme L., et al. (2020). A Lung Tropic AAV Vector Improves Survival in a Mouse Model of Surfactant B Deficiency. Nat. Commun. 11, 3929. doi:10.1038/s41467-020-17577-8
Kawamae K., Pristine G., Chiumello D., Tremblay L. N., Slutsky A. S. (2000). Partial Liquid Ventilation Decreases Serum Tumor Necrosis Factor-Alpha Concentrations in a Rat Acid Aspiration Lung Injury Model. Crit. Care Med. 28, 479–483. doi:10.1097/00003246-200002000-00032
Kazzaz J. A., Strayer M. S., Wu J., Malone D. J., Koo H. C., Shaffer T. H., et al. (2011). Perfluorochemical Liquid-Adenovirus Suspensions Enhance Gene Delivery to the Distal Lung. Pulm. Med. 2011, 918036. doi:10.1155/2011/918036
Keshavarz A., Kadry H., Alobaida A., Ahsan F. (2020). Newer Approaches and Novel Drugs for Inhalational Therapy for Pulmonary Arterial Hypertension. Expert Opin. Drug Deliv. 17, 439–461. doi:10.1080/17425247.2020.1729119
Kim N., Duncan G. A., Hanes J., Suk J. S. (2016). Barriers to Inhaled Gene Therapy of Obstructive Lung Diseases: a Review. J. Controlled Release 240, 465–488. doi:10.1016/j.jconrel.2016.05.031
Komor A. C., Kim Y. B., Packer M. S., Zuris J. A., Liu D. R. (2016). Programmable Editing of a Target Base in Genomic DNA without Double-Stranded DNA Cleavage. Nature 533, 420–424. doi:10.1038/nature17946
Kumar M., Jha A., Dr M., Mishra B. (2020). Targeted Drug Nanocrystals for Pulmonary Delivery: a Potential Strategy for Lung Cancer Therapy. Expert Opin. Drug Deliv. 17, 1459–1472. doi:10.1080/17425247.2020.1798401
Kylstra J. (1967). “Advantages and Limitations of Liquid Breathing,” in Proceedings of the Third Symposium on Underwater Physiology. Editor C. J. Lambertsen (Baltimore: Williams & Wilkins), 341–350.
Kylstra J. A., Paganelli C. V., Lanphier E. H. (1966). Pulmonary Gas Exchange in Dogs Ventilated with Hyperbarically Oxygenated Liquid. J. Appl. Physiol. 21, 177–184. doi:10.1152/jappl.1966.21.1.177
Kylstra J. A., Schoenfisch W. H., Herron J. M., Blenkarn G. D. (1973). Gas Exchange in Saline-Filled Lungs of Man. J. Appl. Physiol. 35, 136–142. doi:10.1152/jappl.1973.35.1.136
Kylstra J. A., Tissing M. O., van der Maëʼn A., de Graaf R. P. (1962). Of Mice as Fish. Trans. Am. Soc. Artif. Intern. Organs. 8, 378–383. doi:10.1097/00002480-196204000-00077
Landrum M. J., Lee J. M., Benson M., Brown G., Chao C., Chitipiralla S., et al. (2016). ClinVar: Public Archive of Interpretations of Clinically Relevant Variants. Nucleic Acids Res. 44, D862–D868. doi:10.1093/nar/gkv1222
Leach C. L., Fuhrman B. P., Morin F. C., Rath M. G. (1993). Perfluorocarbon-associated Gas Exchange (Partial Liquid Ventilation) in Respiratory Distress Syndrome: A Prospective, Randomized, Controlled Study. Crit. Care Med. 21, 1270–1278. doi:10.1097/00003246-199309000-00008
Leach C. L., Greenspan J. S., Rubenstein S. D., Shaffer T. H., Wolfson M. R., Jackson J. C., et al. (1996). Partial Liquid Ventilation with Perflubron in Premature Infants with Severe Respiratory Distress Syndrome. The LiquiVent Study Group. N. Engl. J. Med. 335, 761–767. doi:10.1056/nejm199609123351101
Lisby D. A., Ballard P. L., Fox W. W., Wolfson M. R., Shaffer T. H., Gonzales L. W. (1997). Enhanced Distribution of Adenovirus-Mediated Gene Transfer to Lung Parenchyma by Perfluorochemical Liquid. Hum. Gene Ther. 8, 919–928. doi:10.1089/hum.1997.8.8-919
Lynch P. R., Wilson J. S., Shaffer T. H., Cohen N. (1983). Decompression Incidence in Air- and Liquid-Breathing Hamsters. Undersea Biomed. Res. 10, 1–10. doi:10.1029/gl010i012p01176
Maeder M. L., Stefanidakis M., Wilson C. J., Baral R., Barrera L. A., Bounoutas G. S., et al. (2019). Development of a Gene-Editing Approach to Restore Vision Loss in Leber Congenital Amaurosis Type 10. Nat. Med. 25, 229–233. doi:10.1038/s41591-018-0327-9
Major D., Cadenas M., Cloutier R., Fournier L., Wolfson M. R., Shaffer T. H., et al. (1995). Combined Gas Ventilation and Perfluorochemical Tracheal Instillation as an Alternative Treatment for Lethal Congenital Diaphragmatic Hernia in Lambs. J. Pediatr. Surg. 30, 1178–1182. doi:10.1016/0022-3468(95)90016-0
Mead J., Whittenberger J. L., Radford E. P. (1957). Surface Tension as a Factor in Pulmonary Volume-Pressure Hysteresis. J. Appl. Physiol. 10, 191–196. doi:10.1152/jappl.1957.10.2.191
Merz U., Klosterhalfen B., Häusler M., Kellinghaus M., Peschgens T., Hörnchen H. (2002). Partial Liquid Ventilation Reduces Release of Leukotriene B4 and Interleukin-6 in Bronchoalveolar Lavage in Surfactant-Depleted Newborn Pigs. Pediatr. Res. 51, 183–189. doi:10.1203/00006450-200202000-00010
Miller T. F., Milestone B., Stern R., Shaffer T. H., Wolfson M. R. (1999). Effect of Single versus Multiple Dosing on Perfluorochemical Distribution and Elimination during Partial Liquid Ventilation. Pediatr. Pulmonol. 27, 410–418. doi:10.1002/(sici)1099-0496(199906)27:6<410::aid-ppul8>3.0.co;2-5
Miller T. F., Milestone B., Stern R., Shaffer T. H., Wolfson M. R. (2001). Effects of Perfluorochemical Distribution and Elimination Dynamics on Cardiopulmonary Function. J. Appl. Physiol. 90, 839–849. doi:10.1152/jappl.2001.90.3.839
Modell J. G., Tham M. K., Modell J. H., Calderwood H. W., Ruiz B. C. (1973). Distribution and Retention of Fluorocarbon in Mice and Dogs after Injection or Liquid Ventilation. Toxicol. Appl. Pharmacol. 26, 86–92. doi:10.1016/0041-008x(73)90088-4
Moskowitz G. D., Shaffer T. H., Dubin S. E. (1975). Liquid Breathing Trials and Animal Studies with a Demand-Regulated Liquid Breathing System. Med. Instrum. 9, 28–33.
Muralidharan P., Malapit M., Mallory E., Hayes D., Mansour H. M. (2015). Inhalable Nanoparticulate Powders for Respiratory Delivery. Nanomedicine 11, 1189–1199. doi:10.1016/j.nano.2015.01.007
Musunuru K., Chadwick A. C., Mizoguchi T., Garcia S. P., DeNizio J. E., Reiss C. W., et al. (2021). In Vivo CRISPR Base Editing of PCSK9 Durably Lowers Cholesterol in Primates. Nature 593, 429–434. doi:10.1038/s41586-021-03534-y
Neergard D. V. (1929). Neue Auffassungen über einen Grundbegriff der Atemmechanic. Die Retraktionkraft der Lunge, abhangig von der Oberfl achenspannung in den Alveolen. Z. Gesamte. Exp. Med. 66, 373.
Nogee L. M. (2010). Genetic Basis of Children’s Interstitial Lung Disease. Pediatr. Allergy Immunol. Pulmonol. 23, 15–24. doi:10.1089/ped.2009.0024
Pegg J. H., Horner T. L., Wahrenbrock E. A. (1963). “Breathing of Pressure-Oxygenated Liquids. In: National Academy of Sciences—National Research Council,” in Proceedings of the Second Symposium on Underwater Physiology (Publication No. 1181) (Washington, D.C.: National Academy of Sciences—National Research Council), 166–170.
Pranikoff T., Gauger P. G., Hirschl R. B. (1996). Partial Liquid Ventilation in Newborn Patients with Congenital Diaphragmatic Hernia. J. Pediatr. Surg. 31, 613–618. doi:10.1016/s0022-3468(96)90659-4
Ragaller M., Bleyl J., Tschö U., Winkler T., Regner M., Rasche S., et al. (2001). Effects of Inhalation of Perfluorocarbon Aerosol on Oxygenation and Pulmonary Function Compared to PGI2 Inhalation in a Sheep Model of Oleic Acid-Induced Lung Injury. Intensive Care Med. 27, 889–897. doi:10.1007/s001340100921
Richman P. S., Wolfson M. R., Shaffer T. H. (1993). Lung Lavage with Oxygenated Perfluorochemical Liquid in Acute Lung Injury. Crit. Care Med. 21, 768–774. doi:10.1097/00003246-199305000-00022
Riess J. G. (1992). Overview of Progress in the Fluorocarbon Approach Toin Vivo Oxygen Delivery. Biomater. Artif. Cell Immobilization Biotechnol. 20, 183–202. doi:10.3109/10731199209119635
Roesch E. A., Drumm M. L. (2017). Powerful Tools for Genetic Modification: Advances in Gene Editing. Pediatr. Pulmonol. 52, S15–S20. doi:10.1002/ppul.23791
Rothgangl T., Dennis M. K., Lin P. J. C., Oka R., Witzigmann D., Villiger L., et al. (2021). In Vivo adenine Base Editing of PCSK9 in Macaques Reduces LDL Cholesterol Levels. Nat. Biotechnol. 39, 949–957. doi:10.1038/s41587-021-00933-4
Sekins K. M., Coalson J. J., deLemos R. A., Fields T. K., Flaim S. F., Guerra J. M., et al. (1994). Long-term Partial Liquid Ventilation (PLV) with Perflubron in the Near-Term Baboon Neonate. Artif. Cell Blood Substitutes, Biotechnol. 22, 1381–1387. doi:10.3109/10731199409138841
Sekins K. M. (1995). Lung Cancer Hyperthermia via Ultrasound and PFC Liquids: Final Report. Bethesda, MD: National Institutes of Health. National Institutes of Health Grant R43 CA48611-03.
Servaes S., Epelman M. (2009). Perflubron Residua: 12 Years Following Therapy. Pediatr. Radiol. 39, 393–395. doi:10.1007/s00247-008-1139-8
Shaffer T. H., Wolfson M. R., Greenspan J. S., Hoffman R. E., Davis S. L., Clark L. C. (1996). Liquid Ventilation in Premature Lambs: Uptake, Biodistribution and Elimination of Perfluorodecalin Liquid. Reprod. Fertil. Dev. 8, 409–416. doi:10.1071/rd9960409
Shaffer T. H., Douglas P. R., Lowe C. A., Bhutani V. K. (1983a). The Effects of Liquid Ventilation on Cardiopulmonary Function in Preterm Lambs. Pediatr. Res. 17, 303–306. doi:10.1203/00006450-198304000-00017
Shaffer T. H., Forman D. L., Wolfson M. R. (1984a). Physiological Effects of Ventilation with Liquid Fluorocarbon at Controlled Temperatures. Undersea Biomed. Res. 11, 287–298.
Shaffer T. H., Lowe C. A., Bhutani V. K., Douglas P. R. (1984b). Liquid Ventilation: Effects on Pulmonary Function in Distressed Meconium-Stained Lambs. Pediatr. Res. 18, 47–52.
Shaffer T. H., Rubenstein D., Moskowitz G. D., Delivoria-Papadopoulos M. (1976). Gaseous Exchange and Acid-Base Balance in Premature Lambs during Liquid Ventilation since Birth. Pediatr. Res. 10, 227–231. doi:10.1203/00006450-197604000-00005
Shaffer T. H., Tran N., Bhutani V. K., Sivieri E. M. (1983b). Cardiopulmonary Function in Very Preterm Lambs during Liquid Ventilation. Pediatr. Res. 17, 680–684. doi:10.1203/00006450-198308000-00016
Shaffer T. H., Wolfson M. R. (2011). “Liquid Ventilation,” in Fetal and Neonatal Physiology. Editors R. Polin, W. W. Fox, and S. Abman. 4th Edition (Philadelphia, PA: WB Saunders), 1063–1081. doi:10.1016/b978-1-4160-3479-7.10098-9
Stein S. N., Sonnenschein R. R. (1950). Electrical Activity and Oxygen Tension of Brain during Hyperoxie Convulsions. J. Aviat. Med. 21, 401–405.
Stein S. W., Thiel C. G. (2017). The History of Therapeutic Aerosols: A Chronological Review. J. Aerosol Med. Pulm. Drug Deliv. 30, 20–41. doi:10.1089/jamp.2016.1297
Stenson P. D., Mort M., Ball E. V., Evans K., Hayden M., Heywood S., et al. (2017). The Human Gene Mutation Database: towards a Comprehensive Repository of Inherited Mutation Data for Medical Research, Genetic Diagnosis and Next-Generation Sequencing Studies. Hum. Genet. 136, 665–677. doi:10.1007/s00439-017-1779-6
Tanash H. A., Nilsson P. M., Nilsson J.-Å., Piitulainen E. (2010). Survival in Severe Alpha-1-Antitrypsin Deficiency (PiZZ). Respir. Res. 11, 44–50. doi:10.1186/1465-9921-11-44
Tiruvoipati R., Balasubramanian S. K., Entwisle J. J., Firmin R. K., Peek G. J. (2007). Pseudocalcification on Chest CT Scan. Br. J. Radiol. 80, e125–e127. doi:10.1259/bjr/61486305
von der Hardt K., Kandler M. A., Brenn G., Scheuerer K., Schoof E., Dötsch J., et al. (2004). Comparison of Aerosol Therapy with Different Perfluorocarbons in Surfactant-Depleted Animals. Crit. Care Med. 32, 1200–1206. doi:10.1097/01.ccm.0000124876.31138.f6
Weers J., Johnson C. (1991). Equilibrium Spreading Coefficients of Perfluorocarbons. San Diego, CA: Alliance Pharmaceutical Corporation. Research and Development Report.
Weis C. M., Wolfson M. R., Shaffer T. H. (1997). Liquid-Assisted Ventilation: Physiology and Clinica Application. Ann. Med. 29, 509–517. doi:10.3109/07853899709007475
Weiss D. J., Beckett T., Bonneau L., Young J., Kolls J. K., Wang G. (2003). Transient Increase in Lung Epithelial Tight junction Permeability: An Additional Mechanism for Enhancement of Lung Transgene Expression by Perfluorochemical Liquids. Mol. Ther. 8, 927–935. doi:10.1016/j.ymthe.2003.09.003
Weiss D. J., Bonneau L., Allen J. M., Miller A. D., Halbert C. L. (2000). Perfluorochemical Liquid Enhances Adeno-Associated Virus-Mediated Transgene Expression in Lungs. Mol. Ther. 2, 624–630. doi:10.1006/mthe.2000.0207
Weiss D. J., Bonneau L., Liggitt D. (2001). Use of Perfluorochemical Liquid Allows Earlier Detection of Gene Expression and Use of Less Vector in normal Lung and Enhances Gene Expression in Acutely Injured Lung. Mol. Ther. 3, 734–745. doi:10.1006/mthe.2001.0321
Weiss D. J., Strandjord T. P., Jackson J. C., Clark J. G., Liggitt D. (1999a). Perfluorochemical Liquid-Enhanced Adenoviral Vector Distribution and Expression in Lungs of Spontaneously Breathing Rodents. Exp. Lung Res. 25, 317–333. doi:10.1080/019021499270222
Weiss D. J., Strandjord T. P., Liggitt D., Clark J. G. (1999b). Perflubron Enhances Adenovirus-Mediated Gene Expression in Lungs of Transgenic Mice with Chronic Alveolar Filling. Hum. Gene Ther. 10, 2287–2293. doi:10.1089/10430349950016933
Wessler E. P., Iltis R., Clark L. C. (1977). The Solubility of Oxygen in Highly Fluorinated Liquids. J. Fluorine Chem. 9, 137. doi:10.1080/00091383.1977.10569184
Wilcox D. T., Glick P. L., Karamanokian H. L., Leach C., Morin F. C., Fuhrman B. P. (1994). Perfluorocarbon Associated Gas Exchange (PAGE) and Nitric Oxide in the Lamb with Congenital Diaphragmatic Hernia Model. Pediatr. Res. 35, A260.
Winternitz M. C., Smith G. H. (1920). Preliminary Studies in Intratracheal Therapy. New Haven, CT: Yale University Press, 144–160.
Witt W. P., Weiss A. J., Elixhauser A. (2012). Overview of Hospital Stays for Children in the United States, 2012. Statistical Brief #187. Healthcare Cost and Utilization Project (HCUP) Statistical Briefs [online]. Agency for Healthcare Research and Quality (US). 2006-2014 Dec. Available at: http://www.hcup-us.ahrq.gov/reports/statbriefs/sb187-Hospital-Stays-Children-2012.pdf (Accessed February 5, 2022).
Wolfson M. R., Enkhbaatar P., Fukuda S., Nelson C. L., Williams R. O., Surasarang S. H., et al. (2020). Perfluorochemical-facilitated Plasminogen Activator Delivery to the Airways: A Novel Treatment for Inhalational Smoke-Induced Acute Lung Injury. Clin. Transl. Med. 10, 258–274. doi:10.1002/ctm2.26
Wolfson M. R., Greenspan J. S., Deoras K. S., Rubenstein S. D., Shaffer T. H. (1992). Comparison of Gas and Liquid Ventilation: Clinical, Physiological, and Histological Correlates. J. Appl. Physiol. 72, 1024–1031. doi:10.1152/jappl.1992.72.3.1024
Wolfson M. R., Greenspan J. S., Shaffer T. H. (1996). Pulmonary Administration of Vasoactive Substances by Perfluorochemical Ventilation. Pediatrics 97, 449–455. doi:10.1542/peds.97.4.449
Wolfson M. R., Kechner N. E., Roache R. F., DeChadarevian J.-P., Friss H. E., Rubenstein S. D., et al. (1998). Perfluorochemical rescue after Surfactant Treatment: Effect of Perflubron Dose and Ventilatory Frequency. J. Appl. Physiol. 84, 624–640. doi:10.1152/jappl.1998.84.2.624
Wolfson M. R., Tran N., Bhutani V. K., Shaffer T. H. (1988). A New Experimental Approach for the Study of Cardiopulmonary Physiology during Early Development. J. Appl. Physiol. 65, 1436–1443. doi:10.1152/jappl.1988.65.3.1436
Keywords: drug delivery, gene delivery, partial liquid ventilation, perfluorochemicals, pulmonary
Citation: Alapati D and Shaffer TH (2022) Administration of Drugs/Gene Products to the Respiratory System: A Historical Perspective of the Use of Inert Liquids. Front. Physiol. 13:871893. doi: 10.3389/fphys.2022.871893
Received: 08 February 2022; Accepted: 31 March 2022;
Published: 10 May 2022.
Edited by:
Andrew John Halayko, University of Manitoba, CanadaReviewed by:
Jesus Perez-Gil, Complutense University of Madrid, SpainIan Michael Thornell, The University of Iowa, United States
Copyright © 2022 Alapati and Shaffer. This is an open-access article distributed under the terms of the Creative Commons Attribution License (CC BY). The use, distribution or reproduction in other forums is permitted, provided the original author(s) and the copyright owner(s) are credited and that the original publication in this journal is cited, in accordance with accepted academic practice. No use, distribution or reproduction is permitted which does not comply with these terms.
*Correspondence: Deepthi Alapati, ZGVlcHRoaS5hbGFwYXRpQG5lbW91cnMub3Jn; Thomas H. Shaffer, dGhvbWFzLnNoYWZmZXJAbmVtb3Vycy5vcmc=