- 1Laboratory of Exercise Physiology, Department of Movement, Human and Health Sciences, University of Rome “Foro Italico”, Rome, Italy
- 2Division of Respiratory and Critical Care Physiology and Medicine, The Lundquist Institute for Biomedical Innovation at Harbor-UCLA Medical Center, Institute of Respiratory Medicine and Exercise Physiology, Torrance, CA, United States
Purpose: Growing evidence suggests that respiratory frequency (fR) is a marker of physical effort and a variable sensitive to changes in exercise tolerance. The comparison between arm+leg cycling (Arm+leg) and leg cycling (Leg) has the potential to further test this notion because a greater exercise tolerance is expected in the Arm+leg modality. We systematically compared Arm+leg vs. Leg using different performance tests.
Methods: Twelve males underwent six performance tests in separate, randomized visits. Three tests were performed in each of the two exercise modalities, i.e. an incremental test and two time-to-exhaustion (TTE) tests performed at 90% or 75% of the peak power output reached in the Leg incremental test (PPOLeg). Exercise tolerance, perceived exertion, and cardiorespiratory variables were recorded during all the tests.
Results: A greater exercise tolerance (p < 0.001) was found for Arm+leg in the incremental test (337 ± 32 W vs. 292 ± 28 W), in the TTE test at 90% of PPOLeg (638 ± 154 s vs. 307 ± 67 s), and in the TTE test at 75% of PPOLeg (1,675 ± 525 s vs. 880 ± 363 s). Unlike
Conclusion: Minute ventilation, fR and perceived exertion are sensitive to the improvements in exercise tolerance observed when comparing Arm+leg vs. Leg, unlike
1 Introduction
The comparison between different exercise modalities has the potential to improve our understanding of the physiology of endurance performance. Classical studies have compared leg cycling (Leg) with arm+leg cycling (Arm+leg) to unravel the mechanisms limiting maximal oxygen uptake (Astrand and Saltin, 1961; Secher et al., 1974; Bergh et al., 1976). Findings from these studies have contributed to outlining the important role of cardiocirculatory factors in setting the upper limit for maximal aerobic power. Indeed, the peak value of oxygen uptake (
Growing evidence suggests that fR is a valid marker of physical effort (Nicolò et al., 2014; Nicolò et al., 2016; Nicolò et al., 2017a; Nicolò et al., 2017b; Nicolò et al., 2019; Nicolò and Sacchetti, 2023) and that its time course reflects changes in exercise tolerance (Nicolò and Sacchetti, 2023). In a variety of conditions where exercise tolerance is reduced (experimentally) or lowered (in a cross-sectional comparison), the rate of increase in fR is higher, both during incremental and TTE tests. On the other hand, the rate of increase in fR is lower when assessing exercise strategies, experimental interventions or other conditions leading to an improvement in exercise tolerance (Nicolò and Sacchetti, 2023). The sensitivity of fR to changes in exercise tolerance and the close association between fR and perceived exertion are among the factors suggesting that fR is to a large extent modulated by central command (the activity of motor and premotor brain areas relating to voluntary locomotor muscle contraction) during high-intensity exercise (Nicolò and Sacchetti, 2023). This explains why fR can be considered a marker of physical effort, which is defined as the degree of motor effort (i.e. the magnitude of central command) (Nicolò et al., 2017b). However, measuring the magnitude of central command during “real” exercise conditions is particularly challenging. Hence, it is important to use different approaches (including the comparison of different exercise modalities) to provide indirect evidence on the contribution of central command to fR modulation (Nicolò and Sacchetti, 2023).
The comparison between arm+leg cycling and leg cycling may either challenge or reinforce the notion that the increase in fR during high-intensity exercise reflects changes in exercise tolerance and is influenced by central command. fR is also modulated by muscle afferent feedback from groups III and IV (hereinafter muscle afferent feedback) (Dempsey et al., 2014; Girardi et al., 2021; Nicolò and Sacchetti, 2023), and this drive to breathe may have a greater relative contribution to fR modulation when arm muscles assist leg muscles during arm+leg cycling, in view of a potentially larger amount of muscle mass concomitantly involved in exercise (Dempsey et al., 2014; Nicolò and Sacchetti, 2023). Indeed, even the passive movement of the legs leads to a substantial increase in fR that is at least partially mediated by muscle afferent feedback (Girardi et al., 2021), and this drive to breathe may increase further when adding the movement of the upper limbs. The contribution of muscle afferent feedback to fR may partially confound the association between fR and perceived exertion because the latter is supposed to be largely independent of muscle afferent feedback (Marcora, 2009; Bergevin et al., 2023). While arm+leg cycling may also result in a higher
The purpose of the present study was to systematically assess whether exercise tolerance improves with arm+leg cycling vs. (conventional) leg cycling and whether fR and perceived exertion are sensitive to the expected differences in exercise tolerance. To increase the robustness of our evaluation, we compared arm+leg cycling vs. leg cycling using two exercise paradigms (i.e. incremental test and TTE test) and three comparisons, as the TTE test was performed at two different intensities. We tested the hypotheses that i) arm+leg cycling improves exercise tolerance compared to leg cycling irrespective of the exercise paradigm; and ii) fR is a good marker of physical effort sensitive to between-modality changes in exercise tolerance, unlike other physiological variables such as
2 Materials and methods
2.1 Participants
Twelve recreationally trained males (mean ± SD: age 26 ± 4 years; stature 1.79 ± 0.08 m and body mass: 81 ± 10 kg) volunteered to participate in this study. The volunteers recruited participated in one or more sporting activities requiring the use of both arms and legs (e.g. rugby, extreme conditioning program training, and triathlon), as the benefits of arm+leg cycling vs. leg cycling may be more pronounced for individuals exercising with both upper and lower limbs (Secher et al., 1974). The study was approved by the Institutional Review Board of the University of Rome “Foro Italico” in compliance with the Declaration of Helsinki (CAR 07/2019). Written informed consent was obtained from all participants. They were asked to refrain from vigorous exercise and the consumption of alcohol and caffeine in the 24 h preceding each laboratory visit.
2.2 Experimental overview
Participants reported to the laboratory on 7 different occasions over a 4-week period, with visits separated by at least 48 h. On the first visit, participants were familiarised with the experimental procedures and tests. On the subsequent visits, participants performed three performance tests to exhaustion in each of the two exercise modalities, i.e. arm+leg cycling and leg cycling. The performance tests consisted of a step incremental test and two TTE tests performed at different intensities. Specifically, the incremental tests (Arm+legINC and LegINC) were performed on visits 2 and 3, in random order. The PPO of the LegINC test (PPOLeg) was used to set the power output of the TTE tests (i.e. 90% and 75% of PPOLeg), which were performed on visits 4–7. The order of Arm+leg and Leg tests was always randomized, as well as the order of the TTE tests at 90% and 75% of PPOLeg. All the tests were performed on a multimodal ergometer custom-made by ORF s.r.l Magnetic Days® (Arezzo, Italy) and specifically developed for performing this study. Exhaustion was defined as the decrease in pedaling cadence below 60 rpm, either with the legs or arms. All testing was completed in a laboratory with a room temperature of 23°C ± 1°C. A cooling fan was used during all the tests, and mechanical, physiological, and perceptual variables were recorded as detailed below.
2.3 Multimodal ergometer
The multimodal ergometer was made up of a grinding ergometer and a cycling ergometer, and their combined use allowed the participants to perform arm+leg cycling (see Figure 1). Both the grinding and cycling ergometers were electromagnetically braked, equipped with professional torque transducers (model RT2A) certified by AEP transducers (Cognento, Modena, Italy), and calibrated according to the manufacturer’s instructions. The expanded uncertainty was lower than 0.114% for both torque transducers used. The arm cranks of the grinding ergometer were provided by Harken Italy SPA (Limido Comasco, Como). The chainring of the grinding ergometer was not mechanically connected with that of the cycling ergometer to allow for the separate measurement of the power output provided by the two ergometers. Hence, one of the advantages of this multimodal ergometer is the opportunity to set and register the contribution of arms and legs to the total power output. In all the Arm+leg tests, the relative contribution of the arms was initially set at 20% of the total power output based on the findings reported by Bergh et al. (1976). Thereafter, participants were allowed to request changes in the relative contribution of the arms throughout each test according to preference, and they were familiarised with this procedure on the first visit. The option of individualizing the relative contribution of the arms is supported by previous studies reporting inter-individual variability in preference (Hill et al., 2018) and exercise tolerance (Bergh et al., 1976) for different power output distributions between arms and legs. Participants were free to choose their preferred pedaling cadence in all the tests. In both Arm+leg and Leg modalities, the ergometer settings were set up on the first visit according to participants’ anthropometric characteristics and comfort, and were reproduced in the subsequent visits. The Arm+leg tests were performed on the multimodal ergometer, while the Leg tests were performed on the cycling ergometer (the participants were allowed to use the handlebars).
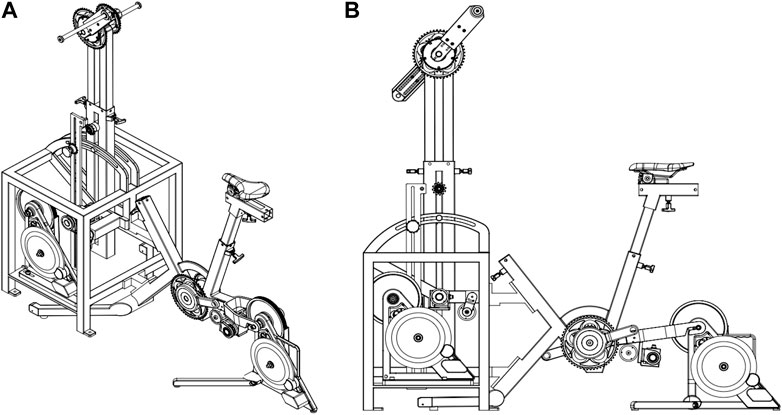
FIGURE 1. Three-dimensional (A) and lateral (B) views of the multimodal ergometer composed of a grinding ergometer and a cycling ergometer. The multimodal ergometer was custom-made by ORF s.r.l Magnetic Days® (Arezzo, Italy). Note that each of the two ergometers is electromagnetically braked and is equipped with a professional torque transducer.
2.4 Step incremental tests
Before the incremental tests, a 5-min warm-up was performed to allow the participants to check the ergometer settings. For both Arm+legINC and LegINC, the first stage of the incremental test consisted of 3 min at 150 W, and the power output was subsequently increased by 20 W every min. The power output of the first stage was chosen to ensure that at least 20% of the total power output could be sustained by the arms in the Arm+leg modality, and the minimum power output for the arm ergometer was about 25 W. Hence, it was not possible to select a power output lower than 150 W, and this limited the opportunity to rigorously determine the gas exchange threshold (GET) and the respiratory compensation point (RCP). The PPO reached in the incremental test was computed as the work rate of the last completed stage plus the fraction of time spent in the last uncompleted stage multiplied by the work-rate increment (i.e. 20 W). The Borg’s 6–20 scale (Borg, 1998) was used to collect ratings of perceived exertion (RPE) data every min. Participants were familiarised with the use of the RPE scale on the first visit and were asked to verbally provide an RPE value, as required by the Arm+leg modality. Breathing artifacts caused by speaking were then removed by data filtering, as described below. Participants did not receive any performance feedback or encouragement during any of the incremental tests performed.
2.5 Time to exhaustion tests
After a 10-min self-paced warm-up, participants performed a TTE test in each of the two exercise modalities (Arm+leg and Leg) and intensities (90% and 75% of PPOLeg) in separate visits. Hereinafter, the TTE tests are abbreviated as Arm+legTTE90 (Arm+leg test at 90% of PPOLeg), LegTTE90 (Leg test at 90% of PPOLeg), Arm+legTTE75 (Arm+leg test at 75% of PPOLeg) and LegTTE75 (Leg test at 75% of PPOLeg). Perceived exertion data were collected every min, while physiological and mechanical variables were measured continuously. Participants did not receive any performance feedback or encouragement during any of the TTE tests performed in this study.
2.6 Cardiorespiratory measures
fR, VT, minute ventilation (
2.7 Data analysis
Data were analyzed with MATLAB (R2016a, The Mathworks, Natick, MA, United States). The comparison of the physiological and perceptual responses between arm+leg cycling and leg cycling was performed in all the tests using the “individual isotime” analysis described by Nicolò et al. (2019). This analysis allows for between-condition comparisons while avoiding the data loss that occurs when the variability in TTE is not addressed on an individual basis (Nicolò et al., 2019). Briefly, breath-by-breath data of fR, VT,
When reporting the relationship between different variables (i.e. RPE vs. fR, RPE vs.
2.8 Statistical analysis
Statistical analyses were conducted using IBM SPSS Statistics 20 (SPSS Inc., Chicago, IL, United States). Data were checked for normality prior to analysis. A paired Student’s t-test was used to compare the performance values of Arm+legINC vs. LegINC, Arm+legTTE90 vs. LegTTE90 and Arm+legTTE75 vs. LegTTE75 separately. The Cohen’s d effect size for paired t-test was then calculated and considered small, moderate or large for values ≥0.2, ≥0.5 and ≥0.8 respectively. A paired Student’s t-test was also used to compare the end-test values of physiological variables between Arm+leg and Leg tests. A two-way repeated-measures ANOVA (condition × time) was used to compare physiological and perceptual responses (processed with the “individual isotime” method) of Arm+leg vs. Leg for each of the three performance tests separately. When the sphericity assumption was violated, the Greenhouse–Geisser adjustment was performed. Partial eta squared (ηp2) effect sizes were calculated for the main effect of condition, the main effect of time, and the interaction; ηp2 values ≥0.01, ≥0.059 and ≥0.138 indicate small, medium and large effects respectively (Cohen, 1988). When a significant interaction was found, pairwise comparisons were performed at each time point using a one-way repeated measures ANOVA to identify differences between Arm+leg and Leg tests. The HR data of the incremental tests and the TTE tests at 75% of PPOLeg were not normally distributed and were analyzed using Friedman’s two-way ANOVA. When statistical significance was found, this test was followed up by a Wilcoxon Signed-Rank test to identify where differences between Arm+leg and Leg tests occurred.
After processing data with the “relative isotime” method, the correlations between RPE and fR, RPE and
3 Results
3.1 Step incremental tests
A significantly greater (p < 0.001; Cohen’s d = 2.78) PPO was found in Arm+legINC (337 ± 32 W) vs. LegINC (292 ± 28 W) (Figure 2A). The average relative contribution of the arms to the total power output was 22% ± 2% in the Arm+legINC test. A higher pedaling cadence (p < 0.01) was found in the LegINC (84 ± 6 rpm) compared to that of the arms (76 ± 6 rpm) and legs (77 ± 7 rpm) of the Arm+legINC test. The two tests (Arm+legINC vs. LegINC) showed significant differences (p < 0.043) in the end-test values of
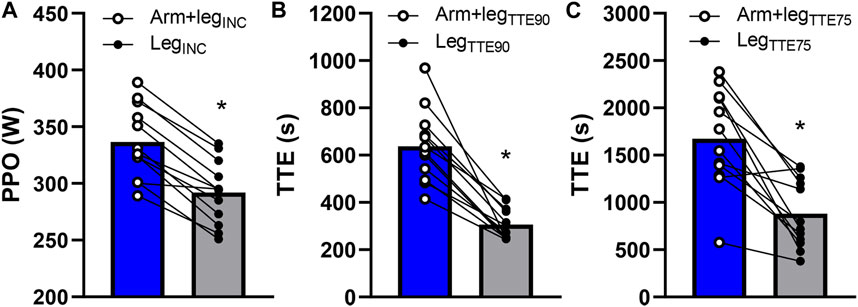
FIGURE 2. Average performance differences between Arm+leg tests (blue bar graphs) and Leg tests (grey bar graphs) for the incremental test (A), the TTE test at 90% of PPOLeg (B), and the TTE test at 75% of PPOLeg (C). The open circles and the filled circles represent individual data during Arm+leg and Leg tests respectively. *p < 0.05 vs. Arm+leg.
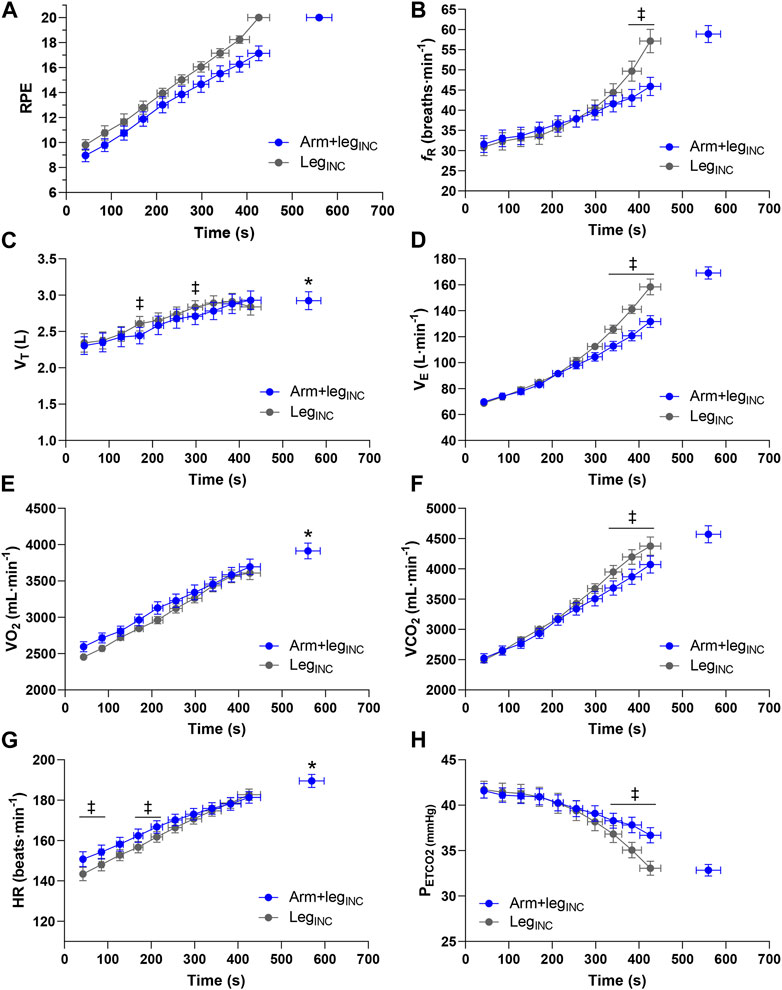
FIGURE 3. Group mean response of ratings of perceived exertion (A), respiratory frequency (B), tidal volume (C), minute ventilation (D), oxygen uptake (E), carbon dioxide output (F), heart rate (G) and end-tidal partial pressure of carbon dioxide (H) for Arm+legINC (blue circles) and LegINC (grey circles). ‡p < 0.05 vs. Arm+legINC, *p < 0.05 vs. LegINC.
3.2 TTE tests at 90% of PPOLeg
A significantly longer (p < 0.001; Cohen’s d = 2.27) TTE was found in Arm+legTTE90 (638 ± 154 s) vs. LegTTE90 (307 ± 67 s) (Figure 2B). The average relative contribution of the arms to the total power output was 22% ± 2% in the Arm+legTTE90 test. A higher pedaling cadence (p < 0.033) was found in the LegINC (83 ± 7 rpm) compared to that of the arms (73 ± 13 rpm) and legs (76 ± 6 rpm) of the Arm+legINC test. The two tests (Arm+legTTE90 vs. LegTTE90) showed significant differences (p < 0.034) in the end-test values of fR (59 ± 10 vs. 53 ± 9 breaths min−1),
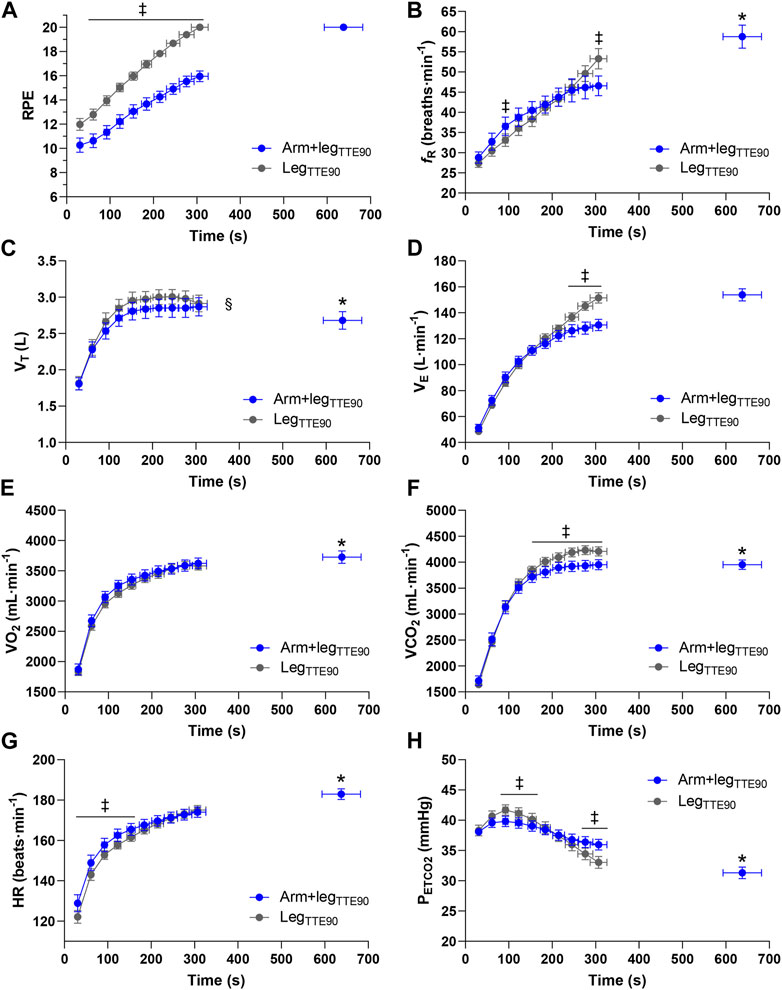
FIGURE 4. Group mean response of ratings of perceived exertion (A), respiratory frequency (B), tidal volume (C), minute ventilation (D), oxygen uptake (E), carbon dioxide output (F), heart rate (G) and end-tidal partial pressure of carbon dioxide (H) for Arm+legTTE90 (blue circles) and LegTTE90 (grey circles). ‡p < 0.05 vs. Arm+legTTE90, *p < 0.05 vs. LegTTE90, § main effect of condition.
3.3 TTE tests at 75% of PPOLeg
A significantly longer (p < 0.001; Cohen’s d = 1.53) TTE was found in Arm+legTTE75 (1,675 ± 525 s) vs. LegTTE75 (880 ± 363 s) (Figure 2C). The average relative contribution of the arms to the total power output was 21% ± 1% in the Arm+legTTE75 test. A higher pedaling cadence (p < 0.016) was found in the LegINC (80 ± 7 rpm) compared to that of the arms (73 ± 8 rpm) and legs (75 ± 6 rpm) of the Arm+legINC test. The two tests (Arm+legTTE75 vs. LegTTE75) also showed significant differences (p < 0.042) in the end-test values of HR (182 ± 10 vs. 175 ± 13 beats min−1) and VT (2.40 ± 0.30 vs. 2.56 ± 0.40 L), while p = 0.084 was found for the end-test values of
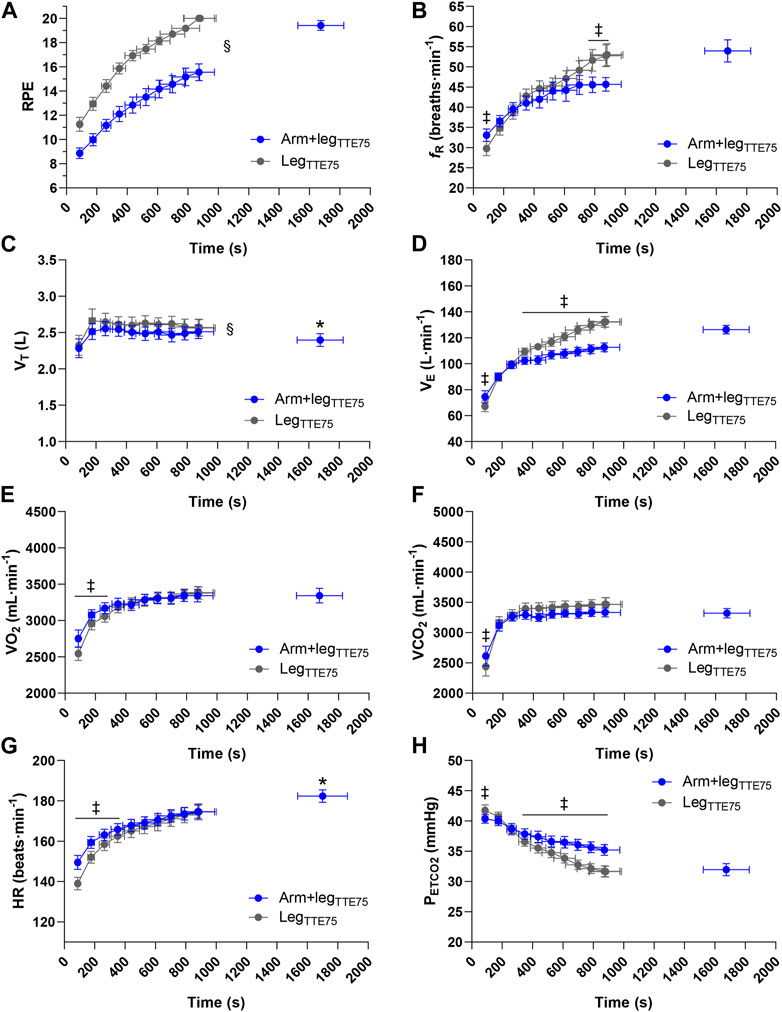
FIGURE 5. Group mean response of ratings of perceived exertion (A), respiratory frequency (B), tidal volume (C), minute ventilation (D), oxygen uptake (E), carbon dioxide output (F), heart rate (G) and end-tidal partial pressure of carbon dioxide (H) for Arm+legTTE75 (blue circles) and LegTTE75 (grey circles). ‡p < 0.05 vs. Arm+legTTE75, *p < 0.05 vs. LegTTE75, § main effect of condition.
3.4 The performance tests considered together
When the performance tests were considered together, a significant correlation was found between fR and RPE (p < 0.001; r = 0.75), HR and RPE (p < 0.001; r = 0.69), and
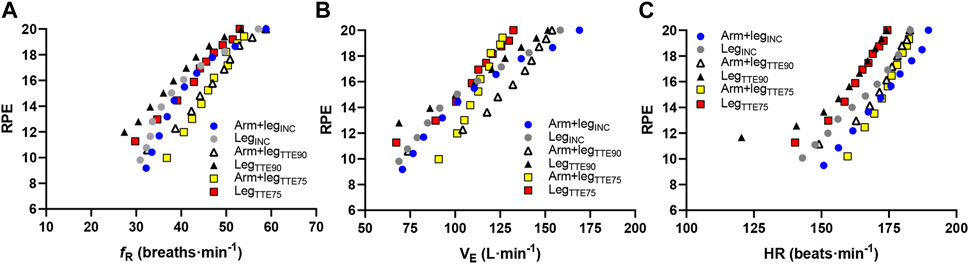
FIGURE 6. Correlations between RPE and respiratory frequency (A), RPE and minute ventilation (B), and RPE and heart rate (C) for the Arm+legINC (blue circles), LegINC (grey circles), Arm+legTTE90 (open triangles), LegTTE90 (black triangles), Arm+legTTE75 (yellow squares) and LegTTE75 (red squares). Each symbol represents the mean value of all participants at each percentage of the TTE.
Figure 7 shows the average response of the group when expressing
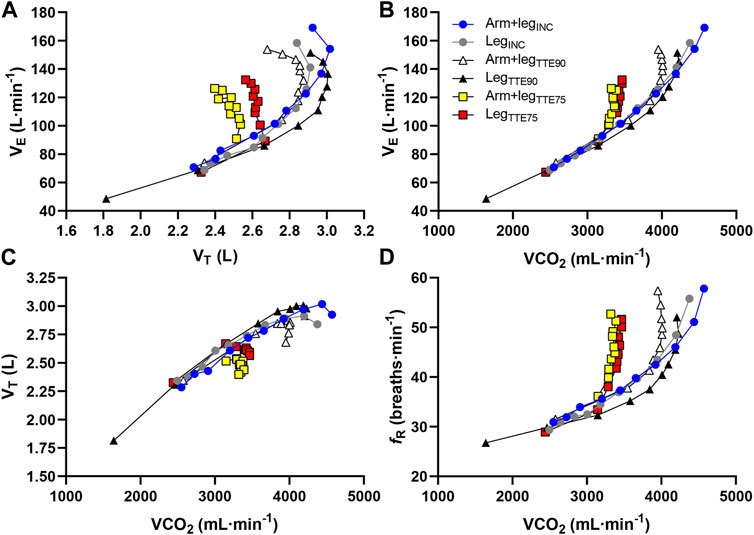
FIGURE 7. Relationship between minute ventilation and tidal volume (A), minute ventilation and carbon dioxide output (B), tidal volume and carbon dioxide output (C), and respiratory frequency and carbon dioxide output (D) for the Arm+legINC (blue circles), LegINC (grey circles), Arm+legTTE90 (open triangles), LegTTE90 (black triangles), Arm+legTTE75 (yellow squares) and LegTTE75 (red squares). Each symbol represents the mean value of all participants at each percentage of the TTE.
Figure 8 shows the individual responses of
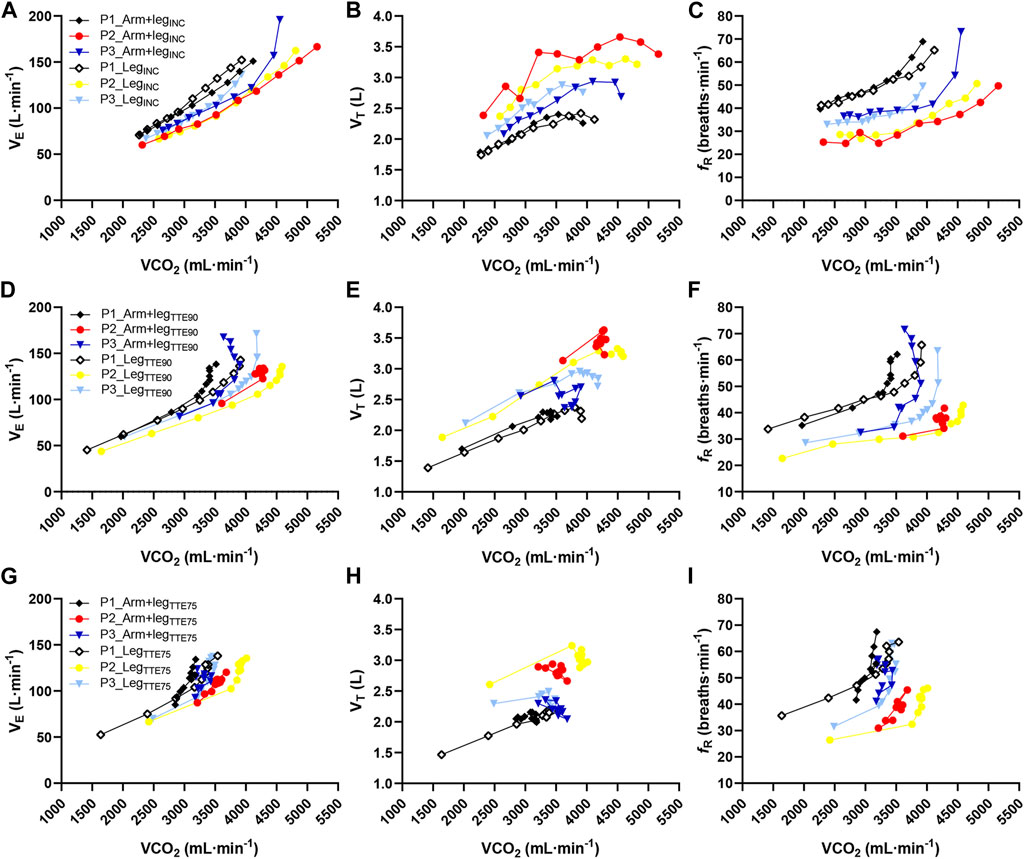
FIGURE 8. Relationship between minute ventilation and carbon dioxide output panels (A,D,G), tidal volume and
4 Discussion
This study aimed to systematically assess whether exercise tolerance improves with arm+leg cycling vs. leg cycling and whether fR and perceived exertion are sensitive to the expected differences in exercise tolerance. This goal was achieved by comparing the two exercise modalities using three performance tests and two exercise paradigms (i.e. incremental test and TTE test). The main findings of the study are as follows: 1) exercise tolerance was substantially improved in all the Arm+leg tests; 2) perceived exertion, minute ventilation and respiratory frequency were particularly sensitive to the between-modality changes in exercise tolerance observed, unlike
Our findings provide convincing evidence that arm+leg cycling substantially increases exercise tolerance when compared to (conventional) leg cycling, hence expanding on the limited literature dealing with performance differences between the two exercise modalities (Secher et al., 1974; Bergh et al., 1976; Nagle et al., 1984). The apparent difference in the percentage improvement in exercise tolerance found between the incremental tests and the TTE tests is, in fact, in line with the different characteristics of the two performance paradigms. Indeed, a 1% improvement in power output in an incremental test, or in a time trial, results in a performance improvement that can exceed 10% in a TTE test (Hopkins et al., 1999). Hence, the average increase in power output of about 15% that we found in the incremental test is compatible with the average increase in TTE found in the TTE tests (i.e. 108% in the Arm+legTTE90 and 90% in the Arm+legTTE75). Such an improvement in exercise tolerance observed in the Arm+leg tests implies that the effort required to sustain a given power output is substantially lower at isotime compared to that of the Leg tests, and this premise is supported by our findings.
The notion that physical effort was lower in the Arm+leg tests than in the Leg tests is substantiated by the lower values of perceived exertion and fR generally found at isotime. While the decrease in perceived exertion during incremental Arm+leg did not reach statistical significance (p = 0.057), it was substantial when comparing Arm+leg vs. Leg during the TTE tests. Indeed, perceived exertion was found to be among the most sensitive variables to variations in exercise tolerance during the TTE tests. These findings extend previous findings showing a lower RPE during arm+leg cycling vs. leg cycling at submaximal work rates (Hoffman et al., 1996; Hill et al., 2018). At isotime, fR was significantly lower (p < 0.003; ηP2 > 0.40) in the three Arm+leg tests than in the Leg tests, although this difference generally reached statistical significance in the last 20% of the Leg tests. This is an important feature of the fR response that might have been missed in previous studies that did not compare Arm+leg and Leg until exhaustion (Robertson et al., 1986). Likewise, the reduction in fR that is observed in the last part of a TTE test when exercise tolerance improves may not appear if the variability in TTE is not addressed on an individual basis when analyzing TTE data (Nicolò et al., 2019). We have overcome this problem by using the previously described “individual isotime” analysis (Nicolò et al., 2019), which reduces extensively the data loss that occurs when using more traditional analyses. Our findings collectively suggest that the time course of fR reflects changes in exercise tolerance both during incremental exercise and TTE exercise, thus supporting the study hypothesis.
The association found between fR and RPE and the sensitivity of fR to changes in exercise tolerance support a substantial modulation of fR by central command (Nicolò and Sacchetti, 2023). However, a partial dissociation was found between fR and RPE, which may suggest that also muscle afferent feedback contributed to the fR response. Although evidence suggests that muscle afferent feedback has a greater relative contribution to fR during moderate exercise than during high-intensity exercise (Amann et al., 2010; Dempsey et al., 2014; Girardi et al., 2021; Nicolò and Sacchetti, 2023), arm+leg cycling implies the simultaneous use of the muscle mass of both the upper and lower limbs, possibly resulting in a greater magnitude of muscle afferent feedback, and especially of its mechanosensitive component (i.e. mechanoreflex). This may explain the slightly higher fR shown in the first part of the TTE tests in the Arm+leg vs. the Leg modality. Indeed, it has been suggested that the relative contribution of muscle afferent feedback to ventilation is higher when the muscle mass recruited is larger (Amann et al., 2011; Dempsey et al., 2014; Nicolò and Sacchetti, 2023). Conversely, it is conceivable that the magnitude of the metabosensitive component of muscle afferent feedback (i.e. metaboreflex) was reduced at isotime in the Arm+leg tests because of the lower intramuscular metabolic perturbation. Hence, the metaboreflex cannot be ruled out as an input contributing to the decrease in fR observed in the Arm+leg tests. Nevertheless, the relative contribution of muscle afferent feedback may reduce over time during a TTE test because the contribution of other inputs increases substantially (e.g. central command) (Dempsey et al., 2014; Nicolò and Sacchetti, 2023). We cannot exclude that afferent feedback from pulmonary mechanoreceptors or alterations in chest wall mechanics might have contributed to the partial dissociation observed between the fR and RPE responses. Arm movements may increase the contribution to ventilation of afferent feedback from pulmonary mechanoreceptors or alter the mechanics of breathing, although these propositions require further investigation. Furthermore, it cannot be excluded that the lower pedaling cadence observed in the Arm+leg tests might have contributed to the between-modality differences observed in fR, although variations in pedaling cadence and fR might not be proportional, especially during high-intensity exercise (Girardi et al., 2021). Conversely, it is less plausible that metabolic acidosis or other metabolic inputs might have provided a substantial direct contribution to the fR modulation, and the reader is referred to previous studies where evidence supporting this proposition has been reviewed (Nicolò et al., 2020a; Nicolò and Sacchetti, 2023).
The ventilatory responses observed in this study can be interpreted in the light of a recently proposed model of ventilatory control during exercise, which suggests that fR and VT are modulated to a large extent by behavioral and metabolic inputs respectively (Nicolò and Sacchetti, 2023). While it was proposed that ventilation is differentially regulated during incremental exercise and TTE exercise (Syabbalo et al., 1994), our findings suggest that the breathing pattern is affected by the magnitude of the inputs modulating ventilation rather than by the type of exercise paradigm. Syabbalo et al. (1994) observed a more rapid and shallow breathing pattern during a TTE test at about 76% of the PPO reached in an incremental exercise than during this latter test. The lower VT reported by Syabbalo et al. (1994) during the TTE test is in line with the VT response observed during the TTE tests at 75% of the PPOLeg in this study. However, the breathing pattern we observed in the TTE tests at 90% of the PPOLeg was, conversely, more similar to that found during the incremental exercise than during the TTE tests at 75% of the PPOLeg (see Figure 7). Hence, the rapid and shallow breathing pattern is not a feature of TTE exercise, which is in contrast with what Syabbalo et al. (1994) had suggested. Conversely, the observed findings can be explained by the differential control of fR and VT (Nicolò et al., 2017a; Nicolò et al., 2018; Nicolò and Sacchetti, 2019; Nicolò and Sacchetti, 2023). While fR generally shows similar peak values during incremental exercise and constant work rate exercise (Syabbalo et al., 1994; Nicolò and Sacchetti, 2023), the VT peak reached during exercise is largely influenced by the magnitude of metabolic inputs and is generally associated with the
The association between
Individual responses further suggest that VT may not change proportionally to
The cardiocirculatory adjustments that occur when exercising simultaneously with the upper and lower limbs may provide further mechanistic support to the improvement in exercise tolerance observed in the Arm+leg modality. Arm+leg cycling may result in a greater peak cardiac output than Leg cycling (Secher et al., 1974; Reybrouck et al., 1975), and the higher
The fact that fR and
The between-modality comparison of exercise tolerance, perceived exertion and
It is worth mentioning that the Arm+leg modality poses some measurement challenges that we had to face in this study. First, we did not attempt to compute the GET and the RCP because of the relatively high initial stage of the incremental tests imposed by the ergometer (i.e. 150 W), which has limited their detection. As such, the TTE tests were not prescribed based on exercise intensity domains. Hence, especially in the Arm+legTTE75 test, it is possible that some participants were exercising in the severe intensity domain while others were in the heavy domain. Second, the measurement of blood lactate is very challenging during the Arm+leg modality, and we decided not to collect it to avoid interfering with the performance tests. Third, perceived exertion can only be rated verbally during the Arm+leg tests, thus generating breathing artifacts and affecting gas exchange measures. However, we used a filtering technique (Lamarra et al., 1987) that addressed this limitation and helped preserve the integrity of the physiological responses. Even the other limitations outlined were partially counteracted by the experimental design and the method of analysis used. Indeed, we performed a detailed between-modality comparison of the responses of some of the main physiological variables commonly used to compute the GET and the RCP. This comparison reveals a greater metabolic perturbation at isotime in the Leg tests, especially when considering the time courses of
5 Conclusion
This study shows that exercise tolerance is substantially higher in the Arm+leg modality than in the leg cycling modality. The average improvement in exercise tolerance was 15% in the incremental test and 108% and 90% in the TTE tests at 90% and 75% of PPOLeg respectively. Perceived exertion, minute ventilation and respiratory frequency were among the most sensitive variables to the improvement in exercise tolerance provided by the Arm+leg modality, hence suggesting that the common mechanism modulating these variables (i.e. central command) plays an important role in endurance performance. These findings reinforce the notion that respiratory frequency is a better marker of physical effort than oxygen uptake and heart rate. Our results have implications for devising exercise strategies to reduce perceived exertion while maintaining a high cardiometabolic demand, and for maximizing energy expenditure for the same level of effort exerted.
Data availability statement
The raw data supporting the conclusion of this article will be made available by the authors, without undue reservation.
Ethics statement
The studies involving human participants were reviewed and approved by Institutional Review Board of the University of Rome “Foro Italico.” The patients/participants provided their written informed consent to participate in this study.
Author contributions
AN, MS, and FF contributed to the conception and design of the study. MG performed data collection. MG and AN analyzed data. AN wrote the first draft of the manuscript. AN, MG, IB, MS, and FF contributed to data interpretation. All authors contributed to the article and approved the submitted version.
Funding
The study was funded by Harken Italy SPA (Limido Comasco, Como). AN was supported by a Post-Doc Research Grant funded by Harken Italy SPA (Limido Comasco, Como). The article publication fees were provided by the University of Rome “Foro Italico” (CDR2. BANDO2022NA).
Acknowledgments
The authors would like to thank Andrea Merello and Michele Cazzaro from Harken Italy SPA for their enthusiasm and valuable help in the different stages of the project.
Conflict of interest
The authors declare that the research was conducted in the absence of any commercial or financial relationships that could be construed as a potential conflict of interest.
Publisher’s note
All claims expressed in this article are solely those of the authors and do not necessarily represent those of their affiliated organizations, or those of the publisher, the editors and the reviewers. Any product that may be evaluated in this article, or claim that may be made by its manufacturer, is not guaranteed or endorsed by the publisher.
Abbreviations
Arm+leg, arm+leg cycling; Arm+legINC, arm+leg cycling incremental test; Arm+legTTE75, Arm+leg TTE test at 75% of PPOLeg; Arm+legTTE90, Arm+leg TTE test at 90% of PPOLeg; fR, respiratory frequency; GET, gas exchange threshold; HR, heart rate; Leg, leg cycling; LegINC, leg cycling incremental test; LegTTE75, Leg TTE test at 75% of PPOLeg; LegTTE90, Leg TTE test at 90% of PPOLeg; PETCO2, end-tidal partial pressure of carbon dioxide; PPO, Peak power output; PPOLeg, peak power output reached in the LegINC; RCP, respiratory compensation point; RPE, ratings of perceived exertion; TTE, time-to-exhaustion;
References
Amann, M., Blain, G. M., Proctor, L. T., Sebranek, J. J., Pegelow, D. F., and Dempsey, J. A. (2010). Group III and IV muscle afferents contribute to ventilatory and cardiovascular response to rhythmic exercise in humans. J. Appl. Physiol. 109, 966–976. doi:10.1152/japplphysiol.00462.2010
Amann, M., Runnels, S., Morgan, D. E., Trinity, J. D., Fjeldstad, A. S., Wray, D. W., et al. (2011). On the contribution of group III and IV muscle afferents to the circulatory response to rhythmic exercise in humans. J. Physiology 589, 3855–3866. doi:10.1113/jphysiol.2011.209353
Astrand, P. O., and Saltin, B. (1961). Maximal oxygen uptake and heart rate in various types of muscular activity. J. Appl. Physiol. 16, 977–981. doi:10.1152/jappl.1961.16.6.977
Bergevin, M., Steele, J., Payen de la Garanderie, M., Feral-Basin, C., Marcora, S. M., Rainville, P., et al. (2023). Pharmacological blockade of muscle afferents and perception of effort: A systematic review with meta-analysis. Sports Med. 53, 415–435. doi:10.1007/s40279-022-01762-4
Bergh, U., Kanstrup, I. L., and Ekblom, B. (1976). Maximal oxygen uptake during exercise with various combinations of arm and leg work. J. Appl. Physiol. 41, 191–196. doi:10.1152/jappl.1976.41.2.191
Bland, J. M., and Altman, D. G. (1995). Calculating correlation coefficients with repeated observations: Part 1-Correlation within subjects. BMJ 310, 446. doi:10.1136/bmj.310.6977.446
Borg, G. (1998). Borg’s perceived exertion and pain scales. Hum. Kinet. 30, 1461. doi:10.1249/00005768-199809000-00018
Cheval, B., and Boisgontier, M. P. (2021). The theory of effort minimization in physical activity. Exerc Sport Sci. Rev. 49, 168–178. doi:10.1249/JES.0000000000000252
Cotes, J. E., Allsopp, D., and Sardi, F. (1969). Human cardiopulmonary responses to exercise: Comparisons between progressive and steady state exercise, between arm and leg exercise, and between subjects differing in body weight. Q. J. Exp. Physiol. Cogn. Med. Sci. 54, 211–222. doi:10.1113/expphysiol.1969.sp002019
Dempsey, J. A., Blain, G. M., and Amann, M. (2014). Are type III-IV muscle afferents required for a normal steady-state exercise hyperpnoea in humans? J. Physiology 592, 463–474. doi:10.1113/jphysiol.2013.261925
Girardi, M., Nicolò, A., Bazzucchi, I., Felici, F., and Sacchetti, M. (2021). The effect of pedalling cadence on respiratory frequency: Passive vs. active exercise of different intensities. Eur. J. Appl. Physiol. 121, 583–596. doi:10.1007/s00421-020-04533-z
Gleser, M. A., Hoestman, D. H., and Mello, R. P. (1974). The effect on
Haouzi, P., and Bell, H. J. (2009). Control of breathing and volitional respiratory rhythm in humans. J. Appl. Physiol. 106, 904–910. doi:10.1152/japplphysiol.90675.2008
Haouzi, P. (2014). Tracking pulmonary gas exchange by breathing control during exercise: Role of muscle blood flow. J. Physiology 592, 453–461. doi:10.1113/jphysiol.2013.261396
Hill, M., Talbot, C., Puddiford, M., and Price, M. (2018). Cardiorespiratory and perceptual responses to self-regulated and imposed submaximal arm–leg ergometry. Eur. J. Appl. Physiol. 118, 1011–1019. doi:10.1007/s00421-018-3838-7
Hoffman, M. D., Kassay, K. M., Zeni, A. I., and Clifford, P. S. (1996). Does the amount of exercising muscle alter the aerobic demand of dynamic exercise? Eur. J. Appl. Physiol. Occup. Physiol. 74, 541–547. doi:10.1007/BF02376770
Hopkins, W. G., Hawley, J. A., and Burke, L. M. (1999). Design and analysis of research on sport performance enhancement. Med. Sci. Sports Exerc 31, 472–485. doi:10.1097/00005768-199903000-00018
Itoh, M., Fukuoka, Y., Grassi, B., Marconi, C., Cerretelli, P., Araki, H., et al. (2002). VE response to VCO2 during exercise is unaffected by exercise training and different exercise limbs. Jpn. J. Physiology 52, 489–496. doi:10.2170/jjphysiol.52.489
Jensen, J. I., Lyager, S., and Pedersen, O. F. (1980). The relationship between maximal ventilation, breathing pattern and mechanical limitation of ventilation. J. Physiol. 309, 521–532. doi:10.1113/jphysiol.1980.sp013524
Kennard, C. D., and Martin, B. J. (1984). Respiratory frequency and the oxygen cost of exercise. Eur. J. Appl. Physiol. Occup. Physiol. 52, 320–323. doi:10.1007/BF01015218
Kitamura, K., Yamaji, K., and Shephard, R. J. (1981). Heart rate predictions of exercise intensity during arm, leg and combined arm/leg exercise. J. Hum. Ergol. (Tokyo) 10, 151–160.
Kokkinos, P., Myers, J., Faselis, C., Panagiotakos, D. B., Doumas, M., Pittaras, A., et al. (2010). Exercise capacity and mortality in older men: A 20-year follow-up study. Circulation 122, 790–797. doi:10.1161/CIRCULATIONAHA.110.938852
Lamarra, N., Whipp, B. J., Ward, S. A., and Wasserman, K. (1987). Effect of interbreath fluctuations on characterizing exercise gas exchange kinetics. J. Appl. Physiol. 62, 2003–2012. doi:10.1152/jappl.1987.62.5.2003
Lamb, T. W., Anthonisen, N. R., and Tenney, S. M. (1965). Controlled frequency breathing during muscular exercise. J. Appl. Physiol. 20, 244–248. doi:10.1152/jappl.1965.20.2.244
Louhevaara, V., Sovijarvi, A., Ilmarinen, J., and Teraslinna, P. (1990). Differences in cardiorespiratory responses during and after arm crank and cycle exercise. Acta Physiol. Scand. 138, 133–143. doi:10.1111/j.1748-1716.1990.tb08825.x
Marcora, S. (2009). Perception of effort during exercise is independent of afferent feedback from skeletal muscles, heart, and lungs. J. Appl. Physiol. 106, 2060–2062. doi:10.1152/japplphysiol.90378.2008
Massaroni, C., Nicolò, A., Lo Presti, D., Sacchetti, M., Silvestri, S., and Schena, E. (2019). Contact-based methods for measuring respiratory rate. Sensors 19, 908. doi:10.3390/s19040908
Nagle, F. J., Richie, J. P., and Giese, M. D. (1984).
Nesti, L., Pugliese, N. R., Sciuto, P., and Natali, A. (2020). Type 2 diabetes and reduced exercise tolerance: A review of the literature through an integrated physiology approach. Cardiovasc Diabetol. 19, 134. doi:10.1186/s12933-020-01109-1
Nicolò, A., Bazzucchi, I., Haxhi, J., Felici, F., and Sacchetti, M. (2014). Comparing continuous and intermittent exercise: An “isoeffort” and “isotime” approach. PLoS One 9, e94990. doi:10.1371/journal.pone.0094990
Nicolò, A., Girardi, M., Bazzucchi, I., Felici, F., and Sacchetti, M. (2018). Respiratory frequency and tidal volume during exercise: Differential control and unbalanced interdependence. Physiol. Rep. 6, e13908. doi:10.14814/phy2.13908
Nicolò, A., Marcora, S. M., Bazzucchi, I., and Sacchetti, M. (2017a). Differential control of respiratory frequency and tidal volume during high-intensity interval training. Exp. Physiol. 102, 934–949. doi:10.1113/EP086352
Nicolò, A., Marcora, S. M., and Sacchetti, M. (2016). Respiratory frequency is strongly associated with perceived exertion during time trials of different duration. J. Sports Sci. 34, 1199–1206. doi:10.1080/02640414.2015.1102315
Nicolò, A., Marcora, S. M., and Sacchetti, M. (2020a). Time to reconsider how ventilation is regulated above the respiratory compensation point during incremental exercise. J. Appl. Physiol. 128, 1447–1449. doi:10.1152/japplphysiol.00814.2019
Nicolò, A., Massaroni, C., and Passfield, L. (2017b). Respiratory frequency during exercise: The neglected physiological measure. Front. Physiol. 8, 922. doi:10.3389/fphys.2017.00922
Nicolò, A., Massaroni, C., Schena, E., and Sacchetti, M. (2020b). The importance of respiratory rate monitoring: From healthcare to sport and exercise. Sensors Switz. 20, 6396. doi:10.3390/s20216396
Nicolò, A., and Sacchetti, M. (2019). A new model of ventilatory control during exercise. Exp. Physiol. 104, 1331–1332. doi:10.1113/EP087937
Nicolò, A., and Sacchetti, M. (2023). Differential control of respiratory frequency and tidal volume during exercise. Eur. J. Appl. Physiol. 123, 215–242. doi:10.1007/s00421-022-05077-0
Nicolò, A., Sacchetti, M., Girardi, M., McCormick, A., Angius, L., Bazzucchi, I., et al. (2019). A comparison of different methods to analyse data collected during time-to-exhaustion tests. Sport Sci. Health 15, 667–679. doi:10.1007/s11332-019-00585-7
Ohashi, S., Izumizaki, M., Atsumi, T., and Homma, I. (2013). CO2 homeostasis is maintained in conscious humans by regulation of tidal volume, but not of respiratory rhythm. Respir. Physiol. Neurobiol. 186, 155–163. doi:10.1016/j.resp.2013.01.008
Reybrouck, T., Heigenhauser, G. F., and Faulkner, J. A. (1975). Limitations to maximum oxygen uptake in arms, leg, and combined arm-leg ergometry. J. Appl. Physiol. 38, 774–779. doi:10.1152/jappl.1975.38.5.774
Robertson, R. J., Falkel, J. E., Drash, A. L., Swank, A. M., Metz, K. F., Spungen, S. A., et al. (1986). Effect of blood pH on peripheral and central signals of perceived exertion. Med. Sci. Sports Exerc 18, 114–122. doi:10.1249/00005768-198602000-00019
Secher, N. H., Clausen, J. P., Klausen, K., Noer, I., and Trap-Jensen, J. (1977). Central and regional circulatory effects of adding arm exercise to leg exercise. Acta Physiol. Scand. 100, 288–297. doi:10.1111/j.1748-1716.1977.tb05952.x
Secher, N. H., Ruberg Larsen, N., Binkhorst, R. A., and Bonde Petersen, F. (1974). Maximal oxygen uptake during arm cranking and combined arm plus leg exercise. J. Appl. Physiol. 36, 515–518. doi:10.1152/jappl.1974.36.5.515
Secher, N. H., and Volianitis, S. (2006). “Are the arms and legs in competition for cardiac output?,” in Medicine and science in sports and exercise. doi:10.1249/01.mss.0000230343.64000.ac
Syabbalo, N. C., Krishnan, B., Zintel, T., and Gallagher, C. G. (1994). Differential ventilatory control during constant work rate and incremental exercise. Respir. Physiol. 97, 175–187. doi:10.1016/0034-5687(94)90024-8
Van De Walle, G. P., and Vukovich, M. D. (2018). The effect of nitrate supplementation on exercise tolerance and performance: A systematic review and meta-analysis. J. Strength Cond. Res. 32, 1796–1808. doi:10.1519/jsc.0000000000002046
Vokac, Z., Bell, H., Bautz Holter, E., and Rodahl, K. (1975). Oxygen uptake/heart rate relationship in leg and arm exercise, sitting and standing. J. Appl. Physiol. 39, 54–59. doi:10.1152/jappl.1975.39.1.54
Keywords: endurance performance, breathing control, respiratory frequency, incremental test, time to exhaustion, fatigue, breathing pattern, oxygen uptake
Citation: Nicolò A, Girardi M, Bazzucchi I, Sacchetti M and Felici F (2023) Ventilation and perceived exertion are sensitive to changes in exercise tolerance: arm+leg cycling vs. leg cycling. Front. Physiol. 14:1226421. doi: 10.3389/fphys.2023.1226421
Received: 21 May 2023; Accepted: 20 July 2023;
Published: 01 August 2023.
Edited by:
Silvia Pogliaghi, University of Verona, ItalyCopyright © 2023 Nicolò, Girardi, Bazzucchi, Sacchetti and Felici. This is an open-access article distributed under the terms of the Creative Commons Attribution License (CC BY). The use, distribution or reproduction in other forums is permitted, provided the original author(s) and the copyright owner(s) are credited and that the original publication in this journal is cited, in accordance with accepted academic practice. No use, distribution or reproduction is permitted which does not comply with these terms.
*Correspondence: Andrea Nicolò, YW5kcmVhLm5pY29sb0B1bmlyb21hNC5pdA==
†ORCID: Andrea Nicolò, orcid.org/0000-0002-4716-1667; Michele Girardi, orcid.org/0000-0001-8102-7384; Ilenia Bazzucchi, orcid.org/0000-0002-6017-1683; Massimo Sacchetti, orcid.org/0000-0002-5365-5161; Francesco Felici, orcid.org/0000-0002-7228-9064