- Guangdong Provincial Key Laboratory of Autophagy and Major Chronic Non-communicable Diseases, Key Laboratory of Prevention and Management of Chronic Kidney Disease of Zhanjiang City, Institute of Nephrology, Affiliated Hospital of Guangdong Medical University, Zhanjiang, Guangdong, China
Peritoneal dialysis (PD) is a widely accepted renal replacement therapy for patients with end-stage renal disease (ESRD). Morphological and functional changes occur in the peritoneal membranes (PMs) of patients undergoing long-term PD. Peritoneal fibrosis (PF) is a common PD-related complication that ultimately leads to PM injury and peritoneal ultrafiltration failure. Autophagy is a cellular process of “self-eating” wherein damaged organelles, protein aggregates, and pathogenic microbes are degraded to maintain intracellular environment homeostasis and cell survival. Growing evidence shows that autophagy is involved in fibrosis progression, including renal fibrosis and hepatic fibrosis, in various organs. Multiple risk factors, including high-glucose peritoneal dialysis solution (HGPDS), stimulate the activation of autophagy, which participates in PF progression, in human peritoneal mesothelial cells (HPMCs). Nevertheless, the underlying roles and mechanisms of autophagy in PF progression remain unclear. In this review, we discuss the key roles and potential mechanisms of autophagy in PF to offer novel perspectives on future therapy strategies for PF and their limitations.
1 Introduction
To date, more than 2 million people suffer from end-stage renal disease (ESRD), and this prevalence continues to increase year by year, leading to a significant economic burden (Howell et al., 2019; Masola et al., 2022). More than 272,000 patients, approximately 11% of the dialysis population, undergo peritoneal dialysis (PD) worldwide (Balzer, 2020). PD is a common renal replacement therapy for patients with ESRD. Owing to advantages such as simple operation, protection of residual renal function, and lower risk of cross-infection, PD offers a higher quality of life and more cost savings than does hemodialysis for patients with ESRD (Mehrotra et al., 2011; Yeates et al., 2012). During the COVID-19 pandemic, the importance of PD was revealed, especially in children or patients from low-income areas. The peritoneum is the first barrier preventing microbial invasion during the peritoneal fluid exchange. Owing to their large surface and dense vascularization, peritoneal membranes (PMs) can facilitate high solute and water transport to the intraperitoneal region and thus are a natural choice for membrane filtration and removal of excess water and uremic and other solutes (Balzer, 2020).
In patients undergoing long-term PD, the peritoneum is repeatedly exposed to a non-physiological environment with high glucose, high permeability, and low pH. Over time, it develops inflammation, fibrosis, and other peritoneal complications, leading to morphological and functional changes in the PMs, resulting in PM injury and ultrafiltration failure (UF) (Davies et al., 1996; Smit et al., 2004; Masola et al., 2022). UF gradually declines within 2–4 years after the initiation of PD (Davies et al., 1996; Smit et al., 2004; Hayat et al., 2021). Initially, the primary causes of PD failure are peritonitis and catheter-related complications (Li et al., 2016a; Hayat et al., 2021). However, the bio-incompatibility of peritoneal dialysis solution (PDS) in the long term, accompanied by impairment of peritoneal integrity and functionality, becomes the main concern (Bajo et al., 2017). In 50%–80% of the patients undergoing long-term PD, the primary signs of peritoneal fibrosis (PF) are detected within 1–2 years (Strippoli et al., 2016). Inhibition of fibrosis and inflammation can prolong the life of PD therapy. Although many mechanisms have been proposed, effective interventions and treatment strategies for PF are lacking (Zhang et al., 2018a). Thus, there is an urgent need to explore the mechanisms underlying PF progression and develop effective prevention strategies.
Autophagy, a process that degrades single proteins and large organelles, is an integral part of all eukaryotic cell types. It is considered a true health modifier (Morishita and Mizushima, 2019; Klionsky et al., 2021) and serves as the primary regulator of cellular and tissue adaptation to various endogenous and exogenous pressures (Morishita and Mizushima, 2019). Autophagy pathways are physiologically correlated, even under basal and non-stressful conditions. In multiple experimental models, pharmacological and genetic interventions that impair autophagy were found to promote or exacerbate diseases (Klionsky et al., 2021). Autophagy plays a critical role in the progression of fibrosis in various organs, such as the kidneys (Dai et al., 2022), liver (Sun et al., 2021), and lungs (Araya et al., 2013). However, the causal connection between autophagy and PF and the pathophysiological mechanisms remains unclear.
Currently, many researches have proved that autophagy is involved in the process of PD and in PD-related complications, and novel interventions targeting autophagy may have clinical transformability. In this review, we highlight the current knowledge about the key roles and potential mechanisms of autophagy in PF. Furthermore, we discuss novel underlying mechanisms of autophagy modulators, which may contribute to developing new clinical therapies for PF.
2 Effect of autophagy in peritoneal fibrosis
PF is one of the most common complications in patients undergoing long-term PD and one of the leading causes of PD failure (Krediet and Struijk, 2013; Krediet, 2018). Epithelial-mesenchymal transition (EMT) of peritoneal mesothelial cells (PMCs), also called mesothelial-mesenchymal transition (MMT), is wildly accepted as the main cause of PF (Strippoli et al., 2016; Zhou et al., 2016; Zhang et al., 2018a; Hayat et al., 2021). Furthermore, in submesothelial areas, transformed mesothelial cells can generate extracellular matrix (ECM) and cause fibrosis, demonstrating their invasive capacity (Yáñez-Mó et al., 2003). Moreover, during PF and MMT, the key fibrogenic molecular machinery, mainly transforming growth factor-β (TGF-β)/Smad-dependent signaling (Margetts et al., 2005; Patel et al., 2010; Loureiro et al., 2011; Yoshizawa et al., 2015) and TGF-β/Smad-independent signaling (Matsuo et al., 2006; Patel et al., 2010; Liu et al., 2012; Fan et al., 2013; Kitterer et al., 2015; Wynn and Vannella, 2016; Padwal et al., 2018), triggers the transcription factors that act on the promoter regions of the cell matrix genes to activate their transcription via specific downstream intracellular signaling.
PF is commonly observed in patients with mild fibrosis undergoing PD (Di Paolo and Sacchi, 2000). However, encapsulating peritoneal sclerosis (EPS), which has low morbidity and high mortality, is an uncommon form of PF (Strippoli et al., 2016). It is a life-threatening complication that may progress even in patients who have discontinued PD.
2.1 Effect of autophagy in peritoneal fibrosis
As a complex and necessary metabolic process, autophagy exists in most mammalian cells. Autophagy is closely related to fibrosis in multiple organs, such as the kidney (Zhao et al., 2019), heart (Zhao et al., 2018), lungs (Cabrera et al., 2015), and liver (Meng et al., 2018). Additionally, high-glucose (HG) levels can modulate autophagy in multiple disease models (Wei et al., 2014; Chang et al., 2015; Lu et al., 2015). However, the potential effects of autophagy during the development of PF remain ambiguous, as some studies suggest that autophagy reduces PF, whereas others show the opposite trend (Figure 1).
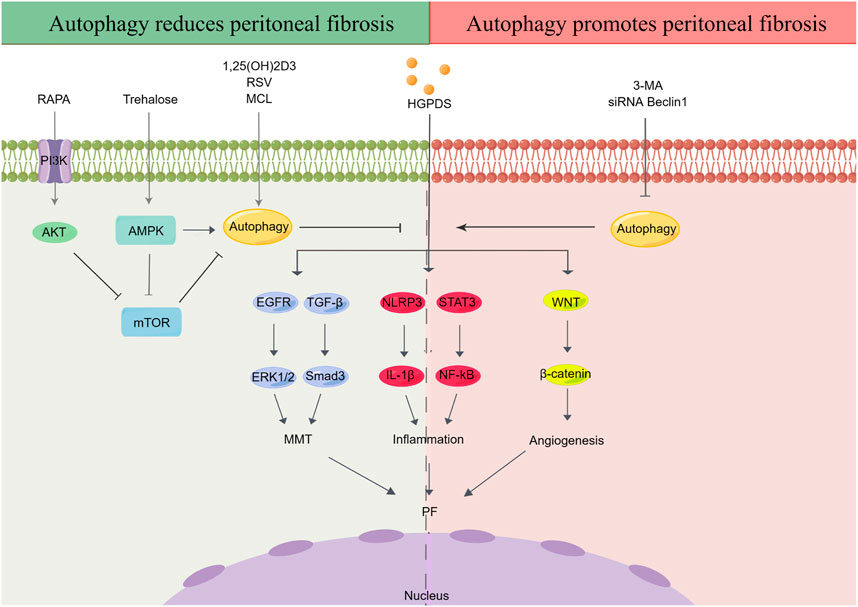
FIGURE 1. Effect of autophagy in peritoneal fibrosis. HGPDS contributes to MMT, inflammation and angiogenesis in peritoneal mesothelial cells, eventually cause PF. Some studies suggest that activation of autophagy reduces PF, whereas some evidences demonstrate that autophagy promotes PF. RAPA: rapamycin, RSV: resveratrol, MCL: micheliolide, HGPDS: high-glucose peritoneal dialysis solution, 3-MA: 3-methyladenine, PI3K: phosphatidylinositide 3-kinases, AMPK: AMP-activated protein kinase, mTOR: mammalian target of rapamycin, EGFR: epidermal growth factor receptor, ERK1/2: extracellular signal-regulated kinase 1/2, TGF-β: transforming growth factor-β, NLRP3: nod-like receptor 3, IL-1β: interleukin-1β, STAT3: signal transducer and activator of transcription 3, NF-κB: nuclear factor kappa-B, MMT: mesothelial-mesenchymal transition.
On the one hand, autophagy is believed to contribute to PF progression. First, autophagy inhibition was observed in the peritoneum of an HG-induced peritoneal injury mouse model (Li et al., 2019a). Simultaneously, studies suggest that blocking autophagy with 3-methyladenine (3-MA) and Beclin-1 or ATG5 small interfering RNA (siRNA) not only enhances the continuous activation of the inflammatory factor nod-like receptor 3 (NLRP3)/interleukin-1β (IL-1β) induced by the stimulation of long-term HGPDS but also promotes MMT progression in the PMs of patients undergoing PD (Li et al., 2017). Moreover, as an autophagy inducer, trehalose has been shown to ameliorate PF by promoting the generation of autophagosomes and suppressing MMT in PMCs (Mayer et al., 2016; Sakai et al., 2017; Miyake et al., 2020) through activating AMP-activated protein kinase (AMPK) pathway, phosphorylating unc-52-like kinase-1 (ULK1) at Ser317, and AMPK/mammalian target of rapamycin (mTOR)pathway, dephosphorylating the inhibitory site of ULK1 (Ser757) (DeBosch et al., 2016; Mayer et al., 2016). Under HG conditions, autophagy inhibition has been observed in peritoneal mesothelial cells and mouse models, with reduction in the expression of light chain 3 (LC3)-II, p62, and Beclin-1; contrastingly, 1,25(OH)2D3 alleviates autophagy inhibition in PMCs through the mTOR pathway (Yang et al., 2017). Increasing evidence has shown that regulation of autophagy through the phosphatidylinositol 3-kinase (PI3K)/AKT/mTOR signaling contributes to the occurrence and pathological progression of diabetic nephropathy and PF (Li et al., 2016b; Lu et al., 2019; Yang et al., 2020a; Jia et al., 2022). In this research, treatment with the mTOR inhibitor rapamycin (RAPA) and PI3K inhibitor LY294002 activated autophagy and alleviated PF in vivo and in vitro, thereby upregulating E-cadherin and zonula occludens-1 (ZO-1) and downregulating alpha-smooth muscle actin (α-SMA) and ferroptosis suppressor protein 1 (Jia et al., 2022). In addition, RAPA, which can induce autophagy by inhibiting MTORC1 expression, relieved peritoneal thickening, angiogenesis, lymphangiogenesis, MMT, and endothelial-to-mesenchymal transition (Endo-MT) and improved UF in a mouse PD model (González-Mateo et al., 2015). Finally, micheliolide (MCL), a natural guaianolide sesquiterpene lactone, which promoted autophagy in db/db mice at a low dose, inhibited TGF-β1-induced ECM accumulation by activating autophagy in PF mouse models and the HPMC cell line (HMrSV5) (Zhong et al., 2018; Li et al., 2019b).
Limited evidence has demonstrated that autophagy promotes PF. Apigenin, a dietary flavonoid synthesized in multiple plants, effectively inhibits PF in a HG-induced mouse model, accompanied by a corresponding alteration of autophagy biomarkers (Zhang et al., 2018a). Moreover, significant activation of autophagy was observed in the PMCs of both two PF rat models induced by PDS and chlorhexidine gluconate (CG) (Shi et al., 2021). However, in above PF rat models and cultured HPMCs, treatment with 3-MA effectively delayed MMT and prevented PF by TGF-β/Smad3 signaling pathway and alleviated peritoneal angiogenesis by downregulation of β-catenin signaling pathway (Shi et al., 2021). Autophagy inhibition significantly reduced MMT, fibrosis, and apoptosis in HPMCs (Wu et al., 2018)(Table 1).
Similar dual characteristics of autophagy have been demonstrated in renal (Liang et al., 2022) and hepatic fibrosis (Sun et al., 2021; Hou et al., 2022). The effect of autophagy activation in the process of PF is complex and multifactorial, and its molecular impact may vary from specific targets in autophagy regulation. Furthermore, different experimental PF models, cell categories, drugs, doses and timing of autophagy inducers, and the diversified molecular mechanisms could result in varying effects of autophagy on PF. It is generally accepted that autophagy functions differently in diverse diseases and at different stages of the same disease. Over-activation of autophagy promotes fibrosis of multiple organs, whereas inhibition of autophagy aggravates cell damage and promotes the occurrence of PF. Nevertheless, further studies are needed to explore whether the different factors mediating autophagy play different roles at different stages of PF. Autophagy activation is usually considered effective in acute pathological damage to maintain cell homeostasis. In contrast, sustained autophagy induced by some chronic diseases may be harmful, causing apoptosis by damaging important organelles.
Furthermore, mitophagy may be involved in PF. With the production of reactive oxygen species (ROS), mitochondrial DNA is more prone to mutations than nuclear DNA, which makes mitochondria more susceptible to damage. Thus, maintaining an intact population of mitochondria via quality control mechanisms, including mitophagy, is essential for cell survival under pathological stress conditions (Lemasters, 2005; Youle and Narendra, 2011). Many studies have shown that HPMCs stimulated with HGPDS can undergo oxidative stress, mitochondrial DNA damage, and even apoptosis (Hung et al., 2014; Ramil-Gómez et al., 2021). However, insufficient or excessive autophagy of mitochondria results in the accumulation of damaged mitochondria, which eventually leads to the disruption of mitochondrial quality control and bioenergy metabolism and even cell death (Kubli and Gustafsson, 2012; Namba et al., 2014; Kawakami et al., 2015). The mitochondrial dysfunction subsequently participates in the occurrence and development of fibrosis (Li et al., 2020). Under long-term hypertonic and HGPDS, HPMCs exhibit progressive PF, and MMT of HPMCs caused by mitochondrial dysfunction is one of the possible mechanisms (Shin et al., 2017; Ramil-Gómez et al., 2021). Furthermore, metformin and other AMPK inhibitors could delay the phenotypic transition of PMCs and PF via modulating oxidative stress, suggesting that AMPK could be a novel therapeutic target to prevent PF. However, there is no direct evidence that mitophagy can inhibit the progression of PF, which requires further experimental proof (Shin et al., 2017).
2.2 Effect of autophagy in encapsulating peritoneal sclerosis
Autophagy may have a potential connection with EPS, a rare but severe complication of patients undergoing PD (Pepereke et al., 2022). EPS, a clinical syndrome characterized by the formation of a fibrous cocoon in the peritoneal cavity, has a high mortality rate of 42% 1 year after diagnosis (Brown et al., 2009). Although there are few treatment methods, and their efficacy is poor. Anti-inflammatory therapy with steroids and antifibrotic therapy with tamoxifen are most commonly used for treating EPS (Lo and Kawanishi, 2009; Garosi et al., 2013; Pepereke et al., 2022).
Recently, mTOR inhibitors (everolimus and sirolimus), widely used as immunosuppressive/antiproliferative agents in renal transplantation and oncology field, have been suggested as a novel treatment for EPS. Two in vivo experiments indicated that oral mTOR inhibitors (everolimus and sirolimus) significantly reduced the progression of PF, including peritoneal thickness, vascularity, and fibrosis score, in a rat model induced by CG (Duman et al., 2008; Ceri et al., 2012). Furthermore, mTOR inhibitors may protect the peritoneum from PF by inhibiting MMT, with an upregulation of the E-cadherin and downregulation of α-SMA (Aguilera et al., 2005). In addition, multiple clinical studies have proposed the therapeutic role of mTOR inhibitors in the management of EPS owing to the antifibrotic and anti-angiogenesis effects. Due to the rarity of EPS, most studies come from case reports rather than systematic research. In particular, Ghadimi et al. reviewed the effects of mTOR inhibitors (everolimus and sirolimus) in the therapy of EPS using 13 case reports (Ghadimi et al., 2016). In one-third of the patients with EPS, mTOR inhibitors were found to allay EPS and reduce mortality. Moreover, 5-aminoimidazole-4-carboxyamide ribonucleoside (AICAR), a classical AMPK agonist that reduces inflammation, fibrosis, and cellular ROS injury and promotes autophagy, inhibits postoperative adhesion formation in vivo and in vitro by inhibiting inflammation and oxidative stress response and accelerating peritoneal mesothelial cell repair (Wu et al., 2022).
The therapeutic effects and mechanism of autophagy against EPS have not been fully confirmed, and more evidence is needed.
3 Effect of autophagy in PD-related peritonitis
PF encompasses two synergistic actions: the fibrosis process itself and inflammation. These two processes are usually bidirectional, with one triggering another (Yáñez-Mó et al., 2003; Loureiro et al., 2011; Yoshizawa et al., 2015; Zhou et al., 2016). PF often progresses after acute and chronic PD-related inflammatory (Kobayashi et al., 2018). Thus, the effects of autophagy on PD-related peritonitis must be elucidated (Figure 2).
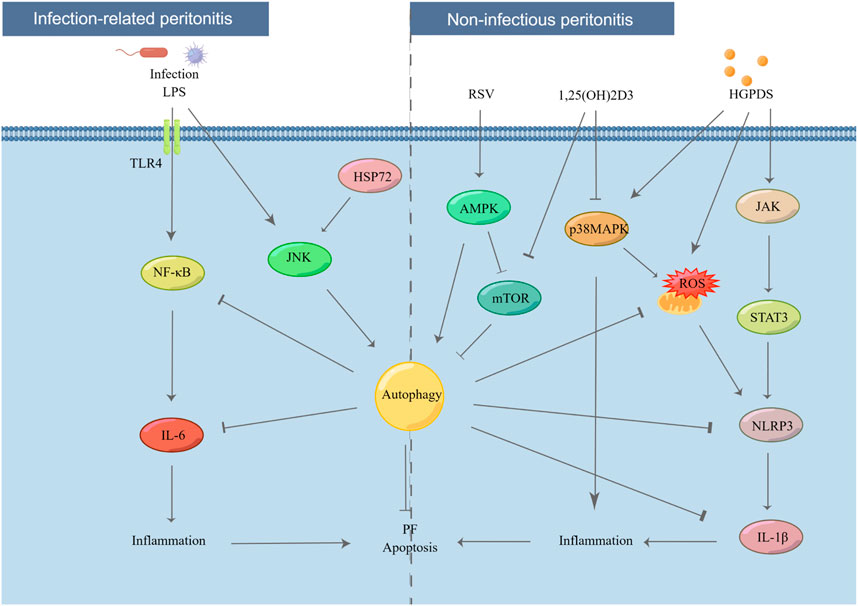
FIGURE 2. Effect of autophagy in PD-related peritonitis. Infectious factors lead to infection-related peritonitis via NF-κB/IL-6 pathway. In addition, under long-term stimulation of HGPDS, HPMCs suffer from non-infectious peritonitis by NLRP3/IL-1β pathway. However, these processes are blocked by the activation of autophagy. LPS: lipopolysaccharide, TLR4: toll like receptor 4, IL-6: interleukin-6, JNK: c-Jun N-terminal kinase, HSP72: heat shock protein 72, p38 MAPK: p38 mitogen activated protein kinases, JAK: Janus kinase.
Recently, PD-related peritonitis, the common and serious complication of PD, has attracted increasing public attention. More than 40% of patients experience UF owing to PD-related peritonitis after 3 years of PD therapy (Baroni et al., 2012). Mild systemic inflammation is usually observed in patients with chronic kidney disease (Stenvinkel and Alvestrand, 2002). PD-related peritonitis can be primarily classified into infection-related and non-infectious peritonitis.
On the one hand, a single microorganism is the main cause of the infection-related peritonitis, with half of these infections come from gram-positive bacteria (Peterson et al., 1987; Golper et al., 1996; Gokal, 2002; Yung and Chan, 2012). According to the comprehensive recommendations on the prevention and treatment of PD-related peritonitis by the International Society for PD, appropriate and adequate antimicrobial treatment is still the most important and main therapy for infection-related peritonitis (Szeto and Li, 2019). However, despite apparent clinical remission of peritonitis, the inflammatory factors and fibrotic mediators persistently increased in the peritoneal cavity cause continuous stimulation to PMCs (Yung and Chan, 2012). In addition, evidence indicates that pro-inflammatory factors are continuously released for at least 6 weeks even after the clinical resolution of PD-related peritonitis (Lai et al., 2000; Lai et al., 2007). On the other hand, when repeatedly exposed to a non-physiological condition of HGPDS, PMCs raise an inflammatory response in the peritoneal cavity, which causes non-infectious peritonitis (Lai and Leung, 2010). Research has established that non-infectious peritonitis also results in PF, neoangiogenesis, and UF (Lai et al., 2000; Myers, 2004; De Vriese, 2005; Zareie et al., 2005; De Vriese et al., 2006; Leung et al., 2006; Aroeira et al., 2007; Guo et al., 2007; Lai et al., 2007; Leung et al., 2009).
3.1 Effect of autophagy in infection-related peritonitis
Several studies have suggested that autophagy has a strong relationship with both infection-related and non-infectious peritonitis, suggesting that autophagy might act as a novel therapeutic target for patients with PD-related peritonitis.
HPMCs are considered as the first barrier to prevent against invading pathogens in patients on PD. Autophagy plays a key role in innate immunity and cell homeostasis (Gutierrez et al., 2004; Nakagawa et al., 2004; Thurston et al., 2009; Sir et al., 2010; Anand et al., 2011). After bacterial invasion, autophagy is activated, degrading bacteria via the autophagy-lysosomal pathway, thus protecting the host against pathogen colonization (Ligeon et al., 2011; Choi et al., 2013). Autophagy activation plays a critical role in the recovery of mesothelium following acute inflammation in a rat model by removing damaged cytoplasmic organelles (Balogh et al., 2015). Lipopolysaccharide (LPS), the active constituent of endotoxins in gram-negative bacteria, is a potential inducer of autophagy in PMCs and macrophages (Xu et al., 2007; Doyle et al., 2011; Meng et al., 2012). In particular, autophagy is induced by LPS in HMrSV5 in a dose- and time-dependent manner, as demonstrated by an increase in the expression of Beclin-1 and LC3-II, number of autophagic vacuoles, and intracellular bactericidal activity (Li et al., 2011). However, these processes in the peritoneal cavity are blocked by autophagy inhibitors, such as 3-MA, Beclin-1 siRNA, or wortmannin, via TLR4 signaling (Wang et al., 2013). A study suggested that heat shock protein 72 (HSP72) protects peritoneal mesothelial cells against LPS-induced mesothelial cell injury by activating c-Jun N-terminal kinase (JNK)-dependent autophagy and partially inhibiting apoptosis (Li et al., 2011). Furthermore, peroxisome proliferator-activated receptor-γ (PPAR-γ) may act as a potential therapeutic target for peritonitis (Zhang et al., 2018b). With the stimulation of LPS, upregulating the expression of nuclear factor kappa-B (NF-κB) activity, ICAM-1, and interleukin (IL)-6 was observed in rat PD models (Zhang et al., 2015). Although fungal peritonitis is rare compared to bacterial peritonitis, accounting for only 1%–12% of PD-related peritonitis (Chavada et al., 2011; Tobudic et al., 2013; Nadeau-Fredette and Bargman, 2015), it has higher morbidity and catheter-related mortality (Levallois et al., 2012; Li et al., 2016a; Giacobino et al., 2016; Xu et al., 2022). Candida species are the most common pathogens involved in most fungal peritonitis cases, with approximately 70%–90% frequency (Kazancioglu et al., 2010; Nadeau-Fredette and Bargman, 2015). In addition, scientific evidence suggests that caspase recruitment domain-containing protein 9 (Card9), an adapter protein, protects against fungal peritonitis by regulating the mucosa-associated lymphoid tissue lymphoma translocation 1 (Malt1)-mediated activation of autophagy in macrophages (Xu et al., 2022).
3.2 Effect of autophagy in non-infectious peritonitis
Autophagy also contributes to non-infectious peritonitis. PMs develop chronic inflammation during long-term PD as a result of bioincompatible PDS with low pH, high concentrations of glucose, and high osmolality (Hayat et al., 2021). IL-6 is a crucial inflammatory factor in patients with PD, and repeated inflammatory activation can lead to fibrotic injury in the peritoneum via the JAK/Signal Transducer And Activator Of Transcription 3 (STAT3) signaling pathway (Fielding et al., 2014; Xiao et al., 2017; Yang et al., 2020b; Yang et al., 2021). Moreover, evidence indicates that the upregulation of IL-17 family cytokines protects the host from infections and chronic inflammation during PD-associated peritoneal injury (Schroder and Tschopp, 2010; Helmke et al., 2021). Long-term continuous exposure to HG PDS leads to mitochondrial ROS production in HPMCs, which subsequently triggers NLRP3 inflammasome activation and IL-1β secretion (Witowski et al., 2001; Yáñez-Mó et al., 2003; Schroder and Tschopp, 2010; Li et al., 2017). However, ROS can induce autophagy, a self-clearance process that reduces oxidative damage via engulfing and degrading the oxidized substances (Wu et al., 2016; Li et al., 2021). Timely initiation of autophagy could block the activation of NLRP3-IL-1β signaling, providing a theoretical basis for a potential therapeutic strategy to suspend inflammation and PF (Li et al., 2017). Resveratrol-induced mitophagy/autophagy by the AMPK pathway may protect against ROS-NLRP3-mediated inflammatory injury in HPMCs (Wu et al., 2016). Research has conclusively demonstrated that HG treatment leads to apoptosis, ROS production, inflammatory activation, and MMT in PMCs via the MAPK/P38 signaling pathway, while 1,25(OH)2D3 blocks the above-mentioned changes (Zhang et al., 2012; Yang et al., 2016). A recent study found that 1,25(OH)2D3 plays a protective role in HG-induced peritoneal injury by increasing autophagy, possibly via the mTOR signaling pathway (Yang et al., 2017). However, opposing views exist. The blockade of autophagy with 3-MA considerably reduced the inflammatory response and macrophage infiltration via the STAT3/NF-κB pathway in both PDS and CG-induced rat models (Shi et al., 2021).
4 Conclusion and perspective
In the last decades, many researchers have suggested a strong relationship between the process of PF and autophagy, but the roles and potential mechanisms are not completely clear, probably due to the versatility of autophagy.
Owing to the continuous research on the role of autophagy in the prevention and treatment of diseases, some drugs targeting autophagy have been used for the treatment of cancer, infection-related diseases, and neuropathy. Meanwhile, drugs targeting autophagy, such as mTOR inhibitors (evolutionus and sirolimus), have also been used in the treatment of EPS. However, most of the clinical data on EPS comes from case reports, while the registries and treatments of EPS provide low-quality evidence owing to its rarity, making it difficult to carry out large-scale systematic research on the role of autophagy in EPS. Histone deacetylase (HDAC) inhibitors or lysosomal acidification inhibitors (such as chloroquine and hydroxychloroquine) may also be used for the regulation of autophagy and are often used in immunotherapy in kidney diseases. However, there is less clinical evidence in PF or peritonitis.
There are several potential aspects of autophagy research in PF. First, the specific mechanism of autophagy in PF and peritonitis needs to be clarified. Selective autophagy, such as mitophagy and macrophagy, may also be involved in PF. Additionally, potential autophagy markers are also attractive. For example, owing to the objective advantages such as simple operation, small invasion, and fast recovery, the ‘pull technology’ for PD catalyst removal is more popular among doctors and patients than the traditional open surgery, which makes it hard to obtain tissue samples. With the simpler, more economical, easier to obtain, and repeatable advantages, peritoneal dialysis fluid, rather than peritoneal tissue, can continuously and remarkably detect autophagy activity and peritoneal fibrosis. Additionally, the application of intervention drugs is equally important. Some autophagy activators can be added to peritoneal dialysis fluid for preventing peritoneal complications. Effective measures should be implemented to promote the transformation of experimental data into clinical practice. Autophagy modulators that can be used to develop novel clinical therapeutic strategies for allaying PF need to be developed to reveal the mechanism of autophagy pathways in PF.
Author contributions
HS, and JY designed and wrote the manuscript. RZ, NA, XC and HY revised the manuscript. HS and CY designed the figures. HS, NA, CY, C-wY, and HL obtained funding. All authors contributed to the article and approved the submitted version
Funding
This work was supported by the Funds for the National Natural Science Foundation of China (grant numbers 81700627, 81670654, and 81974095), National Clinical Key Specialty Construction Project (Institute of Nephrology, Affiliated Hospital of Guangdong Medical University), Science and Technology Innovation Strategy of Guangdong Province (grant numbers 2019A1515010678 and 2018A030313231), Science and Technology Planning Project of Zhanjiang City (grant numbers 2021A05067 and 2021A05083), and Science Foundation of Guangdong Medical University (grant number GDMUM2020009).
Acknowledgments
We thank Editage (www.editage.cn) for English language editing and Figdraw (www.figdraw.com) for figure drawing.
Conflict of interest
The authors declare that the research was conducted in the absence of any commercial or financial relationships that could be construed as a potential conflict of interest.
Publisher’s note
All claims expressed in this article are solely those of the authors and do not necessarily represent those of their affiliated organizations, or those of the publisher, the editors and the reviewers. Any product that may be evaluated in this article, or claim that may be made by its manufacturer, is not guaranteed or endorsed by the publisher.
References
Aguilera, A., Aroeira, L. S., Ramirez-Huesca, M., Perez-Lozano, M. L., Cirugeda, A., Bajo, M. A., et al. (2005). Effects of rapamycin on the epithelial-to-mesenchymal transition of human peritoneal mesothelial cells. Int. J. Artif. Organs 28, 164–169. doi:10.1177/039139880502800213
Anand, P. K., Tait, S. W., Lamkanfi, M., Amer, A. O., Nunez, G., Pagès, G., et al. (2011). TLR2 and RIP2 pathways mediate autophagy of Listeria monocytogenes via extracellular signal-regulated kinase (ERK) activation. J. Biol. Chem. 286, 42981–42991. doi:10.1074/jbc.M111.310599
Araya, J., Kojima, J., Takasaka, N., Ito, S., Fujii, S., Hara, H., et al. (2013). Insufficient autophagy in idiopathic pulmonary fibrosis. Am. J. Physiol. Lung Cell Mol. Physiol. 304, L56–L69. doi:10.1152/ajplung.00213.2012
Aroeira, L. S., Aguilera, A., Sánchez-Tomero, J. A., Bajo, M. A., Del, P. G., Jiménez-Heffernan, J. A., et al. (2007). Epithelial to mesenchymal transition and peritoneal membrane failure in peritoneal dialysis patients: Pathologic significance and potential therapeutic interventions. J. Am. Soc. Nephrol. 18, 2004–2013. doi:10.1681/ASN.2006111292
Bajo, M. A., Del, P. G., and Teitelbaum, I. (2017). Peritoneal membrane preservation. Semin. Nephrol. 37, 77–92. doi:10.1016/j.semnephrol.2016.10.009
Balogh, P., Szabó, A., Likó, I., and Patócs, A. (2015). Autophagy may contribute to the recovery of rat mesothelium following acute inflammation in vivo. Cell Tissue Res. 362, 127–137. doi:10.1007/s00441-015-2188-6
Balzer, M. S. (2020). Molecular pathways in peritoneal fibrosis. Cell Signal 75, 109778. doi:10.1016/j.cellsig.2020.109778
Baroni, G., Schuinski, A., de Moraes, T. P., Meyer, F., and Pecoits-Filho, R. (2012). Inflammation and the peritoneal membrane: Causes and impact on structure and function during peritoneal dialysis. Mediat. Inflamm., 2012, 912595, doi:10.1155/2012/912595
Brown, M. C., Simpson, K., Kerssens, J. J., and Mactier, R. A. (2009). Encapsulating peritoneal sclerosis in the new millennium: A national cohort study. Clin. J. Am. Soc. Nephrol. 4, 1222–1229. doi:10.2215/CJN.01260209
Cabrera, S., Maciel, M., Herrera, I., Nava, T., Vergara, F., Gaxiola, M., et al. (2015). Essential role for the ATG4B protease and autophagy in bleomycin-induced pulmonary fibrosis. Autophagy 11, 670–684. doi:10.1080/15548627.2015.1034409
Ceri, M., Unverdi, S., Dogan, M., Unverdi, H., Karaca, G., Kocak, G., et al. (2012). Effect of sirolimus on the regression of peritoneal sclerosis in an experimental rat model. Int. Urol. Nephrol. 44, 977–982. doi:10.1007/s11255-012-0167-3
Chang, T. C., Hsu, M. F., and Wu, K. K. (2015). High glucose induces bone marrow-derived mesenchymal stem cell senescence by upregulating autophagy. PLoS One 10, e0126537. doi:10.1371/journal.pone.0126537
Chavada, R., Kok, J., van Hal, S., and Chen, S. C. (2011). Seeking clarity within cloudy effluents: Differentiating fungal from bacterial peritonitis in peritoneal dialysis patients. PLoS One 6, e28247. doi:10.1371/journal.pone.0028247
Choi, A. M., Ryter, S. W., and Levine, B. (2013). Autophagy in human health and disease. N. Engl. J. Med. 368, 1845–1846. doi:10.1056/NEJMc1303158
Dai, R., Zhang, L., Jin, H., Wang, D., Cheng, M., Sang, T., et al. (2022). Autophagy in renal fibrosis: Protection or promotion? Front. Pharmacol. 13, 963920. doi:10.3389/fphar.2022.963920
Davies, S. J., Bryan, J., Phillips, L., and Russell, G. I. (1996). Longitudinal changes in peritoneal kinetics: The effects of peritoneal dialysis and peritonitis. Nephrol. Dial. Transpl. 11, 498–506. doi:10.1093/oxfordjournals.ndt.a027318
De Vriese, A. S. (2005). The john F. Maher recipient lecture 2004: Rage in the peritoneum. Perit. Dial. Int. 25, 8–11. doi:10.1177/089686080502500102
De Vriese, A. S., Tilton, R. G., Mortier, S., and Lameire, N. H. (2006). Myofibroblast transdifferentiation of mesothelial cells is mediated by RAGE and contributes to peritoneal fibrosis in uraemia. Nephrol. Dial. Transpl. 21, 2549–2555. doi:10.1093/ndt/gfl271
DeBosch, B. J., Heitmeier, M. R., Mayer, A. L., Higgins, C. B., Crowley, J. R., Kraft, T. E., et al. (2016). Trehalose inhibits solute carrier 2A (SLC2A) proteins to induce autophagy and prevent hepatic steatosis. Sci. Signal 9, a21. doi:10.1126/scisignal.aac5472
Di Paolo, N., and Sacchi, G. (2000). Atlas of peritoneal histology. Perit. Dial. Int. 20 (3), S5–S96.
Doyle, A., Zhang, G., Abdel, F. E., Eissa, N. T., and Li, Y. P. (2011). Toll-like receptor 4 mediates lipopolysaccharide-induced muscle catabolism via coordinate activation of ubiquitin-proteasome and autophagy-lysosome pathways. Faseb J. 25, 99–110. doi:10.1096/fj.10-164152
Duman, S., Bozkurt, D., Sipahi, S., Sezak, M., Ozkan, S., Ertilav, M., et al. (2008). Effects of everolimus as an antiproliferative agent on regression of encapsulating peritoneal sclerosis in a rat model. Adv. Perit. Dial. 24, 104–110.
Fan, Y., Mao, R., and Yang, J. (2013). NF-κB and STAT3 signaling pathways collaboratively link inflammation to cancer. Protein Cell 4, 176–185. doi:10.1007/s13238-013-2084-3
Fielding, C. A., Jones, G. W., McLoughlin, R. M., McLeod, L., Hammond, V. J., Uceda, J., et al. (2014). Interleukin-6 signaling drives fibrosis in unresolved inflammation. Immunity 40, 40–50. doi:10.1016/j.immuni.2013.10.022
Garosi, G., Mancianti, N., Corciulo, R., La Milia, V., and Virga, G. (2013). Encapsulating peritoneal sclerosis. J. Nephrol. 26 (21), 177–187. doi:10.5301/JN.2013.11640
Ghadimi, M., Dashti-Khavidaki, S., and Khalili, H. (2016). mTOR inhibitors for management of encapsulating peritoneal sclerosis: a review of literatures. Ren. Fail 38, 1574–1580. doi:10.1080/0886022X.2016.1209026
Giacobino, J., Montelli, A. C., Barretti, P., Bruder-Nascimento, A., Caramori, J. T., Barbosa, L., et al. (2016). Fungal peritonitis in patients undergoing peritoneal dialysis (PD) in Brazil: Molecular identification, biofilm production and antifungal susceptibility of the agents. Med. Mycol. 54, 725–732. doi:10.1093/mmy/myw030
Gokal, R. (2002). Peritoneal dialysis in the 21st century: An analysis of current problems and future developments. J. Am. Soc. Nephrol. 13 (1), S104–S115. doi:10.1681/asn.v13suppl_1s104
Golper, T. A., Brier, M. E., Bunke, M., Schreiber, M. J., Bartlett, D. K., Hamilton, R. W., et al. (1996). Risk factors for peritonitis in long-term peritoneal dialysis: The network 9 peritonitis and catheter survival studies. Academic subcommittee of the steering committee of the network 9 peritonitis and catheter survival studies. Am. J. Kidney Dis. 28, 428–436. doi:10.1016/s0272-6386(96)90502-8
González-Mateo, G. T., Aguirre, A. R., Loureiro, J., Abensur, H., Sandoval, P., Sánchez-Tomero, J. A., et al. (2015). Rapamycin protects from type-I peritoneal membrane failure inhibiting the angiogenesis, lymphangiogenesis, and endo-MT. Biomed. Res. Int. 2015, 989560. doi:10.1155/2015/989560
Guo, H., Leung, J. C., Lam, M. F., Chan, L. Y., Tsang, A. W., Lan, H. Y., et al. (2007). Smad7 transgene attenuates peritoneal fibrosis in uremic rats treated with peritoneal dialysis. J. Am. Soc. Nephrol. 18, 2689–2703. doi:10.1681/ASN.2007010121
Gutierrez, M. G., Master, S. S., Singh, S. B., Taylor, G. A., Colombo, M. I., and Deretic, V. (2004). Autophagy is a defense mechanism inhibiting BCG and Mycobacterium tuberculosis survival in infected macrophages. Cell 119, 753–766. doi:10.1016/j.cell.2004.11.038
Hayat, A., Collins, J., and Saweirs, W. (2021). Study of early complications associated with peritoneal dialysis catheters: An analysis of the New Zealand peritoneal dialysis registry data. Int. Urol. Nephrol. 53, 1705–1711. doi:10.1007/s11255-021-02785-y
Helmke, A., Hüsing, A. M., Gaedcke, S., Brauns, N., Balzer, M. S., Reinhardt, M., et al. (2021). Peritoneal dialysate-range hypertonic glucose promotes T-cell IL-17 production that induces mesothelial inflammation. Eur. J. Immunol. 51, 354–367. doi:10.1002/eji.202048733
Hou, L. S., Zhang, Y. W., Li, H., Wang, W., Huan, M. L., Zhou, S. Y., et al. (2022). The regulatory role and mechanism of autophagy in energy metabolism-related hepatic fibrosis. Pharmacol. Ther. 234, 108117. doi:10.1016/j.pharmthera.2022.108117
Howell, M., Walker, R. C., and Howard, K. (2019). Cost effectiveness of dialysis modalities: A systematic review of economic evaluations. Appl. Health Econ. Health Policy 17, 315–330. doi:10.1007/s40258-018-00455-2
Hung, K. Y., Liu, S. Y., Yang, T. C., Liao, T. L., and Kao, S. H. (2014). High-dialysate-glucose-induced oxidative stress and mitochondrial-mediated apoptosis in human peritoneal mesothelial cells. Oxid. Med. Cell Longev., 2014, 642793, doi:10.1155/2014/642793
Jia, M., Qiu, H., Lin, L., Zhang, S., Li, D., and Jin, D. (2022). Inhibition of PI3K/AKT/mTOR signalling pathway activates autophagy and suppresses peritoneal fibrosis in the process of peritoneal dialysis. Front. Physiol. 13, 778479. doi:10.3389/fphys.2022.778479
Kawakami, T., Gomez, I. G., Ren, S., Hudkins, K., Roach, A., Alpers, C. E., et al. (2015). Deficient autophagy results in mitochondrial dysfunction and FSGS. J. Am. Soc. Nephrol. 26, 1040–1052. doi:10.1681/ASN.2013111202
Kazancioglu, R., Kirikci, G., Albaz, M., Dolgun, R., and Ekiz, S. (2010). Fungal peritonitis among the peritoneal dialysis patients of four Turkish centres. J. Ren. Care 36, 186–190. doi:10.1111/j.1755-6686.2010.00193.x
Kitterer, D., Latus, J., Ulmer, C., Fritz, P., Biegger, D., Ott, G., et al. (2015). Activation of nuclear factor of activated T cells 5 in the peritoneal membrane of uremic patients. Am. J. Physiol. Ren. Physiol. 308, F1247–F1258. doi:10.1152/ajprenal.00617.2014
Klionsky, D. J., Petroni, G., Amaravadi, R. K., Baehrecke, E. H., Ballabio, A., Boya, P., et al. (2021). Autophagy in major human diseases. Embo J. 40, e108863. doi:10.15252/embj.2021108863
Kobayashi, T., Noguchi, T., Saito, K., Shirasaki, F., Kita, H., Nagata, M., et al. (2018). Eosinophilic inflammation in peritoneal fibrosis patients undergoing peritoneal dialysis. Contrib. Nephrol. 196, 1–4. doi:10.1159/000485689
Krediet, R. T., and Struijk, D. G. (2013). Peritoneal changes in patients on long-term peritoneal dialysis. Nat. Rev. Nephrol. 9, 419–429. doi:10.1038/nrneph.2013.99
Krediet, R. T. (2018). Ultrafiltration failure is a reflection of peritoneal alterations in patients treated with peritoneal dialysis. Front. Physiol. 9, 1815. doi:10.3389/fphys.2018.01815
Kubli, D. A., and Gustafsson, Å. B. (2012). Mitochondria and mitophagy: The yin and yang of cell death control. Circ. Res. 111, 1208–1221. doi:10.1161/CIRCRESAHA.112.265819
Lai, K. N., Lai, K. B., Lam, C. W., Chan, T. M., Li, F. K., and Leung, J. C. (2000). Changes of cytokine profiles during peritonitis in patients on continuous ambulatory peritoneal dialysis. Am. J. Kidney Dis. 35, 644–652. doi:10.1016/s0272-6386(00)70011-4
Lai, K. N., and Leung, J. C. (2010). Inflammation in peritoneal dialysis. Nephron Clin. Pract. 116, c11–c18. doi:10.1159/000314544
Lai, K. N., Tang, S. C., and Leung, J. C. (2007). Mediators of inflammation and fibrosis. Perit. Dial. Int. 27 (2), S65–S71. doi:10.1177/089686080702702s12
Lemasters, J. J. (2005). Selective mitochondrial autophagy, or mitophagy, as a targeted defense against oxidative stress, mitochondrial dysfunction, and aging. Rejuvenation Res. 8, 3–5. doi:10.1089/rej.2005.8.3
Leung, J. C., Chan, L. Y., Tang, S. C., Chu, K. M., and Lai, K. N. (2006). Leptin induces TGF-beta synthesis through functional leptin receptor expressed by human peritoneal mesothelial cell. Kidney Int. 69, 2078–2086. doi:10.1038/sj.ki.5000409
Leung, J. C., Lam, M. F., Tang, S. C., Chan, L. Y., Tam, K. Y., Yip, T. P., et al. (2009). Roles of neutrophil gelatinase-associated lipocalin in continuous ambulatory peritoneal dialysis-related peritonitis. J. Clin. Immunol. 29, 365–378. doi:10.1007/s10875-008-9271-7
Levallois, J., Nadeau-Fredette, A. C., Labbé, A. C., Laverdière, M., Ouimet, D., and Vallée, M. (2012). Ten-year experience with fungal peritonitis in peritoneal dialysis patients: Antifungal susceptibility patterns in a north-American center. Int. J. Infect. Dis. 16, e41–e43. doi:10.1016/j.ijid.2011.09.016
Li, D., Ding, Z., Du, K., Ye, X., and Cheng, S. (2021). Reactive oxygen species as a link between antioxidant pathways and autophagy. Oxid. Med. Cell Longev. 2021, 5583215. doi:10.1155/2021/5583215
Li, D., Lu, Z., Xu, Z., Ji, J., Zheng, Z., Lin, S., et al. (2016). Spironolactone promotes autophagy via inhibiting PI3K/AKT/mTOR signalling pathway and reduce adhesive capacity damage in podocytes under mechanical stress. Biosci. Rep. 36, e00355. doi:10.1042/BSR20160086
Li, P. K., Szeto, C. C., Piraino, B., de Arteaga, J., Fan, S., Figueiredo, A. E., et al. (2016). ISPD peritonitis recommendations: 2016 update on prevention and treatment. Perit. Dial. Int. 36, 481–508. doi:10.3747/pdi.2016.00078
Li, R., Du, J. H., Yao, G. M., Yao, Y., and Zhang, J. (2019). Autophagy: A new mechanism for regulating VEGF and PEDF expression in retinal pigment epithelium cells. Int. J. Ophthalmol. 12, 557–562. doi:10.18240/ijo.2019.04.05
Li, S., Peng, F., Gong, W., Wu, J., Wang, Y., Xu, Z., et al. (2019). Dimethylaminomicheliolide ameliorates peritoneal fibrosis through the activation of autophagy. J. Mol. Med. Berl. 97, 659–674. doi:10.1007/s00109-019-01757-1
Li, S., Zhou, Y., Fan, J., Cao, S., Cao, T., Huang, F., et al. (2011). Heat shock protein 72 enhances autophagy as a protective mechanism in lipopolysaccharide-induced peritonitis in rats. Am. J. Pathol. 179, 2822–2834. doi:10.1016/j.ajpath.2011.08.013
Li, X., Zhang, W., Cao, Q., Wang, Z., Zhao, M., Xu, L., et al. (2020). Mitochondrial dysfunction in fibrotic diseases. Cell Death Discov. 6, 80. doi:10.1038/s41420-020-00316-9
Li, X. Y., Wu, J., Luo, D., Chen, W. X., Zhu, G. L., Zhang, Y. X., et al. (2017). Effect of high glucose-based peritoneal dialysis fluids on NLRP3-IL-1β in human peritoneal mesothelial cells. Beijing Da Xue Xue Bao Yi Xue Ban. 49, 954–960.
Liang, S., Wu, Y. S., Li, D. Y., Tang, J. X., and Liu, H. F. (2022). Autophagy and renal fibrosis. Aging Dis. 13, 712–731. doi:10.14336/AD.2021.1027
Ligeon, L. A., Temime-Smaali, N., and Lafont, F. (2011). Ubiquitylation and autophagy in the control of bacterial infections and related inflammatory responses. Cell Microbiol. 13, 1303–1311. doi:10.1111/j.1462-5822.2011.01628.x
Liu, Q., Zhang, Y., Mao, H., Chen, W., Luo, N., Zhou, Q., et al. (2012). A crosstalk between the Smad and JNK signaling in the TGF-β-induced epithelial-mesenchymal transition in rat peritoneal mesothelial cells. PLoS One 7, e32009. doi:10.1371/journal.pone.0032009
Lo, W. K., and Kawanishi, H. (2009). Encapsulating peritoneal sclerosis-medical and surgical treatment. Perit. Dial. Int. 29 (2), S211–S214. doi:10.1177/089686080902902s43
Loureiro, J., Aguilera, A., Selgas, R., Sandoval, P., Albar-Vizcaíno, P., Pérez-Lozano, M. L., et al. (2011). Blocking TGF-β1 protects the peritoneal membrane from dialysate-induced damage. J. Am. Soc. Nephrol. 22, 1682–1695. doi:10.1681/ASN.2010111197
Lu, Q., Wang, W. W., Zhang, M. Z., Ma, Z. X., Qiu, X. R., Shen, M., et al. (2019). ROS induces epithelial-mesenchymal transition via the TGF-β1/PI3K/Akt/mTOR pathway in diabetic nephropathy. Exp. Ther. Med. 17, 835–846. doi:10.3892/etm.2018.7014
Lu, X., Fan, Q., Xu, L., Li, L., Yue, Y., Xu, Y., et al. (2015). Ursolic acid attenuates diabetic mesangial cell injury through the up-regulation of autophagy via miRNA-21/PTEN/Akt/mTOR suppression. PLoS One 10, e0117400. doi:10.1371/journal.pone.0117400
Margetts, P. J., Bonniaud, P., Liu, L., Hoff, C. M., Holmes, C. J., West-Mays, J. A., et al. (2005). Transient overexpression of TGF-{beta}1 induces epithelial mesenchymal transition in the rodent peritoneum. J. Am. Soc. Nephrol. 16, 425–436. doi:10.1681/ASN.2004060436
Masola, V., Bonomini, M., Borrelli, S., Di Liberato, L., Vecchi, L., Onisto, M., et al. (2022). Fibrosis of peritoneal membrane as target of new therapies in peritoneal dialysis. Int. J. Mol. Sci. 23, 4831. doi:10.3390/ijms23094831
Matsuo, H., Tamura, M., Kabashima, N., Serino, R., Tokunaga, M., Shibata, T., et al. (2006). Prednisolone inhibits hyperosmolarity-induced expression of MCP-1 via NF-kappaB in peritoneal mesothelial cells. Kidney Int. 69, 736–746. doi:10.1038/sj.ki.5000131
Mayer, A. L., Higgins, C. B., Heitmeier, M. R., Kraft, T. E., Qian, X., Crowley, J. R., et al. (2016). SLC2A8 (GLUT8) is a mammalian trehalose transporter required for trehalose-induced autophagy. Sci. Rep. 6, 38586. doi:10.1038/srep38586
Mehrotra, R., Chiu, Y. W., Kalantar-Zadeh, K., Bargman, J., and Vonesh, E. (2011). Similar outcomes with hemodialysis and peritoneal dialysis in patients with end-stage renal disease. Arch. Intern Med. 171, 110–118. doi:10.1001/archinternmed.2010.352
Meng, D., Li, Z., Wang, G., Ling, L., Wu, Y., and Zhang, C. (2018). Carvedilol attenuates liver fibrosis by suppressing autophagy and promoting apoptosis in hepatic stellate cells. Biomed. Pharmacother. 108, 1617–1627. doi:10.1016/j.biopha.2018.10.005
Meng, N., Zhao, J., Su, L., Zhao, B., Zhang, Y., Zhang, S., et al. (2012). A butyrolactone derivative suppressed lipopolysaccharide-induced autophagic injury through inhibiting the autoregulatory loop of p8 and p53 in vascular endothelial cells. Int. J. Biochem. Cell Biol. 44, 311–319. doi:10.1016/j.biocel.2011.11.001
Miyake, T., Sakai, N., Tamai, A., Sato, K., Kamikawa, Y., Miyagawa, T., et al. (2020). Trehalose ameliorates peritoneal fibrosis by promoting Snail degradation and inhibiting mesothelial-to-mesenchymal transition in mesothelial cells. Sci. Rep. 10, 14292. doi:10.1038/s41598-020-71230-4
Morishita, H., and Mizushima, N. (2019). Diverse cellular roles of autophagy. Annu. Rev. Cell Dev. Biol. 35, 453–475. doi:10.1146/annurev-cellbio-100818-125300
Myers, M. J. (2004). Leptin receptor signaling and the regulation of mammalian physiology. Recent Prog. Horm. Res. 59, 287–304. doi:10.1210/rp.59.1.287
Nadeau-Fredette, A. C., and Bargman, J. M. (2015). Characteristics and outcomes of fungal peritonitis in a modern North American cohort. Perit. Dial. Int. 35, 78–84. doi:10.3747/pdi.2013.00179
Nakagawa, I., Amano, A., Mizushima, N., Yamamoto, A., Yamaguchi, H., Kamimoto, T., et al. (2004). Autophagy defends cells against invading group A Streptococcus. Science 306, 1037–1040. doi:10.1126/science.1103966
Namba, T., Takabatake, Y., Kimura, T., Takahashi, A., Yamamoto, T., Matsuda, J., et al. (2014). Autophagic clearance of mitochondria in the kidney copes with metabolic acidosis. J. Am. Soc. Nephrol. 25, 2254–2266. doi:10.1681/ASN.2013090986
Padwal, M., Cheng, G., Liu, L., Boivin, F., Gangji, A. S., Brimble, K. S., et al. (2018). WNT signaling is required for peritoneal membrane angiogenesis. Am. J. Physiol. Ren. Physiol. 314, F1036–F1045. doi:10.1152/ajprenal.00497.2017
Patel, P., Sekiguchi, Y., Oh, K. H., Patterson, S. E., Kolb, M. R., and Margetts, P. J. (2010). Smad3-dependent and -independent pathways are involved in peritoneal membrane injury. Kidney Int. 77, 319–328. doi:10.1038/ki.2009.436
Pepereke, S., Shah, A. D., and Brown, E. A. (2022). Encapsulating peritoneal sclerosis: Your questions answered. Perit. Dial. Int. 43, 119–127. doi:10.1177/08968608221125606
Peterson, P. K., Matzke, G., and Keane, W. F. (1987). Current concepts in the management of peritonitis in patients undergoing continuous ambulatory peritoneal dialysis. Rev. Infect. Dis. 9, 604–612. doi:10.1093/clinids/9.3.604
Ramil-Gómez, O., Rodríguez-Carmona, A., Fernández-Rodríguez, J. A., Pérez-Fontán, M., Ferreiro-Hermida, T., López-Pardo, M., et al. (2021). Mitochondrial dysfunction plays a relevant role in Pathophysiology of peritoneal membrane damage induced by peritoneal dialysis. Antioxidants (Basel) 10, 447. doi:10.3390/antiox10030447
Sakai, N., Nakamura, M., Lipson, K. E., Miyake, T., Kamikawa, Y., Sagara, A., et al. (2017). Inhibition of CTGF ameliorates peritoneal fibrosis through suppression of fibroblast and myofibroblast accumulation and angiogenesis. Sci. Rep. 7, 5392. doi:10.1038/s41598-017-05624-2
Schroder, K., and Tschopp, J. (2010). The inflammasomes. Cell 140, 821–832. doi:10.1016/j.cell.2010.01.040
Shi, Y., Hu, Y., Wang, Y., Ma, X., Tang, L., Tao, M., et al. (2021). Blockade of autophagy prevents the development and progression of peritoneal fibrosis. Front. Pharmacol. 12, 724141. doi:10.3389/fphar.2021.724141
Shin, H. S., Ko, J., Kim, D. A., Ryu, E. S., Ryu, H. M., Park, S. H., et al. (2017). Metformin ameliorates the phenotype transition of peritoneal mesothelial cells and peritoneal fibrosis via a modulation of oxidative stress. Sci. Rep. 7, 5690. doi:10.1038/s41598-017-05836-6
Sir, D., Tian, Y., Chen, W. L., Ann, D. K., Yen, T. S., and Ou, J. H. (2010). The early autophagic pathway is activated by Hepatitis B virus and required for viral DNA replication. Proc. Natl. Acad. Sci. U. S. A. 107, 4383–4388. doi:10.1073/pnas.0911373107
Smit, W., Schouten, N., van den Berg, N., Langedijk, M. J., Struijk, D. G., Krediet, R. T., et al. (2004). Analysis of the prevalence and causes of ultrafiltration failure during long-term peritoneal dialysis: A cross-sectional study. Perit. Dial. Int. 24, 562–570. doi:10.1177/089686080402400616
Stenvinkel, P., and Alvestrand, A. (2002). Inflammation in end-stage renal disease: Sources, consequences, and therapy. Semin. Dial. 15, 329–337. doi:10.1046/j.1525-139x.2002.00083.x
Strippoli, R., Moreno-Vicente, R., Battistelli, C., Cicchini, C., Noce, V., Amicone, L., et al. (2016). Molecular mechanisms underlying peritoneal EMT and fibrosis. Stem Cells Int., 2016, 3543678, doi:10.1155/2016/3543678
Sun, M., Tan, L., and Hu, M. (2021). The role of autophagy in hepatic fibrosis. Am. J. Transl. Res. 13, 5747–5757.
Szeto, C. C., and Li, P. K. (2019). Peritoneal dialysis-associated peritonitis. Clin. J. Am. Soc. Nephrol. 14, 1100–1105. doi:10.2215/CJN.14631218
Thurston, T. L., Ryzhakov, G., Bloor, S., von Muhlinen, N., and Randow, F. (2009). The TBK1 adaptor and autophagy receptor NDP52 restricts the proliferation of ubiquitin-coated bacteria. Nat. Immunol. 10, 1215–1221. doi:10.1038/ni.1800
Tobudic, S., Forstner, C., Schranz, H., Poeppl, W., Vychytil, A., and Burgmann, H. (2013). Comparative in vitro fungicidal activity of echinocandins against Candida albicans in peritoneal dialysis fluids. Mycoses 56, 623–630. doi:10.1111/myc.12079
Wang, J., Feng, X., Zeng, Y., Fan, J., Wu, J., Li, Z., et al. (2013). Lipopolysaccharide (LPS)-induced autophagy is involved in the restriction of Escherichia coli in peritoneal mesothelial cells. BMC Microbiol. 13, 255. doi:10.1186/1471-2180-13-255
Wei, M., Li, Z., and Yang, Z. (2014). Crosstalk between protective autophagy and NF-κB signal in high glucose-induced podocytes. Mol. Cell Biochem. 394, 261–273. doi:10.1007/s11010-014-2102-7
Witowski, J., Wisniewska, J., Korybalska, K., Bender, T. O., Breborowicz, A., Gahl, G. M., et al. (2001). Prolonged exposure to glucose degradation products impairs viability and function of human peritoneal mesothelial cells. J. Am. Soc. Nephrol. 12, 2434–2441. doi:10.1681/ASN.V12112434
Wu, J., Li, X., Zhu, G., Zhang, Y., He, M., and Zhang, J. (2016). The role of Resveratrol-induced mitophagy/autophagy in peritoneal mesothelial cells inflammatory injury via NLRP3 inflammasome activation triggered by mitochondrial ROS. Exp. Cell Res. 341, 42–53. doi:10.1016/j.yexcr.2016.01.014
Wu, J., Xing, C., Zhang, L., Mao, H., Chen, X., Liang, M., et al. (2018). Autophagy promotes fibrosis and apoptosis in the peritoneum during long-term peritoneal dialysis. J. Cell Mol. Med. 22, 1190–1201. doi:10.1111/jcmm.13393
Wu, Y., Duan, X., Gao, Z., Yang, N., and Xue, F. (2022). AICAR attenuates postoperative abdominal adhesion formation by inhibiting oxidative stress and promoting mesothelial cell repair. PLoS One 17, e0272928. doi:10.1371/journal.pone.0272928
Wynn, T. A., and Vannella, K. M. (2016). Macrophages in tissue repair, regeneration, and fibrosis. Immunity 44, 450–462. doi:10.1016/j.immuni.2016.02.015
Xiao, J., Gong, Y., Chen, Y., Yu, D., Wang, X., Zhang, X., et al. (2017). IL-6 promotes epithelial-to-mesenchymal transition of human peritoneal mesothelial cells possibly through the JAK2/STAT3 signaling pathway. Am. J. Physiol. Ren. Physiol. 313, F310–F318. doi:10.1152/ajprenal.00428.2016
Xu, Y., Jagannath, C., Liu, X. D., Sharafkhaneh, A., Kolodziejska, K. E., and Eissa, N. T. (2007). Toll-like receptor 4 is a sensor for autophagy associated with innate immunity. Immunity 27, 135–144. doi:10.1016/j.immuni.2007.05.022
Xu, Z., Qiao, S., Qian, W., Zhu, Y., Yan, W., Shen, S., et al. (2022). Card9 protects fungal peritonitis through regulating Malt1-mediated activation of autophagy in macrophage. Int. Immunopharmacol. 110, 108941. doi:10.1016/j.intimp.2022.108941
Yáñez-Mó, M., Lara-Pezzi, E., Selgas, R., Ramírez-Huesca, M., Domínguez-Jiménez, C., Jiménez-Heffernan, J. A., et al. (2003). Peritoneal dialysis and epithelial-to-mesenchymal transition of mesothelial cells. N. Engl. J. Med. 348, 403–413. doi:10.1056/NEJMoa020809
Yang, F., Qu, Q., Zhao, C., Liu, X., Yang, P., Li, Z., et al. (2020). Paecilomyces cicadae-fermented Radix astragali activates podocyte autophagy by attenuating PI3K/AKT/mTOR pathways to protect against diabetic nephropathy in mice. Biomed. Pharmacother. 129, 110479. doi:10.1016/j.biopha.2020.110479
Yang, L., Fan, Y., Zhang, X., Liu, J., and Ma, J. (2017). Effect of 1,25(OH)2D3 on high glucose-induced autophagy inhibition in peritoneum. Mol. Med. Rep. 16, 7080–7085. doi:10.3892/mmr.2017.7408
Yang, L., Wu, L., Du, S., Hu, Y., Fan, Y., and Ma, J. (2016). 1,25(OH)2D3 inhibits high glucose-induced apoptosis and ROS production in human peritoneal mesothelial cells via the MAPK/P38 pathway. Mol. Med. Rep. 14, 839–844. doi:10.3892/mmr.2016.5323
Yang, X., Bao, M., Fang, Y., Yu, X., Ji, J., and Ding, X. (2021). STAT3/HIF-1α signaling activation mediates peritoneal fibrosis induced by high glucose. J. Transl. Med. 19, 283. doi:10.1186/s12967-021-02946-8
Yang, X., Yan, H., Jiang, N., Yu, Z., Yuan, J., Ni, Z., et al. (2020). IL-6 trans-signaling drives a STAT3-dependent pathway that leads to structural alterations of the peritoneal membrane. Am. J. Physiol. Ren. Physiol. 318, F338–F353. doi:10.1152/ajprenal.00319.2019
Yeates, K., Zhu, N., Vonesh, E., Trpeski, L., Blake, P., and Fenton, S. (2012). Hemodialysis and peritoneal dialysis are associated with similar outcomes for end-stage renal disease treatment in Canada. Nephrol. Dial. Transpl. 27, 3568–3575. doi:10.1093/ndt/gfr674
Yoshizawa, H., Morishita, Y., Watanabe, M., Ishibashi, K., Muto, S., Kusano, E., et al. (2015). TGF-β1-siRNA delivery with nanoparticles inhibits peritoneal fibrosis. Gene Ther. 22, 333–340. doi:10.1038/gt.2014.116
Youle, R. J., and Narendra, D. P. (2011). Mechanisms of mitophagy. Nat. Rev. Mol. Cell Biol. 12, 9–14. doi:10.1038/nrm3028
Yung, S., and Chan, T. M. (2012). Pathophysiological changes to the peritoneal membrane during PD-related peritonitis: The role of mesothelial cells. Mediat. Inflamm., 2012, 484167, doi:10.1155/2012/484167
Zareie, M., Tangelder, G. J., ter Wee, P. M., Hekking, L. H., van Lambalgen, A. A., Keuning, E. D., et al. (2005). Beneficial effects of aminoguanidine on peritoneal microcirculation and tissue remodelling in a rat model of PD. Nephrol. Dial. Transpl. 20, 2783–2792. doi:10.1093/ndt/gfi138
Zhang, X., Wang, J., Fan, Y., Yang, L., Wang, L., and Ma, J. (2012). Zinc supplementation attenuates high glucose-induced epithelial-to-mesenchymal transition of peritoneal mesothelial cells. Biol. Trace Elem. Res. 150, 229–235. doi:10.1007/s12011-012-9451-4
Zhang, Y., Feng, J., Wang, Q., Zhao, S., Xu, J., and Li, H. (2018). PPAR-γ agonist rosiglitazone ameliorates peritoneal deterioration in peritoneal dialysis rats with LPS-induced peritonitis through up-regulation of AQP-1 and ZO-1. Biosci. Rep. 38. doi:10.1042/BSR20180009
Zhang, Y., Sun, Q., Li, X., Ma, X., Li, Y., Jiao, Z., et al. (2018). Apigenin suppresses mouse peritoneal fibrosis by down-regulating miR34a expression. Biomed. Pharmacother. 106, 373–380. doi:10.1016/j.biopha.2018.06.138
Zhang, Y. F., Zou, X. L., Wu, J., Yu, X. Q., and Yang, X. (2015). Rosiglitazone, a peroxisome proliferator-activated receptor (PPAR)-γ agonist, attenuates inflammation via NF-κB inhibition in lipopolysaccharide-induced peritonitis. Inflammation 38, 2105–2115. doi:10.1007/s10753-015-0193-2
Zhao, X. C., Livingston, M. J., Liang, X. L., and Dong, Z. (2019). Cell apoptosis and autophagy in renal fibrosis. Adv. Exp. Med. Biol. 1165, 557–584. doi:10.1007/978-981-13-8871-2_28
Zhao, X. D., Qin, R. H., Yang, J. J., Xu, S. S., Tao, H., Ding, X. S., et al. (2018). DNMT3A controls miR-200b in cardiac fibroblast autophagy and cardiac fibrosis. Inflamm. Res. 67, 681–690. doi:10.1007/s00011-018-1159-2
Zhong, J., Gong, W., Chen, J., Qing, Y., Wu, S., Li, H., et al. (2018). Micheliolide alleviates hepatic steatosis in db/db mice by inhibiting inflammation and promoting autophagy via PPAR-γ-mediated NF-кB and AMPK/mTOR signaling. Int. Immunopharmacol. 59, 197–208. doi:10.1016/j.intimp.2018.03.036
Keywords: autophagy, human peritoneal mesothelial cells, peritoneal dialysis, peritoneal fibrosis, peritoneal dialysis-related peritonitis
Citation: Su H-y, Yang J-j, Zou R, An N, Chen X-c, Yang C, Yang H-j, Yao C-w and Liu H-f (2023) Autophagy in peritoneal fibrosis. Front. Physiol. 14:1187207. doi: 10.3389/fphys.2023.1187207
Received: 15 March 2023; Accepted: 03 May 2023;
Published: 15 May 2023.
Edited by:
Guoshuang Xu, Fourth Military Medical University, ChinaReviewed by:
Yuan Gui, University of Connecticut, United StatesQuan Hong, Chinese PLA General Hospital, China
Copyright © 2023 Su, Yang, Zou, An, Chen, Yang, Yang, Yao and Liu. This is an open-access article distributed under the terms of the Creative Commons Attribution License (CC BY). The use, distribution or reproduction in other forums is permitted, provided the original author(s) and the copyright owner(s) are credited and that the original publication in this journal is cited, in accordance with accepted academic practice. No use, distribution or reproduction is permitted which does not comply with these terms.
*Correspondence: Hua-feng Liu, aGYtbGl1QDI2My5uZXQ=
†These authors have contributed equally to this work