- 1SantéAgri, French Biodiversity Agency (OFB), Orléans & Birieux, France
- 2Consultant, Pluvigner, France
- 3UMR 8025, Centre d’Histoire Judiciaire, University of Lille, Lille, France
- 4Consultant, Andenne, Belgium
Recent awareness of the role of wildlife in the evolution of emerging zoonotic diseases emphasizes the needs to conduct surveillance for public health. Additionally, wildlife surveillance is motivated by animal health, conservation and biodiversity perspectives. Event-based wildlife surveillance involves studying mortality and investigating its causes. Carcass detection thus plays a key role in the surveillance of high-risk diseases. Many factors influence the degradation of a carcass, particularly environmental conditions, the biology and behavior of the species, and the role played by necrophagous insects and scavengers. Various tools and technologies have been tested over the years to improve wildlife carcass detection. Here, we review the main factors that influence carcass detectability and detection in wildlife surveillance and management, alongside the strengths and limitations of key innovative detection tools: detection dogs, drones and thermal imaging. We also list decision criteria to help wildlife surveillance managers and researchers understand and select the targeted search approaches most likely to optimize carcass encounter and recovery during disease outbreaks.
Introduction
Wildlife carcasses may hold important epidemiological information (e.g., whether certain species are affected by or predisposed to a disease, or the spatio-temporal distribution of the disease). Carcasses are often sought as part of epidemiological surveillance efforts since collection and analysis are frequently carried out for diagnostic purposes. Indeed, unusual mortality events in wild animal populations may provide early warning of an emerging threat to the health of wild or domestic animal (Morner et al., 2002). Carcass removal is also part of disease and mortality management. When toxins or pollutants are present, removal may prevent secondary intoxication of scavengers, e.g., from lead or veterinary drugs exposure. For health risk management, carcass removal helps to limit the spread of various pathogens such as African swine fever (ASF) or highly pathogenic avian influenza, or to prevent chain reaction propagation as with the carcass-maggot cycle of botulism (Bollinger et al., 2011; Lange et al., 2018; Pearce-Higgins et al., 2023). However, carcasses are also a fundamental food source for numerous macro- and micro-scavengers. Such species provide a suite of ecosystem services beneficial to humans and wildlife. Therefore, the decision to remove carcasses must always be in equilibrium between potential risks of pathogens spread, the preservation and sustenance requirements of endemic scavengers that depend on this resource, and overall risk to the environment.
In several countries, general and continuous health monitoring includes surveillance of mortality or morbidity events. Such event-based surveillance is sometimes organized through national or regional networks (Kuiken et al., 2011; Cardoso et al., 2022). The sustainability of this type of surveillance network relies on the balance between its early detection capability, and its costs/the efforts it needs (Balajee et al., 2021). The first level of surveillance in a participatory network relies on opportunistic and therefore non-homogeneous sampling. This sampling results in high variability in carcass detection, reporting and collection according to the risk of spread and the challenges posed by the disease. Surveillance could be gradually reinforced by strengthening criteria for reporting, collection and analysis. In a high-risk context, detection can be improved by setting up targeted carcass searches.
Many factors can influence the detectability and the detection of wildlife carcasses in the environment. Detectability, i.e., the probability of a carcass being located (Santos et al., 2016), strongly influences the efficacy of wildlife health surveillance as well as management efficiency. Broadly speaking, during assessments of pesticide-related avian mortality in agricultural systems, and as part of West Nile surveillance efforts, respectively, carcass detectability was found to depend on 1) distribution in the environment, 2) speed of decomposition and 3) the ability of a given observer to find it (Mineau and Collins, 1988; Ward et al., 2006). Detection is strongly influenced by the search effort including protocol designs, human specificity and tools and technologies (Figure 1). Here, we review factors influencing detectability and detection and focus on tools and technologies enhancing detection despite detectability constraints. The effect of protocol designs is well documented elsewhere, and was not included in this review, see for example (Rivera-Milán et al., 2004; Barrientos et al., 2018). Finally, we have listed criteria to help with the choice of the most suitable tools and technologies in light of the environmental context and goals (Figure 2).
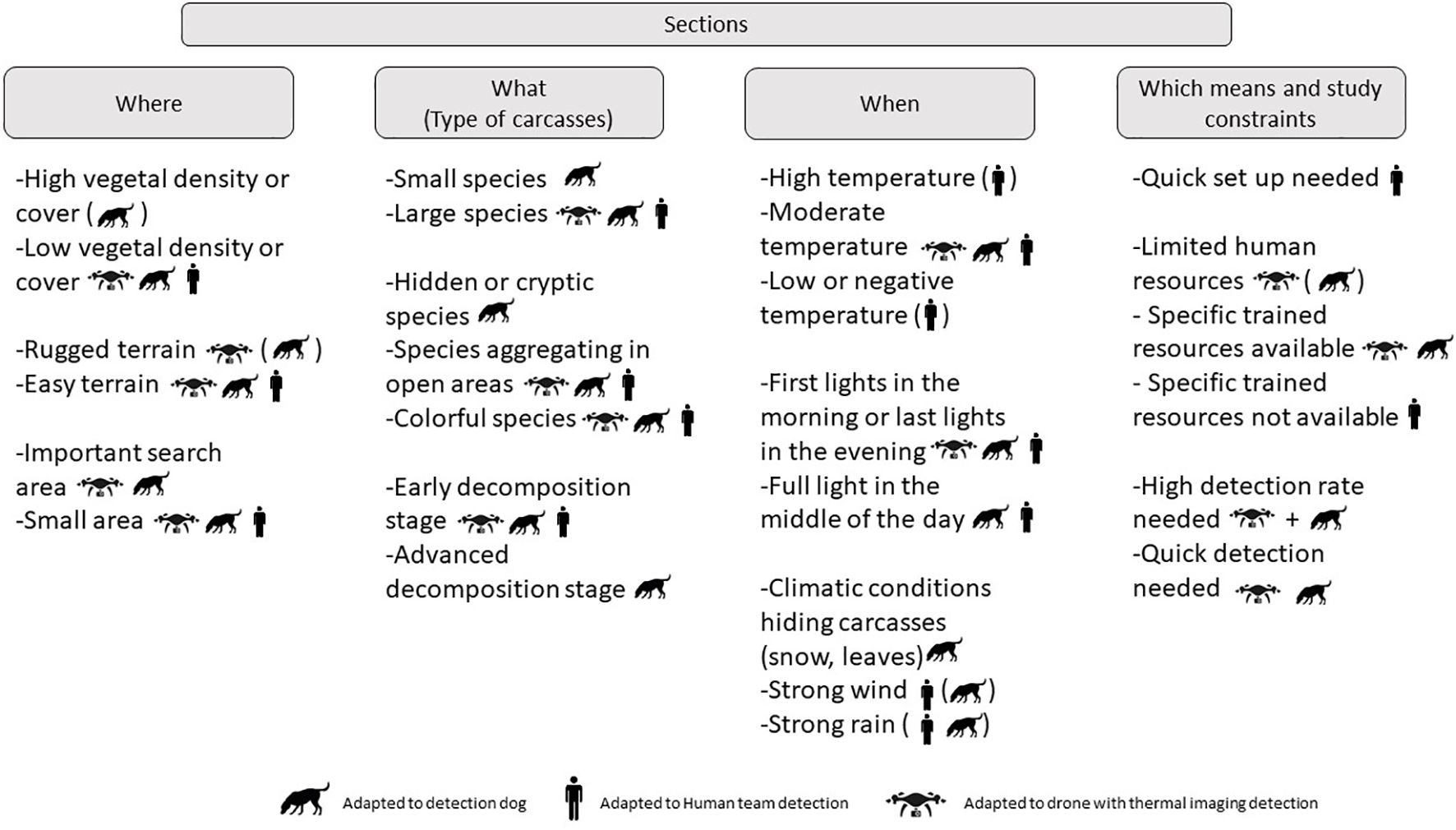
Figure 2. Decision criteria influencing the choice of the most adapted tools for wildlife carcass detection.
Factors influencing carcass detection
Population, species and environmental characteristics
Successful investigation of any mortality event across the various natural environments inhabited by wild animals will hinge on an understanding of the biology and behavior of the affected species. For example, the significance of the location at which a carcass is found must be considered in relation to factors like the animal’s home range, distribution and seasonal movements. The density of animal populations and variation of densities between species in a given environment may influence carcass detection rate (Mineau and Collins, 1988; Morner et al., 2002). Unusual mortality outbreak events tend to be more visible and easily detected in species that aggregate in large numbers, for example at feeding sites, in grasslands or in wetlands. In contrast, the carcasses of animals that conceal themselves, such as rodents living and dying in burrows, or that occur in remote or otherwise inaccessible areas, may inherently be more difficult to detect (Morner et al., 2002). Vegetation complexity also reduces the visibility of carcasses, which are easier for observers to see in open areas than in dense forests and tall grass or bushes (Morner et al., 2002; Peters et al., 2014; Borner et al., 2017; Zimmerman et al., 2019).
Carcass persistence in the environment
All other things being equal, the longer a carcass remains in the environment, the higher the probability of its detection (Henry et al., 2021). However, the process of decomposition can also make a carcass less conspicuous and, hence, reduce detectability. The stages and evolution of animal decomposition depend on endogenous factors such as the size of the animal or the composition and abundance of the necrobiome. Exogenous factors such as climatic parameters or macro-scavenger and necrophagous insect activity may also exert a strong influence on the decomposition process (Zhou, 2011) (Figure 1).
Influence of endogenous factors
Small carcasses degrade more quickly in the environment, and/or are consumed or more readily hidden by other species (Spicka et al., 2011; Santos et al., 2016; Henry et al., 2021). For instance, some silphid beetles bury small birds and rodent carcasses to conceal them from other scavenger competitors, in turn affecting persistence and detectability (Trumbo and Sikes, 2021). Decomposition is accelerated in the presence of lesions which offer an entry point for necrophagous microorganisms, or when an ante mortem infectious process involves a high bacterial load (Zhou, 2011; Whittington, 2019). Conversely, bleeding and dehydration can delay putrefaction. Indeed, these phenomena result in poorer distribution of bacteria in the organism through fluids and deficiency in proteins which is a source of nutrients for bacteria (Zhou, 2011).
Influence of exogenous factors
Environmental (water, soil) and climatic parameters (humidity and temperature) can significantly influence carcass decomposition. Higher temperatures and direct sun usually result in faster rates of carcass decomposition, due to a corresponding increase of insect and microbial activity (Carter et al., 2009; Meyer et al., 2013; Santos et al., 2016; Probst et al., 2020). Generally, moist soils encourage micro-organism presence and hasten decomposition. However, degradation can instead be slowed by extreme moisture conditions (Carter et al., 2010). Cold and humidity along with lack of oxygen may lead to the formation of adipocere - i.e., a waxy substance derived from body fat, as decomposition progresses. Conversely, a dry atmosphere with warm air and air circulation promotes desiccation and mummification (Fiedler and Graw, 2003). In the special case of submerged carcasses there is a dynamic interaction between time since death, the forces conferred by the aquatic medium, and the process of decomposition – all of which influence detectability in the sense that floating carcasses are more observable than those sinking. For marine mammals, the position of the carcass in the water column depends on location of death (at depth, on the surface, near the shoreline), and on temperature, currents, pressures and tides (see e.g., Moore et al., 2020).
In open air conditions, necrophagous insects are often deemed the most influential of factors in carcass decomposition - regardless of environment, species, climatic conditions or body size (Simmons et al., 2010). In parallel, the activity and abundance of necrophagous insects is heavily influenced by climatic factors. It is noteworthy that the presence of toxic substances can alter the speed at which necrophagous insects degrade a carcass. An example of this would be a carcass laced with pesticides for use as bait (Dekeirsschieter et al., 2009; Fernández Verón et al., 2021).
Abundance, diversity and seasonal variation in activity of scavenger species and their interactions, such as competition, attraction but also cooperation, may all influence the location and degradation of carcasses (Guinard et al., 2015; Olson et al., 2016; Orr et al., 2019; Hallingstad et al., 2023). Studies in Poland and Spain reported on a wide diversity of species - both diurnal and nocturnal - congregating around carcasses (Selva et al., 2005; Moleón et al., 2017). No distinction was made, however, between specific feeding activity or more exploratory behavior. For example, a wild boar may interact with and disturb a carcass without expressly consuming it (Probst et al., 2017). Scavengers select carcasses on the basis of their diet, ease of consumption based on decomposition stage, as well as extrinsic factors and behavioral adaptations (Selva et al., 2005; Guinard et al., 2015; Olson et al., 2016). For perspective, an average of two to three is estimated days before roadside carcasses (‘roadkill’) of all species combined are moved, consumed or degraded (Ward et al., 2006; Teixeira et al., 2013; Santos et al., 2016).
Human specificity
Human observers and surveyors are not equally equipped in terms of the motivation, focus and hearing, sight or olfactory senses they might draw upon when searching for carcasses. Some authors have recommended training sessions and standardized procedures to reduce the observer effect on rate of detection (Rivera-Milán et al., 2004). As an example, training and experience can lead to higher probability of detection for human remains (Studebaker-Reed, 2018). Fatigue could also influence focus and should be considered. The human visual observation also depends on species phenotype. For example, the detectability and corresponding detection rate for the carcasses of small species, like amphibians, may be lower (Hels and Buchwald, 2001; Morner et al., 2002; Peters et al., 2014; Borner et al., 2017; Zimmerman et al., 2019; Domínguez del Valle et al., 2020; Kitano et al., 2023). Conversely, bigger, brighter or more colorful species are more conspicuous (Philibert et al., 1993; Linz et al., 2006; Ward et al., 2006). Search strategies and efforts must therefore be specially adapted for the constraints posed by small(er) carcasses, which may involve various approaches, tools and technologies to increase their detection.
In summary, parameters influencing wildlife carcass detection are multiple and complex. Some factors only affect the detectability, but others influence the search effort too. For example, environmental or species characteristics influence not only the degradation of the carcass, but also the feasibility of an observer finding it. These differences in species and environmental factors preclude making overarching recommendations to improve detection. Instead, constraints and goals specific to the study and/or search have to be considered. Understanding factors influencing detectability and detection (Figure 1) is essential to the success of the study design. This knowledge also facilitates selecting the tools and technologies most appropriate to enhancing the detection of wildlife carcasses (see Figure 2).
Innovative detection tools and technologies to facilitate carcass detection
The human detection rate for wildlife carcasses can range widely (e.g., from 2.5 to 42%) depending on protocol designs and human specificity (Rivera-Milán et al., 2004; Paula et al., 2011; Mathews et al., 2013; Domínguez del Valle et al., 2020; Hansen and Winje, 2021). This detection rate can be improved by technologies and innovative application of tools like detection dog team surveys and thermal imaging or drone usage. Different protocols exist to organize observer searches and thereby standardize performance parameters, including walking along a transect line or driving/walking along roadsides to find roadkill carcasses (Ratti et al., 1983; Rivera-Milán et al., 2004; Collinson et al., 2014). Notably, a 1.7 to 17-fold higher detection rate was achieved when observers surveyed on foot rather than from cars (Teixeira et al., 2013).
Important considerations associated with observer searches, relative to costs, include: the time spent in the field and the required frequency or number of searchers to locate a representative proportion of carcasses (MacKenzie et al., 2002; Bailey et al., 2004; Bergman et al., 2020; Licoppe et al., 2023). Consider that a human team searched for desert tortoise (size of a beach ball) for an average of 8.52 hours per day, with an average daily linear distance of 15 km (Nussear et al., 2008). Depending on the type of animal carcass sought and the terrain, these parameters might be inconsistent with swiftly finding and recovering the requisite proportion of carcasses across large areas. If human searchers alone might prove insufficient to detect a large proportion of carcasses very quickly or from a specific decomposition stage, the search may require more effective or additionally complimentary detection tools. Yet reliability and augmented efficacy must still be paired with cost-efficiency. In the following section, we will review the technical aspects, efficiency, limitations and costs of certain innovative tools that could be useful in increasing carcass detection.
Carcass detection dog teams
Efficiency
The olfactory prowess of dogs (Canis lupus familiaris) has been harnessed for various ecological detection targets including: cadavers, wildlife fecal matter (aka ‘scat’) and other signs, as well as illegally poisoned carcasses or baits (Duarte et al., 2017; Deák et al., 2021a). Dogs have been assessed for their capability to detect the volatile organic compounds (VOCs) released by a carcass during its decomposition (Dargan and Forbes, 2021), (Figure 3). Detection dog teams are reportedly 2 to 10 times more effective than observers in detecting bird, bat and sheep carcasses (Table 1) (Homan et al., 2001; Arnett, 2006; Paula et al., 2011; Mathews et al., 2013; Domínguez del Valle et al., 2020; Hansen and Winje, 2021; Reynolds et al., 2021). These teams have also proven effective during searches for carcasses of wildlife species that fall victim to agricultural mowing in different vegetation types (Deák et al., 2021b). An important nuance is the resulting increase in overall detection rate versus augmentation of any likelihood of making a find. In this regard, dog-handler teams are often able to find carcasses which were not (or might not otherwise be) found by humans at all, even if individual variations in performance are observed among the teams or dogs (Hansen and Winje, 2021).
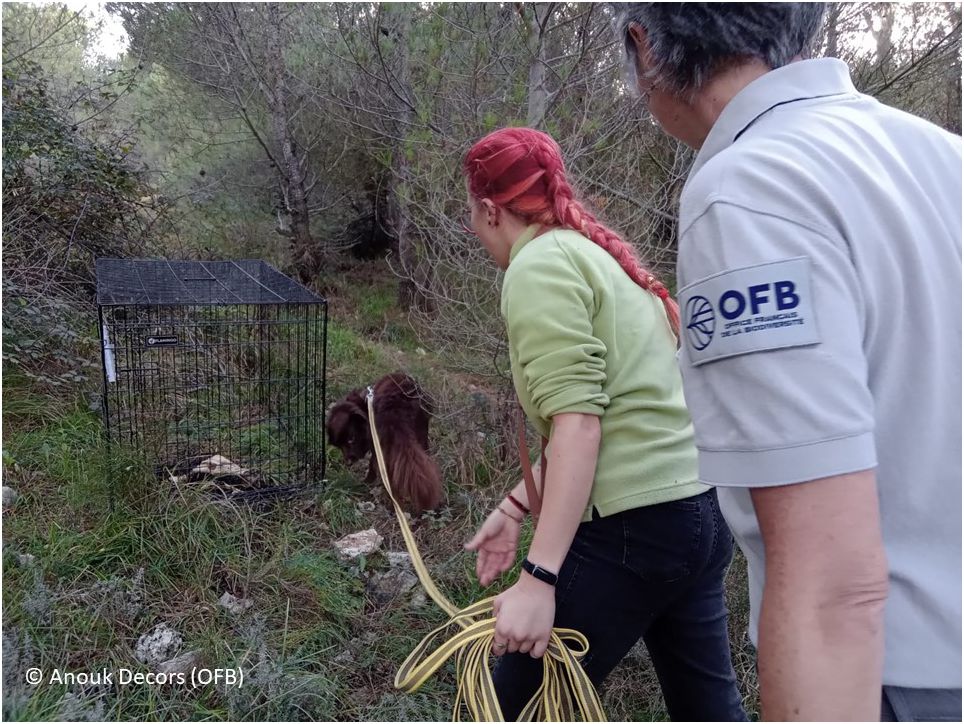
Figure 3. Detection dog and handler during a training session with the French biodiversity agency (OFB) for the SAGIR network. A decomposed wild boar is placed in a cage for training purpose © Anouk Decors (OFB).
There is an additional value of enlisting detection dog teams for carcass searches. Beyond their ability to find quantitatively more carcasses whether concealed or in more open areas (Table 1), dogs have demonstrated the ability to detect carcasses in recent and advanced decay stages. This includes the bones stage, even if the lack of tissue reduces the intensity of VOCs emitted, which increases the difficulty of detection (Dargan and Forbes, 2021). Dogs are also able to detect small animals like newts (Grimm-Seyfarth, 2022; Glover et al., 2023), and discriminate amongst individuals or in presence/absence of disease (Browne et al., 2006; Beebe et al., 2016; Hag-Ali et al., 2021). Note that the efficacy of detection dogs for carcass searches is not always defined in the same way, or reported using the same parameters, among studies. Measurement of the “detection rate” depends to a certain extent on the goal of the studies, which can make it challenging to compare and evaluate performance parameters in a systematic way (McKeague et al., 2024) without taking into account the differences in reported variables and search parameters, among other things. This does not fundamentally change the fact that detection dog teams represent a valuable and effective tool with which to improve wildlife carcass detection.
Influence factors and technical limits
Some types of challenging environments, like tall and dense vegetation, may reduce dog detection performance (Paula et al., 2011; Mathews et al., 2013; Domínguez del Valle et al., 2020, 2020). These require greater physical effort which increases panting and can in turn lead to declining concentration. However, the performance of detection dogs appears to be little to not affected by the duality of small size of carcasses and density of vegetation, in contrast to human searchers (Homan et al., 2001; Arnett, 2006; Paula et al., 2011; Domínguez del Valle et al., 2020; Hansen and Winje, 2021; Reynolds et al., 2021).
Odor dispersion, temperature, humidity and wind conditions could affect a dog’s olfactive capacities (Arnett, 2006; Paula et al., 2011; Mathews et al., 2013; Hansen and Winje, 2021). Increasing temperature correspondingly increases tiredness and above all, panting. Dogs cannot pant and sniff at the same time, so their detection capacity may be reduced in this condition (Homan et al., 2001; Gazit and Terkel, 2003; Mathews et al., 2013). Some authors have reported a ‘best detection temperature’, but overall this parameter seems to exert an inconsistent influence on canine olfaction (Homan et al., 2001; Long et al., 2007; Paula et al., 2011; Reed et al., 2011; Reynolds et al., 2021). Indeed, the effect of temperature on the detection rate depends on multiple factors such as individual differences, time spent in the field at a given temperature and other weather parameters. No general recommendation can be given except avoiding surveys during the hottest weather. The counter effects of wind may be offset by dogs being allowed to move freely around the transect line to scent in multiple directions (Reed et al., 2011). When possible, working off-leash or using a ‘long’ line (e.g., 20 m) may increase the detection rate in windy or dense vegetation conditions (Paula et al., 2011; Reed et al., 2011; Domínguez del Valle et al., 2020; Hansen and Winje, 2021). However, the reality is that dogs are often required or mandated to work on-leash, for example in protected, commercial or urban areas.
Individual differences between dogs, not only breeds but also individual personalities, must be considered. Variations in behavior and reliability may be rooted in different training and detection capacities. Search abilities, trainability, desire to work, concentration, independence, work effort, olfactory capacities and behaviors such as fear of cars and noise have been reported by various authors as main criteria when selecting which dog to train for detection (Sinn et al., 2010; Beebe et al., 2016; Otto et al., 2019; Bray et al., 2021). The handler may also influence the performance of a detection dog (Lazarowski et al., 2020) and so in this regard, their experience and proficiency is crucial. They must communicate with the dog, direct searches efficiently, and both interpret a find and reward correctly. This is why different detection rates can be achieved for the same target under similar operational conditions depending on the degree of handler experience (Furtado et al., 2008; Beebe et al., 2016). For example, 81% of accuracy rate of jaguar and puma scats was obtained with experienced dog-handlers, while 50% of new teams collected non targeted species scat (Furtado et al., 2008). Different effective dog training and conditioning methods exist (Hall et al., 2021). It is also speculated that the skill of the trainer, who can also sometimes be the handler, influences the results achieved by the dog undergoing the training (Johnen et al., 2013, 2017).
Area covered and endurance
This and the next section consider studies focused on scat or other types of wildlife/conservation targets, as they include relevant information on the search and detection capabilities of detection dogs and dog-handler teams. Various linear distances covered by dogs and dog teams during a working session have been reported (Table 2) (Nussear et al., 2008; Brook et al., 2012; Clare et al., 2015; Mumma et al., 2015; Glen and Veltman, 2018; Hollerbach et al., 2018; Desvaux et al., 2021; Hansen and Winje, 2021; Reynolds et al., 2021). Generally, the average distance traveled by dogs is estimated to be 2 to 6 times greater than that walked by their human counterparts (Nussear et al., 2008; Dematteo et al., 2014; Desvaux et al., 2021). Dogs can cover more distance than humans during search work, but concentration and fatigue must be considered so as to maintain detection efficiency and well-being. Various protocols reflect a daily average search time range from 2 to 7 hours, depending on targets and goal of studies (Brook et al., 2012; Oliveira et al., 2012; Mumma et al., 2015; Fuller et al., 2016; Glen and Veltman, 2018; Hollerbach et al., 2018; Desvaux et al., 2021; Deák et al., 2021b).
The target being sought and factors related to its degree of scent are among the main reasons for differences reported in studies. Locations, the nature of the field, including distance to starting point of search and climatic conditions are also capable of influencing dog team efficiency (see corresponding section). Thus, in a protocol design, performance data must be adapted in light of the target, and the goal of the searching (Nussear et al., 2008; Dematteo et al., 2014; Glen and Veltman, 2018). In parallel, regular breaks, resting times and days-off must be scheduled in line with the dogs’ needs and welfare. These breaks ensure that peak concentration and efficiency is maintained (Nussear et al., 2008; Brook et al., 2012; Desvaux et al., 2021).
Costs
The cost of incorporating a detection dog team into ongoing efforts depends on whether or not the team has been professionally trained, their resident country, local prices and the study design and needs. Thus, it is difficult to precisely estimate the cost of the dog detection service/involvement, however some authors have reported costings and expenses according to their study objectives and requirements which can provide useful frames of reference. The cost of the dog-handler service, as shared by the authors, was estimated to be around 40% higher than that of human searchers alone. But the observed improvement in results were considered sufficient to offset the costs, particularly for small carcass searches involving dense vegetation (Domínguez del Valle et al., 2020). The annual cost of detection dogs for bobcat surveillance was estimated at $ 27,539 (USD), corresponding to: the cost of contracting dogs, hourly wages for handlers, training costs at organization headquarters, transport to site, and overhead. A further $ 4,653 was added to this budget for logistical on-site support including gas, housing for dog teams and rental vehicles (Clare et al., 2015). Bearing in mind current inflation rates, the cost of a dog handler is approximated to be $ 480 (USD)/day, which is more expensive than a human team (Nussear et al., 2008). However, with the same time and/or budget availability, twice as many turbines could be visited for bat carcass searches using dogs compared to humans alone. This is based on the hourly labor rate for a human observer, depending on countries, and the average survey time in this study. For such a use, an inclusive cost of $ 8,000 (USD)/animal is estimated based on the dogs used in the study and those trained afterwards (Mathews et al., 2013). Some authors affirmed that using dogs trained by their owners instead of specialized organizations is a way to access detection services when only limited funds are available (Byosiere et al., 2019). However, aside from the failsafe measures around expectation of performance and code of conduct, including safety of the target or species that are built into the professional dog-handler service, the costs of materials, housing and feeding volunteers must be considered. Maintenance and retirement costs must be added when personally owned dogs are used, whereas these costs are largely absorbed by professional organizations.
Set-up, training
In the case of a veteran, accomplished dog, a period of weeks is required for training to a new target odor, allowing for variables arising from multiple factors such as the dog and the target odor itself (Arnett, 2006; Nussear et al., 2008; Domínguez del Valle et al., 2020; Desvaux et al., 2021; Deák et al., 2021b). Some authors observe that dogs with previous fielding experience may show a greater detection rate than novice ones, and so, trained detection dogs are valuable and not easily replaceable (Mathews et al., 2013; Reynolds et al., 2021). But again, this depends on individual variability and many other parameters. Experienced dogs started to be trained by their owners (contracted by ONCFS) on the target “wild boar carcasses” and could begin detection work 3–4 weeks after (Desvaux et al., 2021). Dogs were trained for 7 days, after which they began field testing in the Arnett (2006) study. In another case, initial odor training was scheduled 2 to 3 times a week for 2 months, before proceeding to field acclimation with distractions 5 to 6 times a week and later, field testing (Reynolds et al., 2021). Finally, dogs were trained for 3 months between 2 and 4 hours per week in the Domínguez del Valle et al. (2020) study. Different methods of training and preparing dogs for the field can lead to comparable, desired levels of efficiency. In addition to initial training, dogs must be trained regularly to maintain their memory and detection skills, although the training interval is not well defined and strongly depends on the dog and the handler (Hall et al., 2021). In tandem with efforts to train and maintain detection dogs to certain types of scarcer targets, it may be difficult to rapidly mobilize enough qualified dog teams in response to an emerging problem. This mobilization issue can be a limit of the use of detection dogs.
Aerial thermal imaging
Efficiency
When a bird or mammal dies, its body temperature drops. The evolution of wild boar carcass temperatures showed that carcasses cool at an average rate of 0.5 to 1°C per hour over the first two days with a daily average ambient air temperature 0-17°C. Then, between the third and seventh day, the carcasses warm up, and their daily average temperature mostly ranged between 9–18°C above the daily air temperature (14-21°C) for a period of 12 to 21 days after culling (Hohmann et al., 2021). Thus, thermal imaging is potentially useful for detecting wildlife carcasses at different stages of decomposition (Table 3). Indeed, during the active decomposition phase, heat is released by maggot activity and micro-organisms (i.e. putrefaction bacteria), which contribute to carcass warming (Amendt et al., 2017). The resulting difference between carcass temperature and that of the ambient air enables detection by infrared cameras (see for example Figure 4). This detection is possible during the very first days post mortem and then from the day 3–7 until 21 days, and up to 50 days in some cases, depending on IR signature and conditions (Hohmann et al., 2021). Interestingly, forward-looking infrared (FLIR) cameras are often used for this purpose, and can also be added to aerial devices (Descalzi, 2019; Hohmann et al., 2021; Rietz et al., 2023).
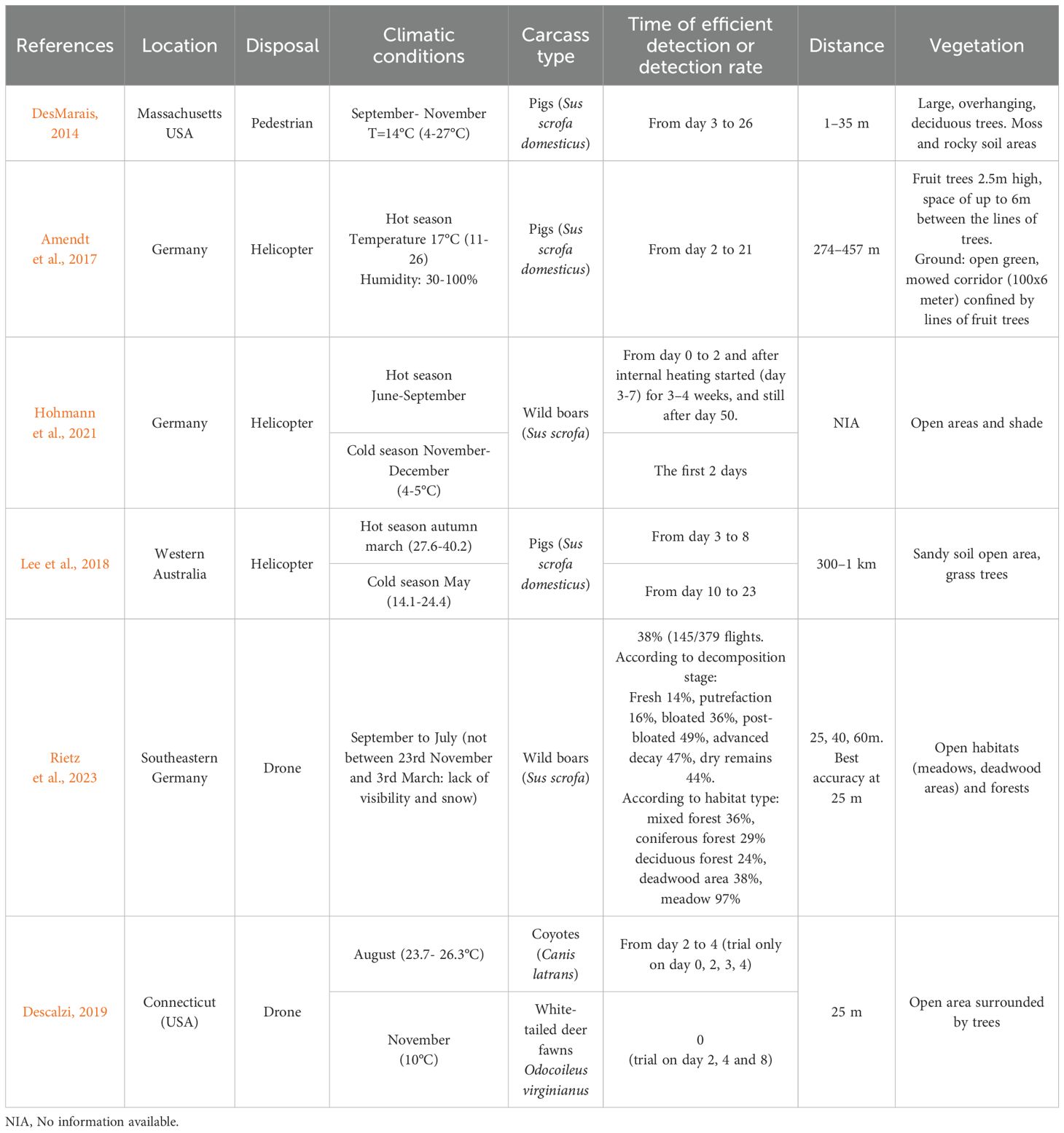
Table 3. Compared efficiency and environmental parameters for detection of wildlife carcass by thermal imaging in different studies.
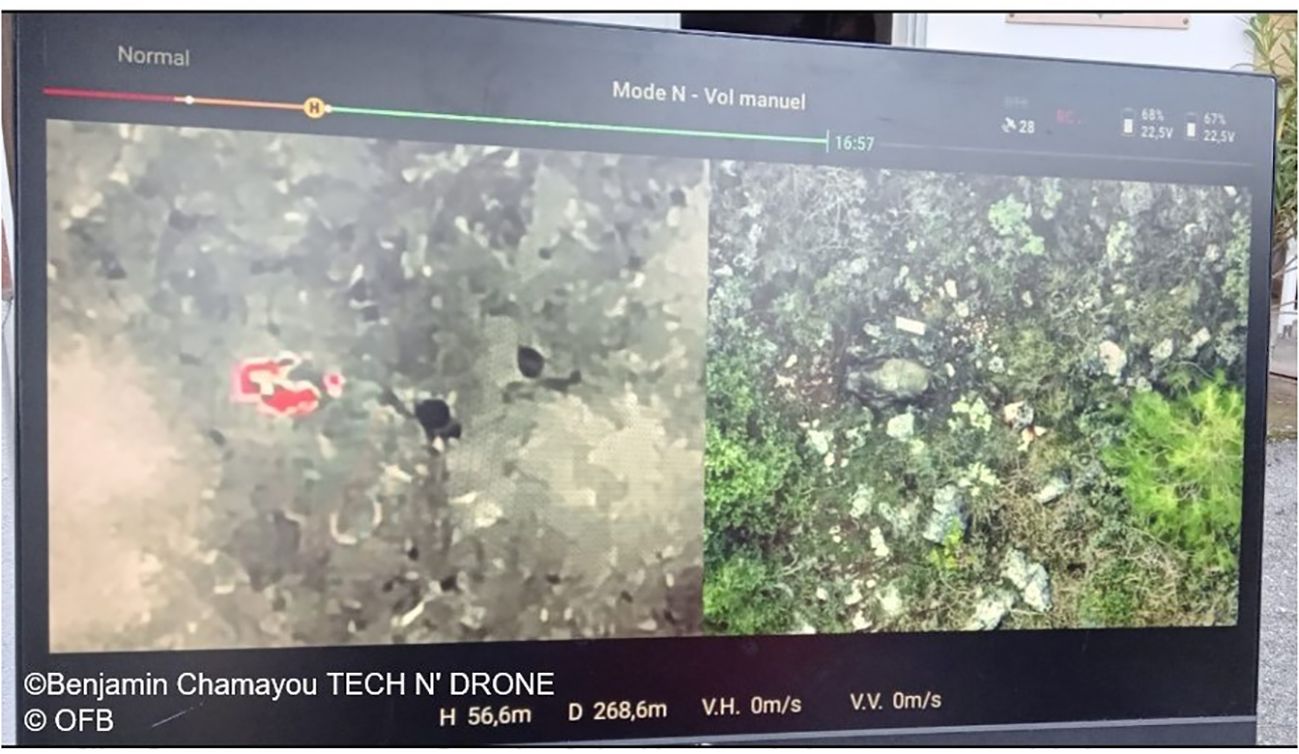
Figure 4. Operational picture of the monitoring screen during a field test with the French Agency (OFB) showing normal and thermal image of a wild boar carcass (about 50kg) 10 days after defrosting with presence of maggot mass (drone height 56.6m) ©Benjamin Chamayou (TECH N’ DRONE), Stéphanie Desvaux, Anouk Decors, Camille Sandor (OFB).
Influence factors and technical limits
Factors impacting thermal imaging include image quality, which varies with climatic conditions, time of day, lighting, carcass decomposition stage and larval density (DesMarais, 2014; Lee et al., 2018; Descalzi, 2019; Hohmann et al., 2021; Rietz et al., 2023). The vegetation density and distance to the animal can also impede or further prevent identification (Gray et al., 2023; Rietz et al., 2023). The decomposition stage will determine internal heating and by extension, emanating radiation. In temperate and continental climatic regions, carcasses found in winter can show little to no internal heating, due to an absence or slowing of entomological activity. In this case, carcasses essentially become ‘invisible’ when sunlight absorption serves as the only source of infrared radiation, because levels mirror that of surrounding objects (e.g. soil, stone, wood) (Descalzi, 2019; Hohmann et al., 2021). Descalzi (2019) and Lee et al. (2018) (see also Table 3) showed that active decomposition was not sufficient to be detected or was delayed during the colder period. The highest probability of detecting wild boar carcasses (>70%) was observed at temperatures >3.0°C. Detection success is indeed shown to match a temperature difference of more than 6.4°C between the carcass and the ambient air.
The size of the carcass is also key in that drones have to fly lower to ensure effective detection of smaller carcasses. Detection by drone has been shown to be higher in open habitats. In very dense forests, with high larval development, i.e. in hot climatic conditions, probability of detection is reduced, potentially to less than 25% (Rietz et al., 2023). Some authors also recommend using drones on cloudy days, as detection can be reduced by direct sunlight. Drone flights conducted in cloudy weather and at first light versus in full sunshine yielded detection probabilities of 70% and 40%, respectively (Descalzi, 2019; Rietz et al., 2023). Weather can also be an operational limitation as drone flight is not recommended in high winds or rain (Whitworth et al., 2022). In conclusion, thermal imaging is most effective for identifying the presence of a carcass in a given landscape when it is in active decomposition and emitting sufficient heat to be distinguishable from surrounding objects. This entails an ambient temperature > 3°C. Aerial devices such as drones are optimally deployed on cloudy days and when sunlight is the lowest. They are also particularly effective with a reduced canopy cover of <70% (DesMarais, 2014; Lee et al., 2018; Descalzi, 2019; Hohmann et al., 2021; Rietz et al., 2023).
Covered area and endurance
In this section we focus on thermal imaging mounted drones. These are considered an asset to wildlife carcass detection because they offer capability to cover a large area in a minimum amount of time (Rietz et al., 2023). Note that helicopters and other aircraft devices are excluded, largely due to cost considerations. An average altitude of 40 meters, with some flight at 25 meters when permitted by vegetation is often used. This altitude results in an optimized compromise between the detection capacity of the drone and a large-coverage area detection rate (Descalzi, 2019; Rietz et al., 2023). An average flight speed of 9 m/s (maximum = 14 m/s) tested during 36 flights was associated with positive detection of carcasses by thermal imaging (Rietz et al., 2023). Thermal camera equipped drone selection will depend on maximum capacity and whether it will meet surveillance protocol expectations. Device battery capacity and weight will determine the covered area and time for which aerial devices such as drones can be used. Drones offer an average range of one kilometer and a maximum flight time between 20 and 45 minutes, depending on the speed and the chosen device. Longer usage will necessitate a change of battery.
Costs
Helicopters are expensive and difficult to access (Descalzi, 2019; Rietz et al., 2023). Thus, thermal equipped drone are often used as they are easier and cheaper despite price differences between countries and models (Nguyen et al., 2021). Ready-to-use drones often cost in excess of $ 20,000 (USD), leading to the suggestion of using homemade devices to reduce costs (Kayan et al., 2018). However, less expensive models exist, with costs varying between $ 6,150 and $ 7,800 (USD). Regardless, the choice of drone must be adapted to the area and specific surveillance needs, and the effectiveness/cost balance must be evaluated on a case by case basis (Descalzi, 2019). This kind of technology evolves rapidly and so does the cost and capacity. Training and repair costs in case of hardware damage must also be considered for personal equipped drones. This must be compared with a drone-pilot service in which such costs are included. Using personal equipped drones or drone-pilot service will depend on the surveillance needs. For occasional needs, a drone-pilot service may be sufficient, whereas for regular needs, training a pilot and buying a drone may become relevant. Furthermore, unless the pilot can also do it, this detection approach requires another person to interpret the thermal images and decide whether there is a chance of finding a carcass. Finally, depending on the surveillance goals, one or several search teams might still be sent to collect the carcass. All of this must be considered in the global costs.
Set-up, training
Drone flight must comply with local regulations pertaining to the training and certification of the pilot and the device used. A different authorization might be needed depending on the weight, the equipment and the flight itself. If the flight is conducted at a high altitude, within a populated area, without visibility or in a particular local aerial zone, authorizations might be required. To be responsive in an emergency, the training time of the pilot must be anticipated and incorporated ahead of time. Working with an established drone pilot or a pilot service facilitates rapid response. Detection also depends on the skills and experience of the operator (Descalzi, 2019). Flight may also not be feasible in certain locations such as aerial restriction zones and populated areas, also dependent on the drone category and the pilot’s certifications. Localized technical assistance may be required for proper field reconnaissance prior to the searching session.
Other possible detection tools
Scavengers equipped with GPS systems
Scavengers such as the European griffon vulture (Gyps fulvus) and opportunist scavengers such as the Iberian wolf (Canis lupus signatus) are routinely equipped with Global Positioning Systems (GPS) to study their behavior. This approach can also be coordinated to identify the location of freely occurring carcasses outside already known feeding-provisioned stations. The interpretation of the GPS data may be indicative of feeding behavior (Planella et al., 2016; Arkumarev et al., 2020; Mateo-Tomás et al., 2023). In Bulgaria, European griffon vultures were fitted with solar-powered GPS attached as backpacks to study their diet (Bodey et al., 2018; Arkumarev et al., 2020). The behavior was designated as a feeding behavior when (1) two or more vultures visited a same location that was considered unsuitable for roosting or perching, and (2) the individual spent more than 15 minutes in a valley, forest or bushy area, which is considered unusual for roosting or perching. This was followed by a field inspection conducted between one and six days after the recorded event with a detection dog team searching for remains. In this protocol, carcasses consumed by vultures were found in 92.1% of the field visits, demonstrating the efficiency of GPS systems in situating feeding locations (Arkumarev et al., 2020). Solar-powered GPS were also used on vultures and GPS collars on wolves to show compliance of sanitary regulations regarding the disposal of livestock carcasses in the field to feed scavengers. Feeding events were characterized by clusters of at least two consecutive positions under a given speed threshold indicating a possible stop. Meanwhile, long periods of inactivity during daylight hours were considered to indicate resting locations. These criteria were sufficient to differentiate feeding sites from resting locations with an accuracy of more than 70% (Mateo-Tomás et al., 2023).
There are few data on the efficiency of this approach, and influence factors have not been investigated so far. However, some limits can be posited. Satiety of tracked individuals may result in a lack of interest and therefore non-detection of some proportion of available carcasses. Furthermore, existing studies mostly focus on large animal carcasses such as ungulates, while the time spent and the number of scavengers feeding on small carcasses may be lower and the cluster missed. In addition, a preliminary step is to anesthetize and/or capture wild scavengers and fit them with GPS systems. This requires an experienced team and raises ethical issues. To avoid these problems, the opportunistic use of data from previously equipped scavengers (for other goals and uses) can be considered as an opportunistic reinforced search approach more than a targeted search. Other species such as corvids could also be considered, but the devices must be quite light and the carcass detection abilities of these species has not been tested thus far.
Sonar detection for submerged carcasses
The effectiveness of a side-scan sonar has been studied on pig carcasses as a model for submerged human bodies. Indeed, such devices allows for larger area searches in less time than dive teams can provide. They can be used in fresh and saltwater and are unaffected by visibility. Medium-sized carcasses were still detectable after 81 days post-submersion, showing that decomposed carcasses can be located for a long time using a side-scan sonar (water temperature 18-23°C, depth of 7.5 m) (Healy et al., 2015). But irregular ground and aquatic vegetation density are important limitations to this approach, as these can obscure the target (Schultz et al., 2013; Healy et al., 2015). Evidently, submerged wildlife carcasses are not a common search target in wildlife surveillance. This approach is still relevant for applications like early detection of cetacean stranding.
Volatile organic compound detection
Many volatile organic compounds (VOCs) are released by a decomposing carcass (Dekeirsschieter et al., 2009; Stadler and Stefanuto, 2013). Certain species can share VOC signatures even while retaining some unique, species-specific VOCs. Since pigs or chickens share certain VOC decomposition signatures with humans, these species are used to train dogs for human remain searching (Cablk et al., 2012). Just as these VOCs can be used to train detection dogs (see, e.g., the review of (Dargan and Forbes, 2021) and (DeGreeff and Furton, 2011)), they can also be directly identified by thermal desorption interfaced with gas chromatography and mass spectrometry (Dekeirsschieter et al., 2009; Stadler and Stefanuto, 2013). They are shown to be mostly acids, cyclic hydrocarbons, oxygenated compounds, sulfur and nitrogen compounds, alcohols, carboxylic acids, aromatics, and sulfides (Dekeirsschieter et al., 2009; Stadler and Stefanuto, 2013; Agapiou et al., 2015). Pig carcasses placed inside tunnels to simulate a building collapse and improve human rescue methods showed that dedicated setups can capture VOCs for subsequent analysis (Agapiou et al., 2015). However, these setups are not developed to search carcasses in real time and over long distances in the field.
Discussion
The surveillance and management of acute wildlife diseases may involve detection and removal of contaminated carcasses, for diagnostic purposes. To enhance detection through the adaptation of search efforts, various techniques are available, each with distinct frameworks, advantages, and limitations. For instance, surveillance of African swine fever (ASF), a disease with significant economic implications and a high fatality rate, depends on the recovery of wild boar carcasses. Sepsis is induced by disease, and the pathogen exhibits significant environmental resistance, allowing its discovery in various organs, including the bone marrow. Consequently, all carcasses, from fresh to skeletal stage, can be utilized for diagnostic purposes. In contrast, the early identification of novel or unidentified diseases relies on a thorough diagnostic methodology and necessitates the analysis of fresh carcasses wherever feasible. Search attempts should consider these aims and constraints accordingly. Upon examining the current knowledge and available data, we have summarized decision criteria influencing the choice of the most adapted tools for facilitating wildlife carcass detectability and detection (Figure 2).
Our review indicates that detection dogs and aerial thermal imaging are effective tools for locating wild animal carcasses. The balance between the advantages and limitations of these two technologies and their integration will ultimately rely on the species of animal carcass targeted, environmental and climatic conditions, and the availability of skilled personnel. Due to the potential lack of formalized networks dedicated to carcass detection dogs and drones, mobilizing qualified resources poses a challenge and necessitate anticipation and quick response. Moreover, planning to test the efficacy of service providers is essential as we are not aware of any existing accreditation for carcass detection dog-handler teams. Furthermore, technologies and regulations evolve rapidly and these approaches are constantly subject to change and improvement. Consequently, surveillance with clear and targeted strategies will enhance carcass detection efficiency.
Figure 2 depicts in list form the selection criteria influencing the most suitable detection tools. Answering to each section and question, the study designers must determine the most significant aspects for the research and select the most suitable tool(s) accordingly. Consider, for example, an early identification of ASF occurring during summer in the French Alps, an environment defined by a compact and dense layer of vegetation. All decomposition stages are valuable for surveillance, including the advanced decomposition stage, but a high detection rate is essential. The surveillance network already possesses detection dog teams. Dogs would thus be the most appropriate instruments in this context. A drone operation could also be incorporated here to improve detection of fresh carcasses. But in this specific environmental situation, marked by elevated daytime temperatures, operators should refrain from utilizing dogs and drones during midday. Consequently, a drone could initially be used to locate carcasses throughout an extensive search at the regional scale. One or several dog teams could then be sent to these particular GPS coordinates, along with the support personnel required to sample or recover carcasses. Any decisions must carefully consider the interplay of local environment, available means and the study goal.
Author contributions
CS: Writing – original draft, Writing – review & editing. SD: Writing – original draft, Writing – review & editing. LP: Writing – original draft, Writing – review & editing. SL: Writing – original draft, Writing – review & editing. DC: Writing – original draft, Writing – review & editing. PD: Writing – original draft, Writing – review & editing. AD: Writing – original draft, Writing – review & editing.
Funding
The author(s) declare that no financial support was received for the research and/or publication of this article.
Acknowledgments
We would like to thank Victoria Weckmann who accepted to be on the picture with the detection dog, and Benjamin CHAMAYOU TECH N’ DRONE for his expertise as a drone pilot during the field trial we made. We received very valuable and relevant inputs on this manuscript from two reviewers and from the editorial team that we would also like to thank.
Conflict of interest
The authors declare that the research was conducted in the absence of any commercial or financial relationships that could be construed as a potential conflict of interest.
Publisher’s note
All claims expressed in this article are solely those of the authors and do not necessarily represent those of their affiliated organizations, or those of the publisher, the editors and the reviewers. Any product that may be evaluated in this article, or claim that may be made by its manufacturer, is not guaranteed or endorsed by the publisher.
References
Agapiou A., Zorba E, Mikedi K, McGregor L, Spiliopoulou C, Statheropoulos M. (2015). Analysis of volatile organic compounds released from the decay of surrogate human models simulating victims of collapsed buildings by thermal desorption–comprehensive two-dimensional gas chromatography–time of flight mass spectrometry. Analytica Chimica Acta 883, 99–108. doi: 10.1016/j.aca.2015.04.024
Amendt J., Rodner S., Schuch C-P., Sprenger H., Weidlich L., Reckel F. (2017). Helicopter thermal imaging for detecting insect infested cadavers. Sci. Justice 57, 366–372. doi: 10.1016/j.scijus.2017.04.008
Arkumarev V., Dobrev D., Stamenov A., Terziev N., Delchev A., Stoychev S. (2020). Using GPS and accelerometry data to study the diet of a top avian scavenger. Bird Study 67, 300–310. doi: 10.1080/00063657.2020.1864285
Arnett E. B. (2006). A preliminary evaluation on the use of dogs to recover bat fatalities at wind energy facilities. Wildlife Soc. Bull. 34, 1440–1445. doi: 10.2193/0091-7648(2006)34[1440:APEOTU]2.0.CO;2
Bailey L. L., Simons T. R., Pollock K. H. (2004). Estimating detection probability parameters for plethodon salamanders using the robust capture-recapture design. J. Wildlife Manage. 68, 1–13. doi: 10.2193/0022-541X(2004)068[0001:EDPPFP]2.0.CO;2
Balajee S. A., Salyer S. J., Greene-Cramer B., Sadek M., Mounts A. W. (2021). The practice of event-based surveillance: concept and methods. Global Security: Health Sci. Policy 6, 1–9. doi: 10.1080/23779497.2020.1848444
Barrientos R., Martins R. C., Ascensão F., D'Amico M., Moreira F., Borda-de-Água L. (2018). A review of searcher efficiency and carcass persistence in infrastructure-driven mortality assessment studies. Biol. Conserv. 222, 146–153. doi: 10.1016/j.biocon.2018.04.014
Beebe S. C., Howell T. J., Bennett P. C. (2016). Using scent detection dogs in conservation settings: A review of scientific literature regarding their selection. Front. Veterinary Sci. 3. doi: 10.3389/fvets.2016.00096
Bergman E. J., Hayes F. P., Lukacs P. M., Bishop C. J. (2020). Moose calf detection probabilities: quantification and evaluation of a ground-based survey technique. Wildlife Biol. 2020, 1. doi: 10.2981/wlb.00599
Bodey T. W., Cleasby I. R., Bell F., Parr N., Schultz A., Votier S. C., et al. (2018). A phylogenetically controlled meta-analysis of biologging device effects on birds: Deleterious effects and a call for more standardized reporting of study data. Methods Ecol. Evol. 9, 946–955. doi: 10.1111/2041-210X.12934
Bollinger T. K., Evelsizer D. D., Zimmer M. (2011).Ecology and Management of Avian Botulism on the Canadian Prairies- PART I EFFICACY OF CARCASS CLEAN-UP DURING AVIAN BOTULISM OUTBREAKS. In: Prairie Habitat Joint Venture Science Committee. Available online at: https://www.phjv.ca/wp-content/uploads/2017/10/BotulismReport_FINAL_FullReport_Aug2011.pdf (Accessed 24 November 2023).
Borner L., Duriez O., Besnard A., Robert A., Carrere V., Jiguet F. (2017). Bird collision with power lines: estimating carcass persistence and detection associated with ground search surveys. Ecosphere 8, e01966. doi: 10.1002/ecs2.1966
Bray E. E., Otto C. M., Udell M. A. R., Hall N. J., Johnston A. M., MacLean E. L. (2021). Enhancing the selection and performance of working dogs. Front. Veterinary Sci. 8. doi: 10.3389/fvets.2021.644431
Brook S. M., van Coeverden de Groot P., Scott C., Boag P., Long B., Ley R. E., et al. (2012). Integrated and novel survey methods for rhinoceros populations confirm the extinction of Rhinoceros sondaicus annamiticus from Vietnam. Biol. Conserv. 155, 59–67. doi: 10.1016/j.biocon.2012.06.008
Browne C., Stafford K., Fordham R. (2006). The use of scent-detection dogs. Irish Veterinary J. 59, 97–104.
Byosiere S.-E., Feng L. C., Rutter N. J. (2019). Factors that may affect the success of scent detection dogs: Exploring non-conventional models of preparation and deployment. Comp. Cogn. Behav. Rev. 14, 81–86. doi: 10.3819/CCBR.2019.140009
Cablk M. E., Szelagowski E. E., Sagebiel J. C. (2012). Characterization of the volatile organic compounds present in the headspace of decomposing animal remains, and compared with human remains. Forensic Sci. Int. 220, 118–125. doi: 10.1016/j.forsciint.2012.02.007
Cardoso B., García-Bocanegra I., Acevedo P., Cáceres G., Alves P. C., Gortázar C. (2022). Stepping up from wildlife disease surveillance to integrated wildlife monitoring in Europe. Res. Veterinary Sci. 144, 149–156. doi: 10.1016/j.rvsc.2021.11.003
Carter D. O., Yellowlees D., Tibbett M. (2009). “Can Temperature Affect the Release of Ninhydrin-Reactive Nitrogen in Gravesoil Following the Burial of a Mammalian (Rattus rattus) Cadaver?,” in Criminal and Environmental Soil Forensics. Eds. Ritz K., Dawson L., Miller D. (Springer Netherlands, Dordrecht), 333–340. doi: 10.1007/978-1-4020-9204-6_21
Carter D. O., Yellowlees D., Tibbett M. (2010). Moisture can be the dominant environmental parameter governing cadaver decomposition in soil. Forensic Sci. Int. 200, 60–66. doi: 10.1016/j.forsciint.2010.03.031
Clare J. D. J., Anderson E. m., Macfarland D. m., Sloss B. l. (2015). Comparing the costs and detectability of bobcat using scat-detecting dog and remote camera surveys in central Wisconsin. Wildlife Soc. Bull. 39, 210–217. doi: 10.1002/wsb.502
Collinson W. J., Parker D. M., Bernard R. T. F., Reilly B. K., Davies-Mostert H. T. (2014). Wildlife road traffic accidents: a standardized protocol for counting flattened fauna. Ecol. Evol. 4, 3060–3071. doi: 10.1002/ece3.1097
Dargan R., Forbes S. L. (2021). Cadaver-detection dogs: A review of their capabilities and the volatile organic compound profile of their associated training aids. WIREs Forensic Sci. 3, e1409. doi: 10.1002/wfs2.1409
Deák G., Árvay M., Horváth M. (2021a). Using detection dogs to reveal illegal pesticide poisoning of raptors in Hungary’. J. Vertebrate Biol. 69. doi: 10.25225/jvb.20110
Deák G., Katona K., Biró Z. (2021b). Exploring the use of a carcass detection dog to assess mowing mortality in Hungary. J. Vertebrate Biol. 69. doi: 10.25225/jvb.20089
DeGreeff L. E., Furton K. G. (2011). Collection and identification of human remains volatiles by non-contact, dynamic airflow sampling and SPME-GC/MS using various sorbent materials. Analytical Bioanalytical Chem. 401, 1295–1307. doi: 10.1007/s00216-011-5167-0
Dekeirsschieter J., Verheggen F. J., Gohy M., Hubrecht F., Bourguignon L., Lognay G. (2009). Cadaveric volatile organic compounds released by decaying pig carcasses (Sus domesticus L.) in different biotopes. Forensic Sci. Int. 189, 46–53. doi: 10.1016/j.forsciint.2009.03.034
Dematteo K. E., Rinas M. A., Argüelles C. F., Holman B. E., Di Bitetti M. S., Davenport B. (2014). Using detection dogs and genetic analyses of scat to expand knowledge and assist felid conservation in Misiones, Argentina. Integr. Zoology 9, 623–639. doi: 10.1111/1749-4877.12113
Descalzi M. (2019). Detection of Larval Aggregations Using a Drone Mounted Thermal Imaging Camera (Connecticut: University of New Haven). Master’s Theses.
DesMarais A. M. (2014). Detection of cadaveric remains by thermal imaging cameras. J. Forensic Identification 64, 489–510.
Desvaux S., Urbaniak C., Petit T., Chaigneau P., Gerbier G., Decors A. (2021). How to strengthen wildlife surveillance to support freedom from disease: example of ASF surveillance in France, at the border with an infected area. Front. Veterinary Sci. 8. doi: 10.3389/fvets.2021.647439
Domínguez del Valle J., Cervantes Peralta F., Jaquero Arjona M. I. (2020). Factors affecting carcass detection at wind farms using dogs and human searchers. J. Appl. Ecol. 57, 1926–1935. doi: 10.1111/1365-2664.13714
Duarte J. M. B., Talarico A. C., Vogliotti A., Garcia J. E., Oliveira M. L., Maldonado J. E. (2017). Scat detection dogs, DNA and species distribution modelling reveal a diminutive geographical range for the Vulnerable small red brocket deer Mazama bororo. Oryx 51, 656–664. doi: 10.1017/S0030605316000405
Fernández Verón I., Zorrilla I., Richards N. L. (2021). Is deliberate pesticide poisoning of wildlife impacting local insect communities? Wildlife and environmental forensic investigations in southern Spain present an opportunity for collaborative entomological monitoring. J. Insect Conserv. 25, 511–519. doi: 10.1007/s10841-021-00319-6
Fiedler S., Graw M. (2003). Decomposition of buried corpses, with special reference to the formation of adipocere. Naturwissenschaften 90, 291–300. doi: 10.1007/s00114-003-0437-0
Fuller A. K., Sutherland C. S., Royle J. A., HAre M. P. (2016). Estimating population density and connectivity of American mink using spatial capture–recapture. Ecol. Appl. 26, 1125–1135. doi: 10.1890/15-0315
Furtado M. M., Carrillo-Percastegui S. E., Jácomo A. T. A., Powell G., Silveira L., Vynne C. (2008). Studying jaguars in the wild: past experiences and future perspectives. CAT News 4, 41–47.
Gazit I., Terkel J. (2003). Explosives detection by sniffer dogs following strenuous physical activity. Appl. Anim. Behav. Sci. 81, 149–161. doi: 10.1016/S0168-1591(02)00274-5
Glen A. S., Veltman C. J. (2018). Search strategies for conservation detection dogs. Wildlife Biol. 2018, 1–9. doi: 10.2981/wlb.00393
Glover N. J., Wilson L. E., LeedaleI A., Jehle R. (2023). An experimental assessment of detection dog ability to locate great crested newts (Triturus cristatus) at distance and through soil. PloS One 18, e0285084. doi: 10.1371/journal.pone.0285084
Gray L. F., McNeil D. J., Larkin J. T., Parker H. A., Shaffer D., Larkin J. L. (2023). Quantifying detection probability of American woodcock (Scolopax minor) on transects sampled with thermal cameras. Wildlife Soc. Bull. 47, e1417. doi: 10.1002/wsb.1417
Grimm-Seyfarth A. (2022). Environmental and training factors affect canine detection probabilities for terrestrial newt surveys. J. Veterinary Behav. 57, 6–15. doi: 10.1016/j.jveb.2022.07.013
Guinard É., Prodon R., Barbraud C. (2015). “Case study: A robust method to Obtain Defendable Data on Wildlife Mortality,” in Handbook of Road Ecology. Eds. Ree R.v. d., Smith D. J., Grilo C. (John Wiley & Sons, Ltd, Chichester, UK), 96–100. doi: 10.1002/9781118568170.ch12
Hag-Ali M., AlShamsi A. S., Boeijen L., Mahmmod Y., Manzoor R., Rutten H. (2021). The detection dogs test is more sensitive than real-time PCR in screening for SARS-CoV-2. Commun. Biol. 4, 686. doi: 10.1038/s42003-021-02232-9
Hall N. J., Johnston A. M., Bray E. E., Otto C. M., MacLean E. L., Udell M. A. R.. (2021). Working dog training for the twenty-first century. Front. Veterinary Sci. 8. doi: 10.3389/fvets.2021.646022
Hallingstad E., Riser-Espinoza D., Brown S., Rabie P., Haddock J., Kosciuch K. (2023). Game bird carcasses are less persistent than raptor carcasses, but can predict raptor persistence dynamics. PloS One 18, e0279997. doi: 10.1371/journal.pone.0279997
Hansen I., Winje E. (2021). Efficiency of livestock carcass detection dogs. Rangelands 43, 194–199. doi: 10.1016/j.rala.2021.03.004
Healy C. A., Schultz J. J., Parker K., Lowers B. (2015). Detecting submerged bodies: controlled research using side-scan sonar to detect submerged proxy cadavers. J. Forensic Sci. 60, 743–752. doi: 10.1111/1556-4029.12671
Hels T., Buchwald E. (2001). The effect of road kills on amphibian populations. Biol. Conserv. 99, 331–340. doi: 10.1016/S0006-3207(00)00215-9
Henry D. A. W., Collinson-Jonker W. J., Davies-Mostert H. T., Nicholson S. K., Roxburgh L., Parker D. M. (2021). Optimising the cost of roadkill surveys based on an analysis of carcass persistence. J. Environ. Manage. 291, 112664. doi: 10.1016/j.jenvman.2021.112664
Hohmann U., Kronenberg M., Scherschlicht M., Schönfeld F. (2021). The possibilities and limitations of thermal imaging to detect wild boar (Sus scrofa) carcasses as a strategy for managing African Swine Fever (ASF) outbreaks. Berliner und Münchener Tierärztliche Wochenschrift 134, 1–14. doi: 10.2376/1439-0299-2020-46
Hollerbach L., Heurich M., Reiners T. E., Nowak C. (2018). Detection dogs allow for systematic non-invasive collection of DNA samples from Eurasian lynx. Mamm. Biol. 90, 42–46. doi: 10.1016/j.mambio.2018.02.003
Homan H. J., Linz G. M., Peer B. D. (2001). Dogs increase recovery of passerine carcasses in dense vegetation. Wildlife Soc. Bull. 29, 292–296. doi: 10.2307/3784011
Johnen D., Heuwieser W., Fischer-Tenhagen C. (2013). Canine scent detection—Fact or fiction? Appl. Anim. Behav. Sci. 148, 201–208. doi: 10.1016/j.applanim.2013.09.002
Johnen D., Heuwieser W., Fischer-Tenhagen C. (2017). An approach to identify bias in scent detection dog testing. Appl. Anim. Behav. Sci. 189, 1–12. doi: 10.1016/j.applanim.2017.01.001
Kayan H., Eslampanah R., Yeganli F., Askar M. (2018). “Heat leakage detection and surveiallance using aerial thermography drone,” in 2018 26th Signal Processing and Communications Applications Conference (SIU), Izmir, Turkey. 1–4. (Turkey: IEEE). doi: 10.1109/SIU.2018.8404366
Kitano M., Smallwood K. S., Fukaya K. (2023). Bird carcass detection from integrated trials at multiple wind farms. J. Wildlife Manage. 87, e22326. doi: 10.1002/jwmg.22326
Kuiken T., Ryser-Degiorgis M.-P., Gavier-Widén D., Gortázar C. (2011). Establishing a European network for wildlife health surveillance: -EN- -FR- Mise en place d’un réseau européen de surveillance sanitaire de la faune sauvage -ES- Creación de una red europea de vigilancia sanitaria de la fauna silvestre. Rev. Scientifique Technique l’OIE 30, 755–761. doi: 10.20506/rst.30.3.2067
Lange M., Guberti V., Thulke H. (2018). Understanding ASF spread and emergency control concepts in wild boar populations using individual-based modelling and spatio-temporal surveillance data. EFSA Supporting Publications 15. doi: 10.2903/sp.efsa.2018.EN-1521
Lazarowski L., Lazarowski L., DeGreeff L. E., Simon A., Singletary M., Angle C. (2020). Methodological considerations in canine olfactory detection research. Front. Veterinary Sci. 7. doi: 10.3389/fvets.2020.00408
Lee M. J., Voss S. C., Franklin D., Dadour I. R. (2018). Preliminary investigation of aircraft mounted thermal imaging to locate decomposing remains via the heat produced by larval aggregations. Forensic Sci. Int. 289, 175–185. doi: 10.1016/j.forsciint.2018.05.028
Licoppe A., De Waele V., Malengreaux C., Paternostre J., Van Goethem A., Desmecht D. (2023). Management of a focal introduction of ASF virus in wild boar: the belgian experience. Pathogens 12, 152. doi: 10.3390/pathogens12020152
Linz G. M., Davis J. E., Engeman R. M., Otis D. L., Avery M. L. (2006). Estimating survival of bird carcasses in cattail marshes. Wildlife Soc. Bull. (1973-2006) 19, 195–199.
Long R. A., Donovan T. M., Mackay P., Zielinski W. J., Buzas J. S. (2007). Effectiveness of scat detection dogs for detecting forest carnivores. J. Wildlife Manage. 71, 2007–2017. doi: 10.2193/2006-230
MacKenzie D. I., Nichols J. D., Lachman G. B., Droege S., Royle J. A., Langtimm C. A. (2002). Estimating site occupancy rates when detection probabilities are less than one. Ecology 83, 2248–2255. doi: 10.1890/0012-9658(2002)083[2248:ESORWD]2.0.CO;2
Mateo-Tomás P., Rodríguez-Pérez J., Fernández-García M., García E. J., Valente e Santos J. P., Gutiérrez I. (2023). Wildlife as sentinels of compliance with law: An example with GPS -tagged scavengers and sanitary regulations. J. Appl. Ecol., 1365–2664.14487. doi: 10.1111/1365-2664.14487
Mathews F., Swindells M., Goodhead R., August T. A., Hardman P., Linton D. M. (2013). Effectiveness of search dogs compared with human observers in locating bat carcasses at wind-turbine sites: A blinded randomized trial. Wildlife Soc. Bull. 37, 34–40. doi: 10.1002/wsb.256
McKeague B., Finlay C., Rooney N. (2024). Conservation detection dogs: A critical review of efficacy and methodology. Ecol. Evol. 14, e10866. doi: 10.1002/ece3.10866
Meyer J., Anderson B., Carter D. O. (2013). Seasonal variation of carcass decomposition and gravesoil chemistry in a cold (Dfa) climate. J. Forensic Sci. 58, 1175–1182. doi: 10.1111/1556-4029.12169
Moleón M., Martínez-Carrasco C., Muellerklein O. C., Getz W. M., Muñoz-Lozano C., Sánchez-Zapata J. A. (2017). Carnivore carcasses are avoided by carnivores. J. Anim. Ecol. 86, 1179–1191. doi: 10.1111/1365-2656.12714
Moore M. J., Mitchell G. H., Rowles T. K., Early G. (2020). ‘Dead cetacean? Beach, bloat, float, sink. Front. Marine Sci. 7. doi: 10.3389/fmars.2020.00333
Morner T., Obendorf D. L., Artois M., Woodford M. H. (2002). Surveillance and monitoring of wildlife diseases: -EN- -FR- -ES-. Rev. Scientifique Technique l’OIE 21, 67–76. doi: 10.20506/rst.21.1.1321
Mumma M. A., Zieminski C., Fuller T. K., Mahoney S. P., Waits L. P. (2015). Evaluating noninvasive genetic sampling techniques to estimate large carnivore abundance. Mol. Ecol. Resour. 15, 1133–1144. doi: 10.1111/1755-0998.12390
Nguyen T. X. B., Rosser K., Chahl J. (2021). A review of modern thermal imaging sensor technology and applications for autonomous aerial navigation. J. Imaging 7, 217. doi: 10.3390/jimaging7100217
Nussear K. E., Esque T. C., Heaton J. S., Cablk M. E., Drake K. K. (2008). ARE WILDLIFE DETECTOR DOGS OR PEOPLE BETTER AT FINDING DESERT TORTOISES (GOPHERUS AGASSIZII). Herpetological Conserv. Biol. 3. doi: 10.3389/frans.2022.932857
Oliveira M. L., Norris D., Ramírez J. F. M., Peres P. H. de F., Galetti M., Duarte J. M. B. (2012). Dogs can detect scat samples more efficiently than humans: an experiment in a continuous Atlantic Forest remnant. Zoologia (Curitiba) 29, 183–186. doi: 10.1590/S1984-46702012000200012
Olson Z. H., Beasley J. C., Rhodes O. E. (2016). Carcass type affects local scavenger guilds more than habitat connectivity. PloS One 11, e0147798. doi: 10.1371/journal.pone.0147798
Orr M. R., Nelson J. D., Watson J. W. (2019). Heterospecific information supports a foraging mutualism between corvids and raptors. Anim. Behav. 153, 105–113. doi: 10.1016/j.anbehav.2019.05.007
Otto C. M., Cobb M. L., Wilsson E. (2019). Editorial: working dogs: form and function. Front. Veterinary Sci. 6. doi: 10.3389/fvets.2019.00351
Paula J., Leal M. C., Silva M. J., Mascarenhas R., Costa H., Mascarenhas M. (2011). Dogs as a tool to improve bird-strike mortality estimates at wind farms. J. Nat. Conserv. 19, 202–208. doi: 10.1016/j.jnc.2011.01.002
Pearce-Higgins J. W., Humphreys E. M., Burton N. H. K., Atkinson P. W., Pollock C., Clewley G. D. (2023). “Highly pathogenic avian influenza in wild birds in the United Kingdom in 2022: impacts, planning for future outbreaks, and conservation and research priorities,” in British Trust for Ornithology, Joint Nature Conservation Committee (Norfolk: BTO Research Report 752).
Peters K. A., Mizrahi D. S., Allen M. C. (2014). Empirical evidence for factors affecting searcher efficiency and scavenging rates at a coastal, terrestrial wind-power facility. J. Fish Wildlife Manage. 5, 330–339. doi: 10.3996/032014-JFWM-019
Philibert H., Wobeser G., Clark R. G. (1993). COUNTING DEAD BIRDS: EXAMINATION OF METHODS. J. Wildlife Dis. 29, 284–289. doi: 10.7589/0090-3558-29.2.284
Planella A., Palacios V., García E. J., Llaneza L., García-Domínguez F., Muñoz-Igualada J. (2016). Influence of different GPS schedules on the detection rate of wolf feeding sites in human-dominated landscapes. Eur. J. Wildlife Res. 62, 471–478. doi: 10.1007/s10344-016-1020-2
Probst C., Globig A., Knoll B., Conraths F. J., Depner K. (2017). Behaviour of free ranging wild boar towards their dead fellows: potential implications for the transmission of African swine fever. R. Soc. Open Sci. 4, 170054. doi: 10.1098/rsos.170054
Probst C., Gethmann J., Amendt J., Lutz L., Teifke J. P., Conraths F. J. (2020). Estimating the postmortem interval of wild boar carcasses. Veterinary Sci. 7, 6. doi: 10.3390/vetsci7010006
Ratti J. T., Smith L. M., Hupp J. W., Laake J. L. (1983). Line transect estimates of density and the winter mortality of gray partridge. J. Wildlife Manage. 47, 1088. doi: 10.2307/3808168
Reed S. E., Bidlack A. L., Hurt A., Getz W. M. (2011). Detection distance and environmental factors in conservation detection dog surveys: Factors Affecting Conservation Dog Surveys. J. Wildlife Manage. 75, 243–251. doi: 10.1002/jwmg.8
Reynolds M. H., Johnson K. N., Schvaneveldt E. R., Dewey D. L., Uyehara K. J., Hess S. C. (2021). Efficacy of detection canines for avian botulism surveillance and mitigation. Conserv. Sci. Pract. 3, e397. doi: 10.1111/csp2.397
Richards N. L., Tomy G., Kinney C. A., Nwanguma F. C., Godwin B., Woollett D. A. S. (2018). “Using Scat Detection Dogs to Monitor Environmental Contaminants in Sentinel Species and Freshwater Ecosystems”, in Richards N. L. (ed.). Cham: Springer International Publishing, pp. 193–262. doi: 10.1007/978-3-319-77356-8_6
Rietz J., van Beeck Calkoen S. T. S., Ferry N., Schlüter J., Wehner H., Schindlatz K.-H. (2023). Drone-based thermal imaging in the detection of wildlife carcasses and disease management. Transboundary Emerging Dis. 2023, 1–12. doi: 10.1155/2023/5517000
Rivera-Milán F. F., Zaccagnini M. E., Canavelli S. B. (2004). Field trials of line-transect surveys of bird carcasses in agro-ecosystems of Argentina’s Pampas region. Wildlife Soc. Bull. 32, 1219–1228. doi: 10.2193/0091-7648(2004)032[1219:FTOLSO]2.0.CO;2
Santos R. A. L., Santos S. M., Santos-Reis M., Picanç̧ode Figueiredo A., Bager A., Aguiar L. M. S. (2016). Carcass persistence and detectability: reducing the uncertainty surrounding wildlife-vehicle collision surveys. PloS One 11, e0165608. doi: 10.1371/journal.pone.0165608
Schultz J. J., Healy C. A., Parker K., Lowers B. (2013). Detecting submerged objects: The application of side scan sonar to forensic contexts. Forensic Sci. Int. 231, 306–316. doi: 10.1016/j.forsciint.2013.05.032
Selva N., Jedrzejewska B., Jedrzejewski W., Wajrak A. (2005). Factors affecting carcass use by a guild of scavengers in European temperate woodland. Can. J. Zoology 83, 1590–1601. doi: 10.1139/z05-158
Simmons T., Adlam R. E., Moffatt C. (2010). Debugging decomposition data—Comparative taphonomic studies and the influence of insects and carcass size on decomposition rate. J. Forensic Sci. 55, 8–13. doi: 10.1111/j.1556-4029.2009.01206.x
Sinn D. L., Gosling S. D., Hilliard S. (2010). Personality and performance in military working dogs: Reliability and predictive validity of behavioral tests. Appl. Anim. Behav. Sci. 127, 51–65. doi: 10.1016/j.applanim.2010.08.007
Spicka A., Johnson R., Bushing J., Higley L. G., Carter D. O. (2011). Carcass mass can influence rate of decomposition and release of ninhydrin-reactive nitrogen into gravesoil. Forensic Sci. Int. 209, 80–85. doi: 10.1016/j.forsciint.2011.01.002
Stadler S., Stefanuto P.-H. (2013). Characterization of volatile organic compounds from human analogue decomposition using thermal desorption coupled to comprehensive two-dimensional gas chromatography–time-of- flight mass spectrometry. Anal. Chem. 85), 998–1005. doi: 10.1021/ac302614y
Studebaker-Reed M. S. E. (2018). The impact of training and experience on the recovery of evidence in outdoor forensic scenes: implications for human remains recovery. (University of Colorado). Available at: https://hdl.handle.net/2144/31280 (Accessed October 28, 2024). Master of Science.
Teixeira F. Z., Pfeifer Coelho A. V., Esperandio I. B., Kindel A. (2013). Vertebrate road mortality estimates: Effects of sampling methods and carcass removal. Biol. Conserv. 157, 317–323. doi: 10.1016/j.biocon.2012.09.006
Trumbo S. T., Sikes D. S. (2021). Resource concealment and the evolution of parental care in burying beetles. J. Zoology 315, 175–182. doi: 10.1111/jzo.12916
Ward M. R., Stallknecht D. E., Willis J., Conroy M. J., Davidson W. R. (2006). WILD BIRD MORTALITY AND WEST NILE VIRUS SURVEILLANCE: BIASES ASSOCIATED WITH DETECTION, REPORTING, AND CARCASS PERSISTENCE. J. Wildlife Dis. 42, 92–106. doi: 10.7589/0090-3558-42.1.92
Whittington A. E. (2019). Effects of peri-mortem infection on the entomofauna of decomposing buried human remains – a metadata analysis. Sci. Justice 59, 452–458. doi: 10.1016/j.scijus.2019.03.006
Whitworth A., Pinto C., Ortiz J., Flatt E., Silman M. (2022). Flight speed and time of day heavily influence rainforest canopy wildlife counts from drone-mounted thermal camera surveys. Biodiversity Conserv. 31, 3179–3195. doi: 10.1007/s10531-022-02483-w
Zhou C. (2011). Factors and processes causing accelerated decomposition in human cadavers e An overview. J. Forensic Legal Med. 18), 6–9. doi: 10.1016/j.jflm.2010.10.003
Keywords: disease surveillance, disease management, wildlife health, carrion ecology, carcass detection
Citation: Sandor C, Desvaux S, Palumbo L, Larrat S, Charabidze D, D’artois P and Decors A (2025) Benefits and limitations of using innovative tools like detection dogs, thermal imaging and drones to increase wildlife carcass finds during health surveillance and management efforts. Front. Conserv. Sci. 6:1443255. doi: 10.3389/fcosc.2025.1443255
Received: 03 June 2024; Accepted: 07 April 2025;
Published: 02 May 2025.
Edited by:
Eric Stolen, University of Central Florida, United StatesReviewed by:
Hernani Gomes Da Cunha Ramos, Humpback Whale Institute, BrazilNgaio Richards, University of Florida, United States
Copyright © 2025 Sandor, Desvaux, Palumbo, Larrat, Charabidze, D’artois and Decors. This is an open-access article distributed under the terms of the Creative Commons Attribution License (CC BY). The use, distribution or reproduction in other forums is permitted, provided the original author(s) and the copyright owner(s) are credited and that the original publication in this journal is cited, in accordance with accepted academic practice. No use, distribution or reproduction is permitted which does not comply with these terms.
*Correspondence: Anouk Decors, YW5vdWsuZGVjb3JzQG9mYi5nb3V2LmZy