- 1Department of Dermatology, Venereology and Allergology, University Medical Center and Medical Faculty Mannheim, Heidelberg University and Center of Excellence in Dermatology, Mannheim, Germany
- 2European Center for Angioscience, Medical Faculty Mannheim, Heidelberg University, Mannheim, Germany
- 3Next Generation Sequencing Core Facility, Medical Faculty Mannheim, Heidelberg University, Mannheim, Germany
- 4Division of Experimental Therapeutics, Graduate School of Medicine, Kyoto University, Kyoto, Japan
- 5Department of Cardiovascular Physiology, Medical Faculty Mannheim, Heidelberg University, Mannheim, Germany
- 6Section of Clinical and Molecular Dermatology, Department of Dermatology, Venereology and Allergology, University Medical Center and Medical Faculty Mannheim, Heidelberg University, Mannheim, Germany
Endothelial wingless-related integration site (Wnt)-/β-catenin signaling is a key regulator of the tightly sealed blood–brain barrier. In the hepatic vascular niche angiokine-mediated Wnt signaling was recently identified as an important regulator of hepatocyte function, including the determination of final adult liver size, liver regeneration, and metabolic liver zonation. Within the hepatic vasculature, the liver sinusoidal endothelial cells (LSECs) are morphologically unique and functionally specialized microvascular endothelial cells (ECs). Pathological changes of LSECs are involved in chronic liver diseases, hepatocarcinogenesis, and liver metastasis. To comprehensively analyze the effects of endothelial Wnt-/β-catenin signaling in the liver, we used endothelial subtype-specific Clec4g-iCre mice to generate hepatic ECs with overexpression of Ctnnb1. In the resultant Clec4g-iCretg/wt;Ctnnb1(Ex3)fl/wt (Ctnnb1OE−EC) mice, activation of endothelial Wnt-/β-catenin signaling resulted in sinusoidal transdifferentiation with disturbed endothelial zonation, that is, loss of midzonal LSEC marker lymphatic vessel endothelial hyaluronic acid receptor 1 (Lyve1) and enrichment of continuous EC genes, such as cluster of differentiation (CD)34 and Apln. Notably, gene set enrichment analysis revealed overrepresentation of brain endothelial transcripts. Activation of endothelial Wnt-/β-catenin signaling did not induce liver fibrosis or alter metabolic liver zonation, but Ctnnb1OE−EC mice exhibited significantly increased plasma triglyceride concentrations, while liver lipid content was slightly reduced. Ctnnb1 overexpression in arterial ECs of the heart has been reported previously to cause cardiomyopathy. As Clec4g-iCre is active in a subset of cardiac ECs, it was not unexpected that Ctnnb1OE−EC mice showed reduced overall survival and cardiac dysfunction. Altogether, balanced endothelial Wnt-/β-catenin signaling in the liver is required for normal LSEC differentiation and for maintenance of normal plasma triglyceride levels.
Introduction
Liver sinusoidal endothelial cells (LSECs) lining the hepatic sinusoids are a prime example for organ-specific endothelial differentiation. They belong to the group of discontinuous ECs characterized by an incomplete basement membrane and the presence of large fenestrations without diaphragm. LSECs exhibit unique molecular, phenotypic, and functional features and are known to instruct the hepatic vascular niche by cellular interactions and the secretion of paracrine-acting factors called angiokines (Nolan et al., 2013; Augustin and Koh, 2017). For example, LSECs were identified to control liver regeneration by angiocrine wingless-related integration site 2 (Wnt2) and hepatocyte growth factor (Hgf) signaling (Ding et al., 2010; Cao et al., 2017; Zhang et al., 2020). Other highly specialized functions include immunological processes, such as tolerance and defense mechanisms and the clearance of noxious factors from the circulation by a repertoire of scavenger receptors (Schledzewski et al., 2011; Wittlich et al., 2017; Shetty et al., 2018). Interestingly, pathological changes of these highly specialized ECs were shown to contribute to severe liver diseases ranging from steatohepatitis to liver cirrhosis and from hepatocarcinogenesis to liver metastasis (Kostallari and Shah, 2016). During disease processes, LSECs are known to transdifferentiate toward a capillary phenotype revealing a loss of fenestrations and formation of a basement membrane, which is termed “sinusoidal capillarization,” thereby aggravating disease progression (Schaffner and Popper, 1963; Lalor et al., 2006). However, the molecular and signaling mechanisms driving sinusoidal capillarization still await detailed analysis.
Notably, recent work by our group could identify the transcription factor GATA-binding factor 4 (GATA4) as a molecular master regulator for LSEC differentiation during liver development and in liver homeostasis. LSEC-restricted deletion of Gata4 was shown to cause transformation of discontinuous liver sinusoids into continuous capillaries. This sinusoidal transformation in the fetal liver inhibited homing of hematopoietic stem and progenitor cells into the fetal liver resulting in fatal anemia (Geraud et al., 2017), while Gata4 deletion in the mature vasculature caused hepatopathy and perisinusoidal liver fibrosis (Winkler et al., 2021). A special form of liver sinusoidal capillarization was also demonstrated when endothelial Notch signaling was enhanced resulting in a partial loss of LSEC-specific markers and increased the expression of continuous endothelial cell (CEC) markers; however, lacking the formation of a solid basement membrane or liver fibrogenesis (Wohlfeil et al., 2019).
Another highly conserved transduction pathway known to be involved in several important biological processes, such as liver development, vascular and hepatic differentiation, and tissue homeostasis is the Wnt-/β-catenin signaling pathway (Decaens et al., 2008; Daneman et al., 2009; Wild et al., 2020). In the liver vasculature, Wnt2 was identified as an LSEC-associated molecule with autocrine growth effects (Klein et al., 2008; Geraud et al., 2010), and also as an angiocrine regulator of liver regeneration (Ding et al., 2010). In the meantime, angiocrine Wnt signaling in the liver has become even more important. Hepatic endothelial cells (ECs) not only express Wnt2, they also express Wnt9b, Wnt ligand secretion mediator (Wls), and Wnt potentiator R-Spondin 3 (Geraud et al., 2010; Rocha et al., 2015). These factors are indispensable for the formation of a Wnt-dependent pericentral hepatocyte subpopulation. Loss of this Wnt-signaling machinery results in decreased liver size, perturbations of liver zonation, metabolic maturation, and impaired liver regeneration capacity (Rocha et al., 2015; Wang et al., 2015; Planas-Paz et al., 2016; Leibing et al., 2018; Preziosi et al., 2018).
Considering EC morphogenesis and specification, Wnt signaling emerged as a major contributor in the past decades (Choi et al., 2012). ECs not only express intracellular Wnt molecules and their corresponding Frizzled receptors, but also β-catenin-dependent transcription factors (Masckauchan et al., 2005; Deb, 2014). Hereby, it was shown that β-catenin induces arterialization and loss of venous fate of the embryonic vasculature during development (Duarte et al., 2004; Corada et al., 2010). Moreover, in vascular beds of the central nervous system Wnt-signaling is a key regulator of the integrity of the highly sealed blood–brain barrier (BBB) by controlling the formation of tight junction (TJ) molecules and solute transporters (Liebner et al., 2008; Zhou et al., 2014; Tran et al., 2016; Profaci et al., 2020). LSECs, on the other hand, do not typically express TJs, since permeability and cell trafficking are facilitated by open fenestrations (Geraud et al., 2012). Constitutive activation of β-catenin in the highly permeable ECs of the circumventricular organs in the brain resulted in the expression of BBB markers and downregulation of non-BBB vasculature markers (Benz et al., 2019; Wang et al., 2019). As our study and other previous studies have shown that Wnt2 is a LSEC-specific growth and differentiation factor required for liver regeneration and that autocrine Wnt/β-catenin signaling cross-stimulates the angiogenetic vascular endothelial growth factor receptor 2 pathway, we hypothesized that unbalanced canonical endothelial Wnt signaling in the liver might also impair LSEC differentiation and LSEC-mediated liver function. To test this hypothesis, we generated a novel mouse line with constitutive β-catenin overactivation in LSECs by crossing Ctnnb1-Ex3fl/wt with EC subtype-specific Clec4g-iCretg/wt mice (Wohlfeil et al., 2019).
Materials and Methods
Animals
To generate endothelial subtype-specific Ctnnb1 gain-of-function (GOF) mice (Ctnnb1OE−EC), Clec4g-iCretg/wt (Tg(Clec4g-icre)1.1Sgoe (Wohlfeil et al., 2019) were crossed with Ctnnb1(Ex3)fl/wt (Ctnnb1tm1Mmt) (Harada et al., 1999) mice. Specificity of Cre-activity was analyzed in crosses of Clec4g-iCretg/wt transgenic mice with R26YFP (B6.129X1-Gt(ROSA)26Sortm1(EYFP)Cos/J) [JAX 006148] (Srinivas et al., 2001) reporter animals. All animals were housed under specific pathogen-free conditions in an animal facility (Heidelberg University). Animal experiments were performed in accordance with Federal Animal Regulations and were institutionally approved by the district government Karlsruhe and performed under institutional guidelines. Mice were sacrificed by cervical dislocation. Liver, heart, kidney, lung, spleen, brain, and intestine weights were measured, and tissue samples were either embedded in the optimum cutting temperature compound (Sakura, Alphen aan den Rijn, The Netherlands) and frozen in liquid nitrogen or fixed in 4% paraformaldehyde at 4°C.
Isolation of Primary Murine LSECs
Livers, pooled from two mice, were perfused in situ via the portal vein with a 0.05% collagenase containing amino acid/saccharide calcium-deprived medium (C2674, Sigma–Aldrich, Taufkirchen, Germany), dissected, mechanically minced, digested at 38°C in a collagenase/Gey's balanced salt solution (G9779, Sigma–Aldrich) and filtered through a 250 μm mesh. Cells were separated by a 35% Nycodenz (1002424, Axis-Shield, Alere Technologies, Oslo, Norway) gradient. Next, LSECs were isolated by magnetic-activated cell sorting using anti-CD146 MicroBeads (ME-9F1, 130-092-007, Miltenyi Biotech, Bergisch Gladbach, Germany) according to the instructions of the manufacturers.
Quantitative Reverse-Transcription PCR
RNA was extracted from primary ECs using EZNA Total-RNA-Kit I (OMEGA Biotec, Norcross, GA, United States). Complementary DNA (cDNA) was synthesized with RevertAid H-Minus M-MuLV Reverse Transcriptase (ThermoScientific, Waltham, MA, United States). Quantitative PCR was performed on a qTOWER 3 G touch thermal cycler (Analytik Jena) using innuMIX qPCR SyGreen Sensitive (845-AS-1310200, Analytik Jena, Jena, Germany). Normalized expression values were calculated using the Pfaffl method considering amplification efficiency values determined by standard curves (Pfaffl, 2001).
RNA in situ Hybridization
Liver tissue was sectioned at 4 μm. RNA in situ hybridization (ISH) was conducted using RNAscope 2.5 HD Red (322350, Advanced Cell Diagnostics, Newark, CA, United States) kits with mouse-specific probes against the positive control mouse Ppib (Cyclophilin B) gene, Mus musculus (Mm)-Bmp2-E3-Channel 1 (1545–2347 NM_007553.3), Mm-Hgf-Channel 1 (1120–2030 NM_010427.4), Mm-Wnt2-Channel 1 (857–2086 NM_023653.5), Mm-Wnt9b-Channel 1 (706–1637 NM_011719.4), Mm-Stab1-Channel 1 (488-1320 NM_138672.2), and Mm-Stab2-Channel 1 (4249–5075 NM_138673.2) according to the protocols of the manufacturer.
Histology and Immunofluorescence
Tissue samples fixed by 4% paraformaldehyde at room temperature for 48–72 h, were subsequently transferred into phosphate-buffered saline (PBS), dehydrated in a graded alcohol series, and embedded in paraffin. Paraffin-embedded tissues were sectioned in 4 μm. For hematoxylin & eosin (H&E), periodic acid–Schiff (PAS), Oil Red O (ORO), Prussian blue, and Sirius red staining, samples were processed according to the standard protocols provided by the manufacturer. For immunofluorescence (IF), cryosections (7 μm) were air-dried, fixed in 4% paraformaldehyde (PFA) or acetone, rehydrated in PBS (A0964.9050, VWR International, Radnor, PA, United States) and blocked in 5% donkey serum (017-000-121, Dianova, Hamburg, Germany) in PBS for 30 min. Primary antibodies were incubated overnight at 4°C. Sections were washed three times in PBS before incubation with appropriate Alexa Fluor–coupled secondary antibodies for 45 min at room temperature. Paraffin-embedded sections were baked at 60°C overnight, after which they were deparaffinized with xylol and rehydrated using ethanol in decreasing concentrations. Antigen retrieval of tissue sections was carried out with epitope retrieval solution (Zytomed Systems, Berlin, Germany) at either pH 6, 8, or 9. Primary antibody was incubated for 2 h at room temperature or overnight at 4°C. Sections were washed three times in PBS before incubation of appropriate secondary antibodies for 1 h at room temperature. Nuclei were counterstained with 4′,6-diamidin-2-phenylindol (DAPI) (D1306, Thermo Fisher Scientific, Waltham, MA, United States). Finally, sections were thoroughly washed in PBS before mounting with Dako fluorescent mounting medium (Dako, Agilent technologies, Santa Clara, CA, United States). Sections were photographed with ECLIPSE Ci microscope (Nikon, Alzenau, Bavaria, Germany) or ECLIPSE Ni-E microscope (Nikon). Immunofluorescence images were acquired in a sequential mode as a series of z-axis images and processed with NIS-Elements AR 5.02 (Nikon Instruments, Tokyo, Japan) and ImageJ 1.52e software (NIH, Bethesda, MD, United States). Using NIS-Elements AR 5.02, images were background corrected (rolling ball 7.5 pixels), deconvoluted, and focused to one plane.
For the quantification of IF images, three representative areas per sample were chosen. For each image, binary masks of the representative channels were created using automated threshold functions (Otsu, MaxEntropy) in ImageJ. The resulting binary masks were quantified for the area, or the number of particles (10-infinite pixels) using ImageJ functions “Measure” and “Analyze Particles.” The “Mean gray value” represents the sum of the gray values of all the pixels within the selected images divided by the number of all pixels. For Ki-67 quantification, only Ki-67 staining was included that overlaid with DAPI-positive nucleus staining to exclude unspecific signal. To this end, we used the “Image Calculator” in ImageJ with “AND” as operator for Ki-67 and DAPI channels. For quantification of RNA ISH or ORO images, three representative areas per sample were chosen. RGB images were split into separate channels corresponding to three determined colors by using the “Color deconvolution” command in ImageJ. The images displaying the region of interest were further processed by setting color thresholds. Finally, the area of particles (>30 pixels) was measured, analyzed, and calculated in percentage (%) of the whole image area.
Antibodies
Primary antibodies: rat anti-Endomucin (14-5851-82, eBioscience, San Diego, CA, United States), goat anti-Lyve1 (AF2125, R&D Systems, Minneapolis, MN, United States), rat anti-mouse/human GATA-4 (14-9980-82, Thermo Fisher Scientific), rat anti-mouse CD68 (137002, BioLegend, San Diego, CA, United States), rabbit anti-Desmin (ab15200, Abcam, Cambridge, Cambs., UK), rabbit anti-glutamine synthetase (G2781, Sigma–Aldrich, Taufkirchen, Bavaria, Germany), goat anti-arginase I (sc-18351, Santa Cruz Biotechnology, Dallas, TX, United States), rat anti-Ki67 (14-5698-82, eBioscience), polyclonal rabbit anti- green fluorescent protein/yellow fluorescent protein (YFP) (A11122, Molecular Probes, Eugene, OR, United States), rat anti-CD31 (102502, BioLegend), SMA-antibody (ab5694, Abcam), goat anti-CD32b (AF1460, R&D Systems), rabbit anti-Collagen type I (R1038, Acris, Hiddenhausen, North Rhine-Westphalia, Germany), rabbit anti-Collagen type III alpha 1 chain (R1040, Acris), rabbit anti-Collagen IV (GTX19808, Genetex, Irvine, CA, United States), rabbit anti-Cyp2E1 (HPA009128, Sigma–Aldrich), rabbit anti-Claudin 5 (34-1600, Thermo Fisher Scientific), goat anti-Podocalyxin (AF1556, R&D Systems), rabbit anti-Cav1 (N-20, Santa Cruz Biotechnology), rabbit anti- intracellular adhesion molecule 1 (ICAM1) (10020-1-AP, Proteintech, Rosemont, IL, United States), goat anti-mouse vascular endothelial (VE)-cadherin (AF1002, R&D Systems), goat anti- vascular cell adhesion molecule (VCAM)-1/CD106 (AF643, R&D Systems). Secondary antibodies: Alexa-Fluor 488, Alexa-Fluor 647, and cyanine 3-conjugated secondary antibodies were purchased from Dianova (Hamburg, Germany).
Microarray Processing and Statistical Analysis
Gene expression profiling was performed using arrays MoGene-2_0-st from Affymetrix (Santa Clara, CA, United States). Biotinylated antisense cDNA and arrays hybridization were performed according to the recommendations of the manufacturer using the GeneChip WT Plus Reagent Kit and the GeneChip Hybridization, Wash and Stain Kit (both from Thermo Fisher Scientific). A Custom CDF Version 22 with ENTREZ-based gene definitions was used to annotate the arrays. The raw fluorescence intensity was robust multiarray analysis background corrected and values were normalized applying quantile normalization. Differential gene expression was analyzed with the one-way-ANOVA, using a commercial software package SAS JMP15 Genomics, version10, from SAS (SAS Institute, Cary, NC, United States). A false-positive rate of a = 0.05 with FDR correction was taken as the level of significance. To determine whether defined lists (or sets) of genes exhibit a statistically significant bias in their distribution, we performed a gene set enrichment analysis (GSEA). GSEA (Subramanian et al., 2005) was carried out using R 3.6.1. clusterProfiler 3.12.0 (Yu et al., 2012), fgsea 1.10.0 (Korotkevich et al., 2021), the molecular signatures database (MSigDB) v6.2 hallmark gene set collection (Liberzon et al., 2015), and self-defined gene lists were used. Gene lists for LSECs and CECs were used as previously described (Winkler et al., 2021). The gene set for brain ECs was defined using published single-cell RNA seq data (Sabbagh et al., 2018). Inclusion criteria were fold change ≥ 2 for brain vs. liver ECs and at least 10 transcripts per million in liver ECs to exclude less-expressed genes. Overrepresentation analysis (ORA) of Gene Ontology terms was performed with the enrichR (Chen et al., 2013) package in R 3.6.1 for all significantly regulated genes. Heatmaps were created with the ComplexHeatmap package (Gu et al., 2016).
The raw and normalized gene expression profiling data have been deposited in the NCBI Gene Expression Omnibus and are accessible through GEO Series accession number GSE175777 (https://www.ncbi.nlm.nih.gov/geo/query/acc.cgi?acc=GSE175777).
Blood Parameters
Serum was analyzed for the following routine parameters: alanine aminotransferase (ALA), aspartate aminotransferase (AST), and glutamate dehydrogenase (GLDH), cholesterol, triglycerides, glucose, and total protein (Roche cobas c 311 analyser, Roche Diagnostics, Basel, Switzerland).
Hepatic Triglycerides
Snap frozen liver tissue (100 mg) was homogenized in 5% NP-40 solution (74385, Merck) and heated for 5 min in a shaking dry incubator (ThermoMixer C, Eppendorf, Hamburg, Germany) at 80–100°C. After cooling to room temperature, the heating was repeated in order to solubilize all triglycerides. After centrifugation for 2 min at top speed (Centrifuge 5417 R, Eppendorf) the supernatant was diluted 10-fold in distilled water and used to determine the triglyceride content based on the protocol of the Triglyceride Quantification Colorimetric/Fluorometric Kit manufacturer (K622, BioVision, Mountain View, CA, United States).
Transthoracic Echocardiography
For echocardiography, mice were anesthetized with 0.5–1.0% isoflurane and placed on a heating pad to maintain body temperature. Non-invasive, echocardiographic parameters were recorded with a linear 50 MHz transducer (Vevo 3100 system with MX700 transducer, Visualsonics, Toronto, Canada) in parasternal long-axis B- & M-mode, and measured post-processing, which comprised heart rate, left ventricle (LV) posterior and anterior wall thickness, and LV internal diameter at both end-systole and end-diastole. From that, LV volume, LV ejection fraction, and cardiac output were calculated with the Vevo Workstation 5.5.0 and the integrated cardiac measurement package.
Statistics
Statistical analysis was performed with SigmaPlot 11 Software (Systat Software GmbH, Germany). For pairwise comparisons, the t-test was used when normality was proved. Differences between data sets with p < 0.05 were considered statistically significant. Data are presented as means with error bars indicating standard error.
Results
Generation and Characterization of Adult β-Catenin-Overactivated HEC Mice
EC subtype-specific Clec4g-iCre mice (Wohlfeil et al., 2019) were used to generate mice with Ctnnb1 GOF in LSECs (Figure 1A). Ctnnb1OE−EC (Clec4g-iCretg/wt;Ctnnb1(Ex3)fl/wt) mice were viable but were born at a lower Mendelian frequency than expected (Figure 1B) and suffered from a reduced overall survival rate (Figure 1C). While bodyweight was not altered, heart weight as well as heart weight/body weight ratio were significantly increased in Ctnnb1OE−EC mice (Figures 1D,E). As Cre-activity was previously described in ECs of the heart in Clec4g-iCre mice (Wohlfeil et al., 2019), a comprehensive analysis of Clec4g-iCre;R26YFP reporter mice was performed for this organ. Reporter activity was present in CD31+ ECs of the heart (Supplementary Figure 1A). Specifically, YFP positivity was observed in the endocardium, including endomucin (Emcn)+ endocardial trabeculae (Rhee et al., 2018) as well as in CD31+ αSMA+ coronary veins and arteries (Zhang et al., 2005) (Supplementary Figures 1A,B). In contrast, LYVE1+ lymphatic vessels were YFP negative (Supplementary Figure 1B). In Ctnnb1OE−EC mice, echocardiography revealed progressive cardiac dysfunction, which is comparable to the phenotype obtained after β-catenin GOF mutation in arterial ECs of the heart by using Bmx-CreERT2 transgenic mice (Nakagawa et al., 2016). Ctnnb1OE−EC mice displayed increased end-diastolic left ventricle internal diameters and volumes (Figure 1F; Supplementary Figure 2A) and a reduction in wall thickness of the left ventricle (Supplementary Figures 2B,C). The ejection fraction was significantly reduced starting with 2 weeks of age (Figure 1G), whereas the cardiac output was first reduced starting with 4 weeks of age (Supplementary Figure 2D). A routine histochemical staining of internal organs such as the kidneys, lungs, spleen, brain, and intestine were gross morphologically unremarkable (Supplementary Figure 3).
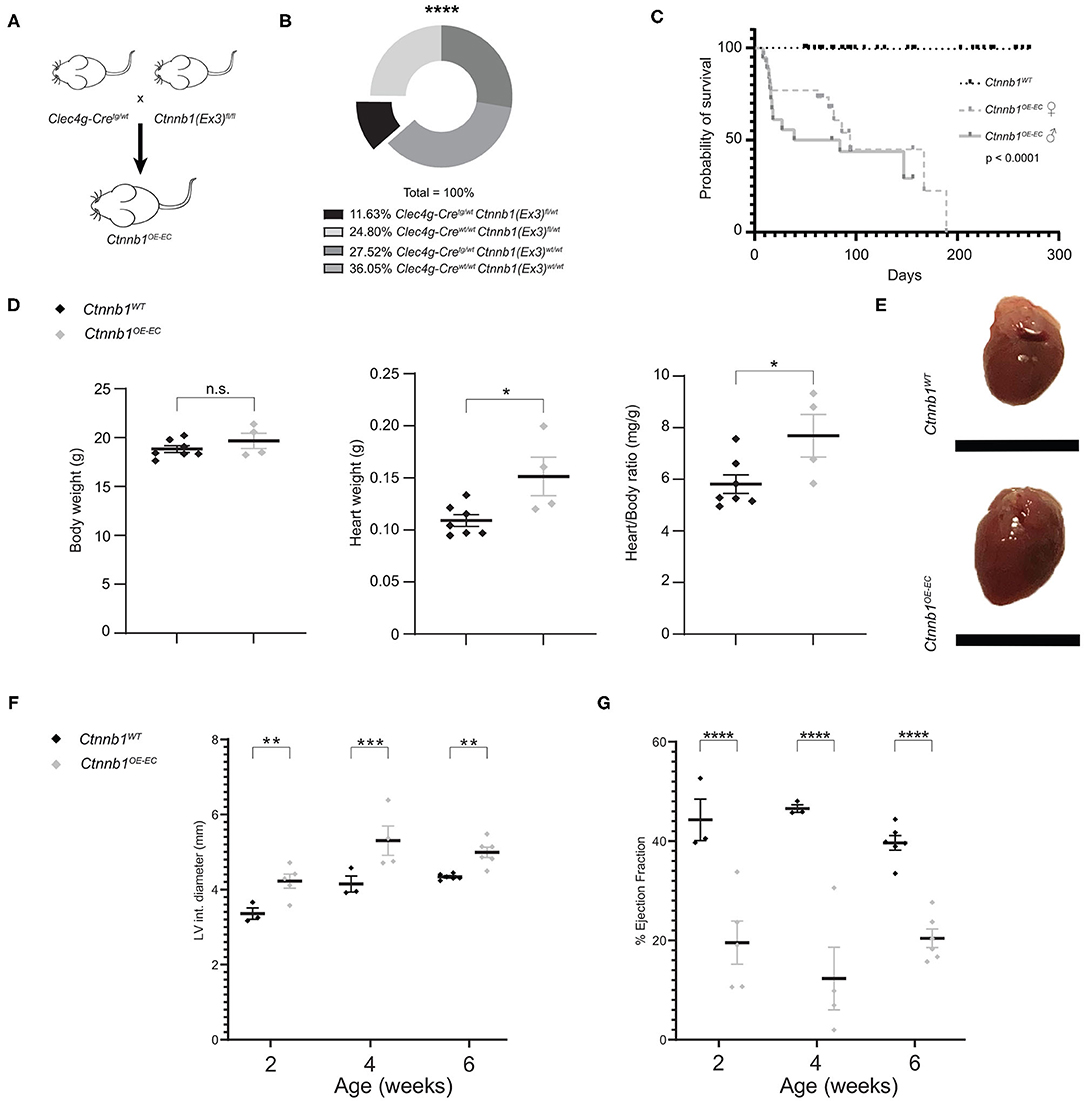
Figure 1. Ctnnb1OE−EC mice have a low survival probability and suffer from cardiac dysfunction (A) Ctnnb1OE−EC mice [Clec4g-iCretg/wt;Ctnnb1(Ex3)fl/wt] were generated by crossing Clec4g-iCretg/wt with Ctnnb1(Ex3)fl/fl mice. (B) Mendelian frequency of Clec4g-iCretg/wt;Ctnnb1(Ex3)fl/wt mice. ****p < 0.0001. (C) Kaplan–Meier survival curves for control (Ctnnb1WT) and Ctnnb1OE−EC mice. The probability of survival is shown for Ctnnb1OE−EC female (n = 26) vs. Ctnnb1OE−SEC male (n = 18) vs. Ctnnb1WT mice (n > 65). (D) Body weight, heart weight, heart-to-body weight ratio of 2- to 3-month-old Ctnnb1WT and Ctnnb1OE−EC mice (female, n ≥ 4). Results are represented as mean ± SEM. ns, not significant; *p < 0.05. (E) Macroscopic heart images of 3-month-old Ctnnb1WT and Ctnnb1OE−EC mice (female, n = 4). Scale bar 1 cm. (F) Left ventricle (LV) interior diameter and (G) ejection fraction as determined by echocardiography of 2-, 4-, and 6-week-old Ctnnb1WT and Ctnnb1OE−EC mice (n ≥ 3). Results are represented as mean ± SEM. **p < 0.01; ***p < 0.001; ****p < 0.0001.
Endothelial β-catenin overactivation in the liver was confirmed by quantitative reverse-transcription PCR (qRT-PCR), which showed significantly elevated expression of Wnt-/β-catenin downstream target gene axis inhibition protein 2 (Axin2) in isolated LSECs from Ctnnb1OE−EC mice (Figure 2A). Liver size, liver weight, and liver/body weight ratio were not significantly altered in Ctnnb1OE−EC mice (Figures 2B,C). Basic liver function tests did not show elevated levels of ALT, AST, and GLDH (Figure 2D). Upon Sirius red staining, no signs of fibrosis were present in the Ctnnb1OE−EC livers (Figure 2E). In line with the absence of collagen deposition upon Sirius red staining, no changes in perisinusoidal collagen I, III, or basement membrane collagen IV deposition were seen (Supplementary Figure 4A). Additionally, no obvious alterations were seen in livers of Ctnnb1OE−EC mice upon H&E histology, PAS, and Prussian blue staining (Supplementary Figure 4B). Likewise, Kupffer cells or hepatic stellate cells (HSC) were not altered in quantity, as analyzed by IF for CD68 or Desmin, respectively (Figure 2F). Co-IF of marker proteins for metabolic liver zonation revealed no changes in zonated expression of Glul/GS and Cyp2E1 in pericentral or Arg1 in periportal and midlobular hepatocytes (Figure 2G; Supplementary Figure 4C). Notably, there was a significant increase in the Ki67-positivity in ECs from Ctnnb1OE−EC livers, while the proliferation of hepatocytes did not show changes (Figure 3A).
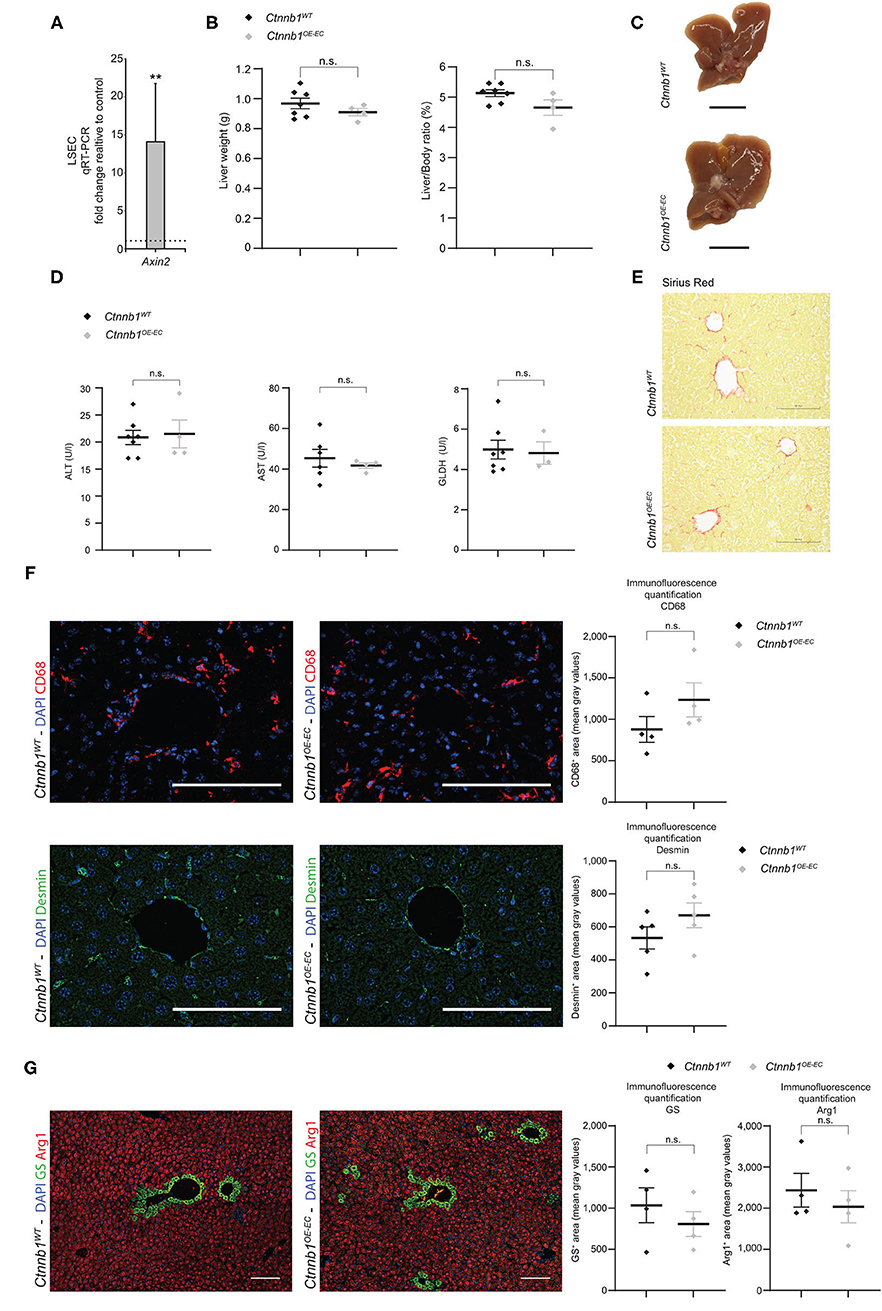
Figure 2. Hepatic endothelial Ctnnb1 overactivation does not lead to hepatopathy and fibrosis. (A) qRT-PCR for axis inhibition protein 2 (Axin2) of cDNA from freshly isolated LSECs of 2-months-old Ctnnb1OE−EC mice compared to corresponding Ctnnb1WT controls (n = 3). β-Actin was used as housekeeping gene. **p < 0.01. (B) Liver weight, liver-to-body weight ratio of 2- to 3-month-old Ctnnb1WT and Ctnnb1OE−EC mice (female, n ≥ 4). Results are represented as mean ± SEM. ns, not significant. (C) Macroscopic liver images of 3-month-old Ctnnb1WT and Ctnnb1OE−EC mice (female, n = 4). Scale bar 1 cm. (D) Liver enzymes [aspartate aminotransferase (AST), alanine aminotransferase (ALT), and glutamate dehydrogenase (GLDH)] in serum of 2- to 3-month-old female Ctnnb1WT and Ctnnb1OE−EC mice (n ≥ 3). Results are represented as mean ± SEM. n.s., not significant. (E) Sirius red staining of liver sections of 2- to 3-month-old male Ctnnb1WT and Ctnnb1OE−EC mice (n = 4). Scale bar 100 μm. (F) Immunofluorescence (IF) staining of DAPI, CD68 and Desmin, and CD68 and Desmin quantification in the liver of 2- to 3-month-old female Ctnnb1WT and Ctnnb1OE−EC mice (n ≥ 4). Scale bar 100 μm. Results are represented as mean ± SEM. ns, not significant. (G) IF staining of DAPI, glutamine synthetase (GS) and arginase (Arg1), and GS and Arg1 quantification in the liver of 2- to 3-month-old Ctnnb1WT and Ctnnb1OE−EC mice (n = 4). Scale bar 100 μm. Results are represented as mean ± SEM. ns, not significant.
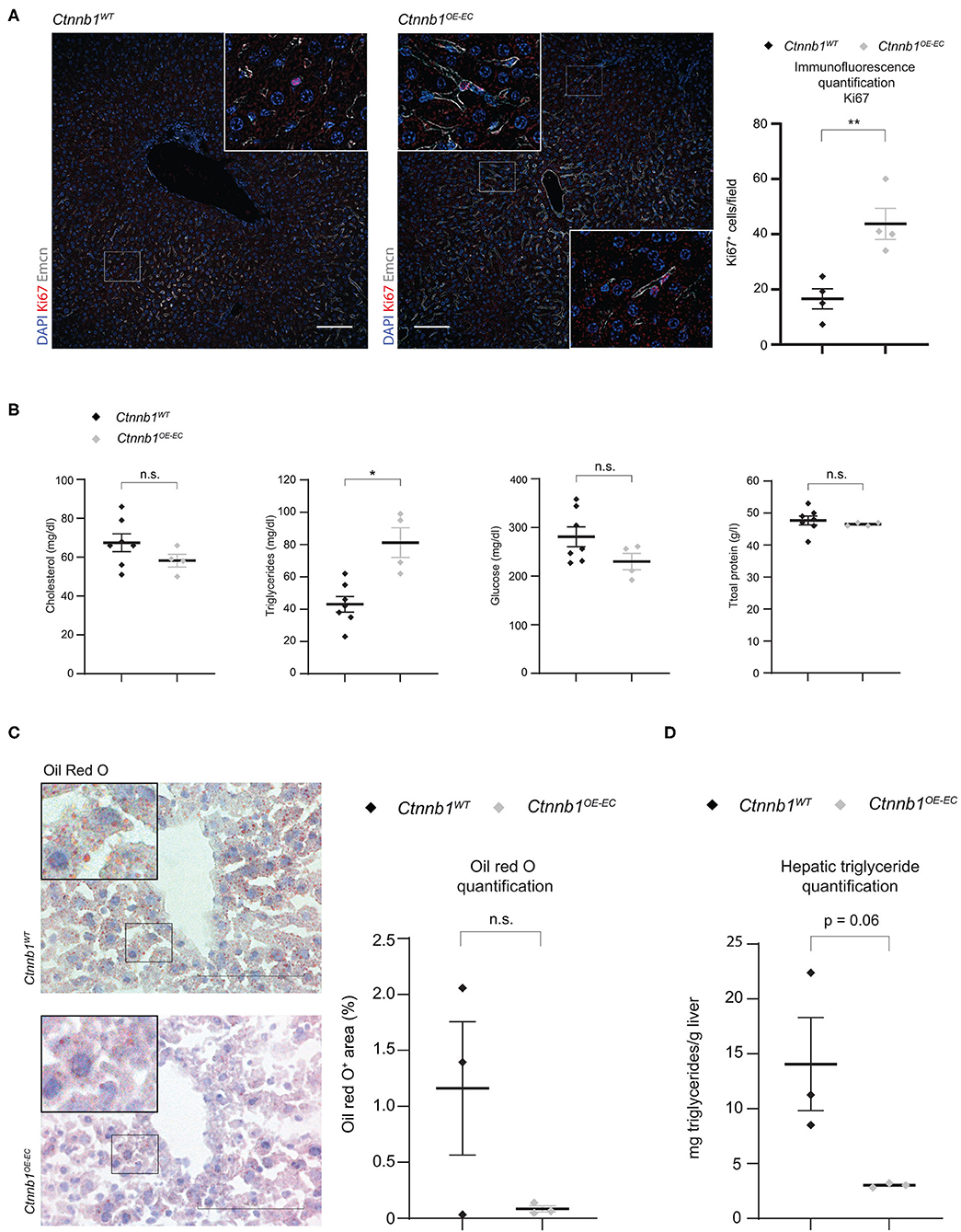
Figure 3. Ctnnb1OE−EC mice display enhanced hepatic endothelial cell proliferation, serum hypertriglyceridemia, and decreased lipid accumulation in the liver. (A) IF staining of DAPI, Ki67, and Emcn, and Ki67 quantification in the liver of 2- to 3-month-old female Ctnnb1WT and Ctnnb1OE−EC mice (n = 3). Scale bar 100 μm. Results are represented as mean ± SEM. **p < 0.01. (B) Serum levels of cholesterol, triglycerides, and glucose in 2- to 3-month-old Ctnnb1WT and Ctnnb1OE−EC mice (female, n ≥ 5). Results are represented as mean ± SEM. **p < 0.01. (C) Oil Red O (ORO) staining and quantification of livers of 3-month-old female Ctnnb1WT and Ctnnb1OE−EC mice (n = 3). Scale bar 100 μm. Results are represented as mean ± SEM. ns, not significant. (D) Hepatic triglyceride concentration of murine liver tissue of 3-month-old female Ctnnb1WT and Ctnnb1OE−EC mice (n = 3). *p < 0.05.
Recently, we could demonstrate that EC-derived Wnt signaling controls metabolic liver zonation and alters lipid metabolism (Leibing et al., 2018). Although metabolic liver zonation was not affected by β-catenin GOF mutation, we performed a comprehensive metabolic screening including serum parameters such as total protein, cholesterol, triglycerides, and glucose (Figure 3B). Interestingly, Ctnnb1OE−EC mice showed significantly increased serum levels of triglycerides (Figure 3B). Liver tissue of Ctnnb1OE−EC mice showed slightly reduced lipid storage upon Oil Red O staining (Figure 3C) and a tendency of decreased levels of hepatic triglycerides as measured by a colorimetric assay (Figure 3D).
Hepatic Endothelial β-Catenin GOF Mutation Causes Molecular Transdifferentiation of LSECs
To identify β-catenin-dependent molecular alterations in LSECs, we performed comprehensive Affymetrix DNA microarray gene expression profiling of isolated primary LSECs from Ctnnb1WT control and Ctnnb1OE−EC animals. β-catenin GOF mutation in LSECs resulted in the significant dysregulation of 128 genes as compared to control LSECs (Table 1). GSEA of LSECs isolated from Ctnnb1WT control and Ctnnb1OE−EC animals revealed significant pathway alterations in the Hallmark gene sets. Among the most regulated gene sets, we found “Myc targets V1 and V2” and “Cholesterol homeostasis” (Figure 4A) followed by “G2M Checkpoint” and “E2F targets.” Furthermore, GSEA confirmed the activation of Wnt/β-catenin signaling in β-catenin GOF mutation in LSECs (Figure 4A). Overrepresentation analysis (ORA) of the significantly dysregulated genes by using Enrichr revealed significant alterations in the gene ontology (GO) biological processes 2018 library (Figure 4B). “Positive regulation of cell differentiation” was identified as the most significant GO term in LSECs with β-catenin GOF mutation (Figure 4B).
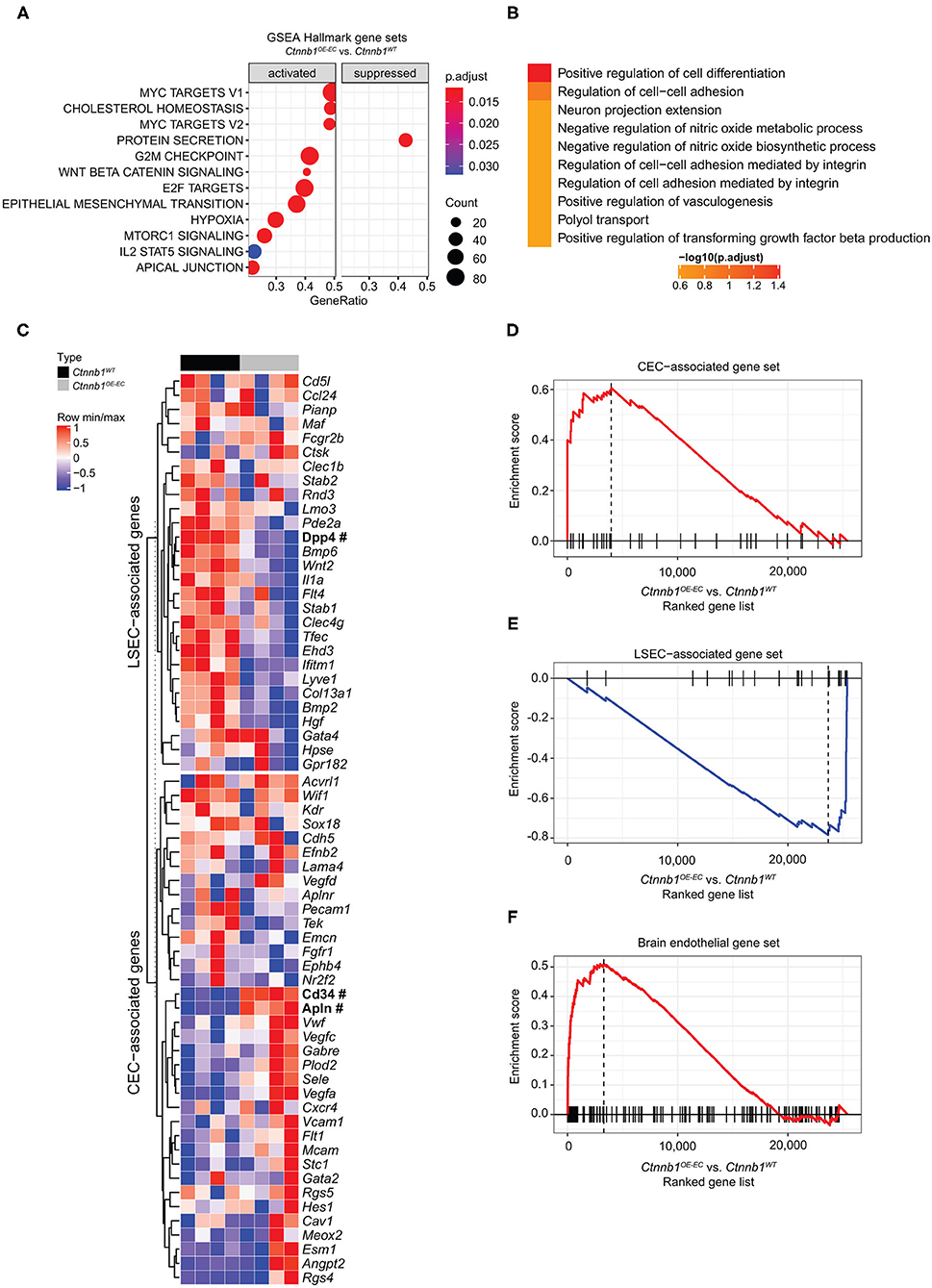
Figure 4. Hepatic endothelial Ctnnb1 overactivation causes sinusoidal transdifferentiation. (A) Gene Set Enrichment Analysis-Kyoto Encyclopedia of Genes and Genomes (GSEA-KEGG) pathway alterations analyzed using MSigDB hallmark gene sets in freshly isolated LSECs from 2-month-old female Ctnnb1WT and Ctnnb1OE−EC (n = 4). (B) Overrepresentation analysis of gene ontology “biological processes” library. (C) Heat map of the liver sinusoidal endothelial cell (LSEC)- and continuous endothelial cell (CEC)-associated genes. Selected genes are shown for isolated LSECs from Ctnnb1WT (black) and Ctnnb1OE−EC mice (gray). Significant samples are written in bold and marked with # (n = 4 samples per group). The heat map color represents the mean and maximum values for each gene. The intensity scale of the standardized expression values ranges from dark blue (low expression) to dark red (high expression). Enrichment plots of (D) LSEC-associated (p = 0.0023; NES = −2.43) and (E) CEC-associated (p = 0.0023; NES = 2.04) genes (n = 4). (F) Enrichment plots of brain endothelial genes (p = 0.0001; NES = 2.16) (n = 4).
An established panel of LSEC-associated and CEC-associated marker genes (Geraud et al., 2010, 2017; Olsavszky et al., 2020) was analyzed in LSECs isolated from Ctnnb1WT control and Ctnnb1OE−EC animals. Gene expression analysis pointed out that β-catenin GOF mutation in LSECs of Ctnnb1OE−EC mice dysregulated LSEC- and CEC-associated genes (Figure 4C). GSEA revealed a significant induction of a CEC-associated gene set (Figure 4D) and a significant loss of an LSEC gene set (Figure 4E). As Wnt-/β-catenin signaling is a well-known driver for brain endothelial differentiation (Liebner et al., 2008), we hypothesized that β-catenin signaling activation in LSECs might result in partial brain EC reprograming. When performing GSEA with a brain endothelial gene set, which was generated by comparing published single-cell RNA-seq data from brain vs. liver ECs (Sabbagh et al., 2018), a significant enrichment for brain EC transcripts was found in Ctnnb1OE−EC LSEC (Figure 4F). Among the genes that were significantly upregulated in Ctnnb1OE−EC LSEC with a fold-change of >2, several genes could be detected that were also highly expressed in brain ECs (Table 2). As the expression of TJ molecule, Cldn5 was previously shown to be upregulated by endothelial Wnt-/β-catenin GOF in the leaky suprafornical organ (Benz et al., 2019), we compared the expression levels of Cldn5 in Ctnnb1OE−EC and control liver. Expression of Cldn5 was not altered in Ctnnb1OE−EC compared with control LSECs (Supplementary Figure 5A).
In addition, the expression of markers for endothelial zonation, that is, Emcn and LYVE1 (Walter et al., 2014) were analyzed. A significant loss of mid-zonal LSEC marker LYVE1 was found, indicating disturbed endothelial liver zonation (Figures 5A,B). However, the expression of pericentral LSEC and CEC marker Emcn was not altered on protein level (Figure 5A). Despite disturbed endothelial zonation, the expression of pan-endothelial marker podocalyxin or CD31 was unaltered indicating no major changes in vascular density in Ctnnb1OE−EC livers (Supplementary Figure 5B). Furthermore, β-catenin GOF in the LSECs did not alter the expression of LSEC marker CD32b (Supplementary Figure 5C), LSEC scavenger receptors Stab1 and Stab2 (Supplementary Figures 5D,E) or CEC markers Vegfr2, Caveolin-1, ICAM1, vascular cell adhesion molecule (VCAM), or VE-cadherin (Supplementary Figures 5C, 6A–C).
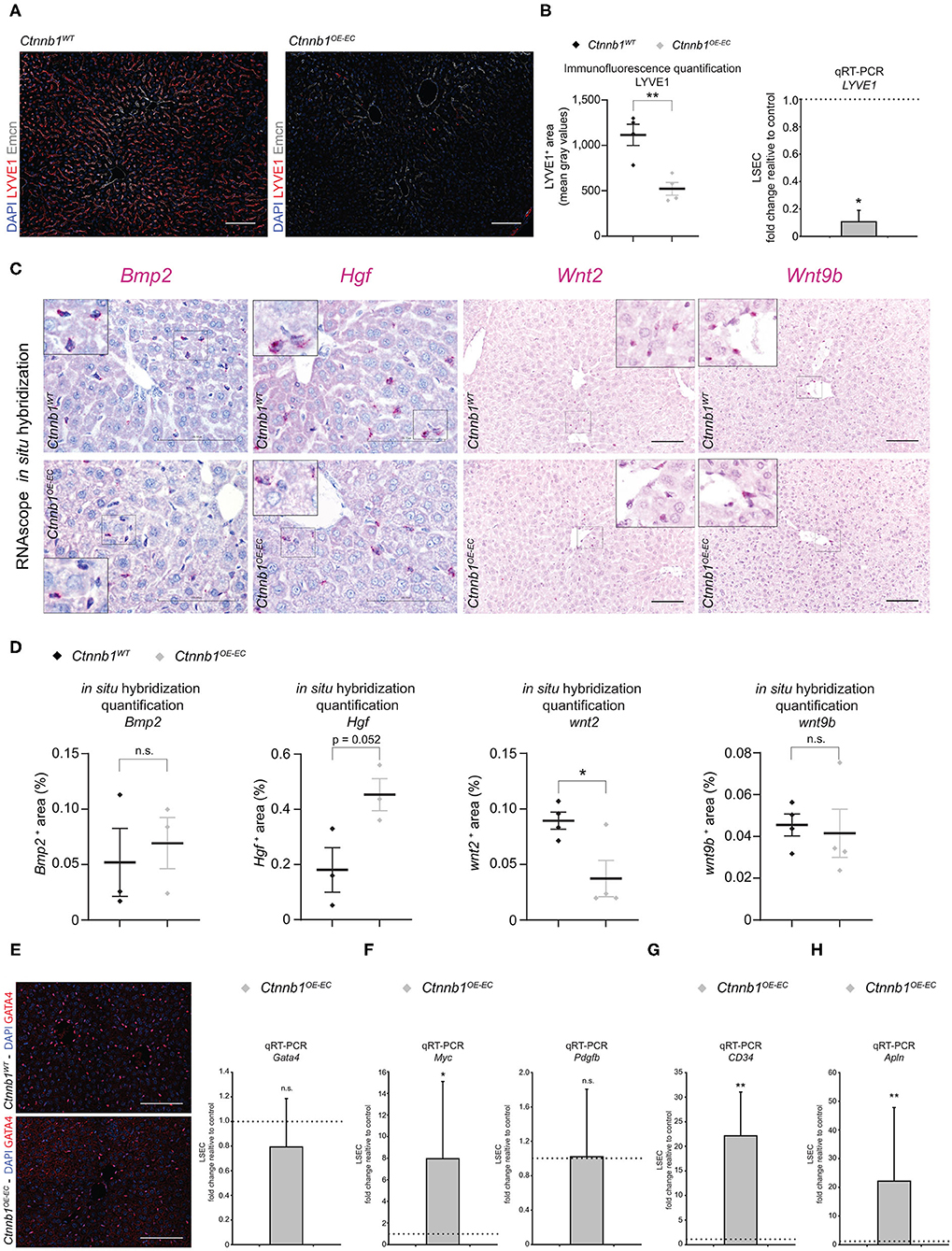
Figure 5. Hepatic endothelial Ctnnb1 overactivation causes loss of LSEC-associated genes and induction of CEC and brain EC genes. (A) IF staining of DAPI, LYVE1, and Emcn in the liver of 2- to 3-month-old female Ctnnb1WT and Ctnnb1OE−EC mice (n = 4). Scale bar 100 μm. (B) Left panel: IF quantification of LYVE1+ area. Results are represented as mean ± SEM. **p < 0.01. Right panel: qRT-PCR for LYVE1 of cDNA from Ctnnb1OE−EC-LSECs compared to Ctnnb1WT control LSECs (n = 4). β-Actin was used as housekeeping gene. *p < 0.05. (C) Bmp2, Hgf, Wnt2, Wnt9b mRNA RNAScope in situ hybridization assay of 2- to 3-month-old female Ctnnb1WT and Ctnnb1OE−EC mice liver sections (n ≥ 3). Scale bar 100 μm. (D) Quantification of Bmp2, Hgf, Wnt2, Wnt9b mRNA RNAScope in situ hybridization assay. Results are represented as mean ± SEM. ns, not significant. *p < 0.05. (E) Left panel: IF staining of DAPI and GATA4 in the liver of 2- to 3-month-old female Ctnnb1WT and Ctnnb1OE−EC mice (n = 4). Scale bar 100 μm. Right panel: qRT-PCR for Gata4 with cDNA from Ctnnb1OE−EC-LSECs compared to Ctnnb1WT control LSECs (n = 4). β-Actin was used as housekeeping gene. n.s., not significant. (F) qRT-PCR for Myc and Pdgfb with cDNA from Ctnnb1OE−EC-LSECs compared with Ctnnb1WT control LSECs (n = 3). β-Actin was used as housekeeping gene. *, p < 0.05; n.s., not significant. (G) qRT-PCR for CD34 with cDNA from Ctnnb1OE−EC-LSECs compared with Ctnnb1WT control LSECs (n = 3). β-Actin was used as housekeeping gene. **p < 0.01. (H) qRT-PCR for Apln with cDNA from Ctnnb1OE−EC-LSECs compared with Ctnnb1WT control LSECs (n = 3). β-Actin was used as housekeeping gene. **p < 0.01.
To confirm the transcriptomic alterations seen in Ctnnb1OE−EC LSEC, we performed immunofluorescent staining, real-time quantitative PCR (qRT-PCR), and ISH for selected EC genes and proteins. The selection was either based on significant regulation among the list of CEC-associated genes (Figure 4C), relation to liver fibrosis [Gata4, Myc, platelet-derived growth factor subunit B (Pdgfb)] (Winkler et al., 2021), or established LSEC angiocrine factors. Upon ISH, the expression of the bone morphogenetic protein (Bmp) 2 was not significantly altered (Figures 5C,D). This was in line with Prussian blue staining of the liver, which did not show iron deposition in the liver of Ctnnb1OE−EC mice (Supplementary Figure 4B). Moreover, Hamp expression in liver lysates was unaltered, indicating preserved BMP2–HAMP signaling (Supplementary Figure 4D). While LSEC angiocrine factor Wnt2 was significantly downregulated, Hgf and Wnt9b were not significantly altered (Figures 5C,D). β-catenin GOF mutation in LSECs did not alter the expression of LSEC master regulator GATA4 on protein or mRNA level (Figure 5E) or pro-fibrotic angiocrine factor Pdgfb (Figure 5F). On the contrary, transcription factor Myc and CEC markers CD34 and Apln were significantly upregulated in Ctnnb1OE−EC LSEC (Figures 4C, 5F–H).
Discussion
Our data show that imbalanced or overactivated β-catenin signaling in LSECs leads to sinusoidal transdifferentiation, including dysregulated lipid homeostasis. Reduced overall survival of Ctnnb1OE−EC mice was most likely independent from LSEC transdifferentiation and dysregulated lipid homeostasis, but rather resulted from progressive heart dysfunction. The heart phenotype observed in Ctnnb1OE−EC mice is comparable to β-catenin GOF mutation studies in arterial ECs by using a Bmx-CreERT2 mice, although reporter activity in heart ECs of Clec4g-iCre;R26YFP mice was identified in more than just arterial ECs, namely in heart capillaries, endocardium, and venous coronary vessels. Mechanistically, activation of Wnt-/β-catenin signaling in arterial ECs of the heart was shown to result in progressive heart failure through suppressing neuregulin-ErbB signaling (Nakagawa et al., 2016).
In the liver, β-catenin GOF mutation in LSECs resulted in sinusoidal-to-continuous transdifferentiation with downregulation of midzonal LSEC marker LYVE1 and angiocrine factor Wnt2, and upregulation of CEC markers CD34 and Apln. This rather “mild” capillarization program lacking HSC activation and perisinusoidal extracellular matrix deposition did not result in hepatopathy or liver fibrosis. Interestingly, Wnt-target gene Myc (He et al., 1998) was significantly induced in Ctnnb1OE−EC LSECs. Previous work by us could show, that loss of LSEC master regulator GATA4 also induced pro-angiogenic Myc in LSECs, to further amplify a pro-fibrotic angiocrine program, including de novo Pdgfb expression, resulting in perisinusoidal liver fibrosis (Winkler et al., 2021). β-catenin GOF in LSECs did not significantly regulate GATA4 expression, which most likely protects against a complete capillarization program and perisinusoidal liver fibrosis by suppressing pro-fibrotic angiocrine factors such as Pdgfb, which was unaltered in Ctnnb1OE−EC LSEC despite a significant Myc induction.
Angiocrine Wnt-signaling is vital for liver growth and metabolic liver zonation and Wnt-signaling in LSECs is linked to autocrine growth effects (Klein et al., 2008; Geraud et al., 2010; Leibing et al., 2018). While activation of β-catenin in LSECs reduced angiocrine Wnt2, this reduction together with unaltered Wnt9b was not sufficient to impair metabolic liver zonation in Ctnnb1OE−EC mice. Interestingly, EC proliferation was significantly induced by activation of β-catenin in LSECs. These findings are supported by GSEA results of Ctnnb1OE−EC LSEC with enrichment in the gene sets “G2M Checkpoint” and “E2F Targets,” both resembling a pro-proliferative state, thereby indicating that β-catenin overactivation in LSECs stimulates endothelial proliferation. This is in line with data observed in postnatal brain and retina, showing that deficiency of endothelial β-catenin signaling impairs endothelial proliferation and sprouting (Martowicz et al., 2019).
Notably, activation of β-catenin in LSECs resulted in the upregulation of genes that are known to be expressed by the brain ECs (Daneman et al., 2010; Wang et al., 2019) and GSEA could confirm the enrichment of brain EC transcripts (Sabbagh et al., 2018) in Ctnnb1OE−EC LSECs. In contrast to LSEC, that belong to discontinuous sinusoidal ECs which enable transfer of fluids, nutrients, and small solutes through open fenestrations within the sinusoidal wall (Wisse et al., 1985; Augustin and Koh, 2017), the brain ECs belong to the group of CECs, expressing specialized TJ molecules and transporters for restricting paracellular passage and transcellular trafficking, thereby generating the tightly sealed blood–brain barrier (Langen et al., 2019). In line with our results, ectopic β-catenin signaling activation in the highly permeable and fenestrated vasculature of the circumventricular organs is sufficient for BBB reprograming (Benz et al., 2019; Wang et al., 2019). Furthermore, inducible pan-endothelial Ctnnb1 GOF showed some overlap with genes dysregulated in Ctnnb1OE−EC LSEC despite using different Cre lines (Munji et al., 2019). Vice-versa, loss of Wnt-signaling activity impairs brain endothelial differentiation by downregulating TJ molecules and transporter proteins, while increasing the expression of the plasmalemma vesicle–associated protein (PLVAP) (Liebner et al., 2008; Stenman et al., 2008; Daneman et al., 2009). However, the expression of TJ molecule Cldn5 was not enhanced in Ctnnb1OE−EC LSEC, which could be a result of maintained expression of LSEC master regulator GATA4. Notably, PLVAP knockout mice developed a reduction of LSEC fenestrations, which led to elevated serum levels of triglycerides, low-density lipoprotein, and cholesterol due to retention of chylomicron remnants in the blood. The authors speculated that compensatory hepatocyte de novo lipogenesis was responsible for steatosis, steatohepatitis, and liver fibrosis (Herrnberger et al., 2014). Ctnnb1OE−EC mice neither showed liver steatosis nor fibrosis, which argue against reduced PLVAP expression as a main driver of isolated hypertriglyceridemia in Ctnnb1OE−EC mice.
As only microvascular ECs in the liver with sinusoidal differentiation allow filtration of chylomicron remnants from the blood (Fraser et al., 1995; Cogger et al., 2006), β-catenin-mediated transdifferentiation of liver sinusoids with partial BBB reprograming in Ctnnb1OE−EC mice may impair uptake of chylomicrons and subsequently lead to elevated serum lipid levels. However, these metabolic alterations are in contrast with previous results, showing that neither sinusoidal capillarization with loss of fenestrations and formation of a basement membrane in Gata4-deficient LSEC (Gata4LSEC−KO), nor partial sinusoidal capillarization/trandifferentiation in mice with enhanced Notch signaling in LSECs (NICDOE−HEC) are associated with reduced levels of serum cholesterol and triglycerides (Wohlfeil et al., 2019; Winkler et al., 2021). This argues against a general impairment of lipid transfer into the space of Disse during sinusoidal capillarization/transdifferentiation and indicates that hypertriglyceridemia in Ctnnb1OE−EC mice is a result of β-catenin-mediated LSEC transdifferentiation by impaired transendothelial transport mechanisms and/or by altered angiocrine signaling that control hepatocyte lipogenesis/lipolysis.
Among the de novo expressed genes in Ctnnb1OE−EC LSEC, Apln was found as the second most upregulated gene. Apelin (APLN) is a secreted peptide, which is widely expressed in different cell types, including CECs and is also known as a regulator of transendothelial lipid transport (Hwangbo et al., 2017). Mice with Apln knockout become obese and show more fat deposition as a consequence of increased vascular permeability with greater uptake of fatty acids. On the other hand, transgenic Apln mice that express apelin under the transcriptional control of the keratin 14 promoter are protected from obesity and show a reduced endothelial permeability (Sawane et al., 2011, 2013). Interestingly, Huang and colleagues were able to show that also Apln signaling in hepatocytes protects against lipid accumulation in the liver (Huang et al., 2017). As the promoter region of the Apln gene has transcription factor–binding sites for Wnt signaling downstream targets Tcf/Lef (Chen et al., 2019), Apln expression might be transcriptionally activated by Wnt-β-catenin signaling activation in Ctnnb1OE−EC LSECs. This is in line with silencing experiments of β-catenin in pulmonary ECs showing that Apln mRNA and protein expression were reduced (Alastalo et al., 2011). Thus, in Ctnnb1OE−EC mice de novo Apln expression in transdifferentiated LSECs may be involved in dysregulated lipid homeostasis. Yet, one has to consider that aberrant Apln expression is also found in CD34+ capillarized LSECs in liver fibrosis (Winkler et al., 2021) and cirrhosis (Yokomori et al., 2012) and also pro-angiogenic effects were similar to the vascular apelin signaling (Helker et al., 2020).
Together, normal sinusoidal differentiation is decisive for the fulfillment of the typical LSEC functions such as scavenging, immunoregulation, protection against stellate cell activation, and fibrosis, but also for the angiocrine regulation of liver regeneration and iron metabolism (Poisson et al., 2017; Shetty et al., 2018; Lafoz et al., 2020; Koch et al., 2021). While endothelial Wnt-signaling activity is largely confined to brain ECs for the maintenance of the BBB (Sabbagh et al., 2018), here we can show for the first time that low-level liver endothelial Wnt-signaling in vivo is crucial for maintaining sinusoidal differentiation, which is required for regulation of proper hepatic lipid metabolism. Further research is necessary to analyze the specific contributions of LSECs in hepatic fat absorption and metabolism. Future work will have to address which angiocrine signaling pathways may be involved in this process, extending the knowledge that liver endothelial fatty acid absorption is not mainly a passive mechanism mediated by open fenestrations in LSECs. This is of particular interest as dyslipidemia is a major risk factor for cardiovascular disease.
Data Availability Statement
The datasets presented in this study can be found in online repositories. The names of the repository/repositories and accession number(s) can be found at: https://www.ncbi.nlm.nih.gov/geo/query/acc.cgi?acc=GSE175777.
Ethics Statement
The animal study was reviewed and approved by Regional Council Karlsruhe. Written informed consent was obtained from the owners for the participation of their animals in this study.
Author Contributions
P-SK, KSa, SG, and VO: study concept and design. P-SK, KSa, JHeil, CDS, SK, JHo, MW, CT, CS, KSc, MT, FT, JHein, CG, SG, and VO: experimental work, analysis, and interpretation of data. P-SK, KSa, and VO: writing original draft. All authors writing and reviewing the manuscript before submission.
Funding
This work was supported by the Deutsche Forschungsgemeinschaft (DFG, German Research Foundation)—Project number 259332240—RTG 2099 (to CG, SG, and P-SK), Project number 5454871—SFB TR23 (to CG and SG), Project number 394046768—SFB 1366 (to CG, SG, and P-SK), Project number 413262200—ICON/EB 187/8-1 and Project number 314905040—CRC/SFB-TR 209 (to SG). The authors gratefully acknowledge the data storage service SDS@hd supported by the Ministry of Science, Research and the Arts Baden-Württemberg (MWK) and the German Research Foundation (DFG) through grant INST 35/1314-1 FUGG and INST 35/1503-1 FUGG. The funders had no role in study design, data collection and analysis, decision to publish, or preparation of the manuscript.
Conflict of Interest
The authors declare that the research was conducted in the absence of any commercial or financial relationships that could be construed as a potential conflict of interest.
Publisher's Note
All claims expressed in this article are solely those of the authors and do not necessarily represent those of their affiliated organizations, or those of the publisher, the editors and the reviewers. Any product that may be evaluated in this article, or claim that may be made by its manufacturer, is not guaranteed or endorsed by the publisher.
Acknowledgments
We thank Hiltrud Schönhaber and Stephanie Riester for excellent technical support. We thank Dr. Stefan Liebner (Goethe University Frankfurt, Frankfurt am Main, Germany) for providing the Ctnnb1(Ex3)fl/wt mouse line from MT (Kyoto University, Kyoto, Japan).
Supplementary Material
The Supplementary Material for this article can be found online at: https://www.frontiersin.org/articles/10.3389/fphys.2021.722394/full#supplementary-material
References
Alastalo, T. P., Li, M., Perez Vde, J., Pham, D., Sawada, H., Wang, J. K., et al. (2011). Disruption of PPARgamma/beta-catenin-mediated regulation of apelin impairs BMP-induced mouse and human pulmonary arterial EC survival. J. Clin. Invest. 121, 3735–3746. doi: 10.1172/JCI43382
Augustin, H. G., and Koh, G. Y. (2017). Organotypic vasculature: from descriptive heterogeneity to functional pathophysiology. Science 357:eaal2379. doi: 10.1126/science.aal2379
Benz, F., Wichitnaowarat, V., Lehmann, M., Germano, R. F., Mihova, D., Macas, J., et al. (2019). Low wnt/beta-catenin signaling determines leaky vessels in the subfornical organ and affects water homeostasis in mice. Elife 8:e43818. doi: 10.7554/eLife.43818.044
Cao, Z., Ye, T., Sun, Y., Ji, G., Shido, K., Chen, Y., et al. (2017). Targeting the vascular and perivascular niches as a regenerative therapy for lung and liver fibrosis. Sci. Transl. Med. 9:eaai8710. doi: 10.1126/scitranslmed.aai8710
Chen, E. Y., Tan, C. M., Kou, Y., Duan, Q., Wang, Z., Meirelles, G. V., et al. (2013). Enrichr: interactive and collaborative HTML5 gene list enrichment analysis tool. BMC Bioinformatics 14:128. doi: 10.1186/1471-2105-14-128
Chen, H., Wong, C. C., Liu, D., Go, M. Y. Y., Wu, B., Peng, S., et al. (2019). APLN promotes hepatocellular carcinoma through activating PI3K/Akt pathway and is a druggable target. Theranostics 9, 5246–5260. doi: 10.7150/thno.34713
Choi, H. J., Park, H., Lee, H. W., and Kwon, Y. G. (2012). The Wnt pathway and the roles for its antagonists, DKKS, in angiogenesis. IUBMB Life 64, 724–731. doi: 10.1002/iub.1062
Cogger, V. C., Hilmer, S. N., Sullivan, D., Muller, M., Fraser, R., and Le Couteur, D. G. (2006). Hyperlipidemia and surfactants: the liver sieve is a link. Atherosclerosis 189, 273–281. doi: 10.1016/j.atherosclerosis.2005.12.025
Corada, M., Nyqvist, D., Orsenigo, F., Caprini, A., Giampietro, C., Taketo, M. M., et al. (2010). The Wnt/beta-catenin pathway modulates vascular remodeling and specification by upregulating Dll4/Notch signaling. Dev. Cell 18, 938–949. doi: 10.1016/j.devcel.2010.05.006
Daneman, R., Agalliu, D., Zhou, L., Kuhnert, F., Kuo, C. J., and Barres, B. A. (2009). Wnt/beta-catenin signaling is required for CNS, but not non-CNS, angiogenesis. Proc. Natl. Acad. Sci. U.S.A. 106, 641–646. doi: 10.1073/pnas.0805165106
Daneman, R., Zhou, L., Agalliu, D., Cahoy, J. D., Kaushal, A., and Barres, B. A. (2010). The mouse blood-brain barrier transcriptome: a new resource for understanding the development and function of brain endothelial cells. PLoS ONE 5:e13741. doi: 10.1371/journal.pone.0013741
Deb, A. (2014). Cell-cell interaction in the heart via Wnt/beta-catenin pathway after cardiac injury. Cardiovasc. Res. 102, 214–223. doi: 10.1093/cvr/cvu054
Decaens, T., Godard, C., De Reynies, A., Rickman, D. S., Tronche, F., Couty, J. P., et al. (2008). Stabilization of beta-catenin affects mouse embryonic liver growth and hepatoblast fate. Hepatology 47, 247–258. doi: 10.1002/hep.21952
Ding, B. S., Nolan, D. J., Butler, J. M., James, D., Babazadeh, A. O., Rosenwaks, Z., et al. (2010). Inductive angiocrine signals from sinusoidal endothelium are required for liver regeneration. Nature 468, 310–315. doi: 10.1038/nature09493
Duarte, A., Hirashima, M., Benedito, R., Trindade, A., Diniz, P., Bekman, E., et al. (2004). Dosage-sensitive requirement for mouse Dll4 in artery development. Genes Dev. 18, 2474–2478. doi: 10.1101/gad.1239004
Fraser, R., Dobbs, B. R., and Rogers, G. W. (1995). Lipoproteins and the liver sieve: the role of the fenestrated sinusoidal endothelium in lipoprotein metabolism, atherosclerosis, and cirrhosis. Hepatology 21, 863–874. doi: 10.1002/hep.1840210337
Geraud, C., Evdokimov, K., Straub, B. K., Peitsch, W. K., Demory, A., Dorflinger, Y., et al. (2012). Unique cell type-specific junctional complexes in vascular endothelium of human and rat liver sinusoids. PLoS ONE 7:e34206. doi: 10.1371/journal.pone.0034206
Geraud, C., Koch, P. S., Zierow, J., Klapproth, K., Busch, K., Olsavszky, V., et al. (2017). GATA4-dependent organ-specific endothelial differentiation controls liver development and embryonic hematopoiesis. J. Clin. Invest. 127, 1099–1114. doi: 10.1172/JCI90086
Geraud, C., Schledzewski, K., Demory, A., Klein, D., Kaus, M., Peyre, F., et al. (2010). Liver sinusoidal endothelium: a microenvironment-dependent differentiation program in rat including the novel junctional protein liver endothelial differentiation-associated protein-1. Hepatology 52, 313–326. doi: 10.1002/hep.23618
Gu, Z., Eils, R., and Schlesner, M. (2016). Complex heatmaps reveal patterns and correlations in multidimensional genomic data. Bioinformatics 32, 2847–2849. doi: 10.1093/bioinformatics/btw313
Harada, N., Tamai, Y., Ishikawa, T., Sauer, B., Takaku, K., Oshima, M., et al. (1999). Intestinal polyposis in mice with a dominant stable mutation of the beta-catenin gene. EMBO J. 18, 5931–5942. doi: 10.1093/emboj/18.21.5931
He, T. C., Sparks, A. B., Rago, C., Hermeking, H., Zawel, L., Da Costa, L. T., et al. (1998). Identification of c-MYC as a target of the APC pathway. Science 281, 1509–1512. doi: 10.1126/science.281.5382.1509
Helker, C. S., Eberlein, J., Wilhelm, K., Sugino, T., Malchow, J., Schuermann, A., et al. (2020). Apelin signaling drives vascular endothelial cells toward a pro-angiogenic state. Elife 9:e55589. doi: 10.7554/eLife.55589.sa2
Herrnberger, L., Hennig, R., Kremer, W., Hellerbrand, C., Goepferich, A., Kalbitzer, H. R., et al. (2014). Formation of fenestrae in murine liver sinusoids depends on plasmalemma vesicle-associated protein and is required for lipoprotein passage. PLoS ONE 9:e115005. doi: 10.1371/journal.pone.0115005
Huang, J., Kang, S., Park, S. J., and Im, D. S. (2017). Apelin protects against liver X receptor-mediated steatosis through AMPK and PPARalpha in human and mouse hepatocytes. Cell. Signal. 39, 84–94. doi: 10.1016/j.cellsig.2017.08.003
Hwangbo, C., Wu, J., Papangeli, I., Adachi, T., Sharma, B., Park, S., et al. (2017). Endothelial APLNR regulates tissue fatty acid uptake and is essential for apelin's glucose-lowering effects. Sci. Transl. Med. 9:eaad4000. doi: 10.1126/scitranslmed.aad4000
Klein, D., Demory, A., Peyre, F., Kroll, J., Augustin, H. G., Helfrich, W., et al. (2008). Wnt2 acts as a cell type-specific, autocrine growth factor in rat hepatic sinusoidal endothelial cells cross-stimulating the VEGF pathway. Hepatology 47, 1018–1031. doi: 10.1002/hep.22084
Koch, P.-S., Lee, K. H., Goerdt, S., and Augustin, H. G. (2021). Angiodiversity and organotypic functions of sinusoidal endothelial cells. Angiogenesis 24, 289–310. doi: 10.1007/s10456-021-09780-y
Korotkevich, G., Sukhov, V., Budin, N., Shpak, B., Artyomov, M. N., and Sergushichev, A. (2021). Fast gene set enrichment analysis. bioRxiv [Preprint] doi: 10.1101/060012
Kostallari, E., and Shah, V. H. (2016). Angiocrine signaling in the hepatic sinusoids in health and disease. Am. J. Physiol. Gastrointest. Liver Physiol. 311, G246–G251. doi: 10.1152/ajpgi.00118.2016
Lafoz, E., Ruart, M., Anton, A., Oncins, A., and Hernandez-Gea, V. (2020). The endothelium as a driver of liver fibrosis and regeneration. Cells 9:929. doi: 10.3390/cells9040929
Lalor, P. F., Lai, W. K., Curbishley, S. M., Shetty, S., and Adams, D. H. (2006). Human hepatic sinusoidal endothelial cells can be distinguished by expression of phenotypic markers related to their specialised functions in vivo. World J. Gastroenterol. 12, 5429–5439. doi: 10.3748/wjg.v12.i34.5429
Langen, U. H., Ayloo, S., and Gu, C. (2019). Development and cell biology of the blood-brain barrier. Annu. Rev. Cell Dev. Biol. 35, 591–613. doi: 10.1146/annurev-cellbio-100617-062608
Leibing, T., Geraud, C., Augustin, I., Boutros, M., Augustin, H. G., Okun, J. G., et al. (2018). Angiocrine Wnt signaling controls liver growth and metabolic maturation in mice. Hepatology 68, 707–722. doi: 10.1002/hep.29613
Liberzon, A., Birger, C., Thorvaldsdottir, H., Ghandi, M., Mesirov, J. P., and Tamayo, P. (2015). The molecular signatures database (MSigDB) hallmark gene set collection. Cell Syst. 1, 417–425. doi: 10.1016/j.cels.2015.12.004
Liebner, S., Corada, M., Bangsow, T., Babbage, J., Taddei, A., Czupalla, C. J., et al. (2008). Wnt/beta-catenin signaling controls development of the blood-brain barrier. J. Cell Biol. 183, 409–417. doi: 10.1083/jcb.200806024
Martowicz, A., Trusohamn, M., Jensen, N., Wisniewska-Kruk, J., Corada, M., Ning, F. C., et al. (2019). Endothelial beta-Catenin signaling supports postnatal brain and retinal angiogenesis by promoting sprouting, tip cell formation, and VEGFR (vascular endothelial growth factor receptor) 2 expression. Arterioscler Thromb. Vasc. Biol. 39, 2273–2288. doi: 10.1161/ATVBAHA.119.312749
Masckauchan, T. N., Shawber, C. J., Funahashi, Y., Li, C. M., and Kitajewski, J. (2005). Wnt/beta-catenin signaling induces proliferation, survival and interleukin-8 in human endothelial cells. Angiogenesis 8, 43–51. doi: 10.1007/s10456-005-5612-9
Munji, R. N., Soung, A. L., Weiner, G. A., Sohet, F., Semple, B. D., Trivedi, A., et al. (2019). Profiling the mouse brain endothelial transcriptome in health and disease models reveals a core blood-brain barrier dysfunction module. Nat. Neurosci. 22, 1892–1902. doi: 10.1038/s41593-019-0497-x
Nakagawa, A., Naito, A. T., Sumida, T., Nomura, S., Shibamoto, M., Higo, T., et al. (2016). Activation of endothelial beta-catenin signaling induces heart failure. Sci. Rep. 6:25009. doi: 10.1038/srep25009
Nolan, D. J., Ginsberg, M., Israely, E., Palikuqi, B., Poulos, M. G., James, D., et al. (2013). Molecular signatures of tissue-specific microvascular endothelial cell heterogeneity in organ maintenance and regeneration. Dev. Cell 26, 204–219. doi: 10.1016/j.devcel.2013.06.017
Olsavszky, V., Sticht, C., Schmid, C. D., Winkler, M., Wohlfeil, S. A., Olsavszky, A., et al. (2020). Exploring the transcriptomic network of multi-ligand scavenger receptor Stabilin-1- and Stabilin-2-deficient liver sinusoidal endothelial cells. Gene 768:145284. doi: 10.1016/j.gene.2020.145284
Pfaffl, M. W. (2001). A new mathematical model for relative quantification in real-time RT-PCR. Nucleic Acids Res. 29:e45. doi: 10.1093/nar/29.9.e45
Planas-Paz, L., Orsini, V., Boulter, L., Calabrese, D., Pikiolek, M., Nigsch, F., et al. (2016). The RSPO-LGR4/5-ZNRF3/RNF43 module controls liver zonation and size. Nat. Cell Biol. 18, 467–479. doi: 10.1038/ncb3337
Poisson, J., Lemoinne, S., Boulanger, C., Durand, F., Moreau, R., Valla, D., et al. (2017). Liver sinusoidal endothelial cells: Physiology and role in liver diseases. J. Hepatol. 66, 212–227. doi: 10.1016/j.jhep.2016.07.009
Preziosi, M., Okabe, H., Poddar, M., Singh, S., and Monga, S. P. (2018). Endothelial Wnts regulate beta-catenin signaling in murine liver zonation and regeneration: a sequel to the Wnt-Wnt situation. Hepatol. Commun. 2, 845–860. doi: 10.1002/hep4.1196
Profaci, C. P., Munji, R. N., Pulido, R. S., and Daneman, R. (2020). The blood-brain barrier in health and disease: important unanswered questions. J. Exp. Med. 217:e20190062. doi: 10.1084/jem.20190062
Rhee, S., Chung, J. I., King, D. A., D'amato, G., Paik, D. T., Duan, A., et al. (2018). Endothelial deletion of Ino80 disrupts coronary angiogenesis and causes congenital heart disease. Nat. Commun. 9:368. doi: 10.1038/s41467-017-02796-3
Rocha, A. S., Vidal, V., Mertz, M., Kendall, T. J., Charlet, A., Okamoto, H., et al. (2015). The angiocrine factor rspondin3 is a key determinant of liver zonation. Cell Rep. 13, 1757–1764. doi: 10.1016/j.celrep.2015.10.049
Sabbagh, M. F., Heng, J. S., Luo, C., Castanon, R. G., Nery, J. R., Rattner, A., et al. (2018). Transcriptional and epigenomic landscapes of CNS and non-CNS vascular endothelial cells. Elife 7:e36187. doi: 10.7554/eLife.36187.042
Sawane, M., Kajiya, K., Kidoya, H., Takagi, M., Muramatsu, F., and Takakura, N. (2013). Apelin inhibits diet-induced obesity by enhancing lymphatic and blood vessel integrity. Diabetes 62, 1970–1980. doi: 10.2337/db12-0604
Sawane, M., Kidoya, H., Muramatsu, F., Takakura, N., and Kajiya, K. (2011). Apelin attenuates UVB-induced edema and inflammation by promoting vessel function. Am. J. Pathol. 179, 2691–2697. doi: 10.1016/j.ajpath.2011.08.024
Schaffner, F., and Popper, H. (1963). Capillarization of hepatic sinusoids in man. Gastroenterology 44, 239–242. doi: 10.1016/S0016-5085(63)80130-4
Schledzewski, K., Geraud, C., Arnold, B., Wang, S., Grone, H. J., Kempf, T., et al. (2011). Deficiency of liver sinusoidal scavenger receptors stabilin-1 and−2 in mice causes glomerulofibrotic nephropathy via impaired hepatic clearance of noxious blood factors. J. Clin. Invest. 121, 703–714. doi: 10.1172/JCI44740
Shetty, S., Lalor, P. F., and Adams, D. H. (2018). Liver sinusoidal endothelial cells–gatekeepers of hepatic immunity. Nat. Rev. Gastroenterol. Hepatol. 15, 555–567. doi: 10.1038/s41575-018-0020-y
Srinivas, S., Watanabe, T., Lin, C. S., William, C. M., Tanabe, Y., Jessell, T. M., et al. (2001). Cre reporter strains produced by targeted insertion of EYFP and ECFP into the ROSA26 locus. BMC Dev. Biol. 1:4. doi: 10.1186/1471-213X-1-4
Stenman, J. M., Rajagopal, J., Carroll, T. J., Ishibashi, M., Mcmahon, J., and Mcmahon, A. P. (2008). Canonical Wnt signaling regulates organ-specific assembly and differentiation of CNS vasculature. Science 322, 1247–1250. doi: 10.1126/science.1164594
Subramanian, A., Tamayo, P., Mootha, V. K., Mukherjee, S., Ebert, B. L., Gillette, M. A., et al. (2005). Gene set enrichment analysis: a knowledge-based approach for interpreting genome-wide expression profiles. Proc. Natl. Acad. Sci. U.S.A. 102, 15545–15550. doi: 10.1073/pnas.0506580102
Tran, K. A., Zhang, X., Predescu, D., Huang, X., Machado, R. F., Gothert, J. R., et al. (2016). Endothelial beta-catenin signaling is required for maintaining adult blood-brain barrier integrity and central nervous system homeostasis. Circulation 133, 177–186. doi: 10.1161/CIRCULATIONAHA.115.015982
Walter, T. J., Cast, A. E., Huppert, K. A., and Huppert, S. S. (2014). Epithelial VEGF signaling is required in the mouse liver for proper sinusoid endothelial cell identity and hepatocyte zonation in vivo. Am. J. Physiol. Gastrointest. Liver Physiol. 306, G849–G862. doi: 10.1152/ajpgi.00426.2013
Wang, B., Zhao, L., Fish, M., Logan, C. Y., and Nusse, R. (2015). Self-renewing diploid Axin2(+) cells fuel homeostatic renewal of the liver. Nature 524, 180–185. doi: 10.1038/nature14863
Wang, Y., Sabbagh, M. F., Gu, X., Rattner, A., Williams, J., and Nathans, J. (2019). Beta-catenin signaling regulates barrier-specific gene expression in circumventricular organ and ocular vasculatures. Elife 8:e43257. doi: 10.7554/eLife.43257.035
Wild, S. L., Elghajiji, A., Grimaldos Rodriguez, C., Weston, S. D., Burke, Z. D., and Tosh, D. (2020). The canonical Wnt pathway as a key regulator in liver development, differentiation and homeostatic renewal. Genes (Basel) 11:1163. doi: 10.3390/genes11101163
Winkler, M., Staniczek, T., Kurschner, S. W., Schmid, C. D., Schonhaber, H., Cordero, J., et al. (2021). Endothelial GATA4 controls liver fibrosis and regeneration by preventing a pathogenic switch in angiocrine signaling. J. Hepatol. 74, 380–393. doi: 10.1016/j.jhep.2020.08.033
Wisse, E., De Zanger, R. B., Charels, K., Van Der Smissen, P., and Mccuskey, R. S. (1985). The liver sieve: considerations concerning the structure and function of endothelial fenestrae, the sinusoidal wall and the space of Disse. Hepatology 5, 683–692. doi: 10.1002/hep.1840050427
Wittlich, M., Dudek, M., Bottcher, J. P., Schanz, O., Hegenbarth, S., Bopp, T., et al. (2017). Liver sinusoidal endothelial cell cross-priming is supported by CD4 T cell-derived IL-2. J. Hepatol. 66, 978–986. doi: 10.1016/j.jhep.2016.12.015
Wohlfeil, S. A., Hafele, V., Dietsch, B., Schledzewski, K., Winkler, M., Zierow, J., et al. (2019). Hepatic endothelial notch activation protects against liver metastasis by regulating endothelial-tumor cell adhesion independent of angiocrine signaling. Cancer Res. 79, 598–610. doi: 10.1158/0008-5472.CAN-18-1752
Yokomori, H., Oda, M., Yoshimura, K., and Hibi, T. (2012). Enhanced expressions of apelin on proliferative hepatic arterial capillaries in human cirrhotic liver. Hepatol. Res. 42, 508–514. doi: 10.1111/j.1872-034X.2011.00945.x
Yu, G., Wang, L. G., Han, Y., and He, Q. Y. (2012). clusterProfiler: an R package for comparing biological themes among gene clusters. OMICS 16, 284–287. doi: 10.1089/omi.2011.0118
Zhang, L., Hoffman, J. A., and Ruoslahti, E. (2005). Molecular profiling of heart endothelial cells. Circulation 112, 1601–1611. doi: 10.1161/CIRCULATIONAHA.104.529537
Zhang, X. J., Olsavszky, V., Yin, Y., Wang, B., Engleitner, T., Ollinger, R., et al. (2020). Angiocrine hepatocyte growth factor signaling controls physiological organ and body size and dynamic hepatocyte proliferation to prevent liver damage during regeneration. Am. J. Pathol. 190, 358–371. doi: 10.1016/j.ajpath.2019.10.009
Keywords: mice, liver, liver sinusoidal endothelial cells, endothelial cells, triglycerides
Citation: Koch P-S, Sandorski K, Heil J, Schmid CD, Kürschner SW, Hoffmann J, Winkler M, Staniczek T, de la Torre C, Sticht C, Schledzewski K, Taketo MM, Trogisch FA, Heineke J, Géraud C, Goerdt S and Olsavszky V (2021) Imbalanced Activation of Wnt-/β-Catenin-Signaling in Liver Endothelium Alters Normal Sinusoidal Differentiation. Front. Physiol. 12:722394. doi: 10.3389/fphys.2021.722394
Received: 08 June 2021; Accepted: 19 August 2021;
Published: 29 September 2021.
Edited by:
Leo A. van Grunsven, Vrije University Brussel, BelgiumReviewed by:
Shishir Shetty, University of Birmingham, United KingdomJulie Siegenthaler, University of Colorado School of Medicine, United States
Copyright © 2021 Koch, Sandorski, Heil, Schmid, Kürschner, Hoffmann, Winkler, Staniczek, de la Torre, Sticht, Schledzewski, Taketo, Trogisch, Heineke, Géraud, Goerdt and Olsavszky. This is an open-access article distributed under the terms of the Creative Commons Attribution License (CC BY). The use, distribution or reproduction in other forums is permitted, provided the original author(s) and the copyright owner(s) are credited and that the original publication in this journal is cited, in accordance with accepted academic practice. No use, distribution or reproduction is permitted which does not comply with these terms.
*Correspondence: Philipp-Sebastian Koch, cGhpbGlwcC5rb2NoQHVtbS5kZQ==
†These authors have contributed equally to this work and share first authorship