- 1KIIT School of Biotechnology, KIIT University, Bhubaneswar, India
- 2Sanford Burnham Prebys Medical Discovery Institute at Lake Nona, Orlando, FL, United States
Introduction
The opinion review by Campbell and Dicke questioned if sarcolipin (SLN) is involved in adaptive thermogenesis especially cold adaptation, while agreeing to SLN-mediated heat production (Campbell and Dicke, 2018). Several interesting questions were raised concerning the data implicating SLN in muscle nonshivering thermogenesis (NST). Here, we intend to clarify to the research community that SLN-mediated heat production and adaptive thermogenesis are inseparable. This is because heat production by SLN-SERCA interaction is a biochemical mechanism, whose physiological implication is adaptive thermogenesis. Recent studies from several groups have identified SLN as an important regulator of SERCA pump (Smith et al., 2002; Tupling et al., 2002; Asahi et al., 2003; MacLennan et al., 2003; Vangheluwe et al., 2005; Mall et al., 2006; Morita et al., 2008; Gorski et al., 2013, 2017; Toyoshima et al., 2013; Winther et al., 2013; Gamu et al., 2014, 2015; Montigny et al., 2014; Fajardo et al., 2015). X-ray Crystallographic studies notably from two groups Toyoshima and Nissen have provided the molecular details of SLN binding to SERCA, inside the TM groove (Toyoshima et al., 2013; Winther et al., 2013). Studies using genetically altered mouse models provided convincing data that SLN is important for muscle thermogenesis and metabolism (Bombardier et al., 2013; Sahoo et al., 2013; Maurya et al., 2015; Rowland et al., 2015a; Bal et al., 2017). Although the molecular details of SLN function in muscle physiology continues to evolve, there is critical evidence that SLN is a key regulator muscle NST.
SLN is an Uncoupler of SERCA Pump Leading to Increased Heat Production
SERCA is a Ca2+ ion transport pump in muscle and its activity is regulated by small peptides including phospholamban (PLB-52 aa) and sarcolipin (SLN-31 aa), whose interaction with SERCA alters the dynamics of Ca2+ cycling (Odermatt et al., 1998; Maclennan, 2004; Bhupathy et al., 2007; Traaseth et al., 2008; Periasamy et al., 2017). SLN expression is muscle specific, and its expression level is highly regulated during muscle development and disease states (Vangheluwe et al., 2005; Babu et al., 2007; Pant et al., 2015). PLB is known as an affinity modulator of SERCA pump for Ca2+. Interestingly PLB binds to SERCA only to the Ca2+-free E2-state, whereas SLN can bind to Ca2+-bound SERCA and can remain bound during the Ca2+-transport cycle. The presence of SLN decreases the Vmax of Ca2+-uptake but not the amount of ATP hydrolyzed (Sahoo et al., 2013, 2015; Shaikh et al., 2016). Anthony Lee and his colleagues were the first to suggest that SLN binding to SERCA could promote uncoupling of SERCA-mediated ATP hydrolysis from Ca2+ transport (Smith et al., 2002; Mall et al., 2006). They further showed that Ca2+ accumulation in the vesicles decreased, but the heat released by SERCA increased in the presence of SLN. Based on this, they suggested that SLN binding to SERCA prevents release of Ca2+ into the lumen and promotes slippage of Ca2+ back to the cytosol. The efficiency of SLN uncoupling of SERCA may depend on several factors including a) the ratio of SLN to SERCA; b) cytosolic Ca2+-concentration; c) SR luminal Ca2+-load; d) ATP availability in the local milieu and e) other factors yet to be defined. A recent study by Nowack et al provides strong evidence that SLN-to-SERCA ratio is utilized by pigs to recruit skeletal muscle thermogenesis independent of shivering. Using muscle SR vesicles expressing high SLN, we determined that the efficiency of SLN uncoupling is not 100% but amounts to no more than 30% of SERCA which suggests while SLN uncoupling function does not interfere with normal muscle function since SERCA pump is in excess. Considering the fact that muscle forms more than 40% of the body weight of all endothermic vertebrates, even a small fraction of heat coming from SLN-mediated uncoupling of SERCA function can have a significant impact on thermoregulation.
The Structural Features Involved in SLN Binding and Uncoupling of SERCA
The detailed mechanism of SLN uncoupling is an evolving area of research, but many structural features of SLN/SERCA interaction were identified by protein cross-linking and using SERCA/SLN co-crystals. The X-ray crystals showed that SLN binds to the SERCA TM groove formed by TMs 2, 6, and 9 (Sahoo et al., 2015; Shaikh et al., 2016) however, these studies could not localize the N-terminus of SLN. Previous studies have shown that the C-terminal residues of SLN (Tyr29 and Tyr31), are important for regulation of SERCA (Odermatt et al., 1998) and or SLN localization and interaction with SERCA (Butler et al., 2011). Employing protein chimeras of SLN and PLB, we determined the function of individual domains in uncoupling. We found addition of SLN C-terminus to PLB can increase PLB binding affinity to SERCA but does not promote uncoupling of SERCA (Sahoo et al., 2015). Recent studies from our laboratory suggest that the N-terminus of SLN is important for SLN function (Sahoo et al., 2015). The short N-terminus varies across species and 2ERSTQE sequence in rodents change to 2ERSTRE in rabbit and to 2GINTRE in primates. It contains a conserved Thr5 residue that has been proposed to be a site of Phosphorylation (Bhupathy et al., 2009). Using mutagenesis and chimeric proteins made between SLN and PLB, we have shown that SLN requires its N-terminus for its uncoupling function. Deletion of the N-terminal residues (MERSTQ) caused SLN to constitutively bind to the SERCA groove but did not possess the uncoupling ability. Recently, Autry et al speculated that the negatively charged Glutamate residues are critical for the uncoupling function (Autry et al., 2016). This suggests that mere occupation of the groove is insufficient for uncoupling function but requires the dynamic interaction between the N-terminus, the TM, and the C-terminus of SLN with SERCA for its regulation.
SLN Plays a Role in Both Shivering and Non-Shivering Thermogenesis
The opinion review questioned if SLN is recruited during adaptive thermogenesis. While skeletal muscle has been known to generate heat through shivering, the role of NST in muscle has not been fully appreciated until recently. Studies in birds and mammals suggest that cold adaptation involves upregulation of SR proteins in addition to metabolic remodeling of the muscle (Rowland et al., 2015a; Bal et al., 2016, 2017). Both shivering and NST rely on SR Ca2+-cycling, so invariably recruits SLN and SERCA, therefore SLN-dependent heat production is a component of both shivering and NST mechanisms. It is interesting to point out that during shivering the level of Ca2+-leak from the SR is very high which would recruit myosin-ATPase as well. Further, as discussed earlier high cytosolic Ca2+-concentration reduce SLN interaction/uncoupling of SERCA, so SLN function during shivering might be an important but minor. During prolonged cold adaptation animals switch from shivering to NST mechanisms; which is activated through an increase in cytosolic Ca2+ either via RYR1-mediated Ca2+-leak or Ca2+-entry through channels in the plasma membrane. It is known that cold adapted mice including UCP1 knockout (UCP1-KO) do not shiver when challenged with cold which argues that NST in muscle is primarily responsible for heat production. This idea is further supported by two independent observations made recently. First, mice with surgical ablation of interscapular BAT acclimated to cold significantly upregulate SLN expression in their skeletal muscles (Bal et al., 2016). Similarly, SLN is upregulated in skeletal muscles of UCP1-KO mice, even when exposed to mild cold (Bal et al., 2017). Despite these progress, there are several gaps in our knowledge including how SLN-based NST is activated, whether this involves neurohormonal signaling to trigger Ca2+-entry through plasma membrane channels and/or release from the SR. At this time the major mechanism appears to be RYR1-mediated Ca2+-leak that can trigger muscle heat production during cold adaptation (Dumonteil et al., 1993, 1995; Aydin et al., 2008; Bal et al., 2016).
SLN is Recruited in Diet Induced Adaptive Thermogenesis
Compared to rodents, in large adult mammals including rabbits, dogs and humans, BAT-mediated thermogenesis is negligent and SLN levels are much more abundant (Babu et al., 2007; Rowland et al., 2015b). To mimic SLN expression found in large mammals, we overexpressed SLN using skeletal α-actin gene promoter (Maurya et al., 2015). Sln over-expression (Sln-OE) did not affect muscle function but increased BMR. When pair-fed, Sln-OE mice showed a higher rate of oxygen consumption and lost its fat mass, whereas Sln-KO mice consumed less oxygen and gained fat mass. We further showed that Sln-OE mice were resistant to high fat diet induced obesity and were protected from lipotoxicity in muscle, suggesting higher SLN leads to enhanced energy expenditure through increased mitochondrial biogenesis (Maurya and Periasamy, 2015; Maurya et al., 2015; Sopariwala et al., 2015). A recent study by Maurya et al, has demonstrated that SLN-mediated Ca2+-signaling promote mitochondrial health and muscle metabolism (Maurya et al., 2018). On the other hand, a manuscript published by Butler et al. shows that SLN could not be detected in rodent muscle and they were unable to increase SLN expression through transgenesis in muscle (Butler et al., 2015). We believe that there might be technical issues with SLN detection since this protein is highly hydrophobic and of very small size (~3.5 kDa) and the use different mouse strain could also contribute to this discrepancy since our Sln-OE mouse model is in C57Bl6/j background. It has been shown that cold intolerance of UCP1-KO varies significantly between different mouse strains (Hofmann et al., 2001).
SLN Based Muscle NST Can Compensate for the Loss of BAT Function in Mice
Recent studies also highlighted that in rodents muscle based thermogenesis is recruited in addition to BAT during cold adaptation (Monemdjou et al., 2000; Anunciado-Koza et al., 2008, 2011; Bruton et al., 2010; Shabalina et al., 2010; Rowland et al., 2015a; Bal et al., 2016, 2017; Stanford et al., 2018). Barbara Cannon's group showed that cold adaptation of UCP1-KO mice affect skeletal muscle metabolism (Aydin et al., 2008), but argued that this is primarily due to muscle shivering. Interestingly, several groups have found that Ca2+-handling in the skeletal muscle is altered in cold adapted UCP1-KO mice. We further investigated the importance of muscle NST in UCP1-KO mice by exposing them to different regimens of cold challenge. We found that skeletal muscles from gradually cold adapted UCP1-KO mice upregulated SLN expression, become redder with increased mitochondrial content, succinate dehydrogenase (SDH) activity as opposed to SLN–KO mice. Interestingly, SLN knockout mice adapted to cold showed significant upregulation of UCP1 and higher mitochondrial content in BAT suggesting that SLN-KO mice increasingly rely on BAT-based NST to compensate for decreased muscle thermogenesis.
Using double knockout (DKO) mice for SLN and UCP1, we studied their ability to adapt to cold. These DKO mice were unable to withstand acute cold (4°C) exposure, while UCP1-KO exhibit only 30% cold sensitivity (Rowland et al., 2015a). Further, we do not believe shivering is the sole mechanism for the survival of UCP1-KO mice during cold adaptation. To gain insight into this question we have performed cold adaptation studies on UCP1-KO mice employing gradual decrease (2.0°C/day) and acute decrease (5°C/day) of ambient temperature of mice reared at 29°C. During this cold exposure, the UCP1-KO show visible shivering only for a short period. When acutely shifted from 29 to 16°C and from 16 to 4°C, mice showed visible shivering for up to 2 days but recognizable shivering decreases and stops by 4th day. Based on these studies we suggest that, subsequent to an initial phase of shivering, SLN-based NST becomes the primary mechanism of thermogenesis as continuous shivering will lead to muscle damage.
SLN Might be the Major Determinant of Muscle Thermogenesis in Birds and BAT-Deficient Mammals
From the studies described here, it is evident that SLN plays a very important role in thermogenesis, both in shivering and muscle NST. Campbell and Dicke rightly pointed out that all our studies have been in the mouse model. We agree that we have not studied large animal models and/or avian species. However, we want to emphasize that we have compared SLN protein expression in mice vs. large animals (like rabbit, dog), and humans (Babu et al., 2007; Rowland et al., 2015b). SLN is highly abundant in all the muscles of larger mammals including human and SLN has a better intracellular milieu for its physiological function in oxidative fibers [discussed by Nowack et al. (2017)]. Based on the expression profile, we do not believe that SLN can have a completely different physiological role in larger mammals compared to mice. We were unable to study SLN expression in birds using our antibody as it detects the C-terminal sequence “RSYQY” that is not conserved in birds (KSYQX). However SLN is highly conserved in the TM region, suggesting avian SLN bind to the same groove of SERCA. The C-terminus of avian SLN does not have conserved sequence but the N-terminus has conserved Glutamate at 2nd and 7th position in sparrow, chicken and pigeon important for uncoupling function (Sahoo et al., 2015; Autry et al., 2016). It is to be noted that avian SERCA bears several residue substitutions especially in the cytosolic and luminal loop regions that SLN can potentially interact as shown in Figure 1. Hence, difference in SLN sequence as seen in Figure 1, might provide better anchoring leading to increased function and not loss of function as indicated by the authors. Complete sequence alignment of SERCA is presented as supplemetal data Data Sheet 1. It shows that transmembrane helices are highly conserved, which provides the groove for SLN to bind to SERCA.
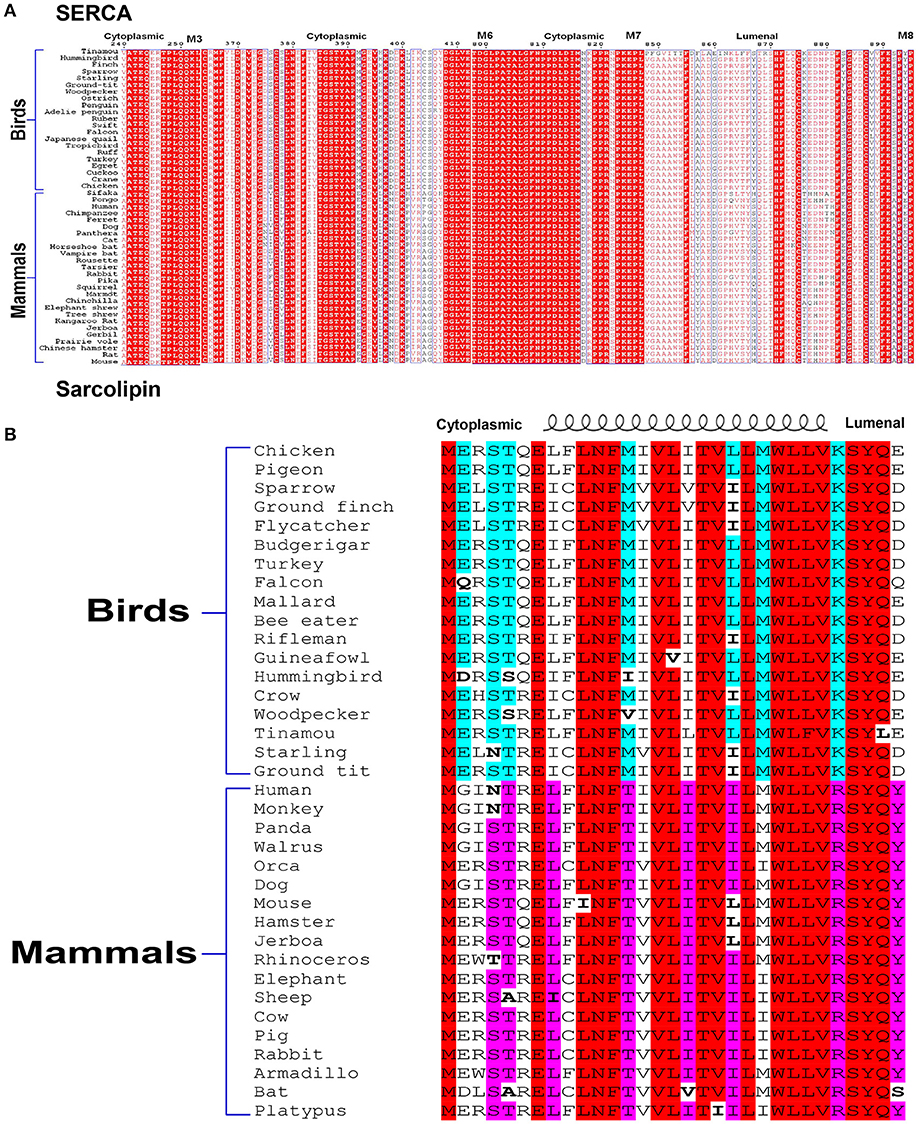
Figure 1. Alignment of SERCA (A) and SLN (B) sequences. Comparison of SERCA sequences from various species of birds and mammals shows that the cytosolic residues that may potentially interact with SLN do not have strict conservation. SLN sequences also show divergence more on the N-terminal cytosolic and C-terminal luminal side. Like other transmembrane proteins, transmembrane residues of both the proteins are more strictly conserved. Therefore, avian SLN may be better suited to uncouple avian SERCA.
Perspectives and Conclusions
While shivering is an important mechanism of heat production in birds and mammals, prolonged shivering would compromise many physiological functions of an animal and even compromise survival in the wild. In addition mild temperature fluctuations do not activate shivering but rely primarily on NST mechanisms. It is of interest and surely significant that SLN is expressed throughout the vertebrate, from fish to man and might be involved in more fundamental role of local heat production. The role of SR Ca2+-cycling in muscle thermogenesis has been documented in the “heater organ”: a modified extraocular muscle found in deep sea fishes (Block and Franzini-Armstrong, 1988; Morrissette et al., 2003) and in malignant hyperthermia, a disease where excessive Ca2+-leaks from RyR coupled with chronic SERCA Ca2+-cycling leading to pathological heat production (Gommans et al., 2002; Rossi and Dirksen, 2006). These examples do suggest that SR can be adapted as a heat producing mechanism. While thermogenesis is often thought as important for whole body temperature regulation, heat production in muscle plays an intrinsic role in peak muscle performance and more importantly survival, especially when faced with a predator–prey situation. True endothermy is seen only in birds and mammals, yet it relies on BAT in only one taxa, the eutherian mammals. The other extant mammals, monotremes and marsupials, lack BAT but are competent endotherms. Birds too lack BAT but manage endothermy at the highest body temperatures of any, 40°C and more. These all must achieve their endothermy by relying on regulatory NST sourced in the skeletal muscle. [reviewed by Nowack et al. (2017)]. Uncoupling mechanisms or futile mechanisms are not entirely new but exist at the expense of increased energy demand. Regulation of SERCA by small peptides including PLB, SLN, myoregulin and others have provided new roles for SERCA pump activity in muscle Physiology (Anderson et al., 2015). We suggest that SLN uncoupling of SERCA activity evolved during vertebrate evolution to support heat production in muscle important for both muscle performance and thermogenesis. Although the molecular details how SLN binding promotes uncoupling of SERCA and recruited under different pathophysiological conditions remain to be solved, the recent studies provide strong evidence that SLN is important for muscle thermogenesis.
Author Contributions
All authors drafted the manuscript together, critically revised the work and approved the final version. NB prepared the figures.
Conflict of Interest Statement
The authors declare that the research was conducted in the absence of any commercial or financial relationships that could be construed as a potential conflict of interest.
Acknowledgments
NB is funded by the Science and Engineering Research Board (SERB), DST, India (ECR/2016/001247) and DBT, India (BT/RLF/Re-entry/41/2014). MP is supported in part by National Institutes of Health Grants R01-HL 088555 and R01 DK098240–01. We thank Sunil Pani for assistance in sequence comparison of SERCA and preparation of the figures for this paper.
Supplementary Material
The Supplementary Material for this article can be found online at: https://www.frontiersin.org/articles/10.3389/fphys.2018.01217/full#supplementary-material
References
Anderson, D. M., Anderson, K. M., Chang, C. L., Makarewich, C. A., Nelson, B. R., et al. (2015). A micropeptide encoded by a putative long noncoding RNA regulates muscle performance. Cell 160, 595–606. doi: 10.1016/j.cell.2015.01.009
Anunciado-Koza, R., Ukropec, J., Koza, R. A., and Kozak, L. P. (2008). Inactivation of UCP1 and the glycerol phosphate cycle synergistically increases energy expenditure to resist diet-induced obesity. J. Biol. Chem. 283, 27688–27697. doi: 10.1074/jbc.M804268200
Anunciado-Koza, R. P., Zhang, J., Ukropec, J., Bajpeyi, S., Koza, R. A., Cefalu, W., et al. (2011). Inactivation of the mitochondrial carrier SLC25A25 (ATP-Mg2+/Pi transporter) reduces physical endurance and metabolic efficiency in mice. J. Biol. Chem. 286, 11659–11671. doi: 10.1074/jbc.M110.203000
Asahi, M., Sugita, Y., Kurzydlowski, K., De Leon, S., Tada, M., Toyoshima, C., et al. (2003). Sarcolipin regulates sarco(endo)plasmic reticulum Ca2+-ATPase (SERCA) by binding to transmembrane helices alone or in association with phospholamban. Proc. Natl. Acad. Sci. U.S.A. 100, 5040–5045. doi: 10.1073/pnas.0330962100
Autry, J. M., Thomas, D. D., and Espinoza-Fonseca, L. M. (2016). Espinoza-fonseca. sarcolipin promotes uncoupling of the SERCA Ca(2+) pump by inducing a structural rearrangement in the energy-transduction domain. Biochemistry 55, 6083–6086. doi: 10.1021/acs.biochem.6b00728
Aydin, J., Shabalina, I. G., Place, N., Reiken, S., Zhang, S., Marks, A., et al. (2008). Nonshivering thermogenesis protects against defective calcium handling in muscle. FASEB J. 22, 3919–3924. doi: 10.1096/fj.08-113712
Babu, G. J., Bhupathy, P., Carnes, C., Billman, G. E., and Periasamy, M. (2007). Differential expression of sarcolipin protein during muscle development and cardiac pathophysiology. J. Mol. Cell Cardiol. 43, 215–222. doi: 10.1016/j.yjmcc.2007.05.009
Bal, N. C., Maurya, S. K., Singh, S., Wehrens, X. H., and Periasamy, M. (2016). Increased reliance on muscle-based thermogenesis upon acute minimization of brown adipose tissue function. J. Biol. Chem. 291, 17247–17257. doi: 10.1074/jbc.M116.728188
Bal, N. C., Singh, S., Reis, F. C., Maurya, S. K., Pani, S., Rowland, L. A., et al. (2017). Both brown adipose tissue and skeletal muscle thermogenesis processes are activated during mild to severe cold adaptation in mice. J. Biol. Chem. 292, 16616–16625. doi: 10.1074/jbc.M117.790451
Bhupathy, P., Babu, G. J., Ito, M., and Periasamy, M. (2009). Threonine-5 at the N-terminus can modulate sarcolipin function in cardiac myocytes. J. Mol. Cell Cardiol. 47, 723–729. doi: 10.1016/j.yjmcc.2009.07.014
Bhupathy, P., Babu, G. J., and Periasamy, M. (2007). Sarcolipin and phospholamban as regulators of cardiac sarcoplasmic reticulum Ca2+ ATPase. J. Mol. Cell. Cardiol. 42, 903–911. doi: 10.1016/j.yjmcc.2007.03.738
Block, B. A., and Franzini-Armstrong, C. (1988). The structure of the membrane systems in a novel muscle cell modified for heat production. J. Cell Biol. 107, 1099–112. doi: 10.1083/jcb.107.3.1099
Bombardier, E., Smith, I. C., Gamu, D., Fajardo, V. A., Vigna, C., Sayer, R. A., et al. (2013). Sarcolipin trumps beta-adrenergic receptor signaling as the favored mechanism for muscle-based diet-induced thermogenesis. FASEB J. 27, 3871–3878. doi: 10.1096/fj.13-230631
Bruton, J. D., Aydin, J., Yamada, T., Shabalina, I. G., Ivarsson, N., Nedergaard, J., et al. (2010). Increased fatigue resistance linked to Ca2+-stimulated mitochondrial biogenesis in muscle fibres of cold-acclimated mice. J. Physiol. 588, 4275–4288. doi: 10.1113/jphysiol.2010.198598
Butler, J., Smyth, N., Broadbridge, R., Council, C. E., Lee, A. G., Arch, J., et al. (2015). The effects of sarcolipin over-expression in mouse skeletal muscle on metabolic activity. Arch. Biochem. Biophys. 569, 26–31. doi: 10.1016/j.abb.2015.01.027
Butler, J., Watson, H. R., Lee, A. G., Schuppe, H. J., and East, J. M. (2011). Retrieval from the ER-golgi intermediate compartment is key to the targeting of c-terminally anchored ER-resident proteins. J. Cell Biochem. 112, 3543–3548. doi: 10.1002/jcb.23281
Campbell, K. L., and Dicke, A. A. (2018). Sarcolipin makes heat, but is it adaptive thermogenesis? Front Physiol. 9:714. doi: 10.3389/fphys.2018.00714
Dumonteil, E., Barre, H., and Meissner, G. (1993). Sarcoplasmic reticulum Ca(2+)-ATPase and ryanodine receptor in cold-acclimated ducklings and thermogenesis. Am. J. Physiol. 265, C507–C513. doi: 10.1152/ajpcell.1993.265.2.C507
Dumonteil, E., Barre, H., and Meissner, G. (1995). Expression of sarcoplasmic reticulum Ca2+ transport proteins in cold-acclimating ducklings. Am. J. Physiol. 269, C955–C960. doi: 10.1152/ajpcell.1995.269.4.C955
Fajardo, V. A., Bombardier, E., Tran, K., Metherel, A. H., Irvine, T., Holloway, G. P., et al. (2015). Tupling. sarcoplasmic reticulum phospholipid fatty acid composition and sarcolipin content in rat skeletal muscle. J. Membr. Biol. 248, 1089–1096. doi: 10.1007/s00232-015-9822-9
Gamu, D., Bombardier, E., Smith, I. C., Fajardo, V. A., and Tupling, A. R. (2014). Sarcolipin provides a novel muscle-based mechanism for adaptive thermogenesis. Exerc. Sport Sci. Rev. 42, 136–142. doi: 10.1249/JES.0000000000000016
Gamu, D., Trinh, A., Bombardier, E., and Tupling, A. R. (2015). Persistence of diet-induced obesity despite access to voluntary activity in mice lacking sarcolipin. Physiol. Rep. 3:e12549. doi: 10.14814/phy2.12549
Gommans, I. M., Vlak, M. H., de Haan, A., and van Engelen, B. G. (2002). Calcium regulation and muscle disease. J. Muscle Res. Cell Motil. 23, 59–63. doi: 10.1023/A:1019984714528
Gorski, P. A., Ceholski, D. K., and Young, H. S. (2017). Structure-function relationship of the SERCA pump and its regulation by phospholamban and sarcolipin. Adv. Exp. Med. Biol. 981, 77–119. doi: 10.1007/978-3-319-55858-5_5
Gorski, P. A., Glaves, J. P., Vangheluwe, P., and Young, H. S. (2013). Sarco(endo)plasmic reticulum calcium ATPase (SERCA) inhibition by sarcolipin is encoded in its luminal tail. J. Biol. Chem. 288, 8456–8467. doi: 10.1074/jbc.M112.446161
Hofmann, W. E., Liu, X., Bearden, C. M., Harper, M. E., and Kozak, L. P. (2001). Effects of genetic background on thermoregulation and fatty acid-induced uncoupling of mitochondria in UCP1-deficient mice. J. Biol. Chem. 276, 12460–12465. doi: 10.1074/jbc.M100466200
Maclennan, D. H. (2004). Interactions of the calcium ATPase with phospholamban and sarcolipin. structure, physiology and pathophysiology. J. Muscle Res. Cell Motil. 25, 600–601.
MacLennan, D. H., Asahi, M., and Tupling, A. R. (2003). The regulation of SERCA-type pumps by phospholamban and sarcolipin. Ann. N. Y. Acad. Sci. 986, 472–480. doi: 10.1111/j.1749-6632.2003.tb07231.x
Mall, S., Broadbridge, R., Harrison, S. L., Gore, M. G., Lee, A. G., and East, J. M. (2006). The presence of sarcolipin results in increased heat production by Ca(2+)-ATPase. J. Biol. Chem. 281, 36597–36602. doi: 10.1074/jbc.M606869200
Maurya, S. K., Bal, N. C., Sopariwala, D. H., Pant, M., Rowland, L. A., Shaikh, S. A., et al. (2015). Sarcolipin is a key determinant of the basal metabolic rate, and its overexpression enhances energy expenditure and resistance against diet-induced obesity. J. Biol. Chem. 290, 10840–10849. doi: 10.1074/jbc.M115.636878
Maurya, S. K., Herrera, J. L., Sahoo, S. K., Reis, F. C. G., Vega, R. B., Kelly, D. P., et al. (2018). Sarcolipin signaling promotes mitochondrial biogenesis and oxidative metabolism in skeletal muscle. Cell Rep. 24, 2919–2931. doi: 10.1016/j.celrep.2018.08.036
Maurya, S. K., and Periasamy, M. (2015). Sarcolipin is a novel regulator of muscle metabolism and obesity. Pharmacol. Res. 102, 270–275. doi: 10.1016/j.phrs.2015.10.020
Monemdjou, S., Hofmann, W. E., Kozak, L. P., and Harper, M. E. (2000). Increased mitochondrial proton leak in skeletal muscle mitochondria of UCP1-deficient mice. Am. J. Physiol. Endocrinol. Metab. 279, E941–E946. doi: 10.1152/ajpendo.2000.279.4.E941
Montigny, C., Decottignies, P., Le Marechal, P., Capy, P., Bublitz, M., Olesen, C., et al. (2014). S-palmitoylation and s-oleoylation of rabbit and pig sarcolipin. J. Biol. Chem. 289, 33850–33861. doi: 10.1074/jbc.M114.590307
Morita, T., Hussain, D., Asahi, M., Tsuda, T., Kurzydlowski, K., Toyoshima, C., et al. (2008). Interaction sites among phospholamban, sarcolipin, and the sarco(endo)plasmic reticulum Ca(2+)-ATPase. Biochem. Biophys. Res. Commun. 369, 188–194. doi: 10.1016/j.bbrc.2007.11.098
Morrissette, J. M., Franck, J. P., and Block, B. A. (2003). Characterization of ryanodine receptor and Ca2+-ATPase isoforms in the thermogenic heater organ of blue marlin (Makaira nigricans). J. Exp. Biol. 206, 805–812. doi: 10.1242/jeb.00158
Nowack, J., Giroud, S., Arnold, W., and Ruf, T. (2017). Muscle non-shivering thermogenesis and its role in the evolution of endothermy. Front. Physiol. 8:889 doi: 10.3389/fphys.2017.00889
Odermatt, A., Becker, S., Khanna, V., Kurzydlowski, K., Leisner, E., Pette, D., et al. (1998). Sarcolipin regulates the activity of SERCA1, the fast-twitch skeletal muscle sarcoplasmic reticulum Ca2+-ATPase. J. Biol. Chem. 273, 12360–12369. doi: 10.1074/jbc.273.20.12360
Pant, M., Bal, N. C., and Periasamy, M. (2015). Cold adaptation overrides developmental regulation of sarcolipin expression in mice skeletal muscle. SOS for muscle-based thermogenesis? J. Exp. Biol. 218, 2321–2325. doi: 10.1242/jeb.119164
Periasamy, M., Maurya, S. K., Sahoo, S. K., Singh, S., Reis, F. C. G., et al. (2017). Role of SERCA pump in muscle thermogenesis and metabolism. Compr. Physiol. 7, 879–890. doi: 10.1002/cphy.c160030
Rossi, A. E., and Dirksen, R. T. (2006). Sarcoplasmic reticulum: the dynamic calcium governor of muscle. Muscle Nerve 33, 715–731. doi: 10.1002/mus.20512
Rowland, L. A., Bal, N. C., Kozak, L. P., and Periasamy, M. (2015a). Uncoupling protein 1 and sarcolipin are required to maintain optimal thermogenesis, and loss of both systems compromises survival of mice under cold stress. J. Biol. Chem. 290, 12282–12289. doi: 10.1074/jbc.M115.637603
Rowland, L. A., Bal, N. C., and Periasamy, M. (2015b). The role of skeletal-muscle-based thermogenic mechanisms in vertebrate endothermy. Biol. Rev. Camb. Philos. Soc. 90, 1279–1297. doi: 10.1111/brv.12157
Sahoo, S. K., Shaikh, S. A., Sopariwala, D. H., Bal, N. C., Bruhn, D. S., Periasamy, M., et al. (2015). The N terminus of sarcolipin plays an important role in uncoupling sarco-endoplasmic reticulum Ca2+-ATPase (SERCA) ATP hydrolysis from Ca2+ Transport. J. Biol. Chem. 290, 14057–14067. doi: 10.1074/jbc.M115.636738
Sahoo, S. K., Shaikh, S. A., Sopariwala, D. H., Bal, N. C., and Periasamy, M. (2013). Sarcolipin protein interaction with sarco(endo)plasmic reticulum Ca2+ ATPase (SERCA) is distinct from phospholamban protein, and only sarcolipin can promote uncoupling of the SERCA pump. J. Biol. Chem. 288, 6881–6889. doi: 10.1074/jbc.M112.436915
Shabalina, I. G., Hoeks, J., Kramarova, T. V., Schrauwen, P., Cannon, B., and Nedergaard, J. (2010). Cold tolerance of UCP1-ablated mice. a skeletal muscle mitochondria switch toward lipid oxidation with marked UCP3 up-regulation not associated with increased basal, fatty acid- or ROS-induced uncoupling or enhanced GDP effects. Biochim. Biophys. Acta 1797, 968–980. doi: 10.1016/j.bbabio.2010.02.033
Shaikh, S. A., Sahoo, S. K., and Periasamy, M. (2016). Phospholamban and sarcolipin: are they functionally redundant or distinct regulators of the Sarco(Endo)plasmic reticulum calcium ATPase? J. Mol. Cell. Cardiol. 91, 81–91. doi: 10.1016/j.yjmcc.2015.12.030
Smith, W. S., Broadbridge, R., East, J. M., and Lee, A. G. (2002). Sarcolipin uncouples hydrolysis of ATP from accumulation of Ca2+ by the Ca2+-ATPase of skeletal-muscle sarcoplasmic reticulum. Biochem. J. 361, 277–286. doi: 10.1042/bj3610277
Sopariwala, D. H., Pant, M., Shaikh, S. A., Goonasekera, S. A., Molkentin, J. D., Periasamy, M., et al. (2015). Sarcolipin overexpression improves muscle energetics and reduces fatigue. J. Appl. Physiol. 118, 1050–1058. doi: 10.1152/japplphysiol.01066.2014
Stanford, K. I., Lynes, M. D., Takahashi, H., Baer, L. A., Arts, P. J., Tseng, Y. H., et al. (2018). 12,13-diHOME. An exercise-induced lipokine that increases skeletal muscle fatty acid uptake. Cell Metab. 27, 1111–1120.e3. doi: 10.1016/j.cmet.2018.03.020
Toyoshima, C., Iwasawa, S., Ogawa, H., Hirata, A., Tsueda, J., and Inesi, G. (2013). Crystal structures of the calcium pump and sarcolipin in the Mg2+-bound E1 state. Nature 495, 260–264. doi: 10.1038/nature11899
Traaseth, N. J., Ha, K. N., Verardi, R., Shi, L., Buffy, J. J., Masterson, L. R., et al. (2008). Structural and dynamic basis of phospholamban and sarcolipin inhibition of Ca(2+)-ATPase. Biochemistry 47, 3–13. doi: 10.1021/bi701668v
Tupling, A. R., Asahi, M., and MacLennan, D. H. (2002). Sarcolipin overexpression in rat slow twitch muscle inhibits sarcoplasmic reticulum Ca2+ uptake and impairs contractile function. J. Biol. Chem. 277, 44740–44746. doi: 10.1074/jbc.M206171200
Vangheluwe, P., Schuermans, M., Zador, E., Waelkens, E., Raeymaekers, L., and Wuytack, F. (2005). Sarcolipin and phospholamban mRNA and protein expression in cardiac and skeletal muscle of different species. Biochem. J. 389, 151–159. doi: 10.1042/BJ20050068
Keywords: sarcolipin (SLN), nonshivering thermogenesis (NST), skeletal muscle, SERCA (sarco(endo)plasmic reticulum calcium ATPase), calcium transport across biological mem branes
Citation: Bal NC, Sahoo SK, Maurya SK and Periasamy M (2018) The Role of Sarcolipin in Muscle Non-shivering Thermogenesis. Front. Physiol. 9:1217. doi: 10.3389/fphys.2018.01217
Received: 22 June 2018; Accepted: 13 August 2018;
Published: 27 September 2018.
Edited by:
Paras Kumar Mishra, University of Nebraska Medical Center, United StatesReviewed by:
Claire Joanne Stocker, University of Buckingham, United KingdomCopyright © 2018 Bal, Sahoo, Maurya and Periasamy. This is an open-access article distributed under the terms of the Creative Commons Attribution License (CC BY). The use, distribution or reproduction in other forums is permitted, provided the original author(s) and the copyright owner(s) are credited and that the original publication in this journal is cited, in accordance with accepted academic practice. No use, distribution or reproduction is permitted which does not comply with these terms.
*Correspondence: Naresh C. Bal, bmFyZXNoLmJhbEBraWl0YmlvdGVjaC5hYy5pbg==
Muthu Periasamy, bXBlcmlhc2FteUBzYnBkaXNjb3Zlcnkub3Jn