- Stress Biology Research Laboratory, Department of Zoology, Savitribai Phule Pune University, Pune, India
The year 2002 marked the tercentenary of Antonie van Leeuwenhoek’s discovery of desiccation tolerance in animals. This remarkable phenomenon to sustain ‘life’ in the absence of water can be revived upon return of hydrating conditions. Today, coping with climate change-related factors, especially temperature-humidity imbalance, is a global challenge. Under such adverse circumstances, desiccation tolerance remains a prime mechanism of several plants and a few animals to escape the hostile consequences of fluctuating hydroperiodicity patterns in their habitats. Among small animals, insects have demonstrated impressive resilience to dehydration and thrive under physiological water deficits without compromising on revival and survival upon rehydration. The focus of this review is to compile research insights on insect desiccation tolerance, gathered over the past several decades from numerous laboratories worldwide working on different insect groups. We provide a comparative overview of species-specific behavioral changes, adjustments in physiological biochemistry and cellular and molecular mechanisms as few of the noteworthy desiccation-responsive survival kits in insects. Finally, we highlight the role of insects as potential mechanistic models in tracking global warming which will form the basis for translational research to mitigate periods of climatic uncertainty predicted for the future.
Introduction
Long-term drought conditions leading to physiological water deficits are a threat to the survival and distribution of all organisms. To this notion, what comes as a delightful surprise is the demonstration of water loss mediated resurrection of apparently ‘dead’ organisms (Keilin, 1959). Such organisms have a remarkable ability of desiccation tolerance whereby they sustain cellular integrity in the desiccated form by activating unique physiological mechanisms (Clegg, 2001). Interestingly, this phase is reversible upon rehydration causing the revival and resumption of active metabolism. At present, global concerns include the challenges associated in coping with climatic stressors, especially the fallout due to humidity-temperature imbalance (Bellard et al., 2012; Boggs, 2016). Under the global sustainable development agendas1, research priorities on “life on land” (item#15) and “climate action” (item#13) have warranted attention. Among small animals, insects have proved to be reliable biological systems to anticipate cause-and-effect relations of climate change stressors (Addo-Bediako et al., 2001; Hoffmann and Todgham, 2010).
This mini-review highlights the notable adaptive mechanisms employed by insects to evade dehydration bouts in their habitats. There have been a few reviews on similar topics (Watanabe, 2006; Cornette and Kikawada, 2011; Chown et al., 2011; Sogame and Kikawada, 2017); however, no recent competent review has emphasized on the profound diversity of hygropreference and associated strategies in insects. Most importantly, we discuss the desiccation tolerance profiles in insects irrespective of whether they possess a lower tolerance potential or are anhydrobiotic with a tolerance for severe water loss. These aspects have not been fully appreciated in the past, therefore, we aim to compile the diverse range of insect desiccation stress responses from a general perspective. Lastly, the present evaluation is by no means an exhaustive list of all desiccation tolerant insects; nonetheless, many case studies have been gathered within the ambit of insect water stress management.
Dry but Not Dead
The documented history of desiccation tolerance dates back to 370 BC when Theophrastus described conditions necessary to store ‘dry seeds alive’ (Leprince and Buitink, 2015). Later, Antonie van Leeuwenhoek described his amazement over the dry dust containing ‘tiny dry animalcules’ that came to life within a few hours after being rehydrated with water (Keilin, 1959). Little did Leeuwenhoek know that his meticulous observations would form the basis of the latent phases of life. To describe this phenomenon, Giard (1894) coined the term ‘anhydrobiosis,’ an extreme form of desiccation tolerance which in Greek implies ‘life without water.’ ‘Desiccation avoidance’ and ‘desiccation tolerance’ are distinguishable phenomena (Pallarés et al., 2016). The former refers to the maintenance of water uptake and/or minimization of body water loss (e.g., Folsomia candida, Collembola: Isotomidae) while the latter includes organisms that can afford loss of water and sustain a dry form without compromising on revival upon rehydration (e.g., all anhydrobiotes). The threshold for tolerance of water loss is highly species-specific and striking differences in desiccation tolerance strategies and traits in congeneric insect species have been linked with their geographic locations and the frequency and duration of drought exposure (Marron et al., 2003; Strachan et al., 2015). However, this is not true in all insects such as few heliconiine butterflies (Lepidoptera: Nymphalidae) (Mazer and Appe, 2001). Contrary to the rationale that desert insects can withstand higher water loss than mesic species, the aquatic beetle, Peltodytes muticus (Coleoptera: Haliplidae) is known for its highest tolerance in comparison to the desert spider beetle, Mezium affine (Coleoptera: Ptinidae) (Pallarés et al., 2016). Closely related Drosophila species (Diptera: Drosophilidae) have evolved different water balance mechanisms as demonstrated in D. nepalensis vs. D. takahashii and D. immigrans vs. D. nasuta (Parkash et al., 2012a,b).
Each organism may have its specific threshold longevity in the dry state; however, desiccation tolerance by no means confers ‘immortality’ or infinite survival but is rather influenced by the mode of desiccation, storage temperature, humidity and oxygen content (Tunnacliffe and Lapinski, 2003; Suemoto et al., 2004; Thorat and Nath, 2016). Depending on these factors, organisms display varying longevities in the desiccated form that may range from 1 day to several years (Figure 1). Notwithstanding these variations and by virtue of qualitative considerations, all such organisms have been considered as desiccation tolerant (Watanabe, 2006). To the best of our knowledge, a numerical method devised for grouping prokaryotes based on their degree of desiccation tolerance, was the first attempt made by Hernández et al. (2009). A recent study in animals proposed the ‘desiccation tolerance index’ (DTi) as a quantitative measure of endurance to desiccation stress (Thorat and Nath, 2016). This mathematical tool is based on the desiccation tolerance in nine oriental Chironomus species (Diptera: Chironomidae) which indicate varying degrees of the tolerance threshold based on their ecological habitats (Figure 2).
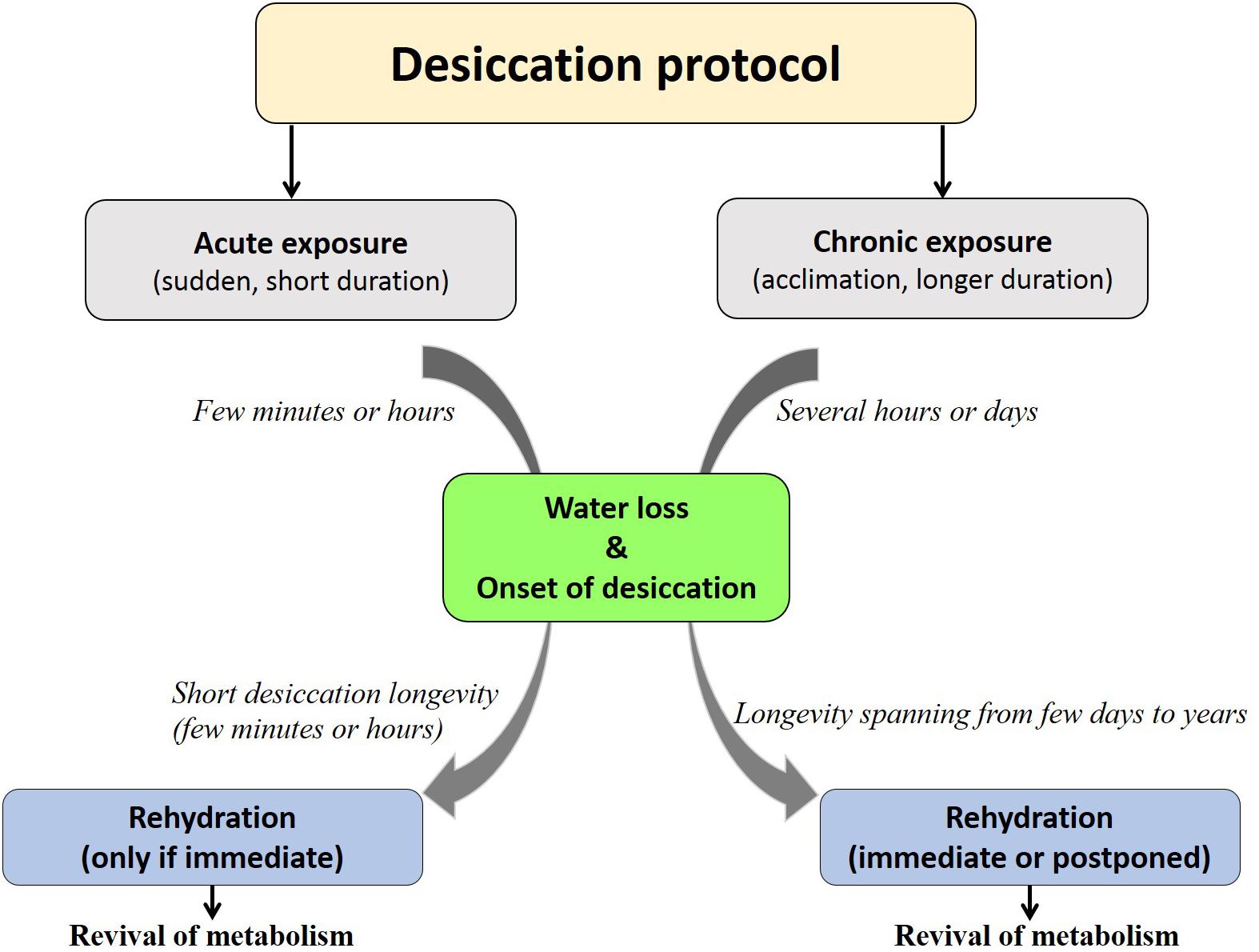
Figure 1. Fate of organisms under desiccation exposure. Acute desiccation permits shorter desiccation longevity (partial desiccation tolerance as seen in stenohygrobiotes) while chronic desiccation facilitates strategic competence to achieve higher desiccation longevity (extreme desiccation tolerance as seen in anhydrobiotes/euryhygrobiotes).
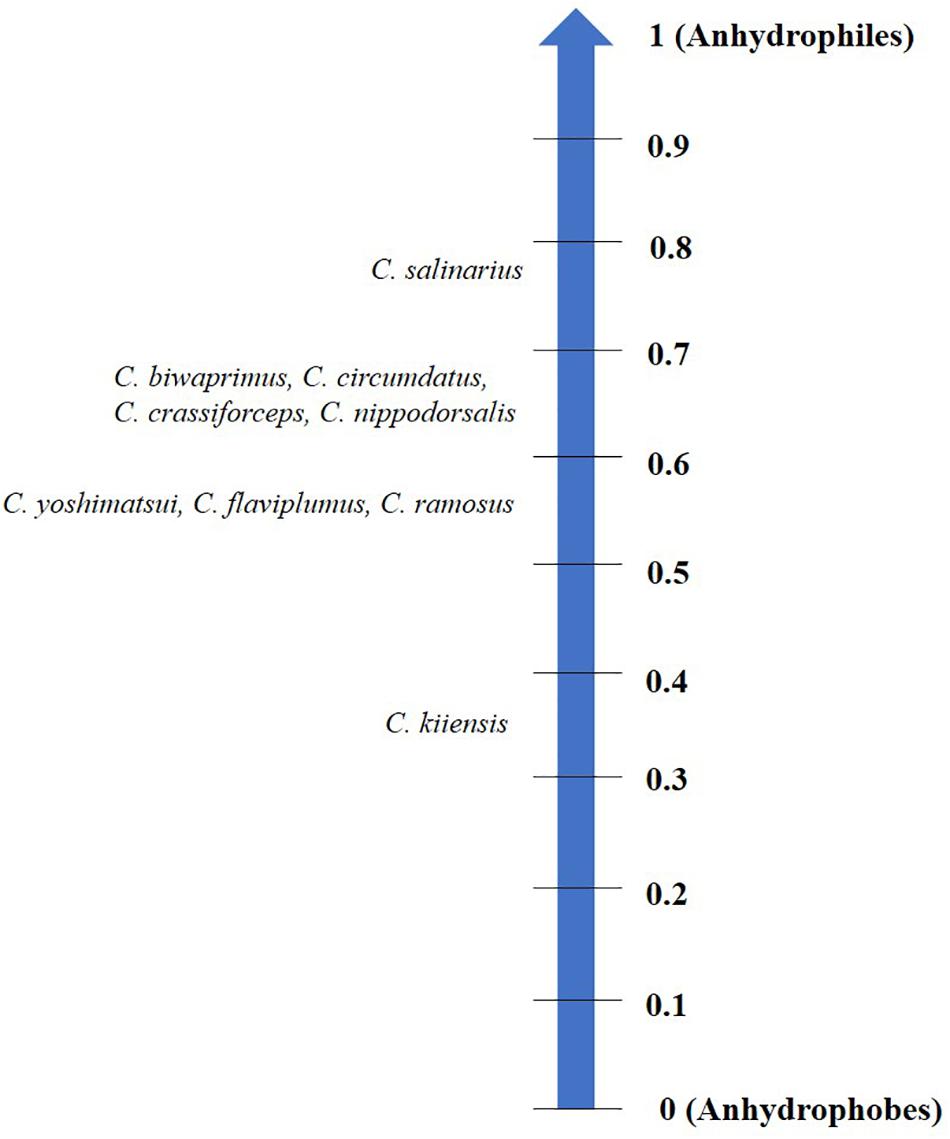
Figure 2. Desiccation tolerance index (DTi) scale categorizing nine oriental chironomids based on their threshold to tolerate water loss. Anhydrophobes lack desiccation tolerance while anhydrophiles are highly desiccation tolerant.
Anhydrobiosis: an Extreme Case of Desiccation Tolerance
Anhydrobiosis is characterized by extreme body water loss, generally over 95% (Benoit, 2010; Sogame and Kikawada, 2017). Thus, anhydrobiosis refers to complete desiccation, unlike desiccation tolerance, which refers to partial dehydration. In this context, we would like to introduce the term, ‘euryhygrobiote’ for such organisms that show a wide range of dehydration tolerance with a high anhydrobiotic potential. Conversely, we coin the term ‘stenohygrobiote’ for organisms that have a narrow dehydration tolerance range and can bear water loss only up to a certain limit. The extremophilic midges (Diptera: Chironomidae), Polypedilum vanderplanki (Hinton, 1951) and Belgica antarctica (Lopez-Martinez et al., 2009) are valuable models in understanding the gamut of molecular and biochemical signatures that render them anhydrobiotic. Anhydrobiotes can also be referred to as ‘anhydrophiles’ in comparison to ‘anhydrophobes,’ which lack desiccation tolerance. P. vanderplanki, the largest known anhydrobiotic eukaryote, endures water content as low as 3% through a gradual and optimized desiccation regime to sustain the dry state for 17 years until rehydration (Cornette and Kikawada, 2011). A new related species, Polypedilum pembai sp.n. also possesses anhydrobiotic potential and shares a few overlapping mechanisms with P. vanderplanki (Cornette et al., 2017). Recent work from our laboratory has demonstrated that the tropical midge, Chironomus ramosus and the fruit fly, Drosophila melanogaster possess a lower ability to tolerate water loss in comparison to the anhydrobiotic midges (Thorat et al., 2017) and are therefore stenohygrobiotic. Among invertebrates, other well-studied non-insect anhydrobiotes include brine shrimps, tardigrades, rotifers and nematodes (Tunnacliffe and Lapinski, 2003; Rebecchi, 2013). Interestingly, desiccation tolerance also confers cross tolerance to a variety of other stressors through multiple physiological defenses including physical and cellular protection via antioxidants, compatible solutes, proteins and DNA repair (Gusev et al., 2010b).
Desiccation Tolerance Strategies in Insects
Environmental cues cause dormancy in insects, a phenomenon triggered by climatic signals including humidity, photoperiod, temperature, etc. (Diniz et al., 2017). Dormancy is further classified into diapause and quiescence. While diapause is a pre-programmed predictive strategy, quiescence is an immediate response to adverse environmental conditions (Denlinger, 1986; Danks, 2002). Aestivation, a form of consequential dormancy is the reason behind the aridity survival strategies of several insect species (Colvin, 1996; Benoit and Denlinger, 2007; North and Godfray, 2018). Anhydrobiosis (ametabolism) is an adaptation against physiological water stress, whereas dormancy is characterized by interrupted or reduced metabolic and hormonal activities (hypometabolism) in response to environmental cues (Watanabe, 2006).
While external milieu trigger desiccation stress responses, interoception is central to tolerance, survival and propagation of species. Below, we discuss a few of the striking and widely established strategies that constitute part of the desiccation tolerance approach of insects (Table 1).
Behavior and Ecology
Hygrosensing abilities and behavioral responses suggest an evolutionary strategy for coping with water loss in insects (Chown et al., 2011). For instance, cockroaches show aggregation in order to control the water loss rate per individual (Dambach and Goehlen, 1999). Similar observations in Chironomus larvae indicate a ‘clumping’ behavior, forming a single bunch to reduce evaporative body water loss (Thorat and Nath, unpublished). Some beetles exhibit bimodal activity patterns in order to escape the hottest hours of the day whereas others display fog-basking for moisture absorption from the surroundings (Bedick et al., 2006; Chown et al., 2011). Other striking evidences for aridity protection, come from niche construction behaviors such as the housing nests of chironomid midges, termite nests, domiciles of some thrips and insect galls (Kikawada et al., 2005; Gilberta, 2014; Zukowski and Su, 2017; Thorat and Nath, 2018). The cuticle is the first portal of water loss in insects and the differential desiccation tolerance patterns in C. ramosus vs. D. melanogaster and P. vanderplanki vs. Paraborniella tonnoiri (Diptera: Chironomidae) have been attributed to striking differences in their cuticular thickness (Nakahara et al., 2008; Thorat et al., 2017). Furthermore, in some insects, restructuring of the cuticle and morphological changes in spiracular features are crucial to minimize water loss. Such restructuring mechanisms are important because water is mainly lost passively and/or actively throughout spiracular respiration and cuticular transpiration (Hadley, 1994; Benoit and Denlinger, 2007; Benoit, 2010; Bazinet et al., 2010; Wadaka et al., 2016; Hidalgo et al., 2018; Ferveur et al., 2018). Other behavioral traits for desiccation protection such as the arrangement of egg laying (layering and density) in the nymphalid butterfly, Chlosyne lacinia (Lepidoptera: Nymphalidae), increases desiccation survival chances of eggs (Clark and Faeth, 1998).
Development and Hormonal Regulation
Our current understanding on the desiccation-mediated developmental consequences in insects is rather fragmented. In the case of the oriental fruit fly, Bactrocera dorsalis (Diptera: Tephritidae), desiccation does not exert significant effects on the average eclosion time (Xie and Zhang, 2007). In C. ramosus and D. melanogaster, modulations in 20-hydroxyecdysone affect recovery patterns and are linked with the desiccation-mediated delay in metamorphosis (Thorat and Nath, 2015; Thorat et al., 2016b). Interestingly, in D. melanogaster, despite the developmental heterochrony, the overall duration of postembryonic development of the life cycle remains almost unaltered. This is reminiscent of Waddington’s ‘canalization’ as an adaptive buffer to adjust their life histories around optimal seasonal conditions (Thorat et al., 2016b). Life cycle and aging in desiccation tolerant animals has been categorized into three hypothetical models, the first, known as the ‘Sleeping Beauty’ model, implies that organisms totally disregard the entire time spent in the dry state, the second model considers that organisms register partial discount of the time spent in the dry state and the third model, whereby organisms record the exact time spent in the dry state, exhibiting non-extended longevity. D. melanogaster follows the Sleeping Beauty model similar to the non-insect anhydrobiotic tardigrade, Milnesium tardigradum (Schill, 2010; Thorat et al., 2016b). Variations in insect hormonal titres are key players in synchronizing developmental changes in order to handle ecological ramifications of stressful environments such as hypoxia, high temperatures, starvation and sleep deprivation; however, investigations in the context of desiccation stress are warranted.
Physiological Biochemistry
A longstanding biochemical adjustment of survival under dry conditions, is the ability of desiccation-responsive synthesis and accumulation of biomolecules including trehalose, mannitol, glycerol, Heat-Shock (HS) and Late Embryonic Abundant (LEA) proteins, proline, glycine-betaine, gamma aminobutyric acid, alanine, and glucosamine (Crowe and Madin, 1974; Tunnacliffe and Lapinski, 2003; Yoder et al., 2006; Kikawada et al., 2008; Philip et al., 2008; Benoit et al., 2009; Mitsumasu et al., 2010; Thorat et al., 2012; Hidalgo et al., 2014; Shukla et al., 2015, 2016, 2018; Yoshida et al., 2016; Thorat et al., 2017; Mazin et al., 2018). These compatible solutes not only offer protection to the drying tissues but also trigger various signaling responses during recovery. Although trehalose was considered indispensable for desiccation tolerance, recent compelling evidences have affirmed that trehalose accumulation may be completely absent in some organisms in which the desiccation protective role is taken up by other biomolecules (Tunnacliffe et al., 2005; Thorat et al., 2017). Differential physiological mechanisms involving carbohydrates, lipids and proteins are known to contribute to the invasive potential of three related Ceratitis fly species (Diptera: Tephritidae) under episodic dehydration (Weldon et al., 2016). Osmoregulatory mechanisms in lepidopteran species have demonstrated the homeostatic control to readjust hemolymph osmolality triggered by body water loss (Willmer, 1980). Interestingly, eggs of Acanthoscelides obtectus (Coleoptera: Bruchidae) show water loss coping mechanisms that enhance egg tolerance and survival (Biemont et al., 1981). In the case of the flea beetle, Longitarsus bethae (Chrysomelidae: Alticinae), while low relative humidity has no influence on oviposition, aridity beyond a critical point is lethal for the eggs (Simelane, 2007). In contrast, egg desiccation did not affect embryo survival in xeric and mesic populations of the tobacco hawk moth, Manduca sexta (Lepidoptera: Sphingidae) (Potter and Woods, 2012).
Antioxidant Defense
Ionic imbalance and changes in osmolarity as a result of cellular water loss leads to the generation of reactive oxygen species (ROS) that are known to damage cellular macromolecules (Alpert, 2005; Benoit and Lopez-Martinez, 2012). Rebecchi (2013) has provided an excellent overview of the whole repertoire of antioxidant defenses under desiccation-responsive oxidative stress management in animals. P. vanderplanki shows the presence of both mitochondrial and cytosolic/extracellular superoxide dismutases (SODs) and abundant glutathione peroxidase and mitochondrial thioredoxin (Cornette et al., 2016; Nesmelov et al., 2016). Furthermore, genes that encode core components of enzymatic antioxidants in P. nubifer are similar to those in insects. However, in P. vanderplanki several groups of antioxidant genes have expanded (Gusev et al., 2014). In contrast, SOD serves as the major antioxidant in B. antarctica (Benoit and Lopez-Martinez, 2012). Recently, the role of unconventional antioxidant molecules such as trehalose, proline, polyamines and polyoils has gained attention (Goyal et al., 2004; Schill et al., 2009; Benoit and Lopez-Martinez, 2012). Trehalose, in particular, has been confirmed for its ROS-scavenging ability in SOD-deficient yeast cells and plants (Kranner and Birtič, 2005; França et al., 2007). Using the advantage of molecular genetic tools in Drosophila and a simple, non-invasive method of whole larval real-time imaging, Thorat et al. (2016a) have demonstrated for the first time that during desiccation, trehalose in collaboration with SOD is involved in the maintenance of redox homeostasis in insects.
Molecular and Evolutionary Biology
Cellular decline in water levels serves as a cue to elicit defensive-responses of molecular indicators. Among the molecular responses mediated via proteins, Hsps, namely, smHsp, Hsp70 and Hsp90 have been linked with desiccation survival in insects (Tammariello et al., 1999; Sjursen et al., 2001; Hayward et al., 2004a; Benoit et al., 2009; Benoit, 2010). LEA proteins are another group of upregulated molecules that act as molecular shields to protect other proteins and bio-membranes against aggregation and denaturation resulting from drying (Goyal et al., 2005; Sogame and Kikawada, 2017). Interestingly, however, B. antarctica lacks genes encoding LEA proteins and Hsps are apparently not involved in conferring desiccation tolerance (Philip et al., 2008). Instead, metabolite synthesis and membrane phospholipids, distinct contractile and cytoskeletal protein patterns and aquaporins are among the key players essential for successful anhydrobiosis in the Antarctic midge (Benoit et al., 2007b; Michaud et al., 2008; Li et al., 2009; Teets et al., 2012; Kelley et al., 2014). In addition, desiccation response was shown to upregulate ‘Frost,’ ‘Desi’ and ‘smp-30’ genes whereas ‘Desat2’ was downregulated during post-desiccation recovery (Sinclair et al., 2007; Kawano et al., 2010). Metabolic fingerprint comparisons in mosquitoes have highlighted specific metabolic alterations, enabling them to survive seasonal aridity (Hidalgo et al., 2015). Diapause in Aedes albopictus (Diptera: Culicidae) promotes desiccation survival by overexpression of a transcript involved in lipid storage with a concomitant increase in hydrocarbon levels (Diniz et al., 2017). Seminal contributions from Davies et al. (2014) have deepened our understanding on the neuroendocrine regulation of salt and water balance in insects (Luan et al., 2015). Recently, the importance of capa neuropeptides as anti-diuretic hormones have been identified in D. melanogaster and is postulated to be a part of desiccation tolerance mechanisms in other insects as well (Davies et al., 2013; Terhzaz et al., 2015).
Conclusion
Adaptive mechanisms vary among organisms based on their ecological and evolutionary background. Thus, stress tolerance physiology is bound to vary even among closely related species and therefore cannot be generalized. In addition, variations in desiccation tolerance physiology is often a result of the desiccation protocols (acute/chronic) employed. It might therefore be possible to judge the desiccation tolerance or anhydrobiotic potential of organisms in the true sense, only when they are studied under a common denominator of reproducible protocols. Nature has a vast array of tactics to safeguard its biodiversity and therefore, exploration of other aridity-induced mechanisms in known and unknown desiccation tolerant organisms will give way to our holistic understanding of the diversity in tolerance patterns from an evolutionary, ecological, physiological, cellular and molecular perspective. As reviewed here, although several molecular and biochemical underpinnings of desiccation tolerance in insects are thoroughly studied and well-established, an understanding of some other basic mechanisms remain elusive. For instance, there is a lack of information on the status of the immune responses elicited during desiccation survival. Another neglected area is the understanding of the neuronal basis governing recovery from desiccation that leads to the reactivation of coordinated sensory circuits. As an example, Pflüger and colleagues have determined the role of insect neurotransmitters in modulating multiple physiological and behavioral processes and have emphasized the involvement of biogenic amines under heat, mechanical stress, starvation and chemicals in insects (Verlinden et al., 2010). Similar studies on physiological water deficits in insects can hold great promise for translational research.
The role of insects as reliable mechanistic models presents endless research possibilities for the prediction of the consequences of climate change. The extreme desiccation tolerance of P. vanderplanki has been exploited as a prototype insect system for investigating the influence of spaceflight environments on life processes (Gusev et al., 2010a). Furthermore, the knowledge of insect desiccation biology offers ample ideas for exciting biomedical and pharmaceutical applications, e.g., anhydrobiotic engineering that targets at improving desiccation tolerance of desiccation-sensitive species, including humans (de Castro et al., 2000; Watanabe et al., 2016). These and many other applications that might have been previously viewed as science fiction, are now possible because of our knowledge of insect responses to water scarcity. Thus, research in desiccation stress response biology has come a long way from curiosity-driven explorations to present day technology-driven applications. Therefore, we hope that this review will trigger impetus for the development of methods and technology to mitigate the consequences of climate change in human and non-human biota.
Author Contributions
LT and BN designed the review layout. LT prepared the manuscript draft, table, and figures. BN revised the manuscript with critical inputs. BN and LT approved the final version of the manuscript.
Funding
BN is thankful to partial funding received from UGC-ISF joint Indo-Israel Research Program [UGC-018 (191)] and UGC-CAS (Phase-III) grant. LT is grateful for logistic support received from the DBT Bio-CARe grant. The funders had no role in manuscript design, preparation or decision to publish.
Conflict of Interest Statement
The authors declare that the research was conducted in the absence of any commercial or financial relationships that could be construed as a potential conflict of interest.
Acknowledgments
We are grateful to Drs. Takashi Okuda (NIAS, Japan), Tetsuo Suemoto (Oita University, Japan) and Koichiro Kawai (Hiroshima University, Japan) for their crucial advice, comments and suggestions on insect desiccation tolerance. We also thank Drs. S. C. Lakhotia (BHU, India) B. J. Rao (TIFR, India) and Amitabh Joshi (Evolutionary and Organismal Biology Unit, JNCSAR, Bengaluru) for their valuable insights.
Footnotes
References
Addo-Bediako, A., Chown, S. L., and Gaston, K. J. (2001). Revisiting water loss in insects: a large scale view. J. Insect Physiol. 47, 1377–1388. doi: 10.1016/S0022-1910(01)00128-7
Alpert, P. (2005). The limits and frontiers of desiccation-tolerant life. Integr. Comp. Biol. 45, 685–695. doi: 10.1093/icb/45.5.685
Alto, B. W., and Juliano, S. A. (2001). Temperature effects on the dynamics of Aedes albopictus (Diptera: Culicidae) populations in the laboratory. J. Med. Entomol. 38, 548–556. doi: 10.1603/0022-2585-38.4.548
Alvarez, T., Frampton, G. K., and Goulson, D. (1999). The effects of drought upon epigeal collembola from arable soils. Agr. For. Entomol. 1, 243–248. doi: 10.1046/j.1461-9563.1999.00032.x
Arlian, L., and Staiger, T. (1979). Water balance in the semiaquatic beetle, Peltodytes muticus. Comp. Biochem. Physiol. Part A Mol. Integr. Physiol. 62A, 1041–1047. doi: 10.1016/0300-9629(79)90047-1
Atmowidjojo, A. H., Wheeler, D. E., Erickson, E. H., and Cohen, A. C. (1997). Temperature tolerance and water balance in feral and domestic honey bees, Apis mellifera L. Comp. Biochem. Physiol. Part A Physiol. 118, 1399–1403. doi: 10.1016/S0300-9629(97)00031-5
Bazinet, A. L., Marshall, K. E., MacMillan, H. A., Williams, C. M., and Sinclair, B. J. (2010). Rapid changes in desiccation resistance in Drosophila melanogaster are facilitated by changes in cuticular permeability. J. Insect Physiol. 56, 2006–2012. doi: 10.1016/j.jinsphys.2010.09.002
Bedick, J. C., Wyatt, W. H., and Albrecht, M. C. (2006). High water-loss rates and rapid dehydration in the burying beetle, Nicrophorus marginatus. Physiol. Entomol. 31, 23–29. doi: 10.1111/j.1365-3032.2005.00477.x
Bellard, C., Bertelsmeier, C., Leadley, P., Thuiller, W., and Courchamp, F. (2012). Impacts of climate change on the future of biodiversity. Ecol. Lett. 15, 365–377. doi: 10.1111/j.1461-0248.2011.01736.x
Benoit, J. B. (2010). “Water management by dormant insects: comparisons between dehydration resistance during summer aestivation and winter diapause and aestivation,” in Progress in Molecular and Subcellular Biology, eds C. Arturo Navas and J. Carvalho (Berlin: Springer).
Benoit, J. B., and Denlinger, D. L. (2007). Suppression of water loss during adult diapause in the northern house mosquito, Culex pipiens. J. Exp. Biol. 210, 217–226. doi: 10.1242/jeb.02630
Benoit, J. B., and Lopez-Martinez, G. (2012). “Role of conventional and unconventional stress proteins during the response of insects to traumatic environmental conditions,” in Hemolymph Proteins and Functional Peptides: Recent Advances in Insects and Other Arthropods, eds M. Tufail and M. Takeda (Oak Park, IL: Bentham Science), 128–160.
Benoit, J. B., Del Grosso, N. A., Yoder, J. A., and Denlinger, D. L. (2007a). Resistance to dehydration between bouts of blood feeding in the bed bug, Cimex lectularius, is enhanced by water conservation, aggregation, and quiescence. Am. J. Trop. Med. Hyg. 76, 987–993.
Benoit, J. B., Lopez-Martinez, G., Michaud, M. R., Elnitsky, M. A., Lee, R. E. Jr., and Denlinger, D. L. (2007b). Mechanisms to reduce dehydration stress in larvae of the Antarctic midge Belgica antarctica. J. Insect Physiol. 53, 656–667. doi: 10.1016/j.jinsphys.2007.04.006
Benoit, J. B., Lopez-Martinez, G., Phillips, Z. P., Patrick, K. R., and Denlinger, D. L. (2009). Heat shock proteins contribute to mosquito dehydration tolerance. J. Insect Physiol. 56, 151–156. doi: 10.1016/j.jinsphys.2009.09.012
Biemont, J. C., Chauvin, G., and Hamon, C. (1981). Ultrastructure and resistance to water loss in eggs of Acanthoscelides obtectus say (Coleoptera: Bruchidae). J. Insect Physiol. 27, 667–679. doi: 10.1016/0022-1910(81)90003-2
Boggs, C. L. (2016). The fingerprints of global climate change on insect populations. Curr. Opin. Insect Sci. 17, 69–73. doi: 10.1016/j.cois.2016.07.004
Chown, S. L., Sørense, J. G., and Terblanche, J. S. (2011). Water loss in insects: an environmental change perspective. J. Insect Physiol. 57, 1070–1084. doi: 10.1016/j.jinsphys.2011.05.004
Clark, B. R., and Faeth, S. H. (1998). The evolution of egg clustering in butterflies: a test of the egg desiccation hypothesis. Evol. Ecol. 12, 543–552. doi: 10.1023/A:1006504725592
Clegg, J. (2001). Cryptobiosis- a peculiar state of biological organization. Comp. Biochem. Physiol. Part B 128, 613–624. doi: 10.1016/S1096-4959(01)00300-1
Colvin, J. (1996). Diapause duration, survival in relation to desiccation and egg-pod morphology of the Senegalese grasshopper, Oedaleus senegalensis. Physiol. Entomol. 21, 173–178. doi: 10.1111/j.1365-3032.1996.tb00852.x
Cooke, B. D., and Skewes, M. K. (1988). The effects of temperature and humidity on the survival and development of the European rabbit flea, Spilopsyllus cuniculi (Dale). Aust. J. Zool. 36, 649–659. doi: 10.1071/ZO9880649
Cornette, R., and Kikawada, T. (2011). The induction of anhydrobiosis in the sleeping chironomid: current status of our knowledge. IUBMB Life 63, 419–429. doi: 10.1002/iub.463
Cornette, R., Kikawada, T., and Shagimardanova, E. I. (2016). New antioxidant genes from an anhydrobiotic insect: unique structural features in functional motifs of thioredoxin. Bionanoscience 6, 568–570. doi: 10.1007/s12668-016-0278-x
Cornette, R., Yamamoto, N., Yamamoto, M., Kobayashi, T., Petrova, N. A., Gusev, O., et al. (2017). A new anhydrobiotic midge from Malawi, Polypedilum pembai sp.n. (Diptera: Chironomidae), closely related to the desiccation tolerant midge, Polypedilum vanderplanki Hinton. Sys. Entomol. 42, 814–825. doi: 10.1111/syen.12248
Crowe, J. H., and Madin, K. A. (1974). Anhydrobiosis in tardigrades and nematodes. Trans. Am. Microsc. Soc. 93, 513–524. doi: 10.2307/3225155
Dambach, M., and Goehlen, B. (1999). Aggregation density and longevity correlate with humidity in first-instar nymphs of the cockroach (Blattella germanica L., Dictyoptera). J. Insect Physiol. 45, 423–429. doi: 10.1016/S0022-1910(98)00141-3
Danks, H. V. (2002). The range of insect dormancy responses. J. Entomol. 99, 127–142. doi: 10.14411/eje.2002.021
Davidson, J. K. (1990). Nonparallel geographic patterns for tolerance to cold and desiccation in Drosophila melanogaster and Drosophila simulans. Aust. J. Zool. 38, 155–161. doi: 10.1071/ZO9900155
Davies, S. A., Cabrero, P., Overend, G., Aitchison, L., Sebastian, S., Terhzaz, S., et al. (2014). Cell sigalling mechanisms for insect stress tolerance. J. Exp. Biol. 217, 119–128. doi: 10.1242/jeb.090571
Davies, S. A., Cabrero, P., Povsic, M., Johnston, N. R., Terhzaz, S., and Dow, J. A. (2013). Signaling by Drosophila capa neuropeptides. Gen. Comp. Endocrinol. 1, 60–66. doi: 10.1016/j.ygcen.2013.03.012
de Castro, A. G., Bredholt, H., Strøm, A. R., and Tunnacliffe, A. (2000). Anhydrobiotic engineering of gram-negative bacteria. Appl. Environ. Microbiol. 66, 4142–4144. doi: 10.1128/AEM.66.9.4142-4144.2000
Denlinger, D. L. (1986). Dormancy in tropical insects. Ann. Rev. Entornol. 31, 239–264. doi: 10.1146/annurev.en.31.010186.001323
Diniz, D. F. A., de Albuquerque, C. M., Oliva, L. O., de Melo-Santos, M. A. V., and Ayres, C. F. J. (2017). Diapause and quiescence: dormancy mechanisms that contribute to the geographical expansion of mosquitoes and their evolutionary success. Parasit. Vectors 10:310. doi: 10.1186/s13071-017-2235-0
Elnitsky, M. A., Benoit, J. B., Denlinger, D. L., and Lee, R. E. Jr. (2008). Desiccation tolerance and drought acclimation in the Antarctic collembolan Cryptopygus antarcticus. J. Insect Physiol. 54, 1432–1439. doi: 10.1016/j.jinsphys.2008.08.004
Ferveur, J.-F., Corto, J., Rihani, K., Cobb, M., and Everaerts, C. (2018). Desiccation resistance: effect of cuticular hydrocarbons and water content in Drosophila melanogaster adults. PeerJ. 6:e4318. doi: 10.7717/peerj.4318
França, M. B., Panek, A. D., and Eleutherio, E. C. A. (2007). Oxidative stress and its effects during dehydration. Comp. Biochem. Physiol. Part A Mol. Intg. Physiol. 146, 621–631. doi: 10.1016/j.cbpa.2006.02.030
Giard, A. (1894). L’anhydrobiose ou ralentissement des phénoménes vitaux. C. R. Soc. Biol. 46, 497–500.
Gibbs, A. G., and Matzkin, L. M. (2001). Evolution of water balance in the genus Drosophila. J. Exp. Biol. 204, 2331–2338.
Gilberta, J. D. J. (2014). Thrips domiciles protect larvae from desiccation in an arid environment. Behav. Ecol. 25, 1338–1346. doi: 10.1093/beheco/aru128
Goto, S. G., Doi, K., Nakayama, S., and Numata, H. (2008). Maternal control of cold and desiccation tolerance in eggs of the band-legged ground cricket Dianemobius nigrofasciatus in relation to embryonic diapause. Entomol. Res. 38, 17–23. doi: 10.1111/j.1748-5967.2008.00140.x
Goyal, K., Browne, J. A., Walton, L. J., Pinelli, C., Rastogi, R. K., Burnell, A. M., et al. (2004). Molecular anhydrobiology: identifying molecules implicated in invertebrate anhydrobiosis. Integr. Comp. Biol. 45, 702–709. doi: 10.1093/icb/45.5.702
Goyal, K., Walton, L. J., and Tunnacliffe, A. (2005). LEA proteins prevent protein aggregation due to water stress. Biochem. J. 388, 151–157. doi: 10.1042/BJ20041931
Gusev, O., Nakahara, Y., Sychev, V., Levinskikh, M., Novikova, N., Alexeev, V., et al. (2010a). An anhydrobiotic insect, Polypedilum vanderplanki as a tool for astrobiology. Space Utiliz. Res. 24, 306–309.
Gusev, O., Nakahara, Y., Vanyagina, V., Malutina, L., Cornette, R., Sakashita, T., et al. (2010b). Anhydrobiosis-associated nuclear dna damage and repair in the sleeping chironomid: linkage with radioresistance. PLoS One 5:e14008. doi: 10.1371/journal.pone.0014008
Gusev, O., Suetsugu, Y., Cornette, R., Kawashima, T., Logacheva, M. D., Kondrashov, A. S., et al. (2014). Comparative genome sequencing reveals genomic signature of extreme desiccation tolerance in the anhydrobiotic midge. Nat. Commun. 5:4784. doi: 10.1038/ncomms5784
Harper, P. P., and Hynes, H. B. N. (1970). Diapause in the nymphs of Canadian winter stoneflies. Ecology 51, 925–927. doi: 10.2307/1933992
Hayward, S. A. L., Rinehart, J. P., and Denlinger, D. L. (2004a). Desiccation and rehydration elicit distinct heat shock protein transcript responses in flesh fly pupae. J. Exp. Biol. 207, 963–997. doi: 10.1242/jeb.00842
Hayward, S. A. L., Worland, M. R., Convey, P., and Bale, J. S. (2004b). Habitat moisture availability and the local distribution of the antarctic collembola Cryptopygus antarcticus and Friesea grisea. Soil Biol. Biochem. 36, 927–934. doi: 10.1016/j.soilbio.2004.02.007
Hernández, A., Zamora, J., González, N., Salazar, E., and Sánchez, M. D. (2009). Anhydrobiosis quotient: a novel approach to evaluate stability in desiccated bacterial cells. J. Appl. Microbiol. 107, 436–442. doi: 10.1111/j.1365-2672.2009.04216.x
Hidalgo, K., Montazeau, C., Siaussat, D., Braman, V., Trabalon, M., Simard, F., et al. (2018). Distinct physiological, biochemical and morphometric adjustments in the malaria vectors Anopheles gambiae and An. coluzzii as means to survive to dry season conditions in Burkina Faso. J. Exp. Biol. 21:jeb.174433. doi: 10.1242/jeb.174433
Hidalgo, K., Mouline, K., Mamai, W., Foucreau, N., Dabiré, K. R., Bouchereau, A., et al. (2014). Novel insights into the metabolic and biochemical underpinnings assisting dry-season survival in female malaria mosquitoes of the Anopheles gambiae complex. J. Insect Physiol. 70, 102–116. doi: 10.1016/j.jinsphys.2014.07.003
Hidalgo, K., Mouline, K., Mamai, W., Foucreau, N., Dabiré, K. R., Bouchereau, A., et al. (2015). Combining two-dimensional gel electrophoresis and metabolomic data in support of dry-season survival in the two main species of the malarial mosquito Anopheles gambiae. Data Brief 5, 255–268. doi: 10.1016/j.dib.2015.08.031
Hinton, H. E. (1951). A new chironomid from Africa, the larva of which can be dehydrated without injury. Proc. Zool. Soc. Lond. 121, 371–380. doi: 10.1111/j.1096-3642.1951.tb00801.x
Hoffmann, A. A., Hallas, R. J., Dean, J. A., and Schiffer, M. (2003). Low potential for climatic stress adaptation in a rainforest Drosophila species. Science 301, 100–102. doi: 10.1126/science.1084296
Hoffmann, A. A., and Parsons, P. A. (1989). An integrated approach to environmental stress tolerance and life-history variation: desiccation tolerance in Drosophila. Biol. J. Linn. Soc. 37, 117–136. doi: 10.1111/j.1095-8312.1989.tb02098.x
Hoffmann, G. E., and Todgham, A. E. (2010). Living in the now: physiological mechanisms to tolerate a rapidly changing environment. Annu. Rev. Physiol. 72, 127–145. doi: 10.1146/annurev-physiol-021909-135900
Holmstrup, M., and Bayley, M. (2013). Protaphorura tricampata, a euedaphic and highly permeable springtail that can sustain activity by osmoregulation during extreme drought. J. Insect Physiol. 59, 1104–1110. doi: 10.1016/j.jinsphys.2013.08.015
Holmstrup, M., Hedlund, K., and Boriss, H. (2002). Drought acclimation and lipid composition in Folsomia candida: implications for cold shock, heat shock and acute desiccation stress. J. Insect Physiol. 48, 961–970. doi: 10.1016/S0022-1910(02)00175-0
Hu, J., Neoh, K. B., Appel, A. G., and Lee, C. Y. (2012). Subterranean termite open-air foraging and tolerance to desiccation: comparative water relation of two sympatric Macrotermes spp. (Blattodea: Termitidae). Comp. Biochem. Physiol. A Mol. Integr. Physiol. 161, 201–217. doi: 10.1016/j.cbpa.2011.10.028
Idrissa, M., Lecoq, M., and Kooyman, C. (2008). Ecology and management of the Senegalese grasshopper, Oedaleus senegalensis (Krauss, 1877) (Orthoptera: Acrididae), in West Africa. review and prospects. Ann. Soc. Entomol. France 44, 271–288. doi: 10.1080/00379271.2008.10697563
Kawano, T., Shimoda, M., Matsumoto, H., Ryuda, M., Tsuzuki, S., and Hayakawa, Y. (2010). Identification of a gene, Desiccate, contributing to desiccation resistance in Drosophila melanogaster. J. Biol. Chem. 285, 38889–38897. doi: 10.1074/jbc.M110.168864
Keilin, D. (1959). The problem of anabiosis or latent life: history and current concept. Proc. R. Soc. Lond. B 150, 149–191. doi: 10.1098/rspb.1959.0013
Kelley, J. L., Peyton, J. T., Fiston-Lavier, A.-S., Teets, N. M., Yee, M.-C., Johnston, J. S., et al. (2014). Compact genome of the antarctic midge is likely an adaptation to an extreme environment. Nat. Commun. 5:4611. doi: 10.1038/ncomms5611
Kharboutli, M. S., and Mack, T. P. (1993). Tolerance of the striped earwig (Dermaptera: Labiduridae) to hot and dry conditions. Envtl. Entomol. 22, 663–668. doi: 10.1093/ee/22.3.663
Kikawada, T., Minakawa, N., Watanabe, M., and Okuda, T. (2005). Factors inducing successful anhydrobiosis in the African chironomid Polypedilum vanderplanki: significance of the larval tubular nest. Integr. Comp. Biol. 45, 710–714. doi: 10.1093/icb/45.5.710
Kikawada, T., Saito, A., Kanamori, Y., Fujita, M., Śnigórska, K., Watanabe, M., et al. (2008). Dehydration-inducible changes in expression of two aquaporins in the sleeping chironomid, Polypedilum vanderplanki. Biochim. Biophys. Acta 1778, 514–520. doi: 10.1016/j.bbamem.2007.11.009
Kranner, I., and Birtič, S. (2005). A modulating role for antioxidants in desiccation tolerance. Integr. Comp. Biol. 45, 451–460. doi: 10.1093/icb/45.5.734
Lancaster, J., Downes, B. J., and Arnold, A. (2010). Environmental constraints on oviposition limit egg supply of a stream insect at multiple scales. Oecologia 163, 373–384. doi: 10.1007/s00442-010-1565-9
Leprince, O., and Buitink, J. (2015). Introduction to desiccation biology: from old borders to new frontiers. Planta 242, 369–378. doi: 10.1007/s00425-015-2357-6
Li, A., Benoit, J. B., Lopez-Martinez, G., Elnitsky, M. A., Lee, R. E. Jr., and Denlinger, D. L. (2009). Distinct contractile and cytoskeletal protein patterns in the Antarctic midge are elicited by desiccation and rehydration. Proteomics 9, 2788–2797. doi: 10.1002/pmic.200800850
Lopez-Martinez, G., Benoit, J. B., Rinehart, J. P., Elnitsky, M. A., Lee, R. E., and Denlinger, D. L. (2009). Dehydration, rehydration, and overhydration alter patterns of gene expression in the Antarctic midge, Belgica antarctica. J. Comp. Physiol. B 179, 481–491. doi: 10.1007/s00360-008-0334-0
Luan, Z., Quigley, C., and Li, H. (2015). The putative Na+/Cl- dependent neurotransmitter/osmolyte transporter inebriated in the Drosophila hindgut is essential for the maintenance of systemic water homeostasis. Sci. Rep. 5:7993. doi: 10.1038/srep07993
Marron, M. T., Markow, T. A., Kain, K. J., and Gibbs, A. G. (2003). Effects of starvation and desiccation on energy metabolism in desert and mesic Drosophila. J. Insect Physiol. 49, 261–270. doi: 10.1016/S0022-1910(02)00287-1
Marten, M., and Zwick, P. (1989). The temperature dependence of embryonic and larval development in Protonemura intricata (Plecoptera: Nemouridae). Freshw. Biol. 22, 1–14. doi: 10.1111/j.1365-2427.1989.tb01079.x
Mazer, C., and Appe, A. (2001). Water loss and desiccation tolerances of longwing butterflies (Lepidoptera: Nymphalidae). Environ. Entomol. 30, 631–636. doi: 10.1603/0046-225X-30.4.631
Mazin, P. V., Shagimardanova, E., Kozlova, O., Cherkasov, A., Sutormin, R., Stepanova, V. V., et al. (2018). Cooption of heat shock regulatory system for anhydrobiosis in the sleeping chironomid Polypedilum vanderplanki. Proc. Natl. Acad. Sci. U.S.A. 6, E2477–E2486. doi: 10.1073/pnas.1719493115
McCluney, K. E., and Date, R. C. (2008). The effects of hydration on growth of the house cricket, Acheta domesticus. J. Insect Sci. 8:32. doi: 10.1673/031.008.3201
Michaud, M. R., Benoit, J. B., Lopez-Martinez, G., Elnitsky, M. A., Lee, R. E., and Denlinger, D. L. (2008). Metabolomics reveals unique and shared metabolic changes in response to heat shock, freezing, and desiccation in the Antarctic midge, Belgica antarctica. J. Insect Physiol. 54, 645–655. doi: 10.1016/j.jinsphys.2008.01.003
Mitsumasu, K., Kanamori, Y., Fujita, M., Iwata, K., Tanaka, D., Kikuta, S., et al. (2010). Enzymatic control of anhydrobiosis-related accumulation of trehalose in the sleeping chironomid, Polypedilum vanderplanki. FEBS J. 277, 4215–4228. doi: 10.1111/j.1742-4658.2010.07811.x
Moriyama, M., and Numata, H. (2010). Desiccation tolerance in fully developed embryos of two cicadas, Cryptotympana facialis and Graptopsaltria nigrofuscata. Entomol. Sci. 13, 68–74. doi: 10.1111/j.1479-8298.2010.00365.x
Moriyama, M., and Numata, H. (2011). A cicada that ensures its fitness during climate warming by synchronizing its hatching time with the rainy season. Zool. Sci. 28, 875–881. doi: 10.2108/zsj.28.875
Nakahara, Y., Watanabe, M., Fujita, A., Kanamori, Y., Tanaka, D., Iwata, K., et al. (2008). Effects of dehydration rate on physiological responses and survival after rehydration in larvae of the anhydrobiotic chironomid. J. Insect Physiol. 54, 1220–1225. doi: 10.1016/j.jinsphys.2008.05.007
Nesmelov, A. A., Devatiyarov, R. M., Voronina, T. A., Kondratyeva, S. A., Cherkasov, A. V., Cornette, R., et al. (2016). New antioxidant genes from an anhydrobiotic insect: unique structural features in functional motifs of thioredoxin. Bionanoscience 6, 568–570. doi: 10.1007/s12668-016-0278-x
Nolte, U., Tietböhl, R. S., and McCafferty, W. P. (1996). A mayfly from tropical Brazil capable of tolerating short-term dehydration. J. North Am. Benthol. Soc. 15, 87–94. doi: 10.2307/1467434
North, A. R., and Godfray, H. C. (2018). Modelling the persistence of mosquito vectors of malaria in Burkina Faso. Malar. J. 17:140. doi: 10.1186/s12936-018-2288-3
Pallarés, S., Velasco, J., Millán, A., Bilton, D. T., and Arribas, P. (2016). Aquatic insects dealing with dehydration: do desiccation resistance traits differ in species with contrasting habitat preferences? PeerJ 4:e2382. doi: 10.7717/peerj.2382
Parkash, R., Aggarwal, D. D., Ranga, P., and Singh, D. (2012a). Divergent strategies for adaptation to desiccation stress in two Drosophila species of immigrans group. J. Comp. Physiol. B 182, 751–769. doi: 10.1007/s00360-012-0655-x
Parkash, R., Ramniwas, S., Kajla, B., and Aggarwal, D. D. (2012b). Divergence of desiccation-related traits in two Drosophila species of the takahashii subgroup from the western Himalayas. J. Exp. Biol. 215, 2181–2191. doi: 10.1242/jeb.065730
Philip, B. N., Yi, S.-X., Elnitsky, M. A., and Lee, R. E. Jr. (2008). Aquaporins play a role in desiccation and freeze tolerance in larvae of the goldenrod gall fly, Eurosta solidaginis. J. Exp. Biol. 211, 1114–1119. doi: 10.1242/jeb.016758
Potter, K. A., and Woods, H. A. (2012). No evidence for the evolution of thermal or desiccation tolerance of eggs among populations of Manduca sexta. Funct. Ecol. 26, 112–122. doi: 10.1111/j.1365-2435.2011.01912.x
Rebecchi, L. (2013). Dry up and survive: the role of antioxidant defences in anhydrobiotic organisms. J. Limnol. 72, 62–72. doi: 10.4081/jlimnol.2013.s1.e8
Rebora, M., Piersanti, S., Salerno, G., Conti, E., and Gaino, E. (2007). Water deprivation tolerance and humidity response in a larval dragonfly: a possible adaptation for survival in drying ponds. Physiol. Entomol. 32, 121–126. doi: 10.1111/j.1365-3032.2006.00553.x
Rowley, M., and Hanson, F. (2007). Humidity detection and hygropreference behavior in larvae of the tobacco hornworm, Manduca sexta. J. Insect Sci. 7:39. doi: 10.1673/031.007.3901
Schill, R. O. (2010). “Anhydrobiotic abilities of tardigrades,” in Dormancy and Resistance in Harsh Environments, eds E. Lubzens, J. Cerda, and M. Clark (Heidelberg: Springer-Verlag), 133–146.
Schill, R. O., Mali, B., Dandekar, T., Schnolzer, M., Reuter, D., and Frohme, M. (2009). Molecular mechanisms of tolerance in tardigrades: new perspectives for preservation and stabilization of biological material. Biotechnol. Adv. 27, 348–352. doi: 10.1016/j.biotechadv.2009.01.011
Shukla, E., Thorat, L., Bendre, A., Jadhav, S., Pal, J. K., Nath, B. B., et al. (2018). Cloning and characterization of trehalase: a conserved glycosidase from oriental midge, Chironomus ramosus. 3 Biotech 8, 352–358. doi: 10.1007/s13205-018-1376-y
Shukla, E., Thorat, L., Bhavnani, V., Bendre, A., Pal, J. K., Nath, B. B., et al. (2016). Molecular cloning and in silico studies of physiologically significant trehalose from Drosophila melanogaster. Int. J. Biol. Macromol. 92, 282–292. doi: 10.1016/j.ijbiomac.2016.06.097
Shukla, E., Thorat, L., Nath, B. B., and Gaikwad, S. M. (2015). Insect trehalase: physiological significance and potential applications. Glycobiology 25, 357–367. doi: 10.1093/glycob/cwu125
Silverman, J., and Rust, M. K. (1983). Some abiotic factors affecting the survival of the cat flea, Ctenocephalides felis (Siphonaptera: Pulicidae). Environ. Entomol. 12, 490–495. doi: 10.1093/ee/12.2.490
Simelane, D. O. (2007). Influence of temperature, photoperiod and humidity on oviposition and egg hatch of the root-feeding flea beetle Longitarsus bethae (Chrysomelidae: Alticinae), a natural enemy of the weed Lantana camara (Verbenaceae). Bull. Entomol. Res. 97, 111–116. doi: 10.1017/S0007485307004713
Sinclair, B. J., Gibbs, A. G., and Roberts, S. P. (2007). Gene transcription during exposure to, and recovery from, cold and desiccation stress in Drosophila melanogaster. Insect Mol. Biol. 16, 435–443. doi: 10.1111/j.1365-2583.2007.00739.x
Sjursen, H., Bayley, M., and Holmstrup, M. (2001). Enhanced drought tolerance of a soil-dwelling springtail by pre-acclimation to mild desiccation stress. J. Insect Physiol. 47, 1021–1027. doi: 10.1016/S0022-1910(01)00078-6
Sogame, Y., and Kikawada, T. (2017). Current findings on the molecular mechanisms underlying anhydrobiosis in Polypedilum vanderplanki. Curr. Opin. Insect Sci. 19, 16–21. doi: 10.1016/j.cois.2016.10.008
Sota, T., and Mogi, M. (1992). Interspecific variation in desiccation survival time of Aedes (stegomyia) mosquito eggs is correlated with habitat and egg size. Oecologia 90, 353–358. doi: 10.1007/BF00317691
Strachan, S. R., Chester, E. T., and Robson, B. J. (2015). Freshwater invertebrate life history strategies for surviving desiccation. Springer Sci. Rev. 3, 57–75. doi: 10.1007/s40362-015-0031-9
Suemoto, T., Kawai, K., and Imabayashi, H. A. (2004). Comparison of desiccation tolerance among 12 species of chironomid larvae. Hydrobiologia 515, 107–114. doi: 10.1023/B:HYDR.0000027322.11005.20
Tammariello, S. P., Rinehart, J. P., and Denlinger, D. L. (1999). Desiccation elicits heat shock protein transcription in the flesh fly, Sacrophaga crassipalpis, but does not enhance tolerance to high or low temperature. J. Insect Physiol. 45, 933–938. doi: 10.1016/S0022-1910(99)00073-6
Teets, N. M., Peyton, J. T., Colinet, H., Renault, D., Kelley, J. L., and Kawarasaki, Y. (2012). Gene expression changes governing extreme dehydration tolerance in an Antarctic insect. Proc. Natl. Acad. Sci. U.S.A. 109, 20744–20749. doi: 10.1073/pnas.1218661109
Terhzaz, S., Teets, N. M., Cabrero, P., Henderson, L., Ritchie, M. G., Nachman, R. J., et al. (2015). Insect capa neuropeptides impact desiccation and cold tolerance. Proc. Natl. Acad. Sci. U.S.A. 112, 2882–2887. doi: 10.1073/pnas.1501518112
Thorat, J. T., Gaikwad, S. M., and Nath, B. B. (2012). Trehalose as an indicator of desiccation stress in Drosophila melanogaster larvae: a potential marker of anhydrobiosis. Biochem. Biophys. Res. Commun. 419, 638–642. doi: 10.1016/j.bbrc.2012.02.065
Thorat, L., Mani, K., Thankgaraj, P., Chatterjee, S., and Nath, B. B. (2016a). Downregulation of dTps1 in Drosophila melanogaster larvae confirms involvement of trehalose in redox regulation following desiccation. Cell Stress Chaperon. 21, 285–294. doi: 10.1007/s12192-015-0658-0
Thorat, L., and Nath, B. B. (2015). Tolerance to desiccation stress in Chironomus ramosus through plasticity in homeostatic control. Eur. J. Environ. Sci. 5, 86–91. doi: 10.14712/23361964.2015.81
Thorat, L., and Nath, B. B. (2016). Quantitative assessment of larval desiccation tolerance in oriental Chironomus species. Curr. Sci. 111, 1448–1449.
Thorat, L., and Nath, B. B. (2018). Aquatic silk proteins in Chironomus: a review. J. Limnol. 77, 95–103. doi: 10.4081/jlimnol.2018.1797
Thorat, L., Oulkar, D., Banerjee, K., Gaikwad, S., and Nath, B. B. (2017). High-throughput mass spectrometry analysis revealed a role for glucosamine in potentiating recovery following desiccation stress in Chironomus. Sci. Rep. 7, 3659–3671. doi: 10.1038/s41598-017-03572-5
Thorat, L., Oulkar, D., Banerjee, K., and Nath, B. B. (2016b). Desiccation stress induces developmental heterochrony in Drosophila melanogaster following desiccation stress. J. Biosci. 41, 331–339.
Tichy, H. J. (1979). Hygro- and thermoreceptive triad in antennal sensillum of the stick insect, Carausius morosus. Comp. Physiol. 132, 149–152. doi: 10.1007/BF00610718
Treherne, J. E., and Willmer, P. G. (1975). Hormonal control of integumentary water loss: evidence for a novel neuroendocrine system in an insect (Periplaneta americana). J. Exp. Biol. 63, 143–159.
Tunnacliffe, A., and Lapinski, J. (2003). Resurrecting Van Leeuwenhoek’s rotifers: a reappraisal of the role of disaccharides in anhydrobiosis. Philos. Trans. R. Soc. Lond. B 358, 1755–1771. doi: 10.1098/rstb.2002.1214
Tunnacliffe, A., Lapinski, J., and McGee, B. A. (2005). Putative LEA protein, but no trehalose, is present in anhydrobiotic bdelloid rotifers. Hydrobiologia 546, 315–321. doi: 10.1007/1-4020-4408-9_32
Verlinden, H., Vleugels, R., Marchal, E., Badisco, L., Pflüger, H.-J., Blenau, W., et al. (2010). The role of octopamine in locusts and other arthropods. J. Insect Physiol. 56, 854–867. doi: 10.1016/j.jinsphys.2010.05.018
Wadaka, M., Mouline, K., Parvy, J.-P., Lannic, J. L., Dabiré, K. R., Ouédraogo, G. A., et al. (2016). Morphological changes in the spiracles of Anopheles gambiae s.l (Diptera) as a response to the dry season conditions in Burkina Faso (West Africa). Parasit. Vectors 69:11. doi: 10.1186/s13071-015-1289-0
Warren, M., Robertson, M. P., and Greeff, J. M. (2010). A comparative approach to understanding factors limiting abundance patterns and distributions in a fig tree-fig wasp mutualism. Ecography 33, 148–158. doi: 10.1111/j.1600-0587.2009.06041.x
Watanabe, K., Imanishi, S., Akiduki, G., Cornette, R., and Okuda, T. (2016). Air-dried cells from the anhydrobiotic insect, Polypedilum vanderplanki, can survive long term preservation at room temperature and retain proliferation potential after rehydration. Cryobiology 73, 93–98. doi: 10.1016/j.cryobiol.2016.05.006
Watanabe, M. (2006). Anhydrobiosis in invertebrates. App. Entomol. Zool. 41, 15–31. doi: 10.1303/aez.2006.15
Weldon, C. W., Boardman, L., Marlin, D., and Terblanche, J. S. (2016). Physiological mechanisms of dehydration tolerance contribute to the invasion potential of Ceratitis capitata (Wiedemann) (Diptera: Tephritidae) relative to its less widely distributed congeners. Front. Zool. 13:15. doi: 10.1186/s12983-016-0147-z
Wickson, S., Chester, E. T., and Robson, B. J. (2012). Aestivation provides flexible mechanisms for survival of stream drying in a larval trichopteran (Leptoceridae). Mar. Freshw. Res. 63, 821–826. doi: 10.1071/MF12095
Willmer, P. G. (1980). The effects of a fluctuating environment on the water relations of larval Lepidoptera. Ecol. Entomol. 5, 271–292. doi: 10.1111/j.1365-2311.1980.tb01150.x
Xie, Q., and Zhang, R. (2007). Responses of oriental fruit fly (diptera: tephritidae) third instars to desiccation and immersion. J. Agric. Urban Entomol. 24, 1–11. doi: 10.3954/1523-5475-24.1.1
Yoder, J. A., Benoit, J. B., Denlinger, D. L., and Rivers, D. B. (2006). Stress-induced accumulation of glycerol in the flesh fly, Sarcophaga bullata: evidence indicating anti-desiccant and cryoprotectant functions of this polyol and a role for the brain in coordinating the response. J. Insect Physiol. 52, 202–214. doi: 10.1016/j.jinsphys.2005.10.005
Yoshida, M., Matsuda, H., Kubo, H., and Nishimura, T. (2016). Molecular characterization of Tps1 and Treh genes in Drosophila and their role in body water homeostasis. Sci. Rep. 6:30582. doi: 10.1038/srep30582
Keywords: insect ecology, humidity, temperature, climate change, stress, desiccation tolerance, anhydrobiosis, adaptation
Citation: Thorat L and Nath BB (2018) Insects With Survival Kits for Desiccation Tolerance Under Extreme Water Deficits. Front. Physiol. 9:1843. doi: 10.3389/fphys.2018.01843
Received: 27 August 2018; Accepted: 06 December 2018;
Published: 21 December 2018.
Edited by:
Bin Tang, Hangzhou Normal University, ChinaReviewed by:
Jose-Luis Martinez-Guitarte, Universidad Nacional de Educación a Distancia (UNED), SpainKevin Hidalgo, INRA UR370 Qualité des Produits Animaux, France
Copyright © 2018 Thorat and Nath. This is an open-access article distributed under the terms of the Creative Commons Attribution License (CC BY). The use, distribution or reproduction in other forums is permitted, provided the original author(s) and the copyright owner(s) are credited and that the original publication in this journal is cited, in accordance with accepted academic practice. No use, distribution or reproduction is permitted which does not comply with these terms.
*Correspondence: Leena Thorat, bGVlbmF0aG9yYXRAZ21haWwuY29t Bimalendu B. Nath, YmJuYXRoQGdtYWlsLmNvbQ==