- 1Department of Sports and Sports Science, University of Freiburg, Freiburg, Germany
- 2Department of Physical Performance, Norwegian School of Sport Sciences, Oslo, Norway
Fatigue impairs sensorimotor performance, reduces spinal reflexes and affects the interaction of antagonistic muscles in complex motor tasks. Although there is literature dealing with the interference of fatigue and postural control, the interpretation is confounded by the variety of paradigms used to study it. This study aimed to evaluate the effects of postural fatigue on balance control and strategy, as well as on neuromuscular modulation, in response to postural perturbation (PERT) during a fatiguing balance task. A fatigue protocol consisting of continuous exposure to perturbations until exhaustion was executed in 24 subjects. Number of failed attempts, paths of center of pressure displacement (COP), ankle, knee, and hip joint kinematics, electromyographic activity of the soleus (SOL), tibialis anterior (TA), rectus femoris (RF), vastus lateralis (VL), biceps femoris (BF), and gluteus maximus muscles (GM) and spinal excitability of SOL at the peak of the short-latency responses (SLR) were recorded after posterior PERT. The co-contraction index (CCI) was calculated for TA_SOL, VL_BF and RF_GM. (1) The number of failed attempts significantly increased while COP amplitude and velocity, as well as angular excursion at the ankle, knee and hip joints, decreased with fatigue (P < 0.05). (2) Concomitantly, CCI of SOL_TA, VL_BF and RF_GM increased and spinal excitability in SOL declined. (3) Adaptations progressively augmented with progressing exhaustion and occurred in the distal prior to proximal segment. Distinctly deteriorated balance ability was accompanied by a modified neuromuscular control—the increase in co-contraction reflected by simultaneously activated antagonists is accompanied by smaller knee and hip joint excursions, indicating an elevated level of articular stiffness. These changes may be associated with an exaggerated postural rigidity and could have caused the delayed and reduced postural reactions that are reflected in the changes in COP displacement when compensating for sudden PERT. The reduction in spinal excitability may either be caused by fatigue itself or by an increase in reciprocal inhibition due to augmented TA activity.
Introduction
Body equilibrium is maintained by joint torques, which are controlled by the central nervous system (CNS). Postural adjustment in response to perturbation requires the detection of body segment movements via visual, vestibular and proprioceptive sensory afferents, integration of that information into the CNS and the execution of an appropriate motor response (Dichgans et al., 1976; Nashner, 1976; Nashner and Berthoz, 1978; Dietz, 1992). Fatigue—by definition, an exercise-induced decline in muscle force—affects this sensorimotor coupling and, thus, considerably threatens body equilibrium (Vuillerme et al., 2002; Gribble and Hertel, 2004).
Fatigue-induced deterioration in balance control is associated with an increased sway path (Nardone et al., 1997; Kelly et al., 2005; Berger et al., 2010) and velocity (Kelly et al., 2005; Bisson et al., 2011), augmented variability of the postural response (Bisson et al., 2011), changes in neuromuscular activation reflected by modulated spinal reflexes (Herrmann et al., 2006; Granacher et al., 2010a), and an impaired interaction of antagonistic muscles in complex postural tasks (Berger et al., 2010; Hassanlouei et al., 2012; Kennedy et al., 2012), and hence is related to an increased fall incidence (Helbostad et al., 2007; Granacher et al., 2010a). In the majority of protocols, fatigue was induced throughout repetitive contractions of selective muscles (Gandevia, 2001; Paillard, 2012). Although there are a substantial number of articles dealing with the interference of fatigue and body equilibrium, the interpretation is confounded by the variety of paradigms used to study it. Fatigue protocols vary in duration (20 s to 15 min), level of exhaustion (−5 to 70% of maximal voluntary contraction), selected muscle topography (musculature encompassing ankle, knee, hip, torso, or neck), as well as the number of muscles involved in the fatiguing exercise (local vs. whole body fatigue, for review see Paillard, 2012). So far, no study has concentrated on fatigue effects that occur during prolonged balance tasks. Despite its daily life relevance, experiments have not been executed within a realistic fatiguing postural scenario, yet. Thus, for a conclusive statement about the neuromuscular compensation for balance recovery after—or even during—fatigue, further considerations to elucidate neuromuscular mechanisms of postural fatigue are still needed (Paillard, 2012).
With regard to body equilibrium, investigations assessing the effect of fatigue on the neuromuscular system focused on the redistribution of compensatory muscle activity and resulting reorganization of multi-joint coordination in response to perturbation after exhaustion (Gandevia, 2001; Paillard, 2012). Scientific protocols involve fatiguing repetitions of mono- or poly-segmental movements (i.e., isolated or coupled contractions of tonic muscles, such as the ankle plantar flexors and dorsi flexors or invertors and evertors, knee extensors, hip flexor-extensors, hip abductor-adductors, erector spinae, or neck extensors) or whole body exercise (running, cycling), interfering with postural control (Paillard, 2012). Particular emphasis is put on the reflex arc that governs the operation of immediate muscle contractions, which are of major relevance for a quick readjustment of the body segments to restore equilibrium after perturbation to avoid falls (Granacher et al., 2010a).
Although study findings diverge according to the nature of the protocols, two major conclusions can be drawn. First, the number of muscles stimulated during a fatiguing exercise is related to the magnitude of the postural deterioration (Enoka and Stuart, 1992; Paillard, 2012). Thus, deficits in body equilibrium grow with the number and size of exhausted muscles, ranging from single (focal muscle) to local (mono-articular muscle set) to complex (poly-articular muscle groups) to whole body fatigue (Boyas et al., 2011; Paillard, 2012).
Second, disregarding the protocol and localization of fatigue, muscles of the non-fatigued segments compensate for neuromuscular deficits of the fatigued region. Thus, it can be assumed that fatigue of the proximal musculature (e.g., knee and/or hip muscles) induces the recruitment of distal muscle groups (e.g., ankle muscles) to counteract its disturbing effects on postural control and vice versa (Gribble and Hertel, 2004; Bellew and Fenter, 2006; Salavati et al., 2007; Bizid et al., 2009).
However, there are still questions regarding crucial issues, such as the effect of fatigue on reflex activity, agonist-antagonist muscle coordination and how these aspects are interlinked with neuro-mechanic coupling and balance strategy (Bonnard et al., 1994; Sparto et al., 1997; Herrmann et al., 2006; Berger et al., 2010; Kennedy et al., 2012). For instance, inhomogeneous findings exist for modulations in postural reflex responses. Herrmann et al. (2006) showed a fatigue-induced increase in contrast to Granacher et al. (2010a), who demonstrated a decrease in response to perturbation. In both studies reflex-relevant phase-specific distinctions between postural responses defined as: short (SLR), medium (MLR), and long (LLR) latency reflex responses after perturbation (Horak and Nashner, 1986; Diener et al., 1988; Taube et al., 2006; Rinalduzzi et al., 2015) in addition to H-reflex measures for an assessment of spinal excitability have not yet been implemented (Nielsen and Kagamihara, 1993). The temporal distinction of reflexes, meaning their latency-dependency and ability to modulate on specific levels within the CNS on different pathways, is of high functional relevance (Horak and Nashner, 1986; Diener et al., 1988; Taube et al., 2006; Rinalduzzi et al., 2015). Therefore, it is emphasized that a more comprehensive understanding can be achieved by distinct subdivision of the reflex response, combined with additive methodological approaches, such as peripheral nerve stimulation (PNS, Taube et al., 2006).
Likewise, inconsistencies in antagonistic muscle co-activation in response to fatigue have been reported. On the one hand, some authors have demonstrated an increased co-contraction in the shank muscles (Berger et al., 2010; Granacher et al., 2010a; Kennedy et al., 2012) associated with increased joint stiffening and shifts in balance strategy due to fatigue (Bonnard et al., 1994; Sparto et al., 1997). In contrast, other authors could not confirm these results and demonstrated a decreased co-contraction accompanied by a reduction in joint control (Hassanlouei et al., 2012; Cheng et al., 2015) after fatigue. Although the reasons and consequences for these inconsistencies remain unclear, it can be speculated that contradictions occur due to differences in fatigue protocols that may impact the magnitude and topography of postural impairment (Enoka and Stuart, 1992; Gandevia, 2001).
Despite the widespread relevance of fatigue in different areas of the rehabilitative sports medicine and geriatrics, as well as the substantial number of related articles, the underlying neuromuscular mechanisms in terms of posture control are poorly understood. Therefore, this study aimed to evaluate the effect of postural fatigue on body equilibrium, neuromuscular control, and joint kinematics in response to perturbation. Fatigue symptoms were deliberately elicited within a balance paradigm to benefit from monitoring the chronological progression of fatigue within the exhausting process, considering both regional and temporal differentiation and affecting the relevant muscle groups. We were particularly interested in identifying if and how fatigue is counterbalanced on a neuromuscular and kinematic level and if this neuro-mechanical coupling can be attributed to particular reflex phases or body segments. We hypothesized that modulations in response to fatigue would be phase (SLR, MLR, and LLR) and segment specific (distal and proximal), and may be associated with differences in the balance strategy, accompanied by differences in kinematic output.
Materials and Methods
Experimental Design
We used a single-group repeated-measures study design to evaluate acute effects of fatigue on postural equilibrium, reflex activity, and balance strategy. For this purpose, two protocols were executed randomly on two different days: the fatigue protocol (FAT), consisting of continuous exposure to perturbations until exhaustion, and the volume-matched control protocol (CON), consisting of consecutive 30 s exposure to eight perturbations which are intermitted by 30 s rest periods (Lesinski et al., 2015). Each ith trial of CON was identical with the ith trial of FAT. For those subjects that performed CON before FAT an upper limit was set for CON. After both protocols have been performed, we deleted the last attempts of CON to individually adjust the number of CON trials to the number of FAT trials. For both protocols, subjects stood on their right leg and perturbations were randomly allocated in eight directions (anterior, posterior, medial, lateral and the four diagonals) in intervals of 4–6 s with an amplitude of 3 cm and a velocity of 1.8 m/s on a Perturmed® (Brüderlin, Göppingen, Germany, Freyler et al., 2015). We chose this experimental setting because fatigue symptoms could be elicited in an all-embracing manner spanned over distal and proximal muscles (disregarding body segment, agonist, or antagonist musculature) and the symptoms could be monitored in continuous progression until exhaustion (Paillard, 2012). The stop criterion was a failure rate of 50% within four perturbations. In order to assess the effects of fatigue on balance control, center of pressure (COP) displacement, joint kinematics, electromyographic (EMG) activity, and spinal excitability at the peak of the short-latency response (SLR) were monitored concomitantly during FAT and CON, after respective posterior perturbation of the foot was carried out. One has to note that this posterior perturbation was executed randomly, but uniformly for both FAT and CON. Prior to data collection, the subjects performed isometric maximal voluntary contractions (MVC) for all recorded muscles (see Freyler et al., 2015 for more details about the procedure of MVC collection).
Data sets of the FAT and CON protocols were divided into four periods with within-subject equity in time and trial numbers (T1, T2, T3, and T4): T1 data of the first quarter was averaged, with data for T2 and T3 of the second and third quarter being averaged and lastly the fourth quarter is presented in T4 (Table 1 and Figure 1). The 30 s rest periods for the CON protocol were not relevant for the volume measurements. In this case only the sum of the 30 s perturbation sets were appropriate.
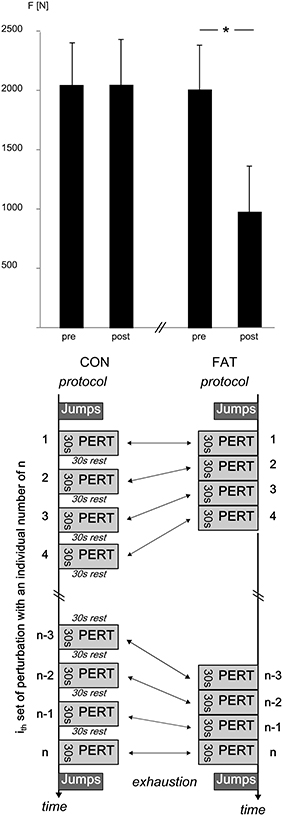
Figure 1. Top: Grand means of peak GRFs [F] before and after the CON and FAT protocol. Peak GRF did not change in response to CON; FAT caused a significant reduction in peak GRF. *Displays a significant difference. Bottom: CON and FAT protocols with n sets for each protocol, all sets contained eight perturbations in eight different directions with each ith set being equal in order and inter-perturbation breaks for FAT and CON. The nth set was the individually last set for each subject before exhaustion.
Subjects
24 subjects (7 females, 17 males, age 25 ± 2 years, height 180 ± 5 cm, weight 74 ± 8 kg; values expressed as mean ± standard deviation) volunteered to participate in this study. All subjects gave written informed consent to the experimental procedure, which was approved by the ethics committee of the University of Freiburg and was in accordance with the latest revision of the Declaration of Helsinki. The subjects were recruited at the sports institute of the University of Freiburg. Thus, we had a homogenous healthy and sportive subpopulation. They were healthy with no previous neurological irregularities or injuries of the lower extremity. A priori, the sample size was estimated by means of a power analysis (f = 0.5; alpha = 0.05; power = 0.8).
Fatigue
As recommended in the literature, strength loss was used as an index of fatigue (Kelly et al., 2005; Paillard, 2012). To monitor fatigue, the subjects performed 10 maximal jumps (both legged hops) before and after FAT and CON on a force platform (Leonardo®, Pforzheim, Deutschland). Peak ground reaction force (GRF) and rate of force development (RFD) was assessed.
Outcome Measures
Number of Failed Attempts
We counted the number of failed attempts. Failed attempts were defined as attempts in which subjects failed to regain postural equilibrium after surface translation and fell (note that due to the safety frame surrounding the subjects, they were secured from falling). Criterions were defined as follows: (i) touching the safety frame of the Perturmed® with at least one hand (Freyler et al., 2015) or (ii) lift off with the unsupported foot to avoid falling. In cases (i) and (ii) subjects would not have been able to regain equilibrium without falling. In contrast, successful attempts were counted when the subjects were able to regain equilibrium within 2 s after surface translation without touching the safety frame. Thus, all attempts in which subjects did not use external support to avoid a fall have been considered to be successful with a regain of equilibrium. Trials were also considered to be successful when subjects: struggled, comprised huge displacements, were swinging, compensated via up or down movements of the COM, demonstrated a fore- or back-wards lean in case they were able to subsequently re-stabilize postural equilibrium.
Postural Sway
Postural sway was quantified by means of a pressure distribution measuring system (Pedar®, Novel, Germany). The sensor mat was placed on the perturbation platform; the COP was recorded by means of 3D sensor deformation with a 100 Hz sampling rate and a spatial resolution of four sensors per square centimeter. COP displacement (COPD) and velocity (COPV) were assessed in the anterior-posterior direction and were averaged over the trials for each subject and each of the five conditions (Cabeza-Ruiz et al., 2011).
Kinematics
Ankle (dorsiflexion and plantar flexion), knee (flexion and extension) and hip (flexion and extension) joint kinematics in the sagittal plane were recorded using electrogoniometers (Biometrics®, Gwent, UK). Goniometers were fixed at the respective joints according to previous research (Ritzmann et al., 2015). All signals were recorded with a sampling frequency of 1 kHz.
EMG Recording
Bipolar Ag/AgCl surface electrodes (Ambu Blue Sensor P, Ballerup, Denmark; diameter 9 mm, center-to-center distance 25 mm) were placed over the M. soleus (SOL), medial gastrocnemius (MG), tibialis anterior (TA), rectus femoris (RF), biceps femoris (BF), vastus medialis (VM), and gluteus maximus (GM) muscles of the right leg. The longitudinal axes of the electrodes were in line with the direction of the underlying muscle fibers. The reference electrode was placed on the patella. Interelectrode resistance was kept below 2.5 kΩ by means of shaving, light abrasion, degreasing and disinfection of the skin. The EMG signals were transmitted to the amplifier (band-pass filter 10 Hz to 1 kHz, 1000x amplified) via shielded cables and recorded with 1 kHz. The cables were carefully taped to the skin.
H-Reflex
Modulation in Ia afferent transmission of the SOL motoneuron pool in response to fatigue was assessed using H-reflex measurements; H-reflexes were elicited via PNS with single rectangular pulses of 1 ms duration (Digitimer DS7, Digitimer, Welwyn Garden City, UK). The cathode (2 cm in diameter) was placed in the popliteal fossa and moved until the best position was found for eliciting an H-reflex in the SOL. The anode (10*5 cm dispersal pad) was fixed directly below the patella on the anterior part of the knee. H-reflexes were elicited by electrically stimulating the posterior tibial nerve. Based on previously recorded H/M recruitment curves, stimulation intensities were set to 25% of the maximal M-wave (Mmax) for all measurements (Crone et al., 1987; Taube et al., 2008). PNS was triggered to occur at the peak of the SLR during posterior perturbation.
Data Processing
Concerning the jumps, GRFs were used to determine the peak GRF (push off and landing threshold 3N) and RFD (peak GRF divided by the time from GC until the force signal reached its peak). The mean value of the 10 jumps was used for statistical analysis (see Ritzmann et al., 2011).
Data sets of the FAT and CON protocols were divided into four periods with within-subject equity in time and trial numbers (T1, T2, T3, and T4); we executed data processing for these periods as follows:
COPD [mm] was calculated for each perturbation as the difference between the COP peak excursion (defined as the maximum value of the COP excursion within the 400 ms window of perturbation) and the onset position expressed as an absolute value. Only the anterior displacement was considered to be of relevance for data processing, due to the COP shifting contrarily to the perturbation direction i.e., a backward translation of the platform caused a forwards shift of the COP and vice versa (Freyler et al., 2015). COPV was calculated according to Freyler et al. (2015): COPV[mm/ms] = COPD/t (t is defined as the time interval from the onset of perturbation to COP peak excursion).
Ankle, knee, and hip joint kinematics were expressed as joint excursions [°] and calculated as the difference between the peak angle position (defined as the maximum value of the angle excursion within the 400 ms window of perturbation) and the onset position (Freyler et al., 2015) for each perturbation.
For each of the recorded muscles, the EMG signals were rectified and integrated (iEMG [mVs]). For data analysis, iEMG was divided into four relevant phases according to literature before and after perturbation: pre-activation–100–0 ms prior to perturbation (PRE), 30–60 ms (SLR, Rinalduzzi et al., 2015), 60–85 ms (MLR), and 85–120 ms (LLR, Taube et al., 2006) post perturbation. Subsequently, the iEMGs were time normalized [mV/s] and normalized to the MVC [%MVC].
In addition, to assess the simultaneous activation of antagonistic muscles encompassing the ankle, knee and hip joint, the co-contraction index (CCI) was calculated for SOL_TA, VM_BF and GM_RF with the rectified and normalized EMG by means of the following equation: CCI = ∑ CCIi, CCIi = ∑ (lower EMGi/higher EMGi) × (lower EMGi+ higher EMGi) for each sample point i, (Lewek et al., 2004). CCIs were expressed for PRE and the entire reflex phase (RP) 30–120 ms after perturbation.
Peak-to-peak amplitudes of the H-reflexes and M-waves were calculated.
For parameters and subjects, we averaged the values for T1, T2, T3, and T4. For EMG, CCI and H-reflexes, we normalized the mean values to T1 for the FAT and CON protocols, respectively.
Statistics
To test for fatigue-induced changes over time, a repeated measures analysis of variance (rmANOVA) was used [time (T1, T2, T3, T4) × protocol (FAT vs. CON)]. The normality of the data was evaluated using Kolmogorov-Smirnov test; data followed a normal distribution. If the assumption of sphericity, as measured via Mauchly's sphericity test, was violated, the Greenhouse-Geisser correction was used. Muscle group (shank vs. thigh) was included as a within-subject factor to detect differences between the CCIs SOL_TA, VM_BF, and GM_RF. Phase was included as a within-subject factor to detect differences between the reflex phases SLR, MLR, and LLR. Segmentation (ankle vs. knee vs. hip) was included as a within-subject factor to detect the dependencies of the different joint flexions of the lower extremities. To correct for multiple testing, we used Bonferroni; each P-value (Pi) for each test was multiplied by the number of tests (Pi adjusted = Pi * n, n = number of tests). If Pi adjusted < 0.05, we considered the respective test i to be of statistical significance. A bivariate two-tailed Pearson correlation analysis was executed to determine the strength of linear relations between the number of failed attempts and the iEMG in the reflex phases SLR, MLR, and LLR for all muscles, the CCIs in RP, and the joint excursions. The false discovery rate was controlled according to the Benjamini–Hochberg–Yekutieli method, a less conservative but still stringent statistical approach conceptualizing the rate of type I errors (Benjamini and Hochberg, 1995; Benjamini and Yekutieli, 2005). All analyses were executed using SPSS 20.0 (SPSS, Inc., Chicago, IL, USA). The values are presented as mean values ± standard deviations (M ± SD).
Results
Changes in peak GRF after FAT and CON are illustrated in Figure 1, grand means for peak GRF and RFD are displayed in Table 1. The rmANOVA revealed a significant time × protocol interaction effect for RFD and peak GRF, indicating a reduced force generation capacity after FAT. Grand means of the failed attempts are displayed in Table 2.
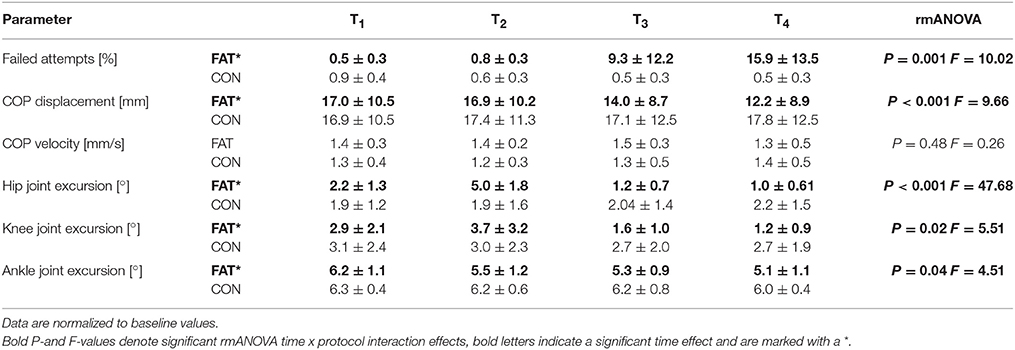
Table 2. Grand means of the failed attempts, anterior center of pressure (COP) displacement and velocity, and hip, knee and ankle joint kinematics are shown: data are displayed for the fatigue (FAT) and control protocol (CON) for the periods T1–T4 (first, second, third, and fourth quarter of the data set including all trial and subjects).
Note that only successful trials were considered for data analysis.
Postural Sway
Fatigue-induced changes in COPD and COPV are displayed in Table 2. The rmANOVA revealed a significant time × protocol interaction effect for COPD, pointing toward a progressively reduced COP displacement in anterior direction with fatigue.
Kinematics
Fatigue-induced changes in joint kinematics are illustrated in Figure 2; grand means are displayed in Table 2. The rmANOVA revealed a significant time × protocol interaction effect for ankle, knee, and hip joint excursions, indicating a progressive decline in joint excursions with fatigue. Furthermore, the rmANOVA revealed a significant interaction effect for time × protocol × segmentation (P = 0.004, F = 8.16) indicating segmental dependencies when compensating perturbation along the lower body segment.
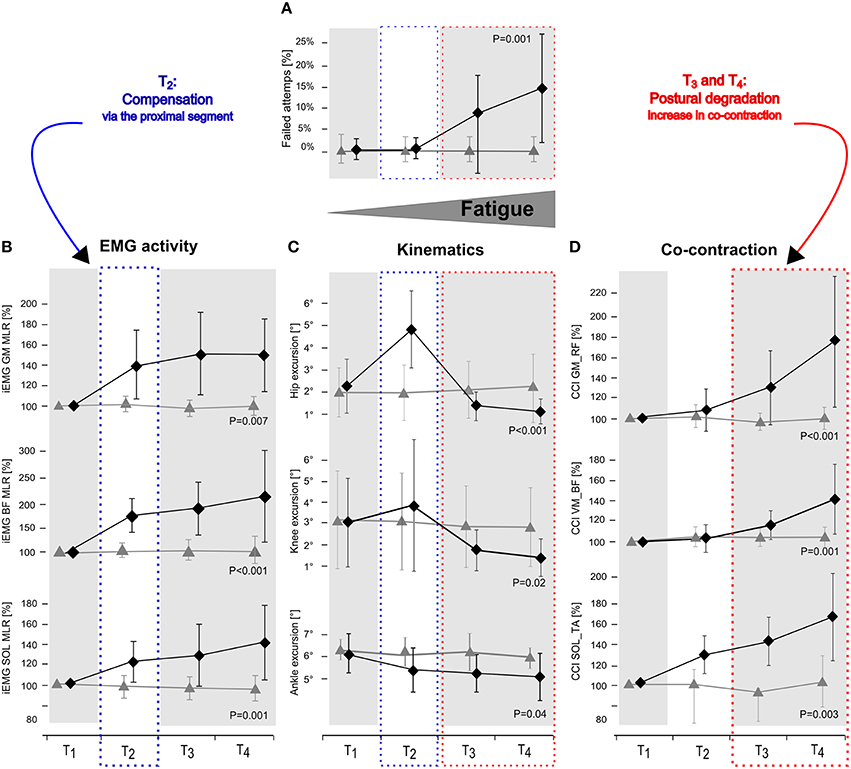
Figure 2. Changes in number of failed attempts (A), EMG activity in GM, BF, and SOL (B), hip, knee, and ankle joint deflection (C) and Co-contraction index (CCI) in the antagonist muscle groups GM_RF, VM_BF, and SOL_TA (D) in response to posterior surface displacement. Grand means (♦ fatigue protocol FAT, ▴ control protocol CON) are illustrated for the periods T1–T4 (first, second, third and fourth quarter: including all trial and subjects). While data showed no changes for CON over time, results reveal segmental compensation for fatigue in T2by augmented knee and hip deflections and an elevated level of neuromuscular activation in GM and BF (blue dotted frame) followed by distinctly reduced knee and hip deflections in T3 and T4 accompanied by increased co-contractions and a raise in number of failed attempts (red dotted frame). P-values are given for time × protocol interaction.
EMG
Fatigue-induced modulations in EMG activity are displayed in Table 3 and Figure 2. The rmANOVA revealed a significant time × protocol × phase interaction effect for GM (P = 0.01, F = 5.22) and TA (P = 0.002, F = 11.81). A significant interaction effect was found for time x protocol x muscle group for PRE (P = 0.007, F = 7.19), indicating topographic differences in the muscle pre-setting. Significant phase- and muscle-specific differences were observed for SOL, TA, GM and BF in each of the recorded reflex phases (SLR, MLR, LLR), as well as for RF and VM in MLR and LLR, indicating a progressive and muscle-specific increase in neuromuscular activation in response to fatigue.
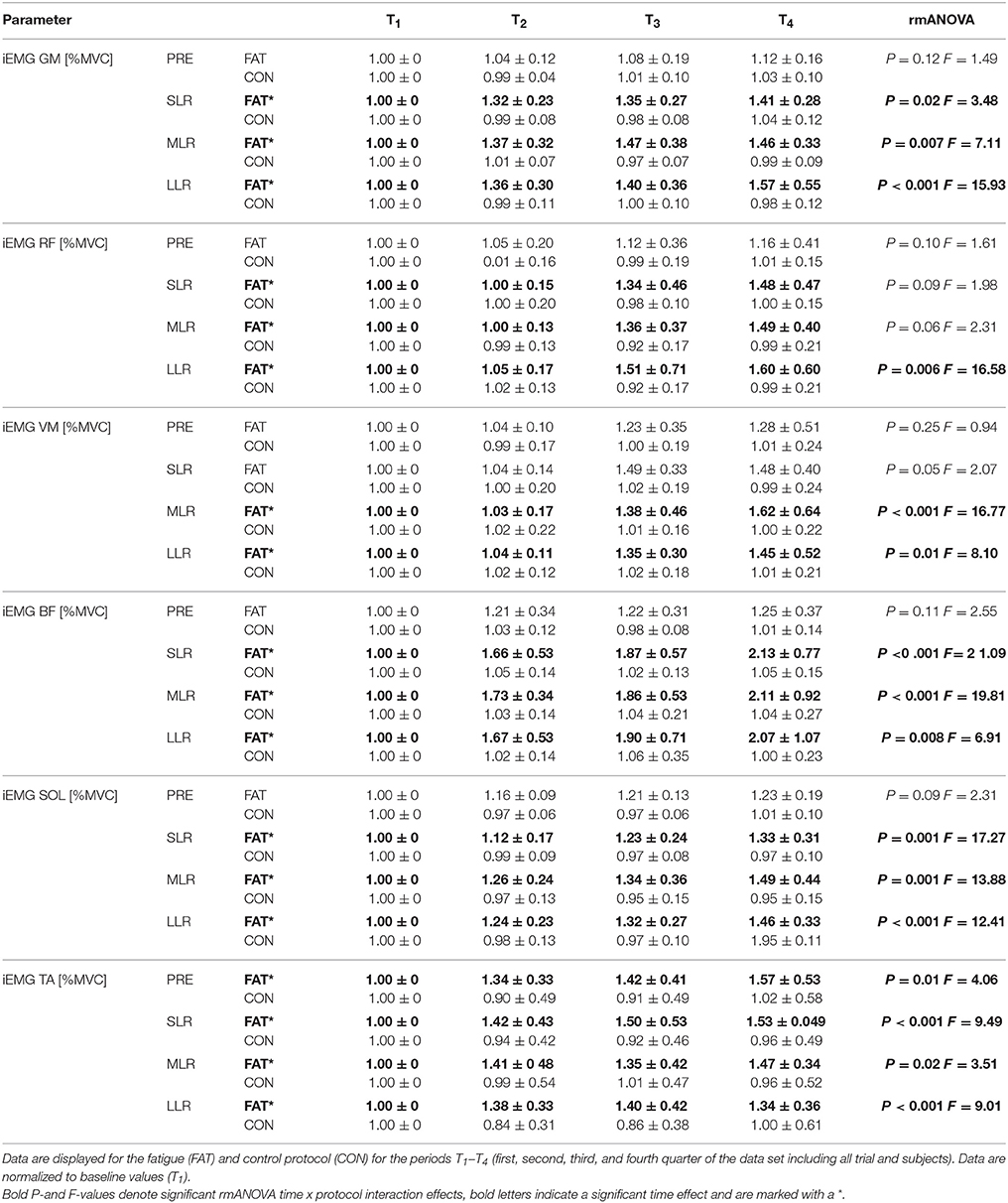
Table 3. Changes in neuromuscular activation: Grand means of the iEMGs of GM, RF, VM, BF, SOL, and TA during pre-activation (PRE) and the relevant reflex phases short-, medium-, and long-latency response (SLR, MLR, and LLR).
Co-Contraction
Fatigue-induced modulations in CCIs are displayed in Table 4 and Figure 2. The rmANOVA revealed a significant time × protocol × muscle group interaction effect for CCI (P = 0.009, F = 6.31), indicating an overall but segment-specific increase in simultaneously activated agonist muscle groups in response to fatigue. Significant interaction effects time x protocol were found for SOL_TA, VM_BF and GM_RF.
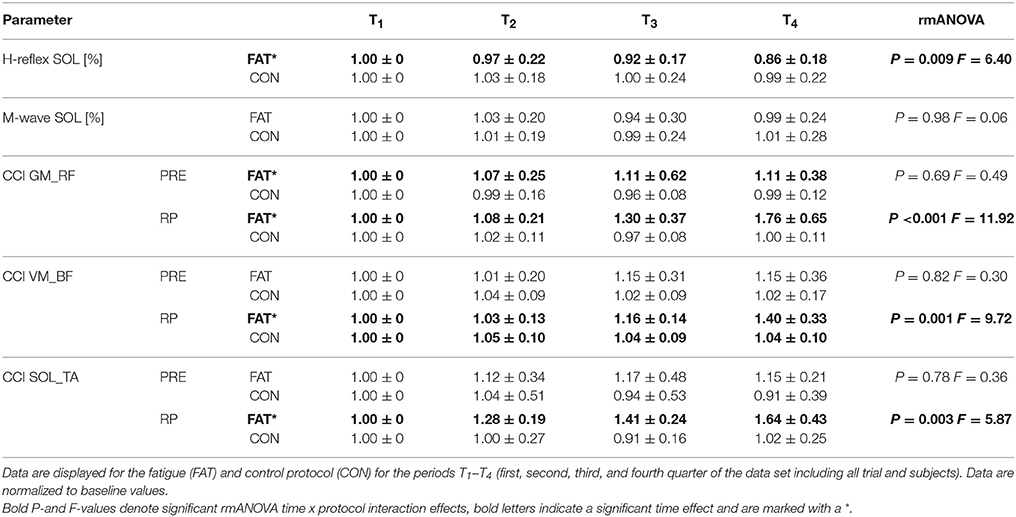
Table 4. Changes in neuromuscular activation: Grand means of H-reflex and M-wave amplitudes as well as co-contraction indices (CCI) for the antagonistic muscle groups encompassing the hip joint (GM_RF), knee joint (VM_BF), and ankle joint (SOL_TA) during pre-activation (PRE) and the entire reflex phase (RP, 30–120ms post perturbation).
H-Reflex
Fatigue-induced modulations in H-Reflex and M-wave amplitudes are displayed in Table 4. The rmANOVA revealed a significant time x protocol interaction effect for the H-reflex amplitude, indicating a fatigue-induced reduction in spinal excitability. M-wave amplitudes and SOL activity during PRE remained unchanged.
Correlations
We detected a significant positive correlation between the number of failed attempts in response to perturbation and CCI of VM_BF (r = 0.49; P = 0.02) and GM_RF (r = 0.72; P = 0.009), indicating that an increased fall incidence is associated with higher antagonistic co-contraction in the proximal limb segment. Furthermore, angular hip excursion was negatively correlated to the number of failed attempts (r = −0.60; P = 0.01). Dependency analysis considering iEMG variables remained insignificant.
Discussion
The objective of this study was to ascertain the effect of postural fatigue on body equilibrium and to compile knowledge about its influence on neuromuscular control and joint kinematics. The study revealed four major findings: Under exhaustion (i) the number of failed attempts in response to perturbation increased while COP amplitude, as well as angular excursions, decreased. These kinematic changes were accompanied by (ii) an increasing co-contraction in the antagonistic muscles encompassing the limb joints and a declined spinal excitability in SOL. (iii) The number of failed attempts positively correlated with antagonistic co-contraction of the upper leg muscles. (iv) All adaptations were progressively augmented with increasing exhaustion and occurred in the distal, prior to proximal, segment.
In contrast to previously published articles in which fatigue was locally induced in particular muscles groups and effects were assessed before and after the protocol (Madigan et al., 2006; Wilson et al., 2006; Kanekar et al., 2008; Berger et al., 2010; Granacher et al., 2010a,b; Bisson et al., 2011; Boyas et al., 2011), in our study, fatigue symptoms were elicited within the balance paradigm in a holistic manner, integrating all body segments. Closer to daily life scenarios when postural equilibrium is deteriorated throughout repetitive balancing, this study gives particular insight into the neuro-mechanical details. This approach is innovative as it allows for monitoring chronological progression of fatigue within the exhausting process, considering both regional and temporal differentiation. Exhaustion was controlled using force measures, demonstrating a loss in Fmax that exceeds 50%, which is sufficiently high for inducing a deterioration of postural control (Kelly et al., 2005; Paillard, 2012). The experiment has been performed in a specific subpopulation of healthy and sportive students with a high-leveled and diversified training. A conclusive statement for other samples such as for geriatric or pathological patients, as well as sedentary, old, adolescent, or rehabilitated subjects cannot be given, as they may respond differently to fatigue.
Fatigue Mechanisms
The fatigue-induced loss in balance performance is associated with a reduced capability to compensate for external perturbation and is reflected by an increased number of failed attempts, referring to fatigue-induced modulations within the neuro-mechanical coupling: a progressively augmented muscle co-contraction caused by simultaneously activated antagonistic muscles encompassing the ankle, knee, and hip joint was observed, concomitant with a rigid articular stiffening of posture. The increased co-contractions may be ascribed to increased motoneuron discharge frequencies and a successive recruitment of motor units (Enoka and Stuart, 1992; Gandevia, 2001), most likely having a substantial impact on spinal excitability: the gradual reduction in H-reflex amplitude is supposed to be attributed to reciprocal inhibition due to an augmented level of activation in the antagonists (Nielsen and Kagamihara, 1993). Reciprocal inhibition is defined as the antagonist alpha motor neuron inhibition, which is evoked by contraction of the agonist muscle (Crone et al., 1987). As TA activity increases with fatigue-induced exhaustion (Table 2), SOL motoneuron excitability may be inhibited and Ia afferent transmission reduced.
Assessing posture in light of neuromuscular aspects, it is known from the literature that subjects with a greater antagonistic co-contraction of leg muscles, coupled with diminished articular deflections, and COP displacements in response to perturbation, display an augmented fall incidence compared to subjects with a smaller co-contraction (Bruhn et al., 2004; Hortobágyi et al., 2009; Nagai et al., 2011; Sayenko et al., 2012). This may be associated with the number of failed attempts, which although rejected for data analysis, may be associated with the presetting of co-contracted musculature (Bruhn et al., 2004; Hortobágyi et al., 2009; Nagai et al., 2011; Sayenko et al., 2012). We, therefore, argue that such fatigue-induced rigid joint stiffening could compromise the ability to react precisely to sudden surface displacements and to move the center of mass accurately above the base of support (Figure 2), and hence could explain the decline in balance performance in T3 and T4 in our study (Allum et al., 2002; Tucker et al., 2008).
As only successful trials were analyzed and failed attempts were excluded from data processing, although failures increased over time in FAT, one could argue that this may cause confounding effects. Particularly, as the number of trials in FAT and CON differed by the number of failed attempts. Failed attempts were defined as falling attempts without a regain of balance. They were not considered for data analysis because we were interested in how our healthy sample compensates for fatigue symptoms without losing balance. Thus, we wanted to know which maneuvers and strategies dominate, when postural safety is required although subjects are impaired due to fatigue-induced modulations reducing the normal motor repertoire.
Fatigue Compensation: Absorption in the Upper Leg Segment
Our results furthermore indicate that postural fatigue was compensated for in T2: The number of failed attempts remained unchanged in T2, although fatigue-induced effects in EMG and kinematics within the lower limb segment were evident (Figure 2). It is supposed that in particular the increased EMG activity of BF and GM in T2, concomitant with distinctly augmented joint deflections in the knee and hip joints, indicating a shift in activation topography from distal (e.g., ankle muscles) to proximal (e.g., knee and/or hip muscles) muscles that absorbed distal deficits and helped to counteract perturbations to keep the number of failed attempts low as indicated by the interaction effect of segmentation. In conjunction with Wilson et al. (2006), who ascertained a general fatigue-induced shift toward the hip strategy, it can be emphasized that distal impairments can be absorbed at the proximal region (Figure 2). In Concerning the underlying mechanisms, activation intensities were particularly high in MLR and LLR. Both reflex phases are supposed to rely on polysynaptic pathways with functional significance to induce appropriate active joint moments for the preservation of postural stability (Nashner, 1977; Horak and Nashner, 1986; Dietz et al., 1988, 1989). Thus, our findings indicate that a reacquisition of center of mass stabilization relies on using long loop reflexes, with the goal to control trunk movements in order to achieve a fast balance recovery in response to surface translation, absorbs fatigue-induced distal deficits (Szturm and Fallang, 1998).
Fatigue Impact: Segmental Distinction
Failed attempts started to considerably appear in T3 and were progressively augmented with increasing exhaustion in T4. Correlations additionally indicate that an increased fall incidence interrelates with higher upper limb, but not lower limb, co-contraction and rigidity, or any other change in neuromuscular activation. Hence, based on our results, we assume that monopedal equilibrium is less disturbed as a result of fatigue in the distal compared to the proximal musculature. This observation is in accordance with previous literature regarding balance research. There is evidence that fatigue symptoms elicited in hip or knee musculature affect postural control, while fatigue of muscles encompassing the ankle joint has less or the same impact on body equilibrium (Miller and Bird, 1976; Gribble and Hertel, 2004; Salavati et al., 2007; Bizid et al., 2009; Bisson et al., 2011). This is also in line with the interaction effect of segmentation: As the lower extremities' segments are interconnected, deficits in neuromuscular activation may cause dependencies reflected in joint deflections (Freyler et al., 2015). Thus, our findings reveal that postural fatigue is a length-dependent phenomenon occurring in the distal prior to the proximal musculature. We observed a reorganization of multi-joint coordination, recruiting proximal muscles, and using the upper limb segment to efficiently compensate for distal deficits.
In conclusion, outcomes address functional relevance and practical application of postural fatigue, identifying two major characteristics within chronological progression of exhaustion in healthy and active subjects: At first, fatigue causes a redistribution of active muscles and a reorganization of multi-joint coordination to stabilize equilibrium. As postural fatigue occurred as a length-dependent phenomenon appearing in the distal prior to the proximal musculature, a priori, the neuromuscular system takes advantage of a shift from the distal to proximal segment for controlling posture and fall avoidance. Second, in contrast to proximal deficits, distal fatigue was not neglectable and considerably affected postural safety and increased fall incidence. For postural safety, in conjunction with fatigue, muscles encompassing the hip, and knee joint seem to be of superior importance.
It may be speculated that elderly, geriatric, and adolescent subjects or patient groups may respond differently to fatigue compared to our healthy and well-trained sample. As a prospective for further research focusing on the interrelation of fatigue and posture control, investigations in a wider spectrum of subjects presenting different subpopulations would be of particular interest.
Author Contributions
All authors RR, KF, AW, and AG made substantial contributions to the conception or design of the work, the acquisition, analysis, and interpretation of data for the work. Further they contributed drafting the work and revising it critically, they helped with the final approval of the version to be published and made the agreement to be accountable for all aspects of the work in ensuring that questions related to the accuracy or integrity of any part of the work are appropriately investigated and resolved.
Conflict of Interest Statement
The authors declare that the research was conducted in the absence of any commercial or financial relationships that could be construed as a potential conflict of interest.
The Review Editor TK and handling Editor JS declared their shared affiliation, and the handling Editor states that the process nevertheless met the standards of a fair and objective review.
Acknowledgments
The article processing charge was funded by the open access publication fund of the Albert-Ludwigs-University Freiburg.
References
Allum, J., Zamani, F., Adkin, A. L., and Ernst, A. (2002). Differences between trunk sway characteristics on a foam support surface and on the Equitest® ankle-sway-referenced support surface. Gait Posture 16, 264–270. doi: 10.1016/s0966-6362(02)00011-5
Bellew, J. W., and Fenter, P. C. (2006). Control of balance differs after knee or ankle fatigue in older women. Arch. Phys. Med. Rehab. 87, 1486–1489. doi: 10.1016/j.apmr.2006.08.020
Benjamini, Y., and Hochberg, Y. (1995). Controlling the false discovery rate: a practical and powerful approach to multiple testing. J. R. Stat. Soc. Series B 57, 289–300. doi: 10.2307/2346101
Benjamini, Y., and Yekutieli, D. (2005). False discovery rate-adjusted multiple confidence intervals for selected parameters. J. Am. Stat. Assoc. 100, 71–81. doi: 10.1198/016214504000001907
Berger, L. L., Regueme, S. C., and Forestier, N. (2010). Unilateral lower limb muscle fatigue induces bilateral effects on undisturbed stance and muscle EMG activities. J. Electromyogr. Kinesiol. 20, 947–952. doi: 10.1016/j.jelekin.2009.09.006
Bisson, E. J., McEwen, D., Lajoie, Y., and Bilodeau, M. (2011). Effects of ankle and hip muscle fatigue on postural sway and attentional demands during unipedal stance. Gait Posture 33, 83–87. doi: 10.1016/j.gaitpost.2010.10.001
Bizid, R., Jully, J. L., Gonzalez, G., Francois, Y., Dupui, P., and Paillard, T. (2009). Effects of fatigue induced by neuromuscular electrical stimulation on postural control. J. Sci. Med. Sports 12, 60–66. doi: 10.1016/j.jsams.2007.10.006
Bonnard, M., Sirin, A. V., Oddsson, L., and Thorstensson, A. (1994). Different strategies to compensate for the effects of fatigue revealed by neuromuscular adaptation processes in humans. Neurosci. Lett. 166, 101–105. doi: 10.1016/0304-3940(94)90850-8
Boyas, S., Remaud, A., Bisson, E. J., Cadieux, S., Morel, B., and Bilodeau, M. (2011). Impairment in postural control is greater when ankle plantarflexors and dorsiflexors are fatigued simultaneously than when fatigued separately. Gait Posture 34, 254–259. doi: 10.1016/j.gaitpost.2011.05.009
Bruhn, S., Kullmann, N., and Gollhofer, A. (2004). The effects of a sensorimotor training and a strength training on postural stabilisation, maximum isometric contraction and jump performance. Int. J. Sports Med. 25, 56–60. doi: 10.1055/s-2003-45228
Cabeza-Ruiz, R., Garcia-Masso, X., Centeno-Prada, R. A., Beas-Jimenez, J. D., Colado, J. C., and Gonzalez, L. M. (2011). Time and frequency analysis of the static balance in young adults with Down syndrome. Gait Posture 33, 23–28. doi: 10.1016/j.gaitpost.2010.09.014
Cheng, C. H., Chien, A., Hsu, W. L., Yen, L. W., Lin, Y. H., and Cheng, H. Y. (2015). Changes of postural control and muscle activation pattern in response to external perturbations after neck flexor fatigue in young subjects with and without chronic neck pain. Gait Posture 41, 801–807. doi: 10.1016/j.gaitpost.2015.02.007
Crone, C., Hultborn, H., Jespersen, B., and Nielsen, J. (1987). Reciprocal Ia inhibition between ankle flexors and extensors in man. J. Physiol. 389, 163–185. doi: 10.1113/jphysiol.1987.sp016652
Dichgans, J., Mauritz, K. H., Allum, J. H., and Brandt, T. (1976). Postural sway in normals and atactic patients: analysis of the stabilising and destabilizing effects of vision. Agressologie 17, 15–24.
Diener, H. C., Horak, F. B., and Nashner, L. M. (1988). Influence of stimulus parameters on human postural responses. J. Neurophysiol. 59, 1888–1905.
Dietz, V. (1992). Human neuronal control of automatic functional movements: interaction between central programs and afferent input. Physiol. Rev. 72, 33–69.
Dietz, V., Horstmann, G., and Berger, W. (1988). Involvement of different receptors in the regulation of human posture. Neurosci. Lett. 94, 82–87. doi: 10.1016/0304-3940(88)90274-1
Dietz, V., Horstmann, G. A., and Berger, W. (1989). Interlimb coordination of leg-muscle activation during perturbation of stance in humans. J. Neurophysiol. 62, 680–693.
Enoka, R. M., and Stuart, D. G. (1992). Neurobiology of muscle fatigue. J. Appl. Physiol. 72, 1631–1648.
Freyler, K., Gollhofer, A., Colin, R., Brüderlin, U., and Ritzmann, R. (2015). Reactive balance control in response to perturbation in unilateral stance: interaction effects of direction, displacement and velocity on compensatory neuromuscular and kinematic responses. PLoS ONE 10:e0144529. doi: 10.1371/journal.pone.0144529
Gandevia, S. C. (2001). Spinal and supraspinal factors in human muscle fatigue. Physiol. Rev. 21, 1725–1789.
Granacher, U., Gruber, M., Förderer, D., Strass, D., and Gollhofer, A. (2010a). Effects of ankle fatigue on functional reflex activity during gait perturbations in young and elderly men. Gait Posture 32, 107–112. doi: 10.1016/j.gaitpost.2010.03.016
Granacher, U., Wolf, I., Wehrle, A., Bridenbaugh, S., and Kressig, R. W. (2010b). Effects of muscle fatigue on gait characteristics under single and dual-task conditions in young and older adults. J. Neuroeng. Rehabil. 7:56. doi: 10.1186/1743-0003-7-56
Gribble, P. A., and Hertel, J. (2004). Effect of hip and ankle muscle fatigue on unipedal postural control. J. Electromyogr. Kinesiol. 14, 641–646. doi: 10.1016/j.jelekin.2004.05.001
Hassanlouei, H., Arendt-Nielsen, L., Kersting, U. G., and Falla, D. (2012). Effect of exercise-induced fatigue on postural control of the knee. J. Electromyogr. Kinesiol. 22, 342–347. doi: 10.1016/j.jelekin.2012.01.014
Helbostad, J. L., Leirfall, S., Moe-Nilssen, R., and Sletvold, O. (2007). Physical fatigue affects gait characteristics in older persons. J. Gerontol. 62A, 1010–1015. doi: 10.1093/gerona/62.9.1010
Herrmann, C. M., Madigan, M. L., Davidson, B. S., and Granata, K. P. (2006). Effect of lumbar extensor fatigue on paraspinal muscle reflexes. J. Electromyogr. Kinesiol. 16, 637–641. doi: 10.1016/j.jelekin.2005.11.004
Horak, F. B., and Nashner, L. M. (1986). Central programming of postural movements: adaptation to altered support-surface configurations. J. Neurophysiol. 55, 1369–1381.
Hortobágyi, T., Solnik, S., Gruber, A., Rider, P., Steinweg, K., Helseth, J., et al. (2009). Interaction between age and gait velocity in the amplitude and timing of antagonist muscle coactivation. Gait Posture 29, 558–564. doi: 10.1016/j.gaitpost.2008.12.007
Kanekar, N., Santos, M. J., and Aruin, A. S. (2008). Anticipatory postural control following fatigue of postural and focal muscles. Clin. Neurophysiol. 119, 2304–2313. doi: 10.1016/j.clinph.2008.06.015
Kelly, M. H., Mattacola, C. G., Uhl, T. L., Malone, T. R., and McCrory, J. L. (2005). Effects of 2 ankle fatigue models on the duration of postural stability dysfunction. J. Athl. Train. 40, 191–196.
Kennedy, A., Guevel, A., and Sveistrup, H. (2012). Impact of ankle muscle fatigue and recovery on the anticipatory postural adjustments to externally initiated perturbations in dynamic postural control. Exp. Brain Res. 223, 553–562. doi: 10.1007/s00221-012-3282-6
Lesinski, M., Hortobagyi, T., Muehlbauer, T., Gollhofer, A., and Granacher, U. (2015). Dose-response relationships of balance training in healthy young adults: a systematic review and meta-analysis. Sports Med. 45, 557–576. doi: 10.1007/s40279-014-0284-5
Lewek, M. D., Rudolph, K. S., and Snyder-Mackler, L. (2004). Control of frontal plane knee laxity during gait in patients with medial compartment knee osteoarthritis. Osteoarthr. Cartilage 12, 745–751. doi: 10.1016/j.joca.2004.05.005
Madigan, M. L., Davidson, B. S., and Nussbaum, M. A. (2006). Postural sway and joint kinematics during quiet standing are affected by lumbar extensor fatigue. Hum. Mov. Sci. 25, 788–799. doi: 10.1016/j.humov.2006.04.004
Miller, P. K., and Bird, A. M. (1976). Localized muscle fatigue and dynamic balance. Percept. Mot. Skills 42, 135–138. doi: 10.2466/pms.1976.42.1.135
Nagai, K., Yamada, M., Uemura, K., Yamada, Y., Ichihashi, N., and Tsuboyama, T. (2011). Differences in muscle coactivation during postural control between healthy older and young adults. Arch. Gerontol. Geriatr. 53, 338–343. doi: 10.1016/j.archger.2011.01.003
Nardone, A., Tarantola, J., Giordano, A., and Schieppati, M. (1997). Fatigue effects on body balance. Electroencephalogr. Clin. Neurophysiol. 105, 309–320. doi: 10.1016/s0924-980x(97)00040-4
Nashner, L., and Berthoz, A. (1978). Visual contribution to rapid motor responses during postural control. Brain Res. 150, 403–407. doi: 10.1016/0006-8993(78)90291-3
Nashner, L. M. (1976). Adapting reflexes controlling the human posture. Exp. Brain Res. 26, 59–72. doi: 10.1007/BF00235249
Nashner, L. M. (1977). Fixed patterns of rapid postural responses among leg muscles during stance. Exp. Brain Res. 30, 13–24. doi: 10.1007/BF00237855
Nielsen, J., and Kagamihara, Y. (1993). The regulation of presynaptic inhibition during co-contraction of antagonistic muscles in man. J. Physiol. 464, 575–593.
Paillard, T. (2012). Effects of general and local fatigue on postural control: a review. Neurosci. Biobehav. Rev. 36, 162–176. doi: 10.1016/j.neubiorev.2011.05.009
Rinalduzzi, S., Trompetto, C., Marinelli, L., Alibardi, A., Missori, P., Fattapposta, F., et al. (2015). Balance dysfunction in Parkinson's disease. Biomed Res. Int. 2015:434683. doi: 10.1155/2015/434683
Ritzmann, R., Freyler, K., Weltin, E., Krause, A., and Gollhofer, A. (2015). Load dependency of postural control - kinematic and neuromuscular changes in response to over and under load conditions. PLoS ONE 6:e128400. doi: 10.1371/journal.pone.0128400
Ritzmann, R., Kramer, A., Gollhofer, A., and Taube, W. (2011). The effect of whole body vibration on the H-reflex, the stretch reflex, and the short-latency response during hopping. Scand. J. Med. Sci. Sports 23, 331–339. doi: 10.1111/j.1600-0838.2011.01388.x
Salavati, M., Moghadam, M., Ebrahimi, I., and Arab, A. M. (2007). Changes in postural stability with fatigue of lower extremity frontal and sagittal plane movers. Gait Posture 26, 214–218. doi: 10.1016/j.gaitpost.2006.09.001
Sayenko, D. G., Masani, K., Vette, A. H., Alekhina, M. I., Propovic, M. R., and Nakazawa, K. (2012). Effects of balance training with visual feedback during mechanically unperturbed standing on postural corrective responses. Gait Posture 35, 339–344. doi: 10.1016/j.gaitpost.2011.10.005
Sparto, P. J., Parnianpour, M., Reinsel, T. E., and Simon, S. (1997). The effect of fatigue on multijoint kinematics, coordination, and postural stability during a repetitive lifting test. J. Orthop. Sports Phys. 25, 3–12. doi: 10.2519/jospt.1997.25.1.3
Szturm, T., and Fallang, B. (1998). Effects of varying acceleration of platform translation and toes-up rotations on the pattern and magnitude of balance reactions in humans. J. Vestibul. Res. Equil. 8, 381–397. doi: 10.1016/S0957-4271(97)00087-6
Taube, W., Gruber, M., and Gollhofer, A. (2008). Spinal and supraspinal adaptations associated with balance training and their functional relevance. Acta Physiol. 193, 101–106. doi: 10.1111/j.1748-1716.2008.01850.x
Taube, W., Schubert, M., Gruber, M., Beck, S., Faist, M., and Gollhofer, A. (2006). Direct cortical pathways contribute to neuromuscular control of perturbed stance. J. Appl. Physiol. 101, 420–429. doi: 10.1152/japplphysiol.01447.2005
Tucker, M. G., Kavanagh, J. J., Barrett, R. S., and Morrison, S. (2008). Age-related differences in postural reaction time and coordination during voluntary sway movements. Hum. Mov. Sci. 27, 728–737. doi: 10.1016/j.humov.2008.03.002
Vuillerme, N., Danion, F., Forestier, N., and Nougier, V. (2002). Postural sway under muscle vibration and muscle fatigue in humans. Neurosci. Lett. 333, 131–135. doi: 10.1016/S0304-3940(02)00999-0
Keywords: postural control, perturbation, electromyography, center of pressure, co-contraction, H-reflex, plasticity, sensorimotor control
Citation: Ritzmann R, Freyler K, Werkhausen A and Gollhofer A (2016) Changes in Balance Strategy and Neuromuscular Control during a Fatiguing Balance Task—A Study in Perturbed Unilateral Stance. Front. Hum. Neurosci. 10:289. doi: 10.3389/fnhum.2016.00289
Received: 02 December 2015; Accepted: 30 May 2016;
Published: 14 June 2016.
Edited by:
Jae Kun Shim, University of Maryland College Park, USAReviewed by:
Giancarlo Zito, Laboratory of Electrophysiology for Translational NeuroScience, National Research Council, Fatebenefratelli Hospital, ItalyTim Kiemel, University of Maryland, USA
Copyright © 2016 Ritzmann, Freyler, Werkhausen and Gollhofer. This is an open-access article distributed under the terms of the Creative Commons Attribution License (CC BY). The use, distribution or reproduction in other forums is permitted, provided the original author(s) or licensor are credited and that the original publication in this journal is cited, in accordance with accepted academic practice. No use, distribution or reproduction is permitted which does not comply with these terms.
*Correspondence: Kathrin Freyler, a2F0aHJpbi5mcmV5bGVyQHNwb3J0LnVuaS1mcmVpYnVyZy5kZQ==