- 1Laboratory of Translational Endocrinology, Carlos Chagas Filho Institute of Biophysics, Federal University of Rio de Janeiro, Rio de Janeiro, Brazil
- 2Laboratory of Molecular Endocrinology, Carlos Chagas Filho Institute of Biophysics, Federal University of Rio de Janeiro, Rio de Janeiro, Brazil
- 3Laboratory of Inflammation, Carlos Chagas Filho Institute of Biophysics, Federal University of Rio de Janeiro, Rio de Janeiro, Brazil
- 4Laboratory of Pulmonary Investigation, Carlos Chagas Filho Institute of Biophysics, Federal University of Rio de Janeiro, Rio de Janeiro, Brazil
Fasting and sepsis induce profound changes in thyroid hormone (TH) central and peripheral metabolism. These changes affect TH action and are called the non-thyroidal illness syndrome (NTIS). To date, it is still debated whether NTIS represents an adaptive response or a real hypothyroid state at the tissue level. Moreover, even though it has been considered the same syndrome, we hypothesized that fasting and sepsis induce a distinct set of changes in thyroid hormone metabolism. Herein, we aimed to evaluate the central and peripheral expression of genes involved in the transport (MCT8/Slc16a2 and MCT10/Slc16a10), metabolism (Dio1, Dio2, and Dio3) and action (Thra and Thrb) of TH during NTIS induced by fasting or sepsis. Male mice were subjected to a 48 h period of fasting or cecal ligation and puncture (CLP)-induced sepsis. At the peripheral level, fasting led to: (1) reduced serum thyroxine (T4) and triiodothyronine (T3), expression of Dio1, Thra, Slc16a2, and MCT8 protein in liver; (2) increased hepatic Slc16a10 and Dio3 expression; and (3) decreased Slc16a2 and Slc16a10 expressions in the thyroid gland. Fasting resulted in reduction of Tshb expression in the pituitary and increased expression of Dio2 in total hypothalamus, arcuate (ARC) and paraventricular (PVN) nucleus. CLP induced sepsis resulted in reduced: (1) T4 serum levels; (2) Dio1, Slc16a2, Slc16a10, Thra, and Thrb expression in liver as well as Slc16a2 expression in the thyroid gland (3) Thrb and Tshb mRNA expression in the pituitary; (4) total leukocyte counts in the bone marrow while increased its number in peritoneal and pleural fluids. In summary, fasting- or sepsis-driven NTIS promotes changes in the set point of hypothalamus-pituitary-thyroid axis through different mechanisms. Reduced hepatic THRs expression in conjunction with reduced TH transporters expression in the thyroid gland may indicate, respectively, reduction in the peripheral action and in the secretion of TH, which may contribute to the low TH serum levels observed in both models.
Introduction
The non-thyroidal illness syndrome (NTIS), a clinical entity typically manifested by reduced conversion of thyroxine (T4) to triiodothyronine (T3) in different acute and chronic systemic conditions, is still a debated topic (Fliers et al., 2015). A long period of fasting induces a decrease in serum thyroid hormone levels (TH, T4, and T3) and an unresponsiveness in the hypothalamus-pituitary-thyroid (HPT) axis (Boelen et al., 2011; Fliers et al., 2014). Fasting also leads to a decrease in peripheral T4 to T3 conversion and reverse-T3 (rT3) clearance in humans (Warner and Beckett, 2010).
Sepsis can also lead to NTIS, with important consequences to the immunological system (van der Spek et al., 2017) and may affect critically ill patients outcome (Iervasi et al., 2003; Scoscia et al., 2004). During sepsis induced by LPS administration, TRH expression decreases through the up regulation of deiodinase type 2 in tanycytes, which further activates local T4, despite the low TH serum levels (Boelen et al., 2004b; De Vries et al., 2014).
It has long been postulated that abnormal levels of thyroid hormones in NTIS could represent an adaptive response to minimize metabolic demands during illness (Van den Berghe, 2014). However, it remains controversial whether NTIS is an adaptive response or a pathophysiological state that requires correction (Golombek, 2008). Additionally, serum thyroid hormone levels may not be indicative of tissue-specific T3 action because of the alterations in intracellular T4 and T3 uptake, local metabolism and receptor binding (Warner and Beckett, 2010).
The analysis of central and peripheral changes in thyroid hormone metabolism during NTIS allows a better understanding of systemic adaptive mechanisms that lead to HPT axis unresponsiveness during fasting and sepsis (Warner and Beckett, 2010; Boelen et al., 2011; Fliers et al., 2014). We hypothesized that sepsis and fasting would differentially change the expression of thyroid hormone metabolism target genes in HPT axis and liver of mice with more pronounced regulation in the Sepsis model. For this purpose, the objective of the present study is to evaluate, in an integrated view, the central and peripheral expression of genes involved in the transport (Slc16a2 and Slc16a10), metabolism (Dio1, Dio2, and Dio3) and action (Thra and Thrb) of thyroid hormones in murine models of NTIS induced by fasting or cecal ligation puncture (CLP)-induced sepsis.
Materials and Methods
Ethics Statement
This study was approved by the Animal Care Committee of the Health Sciences Center, Federal University of Rio de Janeiro (CEUA-190/13), and registered with the Brazilian National Council for Animal Experimentation Control. All animals received humane care in compliance with the “Principles of Laboratory Animal Care” formulated by the National Society for Medical Research and the U.S. National Academy of Sciences Guide for the Care and Use of Laboratory Animals.
Animal Experiment
Male 129/SVxC57/BL6 mice, 10–12 weeks of age, weighting 22–25 g, were kept under a 12/12 h light/darkness cycle, in a controlled-temperature environment (23°C).
For fasting, in three independent experiments, 30 mice were divided into two groups (n = 15/group): one without access to food for 48 h (fasting group) and another group with food available ad libitum (control group). Both groups had free access to water. Twenty animals (n = 10/group) were used to evaluate mRNA expression and hormone levels. Ten animals (n = 5/group) were used to evaluate thyroid monocarboxilate transporter 8 by immunohistochemistry.
For sepsis, three independent experiments were performed. Sepsis was induced by cecal ligation and puncture surgery in 17 animals, whereas, 10 mice were sham-operated and used as control. Briefly, animals were anesthetized with intraperitoneal (i.p.) injection of ketamine and xylazine (0.25 and 0.025 mg/kg, respectively) and a midline laparotomy (2-cm incision) was performed. The cecum was carefully isolated to prevent damage to blood vessels and ligated with a 3-0 cotton suture. The ligature was placed below the ileocecal valve to prevent bowel obstruction. Finally, the cecum was punctured once with an 18-gauge needle and the animals left to recover from anesthesia (de Araujo et al., 2012). In Sham group, the abdominal cavity was opened and the cecum was isolated without ligation and puncture. Mice were fasted for 16 h before surgery in both groups. Postoperative care was similar in both groups, and consisted of a subcutaneous injection of tramadol hydrochloride (20 mg/g body weight) in 1 mL warm (37°C) normal saline (NaCl 0.9%). At 24 h, seven animals from CLP group died.
Mice were killed under anesthesia with isoflurane (Cristalia, Brazil) after 48 h of fasting or 24 h of sepsis induction, at 10:00 am in order to avoid hormonal and gene expression circadian variation. After killing, blood, thyroid, liver, hypothalamus, and pituitary were collected for molecular biology analysis (mRNA and protein expression by qPCR and Western Blot, respectively). Serum was obtained from the blood and stored at −20°C and all tissues were immediately frozen in liquid nitrogen and stored at −70°C. Bone marrow, peritoneal, and pleural fluids were collected for leukocyte analysis.
Thyroid Hormones Serum Measurement
Serum total T3 and T4 concentrations were measured by radioimmunoassay (RIA), using a commercial kit (Total T4 MAb RIA Kit and Total T3 RIA Kit, MP Biomedicals, CA, USA) and following the manufacturer's recommendations. This kit is based on solid phase method and the limit of detection for T4 was 1 μg/dL and for T3 was 25 ng/dL.
mRNA Extraction and Real-Time PCR reaction
Total RNA from liver, hypothalamus, pituitary, and thyroid samples was extracted using the TRIzol method, according to manufacturer's protocol (TRIzol Reagent; Life Technologies, CA, USA). In the fasting experiment, total RNA from PVN and ARC nuclei cryosections was extracted using the RNA easy microkit (Qiagen, Hilden, Germany) following manufacturer's recommendations. The cDNA synthesis was performed using the High Capacity cDNA Reverse Transcription Kit (Applied Biosystems, CA, USA) with 1 or 0.5 μg of total RNA, for tissue or nuclei cryosections respectively. All RNA samples were reverse transcribed in a single reaction. After the cDNA synthesis, mRNA expressions were evaluated by qPCR using the Maxima SYBR Green/ROX qPCR Master Mix 2X (Thermo Fisher Scientific, MA, USA) and the Master Cycler Realplex system (Eppendorf, Germany). Primer pair's sequences are shown in Table 1. The efficiency range accepted for each assay was 95–105%. qPCR quality and genomic DNA contamination was checked using intron-spanning primers, reverse transcriptase-negative samples from cDNA synthesis and melting curve analysis obtained from each reaction. Quantification of the samples mRNA expression was calculated from the quantification cycle (Cq) by the 2−ΔΔCq method (Livak and Schmittgen, 2001) and corrected using 36β4 (Rplp0) expression as the reference gene. The results are expressed relative to the values of the control group (fasting) or Sham (CLP-induced sepsis), which were considered to be equal to 1.
Hypothalamic Paraventricular (PVN) and Arcuate (ARC) Nuclei Microdissection
Hypothalamic PVN and ARC nuclei were obtained from snap frozen mouse brains (n = 10/group), previously collected with the other tissues, as described in “animal experiment” section. The nuclei were collected in cryosections by the punch technique, previously described (Franco et al., 2012, 2015), with particularities for the mouse brain, and following the mouse brain stereotaxic atlas coordinates (Paxinos and Franklin, 2004). The PVN and ARC nucleus were obtained through subsequently 640 and 1,500 μM slices. The slices were collected, respectively, at 0.58 mm and 1.22 mm posterior to the bregma.
Western Blotting
Liver samples were homogenized using the Tissuelyser LT (Qiagen, Hilden, Germany) with lyses buffer (pH 6.4; 50 mM HEPES, 1 mM MgCl2, 10 nM EDTA, and 1% Triton X) and a protease inhibitor cocktail (Roche/DSM Nutritional). Total protein extract (20 μg), previously denatured, was submitted to SDS-PAGE electrophoresis and transferred to a polyvinylidene difluoride membrane (PVDF Hybond-P; Amersham Biosciences, Buckinghamshire, UK). After that, the membrane was blocked for 2 h with 5% non-fat dry milk (Molico; Nestle, São Paulo, Brazil) and incubated overnight with anti-MCT8 antibody (1:750, Santa Cruz Biotechnology) and anti-cyclophilin antibody (1:250.000; Affinity Bioreagents, Golden, CO, USA), the loading control. Both primary antibodies were diluted in Tween-TBS (TBS + 0.1% Tween 20) with 2% non-fat dry milk. Membranes were then incubated for 3 h with secondary antibodies: HRP anti-goat IgG (1:7,000; Santa Cruz Biotechnology) and HRP anti-rabbit igG (1.2:10,000; Amersham Biosciences, Buckinghamshire, UK). After seven wash steps, the membranes were then incubated for chemiluminescence visualization with ECL (ECL Western Blotting System, Amershan Biosciences) and densitometry analysis was performed using the Image Quant Las4000 software (GE HealthCare Life Sciences, Buckinghamshire, UK). The results are expressed relative to the values of the control group (fasting) or Sham (CLP-induced sepsis), which were considered to be equal to 1.
Immunohistochemistry
The thyroid glands were fixed in paraformaldehyde 4%. Cryopreservation was performed with sucrose gradient and posterior OCT embedding. A 5 μm cut was obtained with an ultramicrotome and the sections were then incubated with primary anti-MCT8 antibody (sc-47125, Santa Cruz Biotechnollogy), followed by the appropriate biotinylated secondary antibody (DCMT999, Spring), streptavidin-peroxidase (DHRR999, Spring) and finally 3,3-diamino-benzidine (DAB -ACB060, SCYTEK). The sections were then stained with Mayer's hematoxylin and mounted with glycerol. To quantify the MCT8 protein expression levels in the control and fasting groups, 30 random fields per animal were captured using an AXIOSCOPE microscope with an AXIOCAM HRc camera (CarlZeiss AG, Jena, Germany) at a magnification of 100× and analyzed with Image J analysis software.
Total and Differential Leukocyte Counts
In CLP induced sepsis animals, after 24 h, total and differential leukocyte counts were evaluated in bone marrow, peritoneal lavage fluid, and pleura. Peritoneal and pleural fluids were collected by flushing these cavities twice with 1.0 ml of 37°C sterile, PBS containing 10 mM EDTA using a micropippete. Mice femoral epiphysis were collected, centrifuged (98 g, 10 min, 4°C) and cell pellets were re-suspended in PBS for further quantification of leukocytes in the hemocytometer, as well as peritoneal and pleural washes, after dilution in Türk solution (1:40). After total leukocyte counts, samples from bone marrow and peritoneal/pleural washes were centrifuged (221 g, 10 min, 5°C). From the cell pellets obtained, differential cell counts were performed on May–Grunwald–Giemsa-stained cytospin preparations under an oil immersion objective to determine the percentage of mononuclear cells, eosinophils, and neutrophils in 100 leukocytes counted.
Statistical Analysis
Sample size calculation was based on previous experimental studies of fasting (de Vries et al., 2015) and sepsis (Bloise et al., 2016). A sample size of eight animals per group (allowing for one animal as dropout) would provide the appropriate power (1-β = 0.8) to identify significant (α = 0.05) differences in variable analyzed between injured (fasting or sepsis) and Control or Sham groups, taking into account an effect size d = 1.8, a two-sided test, and a sample size ratio = 1 (G*Power 3.1.9.2, University of Düsseldorf, Germany).
The Kolmogorov-Smirnov test with Lilliefors' corrections was used to test for normality of data, while the Levene median test was used to evaluate the homogeneity of variances. Rout test was used to remove outliers. The data are expressed as the mean ± the standard error of the mean (SEM). The Student's t-test was used for comparisons between two groups when data was normal otherwise Mann Whitney test was used. (mRNA expression, western blotting, RIA, and leukocyte counts). Statistical analyses were performed using the Graphpad prism 6 software (GraphPad Software, Inc., San Diego, CA, USA). Differences were considered to be significant at P < 0.05.
Results
Body Weight and Serum Parameters
In fasting group, serum T4 levels were undetectable and serum T3 levels were lower (38%) in fasting mice compared to control (Table 2, P < 0.001). Fasting group also showed a 22% reduction in total body weight after a 48-h period of fasting (20.1 ± 1.4 g), compared to control (25.9 ± 0.9 g, P < 0.01).
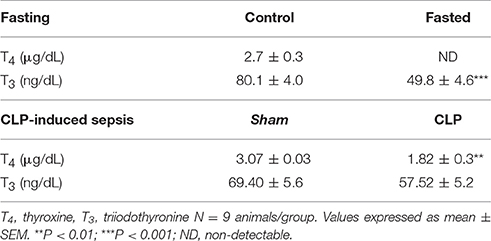
Table 2. Serum T4 and T3 levels, in control and fasted mice (fasting) and sham and CLP-induced sepsis mice.
In CLP-induced sepsis group, serum T4 levels decreased 40% compared to Sham (Table 2, P < 0.01). No significant changes were observed in serum T3 levels between fasting and CLP groups.
Total and Differential Leukocyte Counts in CLP group
Total leukocyte counts (Figure 1) in bone marrow decreased by 37% in CLP-induced sepsis group (P < 0.05) and increased by 2.5- and 1.5-fold, respectively, in the peritoneal and pleural lavages of the CLP group (P < 0.01 and P < 0.05, respectively). Differential leukocyte counts (Figure 1) showed a 67% decrease in bone marrow neutrophils of the CLP-induced sepsis group (P < 0.05). It was also observed an important increase in neutrophils of the peritoneal and pleural lavages in the CLP mice, compared to Sham group (P < 0.05).
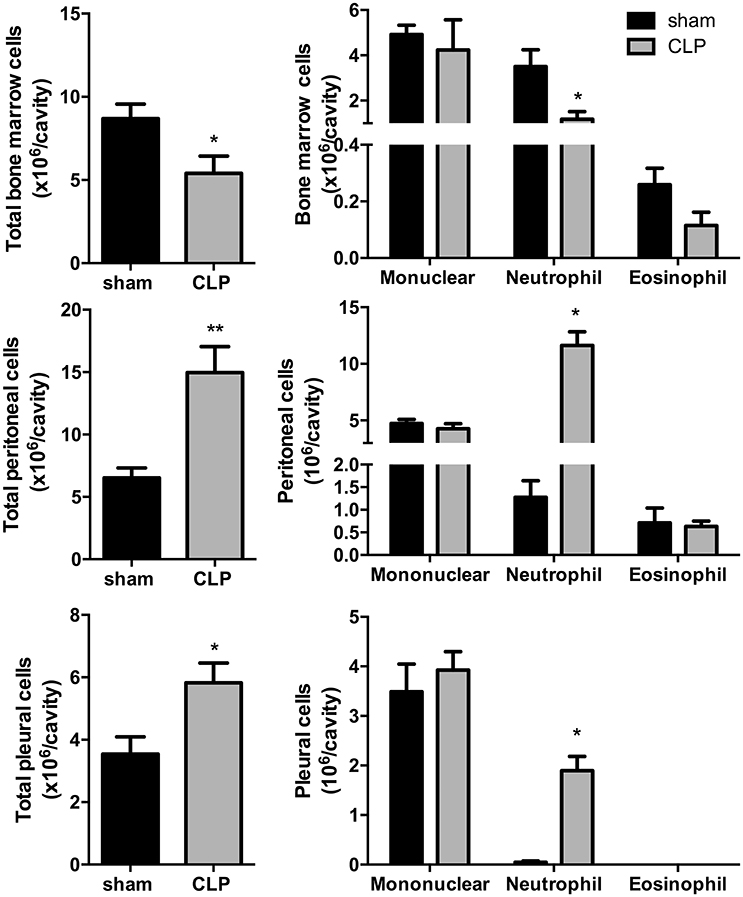
Figure 1. CLP-induced sepsis: total (Left) and differential (Right) leukocyte counts from bone marrow, peritoneal, and pleural lavage fluids. N = 8–9 animals/group. Values are expressed as mean±standard error of the mean (SEM). *P < 0.05. **P < 0.01.
Liver Thyroid Hormone Metabolism
The influence of fasting (Figure 2A) or CLP-induced sepsis (Figure 2B) on liver thyroid hormone metabolism was evaluated through the analysis of deiodinases mRNA expression. Dio1 expression was decreased by 97% (P < 0.001) and Dio3 expression had a 3-fold increase in fasting mice, compared to control group (P < 0.05). Since genomic actions of thyroid hormones depend on their binding to nuclear receptors (THRs), we evaluated the expression of Thra and Thrb, which encode to the transcription factors and T3 nuclear receptors Thrα and Thrβ, respectively. Thra expression decreased by 55% in fasting group compared to control (Figure 2A, P < 0.001), but Thrb expression did not change. To analyze whether the reduced Thra mRNA expression could reflect reduced T3 action, we investigated the mRNA expression of Thrsp in the liver, a T3 positive-regulated gene (Jump et al., 1984). Fasted mice showed a 98% reduction in Thrsp mRNA expression compared to control group (Figure 2A, P < 0.01).
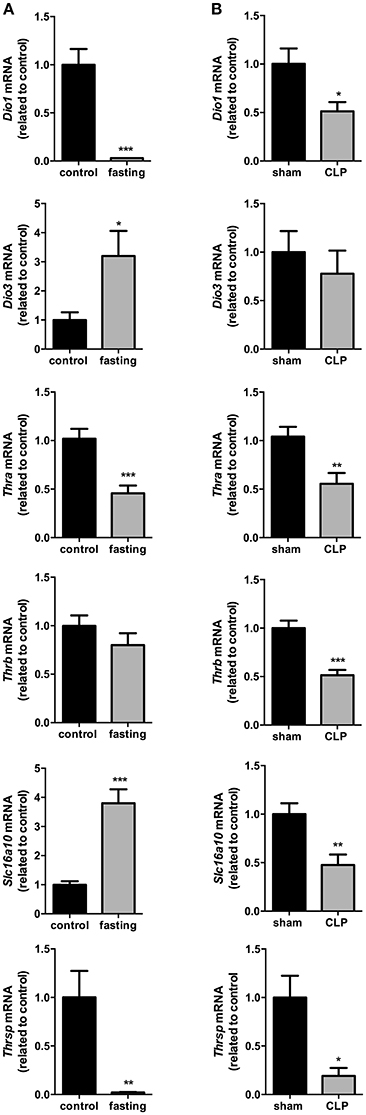
Figure 2. Thyroid hormone metabolism on fasted mice (A) and during CLP-induced sepsis (B): Dio1, Dio3, Thra, Thrb, Slc16a10, and Thrsp mRNA expression in the liver. N = 6–9 animals/group. Values expressed as mean±SEM. *P < 0.05. **P < 0.01. ***P < 0.001.
In CLP-induced sepsis group, Dio1 expression was decreased by 49% (Figure 2B, P < 0.05) and Dio3 expression did not change between CLP-induced sepsis and Sham groups. CLP-induced sepsis mice showed a decrease in both Thra and Thrb mRNA expressions by 45% (P < 0.01) and 49% (P < 0.001), respectively, compared to Sham group. CLP-induced sepsis also promoted 87% decrease Thrsp mRNA expression in the liver (P < 0.05).
We then investigated the expression of the monocarboxylate transporters MCT8 and MCT10. Fasting promoted a 4-fold increase in Slc16a10 mRNA expression (Figure 2A, P < 0.001) and, in contrast, decreased by 77% Slc16a2 gene expression (Figure 3A, P < 0.001). Furthermore, fasted mice showed a 30% reduction in MCT8 protein expression in the liver compared to the control group (Figures 3B,C, P < 0.05). In contrast, CLP-induced sepsis promoted a 53% decrease in liver Slc16a10 mRNA expression (Figure 2B, P < 0.01) and a 49% decrease in liver Slc16a2 mRNA expression (Figure 3D, P < 0.05). No differences were seen on MCT8 protein expression in CLP-induced sepsis (Figures 3E,F).
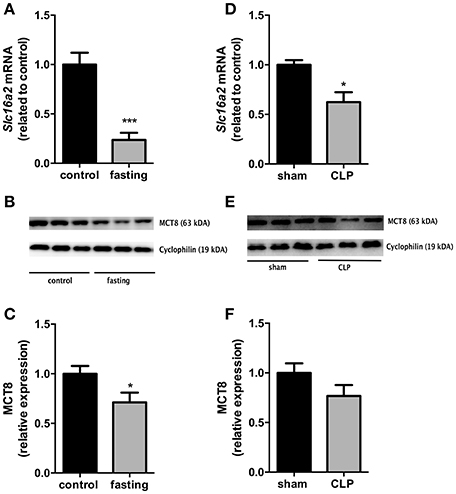
Figure 3. MCT8 expression on fasted mice (A–C) and during CLP-induced sepsis (D–F): Slc16a2 mRNA expression (A,D) and MCT8 protein expression in the liver (B,C,E,F). N = 6 animals/group. Values expressed as mean ± SEM. *P < 0.05. ***P < 0.001.
Expression of Monocarboxilate Transporters in Thyroid
We analyzed the expression of monocarboxilate transporters (MCT8 and MCT10) in the thyroid gland to investigate a possible role of this gland, during fasting and CLP-induced sepsis, causing changes in serum thyroid hormone levels. mRNA expression of Slc16a2 and Slc16a10 was decreased by 50% in fasting mice, compared to control group (Figure 4A, P < 0.05 and P < 0.01, respectively). In CLP-induced sepsis, Slc16a2 mRNA expression decreased 48% compared to control (Figure 4B, P < 0.05). Slc16a10 mRNA expression did not differ between Sham and CLP-induced sepsis (Figure 4B). Furthermore, immunohistochemistry analysis showed that fasting decreased MCT8 staining in the thyrocytes (Figure 4C, P < 0.01).
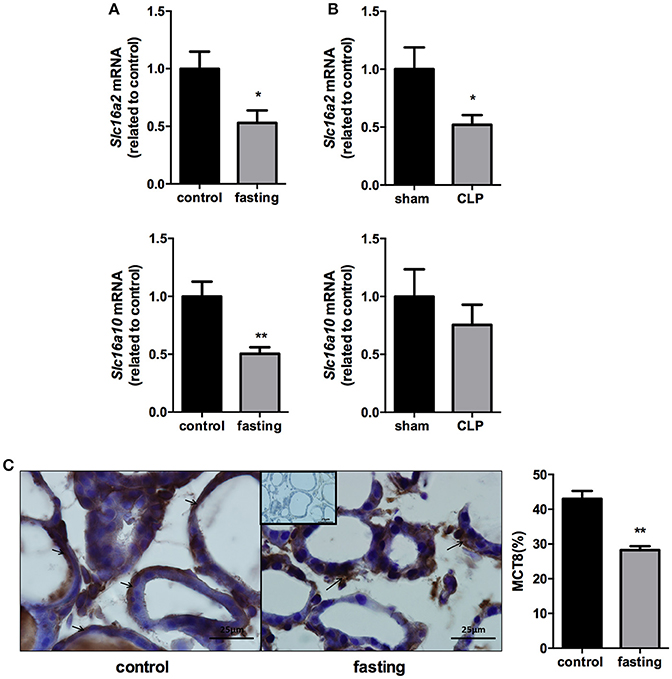
Figure 4. Slc16a2 and Slc16a10 mRNA expression in the thyroid of fasted (A) and CLP-induced sepsis (B). Photomicrography and relative quantification of MCT8 immunostaining in the thyroid gland on fasted mice (C). The black arrows show MCT8 staining in the thyrocytes basolateral membranes. Inset represents the negative control. N = 9 animals/group (A,B) and five animals/group (C). Values expressed as mean±SEM. *P < 0.05. **P < 0.01.
Central Thyroid Hormone Metabolism
Since NTIS changes the set point of HPT axis through different mechanisms in fasting or CLP-induced sepsis, we set out to evaluate the expression of genes involved in thyroid hormone metabolism in the pituitary and the hypothalamus.
The expression of Slc16a2, Dio1, Dio2, and Thrb in the pituitary did no change in fasting mice (Figure 5A), although fasting promoted a 94% reduction in Tshb mRNA expression (Figure 5A, P < 0.0001), which suggest a decrease in the response of the pituitary to the low serum levels of T4.
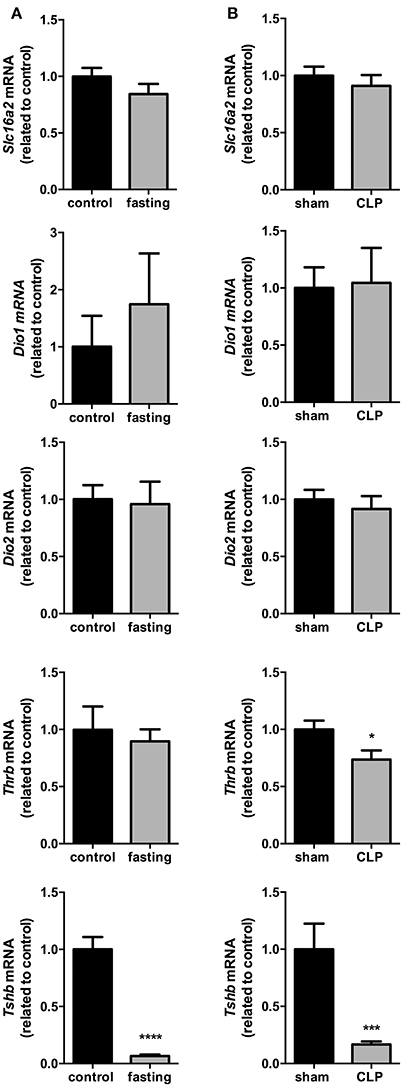
Figure 5. Pituitary thyroid hormones metabolism on fasted mice (A) and during CLP-induced sepsis (B). Slc16a2, Dio1, Dio2, Thrb, and Tshb mRNA expression in the pituitary. N = 6–9 animals/group. Values expressed as mean±SEM. *P < 0.05. ***P < 0.001. ****P < 0.0001.
Slc16a2, Dio1, and Dio2 mRNA expressions did not differ between Sham and CLP-induced sepsis groups (Figure 5B). However, both Thrb and Tshb mRNA expressions reduced 27% (Figure 5B, P < 0.05) and 76% (Figure 5B, P < 0.001), respectively, confirming that in this model of NTIS, HPT axis is also less responsive to low T4 serum levels.
In the total hypothalamus, the expression of Slc16a2, Slc16a10, Dio3, and Thrb did not change in these two models fasting and CLP-induced sepsis (Figures 6A,B). However, we observed a 2-fold increase in the expression of Dio2 mRNA in fasting mice, compared to control group (Figure 6A, P < 0.05). Dio2 expression did not change in the total hypothalamus of CLP-induced sepsis mice, compared to control group (Figure 6B). Thus, we decided to perform a more thorough analysis of Dio2 expression in PVN and ARC nuclei only on the fasting model.
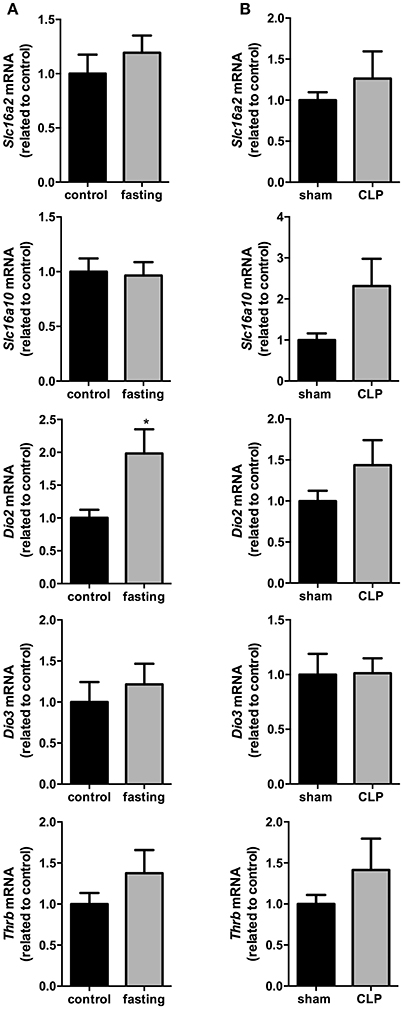
Figure 6. Hypothalamus thyroid hormones metabolism on fasted mice (A) and during CLP-induced sepsis (B). Slc16a2, Slc16a10, Dio2, Dio3, and Thrb mRNA expression in the hypothalamus. N = 6–8 animals/group. Values expressed as mean±SEM. *P < 0.05.
Expression of Dio2 in the PVN and ARC Nucleus (Fasting Experiment)
We then investigated the Dio2 expression in the PVN and ARC nucleus. In the PVN, Dio2 mRNA expression presented a 2.3-fold increase in fasting group (Figure 7, P < 0.01). In the ARC, fasting promoted a 1.6-fold increase in Dio2 mRNA expression (Figure 7, P < 0.01).
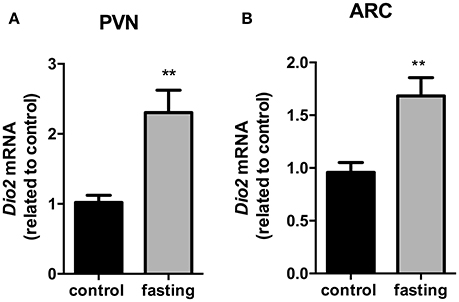
Figure 7. Dio2 mRNA expression in the hypothalamic PVN (A) and ARC (B) nucleus on fasted mice. PVN, paraventricular nucleus. ARC, arcuate nucleus. N = 8 animals/groups. Values expressed as mean±SEM.**P < 0.01.
Discussion
In the present study we showed that fasting and CLP-induced sepsis promoted changes in the systemic TH economy through different mechanisms. Our main finding indicates that, during fasting, Dio2 up-regulation in PVN/ARC nuclei seems to be an adaptive mechanism involved in the HPT-axis impairment, while in CLP-induced sepsis this impairment is probably associated with inflammatory mediators and independent of T3 uptake and action.
CLP model for induction of sepsis in mice is considered the gold standard for sepsis research, inducing a polymicrobial sepsis and mimicking the inflammatory and vascular patterns observed in humans (Dejager et al., 2011). However, CLP-induced sepsis is still an underexplored murine model of NTIS (Dejager et al., 2011). In our study, we observed a decrease in Dio1 liver expression of CLP-induced septic mice, which is considered a hallmark of NTIS according to previous studies (O'Mara et al., 1993; Boelen et al., 1996; de Vries et al., 2015). This finding is related to the influence of inflammatory cytokines on THRβ and THRα expression (Boelen et al., 2004a; Kwakkel et al., 2010) and the redox state of the hepatocytes (Wajner et al., 2011). The reduction in the neutrophils counts in bone marrow and an increase in peritoneal and pleural lavages in the CLP-induced sepsis group confirmed what is observed in abdominal septic patients (Asaduzzaman et al., 2008). We also observed a decrease in serum T4 levels after 24 h of CLP surgery another hallmarks of NTIS in mice, confirming the CLP-induced sepsis as a model for NTIS.
Fasting promotes profound changes in thyroid hormone economy and its effects on central and peripheral TH metabolism in rodents are similar to those observed in humans (Boelen et al., 2008), which makes it an efficient and easy-to-execute experimental model for understanding similarities and differences that may arise from the inflammatory stimulus per se or from the food deprivation state. A recent study from Galton et al. demonstrated that the decrease in serum T3 and T4 levels during fasting occurs even in the absence of deiodinase type I. However, D3 knockout (D3KO) mice demonstrate a blunting of the fasting-induced decrease in serum T4 and T3 levels, revealing that D3 is, in part, responsible for the adaptive systemic changes in thyroid hormone economy during food deprivation (Galton et al., 2014). In contrast to the increase in Dio3 expression during fasting, we did not observe changes in Dio3 expression in the CLP-induced sepsis. However, thyroid hormone serum levels in D3KO mice subjected to bacterial sepsis decreased equally compared to WT mice (Boelen et al., 2009), which indicates that D3 does not play an important role in the decrease in thyroid hormone levels during sepsis.
In both models, we observed decreased TH serum levels and hepatic Dio1 mRNA, which could be caused by a decrease in TH influx into the hepatocytes, therefore we investigated the expression of the two main TH transporters in the liver, MCT8 and MCT10. In both models, MCT8 expression was decreased what suggests, together with hepatic deiodinases decreased part of a particular adaptive mechanism to reduce energy expenditure. In contrast to our results, a recent study in a rabbit model of prolonged critical illness demonstrated an increase in the hepatic expression of Slc16a2, which was inversely correlated with serum thyroid hormone parameters (Mebis et al., 2009). The differences between immunological effectors in acute and prolonged stage of critical illness (Van den Berghe, 2014) and also in the induction of NTIS can be the explanation of such down regulation.
While MCT8 is a specific T3 and T4 transporter, MCT10 transports not only thyroid hormones but also aromatic amino acids (Van Der Deure et al., 2010). In a recent study, de Vries et al. described an increase in Slc16a10 expression after 36 h of fasting in male rats (de Vries et al., 2015). Our results in our mice fasting model, corroborate de Vries' results. However, we did not observe changes in Slc16a10 expression in the liver of CLP-induced sepsis mice. The increase in MCT10 expression in fasting may affect thyroid hormone transport but also can represent a compensatory response to the intense protein catabolism during fasting.
Once we observed a reduction in hepatic Slc16a2 expression in both models, we hypothesized that T3 content in the liver could also be reduced. Then, we investigated the expression of both TH receptors isoforms (Thra and Thrb) and a classic TH up-regulated gene, Thrsp (Jump et al., 1984). While Thrb expression did not change after fasting, Thra expression decreased by half, fact that can explain, in part, the reduction in thyroid hormone action, confirmed by the decrease in liver Thrsp expression in fasted mice. However, once ~60% of liver T3-regulated genes are THRβ-dependent (Boelen et al., 2011), and Dio1 (THRβ-mainly regulated gene) expression was reduced in the liver, we speculate that, in fact, liver T3 content is reduced. In the CLP-induced sepsis, the reduction in both Thra and Thrb mRNA and in Thrsp mRNA expression confirms a decrease in T3 action in liver, in agreement with the literature (Beigneux et al., 2003).
The function of MCT8 in the thyroid gland during NITS models has been unexplored in the literature. Different from other tissues, in the thyroid, MCT8 is involved in the secretion of T4 and T3 (Di Cosmo et al., 2010). We observed a decrease in Slc16a2 expression in both fasting and CLP-sepsis models. Thus, we suggest that the decrease in the expression of TH transporters in the thyroid gland is contributing to low levels of serum TH levels in both models. Altogether, we hypothesize that thyroid has a major role in the systemic thyroid economy during NTIS, with a notable contribution to the low serum T4 levels observed in both experimental models.
CLP-induced sepsis lead to a paradoxical decrease in both Tshb and Thrb mRNA expressions in the pituitary. Although THRβ mainly exerts the negative feedback regulation of the HPT axis, in vitro and in vivo studies show that inflammatory cytokines can regulate independently both genes during acute inflammation (Wassen et al., 1996; Boelen et al., 2004b). Then, we hypothesize that the impairment of HPT-axis during sepsis is due to the effects of inflammatory mediators and occurs independently of T3 uptake and action.
Coppola et al. in a very elegant study, revealed a role for hypothalamic D2, which leads to increased local T3 production, triggering the expression of uncoupling protein 2 (UCP2) and the activation of NPY/AgRP neurons in the arcuate nucleus during fasting (Coppola et al., 2007). In attempt to investigate the dramatic reduction in Tshb expression, and since Dio2 expression did not change in the total hypothalamus of CLP-induced sepsis mice, we decided to perform a more thorough analysis of Dio2 expression in PVN and ARC nuclei only during fasting. We observed an increase of Dio2 gene expression in total hypothalamus and in both PVN and ARC nucleus of fasted mice. It is consistent to conclude that the impairment in HPT axis during fasting is a consequence of the local hypothalamic hyperthyroid state, which in turn leads to the inappropriate Tshb reduced mRNA expression in the pituitary.
Finally, our NTIS models, promotes changes in thyroid hormone metabolism and HPT axis that could be part of a systemic adaptive response to reduce the energy expenditure in both models. At the peripheral level, fasting and sepsis promotes changes in the expression of thyroid hormone transporters and deiodinases in the liver, suggesting a decrease in T4 activation, along with changes in the expression of thyroid hormone transporters in the thyroid, which contribute to reduced thyroid hormone serum levels. At the central level, we suggest a role for the PVN and ARC in the generation of a hypothalamic hyperthyroid state during fasting, one of the NTIS hallmarks. In the septic pituitary, the decrease in Tshb and Thrb expressions, explains the impairment of the HPT axis independently of T3 uptake and action.
Conclusion
Although fasting and CLP-induced sepsis caused similar changes in peripheral thyroid hormone economy, the profound changes in the set point of HPT axis that characterize NITS are caused through different mechanisms.
Author Contributions
Conceptualization of the experiments: KF, AC, FB, CA, LS, LA, CB, PS, PR, and TO. Formal analysis: KF, AC, FB, CA, LS, MW, IT, LA, JS, CB, PS, PR, and TO. Funding acquisition: TO. Performed experiments: KF, AC, FB, CA, LS, MW, LA, JS, and PS. Project supervision: FB, CB, PS, PR, and TO. Writing draft: KF, AC, FB, CA, LS, MW, IT, LA, JS, CB, PS, PR, and TO. Writing review and editing: KF, AC, FB, LS, IT, CB, PS, PR, and TO.
Funding
This research was supported by Fundação Carlos Chagas Filho de Amparo à Pesquisa do Estado do Rio de Janeiro (FAPERJ, CNE 2015/E26/203.190/2015), Conselho Nacional de Pesquisa e Desenvolvimento (CNPq, 304667/2016-1, 422441/2016-3, 303734/2012-4), and Coordenação de Aperfeiçoamento Pessoal de Nível Superior (CAPES).
Conflict of Interest Statement
The authors declare that the research was conducted in the absence of any commercial or financial relationships that could be construed as a potential conflict of interest.
References
Asaduzzaman, M., Zhang, S., Lavasani, S., Wang, Y., and Thorlacius, H. (2008). LFA-1 and MAC-1 mediate pulmonary recruitment of neutrophils and tissue damage in abdominal sepsis. Shock 30, 254–259. doi: 10.1097/shk.0b013e318162c567
Beigneux, A., Moser, A., Shigenaga, J., Grunfeld, C., and Feingold, K. (2003). Sick euthyroid syndrome is associated with decreased TR expression and DNA binding in mouse liver. Am. J. Physiol. Endocrinol. Metab. 284, E228–E236. doi: 10.1152/ajpendo.00155.2002
Bloise, F. F., van der Spek, A. H., Surovtseva, O. V., Ortiga-Carvalho, T. M., Fliers, E., and Boelen, A. (2016). Differential effects of sepsis and chronic inflammation on diaphragm muscle fiber type, thyroid hormone metabolism, and mitochondrial function. Thyroid 26, 600–609. doi: 10.1089/thy.2015.0536
Boelen, A., Kwakkel, J., and Fliers, E. (2011). Beyond low plasma T3: local thyroid hormone metabolism during inflammation and infection. Endocr. Rev. 32, 670–693. doi: 10.1210/er.2011-0007
Boelen, A., Kwakkel, J., Platvoet-ter Schiphorst, M., Mentrup, B., Baur, A., Koehrle, J., et al. (2004a). Interleukin-18, a proinflammatory cytokine, contributes to the pathogenesis of non-thyroidal illness mainly via the central part of the hypothalamus-pituitary-thyroid axis. Eur. J. Endocrinol. 151, 497–502. doi: 10.1530/eje.0.1510497
Boelen, A., Kwakkel, J., Thijssen-Timmer, D. C., Alkemade, A., Fliers, E., and Wiersinga, W. M. (2004b). Simultaneous changes in central and peripheral components of the hypothalamus-pituitary-thyroid axis in lipopolysaccharide-induced acute illness in mice. J. Endocrinol. 182, 315–323. doi: 10.1677/joe.0.1820315
Boelen, A., Kwakkel, J., Wieland, C. W., St Germain, D. L., Fliers, E., and Hernandez, A. (2009). Impaired bacterial clearance in type 3 deiodinase-deficient mice infected with Streptococcus pneumoniae. Endocrinology 150, 1984–1990. doi: 10.1210/en.2008-1133
Boelen, A., Platvoet-ter Schiphorst, M. C., Van Rooijen, N., and Wiersinga, W. M. (1996). Selective macrophage depletion in the liver does not prevent the development of the sick euthyroid syndrome in the mouse. Eur. J. Endocrinol. 134, 513–518.
Boelen, A., Wiersinga, W. M., and Fliers, E. (2008). Fasting-induced changes in the hypothalamus-pituitary-thyroid axis. Thyroid 18, 123–129. doi: 10.1089/thy.2007.0253
Coppola, A., Liu, Z. W., Andrews, Z. B., Paradis, E., Roy, M. C., Friedman, J. M., et al. (2007). A central thermogenic-like mechanism in feeding regulation: an interplay between arcuate nucleus T3 and UCP2. Cell Metab. 5, 21–33. doi: 10.1016/j.cmet.2006.12.002
de Araujo, C. C., Silva, J. D., Samary, C. S., Guimaraes, I. H., Marques, P. S., Oliveira, G. P., et al. (2012). Regular and moderate exercise before experimental sepsis reduces the risk of lung and distal organ injury. J. Appl. Physiol. 112, 1206–1214. doi: 10.1152/japplphysiol.01061.2011
Dejager, L., Pinheiro, I., Dejonckheere, E., and Libert, C. (2011). Cecal ligation and puncture: the gold standard model for polymicrobial sepsis? Trends Microbiol. 19, 198–208. doi: 10.1016/j.tim.2011.01.001
De Vries, E. M., Kwakkel, J., Eggels, L., Kalsbeek, A., Barrett, P., Fliers, E., et al. (2014). NFκB signaling is essential for the lipopolysaccharide-induced increase of type 2 deiodinase in tanycytes. Endocrinology 155, 2000–2008. doi: 10.1210/en.2013-2018
de Vries, E. M., van Beeren, H. C., Ackermans, M. T., Kalsbeek, A., Fliers, E., and Boelen, A. (2015). Differential effects of fasting vs food restriction on liver thyroid hormone metabolism in male rats. J. Endocrinol. 224, 25–35. doi: 10.1530/JOE-14-0533
Di Cosmo, C., Liao, X. H., Dumitrescu, A. M., Philp, N. J., Weiss, R. E., and Refetoff, S. (2010). Mice deficient in MCT8 reveal a mechanism regulating thyroid hormone secretion. J. Clin. Invest. 120, 3377–3388. doi: 10.1172/JCI42113
Fliers, E., Bianco, A. C., Langouche, L., and Boelen, A. (2015). Thyroid function in critically ill patients. Lancet. Diabetes Endocrinol. 3, 816–825. doi: 10.1016/S2213-8587(15)00225-9
Fliers, E., Kalsbeek, A., and Boelen, A. (2014). Mechanisms in endocrinology: beyond the fixed setpoint of the hypothalamus-pituitary-thyroid axis. Eur. J. Endocrinol. 171, R197–R208. doi: 10.1530/EJE-14-0285
Franco, J. G., Dias-Rocha, C. P., Fernandes, T. P., Albuquerque Maia, L., Lisboa, P. C., Moura, E. G., et al. (2015). Resveratrol treatment rescues hyperleptinemia and improves hypothalamic leptin signaling programmed by maternal high-fat diet in rats. Eur. J. Nutr. 55, 601–610. doi: 10.1007/s00394-015-0880-7
Franco, J. G., Fernandes, T. P., Rocha, C. P. D., Calviño, C., Pazos-Moura, C. C., Lisboa, P. C., et al. (2012). Maternal high-fat diet induces obesity and adrenal and thyroid dysfunction in male rat offspring at weaning. J. Physiol. 590, 5503–5518. doi: 10.1113/jphysiol.2012.240655
Galton, V. A., Hernandez, A., and St. Germain, D. L. (2014). The 5-deiodinases are not essential for the fasting-induced decrease in circulating thyroid hormone levels in male mice: possible roles for the type 3 deiodinase and tissue sequestration of hormone. Endocrinology 155, 3172–3181. doi: 10.1210/en.2013-1884
Golombek, S. G. (2008). Nonthyroidal illness syndrome and euthyroid sick syndrome in intensive care patients. Semin. Perinatol. 32, 413–418. doi: 10.1053/j.semperi.2008.09.010
Iervasi, G., Pingitore, A., Landi, P., Raciti, M., Ripoli, A., Scarlattini, M., et al. (2003). Low-T3 syndrome: a strong prognostic predictor of death in patients with heart disease. Circulation 107, 708–713. doi: 10.1161/01.CIR.0000048124.64204.3F
Jump, D. B., Narayan, P., Towle, H., and Oppenheimer, J. H. (1984). Rapid effects of triiodothyronine on hepatic gene expression. Hybridization analysis of tissue-specific triiodothyronine regulation of mRNA(S14). J. Biol. Chem. 259, 2789–2797.
Kwakkel, J., Chassande, O., van Beeren, H. C., Fliers, E., Wiersinga, W. M., and Boelen, A. (2010). Thyroid hormone receptor α modulates lipopolysaccharide-induced changes in peripheral thyroid hormone metabolism. Endocrinology 151, 1959–1969. doi: 10.1210/en.2009-1049
Livak, K. J., and Schmittgen, T. D. (2001). Analysis of relative gene expression data using real-time quantitative PCR and the 2-ΔΔCT Method. Methods 25, 402–408. doi: 10.1006/meth.2001.1262
Mebis, L., Paletta, D., Debaveye, Y., Ellger, B., Langouche, L., D'Hoore, A., et al. (2009). Expression of thyroid hormone transporters during critical illness. Eur. J. Endocrinol. 161, 243–250. doi: 10.1530/EJE-09-0290
O'Mara, B. A., Dittrich, W., Lauterio, T. J., and St. Germain, D. L. (1993). Pretranslational regulation of type I 5′-deiodinase by thyroid hormones and in fasted and diabetic rats. Endocrinology 133, 1715–1723.
Paxinos, G., and Franklin, K. B. J. (2004). The mouse brain in stereotaxic coordinates. Psychoneuroendocrinology 28, 827–828. doi: 10.1016/S0306-4530(03)00088-X
Scoscia, E., Baglioni, S., Eslami, A., Iervasi, G., Monti, S., and Todisco, T. (2004). Low triiodothyronine (T3) state: a predictor of outcome in respiratory failure? Results of a clinical pilot study. Eur. J. Endocrinol. 151, 557–560. doi: 10.1530/eje.0.1510557
Van den Berghe, G. (2014). Non-thyroidal illness in the ICU: a syndrome with different faces. Thyroid 24, 1456–1465. doi: 10.1089/thy.2014.0201
Van Der Deure, W. M., Peeters, R. P., and Visser, T. J. (2010). Molecular aspects of thyroid hormone transporters, including MCT8, MCT10, and OATPs, and the effects of genetic variation in these transporters. J. Mol. Endocrinol. 44, 1–11. doi: 10.1677/JME-09-0042
van der Spek, A. H., Fliers, E., and Boelen, A. (2017). Thyroid hormone metabolism in innate immune cells. J. Endocrinol. 232, R67–R81. doi: 10.1530/JOE-16-0462
Wajner, S. M., Goemann, I. M., Bueno, A. L., Larsen, P. R., and Maia, A. L. (2011). IL-6 promotes nonthyroidal illness syndrome by blocking thyroxine activation while promoting thyroid hormone inactivation in human cells. J. Clin. Invest. 121, 1834–1845. doi: 10.1172/JCI44678
Warner, M. H., and Beckett, G. J. (2010). Mechanisms behind the non-thyroidal illness syndrome: an update. J. Endocrinol. 205, 1–13. doi: 10.1677/JOE-09-0412
Keywords: non-thyroidal illness syndrome, thyroid hormones, fasting, sepsis, CLP, thyroid hormone metabolism
Citation: Fontes KN, Cabanelas A, Bloise FF, Andrade CBV, Souza LL, Wilieman M, Trevenzoli IH, Agra LC, Silva JD, Bandeira-Melo C, Silva PL, Rocco PRM and Ortiga-Carvalho TM (2017) Differential Regulation of Thyroid Hormone Metabolism Target Genes during Non-thyroidal Illness Syndrome Triggered by Fasting or Sepsis in Adult Mice. Front. Physiol. 8:828. doi: 10.3389/fphys.2017.00828
Received: 08 May 2017; Accepted: 06 October 2017;
Published: 25 October 2017.
Edited by:
Jaye Chin-Dusting, Monash University, AustraliaReviewed by:
Antonio Longo, Università degli Studi di Catania, ItalyJohannes Wolfgang Dietrich, Ruhr University Bochum, Germany
Copyright © 2017 Fontes, Cabanelas, Bloise, Andrade, Souza, Wilieman, Trevenzoli, Agra, Silva, Bandeira-Melo, Silva, Rocco and Ortiga-Carvalho. This is an open-access article distributed under the terms of the Creative Commons Attribution License (CC BY). The use, distribution or reproduction in other forums is permitted, provided the original author(s) or licensor are credited and that the original publication in this journal is cited, in accordance with accepted academic practice. No use, distribution or reproduction is permitted which does not comply with these terms.
*Correspondence: Tania M. Ortiga-Carvalho, dGFuaWFvcnRAYmlvZi51ZnJqLmJy