- Health, Exercise and Active Living Research Centre, Department of Exercise and Sport Science, Manchester Metropolitan University, Manchester, United Kingdom
The disproportionate body mass and leg length of Achondroplasic individuals may affect their net oxygen consumption (O2) and metabolic cost (C) when walking at running compared to those of average stature (controls). The aim of this study was to measure submaximal O2 and C during a range of set walking speeds (SWS; 0.56 – 1.94 m⋅s-1, increment 0.28 m⋅s-1), set running speeds (SRS; 1.67 – 3.33 m⋅s-1, increment 0.28 m⋅s-1) and a self-selected walking speed (SSW). O2 and C was scaled to total body mass (TBM) and fat free mass (FFM) while gait speed was scaled to leg length using Froude’s number (Fr). Achondroplasic O2TBM and O2FFM were on average 29 and 35% greater during SWS (P < 0.05) and 12 and 18% higher during SRS (P < 0.05) than controls, respectively. Achondroplasic CTBM and CFFM were 29 and 33% greater during SWS (P < 0.05) and 12 and 18% greater during SRS (P < 0.05) than controls, respectively. There was no difference in SSW O2TBM or O2FFM between groups (P > 0.05), but CTBM and CFFM at SSW were 23 and 29% higher (P < 0.05) in the Achondroplasic group compared to controls, respectively. O2TBM and O2FFM correlated with Fr for both groups (r = 0.984 – 0.999, P < 0.05). Leg length accounted for the majority of the higher O2TBM and O2FFM in the Achondroplasic group, but further work is required to explain the higher Achondroplasic CTBM and CFFM at all speeds compared to controls.
New and Noteworthy: There is a leftward shift of oxygen consumption scaled to total body mass and fat free mass in Achondroplasic adults when walking and running. This is nullified when talking into account leg length. However, despite these scalars, Achondroplasic individuals have a higher walking and metabolic cost compared to age matched non-Achondroplasic individuals, suggesting biomechanical differences between the groups.
Introduction
Achondroplasia is a condition identified by a disproportionate shorter limb length relative to torso length compared to age matched average statured groups, hereafter referred to as ‘controls’ (Hoover-Fong et al., 2007). The absolute shorter lower limbs of the Achondroplasic population compared to controls is likely to affect functional tasks, such as walking, which in turn is likely to affect the oxygen consumption (O2) and metabolic cost of locomotion (C). For example, increased stride frequency is observed in proportionally shorter statured groups compared to taller individuals during walking and running at the same speeds (Minetti et al., 1994). This in turn leads to a higher O2 in the shorter groups at set walking speeds (Rowland and Green, 1988). When gait speed is increased, such as during incremental walking and running, a positive curvilinear trend of absolute O2 exists in numerous cohorts (Rowland and Green, 1988; Minetti et al., 1994; Schepens et al., 2004; van den Hecke et al., 2007). In proportionally shorter statured groups there is a higher O2 compared to taller groups when walking and running at set speeds (Rowland and Green, 1988; Minetti et al., 1994; Schepens et al., 2004; Ludlow and Weyand, 2015). The differences in O2 between groups of different size can be accounted somewhat by total body mass (TBM) and fat free mass (FFM) (Goran et al., 2000; Browning et al., 2006). Scaling horizontal speed to leg length, as done with Froude’s number (Fr), further nullifies the observed difference in O2 between shorter and taller groups during incremental or steady state exercise (Ferretti et al., 1991; Minetti et al., 1994; Steudel and Beattie, 1995; Steudel-Numbers et al., 2007; Kramer and Sylvester, 2012). The size, and therefore mass, of the torso between Achondroplasic individuals and controls is similar (Owen et al., 1990). But with the leg length of Achondroplasic individuals being shorter than controls (Horton et al., 1978), the ratio of torso-to-leg mass is likely to be greater in the Achondroplasic group, which has been shown to lead to a higher O2 and C (Griffin et al., 2003; Browning et al., 2006; Beekley et al., 2007; Peyrot et al., 2009; McCormick, 2014). Furthermore, the shorter Achondroplasic leg would likely lead to a higher O2 than controls when exercising at the same speed, as observed in other shorter to taller comparisons (Minetti et al., 1994). Therefore, separately scaling O2 to either leg length or body mass during incremental exercise would likely under- and over-predict an Achondroplasic individual’s O2 when compared to controls. To the authors’ knowledge though, there are no data pertaining to the measurement of O2 in Achondroplasic individuals during incremental exercise let alone the scaling of O2 during incremental exercise.
O2 is useful to describe the cardiovascular response during exercise, but C describes the oxygen demand over a given distance (Kramer and Sarton-Miller, 2008). For the same incremental walking that exhibits a positive trend of O2 described above, a U-shaped curve of C exists with clear local minima (Ferretti et al., 1991; Minetti et al., 1994; McCann and Adams, 2002; Kramer and Sarton-Miller, 2008). This minima suggests that respective slower and faster walking speeds are less economic, or have a higher C (i.e., more O2 is required for the given distance). A local minima is observed at different speeds when stride frequency is manipulated, suggesting an optimal stride frequency for different speeds (Minetti et al., 1995). The local minima of C during walking is observed around self-selected walking (SSW) speed (Ralston, 1958), but has not been measured alongside set walking speeds in adults groups of shorter stature (Ferretti et al., 1991; Minetti et al., 1994, 2002). In groups of shorter stature, their higher C can be accounted for by their higher stride frequency (Minetti et al., 1994). The inclusion of a leg length scaler, like Fr, can nullify the effect of stride frequency while SSW may explain some of the expected U-shape curve of walking C in Achondroplasia and controls.
Therefore, the overriding aim of this study was to observe the relationship between O2 and incremental walking and running in adults with Achondroplasia. The primary objectives were to: (1) collect submaximal O2 in Achondroplasic adults and controls at differing absolute and relative (SSW) intensities of walking and running; (2) convert O2 into C values and; (3) attempt to account for any differences in O2 and C by normalizing to body masses and leg length. It was hypothesized that Achondroplasia would have a higher O2 and C at all walking and running speeds, but differences would nullify once scaled appropriately to mass and leg length.
Materials and Methods
Participants
Ten Achondroplasic adults and 17 age matched controls free from any lower limb injury volunteered to participate in the study (Table 1). All gave written informed consent, which was approved by the local committee (Manchester Metropolitan University) and conformed with the Helsinki declaration. All participants attended one laboratory session at Manchester Metropolitan University where anthropometric variables and O2 were collected during incremental exercise.
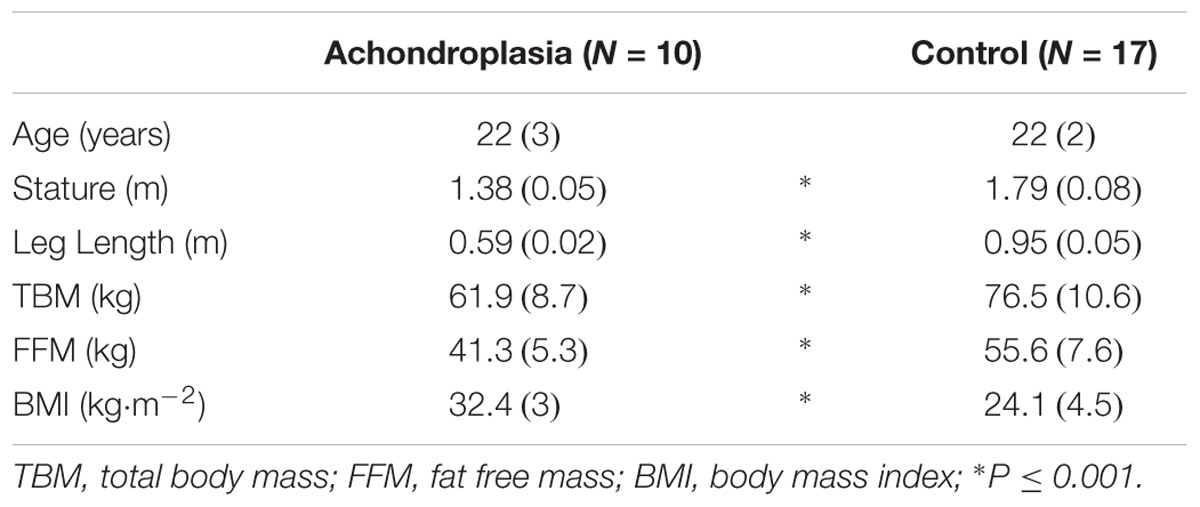
TABLE 1. Anthropometric measures of Achondroplasic adults and controls, values displayed as mean (SD).
Anthropometric Measures
Leg length (m) of all participants was measured as the distance from the anterior iliac spine to the medial malleolus of the ankle while standing. Participants’ TBM (kg) was obtained using electronic scales (SECA 813, CA 91710 Chino, United States) while barefooted and wearing minimal clothing. FFM was obtained using Dual Energy x-ray absorptiometry (DEXA). Following a fasting period of ∼8 h, participants were positioned on a DEXA scanner (Hologic Discovery, Vertec Scientific Ltd, United Kingdom) to measure FFM (kg) of the whole body. Briefly, participants wore a loose-fitting cotton gown and lay supine in a predefined position that ensured enough space was between each arm and the torso and between each leg. The feet were positioned in an internally rotated position and to maintain participant comfort, and reduce the muscle activity during the scan, medical tape (TransporeTM Medical Tape, 3MTM, United States) was wrapped around both feet. A default whole body scan (EF 8.4 lSv) was selected for all trials; the scan emitted duel energy (140/100 kVp) fan-beam x-rays and lasted for ∼7 min. The scanning region was adjusted depending on stature (Achondroplasia ∼160 cm × 65 cm; controls ∼195 cm × 65 cm) with 1.3 cm line spacing and a 0.2 cm point resolution and each participant was exposed to ∼8.4 μSv (Blake et al., 2006). Following all scans manual segmentation of the body was completed using Physician’s View v6.1 software in accordance with Hologic’s recommendations. Glickman et al. (2004) showed that DEXA gives a reliable measurement of TBM (r = 0.940) and FFM (r = 0.890) against computer tomography, while the interrater reliability has been shown to be in excess of 0.998 (Hart et al., 2015).
Speed of Locomotion
Selected walking speed trials were completed by participants conforming to a habitual walking pace around the laboratory (∼40 m). Each participant passed through two timing gates (1 m apart), three times. SSW speeds (m⋅s-1) were calculated and recorded as an average of the three trials and used in O2 assessment where individuals’ SSW intersected absolute speeds described below. O2 collection apparatus (described in the next section) was worn throughout all exercise trials which were conducted on a motorized treadmill (Woodway PPS70). Treadmill speeds were set at 0.56 – 1.94 m⋅s-1 (increment 0.28 m⋅s-1) for walking and 1.67 – 3.33 m⋅s-1 (increment 0.28 m⋅s-1) for running, as described in Ferretti et al. (1991) and Minetti et al. (1994, 2002). All trials were completed at 1% gradient to replicate outdoor conditions (Jones and Doust, 1996) with each stage being 4 min in duration to attain steady state, again replicating Ferretti et al. (1991) and Minetti et al. (1994, 2002). Participants rested for ∼5 min following all walking trials to reduce O2. Where participants could not maintain running speed for the entirety of the 4 min during any walking and running intensity, the stage was omitted from analysis and the testing protocol terminated.
Oxygen Uptake, Metabolic Cost and Scaling
Expired gasses were collected and analyzed using portable breath-by-breath indirect calorimetry (Metamax 3B, Cortex, Leipzig Germany), which was calibrated to the manufacturer’s guidelines prior to testing. The portable indirect calorimeter (weight = 1 kg) and a fitted face mask (Hans Rudolph V2, dead space between 125 – 143 ml) were worn by participants during the exercise bout. Prior to exercise testing, participants lay supine for 5 min so that resting metabolic rate (L⋅min-1) could be measured. Gross O2 for each intensity was recorded with net O2 calculated by subtracting resting metabolic rate from gross O2 as conducted in Minetti et al. (1994, 2002), hereafter net O2 is referred to as ‘O2’. Steady state O2 was determined by a respiratory exchange ratio < 1.0 and by a visual plateau of O2 over the final minute of exercise, with O2 recorded as a rolling average of 6 measurements (every 10 s) for each exercise intensity. C was presented as the amount of O2 required to complete 1 km at each gait speed, given as (L⋅km-1). O2 and C were then normalized to TBM (O2TBM and CTBM, respectively) and FFM (O2FFM and CFFM, respectively). All O2 and C values were presented against absolute walking and running speeds, and against dimensionless Fr, given as: velocity2 (m ⋅ s-1) ÷ leg length (m) ⋅ 9.81(m ⋅ s-1).
Statistical Analysis
All data were collated onto a personal computer (Macintosh, MacBook Pro) and analyzed using SPSS (v22.0, IBM). Data were assumed parametric following Shapiro–Wilk and Levene’s tests. To avoid a Type I error in the comparisons between groups’ O2 and C measures, a repeated measures ANOVA with a between effect was used to identify significant differences. However, only the differences between groups were of interest. Due to the subtle differences in leg length between Achondroplasic and control participants, respectively, interpretation of the interaction between Fr and O2 and C is more difficult. Due to the linear relationship between O2 and Fr presented elsewhere (Kramer and Sarton-Miller, 2008), we correlated the mean of each groups’ Fr and O2 recorded at each walking and running condition using a Pearson’s correlation. Due to the curvilinear relationship between C and speed, the relationship between Fr and C was not inferentially compared. Study power was greater than 0.80 and alpha was set at ≤ 0.05. All results are reported as means (SD).
Results
Participant Anthropometrics
There was no difference in age between groups (P = 0.487, Table 1). Achondroplasia were 23% smaller in stature (P < 0.001), had a 38% shorter leg (P < 0.001), 19% less TBM (P < 0.001), 26% less FFM (P < 0.001) but a 25% greater body mass index (BMI, P < 0.001) than controls (Table 1).
Self-Selected Walking
Achondroplasia were 23% slower than controls at SSW [Achondroplasia, 1.02 (0.13) m⋅s-1; control 1.33 (0.14) m⋅s-1, P < 0.001].
Incremental Exercise
During O2 assessment, both groups completed all walking speeds. However, only 50% of the Achondroplasia group managed to obtain steady state running at 2.50 m⋅s-1 and only 20% maintained steady state running at 2.78 m⋅s-1. Therefore, O2 and C values collected at all walking speeds and at running speeds 1.67 – 2.22 m⋅s-1 were inferentially analyzed (Figures 1, 2).
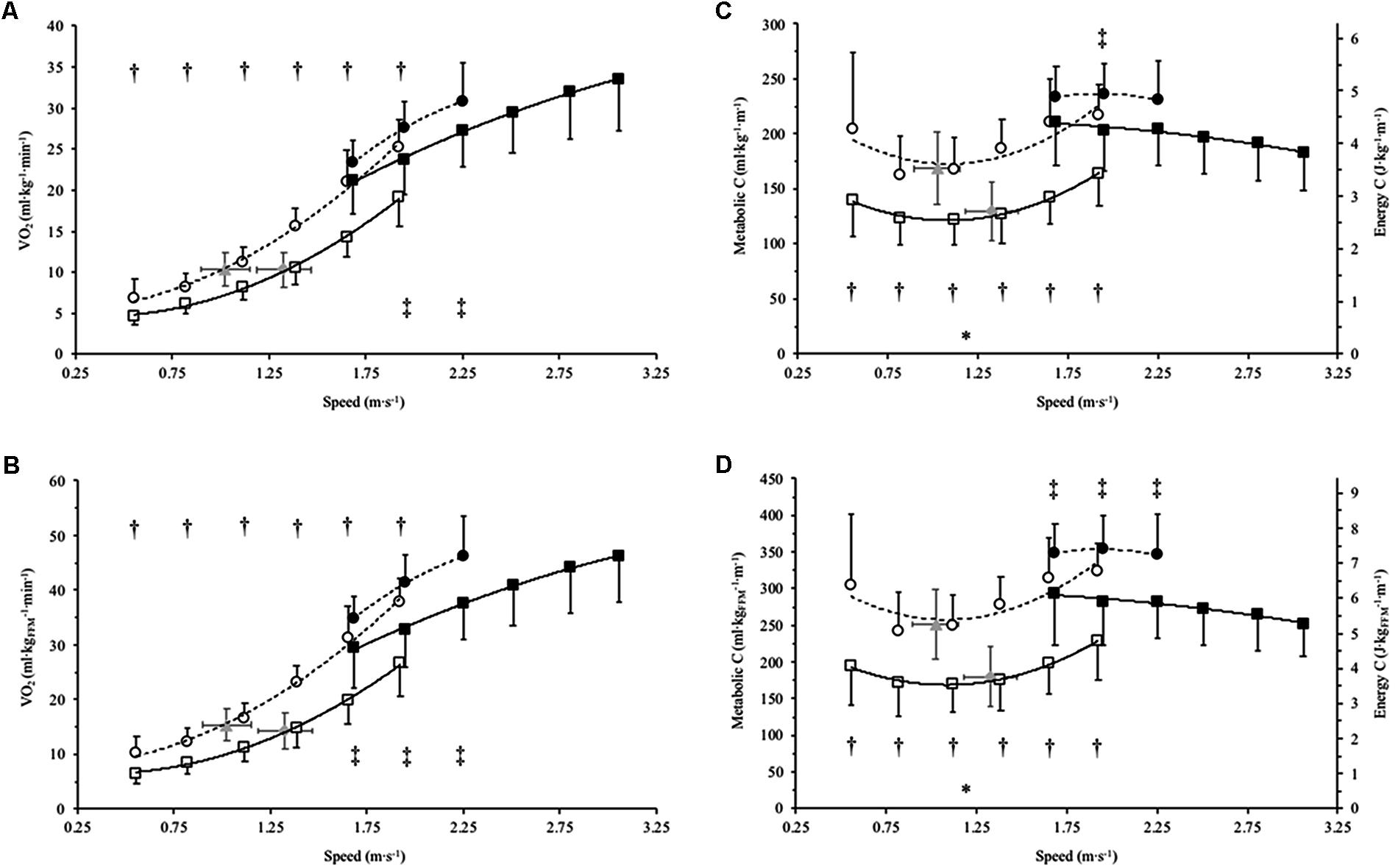
FIGURE 1. Mean SD (error bars) of net oxygen consumption (O2) and metabolic cost (C) for Achondroplasia (◦) and control (□) when walking (open) and running (closed) at absolute and SSW speeds (m⋅s-1); SSW are presented for Achondroplasia () and control (
) respectively. O2 and C are presented relative to total body bass (A and C, respectively) and relative to fat free mass (B and D, respectively); C is also presented as the energy cost by considering 1 ml of O2 = 20.9 J. ∗P < 0.05 at SSW between groups; †P < 0.05 between groups at paired walking speeds; ‡P < 0.05 between groups at paired running speeds. O2 and C and speed relationships are fitted with a 2nd order polynomial, with the broken and continuous lines being the trends for the Achondroplasic and control group, respectively.
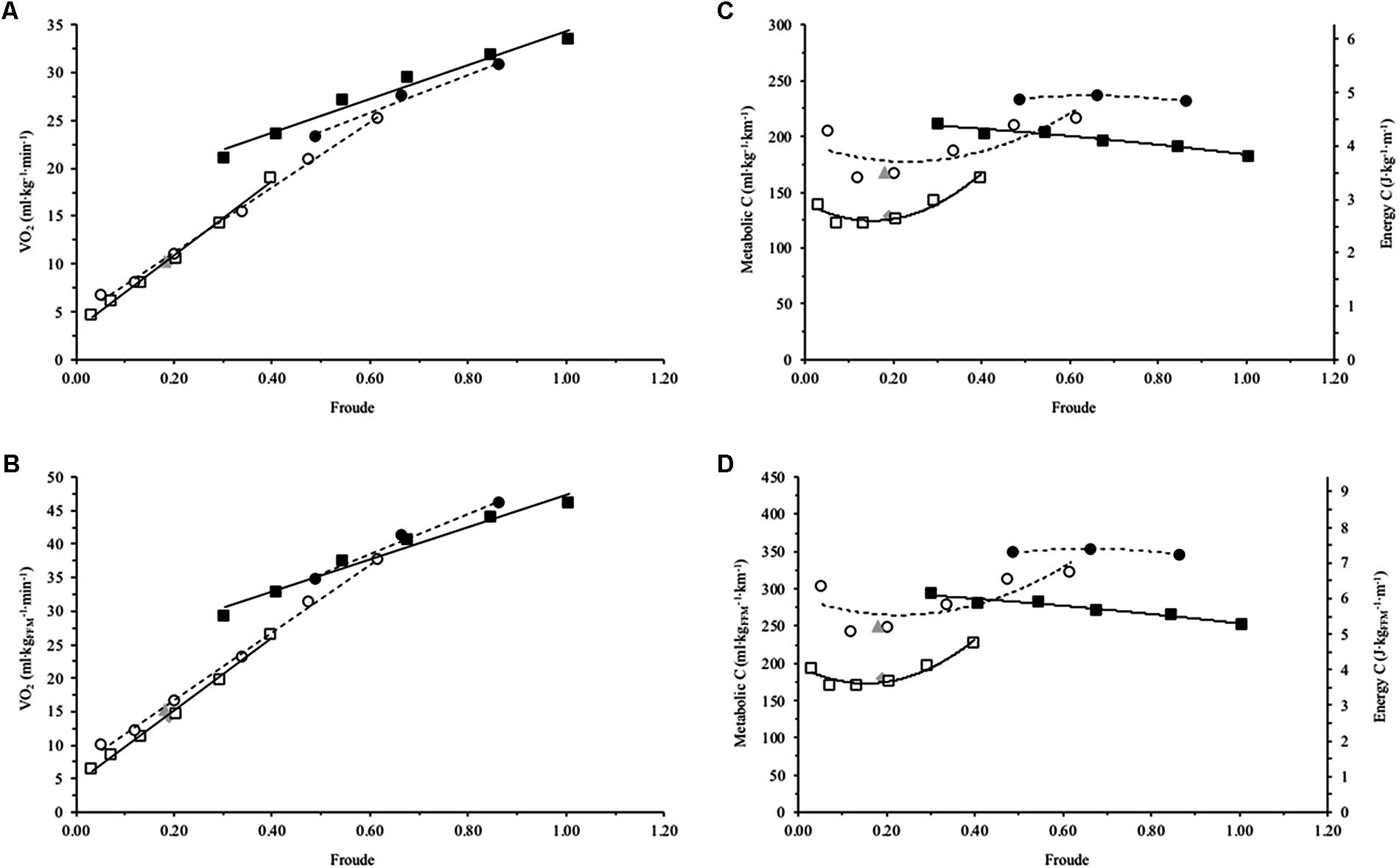
FIGURE 2. Mean net oxygen consumption (O2) and metabolic cost (C) for Achondroplasia (◦) and control (□) when walking (open) and running (closed) at mean Froude’s numbers (Fr); SSW are presented for Achondroplasia () and control (
) respectively. O2 and C are presented relative to total body bass (A and C, respectively) and relative to fat free mass (B and D, respectively); C is also presented as the energy cost by considering 1 ml of O2 = 20.9 J. O2 and Fr relationships are fitted with a linear trend line, while C and Fr relationships are fitted with a 2nd order polynomial with the broken and continuous line being the trends for the Achondroplasic and control group, respectively. SD is omitted for clarity.
Oxygen Consumption
O2TBM were on average 29% greater in Achondroplasia at all absolute walking speeds apart from SSW where no difference was observed (Figure 1A). Similarly, Achondroplasia had an average 35% greater O2FFM at all absolute walking speeds compared to the control group, with no difference being found between groups at SSW (Figure 1B). There was no difference in O2TBM between groups at running speed 1.67 m⋅s-1, but Achondroplasia had a 14 and 12% higher O2TBM than controls at running speeds 1.94 and 2.22 m⋅s-1 (Figure 1A). A higher O2FFM was observed in Achondroplasia for all running speeds (Figure 1B).
Metabolic Cost
On average, Achondroplasia had a 29% higher walking CTBM and 33% higher walking CFFM when compared to controls (Figure 1C). Running CTBM were the same between groups at 1.67 and 2.22 m⋅s-1 (P > 0.05) whereas Achondroplasia had a higher CTBM than controls at 1.94 m⋅s-1 (P < 0.05, Figure 1D). Achondroplasic running CFFM was, on average, 18% higher at all running speeds compared to controls (P < 0.05, Figure 1D).
Comparison of Froude’s Number (Fr)
Strong positive correlations existed for the Achondroplasic group between Fr and O2TBM when walking (r = 0.998, P = 0.001) and running (r = 0.994, P = 0.070), and also for controls when walking (r = 0.997, P < 0.001) and running (r = 0.985, P < 0.001, Figure 2A and Table 2). There were also strong positive correlations for the Achondroplasic group between Fr and O2FFM when walking (r = 0.999, P < 0.001) and running (r = 0.993, P = 0.036) and for the control group when walking (r = 0.997, P < 0.001) and running (r = 0.984, P < 0.001, Figure 2B and Table 2).
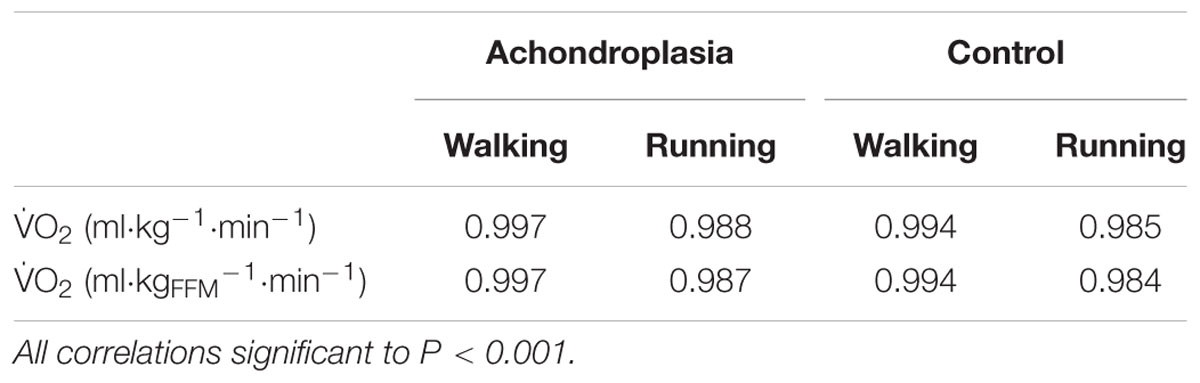
TABLE 2. R2 values for Froude and scaled O2 relationships in Achondroplasic individuals and controls during walking and running.
Discussion
The main aim of this study was to attain O2 and C profiles during incremental walking and running in adult males with Achondroplasia and compare the results to controls. Further to this, we aimed to scale O2 and C to body mass and leg length in both groups. Our hypotheses were partially met in that: (1) Achondroplasia had a greater O2TBM and O2FFM during walking and running compared to controls and had a higher CTBM and CFFM (i.e., a greater O2 for a given distance) at all walking speeds compared to controls; and, (2) leg length can explain some of the difference in O2TBM and O2FFM between groups but not for CTBM and CFFM.
Oxygen Consumption
Achondroplasic O2TBM and O2FFM was higher at every walking and running speed compared to controls other than SSW which were the same between groups. This is similar to previous reports, where shorter statured groups have higher O2TBM than taller counterparts during locomotion (Rowland and Green, 1988; Minetti et al., 1994; Schepens et al., 2004; Ludlow and Weyand, 2015). The higher Achondroplasic O2TBM and O2FFM at set speeds is most likely due to a higher stride frequency of the Achondroplasic group, which is a result of their shorter legs, as Minetti et al. (1994) partially confirmed that higher stride frequency was the cause of higher O2TBM in their African Pygmy group. While stride frequency was not measured in the present study, the data presented by Minetti et al. (1994) would infer some similarities between groups. The Pygmies included in Minetti et al. (1994) were 16 cm taller than the presented Achondroplasic group while the control groups were 1 cm different. With Pygmies being smaller and having a higher stride frequency compared to controls, it is likely that the even smaller Achondroplasic group had a similar, if not higher, stride frequency to the Pygmies.
While this study does not directly measure factors that explain the higher O2 of the Achondroplasic group for a given speed of locomotion, there are a number of mechanisms that could explain these data. Firstly, the probable higher stride frequency of the Achondroplasic group would lead to a greater amount of internal mechanical work being done compared to controls, as observed in other shorter statured groups (Ferretti et al., 1991; Minetti et al., 1994; DeJaeger et al., 2001; Minetti et al., 2002; Schepens et al., 2004; Weyand et al., 2010). A higher internal work not only requires energy to complete the work but would also elicit a greater rate of muscular contraction. Assuming the fiber type distribution is the same between groups, the rate of muscular contraction is likely to alter the force-velocity relationship of the Achondroplasic muscle (Fletcher and MacIntosh, 2017). In this scenario, the Achondroplasic muscle would be producing less force due to the quicker movement of the limbs. Muscle activation could therefore be higher in the Achondroplasic group to recruit the fibers required to maintain locomotion forces, therefore leading to a higher oxygen demand (Mian et al., 2006). However, internal work, force production (relative to gait requirements) and muscle activation have not been measured in Achondroplasic individuals during gait and therefore more work is required in this area to help confirm these theories. To try an account for the assumed higher Achondroplasic stride frequency, and therefore eliminate some of the above, we normalized horizontal speed using leg length in the form of Fr.
Comparisons of Froude’s Number (Fr)
In the current study, Fr could explain the variability of the groups’ O2TBM and O2FFM by as little as 98.7% (Figures 2A,B and Table 2). This matches much of the literature where Fr has been used to compare the relationship between leg length and O2 during locomotion. Ferretti et al. (1991) and Minetti et al. (1994) showed African Pygmy’s O2TBM and Fr trends are similar to controls, with Minetti et al. (2002) also observing similar findings in patients with GHD. The similarity of the O2 and Fr trends between groups suggests that the difference in groups’ O2TBM and O2FFM can be accounted for by the shorter Achondroplasic leg. The similarity in the slopes are not surprising given that O2 correlates well with TBM and FFM (Goran et al., 2000; Weibel and Hoppeler, 2005). It is important to note here though, that although the relationships appear similar between the groups and between mass scalers (Table 2), each mass scaler should not be used to estimate another. For example, in both groups a Fr of ∼0.30 elicits a O2TBM ∼15 ml⋅kg-1⋅min-1, whereas the same Fr elicits a O2FFM ∼20 ml⋅kg-1⋅min-1. While the inclusion of Fr accounted for the difference in O2 by providing similar slopes for both O2TBM and O2FFM between groups respectively, Fr did not explain the difference in C between groups.
Metabolic Cost
While O2 is a useful parameter, it only describes the rate at which O2 is used, not the usage per unit distance. In contrast, C provides O2 usage per unit distance and can be converted into energy cost, assuming an energy equivalent of 20.9 J per 1 ml O2 (Figures 1B,D) and describes the energetic cost per unit mass and distance. Despite accounting for mass and distance traveled, the Achondroplasic group exhibited a higher CTBM and CFFM during walking and running than controls. In addition, CTBM and CFFM at SSW occurred near the local minima of the trend lines for both groups but remained different between groups. The factors that may account for the difference in C between groups are again not investigated directly in this paper, but the available literature does allow insight into potential reasons to why these differences exists.
The torso size of Achondroplasic people and controls is the same when measured as sitting height (Owen et al., 1990). With the Achondroplasic legs being shorter than controls, one would assume that Achondroplasic people have a larger torso to TBM ratio than controls. Where additional mass is added to the torso of individuals exercising on a treadmill, a higher C is observed compared to individuals without additional mass (Griffin et al., 2003; Browning et al., 2006, 2007; Beekley et al., 2007; Peyrot et al., 2009; McCormick, 2014); the converse is observed when body weight is reduced through assisted treadmill running (Grabowski and Kram, 2008). While the present Achondroplasic participants were lighter than the control group, the additional torso mass of the Achondroplasic group would have implications for the contractile properties of their smaller lower leg muscles during stance. Firstly, relative to lower leg muscle mass, a greater vertical ground reaction force (vGRF) would be observed in the Achondroplasic group. The smaller muscle fibers of the Achondroplasic lower limbs (Sims et al., 2017) would therefore do more external work during the braking phase of stance to compensate for the relatively larger vGRF, eliciting a higher C (Pontzer, 2005; Grabowski and Kram, 2008). Furthermore, we recently showed that Achondroplasic adults have a greater co-activation of their antagonists during knee extension (Sims et al., 2017). Although this was during maximal voluntary contraction, it is possible that during the propulsion phase of gait, there is greater co-activation of the hamstrings. Previously, a higher co-activation during gait contributes to negative work which is partly associated with a higher C (Mian et al., 2006). These theories though, require further work to help explain the difference in C between Achondroplasic individuals and controls.
Implications and Future Work
In the present study, the difference in C between groups could possibly be explained by biomechanical differences that have previously been shown to alter or determine C, such as mass distribution and inertial properties (Griffin et al., 2003; Browning et al., 2006, 2007; Beekley et al., 2007; Peyrot et al., 2009; McCormick, 2014), tendon compliance (Arampatzis et al., 2006; Fletcher et al., 2010; Albracht and Arampatzis, 2013) and internal and external work (Ferretti et al., 1991; Minetti et al., 1994, 2002); further work is required in Achondroplasic populations to determine whether these factors play a role in explaining the present results. The current data does show that leg length can explain some of the variance in O2TBM and O2FFM between the presented groups, i.e., longer legs equate to a lower walking and running O2 and in turn suggests a lower walking and running C. Therefore, the lengthening of the Achondroplasic leg would possibly lower C and make some ambulatory based activities of daily living less energetically demanding. Although a viable option, leg lengthening surgery for an Achondroplasic individual is, however, a highly invasive long-term surgical procedure involving prolonged recovery and imposed sedentary behavior (Park et al., 2015). Were other interventions explored to help improve Achondroplasic C during activities of daily living, it is probably that such tasks would be completed more often. It is probable thereafter that their physical activity levels would be improved, as has been observed in an obese population (Weinsier et al., 2000), which is then likely to lower the risk of health complications (Wilmot et al., 2012). Further work should therefore be directed toward identifying exercise interventions that are associated with lowering C in Achondroplasic populations, such as those identified in control populations described above, and then be appropriately addressed in Achondroplasic populations.
Conclusion
This study aimed to observe the relationship between O2 and incremental walking and running speeds in adult males with Achondroplasic. To the author’s knowledge, this is the first study to scale and compare O2 and C during walking and running between Achondroplasic males and controls. The main findings are that (1) Achondroplasic O2TBM and O2FFM are higher at all walking and running speeds compared to controls; (2) O2TBM and O2FFM is not different between groups at SSW; (3) CTBM and CFFM are higher in Achondroplasia than controls at all walking and running speeds, and 4) the inclusion of leg length as a scaler helps explain O2 differences but not C differences between groups. The higher Achondroplasic O2TBM and O2FFM is most likely due to a higher stride frequency, while their higher CTBM and CFFM is likely due to anthropometrical differences compared to controls.
Author Contributions
DS: Lead author, contributed to the inception of the study, participant recruitment and testing, data analysis and interpretation, writing of the main body of work and approval of the article. GO-P and AB: Contributed to the inception of the study, data analysis and interpretation, and revisions of the main body of work and approval of the article. CP: Contributed to data analysis and interpretation and revisions of the main body of work and approval of the article. CM: Contributed to the inception of the study, participant recruitment and testing, data analysis and interpretation, and writing of the main body of work and approval of the article.
Conflict of Interest Statement
The authors declare that the research was conducted in the absence of any commercial or financial relationships that could be construed as a potential conflict of interest.
Acknowledgments
The authors would like to thank the Dwarf Sports Association (Dronfield, United Kingdom. Registered Charity Number 1041961) for their assistance in recruitment and Dream it Believe it Achieve it (Northwich, United Kingdom. Registered Charity Number: 1153116) from which DS received initial postgraduate funding.
References
Albracht, K., and Arampatzis, A. (2013). Exercise-induced changes in triceps surae tendon stiffness and muscle strength affect running economy in humans. Eur. J. Appl. Physiol. 113, 1605–1615. doi: 10.1007/s00421-012-2585-4
Arampatzis, A., De Monte, G., Karamanidis, K., Morey-Klapsing, G., Stafilidis, S., and Brüggemann, G. P. (2006). Influence of the muscle-tendon unit’s mechanical and morphological properties on running economy. J. Exp. Biol. 209, 3345–3357. doi: 10.1242/jeb.02340
Beekley, M. D., Alt, J., Buckley, C. M., Duffey, M., and Crowder, T. A. (2007). Effects of heavy load carriage during constant-speed, simulated, road marching. Mil. Med. 172, 592–595. doi: 10.7205/MILMED.172.6.592
Blake, G. M., Naeem, M., and Boutros, M. (2006). Comparison of effective dose to children and adults from dual X-ray absorptiometry examinations. Bone 38, 935–942. doi: 10.1016/j.bone.2005.11.007
Browning, R. C., Baker, E. A., Herron, J. A., and Kram, R. (2006). Effects of obesity and sex on the energetic cost and preferred speed of walking. J. Appl. Physiol. 100, 390–398. doi: 10.1152/japplphysiol.00767.2005
Browning, R. C., Modica, J. R., Kram, R., and Goswami, A. (2007). The effects of adding mass to the legs on the energetics and biomechanics of walking. Med. Sci. Sports Exerc. 39, 515–525. doi: 10.1249/mss.0b013e31802b3562
DeJaeger, D., Willems, P. A., and Heglund, N. C. (2001). The energy cost of walking in children. Pflügers Arch. 441, 538–543. doi: 10.1007/s004240000443
Ferretti, G., Atchou, G., Grassi, B., Marconi, C., and Cerretelli, P. (1991). Energetics of locomotion in African pygmies. Eur. J. Appl. Physiol. Occup. Physiol. 62, 7–10. doi: 10.1007/BF00635625
Fletcher, J. R., Esau, S. P., and MacIntosh, B. R. (2010). Changes in tendon stiffness and running economy in highly trained distance runners. Eur. J. Appl. Physiol. 110, 1037–1046. doi: 10.1007/s00421-010-1582-8
Fletcher, J. R., and MacIntosh, B. R. (2017). Running economy from a muscle energetics perspective. Front. Physiol. 8:433. doi: 10.3389/fphys.2017.00433
Glickman, S. G., Marn, C. S., Supiano, M. A., and Dengel, D. R. (2004). Validity and reliability of dual-energy X-ray absorptiometry for the assessment of abdominal adiposity. J. Appl. Physiol. 97, 509–514. doi: 10.1152/japplphysiol.01234.2003
Goran, M., Fields, D. A., Hunter, G. R., Herd, S. L., and Weinsier, R. L. (2000). Total body fat does not influence maximal aerobic capacity. Int. J. Obes. 24, 841–848. doi: 10.1038/sj.ijo.0801241
Grabowski, A. M., and Kram, R. (2008). Effects of velocity and weight support on ground reaction forces and metabolic power during running. J. Appl. Biomech. 24, 288–297. doi: 10.1123/jab.24.3.288
Griffin, T. M., Roberts, T. J., and Kram, R. (2003). Metabolic cost of generating muscular force in human walking: insights from load-carrying and speed experiments. J. Appl. Physiol. 95, 172–183. doi: 10.1152/japplphysiol.00944.2002
Hart, N. H., Nimphius, S., Spiteri, T., Cochrane, J. L., and Newton, R. U. (2015). Segmental musculoskeletal examinations using dual-energy X-ray absorptiometry (DXA): positioning and analysis considerations. J. Sports Sci. Med. 14, 620–626.
Hoover-Fong, J. E., McGready, J., Schulze, K. J., Barnes, H., and Scott, C. I. (2007). Weight for age charts for children with achondroplasia. Am. J. Med. Genet. A 143A, 2227–2235. doi: 10.1002/ajmg.a.31873
Horton, W. A., Rotter, J. I., Rimoin, D. L., Scott, C. I., and Hall, J. G. (1978). Standard growth curves for achondroplasia. J. Pediatr. 93, 435–438. doi: 10.1016/S0022-3476(78)81152-4
Jones, A. M., and Doust, J. H. (1996). A 1% treadmill grade most accurately reflects the energetic cost of outdoor running. J. Sports Sci. 14, 321–327. doi: 10.1080/02640419608727717
Kramer, P. A., and Sarton-Miller, I. (2008). The energetics of human walking: is Froude number (Fr) useful for metabolic comparisons? Gait Posture 27, 209–215.
Kramer, P. A., and Sylvester, A. D. (2012). Humans, geometric similarity and the Froude number: is “reasonably close” really close enough? Biol. Open 2, 111–120. doi: 10.1242/bio.20122691
Ludlow, L. W., and Weyand, P. G. (2015). Energy expenditure during level human walking: seeking a simple and accurate predictive solution. J. Appl. Physiol. 120, 481–494. doi: 10.1152/japplphysiol.00864.2015
McCann, D. J., and Adams, W. C. (2002). A dimensional paradigm for identifying the size-independent cost of walking. Med. Sci. Sports Exerc. 34, 1009–1017. doi: 10.1097/00005768-200206000-00017
McCormick, J. J. (2014). The Metabolic Cost of Slow Graded Treadmill Walking with a Weighted Vest in Untrained Females. Doctoral dissertation, The University of New Mexico, Albuquerque, NM.
Mian, O. S., Thom, J. M., Ardigò, L. P., Narici, M. V., and Minetti, A. E. (2006). Metabolic cost, mechanical work, and efficiency during walking in young and older men. Acta Physiol. 186, 127–139. doi: 10.1111/j.1748-1716.2006.01522.x
Minetti, A. E., Capelli, C., Zamparo, P., Di Prampero, P. E., and Saibene, F. (1995). Effects of stride frequency on mechanical power and energy expenditure of walking. Med. Sci. Sports Exerc. 27, 1194–1202. doi: 10.1249/00005768-199508000-00014
Minetti, A. E., Moia, C., Roi, G. S., Susta, D., and Ferretti, G. (2002). Energy cost of walking and running at extreme uphill and downhill slopes. J. Appl. Physiol. 93, 1039–1046. doi: 10.1152/japplphysiol.01177.2001
Minetti, A. E., Saibene, F., Ardigo, L. P., Atchou, G., Schena, F., and Ferretti, G. (1994). Pygmy locomotion. Eur. J. Appl. Physiol. Occup. Physiol. 68, 285–290. doi: 10.1007/BF00571444
Owen, O. E., Smalley, K. J., D’alessio, D. A., Mozzoli, M. A., Knerr, A. N., Kendrick, Z. V., et al. (1990). Resting metabolic rate and body composition of achondroplastic dwarfs. Medicine 69, 56–67. doi: 10.1097/00005792-199001000-00005
Park, K. W., Garcia, R. A., Rejuso, C. A., Choi, J. W., and Song, H. R. (2015). Limb lengthening in patients with Achondroplasia. Yonsei Med. J. 56, 1656–1662. doi: 10.3349/ymj.2015.56.6.1656
Peyrot, N., Thivel, D., Isacco, L., Morin, J. B., Duche, P., and Belli, A. (2009). Do mechanical gait parameters explain the higher metabolic cost of walking in obese adolescents? J. Appl. Physiol. 106, 1763–1770. doi: 10.1152/japplphysiol.91240.2008
Pontzer, H. (2005). A new model predicting locomotor cost from limb length via force production. J. Exp. Biol. 208, 1513–1524. doi: 10.1242/jeb.01549
Ralston, H. J. (1958). Energy-speed relation and optimal speed during level walking. Int. Z. Angew. Physiol. Einschliesslich Arbeitsphysiol. 17, 277–283. doi: 10.1007/BF00698754
Rowland, T. W., and Green, G. M. (1988). Physiological responses to treadmill exercise in females: adult-child differences. Med. Sci. Sports Exerc. 20, 474–478. doi: 10.1249/00005768-198810000-00008
Schepens, B., Bastien, G. J., Heglund, N. C., and Willems, P. A. (2004). Mechanical work and muscular efficiency in walking children. J. Exp. Biol. 207, 587–596. doi: 10.1242/jeb.00793
Sims, D. T., Onambele-Pearson, G. L., Burden, A., Payton, C., and Morse, C. I. (2017). Specific force of the vastus lateralis in adults with Achondroplasia. J. Appl. Physiol. 124, 696–703. doi: 10.1152/japplphysiol.00638.2017
Steudel, K., and Beattie, J. (1995). Does limb length predict the relative energetic cost of locomotion in mammals? J. Zool. 235, 501–514. doi: 10.1111/j.1469-7998.1995.tb01765.x
Steudel-Numbers, K. L., Weaver, T. D., and Wall-Scheffler, C. M. (2007). The evolution of human running: effects of changes in lower-limb length on locomotor economy. J. Hum. Evol. 53, 191–196. doi: 10.1016/j.jhevol.2007.04.001
van den Hecke, A., Malghem, C., Renders, A., Detrembleur, C. S., Palumbo, S., and Lejeune, T. M. (2007). Mechanical work, energetic cost, and gait efficiency in children with cerebral palsy. J. Pediatr. Orthop. 27, 643–647. doi: 10.1097/BPO.0b013e318093f4c3
Weibel, E. R., and Hoppeler, H. (2005). Exercise-induced maximal metabolic rate scales with muscle aerobic capacity. J. Exp. Biol. 208, 1635–1644. doi: 10.1242/jeb.01548
Weinsier, R. L., Hunter, G. R., Zuckerman, P. A., Redden, D. T., Darnell, B. E., Larson, D. E., et al. (2000). Energy expenditure and free-living physical activity in black and white women: comparison before and after weight loss. Am. J. Clin. Nutr. 71, 1138–1146. doi: 10.1093/ajcn/71.5.1138
Weyand, P. G., Smith, B. R., Puyau, M. R., and Butte, N. F. (2010). The mass-specific energy cost of human walking is set by stature. J. Exp. Biol. 213, 3972–3979. doi: 10.1242/jeb.048199
Keywords: Achondroplasia, oxygen consumption, metabolic cost, walking, running
Citation: Sims DT, Onambélé-Pearson GL, Burden A, Payton C and Morse CI (2018) The Oxygen Consumption and Metabolic Cost of Walking and Running in Adults With Achondroplasia. Front. Physiol. 9:410. doi: 10.3389/fphys.2018.00410
Received: 15 January 2018; Accepted: 04 April 2018;
Published: 18 April 2018.
Edited by:
Davide Malatesta, Université de Lausanne, SwitzerlandReviewed by:
Benedicte Schepens, Catholic University of Louvain, BelgiumJared R. Fletcher, University of Calgary, Canada
Copyright © 2018 Sims, Onambélé-Pearson, Burden, Payton and Morse. This is an open-access article distributed under the terms of the Creative Commons Attribution License (CC BY). The use, distribution or reproduction in other forums is permitted, provided the original author(s) and the copyright owner are credited and that the original publication in this journal is cited, in accordance with accepted academic practice. No use, distribution or reproduction is permitted which does not comply with these terms.
*Correspondence: David T. Sims, ZC5zaW1zQG1tdS5hYy51aw==