- 1Department of Surgical and Radiological Sciences, School of Veterinary Medicine, University of California, Davis, Davis, CA, United States
- 2Department of Surgery, School of Medicine, University of California, Davis, Davis, CA, United States
- 3Cardiovascular Research Institute, University of California, San Francisco, San Francisco, CA, United States
- 4Department of Pediatrics, School of Medicine, University of California, San Francisco, San Francisco, CA, United States
In a model of congenital heart disease (CHD), we evaluated if chronically increased pulmonary blood flow and pressure were associated with altered respiratory mechanics and gas exchange. Respiratory mechanics and gas exchange were evaluated in 6 shunt, 7 SHAM, and 7 control age-matched lambs. Lambs were anesthetized and mechanically ventilated for 15 min with tidal volume of 10 mL/kg, positive end-expiratory pressure of 5 cmH2O, and inspired oxygen fraction of 0.21. Respiratory system, lung and chest wall compliances (Crs, CL and Ccw, respectively) and resistances (Rrs, RL and Rcw, respectively), and the profile of the elastic pressure-volume curve (%E2) were evaluated. Arterial blood gases and volumetric capnography variables were collected. Comparisons between groups were performed by one-way ANOVA followed by Tukey-Kramer test for normally distributed data and with Kruskal–Wallis test followed by Steel-Dwass test for non-normally distributed data. Average Crs and CL in shunt lambs were 30% and 58% lower than in control, and 56% and 68% lower than in SHAM lambs, respectively. Ccw was 52% and 47% higher and Rcw was 53% and 40% lower in shunt lambs compared to controls and SHAMs, respectively. No difference in %E2 was identified between groups. No difference in respiratory mechanics was observed between control and SHAM lambs. In shunt lambs, Rcw, Crs and CL were decreased and Ccw was increased when compared to control and SHAM lambs. Pulmonary gas exchange did not seem to be impaired in shunt lambs when compared to controls and SHAMs.
Introduction
Congenital heart disease (CHD) is the most common birth defect, affecting ∼8 in every 1000 live births in the United States (Hoffman and Kaplan, 2002) and the most common of these, ventricular septal defects and patent ductus arteriosus (VSDs, PDA) result in left to right shunting and increased pulmonary blood flow (PBF). Children with this physiology often live with increased PBF and pulmonary artery pressure (PAp) for weeks or months before their defects are surgically corrected (Mitchell et al., 1971), and the associated respiratory dysfunction and morbidity is well-described (Fitzgerald and Sherwood, 2007). Several studies evaluating respiratory mechanics in patients with CHD and increased PBF have consistently found that these patients have restrictive pulmonary function (Healy et al., 2012). For example, Howlett (Howlett, 1972) compared lung mechanics in infants with and without CHD, matched for length and height, and found that those with CHD had decreased lung compliance (CL). Similarly, Bancalari and colleagues (Bancalari et al., 1977), when comparing respiratory mechanics in infants with CHD and either increased or decreased PBF, found that respiratory rate and pulmonary resistance were higher in infants with increased PBF and that their CL was significantly lower than in those with decreased PBF (Bancalari et al., 1977). In these patients, the decrease in CL correlated with elevated mean pulmonary artery pressure. Collectively, these results suggest that the derangements in respiratory mechanics observed in CHD is caused by a combination on increase in PBF and PAp.
Many of these children continue to have abnormal respiratory mechanics several years after successful surgical correction of their cardiac defect. In a study from the mid-1990s, pulmonary function testing in children, who were on average 9 years out from a successful VSD repair, revealed that nearly three-quarters of them had decreased lung compliance and increased lung recoil pressure (Sulc et al., 1996). This respiratory dysfunction lasts into adulthood (Rex et al., 2019) and worsens with age (Maagaard et al., 2020). However, the underlying mechanisms that lead to this abnormal and persistent respiratory physiology are not at all understood. In order to better understand the cardiorespiratory interactions that might contribute to respiratory dysfunction in patients who experience chronically increased PBF, a large animal model is needed.
We have previously developed a surgical large animal model of increased PBF and pressure that we have used to study derangements of the pulmonary vasculature in this setting (Reddy et al., 1995; Black et al., 2000; Steinhorn et al., 2001; Black et al., 2003; Lakshminrusimha et al., 2007; Datar et al., 2012; Oishi et al., 2013; Datar et al., 2014; Johnson Kameny et al., 2019; Zhu et al., 2020; Boehme et al., 2021). In late gestation fetal lambs, a large 8 mm vascular graft is placed between the ascending aortic trunk and the main pulmonary artery (Reddy et al., 1995). After spontaneous delivery and with air-breathing, as pulmonary vascular resistance falls, there develops unrestricted left to right shunting that leads to chronic and torrential PBF (Reddy et al., 1995). Just as with infants and children with CHD and increased PBF (Healy et al., 2012), these ‘shunt’ lambs are tachypneic and have labored work of breathing. In addition, chest radiography and computed tomography demonstrate generalized cardiomegaly with increased vascular markings, arterial and venous distention, and patchy interstitial pulmonary infiltrates in shunt lambs (Datar et al., 2012). Thus, shunt lambs replicate with high fidelity the cardiorespiratory pathophysiology of unrepaired patients with CHD and increased PBF and pressure.
The aim of this study was to evaluate the respiratory mechanics and gas exchange in this large animal model of CHD, and to provide a benchmark for future studies that evaluate respiratory mechanics after repair of the cardiac defect (closure of the shunt).
Materials and methods
Animals
This study followed the ARRIVE guidelines and was approved by the Institutional Animal Care and Use Committee of the University of California, Davis (no 21594).
Twenty lambs aging between 28 and 46 days old and weighing between 10 and 21 kg were allocated in three experimental groups: control, shunt, and SHAM. The shunt group had six lambs with a surgically created anastomosis between the pulmonary artery and the aorta, as a model of CHD with increased PBF and pressure, previously described in detail elsewhere (Reddy et al., 1995; Steinhorn et al., 2001; Datar et al., 2012). In summary, after a lateral thoracotomy, an 8.0-mm Gore-tex vascular graft (W.L. Gore and Associates, Milpitas, CA) was anastomosed between the ascending aorta and main pulmonary artery in fetuses at 135–138 days of gestation (full term is 145 days) from mixed-breed Western pregnant ewes. The control group was composed of seven lambs that did not undergo any fetal surgical procedure but in two cases were twins of shunts. The SHAM group was designed to exclude any unanticipated effects that a fetal thoracotomy procedure might have on our assessment of respiratory mechanics. We performed sham-shunt operations on late-gestation fetal lambs, as above and (Reddy et al., 1995; Steinhorn et al., 2001; Datar et al., 2012). These SHAMs experienced identical anesthesia and instrumentation, including subcutaneous and intramuscular injections of local anesthetic at the thoracotomy site. An incision in the fourth intercostal space through the intercostal muscles was followed by full retraction of the ribs as was done when placing the actual aortopulmonary anastomosis. The deep and superficial planes of the intercostal incision site were subsequently suture-closed in a manner identical to the actual shunt procedure (Steinhorn et al., 2001; Datar et al., 2012).
Anesthesia and instrumentation
Anesthesia was induced in lambs by the intravenous administration of propofol (4 mg/kg) and ketamine (2.5 mg/kg). A cuffed endotracheal tube between 5.0 and 7.0 internal diameter was orally placed into the trachea and connected to a rebreathing circle system. During the entire experiment, anesthesia was maintained by an end-tidal concentration of isoflurane (ETISO) between 1.2% and 2.0% and the lambs were positioned in sternal recumbency. Mechanical ventilation was performed in volume control (constant flow) with a tidal volume (VT) of 10 mL/kg, positive end-expiratory pressure (PEEP) of 5 cmH2O and target inspiratory fraction of oxygen (FIO2) of 0.21 (Flow-I C-20, MAQUET Medical Systems United States of America, Wayne, NJ). Respiratory rate (RR) was adjusted to maintain the end-expiratory partial pressure of CO2 (PETCO2) between 35 and 45 mmHg. Heart rate and rhythm were monitored with electrocardiogram, and pulse oximetry (SpO2) was measured at the tongue, tail, or ear (AS/3, Datex/Ohmeda, Finland). A 22-gauge catheter (Surflo, Terumo Corporation, NJ, United States of America) was placed in the auricular or coccygeal artery for the measurement of blood pressure and sampling to measure blood gases. The blood pressure transducer was maintained at the level of the heart and was calibrated against a mercury column prior to the experiments. A pediatric mainstream flow/CO2 sensor (Respironics Novametrix, LLC, Wallingford, CT) was positioned between the endotracheal tube and the breathing system to measure PETCO2 and to collect the continuous signals of CO2 partial pressure (PCO2) for an offline volumetric capnography analysis (NM3, Philips Respironics, Wallingford, CT). A screen pneumotachometer (4500, Hans Rudolph Inc., Shawnee, KS) connected to a differential pressure transducer (DPL 2.5, Hugo Sacks Elektronik-Harvard Apparatus, Germany) was positioned distal to the flow/CO2 sensor and used to measure airflow (V̇). Airway opening pressure (Pao) was measured from a port located on the pneumotachometer by a calibrated pressure transducer (MPX, Hugo Sacks Elektronik-Harvard Apparatus, Germany). A polypropylene balloon tipped catheter was positioned in the esophagus of the lambs for the measurement of esophageal pressure (Peso) as an estimate of pleural pressure. The esophageal catheter was connected to a pressure transducer (P75, Hugo Sacks Elektronik-Harvard Apparatus, Germany) and its correct position verified by the observation of equal variation in Pao and Peso during the external compression of the thorax while the endotracheal tube was occluded, as described by Lanteri and coworkers (Lanteri et al., 1994). Details of the esophageal balloon catheter used in this study and a graphical representation of the dynamic response of the Peso and Pao during the occlusion maneuver is presented in the Supplementary Figure S1. Rectal temperature was monitored during the experiments and reported in Celsius degrees.
Experimental protocol
After instrumentation, an alveolar recruitment maneuver based on a slow inflation of the lungs with 2–3 L/min until Pao reached 40 cmH2O was performed to standardize the lung volume history in all lambs. After that, the lambs were ventilated for 15 min with the same ventilatory settings used during the instrumentation. During the last minute of ventilation, an arterial blood sample of 1 mL was collected in heparinized syringes (PICO 70, Radiometer America Inc., IL), for the immediate measurement of the arterial partial pressure of O2 (PaO2) and CO2 (PaCO2) (ABL 825, Radiometer America Inc., Brea, CA). Arterial blood gas values were not corrected for body temperature because no algorithm for temperature correction was found for sheep blood. Dynamic respiratory mechanics and volumetric capnography data were collected during the last 2 minutes of ventilation. After the 15 min of ventilation protocol, the delivery of isoflurane was stopped, and the lambs recovered from anesthesia. Once the lambs were extubated and presented coordinated movements, they were placed in a transport crate and returned to the animal vivarium facility. The timeline of the experiment is presented in Figure 1.
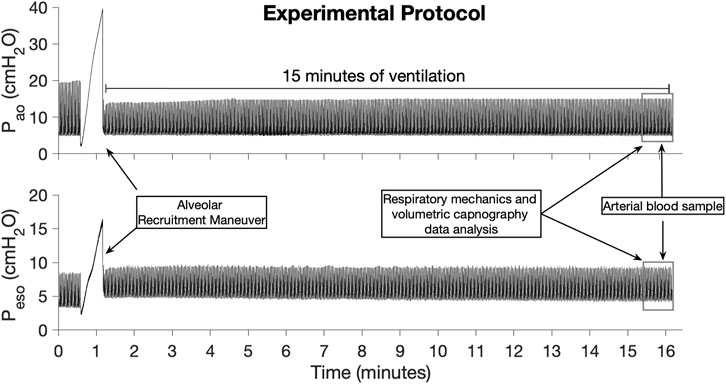
FIGURE 1. Timeline of the experiments illustrated by the airway opening pressure (Pao) and esophageal pressure (Peso) signals collected in one of the control lambs.
Data acquisition and calibrations
The analog signals of arterial blood pressure, V̇, Pao, and Peso were passed through signal conditioner modules (TAM-A Modified, Hugo Sacks Elektronik–Harvard Apparatus GmbH, Germany) and the analog signal of PCO2 and a second V̇ signal were collected from the analog output port of the volumetric capnography monitor (NM3, Philips Respironics, Wallingford, CT) to compute physiologic dead space (VDphys) and anatomic dead space (VDANAT). All analog signals were digitized at 400 Hz using an eight channel, 14-bit analog to digital board (NI USB 6009, NI, Austin, TX). The digital signals were acquired by a dedicated data acquisition system (LabView 2017; NI, Austin, TX) and saved on a personal computer for offline processing.
Calibration curves for the Pao and Peso transducers were estimated by a simple linear regression function of 5 pressure points (0, 10, 20, 30 and 40 cmH2O) obtained with a water column and their respective voltage recorded on the data acquisition system. Calibration of the flow measurements was made by a polynomial curve fitting procedure (Giannella-Neto et al., 1992) applied to a wide range of flows generated by a volumetric calibration syringe (5540, Hans Rudolph Inc., Shawnee, KS). The gas mixture used to calibrate the pneumotachometer had a composition similar to that used during the experiments (O2 and isoflurane concentrations of 21% and 1.5%, respectively). The infrared CO2 sensor was calibrated by simple linear regression using standard calibration tanks containing 3.1, 5.0, 8.1 (Puritan Bennet, Overland Park, KS) and 11.2% CO2 (Radiometer America Inc., Brea, CA). Barometric pressure (PB) was measured by a digital barometer (Fisher Scientific, Hampton, NH) at the time of calibration. All calibrations were performed immediately before each experiment.
Gas exchange
Alveolar partial pressure of oxygen (PAO2) was calculated using the alveolar air equation described below using partial pressure of gases at 37°C.
where PB is the barometric pressure measured in mmHg at the time of the experiment, R was the respiratory quotient considered 0.74 for sheep (Keenan et al., 1990). PAO2—PaO2 (PA-aO2) was calculated for each animal.
A previously validated 4-parameter model was fitted to the volumetric capnography signals using a custom-made implementation of Gauss-Newton algorithm (Motta-Ribeiro et al., 2020).
where V is volume, PCO2 is the partial pressure of CO2 in mmHg and a, b, c and d are parameters of the model.
VDanat and physiologic dead space fraction (VDphys/VT) were calculated as follows. VDanat was considered as the inflexion point of the 4-parameter model (parameter c) (Motta-Ribeiro et al., 2020), and was also expressed as a fraction of VT (VDanat/VT).
where PACO2 is the mean alveolar CO2 partial pressure (mmHg) calculated from the middle part of the phase III of the volumetric capnography curve as described by Tusman et al. (Tusman et al., 2011), and PĒCO2 is the mixed expired CO2 partial pressure. Alveolar dead space fraction (VDalv/VT) was calculated as VDphys/VT–VDanat/VT. The slope of the phase III (SIII) was considered the asymptotic slope of the model (parameter a) and was normalized (SIIIn) for the mixed expired fraction of CO2 (FĒCO2), calculated by the area under the curve of the volumetric capnogram divided by VT.
Respiratory mechanics
Volume (V) was calculated by the numeric integration of the V̇ signal and the values of V̇ and V were reported as BTPS. The endotracheal resistive pressure (PResETT) was calculated using a quadratic volume-dependent model as:
where t is time, V̇̇ was the acceleration of the gases, and K1ETT, K2ETT and InETT were the endotracheal tube values of linear resistance, flow-dependent resistance and inertance, respectively, obtained from reported values of ETT sizes 5.0 to 6.5 (Guttmann et al., 2000). To exclude the resistive properties of the ETT on the estimates of respiratory system and lung mechanics, tracheal pressure (PTracheal) was calculated for the entire respiratory cycle by Pao (t)—PResETT (t).
Dynamic respiratory mechanics was evaluated using a single-compartment model including a volume-dependent elastance and an inertial term. Respiratory system, lung and chest wall respective volume-independent elastance (E1rs, E1L, and E1cw), volume-dependent elastance (E2rs, E2L, and E2cw), resistance (Rrs, RL, and Rcw) and inertance (Inrs, InL, and Incw) were estimated by multiple linear regression (least square method) of the models of the equation of motion using their respective driving pressures (Lanteri et al., 1995a).
where Ptp is transpulmonary pressure (PTracheal–Peso), and PTracheal,0, Ptp,0, and Peso,0 are PTracheal, Ptp and Peso, respectively, when V,
If the elastic pressure-volume curve has an upward concavity, %E2 values are positive indicating that Ers increases during inspiration. On the other hand, a negative %E2 reveals a downward concavity on the elastic pressure-volume curve due to a decrease in Ers during inspiration. If the relation between elastic pressure and volume is linear %E2 approaches 0%. Values of %E2 higher than 20% and lower than −10% have been associated to tidal overdistention and recruitment/derecruitment, respectively, with values between −10% and 20% considered linear distention (Beda et al., 2017). Compliance was calculated for the total respiratory system (Crs), chest wall (Ccw) and lungs (CL) by 1/Ers, 1/Ecw and 1/EL, respectively, and normalized to the body weight in kg of each lamb. Rrs, RL and Rcw were normalized to the body weight of each lamb using a power law described by Stahl (Stahl, 1967).
Other measurements
From the same arterial blood sample used for the PaO2 and PaCO2 measurements, pH was measured and bicarbonate ion (HCO3−) concentration calculated. In addition, the arterial blood concentrations of lactate, glucose, Na+, Cl−, K+ and Ca++ were measured. All those measurements were performed by the same analyzer (ABL 825, Radiometer America Inc., Brea, CA).
Statistical analysis
The main variables of this study were Crs, CL, Ccw, Rrs, RL, and Rcw. Secondary variables were PA-aO2, SIIIn, VDbohr/VT, VDanat and VDalv/VT. Shapiro-Wilk test was used to verify normality of the continuous variables. Normally distributed data were reported as mean ±95% confidence interval and non-normally distributed data as median (range). Comparisons between groups with normal and non-normal distribution were performed by one-way analysis of variance followed by a Tukey-Kramer HSD test and a Kruskal–Wallis test followed by Steel-Dwass test, respectively. Statistical analysis was performed with a commercial statistics software (JMP Pro16.0.0, SAS Institute Inc., NC, United States of America) and p < 0.05 was considered enough to reject the null hypothesis.
Results
All 20 lambs successfully completed the experiments. Arterial blood gas data from one lamb in the control group were not included in the results because of a malfunction in the blood gas analyzer on the day of the experiment. Because the esophageal balloon catheter got displaced during the experiment of one SHAM lamb, data of CL, Ccw, RL and Rcw of only 6 SHAM lambs are presented.
The demographic data and rectal temperature from shunt, control and SHAM lambs are presented in Table 1. There was no difference of age, body weight and rectal temperature between groups. Ventilatory variables and ETISO measured during the experiments are presented in Table 2. As expected, there was no significant difference between groups for VT, PEEP, ETISO, and FIO2 between groups. In addition, fR and peak tracheal pressure were not different between groups.

TABLE 1. Demographic data and rectal temperature in shunt, control and SHAM lambs mechanically ventilated for 15 min (mean ±95% confidence interval).

TABLE 2. Ventilatory variables and isoflurane end-tidal concentration (ETISO) in shunt, control and SHAM lambs mechanically ventilated for 15 min [values are expressed as mean ±95% confidence interval or median (range)].
The main respiratory mechanics results of control, SHAM, and shunt lambs are reported in Figure 2. Average Crs and CL in shunt lambs were 30% and 58% lower than in control, and 56% and 68% lower than in SHAM lambs, respectively. Ccw was 52% and 47% higher and Rcw was 53% and 40% lower in shunt lambs compared to controls and SHAMs, respectively. No significant differences between groups were found for Rrs and RL. Median and range of %E2 were 10.8 (−11.3 21.3), −1.2 (−9.6 40.9) and 11.0 (7.1 32.8) in control, SHAM and shunt lambs, respectively, with no significant difference between groups. Based on the %E2 results, alveolar tidal recruitment/derecruitment was identified in two lambs of the control group (−11.3% and −10.2%%), and overdistention values were found in one lamb of each group (21.3% - control; 40.9% - SHAM, 32.8% - shunt). All parameters of the respiratory mechanics models used in this study are presented in Supplementary Table S2.
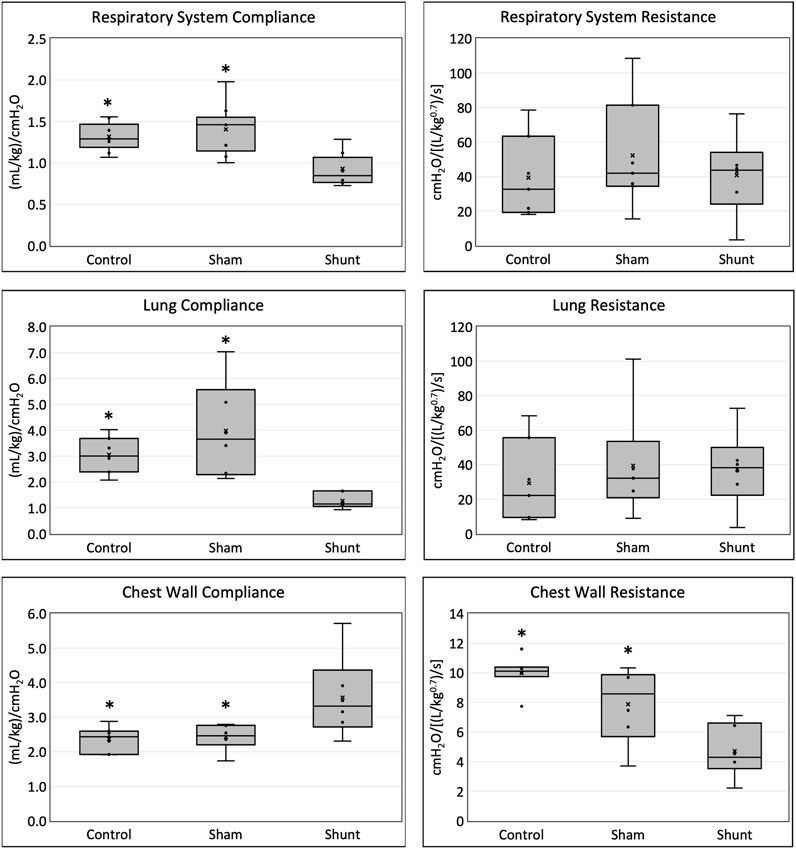
FIGURE 2. Respiratory system, lung and chest wall compliances and resistances in shunt, control and SHAM lambs mechanically ventilated for 15 min (* = significant difference between shunt lambs).
Pulmonary gas exchange variables achieved during the experiments are reported in Table 3 and Figure 3. No significant difference between groups was identified for all pulmonary gas exchange variable. The arterial blood pH, and the concentrations of HCO3−, glucose, lactate, Cl−, K+, and Ca++ were also not different between groups (Table 4).

TABLE 3. Pulmonary gas exchange variables in control, SHAM and shunt lambs mechanically ventilated for 15 min. Values are expressed as mean ±95% confidence interval or median (range).
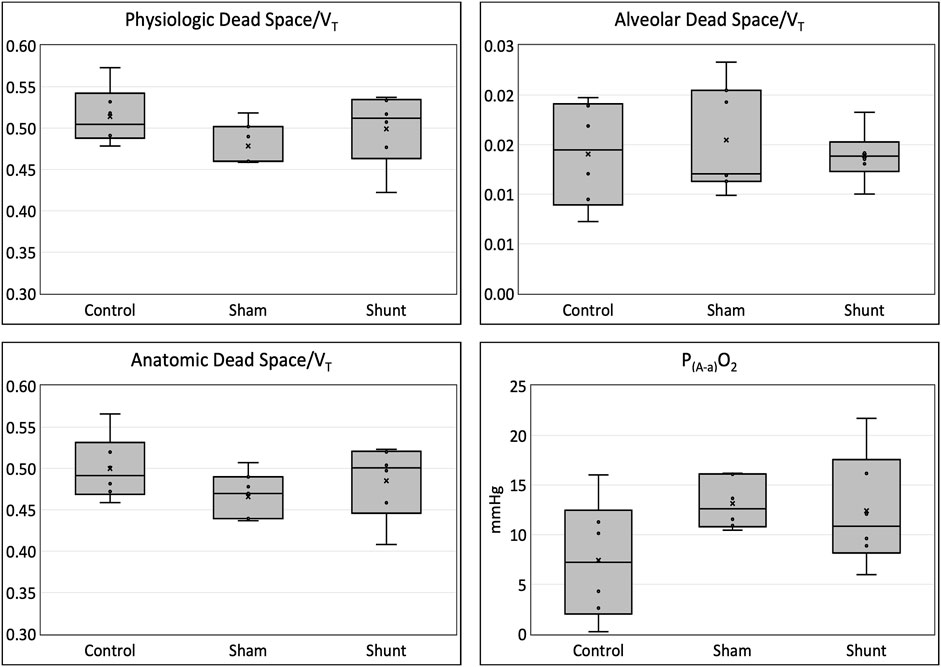
FIGURE 3. Physiologic, Anatomic and alveolar dead space fractions and alveolar-to-arterial oxygen partial pressure difference [P(A-a)O2] in shunt, control and SHAM lambs mechanically ventilated for 15 min.
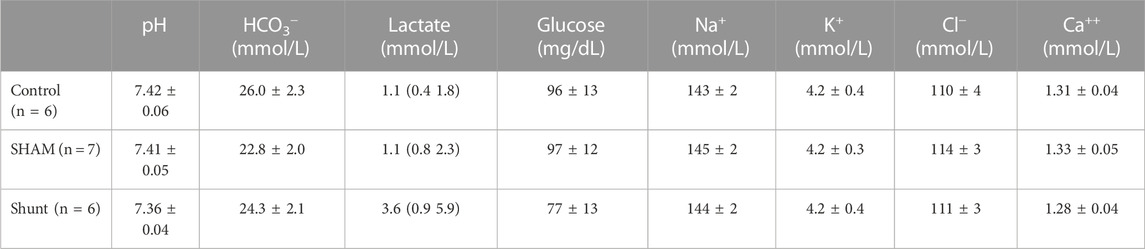
TABLE 4. Acid-base variables, lactate, glucose and electrolytes measured in the arterial blood of control and shunt lambs mechanically ventilated for 15 min. Values are expressed as mean ±95% confidence interval or median (range).
Discussion
The main findings of this study of respiratory mechanics and gas exchange in our surgical ovine model of CHD with increased PBF and pressure were: 1) Crs and CL were lower and Ccw was higher in shunt lambs than in age-matched control and SHAM lambs; 2) Rcw was lower in shunts, but Rrs and RL were no different than in controls and SHAMs; and 3) pulmonary gas exchange was not different among control, SHAM and shunt lambs.
The ovine model of CHD with increased PBF and pressure used in this study replicates the typical features of the human disease, including elevated postnatal pulmonary arterial pressure and blood flow, with the associated derangements of pulmonary vascular structure and function (Reddy et al., 1995; Datar et al., 2012; Datar et al., 2014; Morris et al., 2018; Johnson Kameny et al., 2019; Zhu et al., 2020; Boehme et al., 2021). Although pulmonary artery pressure and pulmonary to systemic blood flow ratio (Q̇p/Q̇s) were not measured during these experiments, hemodynamics were measured in 5 shunt lambs during subsequent evaluations at between 8 and 15 weeks of age while under similar anesthetic conditions, which confirmed that these shunt lambs did experience increased PBF and elevated pulmonary artery pressure (Supplementary Table S4). This model has been used in prior investigations that examined the effects of CHD with increased PBF on pulmonary vascular remodeling (Reddy et al., 1995; Black et al., 2000; Steinhorn et al., 2001; Black et al., 2003; Lakshminrusimha et al., 2007; Oishi et al., 2008; Johnson Kameny et al., 2019), including the pulmonary lymphatic system (Datar et al., 2012; Datar et al., 2014; Datar et al., 2016; Boehme et al., 2021). However, an evaluation of the respiratory mechanics and gas exchange of this model have not been described in detail previously. Consequently, the findings of this study can be used to better understand the effects of CHD-driven increased PBF and pressure on pulmonary function: 1) as baseline measurements for future studies investigating the underlying mechanisms that lead to persistently abnormal respiratory mechanics after the cardiac defect has been repaired (Sulc et al., 1996; Abassi et al., 2019; Rex et al., 2019; Maagaard et al., 2020), and 2) how preservation of pulmonary vascular function in this setting, might improve variables of respiratory function.
Respiratory mechanics
In this ovine model of CHD with increased PBF, Crs was lower than in control and SHAM animals as a result of a lower CL. Similar results have been also reported in infants and children with CHD with increased PBF (Wallgren et al., 1960; Davies et al., 1962; Howlett, 1972; Bancalari et al., 1977; De Troyer and Englert, 1977; Baraldi et al., 1993; Lanteri et al., 1995b; Yau et al., 1996). The reasons for a lower CL and Crs in shunt lambs is likely related to the simultaneous increase in the pulmonary blood volume and pulmonary artery pressure (PAp) (Davies et al., 1962; Griffin et al., 1972; Howlett, 1972; Bancalari et al., 1977). Any discrepancies among these studies may be related to the variable degree of increased PBF and PAp observed in different studies, as suggested by Lanteri et al. (Lanteri et al., 1995b), and/or the different techniques used to evaluate respiratory mechanics used in each study. It has also been suggested that the pulmonary vascular remodeling in response to the chronic increase in PBF and PAp might create a stiffer pulmonary scaffold that contributes to a decreased CL and Crs as well (Davies et al., 1962). Indeed, shunt lambs have a higher density of pulmonary artery branches with thicker walls and an increased smooth muscle layer, particularly in the peripheral intra-acinar arteries (Reddy et al., 1995), which supports this mechanism for the decreased CL and Crs in the shunt lambs that we identified in this study. It is also possible that the increased PBF observed in these shunt lambs could lead to increased left atrial pressure resulting pulmonary venous congestion that also contributes to decreased CL. However, shunt lambs do not demonstrate a clinically relevant elevation in left atrial pressure, and this is not a model of left heart failure (Datar et al., 2012; Datar et al., 2014; Morris et al., 2018; Johnson Kameny et al., 2019). Lastly, Wallgren et al. (Wallgren et al., 1960) proposed that the decrease in CL observed in infants with CHD and increased PBF could be related to compression atelectasis caused by their enlarged heart. This could certainly contribute to the decreased Crs and CL observed in shunt lambs because impressive right ventricular hypertrophy is a typical phenotypic trait of this model of CHD (Reddy et al., 1995; Datar et al., 2012).
The magnitude of the decrease in Crs in the shunt lambs was less than the decrease in their CL because their Ccw was higher than in the control lambs, which counterbalanced the effect of the lower CL upon Crs. The increased Ccw observed in the shunt lambs could be related to the decreased elastic properties of a less muscular, less fatty chest wall, a result of the negative energy balance normally present in CHD with increased PBF (Menon and Poskitt, 1985). Moreover, the possible larger lung volume generated in the control and SHAM when compared to shunt lambs by the use of PEEP could have placed the chest wall in a lower compliance position of its pressure-volume curve. Our results identify an important limitation of studies that evaluate only Crs to assess the degree of respiratory mechanics dysfunction in the individuals with CHD and PBF. Freezer and colleagues (Freezer et al., 1993) suggested that their inability to show decreased compliance in infants with CHD and increased PBF was possibly related to measuring Crs and not CL because the former included the contribution of the chest wall. Moreover, the accurate evaluation of CL in individuals with CHD could have pivotal importance as a prognostic marker of improvement in lung function after surgical or pharmacological shunt closure: infant patients with lower preoperative CL had the best improvement in lung function after surgical ligation of their PDA (Gerhardt and Bancalari, 1980).
The previously reported effects of CHD with increased PBF on Rrs and RL are controversial, with some studies finding no difference (Howlett, 1972; Baraldi et al., 1993) while others an increase in Rrs or RL (Bancalari et al., 1977; Freezer et al., 1993; Lanteri et al., 1995b; Yau et al., 1996). As discussed for the elastic properties, the differences among study results are likely because of differences in the methodologies used to evaluate Rrs and RL, and/or different degrees of pulmonary hemodynamic derangements in their patient population. Increases in Rrs or RL previously reported in infants or children with CHD and increased PBF (Bancalari et al., 1977; Freezer et al., 1993; Lanteri et al., 1995b; Yau et al., 1996) have been attributed to a possible decreased airway diameter from compression by the enlarged heart and vascularly engorged lung structures, or from peribronchial fluid accumulation in the small airways (Hordof et al., 1977). Using a two-compartment model, Freezer and colleagues (Freezer et al., 1993) identified that the two components of Rrs (airway resistance and viscoelastic properties of lungs and chest wall) are affected in infants with CHD with high PBF. In addition, marked increases in Rrs were observed mainly in infants with Q̇p/Q̇s greater than three. Unfortunately, Q̇p/Q̇s was not calculated coincident with these pulmonary function experiments, but Q̇p/Q̇s was subsequently calculated in five of the shunt lambs (at between 8 and 15 weeks of age); this revealed a mean Q̇p/Q̇s of 2.3 (Supplementary Table S4). Even though Q̇p/Q̇s higher than 3.0 is not uncommon in this model (Reddy et al., 1995; Datar et al., 2014), it is possible that the shunt lambs included in the study did not have enough increase in PBF to generate augmented Rrs and RL. Yet another possible reason that we did not observe a difference in Rrs and RL between control and shunt lambs as previous studies (Bancalari et al., 1977; Freezer et al., 1993; Lanteri et al., 1995b; Yau et al., 1996) did, is a potentially important difference in anesthetic conditions. In our study, the lambs were anesthetized with isoflurane and not paralyzed, while in previous studies the individuals were only sedated (Bancalari et al., 1977; Yau et al., 1996) or anesthetized with a different anesthetic and paralyzed (Freezer et al., 1993; Lanteri et al., 1995b). Chloral hydrate and trichloroethylene, the sedative and anesthetic, respectively used in previous studies (Bancalari et al., 1977; Freezer et al., 1993; Lanteri et al., 1995b), do not affect airway caliber or Rrs (Colgan, 1965; Sandberg et al., 2013). On the other hand, isoflurane causes significant bronchodilation (Dikmen et al., 2003) and could have masked small differences in Rrs and RL between shunt and control lambs.
To avoid the influence of the endotracheal tube resistance, the respiratory mechanics evaluation in the lambs of this study was performed using a calculated PTracheal. The endotracheal tube is a major source of resistance and its inclusion on the evaluation of respiratory mechanics can add a significant noise, particularly to the resistance results (Sullivan et al., 1976; Fabry et al., 1994). Furthermore, the exclusion of PresETT by calculating or directly measuring PTracheal can significantly improve the estimates of elastances and resistances (Lanteri et al., 1999; Karason et al., 2000) as well as the calculation of %E2 (Jandre et al., 2008). In the present study, PTracheal was calculated using previously published values of K1, K2, and inertance applied to the quadratic flow-dependent model (Guttmann et al., 2000). The direct measurement of PTracheal in the present study could have provided more accurate values of PTracheal, but it would likely cause an excessive increase in PresETT by the presence of a thin catheter through the small size ETTs used in the lambs of this study. The inclusion of the inertial term in the flow-dependent quadratic model used to calculate PresETT in this study aimed to improve the accuracy of the calculated PTracheal. This strategy significantly improved the accuracy of PTracheal calculation, achieving a maximal deviation between calculated and directly measured PTracheal to approximately 1 cmH2O (Guttmann et al., 2000). Lastly, the inclusion of the inertance term in the flow-dependent quadratic model of PresETT significantly improved the accuracy of the assessment of tidal recruitment/derecruitment and overdistention measured by %E2 in a simulation study (Jandre et al., 2008).
The inclusion of the inertance term on the volume-dependent single compartment model used to estimate respiratory mechanics in this study aimed to account for the effects of inertial forces associated with the relatively high fR used to maintain the target PETCO2. Inertance has been shown to significantly contribute to this model at fR higher than 20 bpm (Lanteri et al., 1999). In addition, Ers and EL were significantly underestimated with no effect on Rrs and RL when inertance was omitted from the model (Lanteri et al., 1999). In a simulation study, Turner et al. (Turner et al., 1991) demonstrated that the overestimation of Crs, when the inertial term of the model was omitted, was more significant in conditions of higher Crs, as in the control lambs. As presented in Supplementary Table S3, the same results were observed in the lambs of this study when the inertance term was omitted. In the control and shunt lambs, Crs and CL were overestimated when the model excluding the inertial term was used. In addition, Ccw and Rcw were underestimated and Rrs was underestimated in control lambs when the model without inertance was applied.
Gas exchange
The most important gas exchange abnormality reported in awake infants (Lees et al., 1967) and anesthetized children (Fletcher et al., 1986) with CHD and increased PBF is an impairment in arterial oxygenation resulting from increased pulmonary ventilation/perfusion (V̇/Q̇) mismatch (Fletcher, 1993). Differently, the shunt lambs of this study presented no significant difference in arterial oxygenation when compared to the control and SHAM animals. However, the small number of lambs used in this study likely precluded us to find significant difference in arterial oxygenation, particularly in P(A-a)O2. As previously reported in children (Fletcher et al., 1986), the physiologic dead space of the shunt individuals was not different than in the control ones. This finding was also observed in pigs where cardiac output was increased with dobutamine (Mosing et al., 2015). In addition, an increase in SIII has also been observed in children (Fletcher et al., 1986) and pigs (Mosing et al., 2015) with increased PBF. This finding was not observed in the shunt lambs even when the SIII was normalized to different sizes of VT. The increase in SIII observed when the pulmonary circulation is overloaded seems to occur due to an increased spread of V̇/Q̇ in the lungs (Fletcher et al., 1986; Fletcher, 1993). The lack of difference in SIIIn between shunt and control lambs could be related to the absence of left ventricular failure in the shunt lambs since the pulmonary gas exchange abnormalities in the presence of increased PBF are expected to be less in the absence of left ventricular failure (Fletcher, 1993). Moreover, the use of PEEP of 5 cmH2O after an alveolar recruitment maneuver in the lambs of this study could have minimized the V̇/Q̇ mismatch caused by the increased PBF, as observed when a similar ventilatory strategy was used in infants with CHD (Sun et al., 2020). Lastly, the prone position used during the experiments possibly contributed to minimize the pulmonary gas exchange derangements caused by the increase in PBF and PAp observed in this model of CHD, as observed in a swine model of ARDS (Katira et al., 2021).
Limitations
The results of this study should be interpreted in the light of important limitations. The absence of functional residual capacity, lung aeration distribution, concurrent pulmonary to systemic blood flow and pulmonary artery pressure measurements, as well as the lack of direct measurement of PTracheal during the experiments were already discussed in previous paragraphs.
The small sample size used in this study may have underpowered the study to detect significant differences in some variables such as body weight and arterial oxygenation. Nevertheless, we were able to identify significant differences in important variables of pulmonary function such as Crs, CL, Ccw and Rcw.
The prone position adopted in the lambs of this study aimed to reproduce the natural position of these animals in their awake state. It is important to highlight that prone position reduces the vertical gradient of pleural pressure maintaining a more homogeneous distribution of ventilation and consequently CL, as well as improve arterial oxygenation (Katira et al., 2021). Therefore, the results of this study should be carefully applied to other recumbencies, especially the supine position, which is the typical position used for sedated and mechanically ventilated human patients undergoing diagnostic procedures. Likely, the respiratory mechanics abnormalities identified in the shunt lambs of this study would be exacerbated in supine position, as previously observed in animal models of early lung injury (Katira et al., 2021).
Previously, we have demonstrated that SHAM fetal surgery did not contribute to the cardiovascular and pulmonary characteristics of shunt lambs (Reddy et al., 1995; Oishi et al., 2008). To evaluate any effect the thoracotomy itself might have subsequent on parameters of respiratory mechanics, we performed pulmonary function testing in SHAM lambs that had undergone a late gestation fetal thoracotomy without placement of an aortopulmonary graft. When compared with unoperated age-matched control lambs, we found no differences in any of the respiratory mechanics variables; therefore, we conclude that the lower Crs and CL in the shunt lambs were intrinsic to the pulmonary derangements resulting from the post-natal effects of the in-utero placement of the aortopulmonary graft. This conclusion is corroborated by previous studies reporting that Crs and CL improved after the correction of the pulmonary overcirculation in children with CHD (Naulty et al., 1978; Lanteri et al., 1995b). In addition, the normalization of compliances and resistances by the actual body weight of the lambs may have overestimated their values in the shunt lambs. Infants with CHD commonly fail to thrive with retarded growth and lower body mass index when compared to normal infants (Menon and Poskitt, 1985). To minimize the possible interference of this issue, previous studies normalized compliances to height (Lanteri et al., 1995b; Yau et al., 1996) or to functional residual capacity (Bancalari et al., 1977; Baraldi et al., 1993). No other morphometric measurements were available for the lambs used in this study. However, this limitation has minimal impact on our results and conclusions since there was no significant difference in body weight between groups. Paralysis was not provided in the lambs of our study mainly because of ethical concerns raised by our IACUC in regard to the monitoring of adequate depth of anesthesia during the experiments (Drummond et al., 1996). Because the use of neuromuscular blockers during passive mechanical ventilation does not seem to affect the respiratory mechanics in anesthetized individuals (Behrakis et al., 1983), we decided to not rebut our IACUC recommendation. Nonetheless, we closely monitored Peso, Paw and V̇ during the experiments to guarantee that no activity of respiratory muscles affected our respiratory mechanics data.
The normal body temperature of lambs varies between 37.9 and 40.2°C (Hamdy and Weaver, 1958), which is higher than the default temperature of our blood gas machine (37°C). To avoid errors related to the human specific algorithm to correct the blood gas results for the actual temperature of the lambs, we decided to not use temperature-corrected values for our blood gas results and for the calculation of P(A-a)O2. In fact, most lambs presented negative P(A-a)O2 when temperature-corrected values of PaO2 we used.
Conclusion
In shunt lambs, Rcw, Crs and CL were decreased and Ccw was increased when compared to control lambs. The characteristics of the respiratory mechanics in this surgical ovine model of CHD are consistent with what has been described previously for infants and children with CHD and increased PBF and pressure.
Data availability statement
The original contributions presented in the study are included in the article/Supplementary Material, further inquiries can be directed to the corresponding authors.
Ethics statement
The animal study was reviewed and approved by University of California Davis Institutional Animal Care and Use Committee.
Author contributions
JS–ORCID 0000-0001-9044-3208 - Conceived and designed research, Performed experiments, Analyzed data, Interpreted results of experiments, Prepared figures, Drafted manuscript, Edited and revised manuscript, Approved final version of manuscript. GR - Performed experiments, Edited and revised manuscript, Approved final version of manuscript. JF - Conceived and designed research, Analyzed data, Interpreted results of experiments, Edited and revised manuscript, Approved final version of manuscript. SD - Conceived and designed research, Performed experiments, Analyzed data, Interpreted results of experiments, Drafted manuscript, Edited and revised manuscript, Approved final version of manuscript. All authors contributed to the article and approved the submitted version.
Funding
This research was supported in part by grants P01HL146369 (JF) and R01HL133034 (SD) from the National Institutes of Health.
Acknowledgments
We are indebted to Frederico C. Jandre and Gabriel C. Motta-Ribeiro for kindly providing the MATLAB script for the volumetric capnography analysis. We are grateful to Rachel Hutchings, Christian Vento, Kirstie Shulman, Victoria Hammitt, and Amy Lesneski for excellent technical support and expert care of our animals.
Conflict of interest
The authors declare that the research was conducted in the absence of any commercial or financial relationships that could be construed as a potential conflict of interest.
Publisher’s note
All claims expressed in this article are solely those of the authors and do not necessarily represent those of their affiliated organizations, or those of the publisher, the editors and the reviewers. Any product that may be evaluated in this article, or claim that may be made by its manufacturer, is not guaranteed or endorsed by the publisher.
Supplementary material
The Supplementary Material for this article can be found online at: https://www.frontiersin.org/articles/10.3389/fphys.2023.1188824/full#supplementary-material
References
Abassi, H., Gavotto, A., Picot, M. C., Bertet, H., Matecki, S., Guillaumont, S., et al. (2019). Impaired pulmonary function and its association with clinical outcomes, exercise capacity and quality of life in children with congenital heart disease. Int. J. Cardiol. 285, 86–92. doi:10.1016/j.ijcard.2019.02.069
Bancalari, E., Jesse, M. J., Gelband, H., and Garcia, O. (1977). Lung mechanics in congenital heart disease with increased and decreased pulmonary blood flow. J. Pediatr. 90 (2), 192–195. doi:10.1016/s0022-3476(77)80628-8
Baraldi, E., Filippone, M., Milanesi, O., Magagnin, G., Vencato, F., Barbieri, P., et al. (1993). Respiratory mechanics in infants and young children before and after repair of left-to-right shunts. Pediatr. Res. 34 (3), 329–333. doi:10.1203/00006450-199309000-00018
Beda, A., Carvalho, A. R., Carvalho, N. C., Hammermuller, S., Amato, M. B., Muders, T., et al. (2017). Mapping regional differences of local pressure-volume curves with electrical impedance tomography. Crit. Care Med. 45 (4), 679–686. doi:10.1097/CCM.0000000000002233
Behrakis, P. K., Higgs, B. D., Baydur, A., Zin, W. A., and Milic-Emili, J. (1983). Respiratory mechanics during halothane anesthesia and anesthesia-paralysis in humans. J. Appl. Physiol. Respir. Environ. Exerc Physiol. 55 (4), 1085–1092. doi:10.1152/jappl.1983.55.4.1085
Black, S. M., Bekker, J. M., Johengen, M. J., Parry, A. J., Soifer, S. J., and Fineman, J. R. (2000). Altered regulation of the ET-1 cascade in lambs with increased pulmonary blood flow and pulmonary hypertension. Pediatr. Res. 47 (1), 97–106. doi:10.1203/00006450-200001000-00018
Black, S. M., Mata-Greenwood, E., Dettman, R. W., Ovadia, B., Fitzgerald, R. K., Reinhartz, O., et al. (2003). Emergence of smooth muscle cell endothelin B-mediated vasoconstriction in lambs with experimental congenital heart disease and increased pulmonary blood flow. Circulation 108 (13), 1646–1654. doi:10.1161/01.CIR.0000087596.01416.2F
Boehme, J. T., Morris, C. J., Chiacchia, S. R., Gong, W., Wu, K. Y., Kameny, R. J., et al. (2021). HIF-1α promotes cellular growth in lymphatic endothelial cells exposed to chronically elevated pulmonary lymph flow. Sci. Rep. 11 (1), 1468. doi:10.1038/s41598-020-80882-1
Carvalho, A. R., Spieth, P. M., Pelosi, P., Vidal Melo, M. F., Koch, T., Jandre, F. C., et al. (2008). Ability of dynamic airway pressure curve profile and elastance for positive end-expiratory pressure titration. Intensive Care Med. 34 (12), 2291–2299. doi:10.1007/s00134-008-1301-7
Colgan, F. J. (1965). Performance of lungs and bronchi during inhalation anesthesia. Anesthesiology 26 (6), 778–785. doi:10.1097/00000542-196511000-00013
Datar, S. A., Gong, W., He, Y., Johengen, M., Kameny, R. J., Raff, G. W., et al. (2016). Disrupted NOS signaling in lymphatic endothelial cells exposed to chronically increased pulmonary lymph flow. Am. J. Physiol. Heart Circ. Physiol. 311 (1), H137–H145. doi:10.1152/ajpheart.00649.2015
Datar, S. A., Johnson, E. G., Oishi, P. E., Johengen, M., Tang, E., Aramburo, A., et al. (2012). Altered lymphatics in an ovine model of congenital heart disease with increased pulmonary blood flow. Am. J. Physiol. Lung Cell. Mol. Physiol. 302 (6), L530–L540. doi:10.1152/ajplung.00324.2011
Datar, S. A., Oishi, P. E., Gong, W., Bennett, S. H., Sun, C. E., Johengen, M., et al. (2014). Altered reactivity and nitric oxide signaling in the isolated thoracic duct from an ovine model of congenital heart disease with increased pulmonary blood flow. Am. J. Physiol. Heart Circ. Physiol. 306 (7), H954–H962. doi:10.1152/ajpheart.00841.2013
Davies, H., Williams, J., and Wood, P. (1962). Lung stiffness in states of abnormal pulmonary blood flow and pressure. Br. Heart J. 24 (2), 129–138. doi:10.1136/hrt.24.2.129
De Troyer, A., and Englert, M. (1977). Mechanics of breathing in patients with atrial septal defect. Am. Rev. Respir. Dis. 115, 413–421. doi:10.1164/arrd.1977.115.3.413
Dikmen, Y., Eminoglu, E., Salihoglu, Z., and Demiroluk, S. (2003). Pulmonary mechanics during isoflurane, sevoflurane and desflurane anaesthesia. Anaesthesia 58 (8), 745–748. doi:10.1046/j.1365-2044.2003.03285.x
Drummond, J. C., Todd, M. M., and Saidman, L. J. (1996). Use of neuromuscular blocking drugs in scientific investigations involving animal subjects. The benefit of the doubt goes to the animal. Anesthesiology 85 (4), 697–699. doi:10.1097/00000542-199610000-00001
Fabry, B., Guttmann, J., Eberhard, L., and Wolff, G. (1994). Automatic compensation of endotracheal tube resistance in spontaneously breathing patients. Technol. Health Care 1 (4), 281–291. doi:10.3233/THC-1994-1405
Fitzgerald, D. A., and Sherwood, M. (2007). Long-term cardio-respiratory consequences of heart disease in childhood. Paediatr. Respir. Rev. 8 (4), 313–321. doi:10.1016/j.prrv.2007.08.006
Fletcher, R. (1993). Gas exchange during anaesthesia and controlled ventilation in children with congenital heart disease. Pediatr. Anesth. 3 (1), 5–17. doi:10.1111/j.1460-9592.1993.tb00027.x
Fletcher, R., Niklason, L., and Drefeldt, B. (1986). Gas exchange during controlled ventilation in children with normal and abnormal pulmonary circulation: A study using the single breath test for carbon dioxide. Anesth. Analg. 65 (6), 645–652. doi:10.1213/00000539-198606000-00014
Freezer, N. J., Lanteri, C. J., and Sly, P. D. (1993). Effect of pulmonary blood flow on measurements of respiratory mechanics using the interrupter technique. J. Appl. Physiol. (1985) 74 (3), 1083–1088. doi:10.1152/jappl.1993.74.3.1083
Gerhardt, T., and Bancalari, E. (1980). Lung compliance in newborns with patent ductus-arteriosus before and after surgical ligation. Biol. Neonate 38 (1-2), 96–105. doi:10.1159/000241348
Giannella-Neto, A., Vale, M. J. O., and Vidal Melo, M. F. (1992). Accurate calibration of pneumotacographs using a syringe and polynomial curve fitting. Eng. Med. Biol. Soc. 2, 693–694.
Griffin, A. J., Ferrara, J. D., Lax, J. O., and Cassels, D. E. (1972). Pulmonary compliance. An index of cardiovascular status in infancy. Am. J. Dis. Child. 123 (2), 89–95. doi:10.1001/archpedi.1972.02110080067001
Guttmann, J., Kessler, V., Mols, G., Hentschel, R., Haberthur, C., and Geiger, K. (2000). Continuous calculation of intratracheal pressure in the presence of pediatric endotracheal tubes. Crit. Care Med. 28 (4), 1018–1026. doi:10.1097/00003246-200004000-00018
Hamdy, A. H., and Weaver, C. R. (1958). Body temperature of young lambs. Am. J. Physiol. 193 (3), 539–540. doi:10.1152/ajplegacy.1958.193.3.539
Healy, F., Hanna, B. D., and Zinman, R. (2012). Pulmonary complications of congenital heart disease. Paediatr. Respir. Rev. 13 (1), 10–15. doi:10.1016/j.prrv.2011.01.007
Hoffman, J. I., and Kaplan, S. (2002). The incidence of congenital heart disease. J. Am. Coll. Cardiol. 39 (12), 1890–1900. doi:10.1016/s0735-1097(02)01886-7
Hordof, A. J., Mellins, R. B., Gersony, W. M., and Steeg, C. N. (1977). Reversibility of chronic obstructive lung disease in infants following repair of ventricular septal defect. J. Pediatr. 90 (2), 187–191. doi:10.1016/s0022-3476(77)80627-6
Howlett, G. (1972). Lung mechanics in normal infants and infants with congenital heart disease. Arch. Dis. Child. 47 (255), 707–715. doi:10.1136/adc.47.255.707
Jandre, F. C., Modesto, F. C., Carvalho, A. R., and Giannella-Neto, A. (2008). The endotracheal tube biases the estimates of pulmonary recruitment and overdistension. Med. Biol. Eng. Comput. 46 (1), 69–73. doi:10.1007/s11517-007-0227-5
Johnson Kameny, R., Datar, S. A., Boehme, J. B., Morris, C., Zhu, T., Goudy, B. D., et al. (2019). Ovine models of congenital heart disease and the consequences of hemodynamic alterations for pulmonary artery remodeling. Am. J. Respir. Cell. Mol. Biol. 60 (5), 503–514. doi:10.1165/rcmb.2018-0305MA
Karason, S., Sondergaard, S., Lundin, S., Wiklund, J., and Stenqvist, O. (2000). Evaluation of pressure/volume loops based on intratracheal pressure measurements during dynamic conditions. Acta Anaesthesiol. Scand. 44 (5), 571–577. doi:10.1034/j.1399-6576.2000.00515.x
Katira, B. H., Osada, K., Engelberts, D., Bastia, L., Damiani, L. F., Li, X., et al. (2021). Positive end-expiratory pressure, pleural pressure, and regional compliance during pronation: An experimental study. Am. J. Respir. Crit. Care Med. 203 (10), 1266–1274. doi:10.1164/rccm.202007-2957OC
Keenan, R. J., Todd, T. R., Demajo, W., and Slutsky, A. S. (1990). Effects of hypercarbia on arterial and alveolar oxygen tensions in a model of gram-negative pneumonia. J. Appl. Physiol. (1985) 68 (5), 1820–1825. doi:10.1152/jappl.1990.68.5.1820
Lakshminrusimha, S., Wiseman, D., Black, S. M., Russell, J. A., Gugino, S. F., Oishi, P., et al. (2007). The role of nitric oxide synthase-derived reactive oxygen species in the altered relaxation of pulmonary arteries from lambs with increased pulmonary blood flow. Am. J. Physiol. Heart Circ. Physiol. 293 (3), H1491–H1497. doi:10.1152/ajpheart.00185.2007
Lanteri, C. J., Kano, S., Duncan, A. W., and Sly, P. D. (1995). Changes in respiratory mechanics in children undergoing cardiopulmonary bypass. Am. J. Respir. Crit. Care Med. 152 (61), 1893–1900. doi:10.1164/ajrccm.152.6.8520752
Lanteri, C. J., Kano, S., Nicolai, T., and Sly, P. D. (1995). Measurement of dynamic respiratory mechanics in neonatal and pediatric intensive care: The multiple linear regression technique. Pediatr. Pulmonol. 19 (1), 29–45. doi:10.1002/ppul.1950190106
Lanteri, C. J., Kano, S., and Sly, P. D. (1994). Validation of esophageal pressure occlusion test after paralysis. Pediatr. Pulmonol. 17 (1), 56–62. doi:10.1002/ppul.1950170110
Lanteri, C. J., Petak, F., Gurrin, L., and Sly, P. D. (1999). Influence of inertance on respiratory mechanics measurements in mechanically ventilated puppies. Pediatr. Pulmonol. 28 (2), 130–138. doi:10.1002/(sici)1099-0496(199908)28:2<130::aid-ppul9>3.0.co;2-x
Lees, M. H., Way, R. C., and Ross, B. B. (1967). Ventilation and respiratory gas transfer of infants with increased pulmonary blood flow. Pediatrics 40 (2), 259–271. doi:10.1542/peds.40.2.259
Maagaard, M., Eckerstrom, F., Boutrup, N., and Hjortdal, V. E. (2020). Functional capacity past age 40 in patients with congenital ventricular septal defects. J. Am. Heart Assoc. 9 (19), e015956. doi:10.1161/JAHA.120.015956
Menon, G., and Poskitt, E. M. (1985). Why does congenital heart disease cause failure to thrive? Arch. Dis. Child. 60 (12), 1134–1139. doi:10.1136/adc.60.12.1134
Mitchell, S. C., Korones, S. B., and Berendes, H. W. (1971). Congenital heart disease in 56,109 births. Incidence and natural history. Circulation 43 (3), 323–332. doi:10.1161/01.cir.43.3.323
Morris, C. J., Kameny, R. J., Boehme, J., Gong, W., He, Y., Zhu, T., et al. (2018). KLF2-mediated disruption of PPAR-gamma signaling in lymphatic endothelial cells exposed to chronically increased pulmonary lymph flow. Am. J. Physiol. Heart Circ. Physiol. 315 (1), H173–H81. doi:10.1152/ajpheart.00635.2017
Mosing, M., Kutter, A. P., Iff, S., Raszplewicz, J., Mauch, J., Bohm, S. H., et al. (2015). The effects of cardiac output and pulmonary arterial hypertension on volumetric capnography derived-variables during normoxia and hypoxia. J. Clin. Monit. Comput. 29 (1), 187–196. doi:10.1007/s10877-014-9588-0
Motta-Ribeiro, G. C., Vidal Melo, M. F., and Jandre, F. C. (2020). A simplified 4-parameter model of volumetric capnograms improves calculations of airway dead space and slope of Phase III. J. Clin. Monit. Comput. 34 (6), 1265–1274. doi:10.1007/s10877-019-00451-4
Naulty, C. M., Horn, S., Conry, J., and Avery, G. B. (1978). Improved lung compliance after ligation of patent ductus arteriosus in hyaline membrane disease. J. Pediatr. 93, 682–684. doi:10.1016/s0022-3476(78)80917-2
Oishi, P. E., Sharma, S., Datar, S. A., Kumar, S., Aggarwal, S., Lu, Q., et al. (2013). Rosiglitazone preserves pulmonary vascular function in lambs with increased pulmonary blood flow. Pediatr. Res. 73 (1), 54–61. doi:10.1038/pr.2012.149
Oishi, P. E., Wiseman, D. A., Sharma, S., Kumar, S., Hou, Y., Datar, S. A., et al. (2008). Progressive dysfunction of nitric oxide synthase in a lamb model of chronically increased pulmonary blood flow: A role for oxidative stress. Am. J. Physiol. Lung Cell. Mol. Physiol. 295 (5), L756–L766. doi:10.1152/ajplung.00146.2007
Reddy, V. M., Meyrick, B., Wong, J., Khoor, A., Liddicoat, J. R., Hanley, F. L., et al. (1995). In utero placement of aortopulmonary shunts. A model of postnatal pulmonary hypertension with increased pulmonary blood flow in lambs. Circulation 92 (3), 606–613. doi:10.1161/01.cir.92.3.606
Rex, C. E., Eckerstrom, F., Heiberg, J., Maagaard, M., Rubak, S., Redington, A., et al. (2019). Surgical closure of a ventricular septal defect in early childhood leads to altered pulmonary function in adulthood: A long-term follow-up. Int. J. Cardiol. 274, 100–105. doi:10.1016/j.ijcard.2018.06.109
Sandberg, K. L., Poole, S. D., and Sundell, H. W. (2013). Cardio-respiratory response to moderate chloral hydrate sedation in young lambs. Acta Paediatr. 102 (4), 391–396. doi:10.1111/apa.12151
Stahl, W. R. (1967). Scaling of respiratory variables in mammals. J. Appl. Physiol. 22 (3), 453–460. doi:10.1152/jappl.1967.22.3.453
Steinhorn, R. H., Russell, J. A., Lakshminrusimha, S., Gugino, S. F., Black, S. M., and Fineman, J. R. (2001). Altered endothelium-dependent relaxations in lambs with high pulmonary blood flow and pulmonary hypertension. Am. J. Physiol. Heart Circ. Physiol. 280 (1), H311–H317. doi:10.1152/ajpheart.2001.280.1.H311
Sulc, J., Samanek, M., Zapletal, A., Voriskova, M., Hucin, B., and Skovranek, J. (1996). Lung function in VSD patients after corrective heart surgery. Pediatr. Cardiol. 17 (1), 1–6. doi:10.1007/BF02505804
Sullivan, M., Paliotta, J., and Saklad, M. (1976). Endotracheal tube as a factor in measurement of respiratory mechanics. J. Appl. Physiol. 41 (4), 590–592. doi:10.1152/jappl.1976.41.4.590
Sun, Y., Shen, S. E., Deng, X. M., Cai, Y., and Du, Y. (2020). Lung protective ventilation in infants undergoing cardiopulmonary bypass surgery for congenital heart disease: A prospective randomized controlled trial. Paediatr. Anaesth. 30 (7), 814–822. doi:10.1111/pan.13894
Turner, M. J., MacLeod, I. M., and Rothberg, A. D. (1991). Effect of airway inertance on linear regression estimates of resistance and compliance in mechanically ventilated infants: A computer model study. Pediatr. Pulmonol. 11 (2), 147–152. doi:10.1002/ppul.1950110212
Tusman, G., Sipmann, F. S., Borges, J. B., Hedenstierna, G., and Bohm, S. H. (2011). Validation of Bohr dead space measured by volumetric capnography. Intensive Care Med. 37 (5), 870–874. doi:10.1007/s00134-011-2164-x
Wallgren, G., Geubelle, F., and Koch, G. (1960). Studies of the mechanics of breathing in children with congenital heart lesions. Acta Paediatr. Stockh. 49, 415–425. doi:10.1111/j.1651-2227.1960.tb07754.x
Yau, K. I., Fang, L. J., and Wu, M. H. (1996). Lung mechanics in infants with left-to-right shunt congenital heart disease. Pediatr. Pulmonol. 21 (1), 42–47. doi:10.1002/(SICI)1099-0496(199601)21:1<42::AID-PPUL7>3.0.CO;2-S
Keywords: congenital heart disease, left-to-right shunt, respiratory mechanics, gas exchange, surgical large animal model
Citation: Soares JHN, Raff GW, Fineman JR and Datar SA (2023) Respiratory mechanics and gas exchange in an ovine model of congenital heart disease with increased pulmonary blood flow and pressure. Front. Physiol. 14:1188824. doi: 10.3389/fphys.2023.1188824
Received: 17 March 2023; Accepted: 30 May 2023;
Published: 09 June 2023.
Edited by:
Christina Maria Pabelick, Mayo Clinic, United StatesReviewed by:
Elizabeth R. Vogel, Mayo Clinic, United StatesGiuseppe Andrea Miserocchi, University of Milano-Bicocca, Italy
Copyright © 2023 Soares, Raff, Fineman and Datar. This is an open-access article distributed under the terms of the Creative Commons Attribution License (CC BY). The use, distribution or reproduction in other forums is permitted, provided the original author(s) and the copyright owner(s) are credited and that the original publication in this journal is cited, in accordance with accepted academic practice. No use, distribution or reproduction is permitted which does not comply with these terms.
*Correspondence: Joao Henrique N. Soares, amhzb2FyZXNAdWNkYXZpcy5lZHU=; Sanjeev A. Datar, c2FuamVldi5kYXRhckB1Y3NmLmVkdQ==