- 1Department of Biomedical Engineering, Center of Injury Biomechanics, Wake Forest School of Medicine, Winston-Salem, NC, United States
- 2School of Biomedical Engineering and Sciences (SBES), Virginia Tech – Wake Forest University, Winston-Salem, NC, United States
- 3Department of Biostatistical Sciences, Wake Forest School of Medicine, Winston-Salem, NC, United States
- 4Department of Radiology, Wake Forest School of Medicine, Winston-Salem, NC, United States
The effects of long-duration spaceflight on crewmember neck musculature have not been adequately studied. The purpose of this study was to evaluate the changes in the neck musculature on pre-flight and post-flight magnetic resonance imaging (MRI) examinations of six crewmembers on 4- to 6-month missions equipped with the advanced resistive exercise device (aRED). The MRI images were resliced to remove variations in spinal curvature, the cross-sectional area (CSA), and muscle fat infiltration (MFI) of neck musculature at the C1-C2, C4-C5, C7-T1, and T1-T2 intervertebral disc levels were measured bilaterally. Percent changes in the neck muscle CSA and fatty infiltration following spaceflight were calculated, and mixed models were used to assess significance of these changes. Crewmembers on missions equipped with the aRED experienced an average 25.1% increase in CSA for the trapezius muscle at C6-C7, an average 11.5% increase in CSA for the semispinalis capitis muscle at C4-C5, an average 9.0% increase in CSA for the sternocleidomastoid muscle at C4-C5, and an average 23.1% increase in CSA for the rhomboid minor at T1-T2. There were no significant changes in the CSA of the levator scapulae, splenius capitis, rectus capitis posterior major, scalenus anterior, scalenus posterior, scalenus medius, longissimus capitis, or obliquus capitis inferior muscles at the locations measured. None of the muscles analyzed experienced statistically significant changes in fatty infiltration with spaceflight. Our study indicates that long-duration spaceflight conditions are associated with preservation of CSA in most neck muscles and significant increases in the CSAs of the trapezius, semispinalis capitis, sternocleidomastoid, and rhomboid minor muscles. This may indicate that cervical muscles are not subjected to the same degradative effects microgravity imparts on the majority of muscles.
Introduction
Lower extremity skeletal muscle atrophy has been established for crewmembers on long-duration spaceflights (LeBlanc et al., 2000a; Ploutz-Snyder et al., 2015). The risk of injury to astronauts while on mission, upon landing, and after returning to gravity can be increased as a result of microgravity induced muscle degradation (LeBlanc et al., 1998, 2000a,b; Adams et al., 2003; Lang et al., 2004; Ploutz-Snyder et al., 2015). One common spinal injury in astronauts is a herniated nucleus pulposus (i.e., herniated disc). Disc herniations occur 4.3 times more often in astronauts’ lifetimes, with the incidence of herniation in the cervical spine being 21.4 times higher than that of the civilian population (Johnston et al., 2010). The immediate risk of disc herniation upon return from mission is even higher; astronauts are 35.9 times more likely to experience a herniation during the first year post-flight (Johnston et al., 2010). Recent studies have found an association between spinal muscle atrophy and decreased lumbar lordosis that can lead to disc herniation (Bailey et al., 2018; Green and Scott, 2018).
Prior studies have not reported any significant changes in overall neck musculature following long-duration spaceflight (LeBlanc et al., 2000a). However, there is limited information on the changes of individual neck muscles and the effects of current in-flight exercise interventions on the neck musculature. Bed rest studies have been used to investigate physiological effects of microgravity, given the ease of conducting tests, and the ability to recruit larger sample sizes. One such study focusing on neck muscle changes after 60 days of bed rest found significant increases in muscle volume for the splenius capitis, spinalis cervicis, longus capitis, longus colli, levator scapulae, sternocleidomastoid, and all three scalenes (Belavý et al., 2013). However, it has been postulated that differences in cervical movements during spaceflight compared to bed rest may lead to differences in spinal muscle changes between the two groups (LeBlanc et al., 1992). Therefore, studies of spaceflight spinal musculature are needed to supplement and validate the results of bed rest studies.
It is important to note that muscle atrophy/hypertrophy is only one component affecting crewmember injury risk following long-duration spaceflight. The combination of spaceflight-induced loss of muscle strength, sensorimotor impairment, reduced postural stability, and bone loss contributes to injury during the dynamic loading encountered in spacecraft landings, post-flight falls, and in daily living (LeBlanc et al., 2000a; Lang et al., 2004; Muir et al., 2011; Wood et al., 2011). To address these concerns, in 2008 the International Space Station (ISS) mission 18 introduced an advanced resistive exercise device (aRED) to simulate earth-based gravitational pull and mimic weight-bearing exercise. Previous studies have investigated the effects of long-duration spaceflight on paraspinal muscles, reporting decreased cross-sectional area (CSA) measurements in the erector spinae, multifidus, and quadratus lumborum muscles (Burkhart et al., 2018; McNamara et al., 2019). However, bed rest studies suggest that these beneficial effects may not translate to the cervical region (Belavý et al., 2013).
Magnetic resonance imaging (MRI) is a commonly used imaging modality for measuring the size and composition of the neck muscles (Elliott et al., 2005; Belavý et al., 2013). Performing these measurements both before and after long-duration spaceflight allows for the investigation of the effects of microgravity on overall muscle health. The primary objective of this study was to assess pre- to post-flight changes in the neck muscle CSA on cervical MRI images of crewmembers (n = 6) on 4- to 6-month aRED-equipped missions. The initial hypothesis was that neck muscles would undergo declines in CSA as a result of prolonged microgravity. In addition, measures of muscle composition were explored as a secondary analysis. It was hypothesized that neck muscles would experience greater levels of fatty infiltration with long-duration spaceflight.
Materials and Methods
The mission durations, pre- and post-flight MRI scans and isokinetic data, and in-flight exercise training logs were obtained from the National Aeronautics and Space Administration (NASA) Life Sciences Data Archive and Lifetime Surveillance of Astronaut Health project. Written informed consent was obtained from each crewmember and the study protocols were approved by the institutional review boards at the Wake Forest School of Medicine and at NASA. Pre- and post-flight T1-weighted MRI scans of the cervical regions were acquired using a Siemens Magnetom Verio 3T scanner for six crewmembers (average age, 48 ± 4.8 years) on expeditions lasting 166 ± 14.8 days in duration (NASA, 2017).
To ensure accurate comparison of pre- and post- flight neck muscle CSA, consistent measurement location was used. Our image analysis protocol accounted for inconsistencies in lordotic curvature during patient positioning. Moreover, a decrease in lordotic curvature has been associated with spaceflight, and our measurement techniques were adjusted to correct for these changes (Stemper et al., 2010). Prior studies have made these corrections by aligning the axial view parallel to each intervertebral disc during scan acquisition (Stemper et al., 2010). Since the MRI scans were collected retrospectively in our study, we employed a method for reslicing the MRI scans, using Mimics software (v20, Materialise, Leuven, Belgium), to allow for consistent measurements. The Mimics reslicing tool axially resliced the MRI images in the direction of a user defined curve. To orient the axial view parallel to the intervertebral discs, a curve was created in the sagittal view which passed through the centroids of each vertebra and traveled perpendicularly through the intervertebral discs (Figure 1A). When resliced along this curve, the newly created axial view aligned parallel to the intervertebral discs at every vertebral level (Figure 1B). To ensure a consistent reslicing method between scans, the resliced curve was generated using the sagittal slice cutting through the center of the spinal cord at the C2-C3 intervertebral disc level. This vertebral level was chosen since the retrospective MRI scans were originally aligned parallel to the C2-C3 intervertebral disc per the scanning protocol.
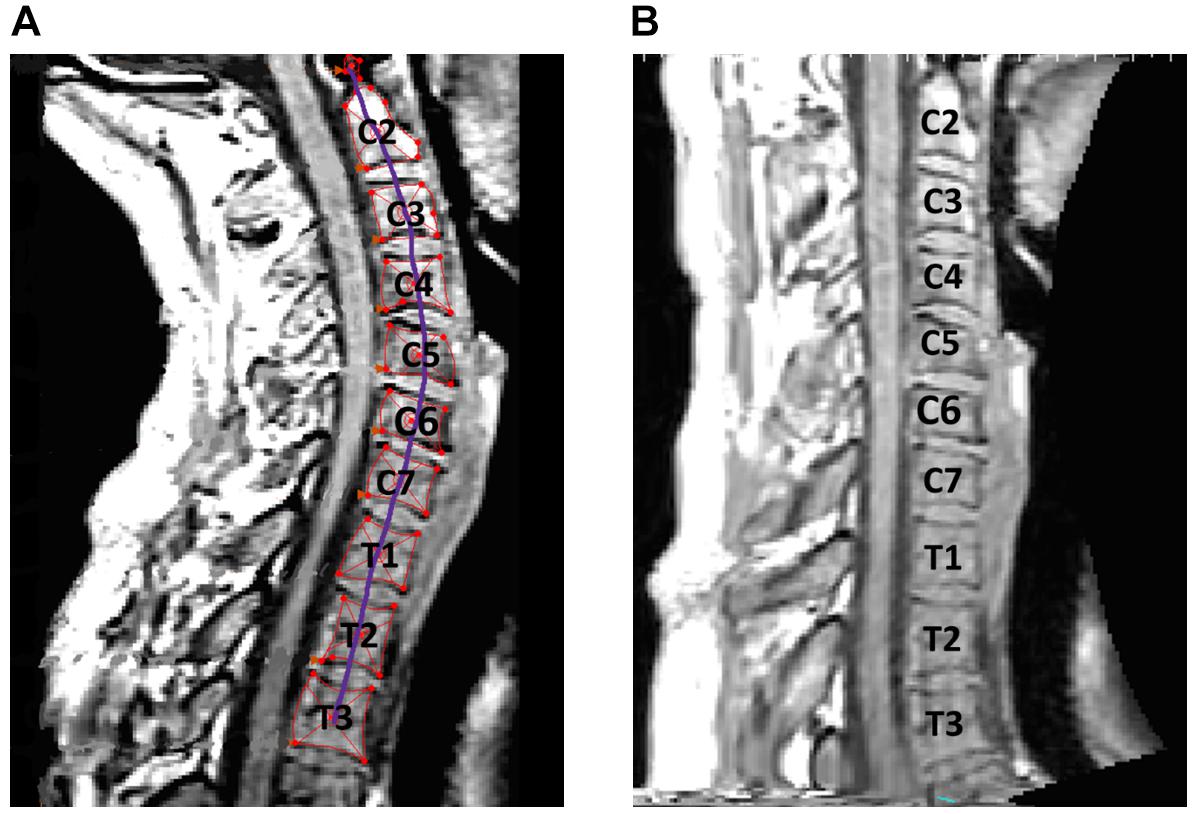
Figure 1. (A) Centroids for each cervical vertebra were determined. (B) Spline curve through centroids was used to produce a re-sliced scan that standardized neck curvature across subjects.
After reslicing the scans, the neck muscle CSA measurements were collected using the Mimics measurement tool. This tool uses an algorithm based on Hounsfield Unit values to interpolate muscle boundaries between manually selected points. Several points were chosen along the perimeter of each neck muscle to create a smooth, accurate muscle boundary and determine muscle CSA.
To ensure accurate comparison of muscle CSA from pre- to post-flight scans, the vertebral level at which the measurements were made was based on previous literature (Elliott et al., 2007; Fernandez-de-Las-Penas et al., 2007; Oksanen et al., 2008; Stemper et al., 2010). Muscles not measured in previous studies were measured at the same vertebral level as the neighboring muscles or at the most inferior intervertebral disc present on all scans (T1-T2). Twelve cervical muscles were measured at one of four measurement locations shown in Figure 2 and Table 1. Measurements were made on both the right and left sides of each muscle, providing a total of 12 pre-flight and 12 post-flight samples for each muscle across the six crewmembers. The mean pre- to post-flight percent change for each muscle was calculated by summing the individual changes and dividing by the sample size (n = 12).
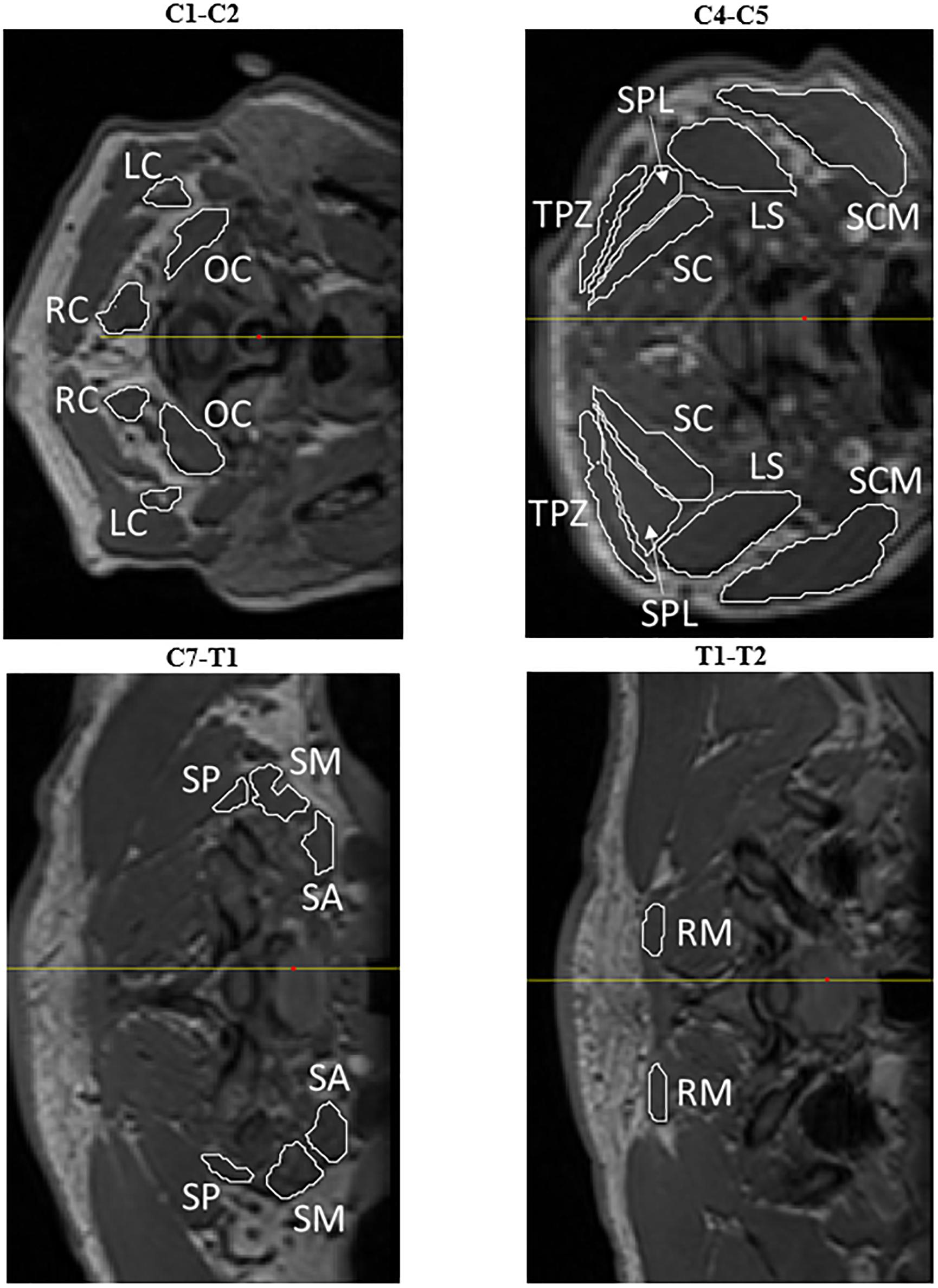
Figure 2. Cross-sectional measurements were acquired at specific intervertebral levels for the longissimus capitis (LC), obliquus capitis inferior (OC), rectus capitis posterior major (RC), sternocleidomastoid (SCM), levator scapulae (LS), trapezius (TPZ), semispinalis capitis (SC), splenius (SPL), scalenus posterior (SP), scalenus medius (SM), scalenus anterior (SA), and rhomboid minor (RM).
Muscle fat infiltration (MFI) was also measured in Mimics (Figure 3). Muscles were segmented in a single slice, creating regions of interests for each muscle at the same vertebral level as the aforementioned CSA measurement. The segmented pixels across the CSA of each muscle were then automatically aggregated and the mean pixel intensity (MPI) extracted. At each examined vertebral level, a selection of subcutaneous fat was also analyzed and the MPI extracted. The MFI was then reported as a ratio of muscle MPI to fat MPI multiplied by 100 to yield an estimate of percent fat content in the muscle, as shown in Equation 1 (Elliott et al., 2005; Valentin et al., 2015).
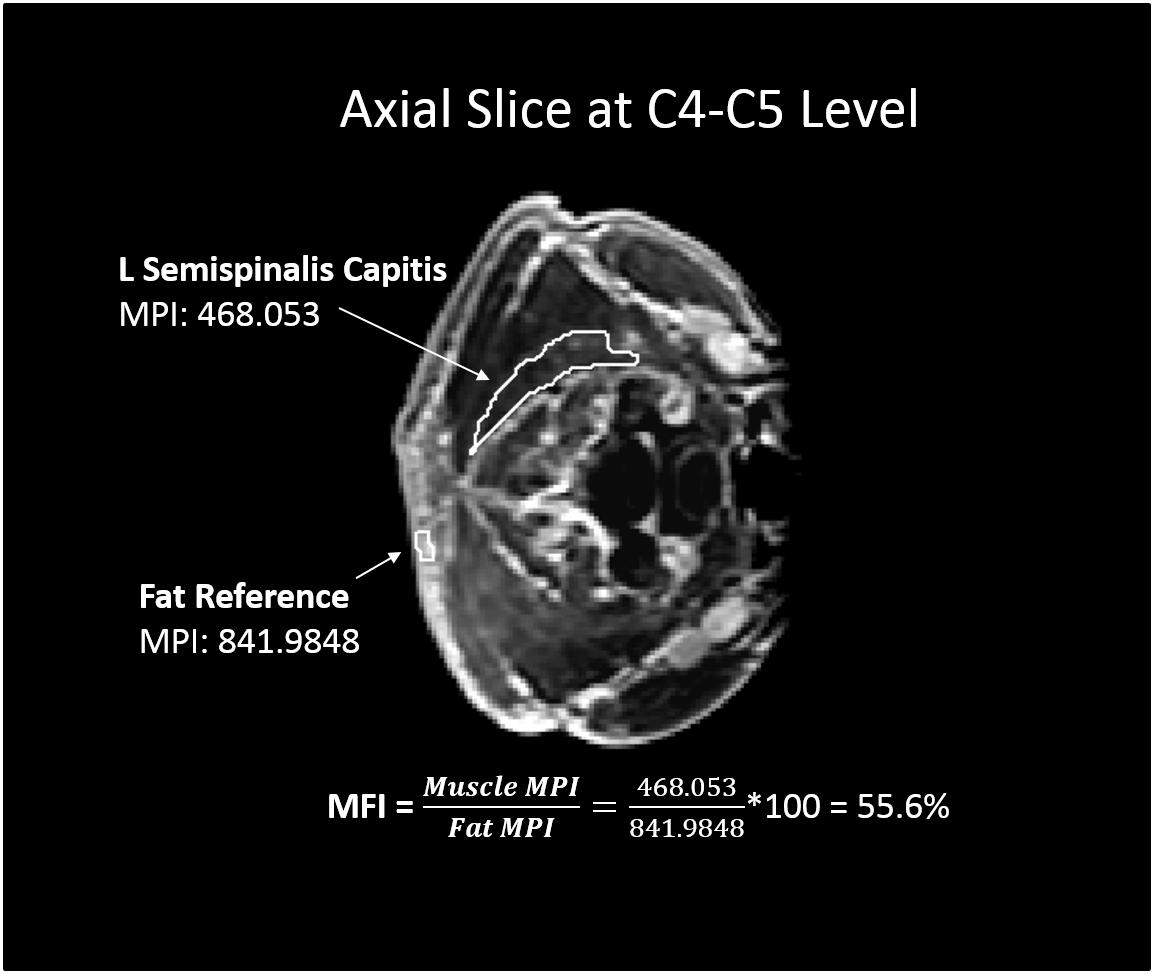
Figure 3. Example of muscle fat infiltration (MFI) calculation. White outlined regions of interest represent a muscle (left semispinalis capitis) and subcutaneous fat reference used for the MFI measurement at the C4-C5 vertebral level. The resulting MFI calculation is shown, in which the mean pixel intensity (MPI) of muscle was reported as a percentage of the reference fat MPI.
Statistical analysis was performed using SAS (Version 9.4. SAS Institute Inc., Cary, NC, United States) using a 0.05 level of significance. Shapiro-Wilk w tests were performed to assess the distribution of data. To account for the repeated measures by subject and side, for each of the outcomes of interest (CSA and MFI), mixed models with a random side effect with an unstructured covariance matrix were used to model changes from pre- to post-spaceflight, and allowing for separate residual variance for each muscle type. This model allows for correlation between measures on the same side and between the left and right sides for each subject. Comparisons between the neck muscle CSA changes in this study and historical volumetric bed rest studies with resistive exercise were performed according to the calculations outlined by Whitley and Ball (2002) to compare two means arising from unpaired data.
A subset of four crewmembers had in-flight exercise logs that documented their time using the cycle ergometer with vibration isolation and stabilization system (CEVIS), time using a treadmill, as well as frequency of aRED usage. These crewmembers also had pre- and post-functional fitness assessments consisting of peak trunk flexion torque. Crewmembers also had pre- and post-fitness assessments for maximum pushups, situps, and pullups performed in two minutes, maximum fingertip distance during a sit and reach exercise, and maximum weight lifted while performing smith bench presses and leg presses. No logs of in-flight nutrition were available in this subset.
Shapiro-wilk tests were performed on all exercise frequencies and trunk flexion torque changes to evaluate for normally distributed results. Trunk flexion torque was individually linearly regressed against lumbopelvic muscle changes to assess for any trunk flexion torque changes as a result of radiologically observed findings. Lumbopelvic muscle changes with normal distributions were individually linearly regressed against CEVIS (min/day), treadmill (min/day), and aRED (uses/day) to evaluate for significant changes resulting from crewmembers’ choice of in-flight fitness routine. These values were determined by taking the total minutes spent using CEVIS or treadmill and total flight use of aRED and normalizing by the mission duration in days.
Results
Pre- to post-flight changes in the muscle CSA are shown in Figure 4 and Table 2. The Shapiro-Wilk w test confirmed a normal distribution of all neck muscle changes (p > 0.05). Post-flight increases in muscle CSA were observed in the semispinalis capitis (mean: 11.5%, SE: 4.4%, p = 0.0106), sternocleidomastoid (mean: 9.0%, SE: 2.3%, p = 0.0001), trapezius (mean: 25.1%, SE: 9.9%, p = 0.0125), and the rhomboid minor (mean: 23.1%, SE: 11.7%, p = 0.0500).
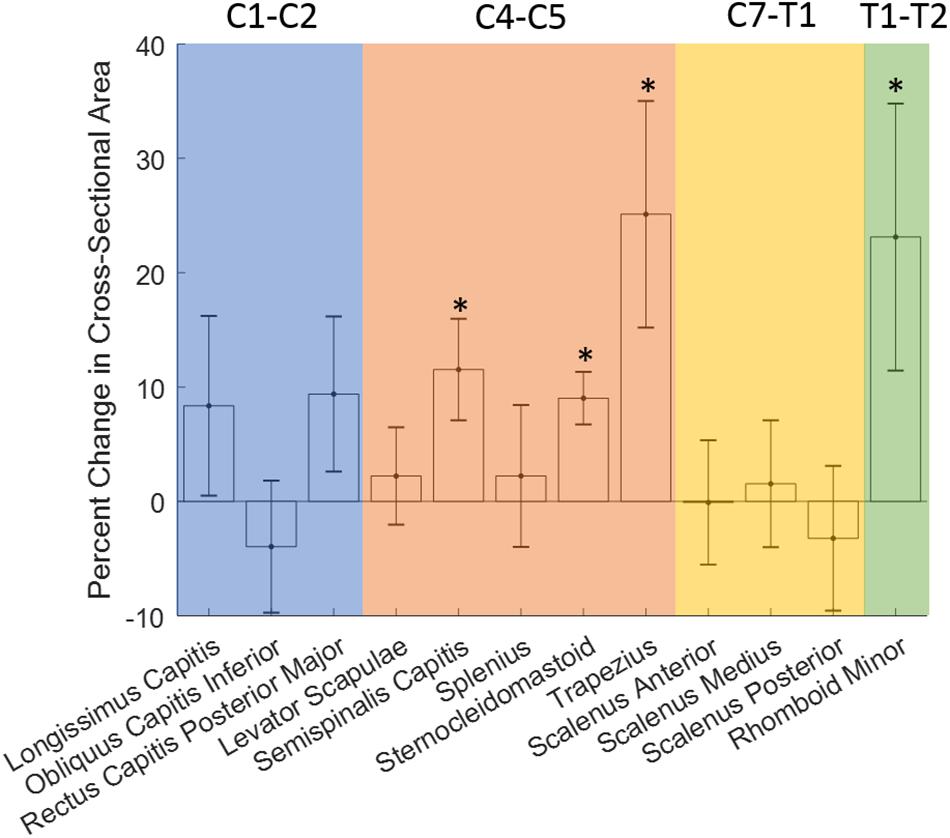
Figure 4. Changes in neck muscle cross-sectional area (CSA) with long-duration spaceflight. Error bar denotes standard error. ∗Denotes significance with p < 0.05.
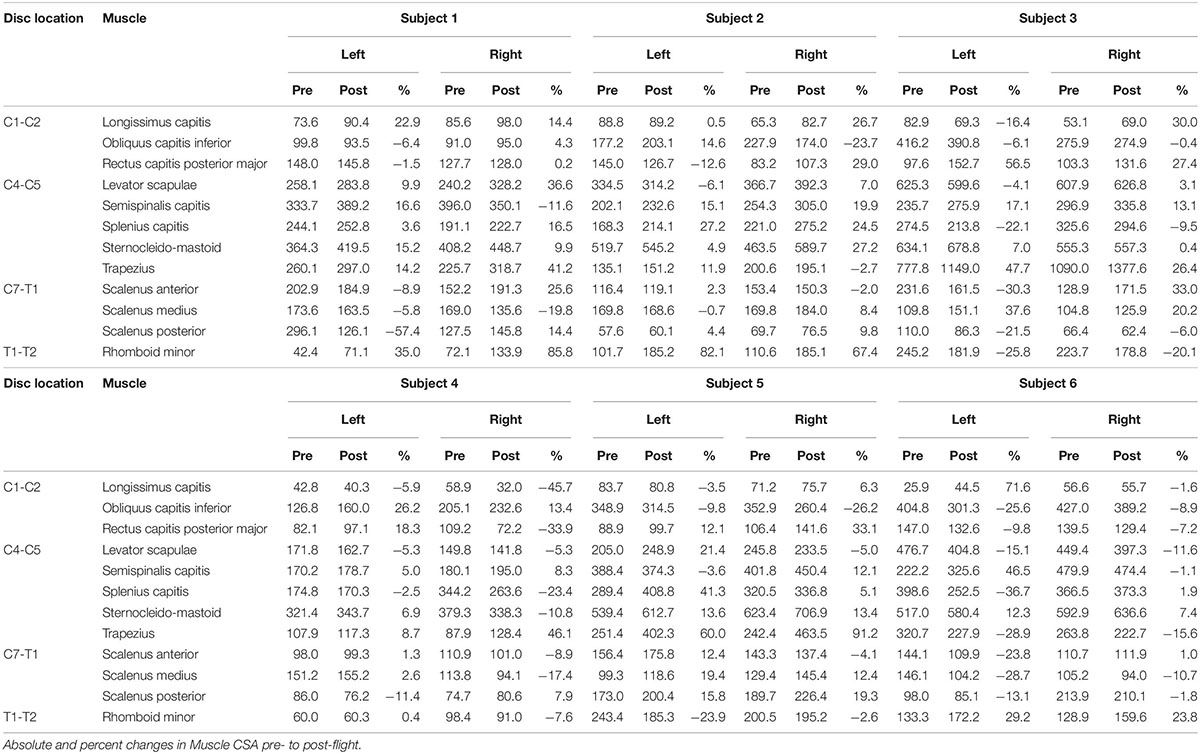
Table 2. Absolute (mm2) and percent changes in muscle cross-sectional area (CSA) for the six crewmembers (Subject 1–Subject 6).
Three of six crewmembers had increases in CSA for both semispinalis capitis muscles following spaceflight ranging from 5.0 to 19.9%. The remaining three crewmembers had one semispinalis capitis muscle with an increase and one with a decrease in CSA following spaceflight of −1.1 to −11.6% on one side, and increases from 12.1 to 46.5% on the other side.
Five of the six crewmembers had increases in CSA for both sternocleidomastoid muscles following spaceflight ranging from 0.4% to 15.2%. The remaining crewmember had one sternocleidomastoid muscle with an increase (6.9%) and one with a decrease (−10.8%) in CSA following spaceflight.
Four of the six crewmembers (67%) had increases in CSA for both trapezius muscles following spaceflight ranging from 8.7 to 91.2%. One crewmember had one trapezius muscle with an increase (11.9%) and one with a decrease (−2.7%) in CSA following spaceflight. One crewmember had decreases in CSA for both trapezius muscles following spaceflight ranging from −15.6 to −28.9%.
Three of the six crewmembers had increases in CSA for both rhomboid minor muscles following spaceflight ranging from 23.8 to 85.8%. Two of the six crewmembers had decreases in CSA for both rhomboid minor muscles following spaceflight ranging from −2.6 to −25.8%. One crewmember had one trapezius muscle with an increase (0.4%) and one with a decrease (−7.6%) in CSA following spaceflight.
Neck muscles with non-significant CSA changes included the longissimus capitis (mean: 8.4%, 7.9% SE), obliquus capitis inferior (mean: −4.0%, 5.8% SE), rectus capitis posterior major (mean: 9.4%, 6.8% SE), levator scapulae (mean: 2.2%, 4.3% SE), splenius capitis (mean: 2.2%, 6.2% SE), scalenus anterior (mean: −0.1%, 5.4% SE), scalenus medius (mean: 1.5%, 5.5% SE), and scalenus posterior (mean: −3.2%, 6.3% SE).
Linear fits of the CSA changes in sternocleidomastoid vs. trapezius, sternocleidomastoid vs. semispinalis capitis, sternocleidomastoid vs. rhomboid minor, trapezius vs. semispinalis capitis, trapezius vs. rhomboid minor, and semispinalis capitis vs. rhomboid minor all resulted in statistically non-significant trends (α = 0.05).
None of the muscles analyzed showed stastistically significant changes in fatty infiltration with spaceflight (Figure 5 and Table 3).
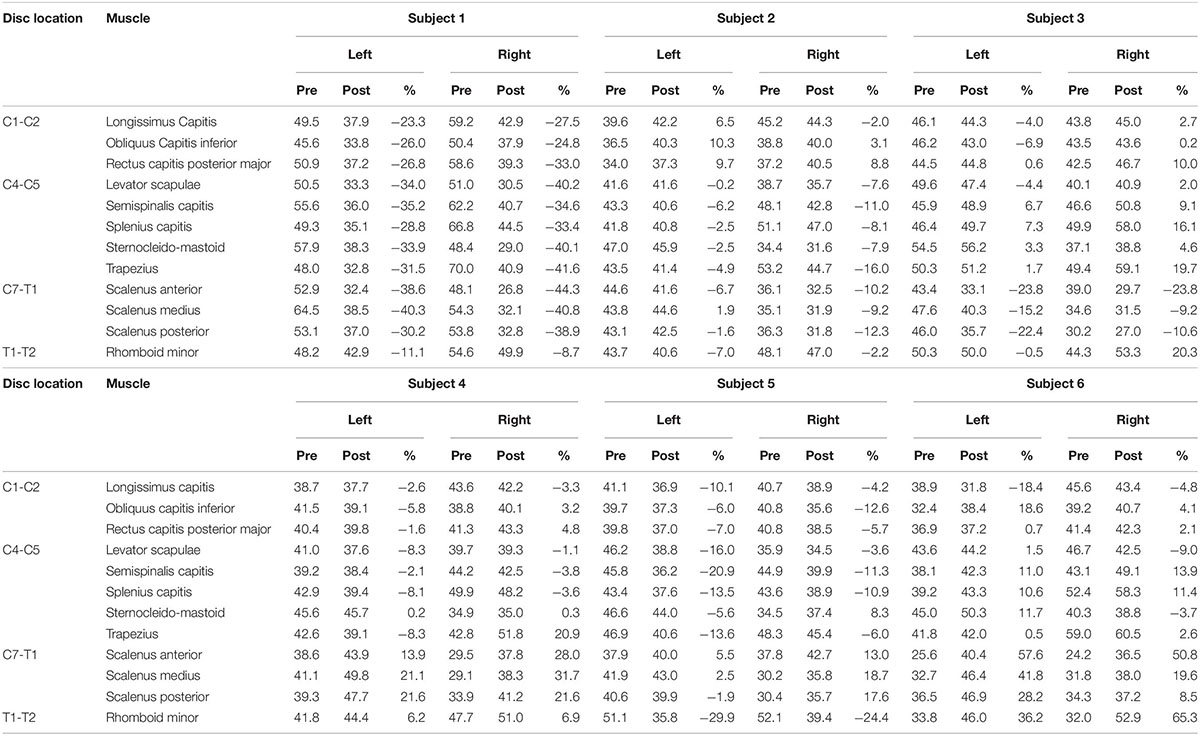
Table 3. Absolute and percent changes in muscle fat infiltration (MFI) for the six crewmembers (Subject 1–Subject 6).
No significant regression equations were found to predict muscle CSA percent change based on the mission duration (n = 4, α = 0.05). However, there was a trend toward significance in that crewmembers who spent longer time at the ISS tended to have larger increases in their semispinalis capitis muscle CSA equivalent to roughly a 2% additional increase per every five days of extra time on the ISS (R2 = 0.88, p < 0.1) (Figure 6).
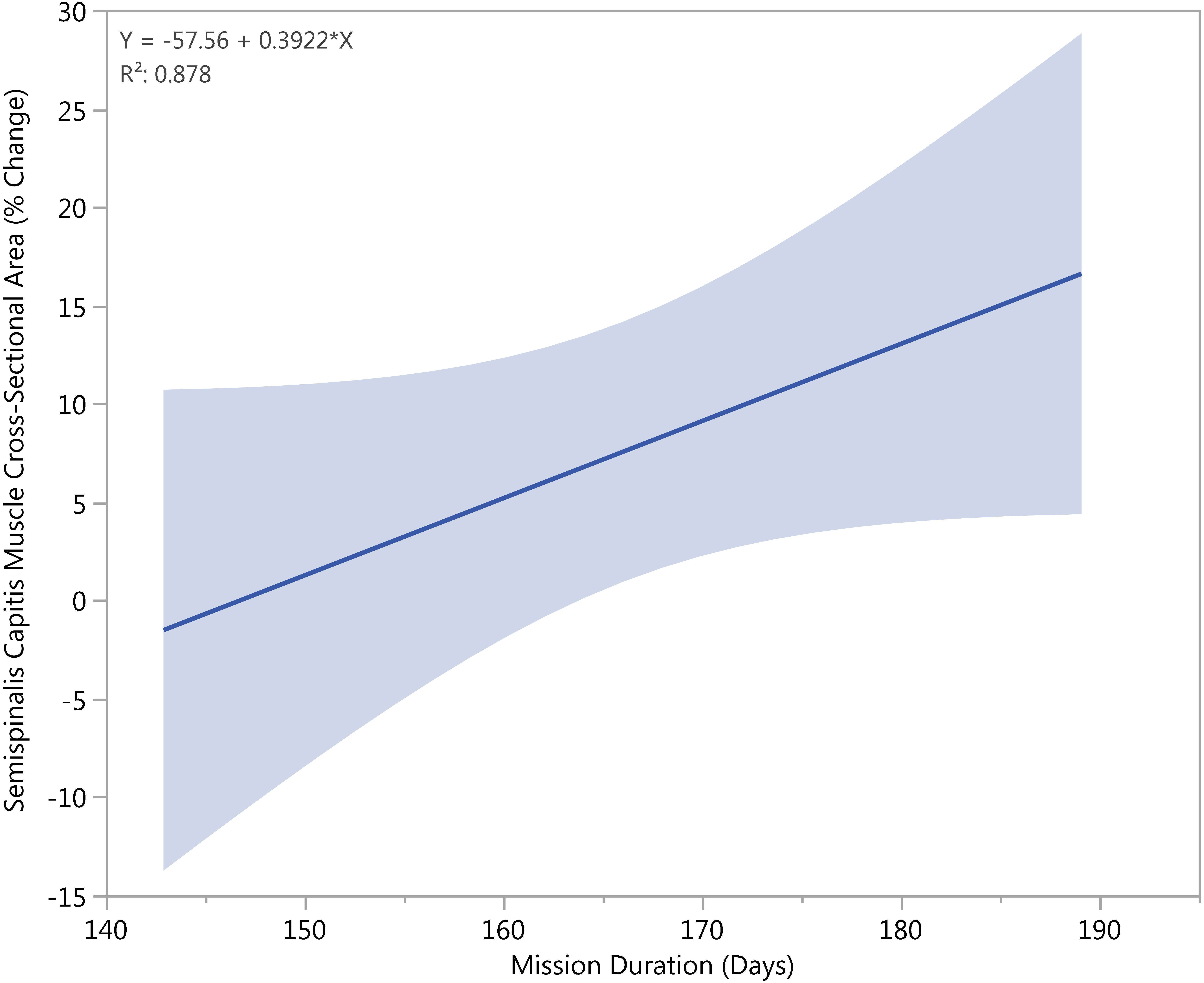
Figure 6. Longer mission durations correlated with increased growth of the semispinalis capitis CSA (p < 0.05).
No significant regression equation to predict trunk flexion strength based on the changes in muscle CSA was found (n = 4, α = 0.05). However, there was a trend toward significance in that crewmembers who had larger increases in their trapezius tended to better preserve their truncal strength compared to their peers. For every 3% increase in trapezius muscle area, a crewmember tended to have an additional 1% preserved truncal strength compared to their peers (R2 = 0.94, p < 0.1) (Figure 7).
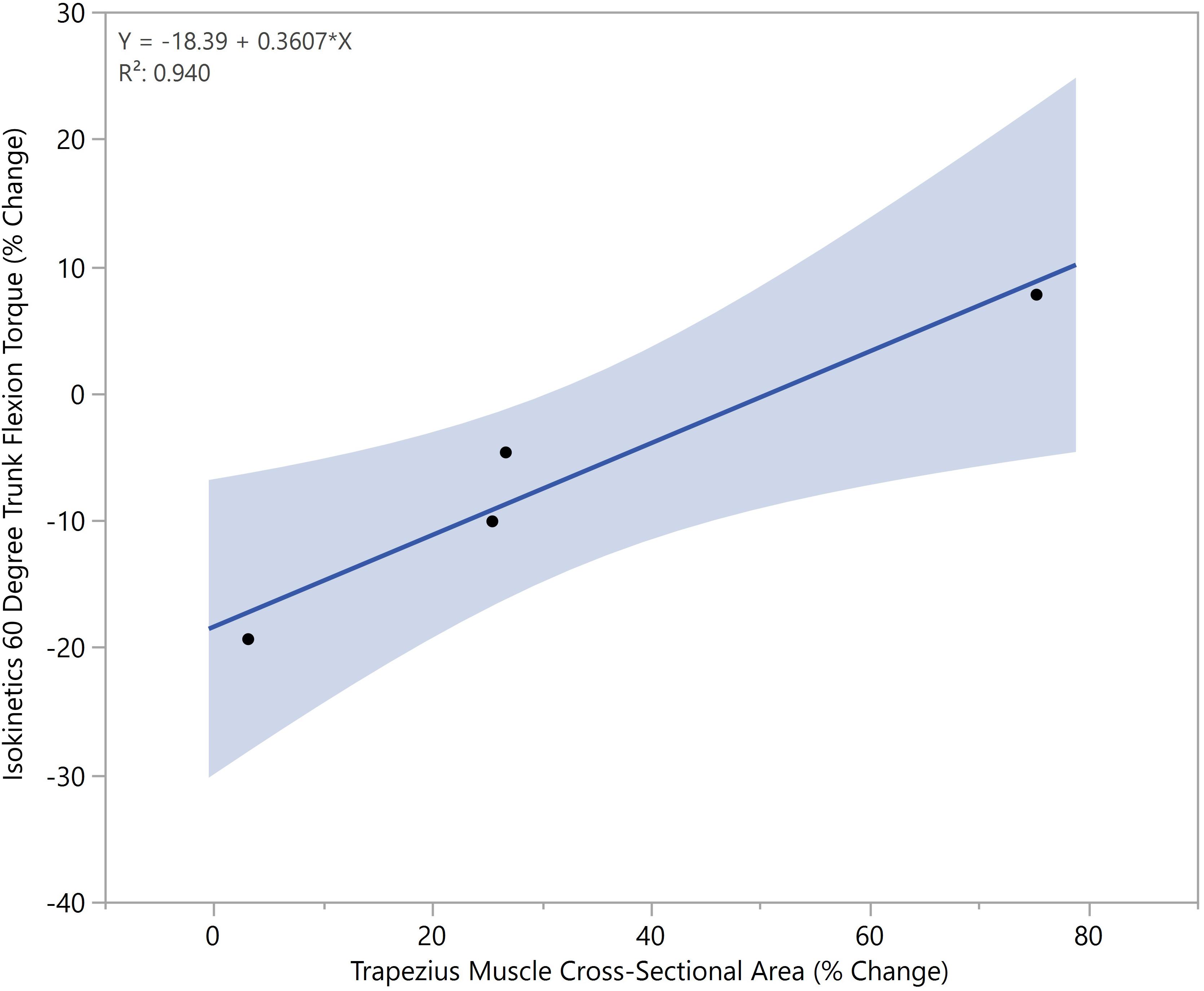
Figure 7. Increased trapezius cross-sectional areas correlated with increased preservation of truncal strength (p < 0.05).
There were no other trends seen when comparing muscle changes to mission duration or isokinetic strength changes.
Discussion
The results of this study support prior studies that found long-duration spaceflight does not lead to atrophy of the neck muscles of astronauts. The study shows the degree to which different neck muscles are affected on current ISS missions.
The novel finding of our study was the increase in the trapezius, semispinalis capitis, rhomboid minor, and sternocleidomastoid CSA. This may be attributed to the constant daily use of these particular neck muscles during spaceflight; however, further studies would be needed to test this hypothesis. It is also possible that the neck muscle hypertrophy occured because the muscles are not as heavily loaded on Earth as lower back and limb musculature, decreasing the potential for atrophy (Belavý et al., 2013). Collectively, these three muscle groups are responsible for the flexion, extension, and torsion movements of the neck that are required for nearly all everyday tasks on the ISS and for maintaining an upright positioning of the head. Furthermore, a lack of gravitational pull could weaken the vestibular nuclei’s sense of balance and require more activity of the neck muscles to move the head in order to take in visual input to compensate. As such, it is reasonable to assume that these muscles are adequately maintained or even strengthened during spaceflight.
These data compare favorably with a volumetric analysis of neck musculature during two months of bed rest with a resistive exercise intervention (Belavý et al., 2013). The semispinalis capitis, longissimus capitis, splenius capitis, levator scapulae, sternocleidomastoid, and the anterior and posterior scalenes all had similar changes or lack thereof. However, the astronaut population had a decrease in posterior scalene size, unlike the bed rest study, although neither study found statistical significance in those results. These comparisons lend credence to the revised hypothesis that lack of spinal loading is driving the enlargement of neck muscles seen in this study since that was the main commonality between the two study setups.
Conditions of decreased muscle strength such as sarcopenia lead to increased mortality and decreased quality of life (Boutin et al., 2015, 2017; Lenchik et al., 2018; Murea et al., 2018). Astronauts are prone to sarcopenia manifesting as increased fatigability and decreased strength of muscles (Thornton and Rummel, 1977; Cruz-Jentoft et al., 2010). Muscle size is just one metric to evaluate muscle strength. Bed rest studies have also shown decreases in type I and type II myofiber CSAs (Bamman et al., 1998). Aging and muscle-wasting diseases have shown a relationship between muscle strength and fat infiltration of muscle (Jones et al., 1983; Liu et al., 1993; Ryan and Nicklas, 1999; Goodpaster et al., 2001; Visser et al., 2002). Investigations into the fat content of neck muscles as well as curvature and intervertebral disc changes may help explain the persistence of herniated nucleus pulposus risk despite preserved muscle size (Johnston et al., 2010; Bailey et al., 2018; Green and Scott, 2018). While MFI was included in this analysis, none of the changes with spaceflight were found to be significant. At this time, the overall results of this study describe the areal changes seen in the neck muscles, but do not explain the persistence of spinal pain and injury in the astronaut population. Further investigation would be necessary to compare these spinal CSA changes to functional changes in the muscles studied.
In-flight countermeasures are thought to aid in the prevention of post-flight injuries (Sayson et al., 2013). However, aRED lacks an exercise protocol that mimics terrestrial cervical spine loading (Sayson et al., 2013), which may explain why spinal pain and herniated discs persist despite the introduction of resistive exercise on ISS missions (Laughlin et al., 2016). NASA’s Astronaut Strength, Conditioning, and Rehabilitation team focuses on the optimization of in-flight exercise regimens as well as post-flight rehabilitation to mitigate the risks of these spinal injuries. While the results of this study demonstrate maintenance of the neck muscles onboard ISS, the risk of spinal injuries persist. The inclusion of in-flight pharmacologic agents could be used to aid in the maintenance of muscle health to offset in-flight musculoskeletal changes and to improve the rates of recovery to baseline values (LeBlanc et al., 2002; Zhang et al., 2011; Smith et al., 2012; Leblanc et al., 2013; Lloyd et al., 2015; MacNabb et al., 2016; Lang et al., 2017; Ramachandran et al., 2018). While many pharmacotherapies such as bisphosphonates, osteoprotegerin, sclerostin antibodies, and parathyroid hormone have been proposed to quell bone loss, a few pharmacotherapies are being studied to prevent muscle atrophy as well (Ramachandran et al., 2018). These include myostatin inhibitors to encourage muscle proliferation and peptide supplements to discourage muscle atrophy (Ramachandran et al., 2018). It is important to continue to quantify the current effects of ISS missions on neck musculature when evaluating the efficacy of in-flight interventions.
Limitations
Study limitations included a small sample size for both the overall study (n = 6), and the sub-study using exercise logs and trunk flexion torque changes (n = 4). However, there were only 18 crewmembers on ISS missions during the data collection period. The study was also limited by the preponderance of male ISS crewmembers during the data collection period. Finally, the study was limited by the MRI scan parameters which did not allow for a comprehensive evaluation of MFI. Ideally, studies should include chemical-shift imaging methods such as the Dixon technique to capture water- and fat-only images that can be utilized to more accurately evaluate muscle fat content (Crawford et al., 2016). The available scan data only made it possible to determine atrophy or hypertrophy of a muscle rather than muscle quality or muscle strength. Despite these limitations, statistically significant changes were found in four of the 12 muscles analyzed. Future studies by our research team will address these limitations by actively recruiting a more representative sample and combining both MRI and computed tomography techniques to evaluate both muscle composition and size.
Conclusion
Using pre- and post-flight MRI scan analysis, we quantified changes in neck musculature in crewmembers of long-duration space missions during the aRED era. None of the muscles showed statistically significant decreases in their CSAs as a result of prolonged microgravity. In addition, post-flight hypertrophy was observed in the semispinalis capitis, sternocleidomastoid, trapezius, and rhomboid minor muscles. Future studies should include assessments of changes in intervertebral disc heights, cervical curvature, and vertebral bone density, and cortical thickness in order to create a more robust picture of how microgravity places astronauts at increased risk for spine injury. Eventually, future studies could allow for in-flight interventions that target musculoskeletal changes and mitigate the risk of injury.
Data Availability
The datasets for this study will not be made publicly available because MRI scans were obtained from the Life Sciences Data Archive and Lifetime Surveillance of Astronaut Health project.
Author Contributions
LL, JT, and AW designed the research. KM, KG, JT, JD, and KK performed the data collection, data analysis, and statistical analysis. LL and AW interpreted the data. KM, KG, JT, LL, and AW wrote and revised the manuscript. AW had primary responsibility for final content. All authors listed have made a substantial, direct and intellectual contribution to the work, and approved it for publication.
Funding
This study was supported by the NASA Human Research Program (Award No. NNX16AP89G) and the National Science Foundation [REU Site: Imaging and Mechanics-Based Projects on Accidental Cases of Trauma (IMPACT), Award No. 1559700].
Conflict of Interest Statement
The authors declare that the research was conducted in the absence of any commercial or financial relationships that could be construed as a potential conflict of interest.
References
Adams, G. R., Caiozzo, V. J., and Baldwin, K. M. (2003). Skeletal muscle unweighting: spaceflight and ground-based models. J. Appl. Physiol. 95, 2185–2201. doi: 10.1152/japplphysiol.00346.2003
Bailey, J. F., Miller, S. L., Khieu, K., O’Neill, C. W., Healey, R. M., Coughlin, D. G., et al. (2018). From the international space station to the clinic: how prolonged unloading may disrupt lumbar spine stability. Spine J. 18, 7–14. doi: 10.1016/j.spinee.2017.08.261
Bamman, M. M., Clarke, M. S., Feeback, D. L., Talmadge, R. J., Stevens, B. R., Lieberman, S. A., et al. (1998). Impact of resistance exercise during bed rest on skeletal muscle sarcopenia and myosin isoform distribution. J. Appl. Physiol. 84, 157–163. doi: 10.1152/jappl.1998.84.1.157
Belavý, D. L., Miokovic, T., Armbrecht, G., and Felsenberg, D. (2013). Hypertrophy in the cervical muscles and thoracic discs in bed rest? J. Appl. Physiol. 115, 586–596. doi: 10.1152/japplphysiol.00376.2013
Boutin, R. D., Bamrungchart, S., Bateni, C. P., Beavers, D. P., Beavers, K. M., Meehan, J. P., et al. (2017). CT of patients with hip fracture: muscle size and attenuation help predict mortality. AJR Am. J. Roentgenol. 208, W208–W215. doi: 10.2214/AJR.16.17226
Boutin, R. D., Yao, L., Canter, R. J., and Lenchik, L. (2015). Sarcopenia: current concepts and imaging implications. AJR Am. J. Roentgenol. 205, W255–W266. doi: 10.2214/AJR.15.14635
Burkhart, K., Allaire, B., and Bouxsein, M. (2018). Negative effects of long-duration spaceflight on paraspinal muscle morphology. Spine 44, 879–886. doi: 10.1097/BRS.0000000000002959
Crawford, R. J., Filli, L., Elliott, J. M., Nanz, D., Fischer, M. A., Marcon, M., et al. (2016). Age- and Level-dependence of fatty infiltration in lumbar paravertebral muscles of healthy volunteers. AJNR Am. J. Neuroradiol. 37, 742–748. doi: 10.3174/ajnr.A4596
Cruz-Jentoft, A. J., Baeyens, J. P., Bauer, J. M., Boirie, Y., Cederholm, T., Landi, F., et al. (2010). Sarcopenia: european consensus on definition and diagnosisreport of the european working group on sarcopenia in older people. Age Ageing 39, 412–423. doi: 10.1093/ageing/afq034
De Loose, V., Van den Oord, M., Keser, I., Burnotte, F., Van Tiggelen, D., Dumarey, A., et al. (2009). MRI study of the morphometry of the cervical musculature in F-16 pilots. Aviat. Space Environ. Med. 80, 727–731. doi: 10.3357/asem.2389.2009
Elliott, J. M., Galloway, G. J., Jull, G. A., Noteboom, J. T., Centeno, C. J., and Gibbon, W. W. (2005). Magnetic resonance imaging analysis of the upper cervical spine extensor musculature in an asymptomatic cohort: an index of fat within muscle. Clin. Radiol. 60, 355–363. doi: 10.1016/j.crad.2004.08.013
Elliott, J. M., Jull, G., Noteboom, J., Durbridge, G., and Gibbon, W. (2007). Magnetic resonance imaging study of cross-sectional area of the cervical extensor musculature in an asymptomatic cohort. Clin. Anat. 20, 35–40. doi: 10.1002/ca.20252
Fernandez-de-Las-Penas, C., Bueno, A., Ferrando, J., Elliott, J., Cuadrado, M., and Pareja, J. (2007). Magnetic resonance imaging study of the morphometry of cervical extensor muscles in chronic tension-type headache. Cephalalgia 27, 355–362. doi: 10.1111/j.1468-2982.2007.01293.x
Goodpaster, B. H., Carlson, C. L., Visser, M., Kelley, D. E., Scherzinger, A., Harris, T. B., et al. (2001). Attenuation of skeletal muscle and strength in the elderly: the health ABC study. J. Appl. Physiol. 90, 2157–2165. doi: 10.1152/jappl.2001.90.6.2157
Green, D. A., and Scott, J. P. (2018). Spinal health during unloading and reloading associated with spaceflight. Front. Physiol. 8:1126. doi: 10.3389/fphys.2017.01126
Johnston, S. L., Campbell, M. R., Scheuring, R., and Feiveson, A. H. (2010). Risk of herniated nucleus pulposus among US astronauts. Aviat. Space Environ. Med. 81, 566–574. doi: 10.3357/asem.2427.2010
Jones, D., Round, J., Edwards, R., Grindwood, S., and Tofts, P. (1983). Size and composition of the calf and quadriceps muscles in duchenne muscular dystrophy: a tomographic and histochemical study. J. Neurol. Sci. 60, 307–322. doi: 10.1016/0022-510x(83)90071-0
Lang, T., LeBlanc, A., Evans, H., Lu, Y., Genant, H., and Yu, A. (2004). Cortical and trabecular bone mineral loss from the spine and hip in long-duration spaceflight. J. Bone Miner. Res. 19, 1006–1012. doi: 10.1359/JBMR.040307
Lang, T., Van Loon, J. J., Bloomfield, S., Vico, L., Chopard, A., Rittweger, J., et al. (2017). Towards human exploration of space: the THESEUS review series on muscle and bone research priorities. NP J. Microgravity 3:8. doi: 10.1038/s41526-017-0013-0
Laughlin, M. S., Murray, J. D., Wear, M. L., and Van Baalen, M. (2016). “Post-flight back pain following international space station missions: evaluation of spaceflight risk factors,” in Proceddings of the Human Research Program Investigators’ Workshop, Galveston, TX.
LeBlanc, A., Lin, C., Shackelford, L., Sinitsyn, V., Evans, H., Belichenko, O., et al. (2000a). Muscle volume, MRI relaxation times (T2), and body composition after spaceflight. J. Appl. Physiol. 89, 2158–2164. doi: 10.1152/jappl.2000.89.6.2158
LeBlanc, A., Schneider, V., Shackelford, L., West, S., Oganov, V., Bakulin, A., et al. (2000b). Bone mineral and lean tissue loss after long duration space flight. J. Musculoskelet. Neuronal Interact. 1, 157–160.
Leblanc, A., Matsumoto, T., Jones, J., Shapiro, J., Lang, T., Shackelford, L., et al. (2013). Bisphosphonates as a supplement to exercise to protect bone during long-duration spaceflight. Osteoporos. Int. 24, 2105–2114. doi: 10.1007/s00198-012-2243-z
LeBlanc, A., Shackelford, L., and Schneider, V. (1998). Future human bone research in space. Bone 22(Suppl. 5), 113S–116S. doi: 10.1016/s8756-3282(98)00013-1
LeBlanc, A. D., Driscol, T. B., Shackelford, L. C., Evans, H. J., Rianon, N. J., Smith, S. M., et al. (2002). Alendronate as an effective countermeasure to disuse induced bone loss. J. Musculoskelet. Neuronal Interact. 2, 335–343.
LeBlanc, A. D., Schneider, V. S., Evans, H. J., Pientok, C., Rowe, R., and Spector, E. (1992). Regional changes in muscle mass following 17 weeks of bed rest. J. Appl. Physiol. 73, 2172–2178. doi: 10.1152/jappl.1992.73.5.2172
Lenchik, L., Lenoir, K. M., Tan, J., Boutin, R. D., Callahan, K. E., Kritchevsky, S. B., et al. (2018). Opportunistic measurement of skeletal muscle size and muscle attenuation on computed tomography predicts 1-year mortality in medicare patients. J. Gerontol. A Biol. Sci. Med. Sci. [Epub ahead of print].
Liu, M., Chino, N., and Ishihara, T. (1993). Muscle damage progression in Duchenne muscular dystrophy evaluated by a new quantitative computed tomography method. Arch. Phys. Med. Rehabil. 74, 507–514. doi: 10.1016/0003-9993(93)90115-q
Lloyd, S. A., Morony, S. E., Ferguson, V. L., Simske, S. J., Stodieck, L. S., Warmington, K. S., et al. (2015). Osteoprotegerin is an effective countermeasure for spaceflight-induced bone loss in mice. Bone 81, 562–572. doi: 10.1016/j.bone.2015.08.021
MacNabb, C., Patton, D., and Hayes, J. (2016). Sclerostin antibody therapy for the treatment of osteoporosis: clinical prospects and challenges. J. Osteoporos. 2016, 1–22. doi: 10.1155/2016/6217286
McNamara, K. P., Greene, K. A., Moore, A. M., Lenchik, L., and Weaver, A. A. (2019). Lumbopelvic muscle changes following long-duration spaceflight. Front. Physiol. 10:627. doi: 10.3389/fphys.2019.00627
Muir, J., Judex, S., Qin, Y. X., and Rubin, C. (2011). Postural instability caused by extended bed rest is alleviated by brief daily exposure to low magnitude mechanical signals. Gait Posture 33, 429–435. doi: 10.1016/j.gaitpost.2010.12.019
Murea, M., Lenchik, L., Register, T. C., Russell, G. B., Xu, J., Smith, S. C., et al. (2018). Psoas and paraspinous muscle index as a predictor of mortality in African American men with type 2 diabetes mellitus. J. Diabetes Complicat. 32, 558–564. doi: 10.1016/j.jdiacomp.2018.03.004
NASA, (2017). Sonographic Astronaut Vertebral Examination (Spinal Ultrasound). Available at: https://www.nasa.gov/mission_pages/station/research/experiments/944.html [accessed July 31, 2017].
Oksanen, A., Erkintalo, M., Metsähonkala, L., Anttila, P., Laimi, K., Hiekkanen, H., et al. (2008). Neck muscles cross-sectional area in adolescents with and without headache–MRI study. Eur. J. Pain 12, 952–959. doi: 10.1016/j.ejpain.2008.01.006
Ploutz-Snyder, L., Ryder, J., English, K., Haddad, F., and Baldwin, K. (2015). “Risk of impaired performance due to reduced muscle mass, strength, and endurance (HRP-47072),” in Proceedings of the HRP HHC, (Houston, TX: NASA Johnson Space Center).
Ramachandran, V., Dalal, S., Scheuring, R. A., and Jones, J. A. (2018). Musculoskeletal Injuries in astronauts: review of pre-flight, in-flight, post-flight, and extravehicular activity injuries. Curr. Pathobiol. Rep. 6, 149–158. doi: 10.1007/s40139-018-0172-z
Ryan, A., and Nicklas, B. (1999). Age-related changes in fat deposition in mid-thigh muscle in women: relationships with metabolic cardiovascular disease risk factors. Int. J. Obes. 23:126. doi: 10.1038/sj.ijo.0800777
Sayson, J. V., Lotz, J., Parazynski, S., and Hargens, A. R. (2013). Back pain in space and post-flight spine injury: mechanisms and countermeasure development. Acta Astronaut. 86, 24–38. doi: 10.1016/j.actaastro.2012.05.016
Smith, S. M., McCoy, T., Gazda, D., Morgan, J. L., Heer, M., and Zwart, S. R. (2012). Space flight calcium: implications for astronaut health, spacecraft operations, and Earth. Nutrients 4, 2047–2068. doi: 10.3390/nu4122047
Stemper, B. D., Baisden, J. L., Yoganandan, N., Pintar, F. A., Paskoff, G. R., and Shender, B. S. (2010). Determination of normative neck muscle morphometry using upright MRI with comparison to supine data. Aviat. Space Environ. Med. 81, 878–882. doi: 10.3357/asem.2758.2010
Thornton, W. E., and Rummel, J. (1977). “Muscular deconditioning and its prevention in space flight,” in Biomed. Results from Skylab. NASA SP-377, eds R. S. Johnston, and L. F. Dietlein, (Houston, TX: NASA Johnson Space Center), 191–197.
Valentin, S., Licka, T., and Elliott, J. (2015). Age and side-related morphometric MRI evaluation of trunk muscles in people without back pain. Man. Ther. 20, 90–95. doi: 10.1016/j.math.2014.07.007
Visser, M., Kritchevsky, S. B., Goodpaster, B. H., Newman, A. B., Nevitt, M., Stamm, E., et al. (2002). Leg muscle mass and composition in relation to lower extremity performance in men and women aged 70 to 79: the health, aging and body composition study. J. Am. Geriatr. Soc. 50, 897–904. doi: 10.1046/j.1532-5415.2002.50217.x
Wood, S. J., Loehr, J. A., and Guilliams, M. E. (2011). Sensorimotor reconditioning during and after spaceflight. Neurorehabilitation 29, 185–195. doi: 10.3233/NRE-2011-2694
Keywords: advanced resistive exercise device, astronaut, cosmonaut, cross-sectional area, cervical muscle, microgravity, muscle hypertrophy, magnetic resonance imaging
Citation: McNamara KP, Greene KA, Tooze JA, Dang J, Khattab K, Lenchik L and Weaver AA (2019) Neck Muscle Changes Following Long-Duration Spaceflight. Front. Physiol. 10:1115. doi: 10.3389/fphys.2019.01115
Received: 27 February 2019; Accepted: 12 August 2019;
Published: 13 September 2019.
Edited by:
Ginés Viscor, University of Barcelona, SpainReviewed by:
Yoav Gimmon, Ben-Gurion University of the Negev, IsraelDaniel Belavy, Deakin University, Australia
Debora Angeloni, Sant’Anna School of Advanced Studies, Italy
Copyright © 2019 McNamara, Greene, Tooze, Dang, Khattab, Lenchik and Weaver. This is an open-access article distributed under the terms of the Creative Commons Attribution License (CC BY). The use, distribution or reproduction in other forums is permitted, provided the original author(s) and the copyright owner(s) are credited and that the original publication in this journal is cited, in accordance with accepted academic practice. No use, distribution or reproduction is permitted which does not comply with these terms.
*Correspondence: Ashley A. Weaver, asweaver@wakehealth.edu