- Graduate School of Pharmaceutical Sciences, The University of Tokyo, Tokyo, Japan
The prefrontal cortex (PFC), located at the anterior region of the cerebral cortex, is a multimodal association cortex essential for higher-order brain functions, including decision-making, attentional control, memory processing, and regulation of social behavior. Structural, circuit-level, and functional abnormalities in the PFC are often associated with neurodevelopmental disorders. Here, we review recent findings on the postnatal development of the PFC, with a particular emphasis on rodent studies, to elucidate how its structural and circuit properties are established during critical developmental windows and how these processes influence adult behaviors. Recent evidence also highlights the lasting effects of early life stress on the PFC structure, connectivity, and function. We explore potential mechanisms underlying these stress-induced alterations, with a focus on epigenetic regulation and its implications for PFC maturation and neurodevelopmental disorders. By integrating these insights, this review provides an overview of the developmental processes shaping the PFC and their implications for brain health and disease.
Introduction
The prefrontal cortex (PFC) is a brain region located in the anterior part of the frontal lobe. In primates, including humans, the PFC is subdivided into distinct subregions such as the medial PFC (mPFC), lateral PFC (lPFC), and orbitofrontal cortex (oFC), each of which contributes to higher-order brain functions, including decision-making, social behavior, memory processing, attentional regulation, and emotional control (Siddiqui et al., 2008; Kolk and Rakic, 2022; Preuss and Wise, 2022). In rodents, the PFC is thought to be composed of the mPFC [infralimbic cortex (IL), prelimbic cortex (PL), and anterior cingulate cortex (ACC)] and oFC, but probably lacking the anatomical equivalent of dorsolateral PFC in primates (Le Merre et al., 2021; Kolk and Rakic, 2022). The PFC integrates and processes information from a wide range of brain regions (Figure 1), enabling it to coordinate functions essential for adaptive behavior (Miller and Cohen, 2001; Friedman and Robbins, 2022). Dysfunction of the PFC has been implicated in the pathophysiology of various neuropsychiatric disorders, including schizophrenia, depression, and autism spectrum disorder (Siddiqui et al., 2008; Xu et al., 2019).
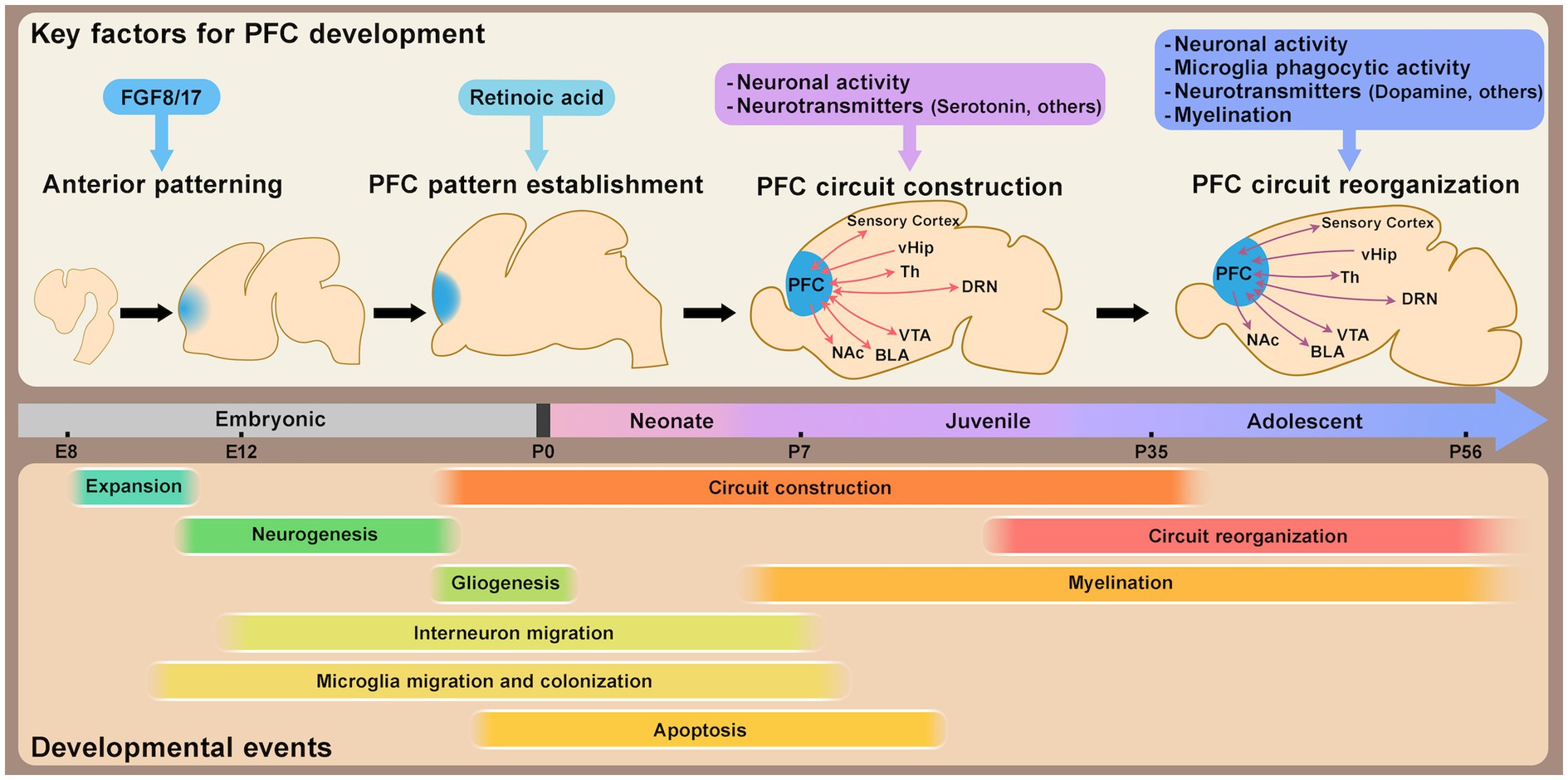
Figure 1. Schematic timeline of mouse prefrontal cortex (PFC) development. This schematic illustrates the approximate timeline of key developmental events shaping PFC structural and circuit organization, along with major factors regulating PFC development and maturation. PFC patterning is largely established by the perinatal stage. Circuit formation progresses from the neonatal stage through adolescence, with synaptic refinement and circuit reorganization occurring during both the juvenile and adolescent stages. Abbreviations: vHip, ventral hippocampus; Th, thalamus; DRN, dorsal raphe nucleus; VTA, ventral tegmental area; BLA, basolateral amygdala; NAc, nucleus accumbens; E, embryonic day; P, postnatal day.
Despite significant evolutionary diversity, certain subdivisions of the PFC demonstrate structural homology across mammals, including humans, non-human primates, and rodents (Preuss and Wise, 2022). In humans, the PFC is particularly enlarged, with its proportion relative to total brain volume being notably greater than in other mammals, even among primates (Donahue et al., 2018). This unique expansion is thought to support advanced cognitive abilities. Although the rodent PFC differs substantially from that of primates, functional similarities in certain circuits, such as the prefrontal-hippocampal circuit for working memory and the prefrontal-amygdala circuit for social behaviors (Tsutsui et al., 2016; Gangopadhyay et al., 2021), provide valuable insights into its conserved functions across species (Ongür and Price, 2000; Euston et al., 2012; Carlén, 2017; Chini and Hanganu-Opatz, 2021). Additionally, the feasibility of manipulation with advanced genetic and molecular tools makes rodents, particularly mice, an indispensable model for studying PFC circuits and functions. Recent studies also demonstrate that rodent models effectively replicate neural circuits and behaviors relevant to neurodevelopmental disorders, underscoring their importance in PFC research (Schubert et al., 2015; Chini and Hanganu-Opatz, 2021). This review draws primarily on findings from rodent studies, specifically in mice and rats, to outline how the structure and circuits of the PFC are established during development.
Early life stress (ELS), such as abuse or social isolation during infancy, childhood, or adolescence, has been linked to an increased risk of psychiatric disorders, including depression, anxiety, impaired social skills, and memory deficits later in life. Studies in both humans and rodents suggest that specific developmental windows are particularly vulnerable to the effects of stress (Schroeder et al., 2018; Nestler and Russo, 2024). The PFC, characterized by its protracted maturation timeline and heightened postnatal plasticity (Chini and Hanganu-Opatz, 2021; Klune et al., 2021), is believed to be especially susceptible to stress during these critical periods. This vulnerability underscores the potential impact of ELS on mental health. This review provides an overview of PFC circuit maturation mechanisms across distinct postnatal stages and explores how ELS disrupts PFC structure, circuitry, and function. The long-term effects of stress may involve epigenetic modifications, which have the potential to induce persistent changes in gene expression. Notably, findings from genome-wide association studies (GWAS) and related analyses indicate that genetic risk factors for psychiatric disorders—such as autism spectrum disorders, schizophrenia, and intellectual disabilities—are enriched in genes associated with epigenetic regulation and transcriptional control (Najmabadi et al., 2011; Satterstrom et al., 2020; Singh et al., 2022), highlighting their potential role in PFC development and disease vulnerability. Building on these findings, this review also examines the interplay between epigenetic regulation, PFC maturation, and stress responses.
Patterning, cell type specification, and cytoarchitecture of the prefrontal cortex
The patterning of the cerebral cortex, which is largely conserved across mammalian species (Krubitzer and Seelke, 2012), is established primarily by morphogen concentration gradients that develop along the anterior–posterior and medial-lateral axes during early embryonic development (O’Leary et al., 2007; Greig et al., 2013; Cadwell et al., 2019). At this stage, neural progenitor cells are in an expansion phase, undergoing symmetric cell division to increase their population before differentiating into specialized cell types (Miyata et al., 2010; Greig et al., 2013). The expression patterns of transcription factors in neural progenitor cells, shaped by these morphogen gradients, lay the foundation for cortical patterning. In the anterior region of the mouse brain, including the prefrontal cortex (PFC), fibroblast growth factors (FGFs), such as FGF8 and FGF17, play critical roles. FGF8 is primarily associated with anteriorization (Fukuchi-Shimogori and Grove, 2001), while FGF17 is involved in the compartmentalization of anterior subdivisions (Cholfin and Rubenstein, 2007) (Figure 1). Recent studies have highlighted the role of retinoic acid (RA) signaling in establishing PFC patterning from the embryonic stage to the perinatal period, and subsequently affecting synaptogenesis and thalamo-prefrontal connectivity (Shibata et al., 2021a). Interestingly, RA signaling appears to be differentially regulated in mice and humans, with evolutionary changes in the enhancer region of Cbln2, a PFC marker, suggested to contribute to interspecies differences in PFC patterning and layer organization (Shibata et al., 2021b) (Figure 1). Since variations in PFC size and organization are often observed in patients with neurodevelopmental disorders (Glahn et al., 2008; De La Fuente et al., 2013; Bakhshi and Chance, 2015; Courchesne et al., 2019; Hashem et al., 2020; Zhao et al., 2022), understanding the mechanisms underlying PFC patterning could provide insights into the pathophysiology of these conditions.
The specification of cell types in the PFC follows a developmental program similar to other cortical areas. After the expansion phase, neural stem cells undergo the neurogenic phase, producing neurons mainly through asymmetric division, followed by the gliogenic phase, where glial cells such as astrocytes or oligodendrocytes are generated (Miyata et al., 2010; Greig et al., 2013). The production of deeper cortical layers precedes that of upper layers, reflecting a well-defined temporal sequence (Greig et al., 2013). Excitatory neurons in each layer of the PFC eventually form layer-specific connections to different brain regions governing specific functions (Murugan et al., 2017). From an evolutionary perspective, significant differences in the granular layer 4 (L4) are evident among species (Le Merre et al., 2021; Preuss and Wise, 2022). In primates, L4 in the PFC is characterized by a well-defined granular cell layer as in other cortical areas, but the granular L4 is largely absent in the PFC of rodents. In addition to excitatory neurons, cortical interneurons, which originate from the ventral telencephalon, migrate into the cortical layers during the perinatal stage (Wamsley and Fishell, 2017; De Marco García and Fishell, 2024), affecting excitatory-inhibitory balance later in postnatal stages (Caballero et al., 2021). Microglia, known for their roles in synaptic development and plasticity (Wu et al., 2015), invade the cortical parenchyma early during embryonic development and continue to proliferate and organize postnatally in mice (Thion et al., 2018). By approximately one or two weeks after birth in rodents, the fundamental cell types in the cerebral cortex are established and their migration is completed.
The cell types mentioned above (e.g., excitatory neurons, inhibitory neurons, and astrocytes) are known to be further classified into subtypes based on factors such as gene expression patterns and their localization within specific cortical layers (Greig et al., 2013; Lanjakornsiripan et al., 2018; De Marco García and Fishell, 2024). While differences in subtypes or gene expression signatures across cortical areas were previously not well understood, recent technological advancements, such as single-cell and spatial transcriptomics, have begun to provide new insights into how diverse cell types acquire region-specific characteristics in humans, macaques, and mice (Nowakowski et al., 2017; Tasic et al., 2018; Bhaduri et al., 2021; Chen et al., 2024; Qian et al., 2024). Although the mechanisms driving area-specific cell type diversification and maturation during postnatal development remain incompletely understood, current evidence suggests that area-specific traits, including those of the PFC, motor, somatosensory, parietal, temporal, and primary visual cortices, first emerge in neural progenitors and become progressively more distinct during differentiation and maturation in the human fetal brain (Nowakowski et al., 2017; Bhaduri et al., 2021). Ongoing single-cell transcriptomic studies that span distinct stages of postnatal development into adulthood (Zhong et al., 2018; Bhattacherjee et al., 2019, 2023; Ortiz et al., 2020; Joglekar et al., 2021) offer valuable opportunities to trace developmental trajectories. While most studies focus on specific developmental time points, integrating findings from these studies may reveal the mechanisms underlying PFC cell type maturation and plasticity, providing deeper insights into its structural and functional complexity. Moreover, epigenetic modifications, which are not fully captured at the transcriptional level, are likely to play a role in PFC maturation processes. Recent studies have begun to uncover the epigenetic landscape of the postnatal PFC, including chromatin accessibility and three-dimensional chromatin interactions at the single-cell level (Lake et al., 2018; Ziffra et al., 2021; Herring et al., 2022; Yao et al., 2022; Heffel et al., 2024; Ma et al., 2024). These findings have shed light on the regulatory landscape that underpins cell type specification and maturation in the PFC. The PFC is thought to undergo a protracted maturation process, extending from birth to adolescence, relative to other cortical regions in both primates and rodents (van Eden et al., 1991; Gogtay et al., 2004; Kolb et al., 2012; Chini and Hanganu-Opatz, 2021; Kolk and Rakic, 2022). However, the mechanisms governing this protracted timeline remain poorly understood. A recent paper using human pluripotent stem cell-derived cortical neurons suggested that epigenetic regulation in neural progenitor cells sets the pace of neuronal maturation after differentiation (Ciceri et al., 2024). Future research is expected to elucidate the molecular and epigenetic factors that regulate the pace of PFC maturation and their implications for both normal development and neurodevelopmental disorders.
Circuit formation and plasticity in prefrontal cortex development
The PFC achieves its complex brain functions through connections with various brain regions, such as the sensory cortex, ventral tegmental area (VTA), basolateral amygdala (BLA), and thalamus, with bi-directional reciprocal connections (Figure 1), being a notable feature that facilitates feedback and integration of information critical for higher-order cognitive functions (Anastasiades and Carter, 2021). While the specific roles of these circuits have been extensively reviewed elsewhere (Le Merre et al., 2021; Yizhar and Levy, 2021; Howland et al., 2022), this review focuses on the maturation of PFC neurons and the formation of circuits during postnatal development.
Neuronal maturation and circuit formation in the PFC begin during the perinatal period as cell production and migration subside (Chini and Hanganu-Opatz, 2021). During postnatal development, which progresses through the neonate, juvenile, and adolescent stages, the PFC matures more slowly than other cortical areas, such as sensory and motor areas. This prolonged maturation is observed in both primates and rodents, at least in certain aspects, including the dynamic reorganization of synaptic properties during adolescence (Paus et al., 2008; Pinto et al., 2013; Pöpplau et al., 2024) and the delayed maturation of parvalbumin-positive (PV) interneurons (Reh et al., 2020; Canetta et al., 2022). This delayed maturation of the PFC likely serves an adaptive function. Sensory and motor areas are required to develop early to process sensory inputs and produce motor outputs. In contrast, the PFC may retain plasticity during later stages to integrate sensory inputs, interpret them, and link them to motor outputs for complex behavioral decision-making. The delayed development of the PFC may also be associated with higher-order cognitive functions, such as social interactions and sexual maturation, which emerge during juvenile and adolescent periods (Chini and Hanganu-Opatz, 2021; Klune et al., 2021).
In sensory systems, it is well-established that critical periods exist during specific developmental stages when neuronal maturation and circuit formation undergo plastic changes (Hensch, 2005; Erzurumlu and Gaspar, 2012; Espinosa and Stryker, 2012; Levelt and Hübener, 2012). These critical periods allow circuits to be appropriately wired to meet environmental demands. Similarly, the PFC is thought to undergo significant plasticity during developmental stages (Larsen and Luna, 2018), which might relate to neurodevelopmental disorders, mood disorders, and addiction (Guirado et al., 2020; Nelson and Gabard-Durnam, 2020). Recent advances in optogenetic and chemogenetic tools, combined with viral vectors and genome-editing technologies, have enabled precise, circuit- or cell-type-specific manipulation of neuronal activity and gene expression at defined developmental stages. These technologies have shed light on the mechanisms underlying the progressive maturation of the PFC.
In the early postnatal stages, from the neonate to the juvenile period, distinct layer-specific changes occur in the mouse mPFC (Kroon et al., 2019). In pyramidal neurons of upper layer 3 (L3) and deep layer 5 (L5), developmental changes in dendritic morphology and intrinsic membrane properties have been observed during the first, second, and fourth postnatal weeks. While these properties develop largely in parallel across layers, excitatory inputs to L3 pyramidal neurons increase more rapidly during the second postnatal week compared to L5 neurons. Conversely, inhibitory inputs develop more rapidly in L5 than in L3. This layer-specific modulation of the excitatory/inhibitory (E/I) balance appears to be a unique feature of the PFC and is not observed in the sensory cortex. The activity of L2/3 neurons during this period drives frequency-specific spiking and enhances network oscillations within the beta–gamma frequency range, but the patterned network was not driven by the activity of L5/6 neurons in the mouse mPFC (Bitzenhofer et al., 2017). The role of neural activity during early postnatal life has also been explored in functional studies. For instance, transient optogenetic activation of excitatory neurons in L2/3 in the mPFC of neonatal mice during postnatal day 7 (P7)–P11 induces premature neuronal maturation, leading to impaired task-related gamma oscillations and deficits in PFC-dependent functions such as working memory and social preference later in life (Bitzenhofer et al., 2021) (Figure 1). These effects are thought to involve inhibitory feedback from PV interneurons. Notably, the same manipulation performed slightly later (P12–P16) results in only partial effects, suggesting the existence of a critical period for plastic changes in early postnatal development. The first one to two postnatal weeks in rodents, corresponding to the breastfeeding period, are dominated by maternal interactions. Open questions remain about the role of external stimuli, from maternal or environmental cues, in activating the PFC, whether spontaneous activity contributes, and how these factors influence long-term outcomes. Connections from the thalamus or dorsal raphe nucleus (DRN) to the PFC are already observed during this stage, with these connections potentially modulating retinoic acid (RA) and serotonin (5-HT), respectively (Larsen et al., 2019; Garcia et al., 2019). As RA plays a significant role in PFC development (Shibata et al., 2021a), external inputs in early life may regulate PFC size or compartmentalization. Following weaning, during the juvenile stage, social behaviors such as juvenile social play become prominent. In rats, this behavior, observed between P21 and P42, has been shown to influence PV-mediated inhibitory inputs and cognitive skills in the PFC later in life (Bijlsma et al., 2022).
By adolescence, sensory areas have largely matured, with significantly reduced plasticity compared to earlier developmental stages. In contrast, PFC maturation and circuit formation accelerate during this period, reflecting its extended developmental timeline and ongoing plasticity (Caballero et al., 2016, 2021). Synaptic density in the PFC undergoes dynamic changes during this period. Recent studies reveal that layer 2/3 pyramidal neuron circuits in the mouse mPFC experience transient disruption due to microglial activity during adolescence, followed by reorganization into adulthood (Pöpplau et al., 2024) (Figure 1). This reorganization is crucial for neural network formation and higher cognitive functions. High-frequency activity patterns in the PFC exhibit a biphasic trajectory during this period. The gamma and spiking activity peaks firstly during pre-juvenile (P16–P23) and early adolescence (P28–P35) but decreases significantly in late adolescence (P36-43) before rising again into adulthood (P53–P60) (Bitzenhofer et al., 2020; Pöpplau et al., 2024). Microglial phagocytic activity is increased during adolescence and promotes period-specific synaptic pruning in the PFC in mice and rats (Mallya et al., 2019; Pöpplau et al., 2024). Furthermore, microglia-driven remodeling during adolescence has been linked to not only dendritic complexity and synaptic structures but also behaviors such as social recognition memory, temporal memory, and fear of extinction later in life at the adult stage in mice (Schalbetter et al., 2022; Pöpplau et al., 2024). Adolescence thus appears to be a critical period when the PFC is particularly sensitive to microglial activity.
During adolescence, connections between the PFC and other brain regions also undergo significant establishment. Connections between the amygdala and subdivisions of the PFC, such as the ACC and IL, exhibited a marked increase during the late postweaning period in rats (Cunningham et al., 2002). Another study has shown that projections from the basolateral amygdala (BLA) and hippocampus to the PL peak at P30, then decline later at P45 into adulthood in mice. These dynamic changes are thought to influence the persistence of fear memories. Interestingly, no such peak is observed in projections to the IL, suggesting subregion-specific plasticity within the mPFC (Pattwell et al., 2016). Bidirectional connections between the mPFC and BLA also show developmental dynamics, with projections from the mPFC to the BLA peaking during adolescence and being pruned into adulthood in mice and rats (Cressman et al., 2010; Arruda-Carvalho et al., 2017). These synchronized peaks in bi-directional connectivity may optimize feedback calculations. Connections from the thalamus to the PFC, observed as early as the first postnatal week, are also highly plastic during adolescence. Chemogenetic inhibition of thalamo-prefrontal (mediodorsal/midline thalamus to medial prefrontal) activity during the postweaning period (P20–P50) impairs mPFC excitability and cognitive functions in adulthood in mice. In contrast, similar manipulations in adulthood have minimal effects, suggesting adolescence is a critical period for thalamo-prefrontal circuit development (Benoit et al., 2022). Interestingly, reduced thalamo-prefrontal connectivity has been reported in young adolescents with psychosis such as schizophrenia even before their diagnosis (Anticevic et al., 2015; Woodward and Heckers, 2016), raising the possibility that adolescence may also represent a period of heightened plasticity for modulating behaviors later in life in humans. Top-down corticocortical projections, such as those from the ACC to the visual cortex, show increased excitability during adolescence (P29–P37) in mice. Evidence suggests that disruptions to this activity during the critical developmental window may affect local and long-range input balance, leading to long-term alterations in PFC excitability and attention-related behaviors (Nabel et al., 2020; Allen and Morishita, 2024).
As described above, the PFC undergoes substantial plasticity changes during specific developmental windows for each circuit. The mechanisms driving these changes remain an active area of research. In sensory cortices, the development of inhibitory neurons is crucial for determining critical period timing (Hensch, 2005). Similarly, changes in PFC plasticity have been linked to GABAergic neurons (Caballero et al., 2016, 2021), particularly PV interneurons (Caballero et al., 2020), whose activity during adolescence (P14–P50) in the mouse mPFC has been shown to be critical for the regulation of gamma oscillations and behaviors later in life (Canetta et al., 2022). Late-adolescent activation of PV interneurons can even rescue deficits in schizophrenia model mice (Mukherjee et al., 2019). Neurotransmitters also play a crucial role in the postnatal development of the PFC, influencing its maturation and the regulation of behaviors. Key examples include serotonin, dopamine, acetylcholine, oxytocin, and endocannabinoids, each contributing to distinct aspects of PFC development (Mukherjee et al., 2019; Soiza-Reilly et al., 2019; Yaseen et al., 2019; Miguelez Fernández et al., 2021; Mastwal et al., 2023; Islam and Blaess, 2024; Molla et al., 2024; Ogelman et al., 2024) (Figure 1). For instance, serotonin signaling during the first two postnatal weeks is essential for regulating PFC maturation, including neuronal development, circuit formation, and the modulation of anxiety/depression-like behaviors in mice (Soiza-Reilly et al., 2019; Ogelman et al., 2024). In contrast, dopaminergic signaling and projections to the PFC increase significantly during adolescence, potentially correlating with pubertal changes and the refinement of cognitive functions (Walker et al., 2017b), partly through the regulation of PV interneuron maturation in mice (Mukherjee et al., 2019; Islam and Blaess, 2024). Future research should aim to clarify the precise timing, cellular targets, and behavioral outcomes of these neurotransmitter signals during critical developmental periods.
Impact of early life stress on prefrontal cortex circuitry and function
During postnatal development, the PFC exhibits significant plasticity, facilitating the establishment of functional neural circuits. This period represents a critical window for experience-dependent brain development but also coincides with increased susceptibility to environmental factors, such as early-life stress (ELS), which may elevate the risk of mental disorders (Takeda et al., 2024; Pechtel and Pizzagalli, 2011; McLaughlin et al., 2010; Green et al., 2010; Nestler and Russo, 2024). Evidence from human studies indicates that childhood neglect or abuse is associated with deficits in social–emotional development and cognitive functions, including IQ, memory processing, and problem-solving abilities (Egeland et al., 1983; Nelson et al., 2007; Spatz Widom et al., 2007; De Bellis et al., 2009; Pollak et al., 2010). Alterations in synaptic dynamics during critical developmental periods have been implicated in the pathophysiology of neurodevelopmental disorders, such as autism spectrum disorder and schizophrenia (Forrest et al., 2018; Faust et al., 2021). Recent studies using rodent models have provided valuable insights into the mechanisms that render the developing brain particularly vulnerable to early-life stress (ELS) and its contributions to the onset of mental disorders later in life. This review focuses primarily on the impact of social stressors, including maternal neglect and social isolation, on the structural and circuit-level development of the PFC.
ELS in neonatal rodents is frequently modeled using maternal separation (MS) or environmental deprivation (e.g., limited bedding and nesting materials), both of which disrupt caregiving environments and lead to behavioral alterations in adulthood, including impaired learning and memory, social deficits, and increased depression-like behaviors (Tractenberg et al., 2016; Walker et al., 2017a). MS has been shown to influence cytoarchitecture by impairing oligodendrocyte differentiation in the mouse mPFC (Teissier et al., 2020) and delaying the onset of neuronal and glial apoptosis in the rat mPFC (Majcher-Maślanka et al., 2019), ultimately altering the composition of each cell type in adulthood. Functionally, it has been reported that MS reduces neuronal activity in the PFC during the stress period. Chemogenetic reduction of mPFC excitability during the first two postnatal weeks (P2–P14) in mice reared under standard facility conditions replicates MS-associated phenotypes, including premature oligodendrocyte differentiation and impairments in emotional behavior and object recognition in adulthood, underscoring a critical period for stress susceptibility (Teissier et al., 2020) (Figure 2). The presence of this critical period is further supported by a study showing that transient chemogenetic activation of PFC neurons during MS in mice ameliorates behavioral deficits in adulthood (Menezes et al., 2024). However, MS has also been reported to increase neuronal excitability, reduce inhibitory neurons, and shift the excitatory/inhibitory (E/I) balance toward excitation in the PFC in mice and rats (Ohta et al., 2020; Oh et al., 2021). Whether stress increases or decreases excitability may depend on specific neuronal subtypes, cortical layers, and circuit connections. Moreover, differences in stress sensitivity have also been reported in circuits between the PFC and specific brain regions (Reincke and Hanganu-Opatz, 2017; Oh et al., 2021) as well as across subregions within the PFC (Luo et al., 2023).
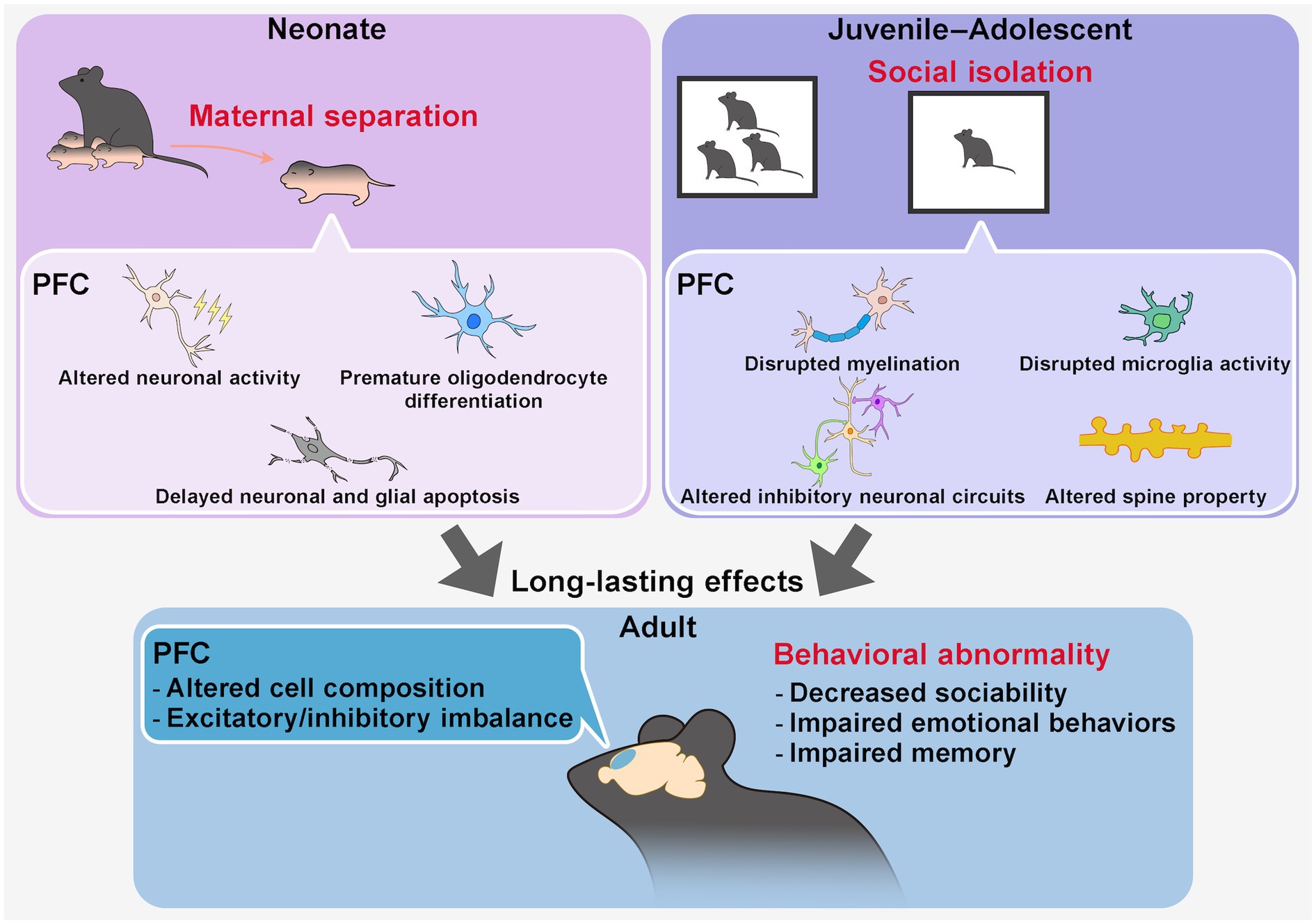
Figure 2. Long-lasting effects of early-life social stress on PFC structure and function. Early-life social stress, such as maternal separation during the neonatal period or social isolation during juvenile–adolescent stages, has been widely used as a model for early-life stress (ELS). These stressors induce persistent alterations in PFC structure, circuit properties, and behaviors into adulthood. During the stress period, maternal separation in neonates affects PFC neuronal activity and cell composition, whereas social isolation in juveniles and adolescents influences neuronal activity, myelination, and microglial function. These changes have been implicated in long-term disruptions in PFC circuit organization, including cell composition and excitatory/inhibitory (E/I) balance, in a circuit- and PFC subregion-specific manner. Ultimately, ELS contributes to behavioral abnormalities in adulthood, including impairments in sociability, emotion regulation, and memory function.
During juvenile and adolescent periods, social isolation is a commonly used stress model to examine long-term effects on PFC function in rodents (Burke et al., 2017; Li et al., 2021; Morishita, 2021). Juvenile social isolation (jSI), particularly during the postweaning period between P21 and P35 in mice, induces greater deficits in social behaviors and cognition in adulthood compared to later isolation periods (P35-P65), indicating a critical period of heightened stress sensitivity during early adolescence (Makinodan et al., 2012). jSI disrupts key elements of neuronal plasticity, including myelination and microglial activity (Makinodan et al., 2012, 2017; Komori et al., 2024), and alters the organization of circuits involving PV or somatostatin (SST)-positive interneurons, leading to long-term changes in E/I balance and behavior (Bicks et al., 2020; Yamamuro et al., 2020; Li et al., 2022) (Figure 2). Social isolation during adolescence in mice also influences actin dynamics in the PFC, which is also observed in rat MS model (Tada et al., 2016), activating cytoskeletal regulatory pathways typically suppressed during this period, thereby affecting dendritic spine properties and long-term brain function (Li et al., 2024a). Furthermore, PFC-associated circuits, including PFC-posterior paraventricular thalamus (pPVT), PFC-nucleus accumbens (NAc), PFC-VTA, and PFC-BLA pathways, have been implicated in mediating the long-term effects of isolation stress in early life on social and cognitive behaviors in adulthood in rodents, with neurotransmitters like dopamine and subregion-dependent differences playing important roles (Baarendse et al., 2013; Yamamuro et al., 2020; Park et al., 2021; Kuniishi et al., 2022; Li et al., 2022; Musardo et al., 2022; Wang et al., 2022).
Epigenetic changes in the prefrontal cortex induced by early life stress: emerging insight
Comprehensive transcriptomic analyses have increasingly been employed in recent years to investigate how ELS influences genome-wide gene expression in the PFC of rodents (Peña et al., 2019; Usui et al., 2021; Wang et al., 2022; Luo et al., 2023). For example, single-cell transcriptomic analysis of PFC tissues from adult mice exposed to MS has revealed long-lasting alterations in gene expression, particularly in genes related to GABAergic and serotonergic pathways (Ohta et al., 2020; Menezes et al., 2024). Environmental factors are thought to influence epigenetic states, which may underlie these persistent transcriptional alterations. Recent studies have shown that stress during early life or adulthood alters histone modifications and DNA methylation states at loci such as Bdnf or serotonin-related genes in the rodent PFC, which are crucial for neuronal plasticity (Roth et al., 2009; Márquez et al., 2013; Xu et al., 2018; Konar et al., 2019; Zhao et al., 2020; Fachim et al., 2021; Jiang et al., 2021; Araki et al., 2024). Moreover, ELS has been shown to induce long-lasting epigenetic changes in cell types critical for neural plasticity, such as PV interneurons and oligodendrocytes, within the rodent PFC (Chen et al., 2020; Noel et al., 2024). Pharmacological inhibition of histone-modifying enzymes, including histone H3K9 methyltransferase, histone H3K4 demethylases, and histone deacetylases (HDACs), has been shown to mitigate behavioral abnormalities caused by ELS in rodent models, suggesting that epigenetic regulation may play a role in both the pathogenesis and potential treatment of stress-related disorders (Wei et al., 2020; Wang et al., 2023; Hernandez Carballo et al., 2024; Li and Yan, 2024). Notably, these inhibitors were administered not only during the stress exposure itself but after the stress period or even later in adulthood, yet still resulted in significant behavioral improvements. This highlights the enduring nature of stress-induced epigenetic changes and their potential reversibility. Furthermore, recent studies in mice suggest that susceptibility to social stress may also be linked to the regulation of gene expression by specific histone-modifying enzymes in the PFC and NAc (Kronman et al., 2021; Li et al., 2024b; Torres-Berrío et al., 2024). Future research should aim to elucidate the specific timing, cell types, and epigenetic mechanisms underlying stress responses to better understand how ELS shapes long-term PFC function and stress vulnerability.
Discussion
This review has highlighted studies, primarily in rodents, illustrating how the PFC undergoes sequential structural and circuit development during postnatal stages. Particular attention has been given to periods of elevated plasticity in the PFC, during which circuit formation and reorganization are especially dynamic. Furthermore, we discussed how these critical windows of plasticity render the PFC particularly vulnerable to environmental stressors, such as ELS, and explored the relationship between ELS and epigenetic regulation in shaping PFC function.
Emerging evidence indicates that the effects of ELS differ substantially across developmental stages, cell types, PFC subregions, and circuits, highlighting the necessity for more detailed investigations. Notably, while the impact of ELS persists into adulthood, epigenetic modifications induced by stress appear to continue evolving even in mature stages. Future research should focus on identifying the genes susceptible to long-term epigenetic changes and elucidating how these alterations influence PFC function over time.
Interestingly, the long-lasting effects of ELS are also implicated in the vulnerability to subsequent stress exposure, consistent with the “two-hit” model (Castillo-Gómez et al., 2017). Differences in responses to second-hit stress have been linked to epigenetic mechanisms, which can either increase or decrease stress resilience depending on the context (Chaby et al., 2020; Reshetnikov et al., 2021). Additionally, even in fully developed adult mice, the PFC retains experience-dependent plasticity (Levy et al., 2019), suggesting that future studies should not only address second-hit vulnerabilities but also investigate the factors that determine plasticity in the adult PFC.
This review did not extensively address how findings from rodent studies translate to humans. While the structural and circuit-level correspondence between the rodent and primate PFC has been discussed extensively (Carlén, 2017; Chini and Hanganu-Opatz, 2021; Le Merre et al., 2021; Preuss and Wise, 2022), further investigation is needed to clarify the similarities in stress responsiveness, particularly given the differences in developmental timelines. Despite the significant differences in the extent of postnatal development, it is worth noting that PFC development appears to progress more slowly than other cortical areas in both humans and rodents, raising intriguing questions about the mechanisms that contribute to the protracted maturation of the PFC.
Another limitation of this review is the lack of discussion on sex differences. Notably, sexual maturation occurs during the juvenile and adolescent periods, and numerous studies have emphasized the sex-specific effects of stress on PFC development and function (Tottenham and Galván, 2016; Heitzeg et al., 2018; Schroeder et al., 2018; Konar et al., 2019; Shaw et al., 2020; Wang et al., 2022). Future research should seek to unravel the intricate interplay between sex differences, developmental stages, and stress responses in shaping PFC development and function.
Achieving a comprehensive understanding of PFC development and its vulnerability to stress will require integrating data across multiple dimensions. By bridging single-cell transcriptional and epigenetic states with circuit-level changes and behavioral outcomes at distinct developmental stages, future research holds the potential to uncover the mechanisms that drive PFC maturation and contribute to the pathophysiology of psychiatric disorders.
Author contributions
XC: Writing – original draft, Writing – review & editing. YK: Writing – review & editing. DK: Writing – original draft, Writing – review & editing.
Funding
The author(s) declare that financial support was received for the research and/or publication of this article. This work was supported by the JSPS KAKENHI grant numbers JP21K06387, JP23H04215, JP23H04667, and JP24K02118 (DK); by the AMED-PRIME under grant number JP23gm6910003 (DK).
Acknowledgments
We apologize to all researchers whose work could not be cited owing to space limitations.
Conflict of interest
The authors declare that the research was conducted in the absence of any commercial or financial relationships that could be construed as a potential conflict of interest.
Generative AI statement
The authors declare that Gen AI was used in the creation of this manuscript. ChatGPT 4o was used to assist with English grammatical corrections for clarity and readability.
Publisher’s note
All claims expressed in this article are solely those of the authors and do not necessarily represent those of their affiliated organizations, or those of the publisher, the editors and the reviewers. Any product that may be evaluated in this article, or claim that may be made by its manufacturer, is not guaranteed or endorsed by the publisher.
References
Allen, S. J., and Morishita, H. (2024). Local and long-range input balance: a framework for investigating frontal cognitive circuit maturation in health and disease. Sci. Adv. 10:eadh3920. doi: 10.1126/sciadv.adh3920
Anastasiades, P. G., and Carter, A. G. (2021). Circuit organization of the rodent medial prefrontal cortex. Trends Neurosci. 44, 550–563. doi: 10.1016/j.tins.2021.03.006
Anticevic, A., Haut, K., Murray, J. D., Repovs, G., Yang, G. J., Diehl, C., et al. (2015). Association of Thalamic Dysconnectivity and Conversion to psychosis in youth and young adults at elevated clinical risk. JAMA Psychiatry 72, 882–891. doi: 10.1001/jamapsychiatry.2015.0566
Araki, R., Kita, A., Ago, Y., and Yabe, T. (2024). Chronic social defeat stress induces anxiety-like behaviors via downregulation of serotonin transporter in the prefrontal serotonergic system in mice. Neurochem. Int. 174:105682. doi: 10.1016/j.neuint.2024.105682
Arruda-Carvalho, M., Wu, W.-C., Cummings, K. A., and Clem, R. L. (2017). Optogenetic examination of prefrontal-amygdala synaptic development. J. Neurosci. 37, 2976–2985. doi: 10.1523/JNEUROSCI.3097-16.2017
Baarendse, P. J. J., Counotte, D. S., O’Donnell, P., and Vanderschuren, L. J. M. J. (2013). Early social experience is critical for the development of cognitive control and dopamine modulation of prefrontal cortex function. Neuropsychopharmacology 38, 1485–1494. doi: 10.1038/npp.2013.47
Bakhshi, K., and Chance, S. A. (2015). The neuropathology of schizophrenia: a selective review of past studies and emerging themes in brain structure and cytoarchitecture. Neuroscience 303, 82–102. doi: 10.1016/j.neuroscience.2015.06.028
Benoit, L. J., Holt, E. S., Posani, L., Fusi, S., Harris, A. Z., Canetta, S., et al. (2022). Adolescent thalamic inhibition leads to long-lasting impairments in prefrontal cortex function. Nat. Neurosci. 25, 714–725. doi: 10.1038/s41593-022-01072-y
Bhaduri, A., Sandoval-Espinosa, C., Otero-Garcia, M., Oh, I., Yin, R., Eze, U. C., et al. (2021). An atlas of cortical arealization identifies dynamic molecular signatures. Nature 598, 200–204. doi: 10.1038/s41586-021-03910-8
Bhattacherjee, A., Djekidel, M. N., Chen, R., Chen, W., Tuesta, L. M., and Zhang, Y. (2019). Cell type-specific transcriptional programs in mouse prefrontal cortex during adolescence and addiction. Nat. Commun. 10:4169. doi: 10.1038/s41467-019-12054-3
Bhattacherjee, A., Zhang, C., Watson, B. R., Djekidel, M. N., Moffitt, J. R., and Zhang, Y. (2023). Spatial transcriptomics reveals the distinct organization of mouse prefrontal cortex and neuronal subtypes regulating chronic pain. Nat. Neurosci. 26, 1880–1893. doi: 10.1038/s41593-023-01455-9
Bicks, L. K., Yamamuro, K., Flanigan, M. E., Kim, J. M., Kato, D., Lucas, E. K., et al. (2020). Prefrontal parvalbumin interneurons require juvenile social experience to establish adult social behavior. Nat. Commun. 11:1003. doi: 10.1038/s41467-020-14740-z
Bijlsma, A., Omrani, A., Spoelder, M., Verharen, J. P. H., Bauer, L., Cornelis, C., et al. (2022). Social play behavior is critical for the development of prefrontal inhibitory synapses and cognitive flexibility in rats. J. Neurosci. 42, 8716–8728. doi: 10.1523/JNEUROSCI.0524-22.2022
Bitzenhofer, S. H., Ahlbeck, J., Wolff, A., Wiegert, J. S., Gee, C. E., Oertner, T. G., et al. (2017). Layer-specific optogenetic activation of pyramidal neurons causes beta–gamma entrainment of neonatal networks. Nat. Commun. 8:14563. doi: 10.1038/ncomms14563
Bitzenhofer, S. H., Pöpplau, J. A., Chini, M., Marquardt, A., and Hanganu-Opatz, I. L. (2021). A transient developmental increase in prefrontal activity alters network maturation and causes cognitive dysfunction in adult mice. Neuron 109, 1350–1364.e6. doi: 10.1016/j.neuron.2021.02.011
Bitzenhofer, S. H., Pöpplau, J. A., and Hanganu-Opatz, I. (2020). Gamma activity accelerates during prefrontal development. eLife 9:e56795. doi: 10.7554/eLife.56795
Burke, A. R., McCormick, C. M., Pellis, S. M., and Lukkes, J. L. (2017). Impact of adolescent social experiences on behavior and neural circuits implicated in mental illnesses. Neurosci. Biobehav. Rev. 76, 280–300. doi: 10.1016/j.neubiorev.2017.01.018
Caballero, A., Flores-Barrera, E., Thomases, D. R., and Tseng, K. Y. (2020). Downregulation of parvalbumin expression in the prefrontal cortex during adolescence causes enduring prefrontal disinhibition in adulthood. Neuropsychopharmacology 45, 1527–1535. doi: 10.1038/s41386-020-0709-9
Caballero, A., Granberg, R., and Tseng, K. Y. (2016). Mechanisms contributing to prefrontal cortex maturation during adolescence. Neurosci. Biobehav. Rev. 70, 4–12. doi: 10.1016/j.neubiorev.2016.05.013
Caballero, A., Orozco, A., and Tseng, K. Y. (2021). Developmental regulation of excitatory-inhibitory synaptic balance in the prefrontal cortex during adolescence. Semin. Cell Dev. Biol. 118, 60–63. doi: 10.1016/j.semcdb.2021.02.008
Cadwell, C. R., Bhaduri, A., Mostajo-Radji, M. A., Keefe, M. G., and Nowakowski, T. J. (2019). Development and Arealization of the cerebral cortex. Neuron 103, 980–1004. doi: 10.1016/j.neuron.2019.07.009
Canetta, S. E., Holt, E. S., Benoit, L. J., Teboul, E., Sahyoun, G. M., Ogden, R. T., et al. (2022). Mature parvalbumin interneuron function in prefrontal cortex requires activity during a postnatal sensitive period. eLife 11:e80324. doi: 10.7554/eLife.80324
Carlén, M. (2017). What constitutes the prefrontal cortex? Science 358, 478–482. doi: 10.1126/science.aan8868
Castillo-Gómez, E., Pérez-Rando, M., Bellés, M., Gilabert-Juan, J., Llorens, J. V., Carceller, H., et al. (2017). Early social isolation stress and perinatal NMDA receptor antagonist treatment induce changes in the structure and neurochemistry of inhibitory neurons of the adult amygdala and prefrontal cortex. eNeuro 4:2017. doi: 10.1523/ENEURO.0034-17.2017
Chaby, L. E., Sadik, N., Burson, N. A., Lloyd, S., O’Donnel, K., Winters, J., et al. (2020). Repeated stress exposure in mid-adolescence attenuates behavioral, noradrenergic, and epigenetic effects of trauma-like stress in early adult male rats. Sci. Rep. 10:17935. doi: 10.1038/s41598-020-74481-3
Chen, X., Fischer, S., Rue, M. C. P., Zhang, A., Mukherjee, D., Kanold, P. O., et al. (2024). Whole-cortex in situ sequencing reveals input-dependent area identity. Nature, 1–10. doi: 10.1038/s41586-024-07221-6
Chen, X., Liu, H., Gan, J., Wang, X., Yu, G., Li, T., et al. (2020). Quetiapine modulates histone methylation status in oligodendroglia and rescues adolescent behavioral alterations of socially isolated mice. Front. Psych. 10:984. doi: 10.3389/fpsyt.2019.00984
Chini, M., and Hanganu-Opatz, I. L. (2021). Prefrontal cortex development in health and disease: lessons from rodents and humans. Trends Neurosci. 44, 227–240. doi: 10.1016/j.tins.2020.10.017
Cholfin, J. A., and Rubenstein, J. L. R. (2007). Patterning of frontal cortex subdivisions by Fgf17. Proc. Natl. Acad. Sci. 104, 7652–7657. doi: 10.1073/pnas.0702225104
Ciceri, G., Baggiolini, A., Cho, H. S., Kshirsagar, M., Benito-Kwiecinski, S., Walsh, R. M., et al. (2024). An epigenetic barrier sets the timing of human neuronal maturation. Nature 626, 881–890. doi: 10.1038/s41586-023-06984-8
Courchesne, E., Pramparo, T., Gazestani, V. H., Lombardo, M. V., Pierce, K., and Lewis, N. E. (2019). The ASD living biology: from cell proliferation to clinical phenotype. Mol. Psychiatry 24, 88–107. doi: 10.1038/s41380-018-0056-y
Cressman, V. L., Balaban, J., Steinfeld, S., Shemyakin, A., Graham, P., Parisot, N., et al. (2010). Prefrontal cortical inputs to the basal amygdala undergo pruning during late adolescence in the rat. J. Comp. Neurol. 518, 2693–2709. doi: 10.1002/cne.22359
Cunningham, M. G., Bhattacharyya, S., and Benes, F. M. (2002). Amygdalo-cortical sprouting continues into early adulthood: implications for the development of normal and abnormal function during adolescence. J. Comp. Neurol. 453, 116–130. doi: 10.1002/cne.10376
De Bellis, M. D., Hooper, S. R., Spratt, E. G., and Woolley, D. P. (2009). Neuropsychological findings in childhood neglect and their relationships to pediatric PTSD. J. Int. Neuropsychol. Soc. 15, 868–878. doi: 10.1017/S1355617709990464
De La Fuente, A., Xia, S., Branch, C., and Li, X. (2013). A review of attention-deficit/hyperactivity disorder from the perspective of brain networks. Front. Hum. Neurosci. 7:192. doi: 10.3389/fnhum.2013.00192
De Marco García, N. V., and Fishell, G. (2024). Interneuron diversity: How form becomes function. Cold Spring Harb Perspect Biol :a041513. doi: 10.1101/cshperspect.a041513
Donahue, C. J., Glasser, M. F., Preuss, T. M., Rilling, J. K., and Van Essen, D. C. (2018). Quantitative assessment of prefrontal cortex in humans relative to nonhuman primates. Proc. Natl. Acad. Sci. 115, E5183–E5192. doi: 10.1073/pnas.1721653115
Egeland, B., Sroufe, L. A., and Erickson, M. (1983). The developmental consequence of different patterns of maltreatment. Child Abuse Negl. 7, 459–469. doi: 10.1016/0145-2134(83)90053-4
Erzurumlu, R. S., and Gaspar, P. (2012). Development and critical period plasticity of the barrel cortex. Eur. J. Neurosci. 35, 1540–1553. doi: 10.1111/j.1460-9568.2012.08075.x
Espinosa, J. S., and Stryker, M. P. (2012). Development and plasticity of the primary visual cortex. Neuron 75, 230–249. doi: 10.1016/j.neuron.2012.06.009
Euston, D. R., Gruber, A. J., and McNaughton, B. L. (2012). The role of medial prefrontal cortex in memory and decision making. Neuron 76, 1057–1070. doi: 10.1016/j.neuron.2012.12.002
Fachim, H. A., Corsi-Zuelli, F., Loureiro, C. M., Iamjan, S., Shuhama, R., Joca, S., et al. (2021). Early-life stress effects on BDNF DNA methylation in first-episode psychosis and in rats reared in isolation. Prog. Neuro-Psychopharmacol. Biol. Psychiatry 108:110188. doi: 10.1016/j.pnpbp.2020.110188
Faust, T. E., Gunner, G., and Schafer, D. P. (2021). Mechanisms governing activity-dependent synaptic pruning in the developing mammalian CNS. Nat. Rev. Neurosci. 22, 657–673. doi: 10.1038/s41583-021-00507-y
Forrest, M. P., Parnell, E., and Penzes, P. (2018). Dendritic structural plasticity and neuropsychiatric disease. Nat. Rev. Neurosci. 19, 215–234. doi: 10.1038/nrn.2018.16
Friedman, N. P., and Robbins, T. W. (2022). The role of prefrontal cortex in cognitive control and executive function. Neuropsychopharmacology 47, 72–89. doi: 10.1038/s41386-021-01132-0
Fukuchi-Shimogori, T., and Grove, E. A. (2001). Neocortex patterning by the secreted signaling molecule FGF8. Science 294, 1071–1074. doi: 10.1126/science.1064252
Gangopadhyay, P., Chawla, M., Dal Monte, O., and Chang, S. W. C. (2021). Prefrontal–amygdala circuits in social decision-making. Nat. Neurosci. 24, 5–18. doi: 10.1038/s41593-020-00738-9
Garcia, L. P., Witteveen, J. S., Middelman, A., van Hulten, J. A., Martens, G. J. M., Homberg, J. R., et al. (2019). Perturbed developmental serotonin signaling affects prefrontal Catecholaminergic innervation and cortical integrity. Mol. Neurobiol. 56, 1405–1420. doi: 10.1007/s12035-018-1105-x
Glahn, D. C., Laird, A. R., Ellison-Wright, I., Thelen, S. M., Robinson, J. L., Lancaster, J. L., et al. (2008). Meta-analysis of gray matter anomalies in schizophrenia: application of anatomic likelihood estimation and network analysis. Biol. Psychiatry 64, 774–781. doi: 10.1016/j.biopsych.2008.03.031
Gogtay, N., Giedd, J. N., Lusk, L., Hayashi, K. M., Greenstein, D., Vaituzis, A. C., et al. (2004). Dynamic mapping of human cortical development during childhood through early adulthood. Proc. Natl. Acad. Sci. 101, 8174–8179. doi: 10.1073/pnas.0402680101
Green, J. G., McLaughlin, K. A., Berglund, P. A., Gruber, M. J., Sampson, N. A., Zaslavsky, A. M., et al. (2010). Childhood adversities and adult psychiatric disorders in the National Comorbidity Survey Replication I. Arch. Gen. Psychiatry 67, 113–123. doi: 10.1001/archgenpsychiatry.2009.186
Greig, L. C., Woodworth, M. B., Galazo, M. J., Padmanabhan, H., and Macklis, J. D. (2013). Molecular logic of neocortical projection neuron specification, development and diversity. Nat. Rev. Neurosci. 14, 755–769. doi: 10.1038/nrn3586
Guirado, R., Perez-Rando, M., Ferragud, A., Gutierrez-Castellanos, N., Umemori, J., Carceller, H., et al. (2020). A critical period for prefrontal network configurations underlying psychiatric disorders and addiction. Front. Behav. Neurosci. 14:51. doi: 10.3389/fnbeh.2020.00051
Hashem, S., Nisar, S., Bhat, A. A., Yadav, S. K., Azeem, M. W., Bagga, P., et al. (2020). Genetics of structural and functional brain changes in autism spectrum disorder. Transl. Psychiatry 10:229. doi: 10.1038/s41398-020-00921-3
Heffel, M. G., Zhou, J., Zhang, Y., Lee, D.-S., Hou, K., Pastor-Alonso, O., et al. (2024). Temporally distinct 3D multi-omic dynamics in the developing human brain. Nature 635, 481–489. doi: 10.1038/s41586-024-08030-7
Heitzeg, M. M., Hardee, J. E., and Beltz, A. M. (2018). Sex differences in the developmental neuroscience of adolescent substance use risk. Curr. Opin. Behav. Sci. 23, 21–26. doi: 10.1016/j.cobeha.2018.01.020
Hensch, T. K. (2005). Critical period plasticity in local cortical circuits. Nat. Rev. Neurosci. 6, 877–888. doi: 10.1038/nrn1787
Hernandez Carballo, L. G., Li, P., Senek, R., and Yan, Z. (2024). Systemic histone deacetylase inhibition ameliorates the aberrant responses to acute stress in socially isolated male mice. J. Physiol. 602, 2047–2060. doi: 10.1113/JP285875
Herring, C. A., Simmons, R. K., Freytag, S., Poppe, D., Moffet, J. J. D., Pflueger, J., et al. (2022). Human prefrontal cortex gene regulatory dynamics from gestation to adulthood at single-cell resolution. Cell 185, 4428–4447.e28. doi: 10.1016/j.cell.2022.09.039
Howland, J. G., Ito, R., Lapish, C. C., and Villaruel, F. R. (2022). The rodent medial prefrontal cortex and associated circuits in orchestrating adaptive behavior under variable demands. Neurosci. Biobehav. Rev. 135:104569. doi: 10.1016/j.neubiorev.2022.104569
Islam, K. U. S., and Blaess, S. (2024). The impact of the mesoprefrontal dopaminergic system on the maturation of interneurons in the murine prefrontal cortex. Front. Neurosci. 18:3402. doi: 10.3389/fnins.2024.1403402
Jiang, Z., Zhu, Z., Zhao, M., Wang, W., Li, H., Liu, D., et al. (2021). H3K9me2 regulation of BDNF expression in the hippocampus and medial prefrontal cortex is involved in the depressive-like phenotype induced by maternal separation in male rats. Psychopharmacology 238, 2801–2813. doi: 10.1007/s00213-021-05896-7
Joglekar, A., Prjibelski, A., Mahfouz, A., Collier, P., Lin, S., Schlusche, A. K., et al. (2021). A spatially resolved brain region- and cell type-specific isoform atlas of the postnatal mouse brain. Nat. Commun. 12:463. doi: 10.1038/s41467-020-20343-5
Klune, C. B., Jin, B., and DeNardo, L. A. (2021). Linking mPFC circuit maturation to the developmental regulation of emotional memory and cognitive flexibility. eLife 10:e64567. doi: 10.7554/eLife.64567
Kolb, B., Mychasiuk, R., Muhammad, A., Li, Y., Frost, D. O., and Gibb, R. (2012). Experience and the developing prefrontal cortex. Proc. Natl. Acad. Sci. 109, 17186–17193. doi: 10.1073/pnas.1121251109
Kolk, S. M., and Rakic, P. (2022). Development of prefrontal cortex. Neuropsychopharmacology 47, 41–57. doi: 10.1038/s41386-021-01137-9
Komori, T., Okamura, K., Ikehara, M., Yamamuro, K., Endo, N., Okumura, K., et al. (2024). Brain-derived neurotrophic factor from microglia regulates neuronal development in the medial prefrontal cortex and its associated social behavior. Mol. Psychiatry 29, 1338–1349. doi: 10.1038/s41380-024-02413-y
Konar, A., Rastogi, M., and Bhambri, A. (2019). Brain region specific methylation and Sirt1 binding changes in MAOA promoter is associated with sexual dimorphism in early life stress induced aggressive behavior. Neurochem. Int. 129:104510. doi: 10.1016/j.neuint.2019.104510
Kronman, H., Torres-Berrío, A., Sidoli, S., Issler, O., Godino, A., Ramakrishnan, A., et al. (2021). Long-term behavioral and cell-type-specific molecular effects of early life stress are mediated by H3K79me2 dynamics in medium spiny neurons. Nat. Neurosci. 24, 667–676. doi: 10.1038/s41593-021-00814-8
Kroon, T., van Hugte, E., van Linge, L., Mansvelder, H. D., and Meredith, R. M. (2019). Early postnatal development of pyramidal neurons across layers of the mouse medial prefrontal cortex. Sci. Rep. 9:5037. doi: 10.1038/s41598-019-41661-9
Krubitzer, L. A., and Seelke, A. M. H. (2012). Cortical evolution in mammals: the bane and beauty of phenotypic variability. Proc. Natl. Acad. Sci. 109, 10647–10654. doi: 10.1073/pnas.1201891109
Kuniishi, H., Nakatake, Y., Sekiguchi, M., and Yamada, M. (2022). Adolescent social isolation induces distinct changes in the medial and lateral OFC-BLA synapse and social and emotional alterations in adult mice. Neuropsychopharmacology 47, 1597–1607. doi: 10.1038/s41386-022-01358-6
Lake, B. B., Chen, S., Sos, B. C., Fan, J., Kaeser, G. E., Yung, Y. C., et al. (2018). Integrative single-cell analysis of transcriptional and epigenetic states in the human adult brain. Nat. Biotechnol. 36, 70–80. doi: 10.1038/nbt.4038
Lanjakornsiripan, D., Pior, B.-J., Kawaguchi, D., Furutachi, S., Tahara, T., Katsuyama, Y., et al. (2018). Layer-specific morphological and molecular differences in neocortical astrocytes and their dependence on neuronal layers. Nat. Commun. 9:1623. doi: 10.1038/s41467-018-03940-3
Larsen, B., and Luna, B. (2018). Adolescence as a neurobiological critical period for the development of higher-order cognition. Neurosci. Biobehav. Rev. 94, 179–195. doi: 10.1016/j.neubiorev.2018.09.005
Larsen, R., Proue, A., Scott, E. P., Christiansen, M., and Nakagawa, Y. (2019). The thalamus regulates retinoic acid signaling and development of Parvalbumin interneurons in postnatal mouse prefrontal cortex. eNeuro 6:ENEURO.0018-19.2019. doi: 10.1523/ENEURO.0018-19.2019
Le Merre, P., Ährlund-Richter, S., and Carlén, M. (2021). The mouse prefrontal cortex: Unity in diversity. Neuron 109, 1925–1944. doi: 10.1016/j.neuron.2021.03.035
Levelt, C. N., and Hübener, M. (2012). Critical-period plasticity in the visual cortex. Annu. Rev. Neurosci. 35, 309–330. doi: 10.1146/annurev-neuro-061010-113813
Levy, D. R., Tamir, T., Kaufman, M., Parabucki, A., Weissbrod, A., Schneidman, E., et al. (2019). Dynamics of social representation in the mouse prefrontal cortex. Nat. Neurosci. 22, 2013–2022. doi: 10.1038/s41593-019-0531-z
Li, D. C., Hinton, E. A., and Gourley, S. L. (2021). Persistent behavioral and neurobiological consequences of social isolation during adolescence. Semin. Cell Dev. Biol. 118, 73–82. doi: 10.1016/j.semcdb.2021.05.017
Li, D. C., Hinton, E. A., Guo, J., Knight, K. A., Sequeira, M. K., Wynne, M. E., et al. (2024a). Social experience in adolescence shapes prefrontal cortex structure and function in adulthood. Mol. Psychiatry 29, 2787–2798. doi: 10.1038/s41380-024-02540-6
Li, H., Kawatake-Kuno, A., Inaba, H., Miyake, Y., Itoh, Y., Ueki, T., et al. (2024b). Discrete prefrontal neuronal circuits determine repeated stress-induced behavioral phenotypes in male mice. Neuron 112, 786–804.e8. doi: 10.1016/j.neuron.2023.12.004
Li, X., Sun, H., Zhu, Y., Wang, F., Wang, X., Han, L., et al. (2022). Dysregulation of prefrontal parvalbumin interneurons leads to adult aggression induced by social isolation stress during adolescence. Front. Mol. Neurosci. 15:1010152. doi: 10.3389/fnmol.2022.1010152
Li, P., and Yan, Z. (2024). An epigenetic mechanism of social isolation stress in adolescent female mice. Neurobiol Stress 29:100601. doi: 10.1016/j.ynstr.2023.100601
Luo, F., Deng, J., Sun, X., Zhen, J., and Luo, X. (2023). Anterior cingulate cortex orexin signaling mediates early-life stress-induced social impairment in females. Proc. Natl. Acad. Sci. 120:e2220353120. doi: 10.1073/pnas.2220353120
Ma, Y., Guo, S., Chen, Y., Peng, Y., Su, X., Jiang, H., et al. (2024). Single-nucleus chromatin landscape dataset of mouse brain development and aging. Sci Data 11:616. doi: 10.1038/s41597-024-03382-1
Majcher-Maślanka, I., Solarz, A., and Chocyk, A. (2019). Maternal separation disturbs postnatal development of the medial prefrontal cortex and affects the number of neurons and glial cells in adolescent rats. Neuroscience 423, 131–147. doi: 10.1016/j.neuroscience.2019.10.033
Makinodan, M., Ikawa, D., Yamamuro, K., Yamashita, Y., Toritsuka, M., Kimoto, S., et al. (2017). Effects of the mode of re-socialization after juvenile social isolation on medial prefrontal cortex myelination and function. Sci. Rep. 7:5481. doi: 10.1038/s41598-017-05632-2
Makinodan, M., Rosen, K. M., Ito, S., and Corfas, G. (2012). A critical period for social experience-dependent oligodendrocyte maturation and myelination. Science 337, 1357–1360. doi: 10.1126/science.1220845
Mallya, A. P., Wang, H.-D., Lee, H. N. R., and Deutch, A. Y. (2019). Microglial pruning of synapses in the prefrontal cortex during adolescence. Cereb. Cortex 29, 1634–1643. doi: 10.1093/cercor/bhy061
Márquez, C., Poirier, G. L., Cordero, M. I., Larsen, M. H., Groner, A., Marquis, J., et al. (2013). Peripuberty stress leads to abnormal aggression, altered amygdala and orbitofrontal reactivity and increased prefrontal MAOA gene expression. Transl. Psychiatry 3:e216. doi: 10.1038/tp.2012.144
Mastwal, S., Li, X., Stowell, R., Manion, M., Zhang, W., Kim, N.-S., et al. (2023). Adolescent neurostimulation of dopamine circuit reverses genetic deficits in frontal cortex function. eLife 12:RP87414. doi: 10.7554/eLife.87414.3
McLaughlin, K. A., Green, J. G., Gruber, M. J., Sampson, N. A., Zaslavsky, A. M., and Kessler, R. C. (2010). Childhood adversities and adult psychiatric disorders in the National Comorbidity Survey Replication II. Arch. Gen. Psychiatry 67, 124–132. doi: 10.1001/archgenpsychiatry.2009.187
Menezes, E. C., Geiger, H., Abreu, F. F., Rachmany, L., Wilson, D. A., Alldred, M. J., et al. (2024). Early-life prefrontal cortex inhibition and early-life stress lead to long-lasting behavioral, transcriptional, and physiological impairments. Mol. Psychiatry 29, 2359–2371. doi: 10.1038/s41380-024-02499-4
Miguelez Fernández, A. M. M., Molla, H. M., Thomases, D. R., and Tseng, K. Y. (2021). Prefrontal α7nAChR signaling differentially modulates afferent drive and trace fear conditioning behavior in adolescent and adult rats. J. Neurosci. 41, 1908–1916. doi: 10.1523/JNEUROSCI.1941-20.2020
Miller, E. K., and Cohen, J. D. (2001). An integrative theory of prefrontal cortex function. Annu. Rev. Neurosci. 24, 167–202. doi: 10.1146/annurev.neuro.24.1.167
Miyata, T., Kawaguchi, D., Kawaguchi, A., and Gotoh, Y. (2010). Mechanisms that regulate the number of neurons during mouse neocortical development. Curr. Opin. Neurobiol. 20, 22–28. doi: 10.1016/j.conb.2010.01.001
Molla, H. M., Miguelez Fernández, A. M. M., and Tseng, K. Y. (2024). Late-adolescent onset of prefrontal endocannabinoid control of hippocampal and amygdalar inputs and its impact on trace-fear conditioning behavior. Neuropsychopharmacology 49, 1417–1424. doi: 10.1038/s41386-024-01844-z
Morishita, H. (2021). A prefrontal social circuit vulnerable to juvenile social isolation. Neuropsychopharmacology 46, 229–230. doi: 10.1038/s41386-020-00821-6
Mukherjee, A., Carvalho, F., Eliez, S., and Caroni, P. (2019). Long-lasting Rescue of Network and Cognitive Dysfunction in a genetic schizophrenia model. Cell 178, 1387–1402.e14. doi: 10.1016/j.cell.2019.07.023
Murugan, M., Jang, H. J., Park, M., Miller, E. M., Cox, J., Taliaferro, J. P., et al. (2017). Combined social and spatial coding in a descending projection from the prefrontal cortex. Cell 171, 1663–1677.e16. doi: 10.1016/j.cell.2017.11.002
Musardo, S., Contestabile, A., Knoop, M., Baud, O., and Bellone, C. (2022). Oxytocin neurons mediate the effect of social isolation via the VTA circuits. eLife 11:e73421. doi: 10.7554/eLife.73421
Nabel, E. M., Garkun, Y., Koike, H., Sadahiro, M., Liang, A., Norman, K. J., et al. (2020). Adolescent frontal top-down neurons receive heightened local drive to establish adult attentional behavior in mice. Nat. Commun. 11:3983. doi: 10.1038/s41467-020-17787-0
Najmabadi, H., Hu, H., Garshasbi, M., Zemojtel, T., Abedini, S. S., Chen, W., et al. (2011). Deep sequencing reveals 50 novel genes for recessive cognitive disorders. Nature 478, 57–63. doi: 10.1038/nature10423
Nelson, C. A., and Gabard-Durnam, L. J. (2020). Early adversity and critical periods: neurodevelopmental consequences of violating the expectable environment. Trends Neurosci. 43, 133–143. doi: 10.1016/j.tins.2020.01.002
Nelson, C. A., Zeanah, C. H., Fox, N. A., Marshall, P. J., Smyke, A. T., and Guthrie, D. (2007). Cognitive recovery in socially deprived young children: the Bucharest early intervention project. Science 318, 1937–1940. doi: 10.1126/science.1143921
Nestler, E. J., and Russo, S. J. (2024). Neurobiological basis of stress resilience. Neuron 112, 1911–1929. doi: 10.1016/j.neuron.2024.05.001
Noel, E. S., Chen, A., Peña, Y. A., and Honeycutt, J. A. (2024). Early life adversity drives sex-dependent changes in 5-mC DNA methylation of parvalbumin cells in the prefrontal cortex in rats. bioRxiv. doi: 10.1101/2024.01.31.578313
Nowakowski, T. J., Bhaduri, A., Pollen, A. A., Alvarado, B., Mostajo-Radji, M. A., Di Lullo, E., et al. (2017). Spatiotemporal gene expression trajectories reveal developmental hierarchies of the human cortex. Science 358, 1318–1323. doi: 10.1126/science.aap8809
O’Leary, D. D. M., Chou, S.-J., and Sahara, S. (2007). Area patterning of the mammalian cortex. Neuron 56, 252–269. doi: 10.1016/j.neuron.2007.10.010
Ogelman, R., Gomez Wulschner, L. E., Hoelscher, V. M., Hwang, I.-W., Chang, V. N., and Oh, W. C. (2024). Serotonin modulates excitatory synapse maturation in the developing prefrontal cortex. Nat. Commun. 15:1368. doi: 10.1038/s41467-024-45734-w
Oh, W. C., Rodríguez, G., Asede, D., Jung, K., Hwang, I.-W., Ogelman, R., et al. (2021). Dysregulation of the mesoprefrontal dopamine circuit mediates an early-life stress-induced synaptic imbalance in the prefrontal cortex. Cell Rep. 35:109074. doi: 10.1016/j.celrep.2021.109074
Ohta, K., Suzuki, S., Warita, K., Sumitani, K., Tenkumo, C., Ozawa, T., et al. (2020). The effects of early life stress on the excitatory/inhibitory balance of the medial prefrontal cortex. Behav. Brain Res. 379:112306. doi: 10.1016/j.bbr.2019.112306
Ongür, D., and Price, J. L. (2000). The organization of networks within the orbital and medial prefrontal cortex of rats, monkeys and humans. Cereb. Cortex 10, 206–219. doi: 10.1093/cercor/10.3.206
Ortiz, C., Navarro, J. F., Jurek, A., Märtin, A., Lundeberg, J., and Meletis, K. (2020). Molecular atlas of the adult mouse brain. Sci. Adv. 6:eabb3446. doi: 10.1126/sciadv.abb3446
Park, G., Ryu, C., Kim, S., Jeong, S. J., Koo, J. W., Lee, Y.-S., et al. (2021). Social isolation impairs the prefrontal-nucleus accumbens circuit subserving social recognition in mice. Cell Rep. 35:109104. doi: 10.1016/j.celrep.2021.109104
Pattwell, S. S., Liston, C., Jing, D., Ninan, I., Yang, R. R., Witztum, J., et al. (2016). Dynamic changes in neural circuitry during adolescence are associated with persistent attenuation of fear memories. Nat. Commun. 7:11475. doi: 10.1038/ncomms11475
Paus, T., Keshavan, M., and Giedd, J. N. (2008). Why do many psychiatric disorders emerge during adolescence? Nat. Rev. Neurosci. 9, 947–957. doi: 10.1038/nrn2513
Pechtel, P., and Pizzagalli, D. A. (2011). Effects of early life stress on cognitive and affective function: an integrated review of human literature. Psychopharmacology 214, 55–70. doi: 10.1007/s00213-010-2009-2
Peña, C. J., Smith, M., Ramakrishnan, A., Cates, H. M., Bagot, R. C., Kronman, H. G., et al. (2019). Early life stress alters transcriptomic patterning across reward circuitry in male and female mice. Nat. Commun. 10:5098. doi: 10.1038/s41467-019-13085-6
Pinto, J. G. A., Jones, D. G., and Murphy, K. M. (2013). Comparing development of synaptic proteins in rat visual, somatosensory, and frontal cortex. Front Neural Circuits 7:97. doi: 10.3389/fncir.2013.00097
Pollak, S. D., Nelson, C. A., Schlaak, M. F., Roeber, B. J., Wewerka, S. S., Wiik, K. L., et al. (2010). Neurodevelopmental effects of early deprivation in Postinstitutionalized children. Child Dev. 81, 224–236. doi: 10.1111/j.1467-8624.2009.01391.x
Pöpplau, J. A., Schwarze, T., Dorofeikova, M., Pochinok, I., Günther, A., Marquardt, A., et al. (2024). Reorganization of adolescent prefrontal cortex circuitry is required for mouse cognitive maturation. Neuron 112, 421–440.e7. doi: 10.1016/j.neuron.2023.10.024
Preuss, T. M., and Wise, S. P. (2022). Evolution of prefrontal cortex. Neuropsychopharmacology 47, 3–19. doi: 10.1038/s41386-021-01076-5
Qian, X., Coleman, K., Jiang, S., Kriz, A. J., Marciano, J. H., Luo, C., et al. (2024). Spatial single-cell analysis decodes cortical layer and area specification. bioRxiv. doi: 10.1101/2024.06.05.597673
Reh, R. K., Dias, B. G., Nelson, C. A., Kaufer, D., Werker, J. F., Kolb, B., et al. (2020). Critical period regulation across multiple timescales. Proc. Natl. Acad. Sci. 117, 23242–23251. doi: 10.1073/pnas.1820836117
Reincke, S. A. J., and Hanganu-Opatz, I. L. (2017). Early-life stress impairs recognition memory and perturbs the functional maturation of prefrontal-hippocampal-perirhinal networks. Sci. Rep. 7:42042. doi: 10.1038/srep42042
Reshetnikov, V. V., Kisaretova, P. E., Ershov, N. I., Merkulova, T. I., and Bondar, N. P. (2021). Social defeat stress in adult mice causes alterations in gene expression, alternative splicing, and the epigenetic landscape of H3K4me3 in the prefrontal cortex: An impact of early-life stress. Prog. Neuro-Psychopharmacol. Biol. Psychiatry 106:110068. doi: 10.1016/j.pnpbp.2020.110068
Roth, T. L., Lubin, F. D., Funk, A. J., and Sweatt, J. D. (2009). Lasting epigenetic influence of early-life adversity on the BDNF gene. Biol. Psychiatry 65, 760–769. doi: 10.1016/j.biopsych.2008.11.028
Satterstrom, F. K., Kosmicki, J. A., Wang, J., Breen, M. S., De Rubeis, S., An, J.-Y., et al. (2020). Large-scale exome sequencing study implicates both developmental and functional changes in the neurobiology of autism. Cell 180, 568–584.e23. doi: 10.1016/j.cell.2019.12.036
Schalbetter, S. M., von Arx, A. S., Cruz-Ochoa, N., Dawson, K., Ivanov, A., Mueller, F. S., et al. (2022). Adolescence is a sensitive period for prefrontal microglia to act on cognitive development. Sci. Adv. 8:eabi6672. doi: 10.1126/sciadv.abi6672
Schroeder, A., Notaras, M., Du, X., and Hill, R. A. (2018). On the developmental timing of stress: delineating sex-specific effects of stress across development on adult behavior. Brain Sci. 8:121. doi: 10.3390/brainsci8070121
Schubert, D., Martens, G. J. M., and Kolk, S. M. (2015). Molecular underpinnings of prefrontal cortex development in rodents provide insights into the etiology of neurodevelopmental disorders. Mol. Psychiatry 20, 795–809. doi: 10.1038/mp.2014.147
Shaw, G. A., Dupree, J. L., and Neigh, G. N. (2020). Adolescent maturation of the prefrontal cortex: role of stress and sex in shaping adult risk for compromise. Genes Brain Behav. 19:e12626. doi: 10.1111/gbb.12626
Shibata, M., Pattabiraman, K., Lorente-Galdos, B., Andrijevic, D., Kim, S.-K., Kaur, N., et al. (2021a). Regulation of prefrontal patterning and connectivity by retinoic acid. Nature 598, 483–488. doi: 10.1038/s41586-021-03953-x
Shibata, M., Pattabiraman, K., Muchnik, S. K., Kaur, N., Morozov, Y. M., Cheng, X., et al. (2021b). Hominini-specific regulation of CBLN2 increases prefrontal spinogenesis. Nature 598, 489–494. doi: 10.1038/s41586-021-03952-y
Siddiqui, S., Chatterjee, U., Kumar, D., Siddiqui, A., and Goyal, N. (2008). Neuropsychology of prefrontal cortex. Indian J. Psychiatry 50, 202–208. doi: 10.4103/0019-5545.43634
Singh, T., Poterba, T., Curtis, D., Akil, H., Al Eissa, M., Barchas, J. D., et al. (2022). Rare coding variants in ten genes confer substantial risk for schizophrenia. Nature 604, 509–516. doi: 10.1038/s41586-022-04556-w
Soiza-Reilly, M., Meye, F. J., Olusakin, J., Telley, L., Petit, E., Chen, X., et al. (2019). SSRIs target prefrontal to raphe circuits during development modulating synaptic connectivity and emotional behavior. Mol. Psychiatry 24, 726–745. doi: 10.1038/s41380-018-0260-9
Spatz Widom, C., DuMont, K., and Czaja, S. J. (2007). A prospective investigation of major depressive disorder and comorbidity in abused and neglected children grown up. Arch. Gen. Psychiatry 64, 49–56. doi: 10.1001/archpsyc.64.1.49
Tada, H., Miyazaki, T., Takemoto, K., Takase, K., Jitsuki, S., Nakajima, W., et al. (2016). Neonatal isolation augments social dominance by altering actin dynamics in the medial prefrontal cortex. Proc. Natl. Acad. Sci. 113, E7097–E7105. doi: 10.1073/pnas.1606351113
Takeda, T., Makinodan, M., Toritsuka, M., and Iwata, N. (2024). Impacts of adverse childhood experiences on individuals with autism spectrum disorder. Curr. Opin. Neurobiol. 89:102932. doi: 10.1016/j.conb.2024.102932
Tasic, B., Yao, Z., Graybuck, L. T., Smith, K. A., Nguyen, T. N., Bertagnolli, D., et al. (2018). Shared and distinct transcriptomic cell types across neocortical areas. Nature 563, 72–78. doi: 10.1038/s41586-018-0654-5
Teissier, A., Le Magueresse, C., Olusakin, J., Andrade da Costa, B. L. S., De Stasi, A. M., Bacci, A., et al. (2020). Early-life stress impairs postnatal oligodendrogenesis and adult emotional behaviour through activity-dependent mechanisms. Mol. Psychiatry 25, 1159–1174. doi: 10.1038/s41380-019-0493-2
Thion, M. S., Ginhoux, F., and Garel, S. (2018). Microglia and early brain development: An intimate journey. Science 362, 185–189. doi: 10.1126/science.aat0474
Torres-Berrío, A., Estill, M., Patel, V., Ramakrishnan, A., Kronman, H., Minier-Toribio, A., et al. (2024). Mono-methylation of lysine 27 at histone 3 confers lifelong susceptibility to stress. Neuron 112, 2973–2989.e10. doi: 10.1016/j.neuron.2024.06.006
Tottenham, N., and Galván, A. (2016). Stress and the adolescent brain. Neurosci. Biobehav. Rev. 70, 217–227. doi: 10.1016/j.neubiorev.2016.07.030
Tractenberg, S. G., Levandowski, M. L., de Azeredo, L. A., Orso, R., Roithmann, L. G., Hoffmann, E. S., et al. (2016). An overview of maternal separation effects on behavioural outcomes in mice: evidence from a four-stage methodological systematic review. Neurosci. Biobehav. Rev. 68, 489–503. doi: 10.1016/j.neubiorev.2016.06.021
Tsutsui, K.-I., Oyama, K., Nakamura, S., and Iijima, T. (2016). Comparative overview of visuospatial working memory in monkeys and rats. Front. Syst. Neurosci. 10:99. doi: 10.3389/fnsys.2016.00099
Usui, N., Ono, Y., Aramaki, R., Berto, S., Konopka, G., Matsuzaki, H., et al. (2021). Early life stress alters gene expression and Cytoarchitecture in the prefrontal cortex leading to social impairment and increased anxiety. Front. Genet. 12:754198. doi: 10.3389/fgene.2021.754198
van Eden, C. G., Kros, J. M., and Uylings, H. B. M. (1991). Chapter 8 the development of the rat prefrontal cortex: its size and development of connections with thalamus, spinal cord and other cortical areas. Progress Brain Res., 85:169–183. doi: 10.1016/S0079-6123(08)62680-1
Walker, C.-D., Bath, K. G., Joels, M., Korosi, A., Larauche, M., Lucassen, P. J., et al. (2017a). Chronic early life stress induced by limited bedding and nesting (LBN) material in rodents: critical considerations of methodology, outcomes and translational potential. Stress 20, 421–448. doi: 10.1080/10253890.2017.1343296
Walker, D. M., Bell, M. R., Flores, C., Gulley, J. M., Willing, J., and Paul, M. J. (2017b). Adolescence and reward: making sense of neural and behavioral changes amid the Chaos. J. Neurosci. 37, 10855–10866. doi: 10.1523/JNEUROSCI.1834-17.2017
Wamsley, B., and Fishell, G. (2017). Genetic and activity-dependent mechanisms underlying interneuron diversity. Nat. Rev. Neurosci. 18, 299–309. doi: 10.1038/nrn.2017.30
Wang, Z.-J., Shwani, T., Liu, J., Zhong, P., Yang, F., Schatz, K., et al. (2022). Molecular and cellular mechanisms for differential effects of chronic social isolation stress in males and females. Mol. Psychiatry 27, 3056–3068. doi: 10.1038/s41380-022-01574-y
Wang, J., Zhang, W., Xu, H., Ellenbroek, B., Dai, J., Wang, L., et al. (2023). The changes of histone methylation induced by adolescent social stress regulate the resting-state activity in mPFC. Research 6:0264. doi: 10.34133/research.0264
Wei, J., Ma, L., Ju, P., Yang, B., Wang, Y.-X., and Chen, J. (2020). Involvement of oxytocin receptor/Erk/MAPK signaling in the mPFC in early life stress-induced autistic-like behaviors. Front. Cell Dev. Biol. 8:564485. doi: 10.3389/fcell.2020.564485
Woodward, N. D., and Heckers, S. (2016). Mapping Thalamocortical functional connectivity in chronic and early stages of psychotic disorders. Biol. Psychiatry 79, 1016–1025. doi: 10.1016/j.biopsych.2015.06.026
Wu, Y., Dissing-Olesen, L., MacVicar, B. A., and Stevens, B. (2015). Microglia: dynamic mediators of synapse development and plasticity. Trends Immunol. 36, 605–613. doi: 10.1016/j.it.2015.08.008
Xu, P., Chen, A., Li, Y., Xing, X., and Lu, H. (2019). Medial prefrontal cortex in neurological diseases. Physiol. Genomics 51, 432–442. doi: 10.1152/physiolgenomics.00006.2019
Xu, H., Wang, J., Zhang, K., Zhao, M., Ellenbroek, B., Shao, F., et al. (2018). Effects of adolescent social stress and antidepressant treatment on cognitive inflexibility and Bdnf epigenetic modifications in the mPFC of adult mice. Psychoneuroendocrinology 88, 92–101. doi: 10.1016/j.psyneuen.2017.11.013
Yamamuro, K., Bicks, L. K., Leventhal, M. B., Kato, D., Im, S., Flanigan, M. E., et al. (2020). A prefrontal–paraventricular thalamus circuit requires juvenile social experience to regulate adult sociability in mice. Nat. Neurosci. 23, 1240–1252. doi: 10.1038/s41593-020-0695-6
Yao, X., Lu, Z., Feng, Z., Gao, L., Zhou, X., Li, M., et al. (2022). Comparison of chromatin accessibility landscapes during early development of prefrontal cortex between rhesus macaque and human. Nat. Commun. 13:3883. doi: 10.1038/s41467-022-31403-3
Yaseen, A., Shrivastava, K., Zuri, Z., Hatoum, O. A., and Maroun, M. (2019). Prefrontal oxytocin is involved in impairments in prefrontal plasticity and social memory following acute exposure to high fat diet in juvenile animals. Cereb. Cortex 29, 1900–1909. doi: 10.1093/cercor/bhy070
Yizhar, O., and Levy, D. R. (2021). The social dilemma: prefrontal control of mammalian sociability. Curr. Opin. Neurobiol. 68, 67–75. doi: 10.1016/j.conb.2021.01.007
Zhao, M., Wang, W., Jiang, Z., Zhu, Z., Liu, D., and Pan, F. (2020). Long-term effect of post-traumatic stress in adolescence on dendrite development and H3K9me2/BDNF expression in male rat Hippocampus and prefrontal cortex. Front. Cell Dev. Biol. 8:682. doi: 10.3389/fcell.2020.00682
Zhao, Y., Zhang, Q., Shah, C., Li, Q., Sweeney, J. A., Li, F., et al. (2022). Cortical thickness abnormalities at different stages of the illness course in schizophrenia. JAMA Psychiatry 79, 560–570. doi: 10.1001/jamapsychiatry.2022.0799
Zhong, S., Zhang, S., Fan, X., Wu, Q., Yan, L., Dong, J., et al. (2018). A single-cell RNA-seq survey of the developmental landscape of the human prefrontal cortex. Nature 555, 524–528. doi: 10.1038/nature25980
Keywords: prefrontal cortex, critical period, plasticity, neural circuit, development, epigenetics, early life stress, neurodevelopmental disorder
Citation: Chen X, Kim Y and Kawaguchi D (2025) Development of the rodent prefrontal cortex: circuit formation, plasticity, and impacts of early life stress. Front. Neural Circuits. 19:1568610. doi: 10.3389/fncir.2025.1568610
Edited by:
Mariko Miyata, Tokyo Women’s Medical University, JapanReviewed by:
Naoki Nakagawa, Kyoto University, JapanCopyright © 2025 Chen, Kim and Kawaguchi. This is an open-access article distributed under the terms of the Creative Commons Attribution License (CC BY). The use, distribution or reproduction in other forums is permitted, provided the original author(s) and the copyright owner(s) are credited and that the original publication in this journal is cited, in accordance with accepted academic practice. No use, distribution or reproduction is permitted which does not comply with these terms.
*Correspondence: Daichi Kawaguchi, ZGthd2FndWNoaUBtb2wuZi51LXRva3lvLmFjLmpw