- 1Lambe Institute for Translational Research, Discipline of Pathology, School of Medicine, University of Galway, Galway, Ireland
- 2Houston Methodist Research Institute, Houston, TX, United States
- 3Dr Mary and Ron Neal Cancer Center, Houston Methodist Hospital, Houston, TX, United States
The human epidermal growth factor receptor (HER) family consists of four members, activated by two families of ligands. They are known for mediating cell–cell interactions in organogenesis, and their deregulation has been associated with various cancers, including breast and esophageal cancers. In particular, aberrant epidermal growth factor receptor (EGFR) and HER2 signaling drive disease progression and result in poorer patient outcomes. Nitric oxide (NO) has been proposed as an alternative activator of the HER family and may play a role in this aberrant activation due to its ability to induce s-nitrosation and phosphorylation of the EGFR. This review discusses the potential impact of NO on HER family activation and downstream signaling, along with its role in the efficacy of therapeutics targeting the family.
1 Introduction
Nitric oxide (NO) is a gaseous signaling molecule with a short half-life. In 1987, it was discovered to be the molecule responsible for the ability of endothelium-derived relaxing factor to induce the relaxation of vascular smooth muscle (Ignarro et al., 1987). NO has also been associated with the regulation of other biological systems, including the cardiovascular, nervous, and immune systems (Ignarro, 2000). NO is generated physiologically from oxygen, NADPH, and L-arginine by a family of three nitric oxide synthases: neuronal (nNOS/NOS1), inducible (iNOS/NOS2), and endothelial (eNOS/NOS3). NO synthesis requires the binding of NOS and calmodulin. High levels of intracellular calcium are needed to facilitate the binding of nNOS and eNOS to calmodulin (Nathan and Xie, 1994). This results in the generation of nanomolar levels of NO over a short period of time (seconds/minutes) (Michel and Feron, 1997). However, iNOS has a high affinity for calmodulin, so it does not require high calcium levels, allowing it to produce micromolar NO levels over long periods of time (hours/days). nNOS is predominantly expressed in neurons, where it plays a role in synaptic plasticity and the regulation of blood pressure. The expression of iNOS can be induced in various cell types, following an interaction with stimuli such as lipopolysaccharide or cytokines. The large amounts of NO generated by iNOS have protective effects against pathogens. eNOS is expressed in endothelial cells, where it functions to regulate vasodilation and blood pressure (Förstermann and Sessa, 2012).
NO generated enzymatically by NOS can be rapidly inactivated through conversion to inorganic nitrate (NO3) and nitrite (NO2−). However, NO can also be produced from dietary nitrate and nitrite. Bacteria in the mouth reduce nitrate to nitrite, which travels to the stomach. In the stomach, nitrite undergoes non-enzymatic disproportionation (Lundberg and Weitzberg, 2013). There are various other methods, both enzymatic and non-enzymatic, that convert nitrate to NO, all of which occur at an accelerated rate in acidic and hypoxic conditions, where NOS enzymes may be inactive. NO generated by this pathway is also involved in NO signaling (Lundberg and Weitzberg, 2022).
NO is a key component of redox signaling within biological systems. Its unpaired electron allows it to act as both a reductant and a weak oxidant (Kanner et al., 1991). NO is not stored but simply diffuses to its active site and covalently binds to its targets. These targets include the ions of transition metals such as iron. NO’s interaction with heme facilitates its interactions with hemoglobin and soluble guanylate cyclase (sGC) (Ignarro, 1989). sGC activation by NO mediates NO’s effects on vasodilation and blood pressure and is considered to be classical NO signaling. An alternative signaling mechanism of NO is via s-nitrosation. NO and the related nitrosonium ion (NO+) also react with proteins to form S-nitrosothiols (R-SNO), which, as discussed later in this review, allows NO to regulate protein signaling via s-nitrosation.
NO has been found to play a role in various cancers, such as breast, prostate, lung, pancreas, and colon cancers (Fujimoto et al., 1997; Reveneau et al., 1999; Hundley and Rigas, 2006; Stewart et al., 2009; Wang et al., 2016). In cancer, NO can regulate various key components, such as tumor growth, metastasis, and angiogenesis. The effects of NO in cancer are dichotomous and based on the concentration, duration of exposure, NOS isoform, tumor microenvironment, and type of cancer (Burke et al., 2013; Kamm et al., 2019). Low NO concentrations are associated with metastasis and drug resistance, whereas high NO concentrations are linked to increased apoptosis (Vannini et al., 2015).
The human epidermal growth factor receptor (HER) family’s discovery began in the 1960s, when epidermal growth factor (EGF) was first discovered (Cohen, 1965). It was not until the 1980s that the corresponding receptor, epidermal growth factor receptor (EGFR), was successfully cloned and found to be amplified in A431 epidermoid carcinoma cells (Ullrich et al., 1984). It was subsequently discovered that the avian erythroblastosis tumor virus encoded an aberrant variant of EGFR (HER1) (Downward et al., 1984a). This led to the investigation into the oncogenic role of EGFR. Following this, a gene similar to EGFR, which is known as the human epidermal growth factor receptor 2 (HER2) gene, was found to be amplified in a human breast cancer cell line (King et al., 1985). HER2 (human) and neu (rodent) are homologs of a growth factor receptor that were discovered independently and found to be oncogenic. Neu was initially found to be homologous to v-erbB (avian erythroblastosis virus), a viral oncogene, and the EGFR (Schechter et al., 1984; 1985). It was later discovered that HER2 also had tyrosine kinase activity (Akiyama et al., 1986). HER2 gene amplification by 2–50 fold was found in ∼30% of breast tumors and was found to be a significant predictor of both overall survival and time to relapse (Slamon et al., 1987). HER3 and HER4 were discovered in the early 1990s (Plowman et al., 1990; 1993). This led to the completion of the HER family as we know it today, consisting of EGFR (HER1, erbB1), HER2 (erbB2, HER2/neu), HER3 (erbB3), and HER4 (erbB4).
HER family members are expressed throughout the body in non-hematopoietic cells. The predominant physiological role of the HER family is in the mediation of cell–cell interactions both during organogenesis and adulthood (Burden and Yarden, 1997). They play a key role in the development of several organ systems, such as the nervous system, heart, skin, lungs, and gastrointestinal tract (Lee et al., 1995; Miettinen et al., 1995). The family has also been shown to play a role in the development of the mammary gland during puberty (Xie et al., 1997; Andrechek et al., 2005). The HER family, particularly EGFR and HER2, has also been implicated in various cancers. Tumors with dysregulated EGFR or HER2 are linked to more aggressive disease and poor clinical outcomes (Slamon et al., 1987; Nicholson et al., 2001).
Classically regulated by ligand binding and dimerization, in this review, we present an alternative form of HER receptor family activation via NO. HER family activation by NO may lead to enhanced tumor HER receptor signaling, with clinical consequences for patient prognosis and therapeutic outcomes. In particular, if NO and HER receptors interact to drive tumor progression, this may represent an opportunity for combination targeting for the treatment of cancer. This review describes the role of the HER receptor family in cancer progression and introduces how these receptors and their downstream signaling pathways are impacted by NO signaling. Understanding the interplay between these diverse processes is key for the future design of dual combinations of HER- and NO-targeting therapeutics.
2 HER family receptor structure and activation
HER receptors are type I transmembrane growth factor receptors. They respond to extracellular stimuli by activating intracellular signaling pathways. Structurally, they consist of four domains: an extracellular N-terminal containing two cysteine-rich regions, a transmembrane domain, an intracytoplasmic domain, and a C-terminal tail (Carpenter, 1987).
The HER family is activated by two families of ligands: EGF-related ligands and neuregulins. All of these ligands share an EGF-like domain and three disulfide-bonded loops. The receptor-binding domain tends to be part of a large precursor that undergoes a highly regulated cleavage to release the ligand (Prenzel et al., 1999). Different ligands have differential specificities for each of the family members, as summarized in Table 1. The EGFR is predominantly activated by the EGF, whereas HER3/HER4 is activated by neuregulins. Despite HER2 not having a ligand-binding domain, it is frequently hyperactivated.
Upon extracellular binding with their given ligand, the extracellular domain undergoes a conformational change from a closed inhibited state to an open active state that promotes dimerization (Figure 1) (Lemmon et al., 1997). Dimer partner selection is an important factor in determining the downstream signaling pathways activated by receptor activation. There is a hierarchy of interactions between the transmembrane domains of the four receptors, ranging from non-interactive pairs to strong dimerization. The preferred dimer partner was found to be HER2, with HER3 ranking next ahead of EGFR (Duneau et al., 2007). HER2 has the strongest kinase activity; therefore, dimers containing HER2 have stronger downstream signaling (Graus-Porta et al., 1997). The HER3–HER4 heterodimer is the least favored thermodynamically and could not be induced by ligand binding (Tzahar et al., 1996). Despite this, HER4 signaling has been documented in breast and nervous system development (Tidcombe et al., 2003).
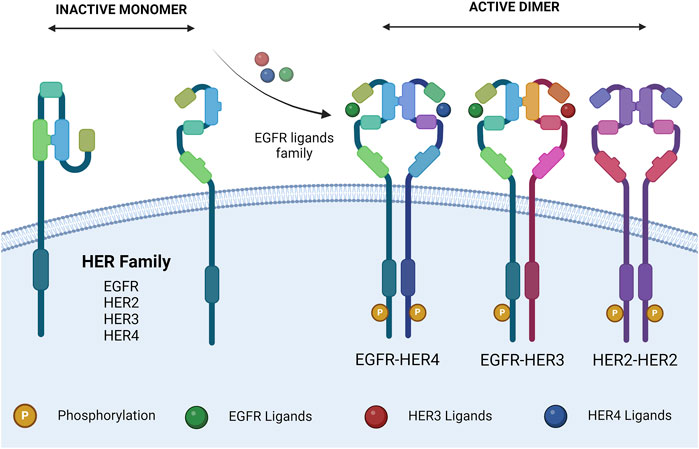
Figure 1. HER family dimerization. HER family ligand interactions, and subsequent dimerization. Ligands are described in Table 1. The dimers shown are representative. Created with BioRender.com.
HER2 exists in a constitutively active state (Garrett et al., 2003) and lacks the ability to bind a ligand; therefore, the ability of ligand binding to induce HER2 signaling is dependent on a heterodimeric partnership (Sliwkowski, 2003). On the other hand, HER3 is missing an ATP-binding site within its catalytic domain, preventing its kinase activity (Sierke et al., 1997), meaning that HER3’s downstream signaling is also dependent on a heterodimeric partnership (Kim et al., 1998). Despite being incomplete individually, the HER2–HER3 heterodimer is the most active of the family and is essential for various processes.
The ligand(s) involved in receptor activation can also play a role in dimer selection. In dimers where two ligands can be present (e.g., EGFR–HER3), they are more stabilized by neuregulin than the EGF (Tzahar et al., 1996). Dimer formation allows for the activation of the kinase domain and trans-auto-phosphorylation of the intracellular domain. Each member of the HER family has different C-terminal sites, which becomes trans-auto-phosphorylated upon dimer formation. This allows for the subsequent docking of signaling molecules, triggering downstream signaling and the various biological effects associated with HER family signaling (Olayioye et al., 2000).
There are two pathways involved in the post-activation processing of the HER family. The EGFR undergoes endocytic degradation, whereas HER2, HER3, and HER4 undergo endocytic recycling (Baulida et al., 1996). When the EGFR dimerizes with HER2, such as in HER2 overexpression, it is redirected down the endocytic recycling pathway. This results in increased levels of EGFR on the cell membrane, along with longer and more potent signaling activity (Lenferink et al., 1998; Waterman et al., 1998; Hendriks et al., 2003). Overall, HER2 is the least frequently inactivated member of the HER family. HER2-containing dimers can trigger downstream signaling for prolonged periods by evading signal attenuation. This leads to increased MAPK and c-Jun activation in HER2-overexpressing cells, following treatment with EGFR or HER3 ligands (Karunagaran et al., 1996).
3 Interaction of NO with HER family signaling
HER family signaling varies in complexity from organism to organism. In C. elegans, signaling is driven by a single ligand and receptor; in Drosophila, this increases to four ligands and one receptor (Lacenere and Sternberg, 2000), whereas in mammals, there are at least 12 ligands and 4 receptors. The extent of the HER family in mammals compared to other animals is believed to result from functional differentiation, requiring all members of the family to interact in their various heterodimers to carry out different functions downstream. The fact that HER2 and HER3 are functionally incomplete facilitates this concept.
The specific tyrosine phosphorylation residues of each member of the HER family control the binding ability of downstream signaling molecules. Some molecules are common among multiple members of the family, such as Grb2 and Shc, whereas others are more specific, like Cbl, which only binds to the EGFR (Levkowitz et al., 1996). Therefore, each of the 10 HER family dimers exhibits differences in the downstream signaling pathways they activate (Figure 2).
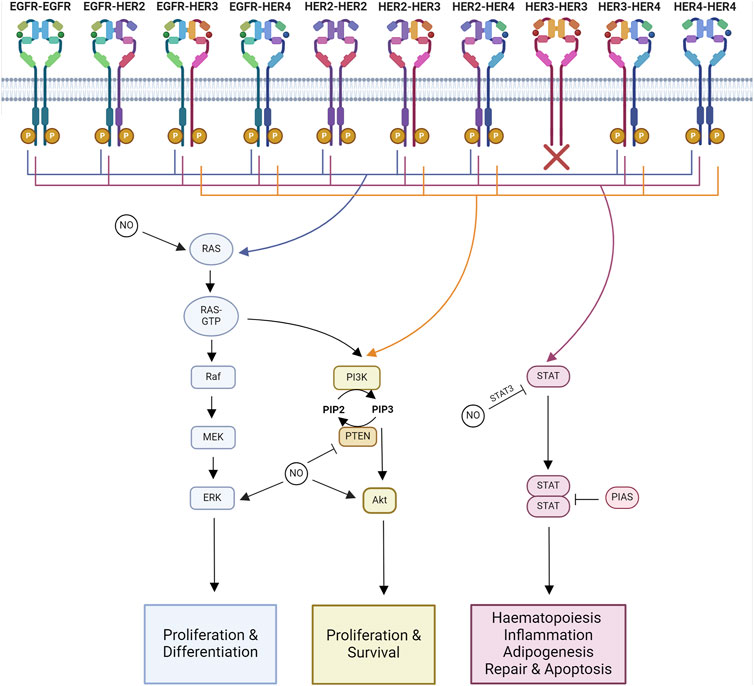
Figure 2. Major signaling pathways impacted by NO-HER family interactions. Major downstream signaling pathways activated by each HER family dimer pair and known interactions of NO. Ras-MAPK signaling regulates proliferation and differentiation. PI3K-Akt signaling regulates proliferation and survival. STAT signaling regulates hematopoiesis, inflammation, adipogenesis, repair, and apoptosis. Created with BioRender.com.
Homodimers tend to be less able to stimulate proliferation compared to heterodimers. Within the heterodimers, those containing HER2 are the most active, while those containing HER3–HER4 are the least active, linking into the hierarchal process of dimer formation. HER2’s highly active signaling is due to its ability to decrease the rate of NRG and EGF dissociation from their receptors alongside HER2’s slow endocytosis rate, leading to the amplification and increased duration of signaling (Graus-Porta et al., 1995; Karunagaran et al., 1996). HER2 overexpression alters the profile of HER family dimers by increasing the formation of HER2–EGFR and HER2–HER3 heterodimers (Karunagaran et al., 1996; Hendriks et al., 2003).
The functional results of HER family signaling are varied and include migration, mitosis, adhesion, differentiation, and apoptosis. The end result is dependent on the cell type and the ligand and receptor combinations (Yarden and Sliwkowski, 2001). The core pathways downstream of HER family activation are Ras-MAPK, PI3K-Akt, and JAK-STAT signaling.
3.1 Ras-MAPK
The Ras-MAPK pathway controls proliferation and differentiation (Iwakura and Nawa, 2013). MAPK signaling activated through Ras and Shc is a downstream target of all HER family dimers (Yarden and Sliwkowski, 2001).
Activated HER family dimers, once phosphorylated at an appropriate residue, become associated with Shc and Grb2. Grb2 and Shc both have phospho-tyrosine-binding sites in all members of the HER family, as outlined above. Grb2 or Shc binding then recruits SOS, a Ras-guanine nucleotide exchange factor, leading to the activation of Ras and setting off the kinase cascade that activates Raf, MEK, and ERK (Iwakura and Nawa, 2013). ERK1/2 further interacts with various molecules that promote cell division (Yamamoto et al., 2006).
In addition to direct NO action on HER receptors, Ras s-nitrosation modulates the effects of NO on the Raf/MEK/ERK and PI3K/Akt pathways (Pervin et al., 2007). Ras is aberrantly activated in breast cancers overexpressing EGFR or HER2 (von Lintig et al., 2000). S-nitrosation of Ras has been linked to metastasis through MAPK-dependent Ets-1 activation (Marshall and Foster, 2012). NO also phosphorylates ERK1/2 and increases cell migration in an EGFR-ERK1/2-dependent manner in triple-negative breast cancer (Garrido et al., 2017). NO induces tumor growth through MEK1/2 and ERK1/2 phosphorylation, potentially due to a combination of HER receptor and Ras modifications (Rice et al., 2010; Sen et al., 2013; Chen et al., 2018). In colon cancer, NO’s phosphorylation of ERK1/2 also leads to the upregulation of MMP-2 and MMP-9 expression (Babykutty et al., 2012).
3.2 PI3K-Akt
The PI3K-Akt signaling pathway regulates cell growth and anti-apoptotic signaling (Iwakura and Nawa, 2013). The PI3K pathway is activated downstream of most HER dimer pairs. However, the extent and kinetics of the activation are varied. This is due to the ability of PI3K to directly interact with HER3 and HER4, whereas it can only interact indirectly with EGFR and HER2 (Soltoff and Cantley, 1996).
HER3 plays a major role in the activation of pro-survival signaling through PI3K due to its six binding sites for p85, PI3K’s regulatory subunit, whereas HER4 only has one p85-binding site.
Aberrant NOS expression is linked to the ability of oncogenic PI3K/Akt signaling to induce inflammation and immunosuppression (Villegas et al., 2018). In breast cancer, Akt phosphorylation and iNOS expression are strongly correlated (Smeda et al., 2018). NO is capable of inducing Akt phosphorylation and tumor growth (Ridnour et al., 2012; Sen et al., 2013). The threshold for Akt phosphorylation is 100 nM of NO (Thomas et al., 2008). NO also protects against H2O2-induced cell death neuroblastoma through Akt phosphorylation (Yoo et al., 2018). In ovarian cancer, GSNO was found to induce the s-nitrosation of EGFR and Akt but decrease Akt phosphorylation (Giri et al., 2014). In gastric cancer, NO has an anti-proliferative effect and also inhibits Akt phosphorylation (Sang et al., 2011). In ER-negative breast cancer with high levels of iNOS, Akt phosphorylation at S473 is present in 87%–89% of tumors, whereas abundant phosphorylation of Akt at T308 is linked with co-expression of iNOS and COX2. Downstream of Akt, phosphorylated forms of BAD and caspase 9 were also more abundant in tumors co-expressing iNOS and COX2, showing an association with activation of the Akt signaling pathway. Additionally, Ras-MAPK and/or PI3K-Akt signaling are required for NO to induce COX2 expression (Basudhar et al., 2017).
PTEN, the negative regulator of PI3K signaling, is also highly regulated by NO. S-nitrosation of PTEN at C83 results in the inhibition of its enzymatic activity and the induction of its degradation via NEDD4-1-mediated ubiquitination (Kwak et al., 2010; Numajiri et al., 2011; Ohno et al., 2015). S-nitrosation of PTEN has been shown to occur via trans-nitrosation in the brain, with s-nitrosated DJ-1 donating its NO group to PTEN (Choi et al., 2014). nNOS activity has been shown to induce s-nitrosation of PTEN, resulting in the activation of Akt/mTOR signaling and inhibition of autophagy in nasopharyngeal carcinoma (Zhu et al., 2019). S-sulfhydration of PTEN at C71 and C124 occurs endogenously and prevents s-nitrosation of PTEN (Ohno et al., 2015). Therefore, s-sulfhydration of PTEN allows it to inhibit PI3K signaling, whereas s-nitrosation of PTEN allows for increased PI3K signaling. iNOS expression and the resulting increase in PI3K signaling have been associated with poor clinical outcomes in a subset of melanoma patients expressing PTEN (Ding et al., 2021).
3.3 JAK-STAT
JAK/STAT signaling is involved in hematopoiesis, tissue repair, inflammation, apoptosis, and adipogenesis (Bach et al., 1996; Stephens et al., 1996; Fulda and Debatin, 2002; Owen et al., 2019; Jaiswal et al., 2023). JAKs are known to be recruited to activated EGFR, where they become transphosphorylated (Iwakura and Nawa, 2013). Phosphorylated JAK is active and further phosphorylates the receptor, forming a STAT docking site. When STAT binds, it is phosphorylated by JAK, triggering the disassociation of active STAT. Active STAT then forms dimers capable of translocation to the nucleus, where they regulate gene transcription (Bharadwaj et al., 2020).
EGFR activation leads to STAT1 activation and the formation of STAT1 and STAT3 complexes with JAK1 and JAK2 (Andl et al., 2004). JAK2’s phosphorylation of the EGFR has been linked to the activation of Ras-MAPK signaling (Wong et al., 1992; Yamauchi et al., 1997). The EGFR can interact with all STATs, with the exception of STAT6 (Erdogan et al., 2022). In breast cancer, HER2 overexpression has been linked to STAT3 expression and a HER2-STAT3 signaling network (Diaz et al., 2006; Duru et al., 2012). NRG-1, the ligand for HER3 and HER4, can activate JAK/STAT signaling through JAK3, STAT3, and STAT5 in an HER2–HER3-dependent manner. This has been linked to an induction of proliferation (Liu and Kern, 2002). HER4 becomes truncated by γ-secretase, forming a soluble intracellular domain with signaling activity (Ni et al., 2001). This truncated HER4 acts as a chaperone for the translocation of STAT5A to the nucleus (Long et al., 2003).
Both STAT3 and its repressor, Pias3, have been reported to undergo s-nitrosation. S-nitrosation of STAT3 results in a decrease in its phosphorylation at Y705 (Giri et al., 2014). S-nitrosation of Pias3 promoted its degradation (Qu et al., 2007). High concentrations of NO have been reported to induce apoptosis in ovarian cancer. This has been linked to a decrease in STAT3 and Akt phosphorylation (Kielbik et al., 2013). The reduction in phosphorylation may be due to the reported inverse relationship between s-nitrosation and phosphorylation in STAT3 and Akt (Giri et al., 2014).
4 HER family post-translational modifications
The HER family undergoes extensive post-translational modifications (PTMs) (Figure 3). The PTM of proteins involves the addition of functional groups, cleavage, or degradation of translated proteins by various enzymes. This review will focus on the addition of functional groups. S-nitrosation is a PTM mediated by NO. Other common PTMs include phosphorylation, glycosylation, acetylation, methylation, and ubiquitination, all of which can be regulated by NO signaling. PTMs can be classified by the amino acids they modify, the type of enzyme, and the reversibility of the modification (Walsh et al., 2005). The role of PTMs is to regulate protein function. This can be done allosterically or through the creation of binding sites to facilitate protein–protein interactions (Seet et al., 2006).
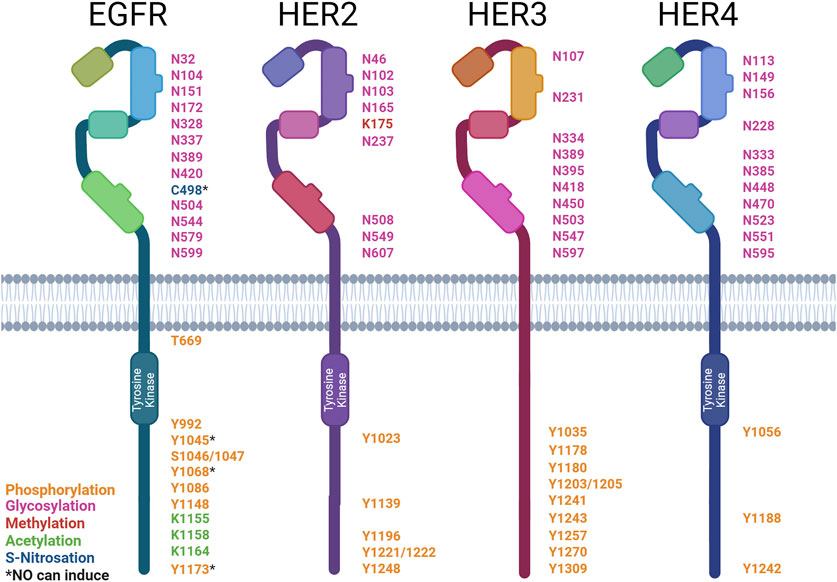
Figure 3. Post-translational modification sites of HER family receptors. HER family receptor structures indicating the sites of post-translational modification (PTM): asparagine (N), cysteine (C), lysine (K), serine (S), tyrosine (Y), and threonine (T). The types of modification are color-coded: phosphorylation (orange), glycosylation (pink), methylation (red), acetylation (green), and S-nitrosation (blue). * denotes PTMs that can be induced by NO. Created with BioRender.com.
4.1 Phosphorylation
The phosphorylation of proteins is the most common PTM and plays a role in the regulation of almost all cellular processes. Kinases are the enzymes that mediate the addition of a phosphate group to a protein; they catalyze the transfer of γ-phosphate from ATP to an amino acid. The most commonly phosphorylated amino acids are serine, threonine, and tyrosine. On the other hand, phosphatases remove phosphate groups from proteins, further allowing for the regulation of cellular functions (Ubersax and Ferrell Jr, 2007).
The HER family functions as kinases and undergoes trans-phosphorylation in dimer pairs, as previously discussed. Each member of the HER family has different C-terminal tyrosine sites that become trans-auto-phosphorylated. These sites act as docking sites for SH2 and PTB domains on various enzymes and adapter proteins involved in downstream signaling. Protein microarrays have been used to screen all SH2 and PTB domains within the human genome for interactions with the HER family (Jones et al., 2006).
EGFR’s activation leads to the autophosphorylation of six tyrosine residues (Downward et al., 1984b; Hsuan et al., 1989; Margolis et al., 1989; Walton et al., 1990). EGFR’s phospho-tyrosines then interact with various downstream signaling molecules (∼7.2 proteins per site) (Jones et al., 2006). Some of these downstream signaling molecules are Shc (Y1173 and Y1148), PLCγ (Y1173 and Y992), SHP1 (Y1173), Grb2 (Y1068 and Y1086), and Cbl (Y1045) (Rotin et al., 1992; Batzer et al., 1994; Okabayashi et al., 1994; Okutani et al., 1994; Keilhack et al., 1998; Sakaguchi et al., 1998; Chattopadhyay et al., 1999; Levkowitz et al., 1999). The EGFR phosphorylates PLCγ at Y771 and Y1254 (Wahl et al., 1990). This increases the phospholipase activity required for EGF-associated cell motility (Xie et al., 1998). Cbl docking facilitates the ubiquitination and subsequent degradation of the EGFR (Waterman et al., 2002). Shc and Grb2 interactions allow for the activation of MAPK/ERK signaling (Rojas et al., 1996; Sakaguchi et al., 1998). NO signaling via the EGFR has been well-documented in breast cancer, with high levels of iNOS expression correlated with EGFR Y1173 phosphorylation in ER-negative patient samples. NO has also been shown to rapidly induce EGFR Y1045, Y1068, and Y1173 phosphorylation in triple-negative breast cancer cells (Glynn et al., 2010; Garrido et al., 2017). EGFR phosphorylation at Y1173 by NO has also been documented in lung cancer (Lee et al., 2008). In contrast, NO has also been shown to inhibit the phosphorylation of the EGFR by the EGF, causing a downstream reduction in ERK phosphorylation in gastric cancer cells through the activation of type II cGMP-dependent protein kinase (PKG II), demonstrating both pro- and anti-stimulatory effects on the EGFR (YAO et al., 2015).
HER2 activation leads to the autophosphorylation of five tyrosine residues (Hazan et al., 1990; Segatto et al., 1990). These phospho-tyrosines then interact with various downstream signaling molecules (∼17 proteins per site) (Jones et al., 2006). Similarly to the EGFR, HER2’s phospho-tyrosines interact with Shc’s PTB domain (Y1196 and Y1248), Shc’s SH2 domain (Y1248 and Y1221/2), and Grb2 (Y1139) (Ricci et al., 1995). HER2 also interacts with Chk (Y1248) (Zrihan-Licht et al., 1998). The HER2-pY1112 site was found to specifically regulate HER2 ubiquitination, the HER2-pY1196 site is involved in the regulation of cell motility, and the HER2 pY1248 site regulates both migration and proliferation (Li et al., 2016).
HER3 activation leads to the autophosphorylation of nine tyrosine residues These phospho-tyrosines then interact with various downstream signaling molecules (∼8.8 proteins per site) (Jones et al., 2006). These phospho-tyrosines then interact with various downstream signaling molecules, such as Shc (Y1309), PI3K’s p85 subunit (Y1035, Y1178, Y1203, Y1241, Y1257, and Y1270), and Grb7 (Y1180) (Prigent and Gullick, 1994; Fiddes et al., 1998). HER3 differs from EGFR and HER2 in the absence of a Grb2- and PLCγ-binding site and the presence of PI3K-binding sites (Prigent and Gullick, 1994; Songyang et al., 1995).
HER4 activation leads to autophosphorylation of three tyrosine residues. These phospho-tyrosines then interact with various downstream signaling molecules (∼2.3 proteins per site) (Jones et al., 2006). These phospho-tyrosines then interact with various downstream signaling molecules, such as Shc (Y1242 and Y1188) and PI3K’s p85 subunit (Y1056) (Cohen et al., 1996; Elenius et al., 1999).
Little is known about the impact of NO on the activation status of HER2, HER3, or HER4. Given NO’s ability to regulate the EGFR and other tyrosine kinase inhibitors, there is potential for NO to also regulate the other members of this receptor family in a similar fashion. Indeed, disruption in the equilibrium of tyrosine phosphorylation has been linked to many disease states, including cancer (Hunter, 2009). Aberrant phosphorylation of the HER family occurs in various cancers, including breast, lung, and brain cancers (Slamon et al., 1987; Moscatello et al., 1995).
Phosphatases form the other piece of the puzzle that regulates the equilibrium of phosphorylation. They act by removing the phosphate groups added by kinases. Therefore, they act as antagonists to kinase receptor signaling and play a tumor-suppressive role (Li et al., 1997; Li and Sun, 1997). Two PEST-containing phosphatases, PTPN12 and BDP1 (PTPN18), have been identified as potent negative regulators of HER2 signaling. BDP1 dephosphorylates HER2 at pY1112, pY1196, and pY1248. On the contrary, PTPN12 acts on EGFR pY1148 and HER2 pY1112, pY1196, pY1221/1222, and pY1248 (Li et al., 2016). PTPN12 acts as a potent suppressor of proliferation, transformation, and metastasis through the inhibition of EGFR/HER2 signaling in mammary epithelial cells (Sun et al., 2011). NO has been shown to increase PTPN12 activity via cGMP signaling; therefore, this is a possible mechanism for NO to downregulate EGFR/HER2 signaling. This also demonstrates a precedent for NO to regulate phosphatases (Lin et al., 2003).
The HER family is also phosphorylated at serine and threonine residues. TNFα has been shown to induce the phosphorylation of EGFR S1046/7 and T669 through the activation of the ERK and p38 MAPK pathways (Noguchi et al., 2013). The phosphorylation of S1046/7 is linked to the internalization of the EGFR, whereas phosphorylation at T669 has been shown to suppress the constitutive tyrosine phosphorylation in EGFR homo- and heterodimers (Sato et al., 2013).
4.2 S-nitrosation
S-nitrosation is the reversible addition of NO to cysteine via sulfur, forming an S-NO bond known as S-nitrosothiol (Gaston et al., 2003). S-nitrosation occurs spontaneously upon the generation of NO by the NO synthase enzymes (NOS). Once a protein becomes s-nitrosated, it can trans-nitrosate other proteins it interacts with, thus amplifying the signal (Mitchell and Marletta, 2005; Kornberg et al., 2010; Nakamura and Lipton, 2013). S-nitrosation can also be regulated by nitrosylase and denitrosylase enzymes (Kornberg et al., 2010; Anand et al., 2014). NO is known to interact with over 3,000 proteins, predominantly via s-nitrosation (Mnatsakanyan et al., 2019). S-nitrosation of cysteines can alter protein activity and, therefore, cellular signaling (Park, 1988). Alterations in protein s-nitrosation have been associated with various disease states, including cancer (Foster et al., 2009).
S-nitrosation of the EGFR at C498 has been shown to activate the receptor. Downstream of EGFR s-nitrosation, oncogenic signaling pathways, including c-Myc, Akt, STAT3, and β-catenin, are activated in breast cancer. The NO concentration threshold for EGFR activation is between 200 and 300 nM; therefore, an autoxidation product of NO such as N2O3 is considered to be responsible (Switzer et al., 2012). Given that NO can s-nitrosate the EGFR and impact its signaling, it is important that future studies also examine whether NO plays a similar role on the other HER family members.
4.3 Other PTMs
The HER family undergoes various other post-translational modifications, such as glycosylation, acetylation, methylation, and ubiquitination. However, any role of NO in the formation of these modifications has yet to be determined.
The extracellular domain of the HER family receptors undergoes extensive post-translational glycosylation, which regulates their ability to bind ligands, form dimers, and activate downstream signaling. Glycosylation has also been shown to modulate the response to anti-HER2 therapeutics. α2,6-Sialylation of HER has been associated with increased resistance to trastuzumab and increased Akt and ERK phosphorylation despite reduced HER2 phosphorylation (Liu et al., 2018). However, no direct link between NO and HER family glycosylation has been reported. NO has been found to increase N-glycan, α2,6-sialylation, and O-GlcNAcylation levels in neuroblastoma (Van de Wouwer et al., 2011). In plants, a link was also found between s-nitrosation and N-glycosylation through the co-substrate thioglucoside glucohydrolase-2 (Du et al., 2019).
Acetylation of the EGFR has been shown to affect tyrosine phosphorylation. K-deacetylase inhibition induces EGFR phosphorylation (Zhou et al., 2006; Song et al., 2011). Receptor turnover and endocytosis are also regulated (Gao et al., 2010; Goh et al., 2010). Although no acetylation sites have been reported on HER2, HER3, or HER4, their structural similarity to the EGFR makes acetylation likely. Using MusiteDeep, a deep learning framework (Wang et al., 2020), we predicted the following acetylation sites: EGFR—K229, K238, K262, K438, K807, K928, K969, K981, K984, K997, and K1283; HER2—K753 and K854; HER3—K177, K383, K602, K705, and K926; HER4—K751, K852, K1223, and K1269. NO has not yet been shown to induce EGFR acetylation. However, NO has been associated with the modulation of histone acetylation by s-nitrosation of histone deacetylases (Colussi et al., 2008).
The methylation of HER2 at K175 by SMYD3 results in increased receptor phosphorylation and formation of HER2–HER2 homodimers (Yoshioka et al., 2017). There is neither any literature report on the direct methylation of EGFR, HER3, or HER4, nor on the ability of NO to induce acetylation of the HER family. However, NO plays a role in DNA and histone methylation (Socco et al., 2017). Although no methylation sites have been reported on EGFR, HER3, or HER4, their structural similarity to HER2 makes acetylation likely. We predicted the following methylation sites: EGFR—K1047; HER3—R525, K959, R1042, and R1089; and HER4—K2 and K935 (Wang et al., 2020).
All HER family receptors undergo ubiquitination, a regulator of their degradation. Ubiquitin-specific protease 2a (USP2a) inhibits EGFR’s endocytosis and subsequent degradation, therefore increasing its stability (Liu et al., 2013). PTPN18 ubiquitinates HER2 at K48, triggering rapid proteasomal degradation. Conversely, PTPN’s de-phosphorylase activity at Y1112 inhibits the trafficking of HER2 to the lysosome, preventing degradation (Wang et al., 2014). HER3’s ubiquitination is mediated by Nrdp1, an E3 enzyme. This regulates receptor expression levels. In breast cancer, Nrdp1 expression is lost, facilitating increased HER3 expression and downstream signaling (Printsev et al., 2014). HER4 is polyubiquitinated through an interaction with the WW domains of the E3 enzyme, AIP4/Itch (Omerovic et al., 2007). NO has not been reported to affect the ubiquitination of the HER family but has been found to interact with ubiquitination machinery within the cell. NO alters ubiquitination through the inhibition of ubiquitin E1 (Kitagaki et al., 2009) and s-nitrosation of E2 and E3 enzymes (Qu et al., 2007; Bailey et al., 2018).
5 Targeting the NO-HER family axis in tumors
As all HER family dimers activate the pro-proliferative Ras-MAPK pathway, the family is frequently dysregulated in cancer. Aberrant phosphorylation of the family has been documented in various cancers, including breast, lung, and brain cancers (Slamon et al., 1987; Moscatello et al., 1995), making the family a well-exploited drug target in cancer (Table 2). The use of therapeutics targeting NO and HER family activity is discussed below, along with interactions between NO signaling and responses to HER family-targeted therapeutics.
5.1 NOS inhibitors
A variety of NOS inhibitors, with differing specificities for the three NOS isoforms, are in use by researchers in the NO field. Commonly used NOS inhibitors such as L-NMMA and L-NAME are L-arginine analogs (Figure 4). They bind to NOS’s arginine-binding site, acting as competitive antagonists of the enzyme. These inhibitors have been investigated in clinical trials for various disease states, including cancer (Table 3) (Dao et al., 2021). One such inhibitor, NG-monomethyl-L-arginine (L-NMMA), is currently being investigated in the context of triple-negative breast cancer. L-NMMA is a pan-NOS inhibitor that was well-tolerated in an international phase III placebo-controlled trial for cardiogenic shock (TRIUMPH Investigators et al., 2007). L-NMMA, in combination with taxane chemotherapy in locally advanced and metastatic triple-negative breast cancer, was explored in a phase Ib/II clinical trial (Chung et al., 2021). The phase 1 dose-finding wing of the trial recommended a dose of 20 mg/kg for L-NMMA and 100 mg/m2 for docetaxel, along with amlodipine and aspirin, to prevent hypertension and thromboembolism, respectively, for the phase II portion. L-NMMA was found to significantly reduce serum nitrates and nitrites, showing successful inhibition of NOS. The overall response was 45.8%, with immune remodeling and a decrease in iNOS expression, following treatment seen in responders. Non-responders had increased levels of circulating fibroblast growth factor (FGF-2), VEGF, IL-8, IL-12p40, IL-1a, and IL-6, which may act as a method of monitoring response. IL-6 can induce STAT3 signaling, a well-known mechanism behind metastasis and proliferation in cancer (Hemmann et al., 1996). Currently, L-NMMA, in combination with nab-paclitaxel and the PI3K inhibitor alpelisib, is being studied in metastatic metaplastic breast cancer in a phase I/II trial (Trial ID: NCT05660083) (The Methodist Hospital Research Institute, 2023). Alternatively, L-NAME, another pan-NOS inhibitor, has been shown to inhibit ERK activation in triple-negative breast cancer in vitro (Sciacca et al., 2019). Both STAT3 and ERK signaling are modulated by both NO and HER family signaling, as previously discussed.
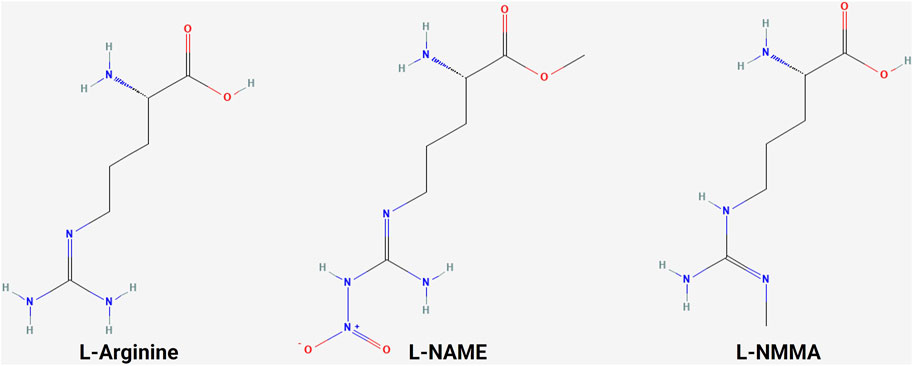
Figure 4. Structure of L-arginine and NOS inhibitors. Chemical structure of L-arginine and NOS inhibitors L-NAME and L-NMMA, showing the structural similarity between the molecules (NCBI, 2024b; NCBI, 2024a; NCBI, 2024c).
5.2 Anti-EGFR therapeutics
The overexpression and mutation of the EGFR have been associated with cancers. EGFR overexpression occurs in gliomas, NSCLCs, and pancreatic adenocarcinomas (Rusch et al., 1997; Wikstrand et al., 1998; Ueda et al., 2004). In gliomas, it is associated with a higher tumor grade and reduced survival (Wikstrand et al., 1998). An EGFR mutation resulting in the loss of its extracellular domain in EGFR type III can cause constitutive activation and has been associated with lung, ovary, and breast cancers (Moscatello et al., 1995). The EGFR has become one of the most popular cancer treatment targets. To date, there are two main drug types for cancer-targeted therapy based on high EGFR expression: tyrosine kinase inhibitors (TKIs) and EGFR monoclonal antibodies. These therapeutic agents have been most successful in the treatment of lung, head and neck, and colorectal cancers.
EGFR TKIs targeting activating EGFR mutations in NSCLC have led to a paradigm shift in the treatment of advanced NSCLC. First- and second-generation TKIs, including gefitinib, erlotinib, afatinib, and dacomitinib, have shown superior overall survival (OS) and progression-free survival (PFS) when compared to platinum-containing chemotherapy (Rosell et al., 2012; Wu et al., 2017; Yoshioka et al., 2019; Sequist et al., 2023). Third-generation osimertinib is an irreversible TKI with significantly prolonged PFS and OS when compared with earlier generations of TKIs (Ramalingam et al., 2020).
EGFR monoclonal antibodies, including cetuximab, panitumumab, nimotuzumab, and necitumumab, exert antitumor activity by competitively binding to different regions of the EGFR extracellular region and inhibiting downstream signaling pathways. In addition, the Fc region of monoclonal antibodies such as cetuximab, panitumumab, and nimotuzumab can bind to the FcR on the surface of different immune cells like natural killer cells, macrophages, and dendritic cells to mediate different innate immune responses (Mazorra et al., 2017). Cetuximab and panitumumab are the most commonly used monoclonal antibodies. When they are combined with chemotherapy, cetuximab and panitumumab improve the response rate and PFS in K-Ras wild-type metastatic colorectal cancer (Cunningham et al., 2004; Karapetis et al., 2008; Douillard et al., 2010). Cetuximab, in combination with radiotherapy, significantly improved overall survival in patients with locoregionally advanced squamous cell carcinoma of the head and neck (Bonner et al., 2010).
Both TKIs and EGFR monoclonal antibodies could interact with the NO pathway, providing potential targets and therapeutic strategies to overcome TKI and monoclonal antibody resistance. Cetuximab, in combination with chemotherapy, has been found to downregulate iNOS and NO levels in colorectal cancer (Benkhelifa et al., 2019). The conjugation of cetuximab with S-nitrosothiol enhances the tumor accumulation of the co-administered antibody (Yoshikawa et al., 2020). Gefitinib is found to act synergistically with NO to induce cell death in metastatic prostate cancer cells (Mimeault et al., 2005). NO-aspirin significantly reduced the number and size of lung tumors in vivo, which was linked to reduced levels of EGFR and Akt phosphorylation (Song et al., 2018). A novel hederagenin-NO donor has been found to inhibit proliferation and EGFR kinase activity, even in gefitinib- and osimertinib-resistant NSCLC (Chen et al., 2019).
5.3 Anti-HER2 therapeutics
Only HER2 gene amplification with resultant overexpression of the HER2 protein is needed for cellular transformation. HER2 overexpression or amplification leads to ligand-independent dimerization and abnormal downstream signaling. In both mouse fibroblasts and highly transformed tumorigenic cells (Chazin et al., 1992) and in human breast cancer cells, HER2 overexpression results in increased tumorigenicity (Benz et al., 1992). It is found in approximately 25% of breast cancers and is historically associated with aggressive disease and a poor prognosis (Slamon et al., 1989). The discovery that HER2 overexpression was associated with an extremely poor outcome in breast cancer led to the development of the monoclonal antibody trastuzumab and many other agents later, which revolutionized the outcome of patients with HER2-positive breast cancer.
Similar to EGFR-targeted therapies, anti-HER2 therapeutics also include TKIs, such as lapatinib, neratinib, pyrotinib, and tucatinib, and monoclonal antibodies, like trastuzumab and pertuzumab (Cho et al., 2003; Franklin et al., 2004; Arcila et al., 2012). Trastuzumab was the first humanized monoclonal antibody developed that achieved remarkable success. Another successfully developed monoclonal antibody is pertuzumab. Although trastuzumab binds to the extracellular domain IV of HER2, pertuzumab binds to the extracellular domain II, which prevents HER2 heterodimerization with EGFR, HER3, and HER4. Combinations of trastuzumab and pertuzumab provide complementary mechanisms of action and were proven superior to single-agent trastuzumab in neoadjuvant, adjuvant, and metastatic settings in breast cancer (Nahta et al., 2004; Gianni et al., 2012; Swain et al., 2020). The success of targeting HER2 as a therapeutic strategy was seen in other malignancies that overexpress HER2. Trastuzumab, in combination with chemotherapy, improved OS in patients with HER2-positive gastric or gastro-esophageal junction cancer and endometrial cancer (Bang et al., 2010; Fader et al., 2020).
TKIs are small molecules that target the intracellular catalytic kinase domain of HER2, competing with ATP, blocking phosphorylation and the activation of downstream signaling cascades. Because of their small molecular size, some TKIs have shown the ability of penetrating the blood–brain barrier and anti-tumor efficacy in the CNS. Lapatinib monotherapy and combination therapy demonstrated some efficacy in patients with HER2-positive breast cancer and CNS diseases (Geyer et al., 2006; Lin et al., 2009). Most recently, a newer-generation TKI tucatinib, in combination with capecitabine and trastuzumab, for the first time, demonstrated clinically meaningful benefits in patients with HER2-positive active brain metastases (Murthy et al., 2020).
Recently, the anti-HER2 therapeutics have been expanded to include antibody–drug conjugates (ADCs) such as ado-trastuzumab emtansine (T-DM1) and fam-trastuzumab deruxtecan-nxki (T-DXd). ADCs contain a tumor-targeting antibody covalently bound to a cytotoxic drug (payload) via a synthetic linker. The ADC is directed to cancer cells expressing the target on the cell surface, followed by the internalization of the ADC and release of the cytotoxic payload, resulting in tumor cell death. T-DM1 was the first anti-HER2 ADC developed that contains DM1, a maytansine derivative, as a payload with a drug-to-antibody ratio of 3.5. T-DM1 prolonged PFS and OS in patients with HER2-positive breast cancer in the metastatic setting (Verma et al., 2012) and in the adjuvant setting in patients with residual disease after neoadjuvant treatment (von Minckwitz et al., 2019). T-DXd is a newer ADC that has deruxtecan as a payload and a drug-to-payload ratio of 8. T-DXd demonstrated unprecedented improvement in PFS when compared head-to-head with T-DM1 in patients with metastatic HER2-positive breast cancer, leading to its FDA approval (Cortés et al., 2022).
In addition to HER2 gene amplification, HER2 mutation can also activate the downstream signaling pathway and drive tumorigenesis. In contrast to HER2 overexpression, HER2 mutations are identified in a wider variety of solid organ malignancies. HER2 mutations can be detected in up to 15%–19% of prostate neuroendocrine tumor and bladder cancer; 3%–6% of colorectal, gastric, and esophageal cancers; and less than 3% in breast and lung cancers (Connell and Doherty, 2017). Despite the success of monoclonal antibodies and TKIs in HER2 overexpressed cancer, they have only shown minor benefits in HER2-mutated malignancies. In contrast, ADCs, especially T-DXd, have shown encouraging results in HER2-mutated advanced lung cancer and gastroesophageal cancer that led to FDA approvals (Shitara et al., 2020; Li et al., 2022).
Despite the success in the development of anti-HER2 therapeutics, resistance inevitably happens in many patients. In addition to mutations like ΔHER2 (Siegel et al., 1999), which results in a higher level of homodimer formation and phosphorylation, altered s-nitrosation in HER2+ breast cancer through GSNOR inhibition has also been identified to induce trastuzumab resistance (Cañas et al., 2016). The interactions between gefitinib and NO discussed above are also relevant in the context of HER2 as the response to gefitinib in breast cancer was found to be independent of EGFR expression but influenced by HER2 overexpression (Campiglio et al., 2004).
5.4 Anti-HER3 therapeutics
The presence of HER3 has been documented in multiple cancers (Ciardiello et al., 1991; Rajkumar et al., 1993; 1996; Friess et al., 1995; Simpson et al., 1995; Bobrow et al., 1997; Leung et al., 1997; Yi et al., 1997; Slesak et al., 1998; Reschke et al., 2008; Ocana et al., 2013; Zhang et al., 2015) and linked to both treatment failure and drug resistance in breast, prostate, ovarian, and NSCLC (Holbro et al., 2003; Engelman et al., 2007; Mills and Yarden, 2010; Jathal et al., 2011). The upregulation of HER3 expression or signaling is associated with resistance to HER2 inhibitors in HER2-overexpressed breast cancer and to EGFR inhibitors in lung cancer; HER3 mutations have been reported as an oncogenic driver in colon and gastric cancers. These findings suggest that HER3 plays a pivotal role in the upregulation of tumor growth and drug resistance (Jacob et al., 2018). Despite the role of HER3 in mediating resistance, the inhibition of HER3 with either anti-HER3 monoclonal antibodies or in combination with anti-EGFR, anti-HER2, or chemotherapy only provided marginal clinical benefit. An increased incidence of diarrhea was also observed when anti-HER3 therapies were combined with anti-HER2 therapies (Aurisicchio et al., 2012; McDonagh et al., 2012; Huang et al., 2013).
The interactions between gefitinib and NO discussed above are also relevant in the context of HER3 as gefitinib induces the formation of inactive EGFR/HER2 and EGFR/HER3 dimers, along with inhibiting the formation of active HER2/HER3 dimers in HER2-amplified breast cancer (Anido et al., 2003).
5.5 Anti-HER4 therapeutics
HER4 has been linked to various cancers, such as breast, colorectal, lung, hepatocellular, prostate, bladder, ovarian, endometrial, and glioblastoma (Edwards et al., 2006; Memon et al., 2006; Ejskjaer et al., 2007; Sundvall et al., 2008; de Wit et al., 2013; Liu et al., 2017; Saglam et al., 2017; Zhang et al., 2017; Donoghue et al., 2018). However, the role of HER4 is less straightforward.
HER4 expression in breast cancer has been linked to improved outcomes in ER + disease due to its anti-proliferative activity (Tovey et al., 2004). HER4 expression has also been linked to improved sensitivity to trastuzumab (Portier et al., 2013). In bladder and hepatocellular carcinoma, decreased HER4 expression is linked to a poor prognosis (Memon et al., 2006; Liu et al., 2017). On the other hand, in lung cancer, specific HER4 polymorphisms are linked to a higher risk of developing the disease (Zhang et al., 2017). In glioblastoma and endometrial cancer, HER4 expression is not correlated with survival (Ejskjaer et al., 2007; Donoghue et al., 2018). Afatinib and allitinib are kinase inhibitors that act on EGFR, HER2, and HER4 (Solca et al., 2012; Zhang et al., 2014). Recently, novel imidazothiazole derivatives were found to act as specific HER4 kinase inhibitors (Zaraei et al., 2021), which might help delineate the role of HER4 in various solid tumor malignancies.
6 Conclusion
Since its discovery in the 1960s, the role of the EGFR and its sister receptors in cancer has become increasingly apparent. Aberrant EGFR and HER2 signaling is widely accepted to drive disease progression and result in poorer patient outcomes. Nitric oxide has been proposed as an alternative activator of the HER family and may play a role in this aberrant activation as high iNOS expression has been associated with outcomes in the ER-negative and triple-negative breast cancer setting. NO is a promising druggable target with widespread involvement in oncogenic signaling through the induction of EGFR phosphorylation and s-nitrosation. Due to the structural similarity between the EGFR and the rest of the receptor family, NO is also likely to induce their phosphorylation and s-nitrosation. Signaling molecules downstream of the HER family, Ras and PTEN, also undergo s-nitrosation, resulting in increased Ras and PI3K signal transduction, further demonstrating the role of NO in signaling associated with these receptors. Additionally, NO has been shown to reduce EGFR signaling and act synergistically with gefitinib, an EGFR tyrosine kinase inhibitor, in prostate and lung cancers, while altered s-nitrosation in HER2+ breast cancer is linked to trastuzumab resistance. This demonstrates a role for NO in the treatment of patients with tumors driven by HER family signaling. However, further research is needed to fully unravel the role of NO in the activation of HER family signaling and its impact on treatment outcomes as research to date has predominantly focused on the EGFR.
Author contributions
CO: conceptualization, writing–original draft, and writing–review and editing. KS: writing–original draft and writing–review and editing. SS: visualization and writing–review and editing. JC: conceptualization, funding acquisition, and writing–review and editing. SG: conceptualization, funding acquisition, project administration, supervision, writing–original draft, and writing–review and editing.
Funding
The author(s) declare that financial support was received for the research, authorship, and/or publication of this article. This research was funded in part by the Galway University Foundation (grant number RNR1008), the Science Foundation Ireland (SFI) (grant number 17/CDA/4638), the European Regional Development Fund (ERDF) (grant number 13/RC/2073), Horizon Europe MSCA (grant number 101119873), a University of Galway Hardiman PhD Scholarship, the NCI (grant numbers U01 CA268813 and R01CA284315), the Breast Cancer Research Foundation (BCRF), the Cancer Prevention and Research Institute of Texas (CPRIT) (grant number RP220650), CREDO, and philanthropic support from M. Neal and R. Neal.
Conflict of interest
The authors declare that the research was conducted in the absence of any commercial or financial relationships that could be construed as a potential conflict of interest.
The author(s) declared that they were an editorial board member of Frontiers, at the time of submission. This had no impact on the peer review process and the final decision.
Publisher’s note
All claims expressed in this article are solely those of the authors and do not necessarily represent those of their affiliated organizations, or those of the publisher, the editors, and the reviewers. Any product that may be evaluated in this article, or claim that may be made by its manufacturer, is not guaranteed or endorsed by the publisher.
References
Akiyama T., Sudo C., Ogawara H., Toyoshima K., Yamamoto T. (1986). The product of the human c-erbB-2 gene: a 185-kilodalton glycoprotein with tyrosine kinase activity. Sci. (New York, N.Y.) 232 (4758), 1644–1646. doi:10.1126/science.3012781
Anand P., Hausladen A., Wang Y. J., Zhang G. F., Stomberski C., Brunengraber H., et al. (2014). Identification of S-nitroso-CoA reductases that regulate protein S-nitrosylation. Proc. Natl. Acad. Sci. U. S. A. 111 (52), 18572–18577. doi:10.1073/pnas.1417816112
Andl C. D., Mizushima T., Oyama K., Bowser M., Nakagawa H., Rustgi A. K. (2004). EGFR-induced cell migration is mediated predominantly by the JAK-STAT pathway in primary esophageal keratinocytes. Am. J. Physiology-Gastrointestinal Liver Physiology 287 (6), G1227–G1237. doi:10.1152/ajpgi.00253.2004
Andrechek E. R., White D., Muller W. J. (2005). Targeted disruption of ErbB2/Neu in the mammary epithelium results in impaired ductal outgrowth. Oncogene 24 (5), 932–937. doi:10.1038/sj.onc.1208230
Anido J., Matar P., Albanell J., Guzmán M., Rojo F., Arribas J., et al. (2003). ZD1839, a specific epidermal growth factor receptor (EGFR) tyrosine kinase inhibitor, induces the formation of inactive EGFR/HER2 and EGFR/HER3 heterodimers and prevents heregulin signaling in HER2-overexpressing breast cancer cells. Clin. Cancer Res. An Official J. Am. Assoc. Cancer Res. 9 (4), 1274–1283.
Arcila M. E., Chaft J. E., Nafa K., Roy-Chowdhuri S., Lau C., Zaidinski M., et al. (2012). Prevalence, clinicopathologic associations, and molecular spectrum of ERBB2 (HER2) tyrosine kinase mutations in lung adenocarcinomas. Clin. Cancer Res. 18 (18), 4910–4918. doi:10.1158/1078-0432.CCR-12-0912
Aurisicchio L., Marra E., Luberto L., Carlomosti F., De Vitis C., Noto A., et al. (2012). Novel anti-ErbB3 monoclonal antibodies show therapeutic efficacy in xenografted and spontaneous mouse tumors. J. Cell. Physiology 227 (10), 3381–3388. doi:10.1002/jcp.24037
Babykutty S., Suboj P., Srinivas P., Nair A. S., Chandramohan K., Gopala S. (2012). Insidious role of nitric oxide in migration/invasion of colon cancer cells by upregulating MMP-2/9 via activation of cGMP-PKG-ERK signaling pathways. Clin. Exp. Metastasis 29 (5), 471–492. doi:10.1007/s10585-012-9464-6
Bach E. A., Tanner J. W., Marsters S., Ashkenazi A., Aguet M., Shaw A. S., et al. (1996). Ligand-induced assembly and activation of the gamma interferon receptor in intact cells. Mol. Cell. Biol. 16 (6), 3214–3221. doi:10.1128/mcb.16.6.3214
Bailey J., Davis S., Shaw A., Diotallevi M., Fischer R., Benson M. A., et al. (2018). Tetrahydrobiopterin modulates ubiquitin conjugation to UBC13/UBE2N and proteasome activity by S-nitrosation. Sci. Rep. 8 (1), 14310. doi:10.1038/s41598-018-32481-4
Bang Y.-J., Van Cutsem E., Feyereislova A., Chung H. C., Shen L., Sawaki A., et al. (2010). Trastuzumab in combination with chemotherapy versus chemotherapy alone for treatment of HER2-positive advanced gastric or gastro-oesophageal junction cancer (ToGA): a phase 3, open-label, randomised controlled trial. Lancet 376 (9742), 687–697. doi:10.1016/S0140-6736(10)61121-X
Basudhar D., Glynn S. A., Greer M., Somasundaram V., No J. H., Scheiblin D. A., et al. (2017). Coexpression of NOS2 and COX2 accelerates tumor growth and reduces survival in estrogen receptor-negative breast cancer. Proc. Natl. Acad. Sci. U. S. A. 114 (49), 13030–13035. doi:10.1073/pnas.1709119114
Batzer A. G., Rotin D., Ureña J. M., Skolnik E. Y., Schlessinger J. (1994). Hierarchy of binding sites for Grb2 and Shc on the epidermal growth factor receptor. Mol. Cell. Biol. 14 (8), 5192–5201. doi:10.1128/mcb.14.8.5192
Baulida J., Kraus M. H., Alimandi M., Di Fiore P. P., Carpenter G. (1996). All ErbB receptors other than the epidermal growth factor receptor are endocytosis impaired. J. Biol. Chem. 271 (9), 5251–5257. doi:10.1074/jbc.271.9.5251
Benkhelifa S., Rafa H., Belhadef S., Ait-Kaci H., Medjeber O., Belkhelfa M., et al. (2019). Aberrant up-regulation of iNOS/NO system is correlated with an increased abundance of Foxp3+ cells and reduced effector/memory cell markers expression during colorectal cancer: immunomodulatory effects of cetuximab combined with chemotherapy. Inflammopharmacology 27 (4), 685–700. doi:10.1007/s10787-019-00566-9
Benz C. C., Scott G. K., Sarup J. C., Johnson R. M., Tripathy D., Coronado E., et al. (1992). Estrogen-dependent, tamoxifen-resistant tumorigenic growth of MCF-7 cells transfected with HER2/neu. Breast Cancer Res. Treat. 24 (2), 85–95. doi:10.1007/BF01961241
Bharadwaj U., Kasembeli M. M., Robinson P., Tweardy D. J. (2020). Targeting janus kinases and signal transducer and activator of transcription 3 to treat inflammation, fibrosis, and cancer: rationale, progress, and caution. Pharmacol. Rev. 72 (2), 486–526. doi:10.1124/pr.119.018440
Bobrow L. G., Millis R. R., Happerfield L. C., Gullick W. J. (1997). c-erbB-3 protein expression in ductal carcinoma in situ of the breast. Eur. J. Cancer 33 (11), 1846–1850. doi:10.1016/s0959-8049(97)00244-x
Bonner J. A., Harari P. M., Giralt J., Cohen R. B., Jones C. U., Sur R. K., et al. (2010). Radiotherapy plus cetuximab for locoregionally advanced head and neck cancer: 5-year survival data from a phase 3 randomised trial, and relation between cetuximab-induced rash and survival. Lancet. Oncol. 11 (1), 21–28. doi:10.1016/S1470-2045(09)70311-0
Burden S., Yarden Y. (1997). Neuregulins and their receptors: a versatile signaling module in organogenesis and oncogenesis. Neuron 18 (6), 847–855. doi:10.1016/s0896-6273(00)80324-4
Burke A. J., Sullivan F. J., Giles F. J., Glynn S. A. (2013). The yin and yang of nitric oxide in cancer progression. Carcinogenesis 34 (3), 503–512. doi:10.1093/carcin/bgt034
Campiglio M., Locatelli A., Olgiati C., Normanno N., Somenzi G., Viganò L., et al. (2004). Inhibition of proliferation and induction of apoptosis in breast cancer cells by the epidermal growth factor receptor (EGFR) tyrosine kinase inhibitor ZD1839 (’Iressa’) is independent of EGFR expression level. J. Cell. Physiology 198 (2), 259–268. doi:10.1002/jcp.10411
Cañas A., López-Sánchez L. M., Peñarando J., Valverde A., Conde F., Hernández V., et al. (2016). Altered S-nitrosothiol homeostasis provides a survival advantage to breast cancer cells in HER2 tumors and reduces their sensitivity to trastuzumab. Biochimica Biophysica Acta 1862 (4), 601–610. doi:10.1016/j.bbadis.2016.02.005
Carpenter G. (1987). Receptors for epidermal growth factor and other polypeptide mitogens. Annu. Rev. Biochem. 56, 881–914. doi:10.1146/annurev.bi.56.070187.004313
Carraway K. L., Weber J. L., Unger M. J., Ledesma J., Yu N., Gassmann M., et al. (1997). Neuregulin-2, a new ligand of ErbB3/ErbB4-receptor tyrosine kinases. Nature 387 (6632), 512–516. doi:10.1038/387512a0
Chattopadhyay A., Vecchi M., Ji Q. s., Mernaugh R., Carpenter G. (1999). The role of individual SH2 domains in mediating association of phospholipase C-gamma1 with the activated EGF receptor. J. Biol. Chem. 274 (37), 26091–26097. doi:10.1074/jbc.274.37.26091
Chazin V. R., Kaleko M., Miller A. D., Slamon D. J. (1992). Transformation mediated by the human HER-2 gene independent of the epidermal growth factor receptor. Oncogene 7 (9), 1859–1866.
Chen S., Guo D., Zhang W., Xie Y., Yang H., Cheng B., et al. (2018). Biglycan, a nitric oxide-downregulated proteoglycan, prevents nitric oxide-induced neuronal cell apoptosis via targeting erk1/2 and p38 signaling pathways. J. Mol. Neurosci. MN 66 (1), 68–76. doi:10.1007/s12031-018-1151-x
Chen Z., Huang K. Y., Ling Y., Goto M., Duan H. Q., Tong X. H., et al. (2019). Discovery of an oleanolic acid/hederagenin-nitric oxide donor hybrid as an EGFR tyrosine kinase inhibitor for non-small-cell lung cancer. J. Nat. Prod. 82 (11), 3065–3073. doi:10.1021/acs.jnatprod.9b00659
Cho H.-S., Mason K., Ramyar K. X., Stanley A. M., Gabelli S. B., Denney D. W., et al. (2003). Structure of the extracellular region of HER2 alone and in complex with the Herceptin Fab. Nature 421 (6924), 756–760. doi:10.1038/nature01392
Choi M. S., Nakamura T., Cho S. J., Han X., Holland E. A., Qu J., et al. (2014). Transnitrosylation from DJ-1 to PTEN attenuates neuronal cell death in Parkinson’s disease models. J. Neurosci. Official J. Soc. Neurosci. 34 (45), 15123–15131. doi:10.1523/JNEUROSCI.4751-13.2014
Chung A. W., Anand K., Anselme A. C., Chan A. A., Gupta N., Venta L. A., et al. (2021). A phase 1/2 clinical trial of the nitric oxide synthase inhibitor L-NMMA and taxane for treating chemoresistant triple-negative breast cancer. Sci. Transl. Med. 13 (624), eabj5070. doi:10.1126/scitranslmed.abj5070
Ciardiello F., Kim N., Saeki T., Dono R., Persico M. G., Plowman G. D., et al. (1991). Differential expression of epidermal growth factor-related proteins in human colorectal tumors. Proc. Natl. Acad. Sci. U. S. A. 88 (17), 7792–7796. doi:10.1073/pnas.88.17.7792
Cohen B. D., Green J. M., Foy L., Fell H. P. (1996). HER4-mediated biological and biochemical properties in NIH 3T3 cells. Evidence for HER1-HER4 heterodimers. J. Biol. Chem. 271 (9), 4813–4818. doi:10.1074/jbc.271.9.4813
Cohen S. (1965). The stimulation of epidermal proliferation by a specific protein (EGF). Dev. Biol. 12 (3), 394–407. doi:10.1016/0012-1606(65)90005-9
Colussi C., Mozzetta C., Gurtner A., Illi B., Rosati J., Straino S., et al. (2008). HDAC2 blockade by nitric oxide and histone deacetylase inhibitors reveals a common target in Duchenne muscular dystrophy treatment. Proc. Natl. Acad. Sci. U. S. A. 105 (49), 19183–19187. doi:10.1073/pnas.0805514105
Connell C. M., Doherty G. J. (2017). Activating HER2 mutations as emerging targets in multiple solid cancers. ESMO open 2 (5), e000279. doi:10.1136/esmoopen-2017-000279
Cortés J., Kim S. B., Chung W. P., Im S. A., Park Y. H., Hegg R., et al. (2022). Trastuzumab deruxtecan versus trastuzumab emtansine for breast cancer. N. Engl. J. Med. 386 (12), 1143–1154. doi:10.1056/NEJMoa2115022
Cunningham D., Humblet Y., Siena S., Khayat D., Bleiberg H., Santoro A., et al. (2004). Cetuximab monotherapy and cetuximab plus irinotecan in irinotecan-refractory metastatic colorectal cancer. N. Engl. J. Med. 351 (4), 337–345. doi:10.1056/NEJMoa033025
Dao V.T.-V., Elbatreek M. H., Fuchß T., Grädler U., Schmidt HHHW, Shah A. M., et al. (2021). “Nitric oxide synthase inhibitors into the clinic at last,” in Reactive oxygen species: network pharmacology and therapeutic applications. Editors H. H. H. W Schmidt, P Ghezzi, and A Cuadrado (Cham: Springer International Publishing (Handbook of Experimental Pharmacology), 169–204. doi:10.1007/164_2020_382
de Wit M., Fijneman R. J. A., Verheul H. M. W., Meijer G. A., Jimenez C. R. (2013). Proteomics in colorectal cancer translational research: biomarker discovery for clinical applications. Clin. Biochem. 46 (6), 466–479. doi:10.1016/j.clinbiochem.2012.10.039
Diaz N., Minton S., Cox C., Bowman T., Gritsko T., Garcia R., et al. (2006). Activation of stat3 in primary tumors from high-risk breast cancer patients is associated with elevated levels of activated SRC and survivin expression. Clin. Cancer Res. An Official J. Am. Assoc. Cancer Res. 12 (1), 20–28. doi:10.1158/1078-0432.CCR-04-1749
Ding Z., Ogata D., Roszik J., Qin Y., Kim S. H., Tetzlaff M. T., et al. (2021). iNOS associates with poor survival in melanoma: a role for nitric oxide in the PI3K-akt pathway stimulation and PTEN S-nitrosylation. Front. Oncol. 11, 631766. doi:10.3389/fonc.2021.631766
Dingemans A.-M. C., Groen H. J. M., Herder G. J. M., Stigt J. A., Smit E. F., Bahce I., et al. (2015). A randomized phase II study comparing paclitaxel-carboplatin-bevacizumab with or without nitroglycerin patches in patients with stage IV nonsquamous nonsmall-cell lung cancer: NVALT12 (NCT01171170)†. Ann. Oncol. Official J. Eur. Soc. Med. Oncol. 26 (11), 2286–2293. doi:10.1093/annonc/mdv370
Donoghue J. F., Kerr L. T., Alexander N. W., Greenall S. A., Longano A. B., Gottardo N. G., et al. (2018). Activation of ERBB4 in glioblastoma can contribute to increased tumorigenicity and influence therapeutic response. Cancers 10 (8), 243. doi:10.3390/cancers10080243
Douillard J.-Y., Siena S., Cassidy J., Tabernero J., Burkes R., Barugel M., et al. (2010). Randomized, phase III trial of panitumumab with infusional fluorouracil, leucovorin, and oxaliplatin (FOLFOX4) versus FOLFOX4 alone as first-line treatment in patients with previously untreated metastatic colorectal cancer: the PRIME study. J. Clin. Oncol. Official J. Am. Soc. Clin. Oncol. 28 (31), 4697–4705. doi:10.1200/JCO.2009.27.4860
Downward J., Parker P., Waterfield M. D. (1984b). Autophosphorylation sites on the epidermal growth factor receptor. Nature 311 (5985), 483–485. doi:10.1038/311483a0
Downward J., Yarden Y., Mayes E., Scrace G., Totty N., Stockwell P., et al. (1984a). Close similarity of epidermal growth factor receptor and v-erb-B oncogene protein sequences. Nature 307 (5951), 521–527. doi:10.1038/307521a0
Du H., Chen L., Zhan N., Mu J., Ren B., Zuo J. (2019). A new insight to explore the regulation between S-nitrosylation and N-glycosylation. Plant Direct 3 (2), e00110. doi:10.1002/pld3.110
Duneau J.-P., Vegh A. P., Sturgis J. N. (2007). A dimerization hierarchy in the transmembrane domains of the HER receptor family. Biochemistry 46 (7), 2010–2019. doi:10.1021/bi061436f
Duru N., Fan M., Candas D., Menaa C., Liu H. C., Nantajit D., et al. (2012). HER2-associated radioresistance of breast cancer stem cells isolated from HER2-negative breast cancer cells. Clin. Cancer Res. An Official J. Am. Assoc. Cancer Res. 18 (24), 6634–6647. doi:10.1158/1078-0432.CCR-12-1436
Edwards J., Traynor P., Munro A. F., Pirret C. F., Dunne B., Bartlett J. M. S. (2006). The role of HER1-HER4 and EGFRvIII in hormone-refractory prostate cancer. Clin. Cancer Res. An Official J. Am. Assoc. Cancer Res. 12 (1), 123–130. doi:10.1158/1078-0432.CCR-05-1445
Ejskjaer K., Sørensen B. S., Poulsen S. S., Forman A., Nexø E., Mogensen O. (2007). Expression of the epidermal growth factor system in endometrioid endometrial cancer. Gynecol. Oncol. 104 (1), 158–167. doi:10.1016/j.ygyno.2006.07.015
Elenius K., Choi C. J., Paul S., Santiestevan E., Nishi E., Klagsbrun M. (1999). Characterization of a naturally occurring ErbB4 isoform that does not bind or activate phosphatidyl inositol 3-kinase. Oncogene 18 (16), 2607–2615. doi:10.1038/sj.onc.1202612
EMA (2021a). Perjeta | European medicines agency. Available at: https://www.ema.europa.eu/en/medicines/human/EPAR/perjeta (Accessed: February 5, 2024).
EMA (2021b). Vizimpro | European medicines agency. Available at: https://www.ema.europa.eu/en/medicines/human/EPAR/vizimpro (Accessed: February 5, 2024).
EMA (2022a). Erbitux | European medicines agency. Available at: https://www.ema.europa.eu/en/medicines/human/EPAR/erbitux (Accessed: February 5, 2024).
EMA (2022b). Vectibix | European medicines agency. Available at: https://www.ema.europa.eu/en/medicines/human/EPAR/vectibix (Accessed: February 5, 2024).
EMA (2023a). Enhertu | European medicines agency. Available at: https://www.ema.europa.eu/en/medicines/human/EPAR/enhertu (Accessed: February 5, 2024).
EMA (2023b). Giotrif | European medicines agency. Available at: https://www.ema.europa.eu/en/medicines/human/EPAR/giotrif (Accessed: February 5, 2024).
EMA (2023c). Herceptin | European medicines agency. Available at: https://www.ema.europa.eu/en/medicines/human/EPAR/herceptin (Accessed: February 5, 2024).
EMA (2023d). Iressa | European medicines agency. Available at: https://www.ema.europa.eu/en/medicines/human/EPAR/iressa (Accessed: February 5, 2024).
EMA (2023e). Kadcyla | European medicines agency. Available at: https://www.ema.europa.eu/en/medicines/human/EPAR/kadcyla (Accessed: February 5, 2024).
EMA (2023f). Nerlynx | European medicines agency. Available at: https://www.ema.europa.eu/en/medicines/human/EPAR/nerlynx (Accessed: February 5, 2024).
EMA (2023g). Tagrisso | European medicines agency. Available at: https://www.ema.europa.eu/en/medicines/human/EPAR/tagrisso (Accessed: February 5, 2024).
EMA (2023h). Tarceva | European medicines agency. Available at: https://www.ema.europa.eu/en/medicines/human/EPAR/tarceva (Accessed: February 5, 2024).
EMA (2023i). Tukysa | European medicines agency. Available at: https://www.ema.europa.eu/en/medicines/human/EPAR/tukysa (Accessed: February 5, 2024).
EMA (2023j). Tyverb | European medicines agency. Available at: https://www.ema.europa.eu/en/medicines/human/EPAR/tyverb (Accessed: February 5, 2024).
Engelman J. A., Zejnullahu K., Mitsudomi T., Song Y., Hyland C., Park J. O., et al. (2007). MET amplification leads to gefitinib resistance in lung cancer by activating ERBB3 signaling. Sci. (New York, N.Y.) 316 (5827), 1039–1043. doi:10.1126/science.1141478
Erdogan F., Radu T. B., Orlova A., Qadree A. K., de Araujo E. D., Israelian J., et al. (2022). JAK-STAT core cancer pathway: an integrative cancer interactome analysis. J. Cell. Mol. Med. 26 (7), 2049–2062. doi:10.1111/jcmm.17228
Fader A. N., Roque D. M., Siegel E., Buza N., Hui P., Abdelghany O., et al. (2020). Randomized phase II trial of carboplatin-paclitaxel compared with carboplatin-paclitaxel-trastuzumab in advanced (stage III-IV) or recurrent uterine serous carcinomas that overexpress her2/neu (NCT01367002): updated overall survival analysis. Clin. Cancer Res. An Official J. Am. Assoc. Cancer Res. 26 (15), 3928–3935. doi:10.1158/1078-0432.CCR-20-0953
Fiddes R. J., Campbell D. H., Janes P. W., Sivertsen S. P., Sasaki H., Wallasch C., et al. (1998). Analysis of Grb7 recruitment by heregulin-activated erbB receptors reveals a novel target selectivity for erbB3. J. Biol. Chem. 273 (13), 7717–7724. doi:10.1074/jbc.273.13.7717
Förstermann U., Sessa W. C. (2012). Nitric oxide synthases: regulation and function. Eur. Heart J. 33 (7), 829–837. doi:10.1093/eurheartj/ehr304
Foster M. W., Hess D. T., Stamler J. S. (2009). Protein S-nitrosylation in health and disease: a current perspective. Trends Mol. Med. 15 (9), 391–404. doi:10.1016/j.molmed.2009.06.007
Franklin M. C., Carey K. D., Vajdos F. F., Leahy D. J., de Vos A. M., Sliwkowski M. X. (2004). Insights into ErbB signaling from the structure of the ErbB2-pertuzumab complex. Cancer Cell 5 (4), 317–328. doi:10.1016/S1535-6108(04)00083-2
Friess H., Yamanaka Y., Kobrin M. S., Do D. A., Büchler M. W., Korc M. (1995). Enhanced erbB-3 expression in human pancreatic cancer correlates with tumor progression. Clin. Cancer Res. An Official J. Am. Assoc. Cancer Res. 1 (11), 1413–1420.
Fujimoto H., Ando Y., Yamashita T., Terazaki H., Tanaka Y., Sasaki J., et al. (1997). Nitric oxide synthase activity in human lung cancer. Jpn. J. Cancer Res. Gann 88 (12), 1190–1198. doi:10.1111/j.1349-7006.1997.tb00348.x
Fulda S., Debatin K.-M. (2002). IFNgamma sensitizes for apoptosis by upregulating caspase-8 expression through the Stat1 pathway. Oncogene 21 (15), 2295–2308. doi:10.1038/sj.onc.1205255
Gao Y., Hubbert C. C., Yao T.-P. (2010). The microtubule-associated histone deacetylase 6 (HDAC6) regulates epidermal growth factor receptor (EGFR) endocytic trafficking and degradation. J. Biol. Chem. 285 (15), 11219–11226. doi:10.1074/jbc.M109.042754
Garrett T. P. J., McKern N. M., Lou M., Elleman T. C., Adams T. E., Lovrecz G. O., et al. (2003). The crystal structure of a truncated ErbB2 ectodomain reveals an active conformation, poised to interact with other ErbB receptors. Mol. Cell 11 (2), 495–505. doi:10.1016/s1097-2765(03)00048-0
Garrido P., Shalaby A., Walsh E. M., Keane N., Webber M., Keane M. M., et al. (2017). Impact of inducible nitric oxide synthase (iNOS) expression on triple negative breast cancer outcome and activation of EGFR and ERK signaling pathways. Oncotarget 8 (46), 80568–80588. doi:10.18632/oncotarget.19631
Gaston B. M., Carver J., Doctor A., Palmer L. A. (2003). S-nitrosylation signaling in cell biology. Mol. Interv. 3 (5), 253–263. doi:10.1124/mi.3.5.253
Geyer C. E., Forster J., Lindquist D., Chan S., Romieu C. G., Pienkowski T., et al. (2006). Lapatinib plus capecitabine for HER2-positive advanced breast cancer. N. Engl. J. Med. 355 (26), 2733–2743. doi:10.1056/NEJMoa064320
Gianni L., Pienkowski T., Im Y. H., Roman L., Tseng L. M., Liu M. C., et al. (2012). Efficacy and safety of neoadjuvant pertuzumab and trastuzumab in women with locally advanced, inflammatory, or early HER2-positive breast cancer (NeoSphere): a randomised multicentre, open-label, phase 2 trial. Lancet. Oncol. 13 (1), 25–32. doi:10.1016/S1470-2045(11)70336-9
Giri S., Rattan R., Deshpande M., Maguire J. L., Johnson Z., Graham R. P., et al. (2014). Preclinical therapeutic potential of a nitrosylating agent in the treatment of ovarian cancer. PloS One 9 (6), e97897. doi:10.1371/journal.pone.0097897
Glynn S. A., Boersma B. J., Dorsey T. H., Yi M., Yfantis H. G., Ridnour L. A., et al. (2010). Increased NOS2 predicts poor survival in estrogen receptor-negative breast cancer patients. J. Clin. Investigation 120 (11), 3843–3854. doi:10.1172/JCI42059
Goh L. K., Huang F., Kim W., Gygi S., Sorkin A. (2010). Multiple mechanisms collectively regulate clathrin-mediated endocytosis of the epidermal growth factor receptor. J. Cell Biol. 189 (5), 871–883. doi:10.1083/jcb.201001008
Graus-Porta D., Beerli R. R., Daly J. M., Hynes N. E. (1997). ErbB-2, the preferred heterodimerization partner of all ErbB receptors, is a mediator of lateral signaling. EMBO J. 16 (7), 1647–1655. doi:10.1093/emboj/16.7.1647
Graus-Porta D., Beerli R. R., Hynes N. E. (1995). Single-chain antibody-mediated intracellular retention of ErbB-2 impairs Neu differentiation factor and epidermal growth factor signaling. Mol. Cell. Biol. 15 (3), 1182–1191. doi:10.1128/MCB.15.3.1182
Harari D., Tzahar E., Romano J., Shelly M., Pierce J. H., Andrews G. C., et al. (1999). Neuregulin-4: a novel growth factor that acts through the ErbB-4 receptor tyrosine kinase. Oncogene 18 (17), 2681–2689. doi:10.1038/sj.onc.1202631
Hazan R., Margolis B., Dombalagian M., Ullrich A., Zilberstein A., Schlessinger J. (1990). Identification of autophosphorylation sites of HER2/neu. Cell Growth and Differ. Mol. Biol. J. Am. Assoc. Cancer Res. 1 (1), 3–7.
Hemmann U., Gerhartz C., Heesel B., Sasse J., Kurapkat G., Grötzinger J., et al. (1996). Differential activation of acute phase response factor/Stat3 and Stat1 via the cytoplasmic domain of the interleukin 6 signal transducer gp130. II. Src homology SH2 domains define the specificity of stat factor activation. J. Biol. Chem. 271 (22), 12999–13007. doi:10.1074/jbc.271.22.12999
Hendriks B. S., Opresko L. K., Wiley H. S., Lauffenburger D. (2003). Quantitative analysis of HER2-mediated effects on HER2 and epidermal growth factor receptor endocytosis: distribution of homo- and heterodimers depends on relative HER2 levels. J. Biol. Chem. 278 (26), 23343–23351. doi:10.1074/jbc.M300477200
Higashiyama S., Abraham J. A., Miller J., Fiddes J. C., Klagsbrun M. (1991). A heparin-binding growth factor secreted by macrophage-like cells that is related to EGF. Sci. (New York, N.Y.) 251 (4996), 936–939. doi:10.1126/science.1840698
Holbro T., Beerli R. R., Maurer F., Koziczak M., Barbas C. F., Hynes N. E. (2003). The ErbB2/ErbB3 heterodimer functions as an oncogenic unit: ErbB2 requires ErbB3 to drive breast tumor cell proliferation. Proc. Natl. Acad. Sci. U. S. A. 100 (15), 8933–8938. doi:10.1073/pnas.1537685100
Hsuan J. J., Totty N., Waterfield M. D. (1989). Identification of a novel autophosphorylation site (P4) on the epidermal growth factor receptor. Biochem. J. 262 (2), 659–663. doi:10.1042/bj2620659
Huang S., Li C., Armstrong E. A., Peet C. R., Saker J., Amler L. C., et al. (2013). Dual targeting of EGFR and HER3 with MEHD7945A overcomes acquired resistance to EGFR inhibitors and radiation. Cancer Res. 73 (2), 824–833. doi:10.1158/0008-5472.CAN-12-1611
Hundley T. R., Rigas B. (2006). Nitric oxide-donating aspirin inhibits colon cancer cell growth via mitogen-activated protein kinase activation. J. Pharmacol. Exp. Ther. 316 (1), 25–34. doi:10.1124/jpet.105.091363
Hunter T. (2009). Tyrosine phosphorylation: thirty years and counting. Curr. Opin. Cell Biol. 21 (2), 140–146. doi:10.1016/j.ceb.2009.01.028
Ignarro L. J. (1989). Biological actions and properties of endothelium-derived nitric oxide formed and released from artery and vein. Circulation Res. 65 (1), 1–21. doi:10.1161/01.res.65.1.1
Ignarro L. J., Buga G. M., Wood K. S., Byrns R. E., Chaudhuri G. (1987). Endothelium-derived relaxing factor produced and released from artery and vein is nitric oxide. Proc. Natl. Acad. Sci. U. S. A. 84 (24), 9265–9269. doi:10.1073/pnas.84.24.9265
Illum H., Wang D. H., Dowell J. E., Hittson W. J., Torrisi J. R., Meyer J., et al. (2015). Phase I dose escalation trial of nitroglycerin in addition to 5-fluorouracil and radiation therapy for neoadjuvant treatment of operable rectal cancer. Surgery 158 (2), 460–465. doi:10.1016/j.surg.2015.04.007
Iwakura Y., Nawa H. (2013). ErbB1-4-dependent EGF/neuregulin signals and their cross talk in the central nervous system: pathological implications in schizophrenia and Parkinson’s disease. Front. Cell. Neurosci. 7, 4. doi:10.3389/fncel.2013.00004
Jacob W., James I., Hasmann M., Weisser M. (2018). Clinical development of HER3-targeting monoclonal antibodies: perils and progress. Cancer Treat. Rev. 68, 111–123. doi:10.1016/j.ctrv.2018.06.011
Jaiswal J., Egert J., Engesser R., Peyrotón A. A., Nogay L., Weichselberger V., et al. (2023). Mutual repression between JNK/AP-1 and JAK/STAT stratifies senescent and proliferative cell behaviors during tissue regeneration. PLOS Biol. 21 (5), e3001665. doi:10.1371/journal.pbio.3001665
Jathal M. K., Chen L., Mudryj M., Ghosh P. M. (2011). Targeting ErbB3: the new RTK(id) on the prostate cancer block. Immunol. Endocr. Metabolic Agents Med. Chem. 11 (2), 131–149. doi:10.2174/187152211795495643
Jones R. B., Gordus A., Krall J. A., MacBeath G. (2006). A quantitative protein interaction network for the ErbB receptors using protein microarrays. Nature 439 (7073), 168–174. doi:10.1038/nature04177
Kamm A., Przychodzen P., Kuban-Jankowska A., Jacewicz D., Dabrowska A. M., Nussberger S., et al. (2019). Nitric oxide and its derivatives in the cancer battlefield. Nitric Oxide Biol. Chem. 93, 102–114. doi:10.1016/j.niox.2019.09.005
Kanner J., Harel S., Granit R. (1991). Nitric oxide as an antioxidant. Archives Biochem. Biophysics 289 (1), 130–136. doi:10.1016/0003-9861(91)90452-o
Karapetis C. S., Khambata-Ford S., Jonker D. J., O’Callaghan C. J., Tu D., Tebbutt N. C., et al. (2008). K-ras mutations and benefit from cetuximab in advanced colorectal cancer. N. Engl. J. Med. 359 (17), 1757–1765. doi:10.1056/NEJMoa0804385
Karunagaran D., Tzahar E., Beerli R. R., Chen X., Graus-Porta D., Ratzkin B. J., et al. (1996). ErbB-2 is a common auxiliary subunit of NDF and EGF receptors: implications for breast cancer. EMBO J. 15 (2), 254–264. doi:10.1002/j.1460-2075.1996.tb00356.x
Keilhack H., Tenev T., Nyakatura E., Godovac-Zimmermann J., Nielsen L., Seedorf K., et al. (1998). Phosphotyrosine 1173 mediates binding of the protein-tyrosine phosphatase SHP-1 to the epidermal growth factor receptor and attenuation of receptor signaling. J. Biol. Chem. 273 (38), 24839–24846. doi:10.1074/jbc.273.38.24839
Kielbik M., Klink M., Brzezinska M., Szulc I., Sulowska Z. (2013). Nitric oxide donors: spermine/NO and diethylenetriamine/NO induce ovarian cancer cell death and affect STAT3 and AKT signaling proteins. Nitric Oxide Biol. Chem. 35, 93–109. doi:10.1016/j.niox.2013.09.001
Kim H. H., Vijapurkar U., Hellyer N. J., Bravo D., Koland J. G. (1998). Signal transduction by epidermal growth factor and heregulin via the kinase-deficient ErbB3 protein. Biochem. J. 334 (1), 189–195. doi:10.1042/bj3340189
Kim M. M., Parmar H. A., Schipper M., Devasia T., Aryal M. P., Kesari S., et al. (2020). BRAINSTORM: a multi-institutional phase 1/2 study of RRx-001 in combination with whole brain radiation therapy for patients with brain metastases. Int. J. Radiat. Oncol. Biol. Phys. 107 (3), 478–486. doi:10.1016/j.ijrobp.2020.02.639
King C. R., Kraus M. H., Aaronson S. A. (1985). Amplification of a novel v-erbB-related gene in a human mammary carcinoma. Sci. (New York, N.Y.) 229 (4717), 974–976. doi:10.1126/science.2992089
Kitagaki J., Yang Y., Saavedra J. E., Colburn N. H., Keefer L. K., Perantoni A. O. (2009). Nitric oxide prodrug JS-K inhibits ubiquitin E1 and kills tumor cells retaining wild type p53. Oncogene 28 (4), 619–624. doi:10.1038/onc.2008.401
Kornberg M. D., Sen N., Hara M. R., Juluri K. R., Nguyen J. V. K., Snowman A. M., et al. (2010). GAPDH mediates nitrosylation of nuclear proteins. Nat. Cell Biol. 12 (11), 1094–1100. doi:10.1038/ncb2114
Kwak Y.-D., Ma T., Diao S., Zhang X., Chen Y., Hsu J., et al. (2010). NO signaling and S-nitrosylation regulate PTEN inhibition in neurodegeneration. Mol. Neurodegener. 5, 49. doi:10.1186/1750-1326-5-49
Lacenere C. J., Sternberg P. W. (2000). Regulation of EGF receptor signaling in the fruitfly D. melanogaster and the nematode C. elegans. Breast Dis. 11, 19–30. doi:10.3233/bd-1999-11103
Lee H.-C., An S., Lee H., Woo S. H., Jin H. O., Seo S. K., et al. (2008). Activation of epidermal growth factor receptor and its downstream signaling pathway by nitric oxide in response to ionizing radiation. Mol. cancer Res. MCR 6 (6), 996–1002. doi:10.1158/1541-7786.MCR-08-0113
Lee K. F., Simon H., Chen H., Bates B., Hung M. C., Hauser C. (1995). Requirement for neuregulin receptor erbB2 in neural and cardiac development. Nature 378 (6555), 394–398. doi:10.1038/378394a0
Lee M.-J., Tomita Y., Yuno A., Lee S., Abrouk N. E., Oronsky B., et al. (2021). Results from a biomarker study to accompany a phase II trial of RRx-001 with reintroduced platinum-based chemotherapy in relapsed small cell carcinoma. Expert Opin. Investigational Drugs 30 (2), 177–183. doi:10.1080/13543784.2021.1863947
Lemmon M. A., Bu Z., Ladbury J. E., Zhou M., Pinchasi D., Lax I., et al. (1997). Two EGF molecules contribute additively to stabilization of the EGFR dimer. EMBO J. 16 (2), 281–294. doi:10.1093/emboj/16.2.281
Lenferink A. E., Pinkas-Kramarski R., van de Poll M. L., van Vugt M. J., Klapper L. N., Tzahar E., et al. (1998). Differential endocytic routing of homo- and hetero-dimeric ErbB tyrosine kinases confers signaling superiority to receptor heterodimers. EMBO J. 17 (12), 3385–3397. doi:10.1093/emboj/17.12.3385
Leung H. Y., Weston J., Gullick W. J., Williams G. (1997). A potential autocrine loop between heregulin-alpha and erbB-3 receptor in human prostatic adenocarcinoma. Br. J. Urology 79 (2), 212–216. doi:10.1046/j.1464-410x.1997.30412.x
Levkowitz G., Klapper L. N., Tzahar E., Freywald A., Sela M., Yarden Y. (1996). Coupling of the c-Cbl protooncogene product to ErbB-1/EGF-receptor but not to other ErbB proteins. Oncogene 12 (5), 1117–1125.
Levkowitz G., Waterman H., Ettenberg S. A., Katz M., Tsygankov A. Y., Alroy I., et al. (1999). Ubiquitin ligase activity and tyrosine phosphorylation underlie suppression of growth factor signaling by c-Cbl/Sli-1. Mol. Cell 4 (6), 1029–1040. doi:10.1016/s1097-2765(00)80231-2
Li B. T., Smit E. F., Goto Y., Nakagawa K., Udagawa H., Mazières J., et al. (2022). Trastuzumab deruxtecan in HER2-mutant non-small-cell lung cancer. N. Engl. J. Med. 386 (3), 241–251. doi:10.1056/NEJMoa2112431
Li D. M., Sun H. (1997). TEP1, encoded by a candidate tumor suppressor locus, is a novel protein tyrosine phosphatase regulated by transforming growth factor beta. Cancer Res. 57 (11), 2124–2129.
Li H., Yang F., Liu C., Xiao P., Xu Y., Liang Z., et al. (2016). Crystal structure and substrate specificity of PTPN12. Cell Rep. 15 (6), 1345–1358. doi:10.1016/j.celrep.2016.04.016
Li J., Yen C., Liaw D., Podsypanina K., Bose S., Wang S. I., et al. (1997). PTEN, a putative protein tyrosine phosphatase gene mutated in human brain, breast, and prostate cancer. Sci. (New York, N.Y.) 275 (5308), 1943–1947. doi:10.1126/science.275.5308.1943
Lin N. U., Diéras V., Paul D., Lossignol D., Christodoulou C., Stemmler H. J., et al. (2009). Multicenter phase II study of lapatinib in patients with brain metastases from HER2-positive breast cancer. Clin. Cancer Res. An Official J. Am. Assoc. Cancer Res. 15 (4), 1452–1459. doi:10.1158/1078-0432.CCR-08-1080
Lin Y., Ceacareanu A. C., Hassid A. (2003). Nitric oxide-induced inhibition of aortic smooth muscle cell motility: role of PTP-PEST and adaptor proteins p130cas and Crk. Am. J. Physiology-Heart Circulatory Physiology 285 (2), H710–H721. doi:10.1152/ajpheart.01127.2002
Liu J., Kern J. A. (2002). Neuregulin-1 activates the JAK-STAT pathway and regulates lung epithelial cell proliferation. Am. J. Respir. Cell Mol. Biol. 27 (3), 306–313. doi:10.1165/rcmb.4850
Liu N., Zhu M., Linhai Y., Song Y., Gui X., Tan G., et al. (2018). Increasing HER2 α2,6 sialylation facilitates gastric cancer progression and resistance via the Akt and ERK pathways. Oncol. Rep. 40 (5), 2997–3005. doi:10.3892/or.2018.6680
Liu X., Zhang Y., Wang Y., Yang M., Hong F., Yang S. (2021). Protein phosphorylation in cancer: role of nitric oxide signaling pathway. Biomolecules 11 (7), 1009. doi:10.3390/biom11071009
Liu Y., Song L., Ni H., Sun L., Jiao W., Chen L., et al. (2017). ERBB4 acts as a suppressor in the development of hepatocellular carcinoma. Carcinogenesis 38 (4), 465–473. doi:10.1093/carcin/bgx017
Liu Z., Zanata S. M., Kim J., Peterson M. A., Di Vizio D., Chirieac L. R., et al. (2013). The ubiquitin-specific protease USP2a prevents endocytosis-mediated EGFR degradation. Oncogene 32 (13), 1660–1669. doi:10.1038/onc.2012.188
Long W., Wagner K. U., Lloyd K. C. K., Binart N., Shillingford J. M., Hennighausen L., et al. (2003). Impaired differentiation and lactational failure of Erbb4-deficient mammary glands identify ERBB4 as an obligate mediator of STAT5. Development 130 (21), 5257–5268. doi:10.1242/dev.00715
Luke J. J., LoRusso P., Shapiro G. I., Krivoshik A., Schuster R., Yamazaki T., et al. (2016). ASP9853, an inhibitor of inducible nitric oxide synthase dimerization, in combination with docetaxel: preclinical investigation and a Phase I study in advanced solid tumors. Cancer Chemother. Pharmacol. 77 (3), 549–558. doi:10.1007/s00280-016-2967-0
Lundberg J. O., Weitzberg E. (2013). Biology of nitrogen oxides in the gastrointestinal tract. Gut 62 (4), 616–629. doi:10.1136/gutjnl-2011-301649
Lundberg J. O., Weitzberg E. (2022). Nitric oxide signaling in health and disease. Cell 185 (16), 2853–2878. doi:10.1016/j.cell.2022.06.010
Margolis B. L., Lax I., Kris R., Dombalagian M., Honegger A. M., Howk R., et al. (1989). All autophosphorylation sites of epidermal growth factor (EGF) receptor and HER2/neu are located in their carboxyl-terminal tails. J. Biol. Chem. 264 (18), 10667–10671. doi:10.1016/s0021-9258(18)81674-x
Marquardt H., Hunkapiller M. W., Hood L. E., Todaro G. J. (1984). Rat transforming growth factor type 1: structure and relation to epidermal growth factor. Sci. (New York, N.Y.) 223 (4640), 1079–1082. doi:10.1126/science.6320373
Marshall H. E., Foster M. W. (2012). S-nitrosylation of Ras in breast cancer. Breast cancer Res. BCR 14 (6), 113. doi:10.1186/bcr3331
Mazorra Z., Lavastida A., Concha-Benavente F., Valdés A., Srivastava R. M., García-Bates T. M., et al. (2017). Nimotuzumab induces NK cell activation, cytotoxicity, dendritic cell maturation and expansion of EGFR-specific T cells in head and neck cancer patients. Front. Pharmacol. 8, 382. doi:10.3389/fphar.2017.00382
McDonagh C. F., Huhalov A., Harms B. D., Adams S., Paragas V., Oyama S., et al. (2012). Antitumor activity of a novel bispecific antibody that targets the ErbB2/ErbB3 oncogenic unit and inhibits heregulin-induced activation of ErbB3. Mol. Cancer Ther. 11 (3), 582–593. doi:10.1158/1535-7163.MCT-11-0820
Memon A. A., Sorensen B. S., Meldgaard P., Fokdal L., Thykjaer T., Nexo E. (2006). The relation between survival and expression of HER1 and HER2 depends on the expression of HER3 and HER4: a study in bladder cancer patients. Br. J. Cancer 94 (11), 1703–1709. doi:10.1038/sj.bjc.6603154
Michel T., Feron O. (1997). Nitric oxide synthases: which, where, how, and why? J. Clin. Investigation 100 (9), 2146–2152. doi:10.1172/JCI119750
Miettinen P. J., Berger J. E., Meneses J., Phung Y., Pedersen R. A., Werb Z., et al. (1995). Epithelial immaturity and multiorgan failure in mice lacking epidermal growth factor receptor. Nature 376 (6538), 337–341. doi:10.1038/376337a0
Mills G. B., Yarden Y. (2010). The rebirth of a phoenix: ovarian cancers are addicted to ErbB-3. Cancer Cell 17 (3), 217–218. doi:10.1016/j.ccr.2010.02.023
Mimeault M., Jouy N., Depreux P., Hénichart J. P. (2005). Synergistic antiproliferative and apoptotic effects induced by mixed epidermal growth factor receptor inhibitor ZD1839 and nitric oxide donor in human prostatic cancer cell lines. Prostate 62 (2), 187–199. doi:10.1002/pros.20138
Mitchell D. A., Marletta M. A. (2005). Thioredoxin catalyzes the S-nitrosation of the caspase-3 active site cysteine. Nat. Chem. Biol. 1 (3), 154–158. doi:10.1038/nchembio720
Mnatsakanyan R., Markoutsa S., Walbrunn K., Roos A., Verhelst S. H. L., Zahedi R. P. (2019). Proteome-wide detection of S-nitrosylation targets and motifs using bioorthogonal cleavable-linker-based enrichment and switch technique. Nat. Commun. 10 (1), 2195. doi:10.1038/s41467-019-10182-4
Moscatello D. K., Holgado-Madruga M., Godwin A. K., Ramirez G., Gunn G., Zoltick P. W., et al. (1995). Frequent expression of a mutant epidermal growth factor receptor in multiple human tumors. Cancer Res. 55 (23), 5536–5539.
Murthy R. K., Loi S., Okines A., Paplomata E., Hamilton E., Hurvitz S. A., et al. (2020). Tucatinib, trastuzumab, and capecitabine for HER2-positive metastatic breast cancer. N. Engl. J. Med. 382 (7), 597–609. doi:10.1056/NEJMoa1914609
Nahta R., Hung M.-C., Esteva F. J. (2004). The HER-2-targeting antibodies trastuzumab and pertuzumab synergistically inhibit the survival of breast cancer cells. Cancer Res. 64 (7), 2343–2346. doi:10.1158/0008-5472.can-03-3856
Nakamura T., Lipton S. A. (2013). Emerging role of protein-protein transnitrosylation in cell signaling pathways. Antioxidants Redox Signal. 18 (3), 239–249. doi:10.1089/ars.2012.4703
Nathan C., Xie Q. W. (1994). Nitric oxide synthases: roles, tolls, and controls. Cell 78 (6), 915–918. doi:10.1016/0092-8674(94)90266-6
NCBI (2024a). L-Name. Available at: https://pubchem.ncbi.nlm.nih.gov/compound/39836 (Accessed February 6, 2024).
NCBI (2024b). Arginine. Available at: https://pubchem.ncbi.nlm.nih.gov/compound/6322 (Accessed: February 6, 2024).
NCBI (2024c). Tilarginine. Available at: https://pubchem.ncbi.nlm.nih.gov/compound/132862 (Accessed: February 6, 2024).
Ni C.-Y., Murphy M. P., Golde T. E., Carpenter G. (2001). Gamma -Secretase cleavage and nuclear localization of ErbB-4 receptor tyrosine kinase. Science 294 (5549), 2179–2181. doi:10.1126/science.1065412
Nicholson R. I., Gee J. M. W., Harper M. E. (2001). EGFR and cancer prognosis. Eur. J. Cancer 37, 9–15. doi:10.1016/S0959-8049(01)00231-3
Niravath P. A. (2023). Clinical phase Ib/II trial of L-NMMA plus taxane chemotherapy in the treatment of refractory locally advanced or metastatic triple negative breast cancer patients. Clinical trial registration NCT02834403. clinicaltrials.gov. Available at: https://clinicaltrials.gov/study/NCT02834403 (Accessed January 1, 2024).
Noguchi N., Kondo Y., Maeda N., Higa-Nakamine S., Toku S., Maruyama J., et al. (2013). Phosphorylation of epidermal growth factor receptor at serine 1047 by MAP kinase-activated protein kinase-2 in cultured lung epithelial cells treated with flagellin. Archives Biochem. Biophysics 529 (2), 75–85. doi:10.1016/j.abb.2012.11.006
Numajiri N., Takasawa K., Nishiya T., Tanaka H., Ohno K., Hayakawa W., et al. (2011). On–off system for PI3-kinase–Akt signaling through S-nitrosylation of phosphatase with sequence homology to tensin (PTEN). Proc. Natl. Acad. Sci. 108 (25), 10349–10354. doi:10.1073/pnas.1103503108
Ocana A., Vera-Badillo F., Seruga B., Templeton A., Pandiella A., Amir E. (2013). HER3 overexpression and survival in solid tumors: a meta-analysis. J. Natl. Cancer Inst. 105 (4), 266–273. doi:10.1093/jnci/djs501
Ohno K., Okuda K., Uehara T. (2015). Endogenous S-sulfhydration of PTEN helps protect against modification by nitric oxide. Biochem. Biophysical Res. Commun. 456 (1), 245–249. doi:10.1016/j.bbrc.2014.11.066
Okabayashi Y., Kido Y., Okutani T., Sugimoto Y., Sakaguchi K., Kasuga M. (1994). Tyrosines 1148 and 1173 of activated human epidermal growth factor receptors are binding sites of Shc in intact cells. J. Biol. Chem. 269 (28), 18674–18678. doi:10.1016/s0021-9258(17)32363-3
Okutani T., Okabayashi Y., Kido Y., Sugimoto Y., Sakaguchi K., Matuoka K., et al. (1994). Grb2/Ash binds directly to tyrosines 1068 and 1086 and indirectly to tyrosine 1148 of activated human epidermal growth factor receptors in intact cells. J. Biol. Chem. 269 (49), 31310–31314. doi:10.1016/s0021-9258(18)47424-8
Olayioye M. A., Neve R. M., Lane H. A., Hynes N. E. (2000). The ErbB signaling network: receptor heterodimerization in development and cancer. EMBO J. 19 (13), 3159–3167. doi:10.1093/emboj/19.13.3159
Omerovic J., Santangelo L., Puggioni E. M. R., Marrocco J., Dall’Armi C., Palumbo C., et al. (2007). The E3 ligase Aip4/Itch ubiquitinates and targets ErbB-4 for degradation. FASEB J. official Publ. Fed. Am. Soc. Exp. Biol. 21 (11), 2849–2862. doi:10.1096/fj.06-7925com
Oronsky B., Reid T. R., Larson C., Caroen S., Quinn M., Burbano E., et al. (2019). REPLATINUM Phase III randomized study: RRx-001 + platinum doublet versus platinum doublet in third-line small cell lung cancer. Future Oncol. Lond. Engl. 15 (30), 3427–3433. doi:10.2217/fon-2019-0317
Owen K. L., Brockwell N. K., Parker B. S. (2019). JAK-STAT signaling: a double-edged sword of immune regulation and cancer progression. Cancers 11 (12), 2002. doi:10.3390/cancers11122002
Park J. W. (1988). Reaction of S-nitrosoglutathione with sulfhydryl groups in protein. Biochem. Biophysical Res. Commun. 152 (2), 916–920. doi:10.1016/s0006-291x(88)80127-x
Pervin S., Singh R., Hernandez E., Wu G., Chaudhuri G. (2007). Nitric oxide in physiologic concentrations targets the translational machinery to increase the proliferation of human breast cancer cells: involvement of mammalian target of rapamycin/eIF4E pathway. Cancer Res. 67 (1), 289–299. doi:10.1158/0008-5472.CAN-05-4623
Plowman G. D., Culouscou J. M., Whitney G. S., Green J. M., Carlton G. W., Foy L., et al. (1993). Ligand-specific activation of HER4/p180erbB4, a fourth member of the epidermal growth factor receptor family. Proc. Natl. Acad. Sci. 90 (5), 1746–1750. doi:10.1073/pnas.90.5.1746
Plowman G. D., Whitney G. S., Neubauer M. G., Green J. M., McDonald V. L., Todaro G. J., et al. (1990). Molecular cloning and expression of an additional epidermal growth factor receptor-related gene. Proc. Natl. Acad. Sci. 87 (13), 4905–4909. doi:10.1073/pnas.87.13.4905
Portier B. P., Minca E. C., Wang Z., Lanigan C., Gruver A. M., Downs-Kelly E., et al. (2013). HER4 expression status correlates with improved outcome in both neoadjuvant and adjuvant Trastuzumab treated invasive breast carcinoma. Oncotarget 4 (10), 1662–1672. doi:10.18632/oncotarget.1232
Prenzel N., Zwick E., Daub H., Leserer M., Abraham R., Wallasch C., et al. (1999). EGF receptor transactivation by G-protein-coupled receptors requires metalloproteinase cleavage of proHB-EGF. Nature 402 (6764), 884–888. doi:10.1038/47260
Prigent S. A., Gullick W. J. (1994). Identification of c-erbB-3 binding sites for phosphatidylinositol 3’-kinase and SHC using an EGF receptor/c-erbB-3 chimera. EMBO J. 13 (12), 2831–2841. doi:10.1002/j.1460-2075.1994.tb06577.x
Printsev I., Yen L., Sweeney C., Carraway K. L. (2014). Oligomerization of the Nrdp1 E3 ubiquitin ligase is necessary for efficient autoubiquitination but not ErbB3 ubiquitination. J. Biol. Chem. 289 (12), 8570–8578. doi:10.1074/jbc.M113.527036
Qu J., Liu G. H., Wu K., Han P., Wang P., Li J., et al. (2007). Nitric oxide destabilizes Pias3 and regulates sumoylation. PloS One 2 (10), e1085. doi:10.1371/journal.pone.0001085
Rajkumar T., Gooden C. S., Lemoine N. R., Gullick W. J., Goden C. S. (1993). Expression of the c-erbB-3 protein in gastrointestinal tract tumours determined by monoclonal antibody RTJ1. J. Pathology 170 (3), 271–278. doi:10.1002/path.1711700309
Rajkumar T., Stamp G. W., Hughes C. M., Gullick W. J. (1996). c-erbB3 protein expression in ovarian cancer. Clin. Mol. Pathol. 49 (4), M199–M202. doi:10.1136/mp.49.4.m199
Ramalingam S. S., Vansteenkiste J., Planchard D., Cho B. C., Gray J. E., Ohe Y., et al. (2020). Overall survival with osimertinib in untreated, EGFR-mutated advanced NSCLC. N. Engl. J. Med. 382 (1), 41–50. doi:10.1056/NEJMoa1913662
Rayego-Mateos S., Rodrigues-Díez R., Morgado-Pascual J. L., Rodrigues Díez R. R., Mas S., Lavoz C., et al. (2013). Connective tissue growth factor is a new ligand of epidermal growth factor receptor. J. Mol. Cell Biol. 5 (5), 323–335. doi:10.1093/jmcb/mjt030
Reid T., Oronsky B., Scicinski J., Scribner C. L., Knox S. J., Ning S., et al. (2015). Safety and activity of RRx-001 in patients with advanced cancer: a first-in-human, open-label, dose-escalation phase 1 study. Lancet Oncol. 16 (9), 1133–1142. doi:10.1016/S1470-2045(15)00089-3
Reid T. R., Abrouk N., Caroen S., Oronsky B., Stirn M., Larson C., et al. (2023). ROCKET: phase II randomized, active-controlled, multicenter trial to assess the safety and efficacy of RRx-001 + irinotecan vs single-agent regorafenib in third/fourth line colorectal cancer. Clin. Colorectal Cancer 22 (1), 92–99. doi:10.1016/j.clcc.2022.11.003
Reschke M., Mihic-Probst D., van der Horst E. H., Knyazev P., Wild P. J., Hutterer M., et al. (2008). HER3 is a determinant for poor prognosis in melanoma. Clin. Cancer Res. An Official J. Am. Assoc. Cancer Res. 14 (16), 5188–5197. doi:10.1158/1078-0432.CCR-08-0186
Reveneau S., Arnould L., Jolimoy G., Hilpert S., Lejeune P., Saint-Giorgio V., et al. (1999). Nitric oxide synthase in human breast cancer is associated with tumor grade, proliferation rate, and expression of progesterone receptors. Laboratory Investigation; a J. Tech. Methods Pathology 79 (10), 1215–1225.
Ricci A., Lanfrancone L., Chiari R., Belardo G., Pertica C., Natali P. G., et al. (1995). Analysis of protein-protein interactions involved in the activation of the Shc/Grb-2 pathway by the ErbB-2 kinase. Oncogene 11 (8), 1519–1529.
Rice P. L., Barrett B. S., Fritz J. M., Srebernak M. C., Kisley L. R., Malkinson A. M., et al. (2010). Regulation of cytokine-induced prostanoid and nitric oxide synthesis by extracellular signal–regulated kinase 1/2 in lung epithelial cells. Exp. Lung Res. 36 (9), 558–571. doi:10.3109/01902148.2010.491891
Ridnour L. A., Barasch K. M., Windhausen A. N., Dorsey T. H., Lizardo M. M., Yfantis H. G., et al. (2012). Nitric oxide synthase and breast cancer: role of TIMP-1 in NO-mediated Akt activation. PloS One 7 (9), e44081. doi:10.1371/journal.pone.0044081
Riese D. J., van Raaij T. M., Plowman G. D., Andrews G. C., Stern D. F. (1995). The cellular response to neuregulins is governed by complex interactions of the erbB receptor family. Mol. Cell. Biol. 15 (10), 5770–5776. doi:10.1128/mcb.15.10.5770
Rigas B. (2009). Phase I multiple-dose safety, pharmacokinetic and pharmacodynamic clinical study of nitric oxide releasing aspirin (NCX 4016). Clinical trial registration NCT00331786. clinicaltrials.gov. Available at: https://clinicaltrials.gov/study/NCT00331786 (Accessed January 1, 2024).
Rojas M., Yao S., Lin Y. Z. (1996). Controlling epidermal growth factor (EGF)-stimulated Ras activation in intact cells by a cell-permeable peptide mimicking phosphorylated EGF receptor. J. Biol. Chem. 271 (44), 27456–27461. doi:10.1074/jbc.271.44.27456
Rosell R., Carcereny E., Gervais R., Vergnenegre A., Massuti B., Felip E., et al. (2012). Erlotinib versus standard chemotherapy as first-line treatment for European patients with advanced EGFR mutation-positive non-small-cell lung cancer (EURTAC): a multicentre, open-label, randomised phase 3 trial. Lancet. Oncol. 13 (3), 239–246. doi:10.1016/S1470-2045(11)70393-X
Rotin D., Margolis B., Mohammadi M., Daly R. J., Daum G., Fischer E. H., et al. (1992). SH2 domains prevent tyrosine dephosphorylation of the EGF receptor: identification of Tyr992 as the high-affinity binding site for SH2 domains of phospholipase C gamma. EMBO J. 11 (2), 559–567. doi:10.1002/j.1460-2075.1992.tb05087.x
Rusch V., Klimstra D., Venkatraman E., Pisters P. W., Langenfeld J., Dmitrovsky E. (1997). Overexpression of the epidermal growth factor receptor and its ligand transforming growth factor alpha is frequent in resectable non-small cell lung cancer but does not predict tumor progression. Clin. Cancer Res. An Official J. Am. Assoc. Cancer Res. 3 (4), 515–522.
Saglam O., Xiong Y., Marchion D. C., Strosberg C., Wenham R. M., Johnson J. J., et al. (2017). ERBB4 expression in ovarian serous carcinoma resistant to platinum-based therapy. Cancer Control J. Moffitt Cancer Cent. 24 (1), 89–95. doi:10.1177/107327481702400115
Sakaguchi K., Okabayashi Y., Kido Y., Kimura S., Matsumura Y., Inushima K., et al. (1998). Shc phosphotyrosine-binding domain dominantly interacts with epidermal growth factor receptors and mediates Ras activation in intact cells. Mol. Endocrinol. 12 (4), 536–543. doi:10.1210/mend.12.4.0094
Sang J., Chen Y., Tao Y. (2011). Nitric oxide inhibits gastric cancer cell growth through the modulation of the Akt pathway. Mol. Med. Rep. 4 (6), 1163–1167. doi:10.3892/mmr.2011.535
Sato K., Shin M. S., Sakimura A., Zhou Y., Tanaka T., Kawanishi M., et al. (2013). Inverse correlation between Thr-669 and constitutive tyrosine phosphorylation in the asymmetric epidermal growth factor receptor dimer conformation. Cancer Sci. 104 (10), 1315–1322. doi:10.1111/cas.12225
Schechter A. L., Hung M. C., Vaidyanathan L., Weinberg R. A., Yang-Feng T. L., Francke U., et al. (1985). The neu gene: an erbB-homologous gene distinct from and unlinked to the gene encoding the EGF receptor. Sci. (New York, N.Y.) 229 (4717), 976–978. doi:10.1126/science.2992090
Schechter A. L., Stern D. F., Vaidyanathan L., Decker S. J., Drebin J. A., Greene M. I., et al. (1984). The neu oncogene: an erb-B-related gene encoding a 185,000-Mr tumour antigen. Nature 312 (5994), 513–516. doi:10.1038/312513a0
Sciacca M., Belgorosky D., Zambrano M., Gómez Escalante J. I., Roca F., Langle Y. V., et al. (2019). Inhibition of breast tumor growth by N(G)-nitro-l-arginine methyl ester (l-NAME) is accompanied by activation of fibroblasts. Nitric Oxide Biol. Chem. 93, 34–43. doi:10.1016/j.niox.2019.09.008
Seet B. T., Dikic I., Zhou M. M., Pawson T. (2006). Reading protein modifications with interaction domains. Nat. Rev. Mol. Cell Biol. 7 (7), 473–483. doi:10.1038/nrm1960
Segatto O., Lonardo F., Pierce J. H., Bottaro D. P., Di Fiore P. P. (1990). The role of autophosphorylation in modulation of erbB-2 transforming function. New Biol. 2 (2), 187–195.
Sen S., Kawahara B., Chaudhuri G. (2013). Mitochondrial-associated nitric oxide synthase activity inhibits cytochrome c oxidase: implications for breast cancer. Free Radic. Biol. Med. 57, 210–220. doi:10.1016/j.freeradbiomed.2012.10.545
Sequist L. V., Yang J. C. H., Yamamoto N., O’Byrne K., Hirsh V., Mok T., et al. (2023). Phase III study of afatinib or cisplatin plus pemetrexed in patients with metastatic lung adenocarcinoma with EGFR mutations. J. Clin. Oncol. Official J. Am. Soc. Clin. Oncol. 41 (16), 2869–2876. doi:10.1200/JCO.22.02547
Shing Y., Christofori G., Hanahan D., Ono Y., Sasada R., Igarashi K., et al. (1993). Betacellulin: a mitogen from pancreatic beta cell tumors. Sci. (New York, N.Y.) 259 (5101), 1604–1607. doi:10.1126/science.8456283
Shitara K., Bang Y. J., Iwasa S., Sugimoto N., Ryu M. H., Sakai D., et al. (2020). Trastuzumab deruxtecan in previously treated HER2-positive gastric cancer. N. Engl. J. Med. 382 (25), 2419–2430. doi:10.1056/NEJMoa2004413
Shoyab M., Plowman G. D., McDonald V. L., Bradley J. G., Todaro G. J. (1989). Structure and function of human amphiregulin: a member of the epidermal growth factor family. Science 243 (4894), 1074–1076. doi:10.1126/science.2466334
Siegel P. M., Ryan E. D., Cardiff R. D., Muller W. J. (1999). Elevated expression of activated forms of Neu/ErbB-2 and ErbB-3 are involved in the induction of mammary tumors in transgenic mice: implications for human breast cancer. EMBO J. 18 (8), 2149–2164. doi:10.1093/emboj/18.8.2149
Siemens D. R., Heaton J. P. W., Adams M. A., Kawakami J., Graham C. H. (2009). Phase II study of nitric oxide donor for men with increasing prostate-specific antigen level after surgery or radiotherapy for prostate cancer. Urology 74 (4), 878–883. doi:10.1016/j.urology.2009.03.004
Sierke S. L., Cheng K., Kim H. H., Koland J. G. (1997). Biochemical characterization of the protein tyrosine kinase homology domain of the ErbB3 (HER3) receptor protein. Biochem. J. 322 (3), 757–763. doi:10.1042/bj3220757
Simpson B. J., Weatherill J., Miller E. P., Lessells A. M., Langdon S. P., Miller W. R. (1995). c-erbB-3 protein expression in ovarian tumours. Br. J. Cancer 71 (4), 758–762. doi:10.1038/bjc.1995.147
Slamon D. J., Clark G. M., Wong S. G., Levin W. J., Ullrich A., McGuire W. L. (1987). Human breast cancer: correlation of relapse and survival with amplification of the HER-2/neu oncogene. Sci. (New York, N.Y.) 235 (4785), 177–182. doi:10.1126/science.3798106
Slamon D. J., Godolphin W., Jones L. A., Holt J. A., Wong S. G., Keith D. E., et al. (1989). Studies of the HER-2/neu proto-oncogene in human breast and ovarian cancer. Sci. (New York, N.Y.) 244 (4905), 707–712. doi:10.1126/science.2470152
Slesak B., Harlozinska A., Porebska I., Bojarowski T., Lapinska J., Rzeszutko M., et al. (1998). Expression of epidermal growth factor receptor family proteins (EGFR, c-erbB-2 and c-erbB-3) in gastric cancer and chronic gastritis. Anticancer Res. 18 (4A), 2727–2732.
Sliwkowski M. X. (2003). Ready to partner. Nat. Struct. Biol. 10 (3), 158–159. doi:10.1038/nsb0303-158
Smeda M., Kieronska A., Adamski M. G., Proniewski B., Sternak M., Mohaissen T., et al. (2018). Nitric oxide deficiency and endothelial-mesenchymal transition of pulmonary endothelium in the progression of 4T1 metastatic breast cancer in mice. Breast cancer Res. BCR 20 (1), 86. doi:10.1186/s13058-018-1013-z
Socco S., Bovee R. C., Palczewski M. B., Hickok J. R., Thomas D. D. (2017). Epigenetics: the third pillar of nitric oxide signaling. Pharmacol. Res. 121, 52–58. doi:10.1016/j.phrs.2017.04.011
Solca F., Dahl G., Zoephel A., Bader G., Sanderson M., Klein C., et al. (2012). Target binding properties and cellular activity of afatinib (BIBW 2992), an irreversible ErbB family blocker. J. Pharmacol. Exp. Ther. 343 (2), 342–350. doi:10.1124/jpet.112.197756
Soltoff S. P., Cantley L. C. (1996). p120cbl is a cytosolic adapter protein that associates with phosphoinositide 3-kinase in response to epidermal growth factor in PC12 and other cells. J. Biol. Chem. 271 (1), 563–567. doi:10.1074/jbc.271.1.563
Song H., Li C. W., Labaff A. M., Lim S. O., Li L. Y., Kan S. F., et al. (2011). Acetylation of EGF receptor contributes to tumor cell resistance to histone deacetylase inhibitors. Biochem. Biophysical Res. Commun. 404 (1), 68–73. doi:10.1016/j.bbrc.2010.11.064
Song J. M., Upadhyaya P., Kassie F. (2018). Nitric oxide-donating aspirin (NO-Aspirin) suppresses lung tumorigenesis in vitro and in vivo and these effects are associated with modulation of the EGFR signaling pathway. Carcinogenesis 39 (7), 911–920. doi:10.1093/carcin/bgy049
Songyang Z., Margolis B., Chaudhuri M., Shoelson S. E., Cantley L. C. (1995). The phosphotyrosine interaction domain of SHC recognizes tyrosine-phosphorylated NPXY motif. J. Biol. Chem. 270 (25), 14863–14866. doi:10.1074/jbc.270.25.14863
Stephens J. M., Morrison R. F., Pilch P. F. (1996). The expression and regulation of STATs during 3T3-L1 adipocyte differentiation. J. Biol. Chem. 271 (18), 10441–10444. doi:10.1074/jbc.271.18.10441
Stewart G. D., Nanda J., Brown D. J. G., Riddick A. C. P., Ross J. A., Habib F. K. (2009). NO-sulindac inhibits the hypoxia response of PC-3 prostate cancer cells via the Akt signalling pathway. Int. J. Cancer 124 (1), 223–232. doi:10.1002/ijc.23934
Strachan L., Murison J. G., Prestidge R. L., Sleeman M. A., Watson J. D., Kumble K. D. (2001). Cloning and biological activity of epigen, a novel member of the epidermal growth factor superfamily. J. Biol. Chem. 276 (21), 18265–18271. doi:10.1074/jbc.M006935200
Sun T., Aceto N., Meerbrey K. L., Kessler J. D., Zhou C., Migliaccio I., et al. (2011). Activation of multiple proto-oncogenic tyrosine kinases in breast cancer via loss of the PTPN12 phosphatase. Cell 144 (5), 703–718. doi:10.1016/j.cell.2011.02.003
Sundvall M., Iljin K., Kilpinen S., Sara H., Kallioniemi O. P., Elenius K. (2008). Role of ErbB4 in breast cancer. J. Mammary Gl. Biol. Neoplasia 13 (2), 259–268. doi:10.1007/s10911-008-9079-3
Swain S. M., Miles D., Kim S. B., Im Y. H., Im S. A., Semiglazov V., et al. (2020). Pertuzumab, trastuzumab, and docetaxel for HER2-positive metastatic breast cancer (CLEOPATRA): end-of-study results from a double-blind, randomised, placebo-controlled, phase 3 study. Lancet. Oncol. 21 (4), 519–530. doi:10.1016/S1470-2045(19)30863-0
Switzer C. H., Glynn S. A., Cheng R. Y. S., Ridnour L. A., Green J. E., Ambs S., et al. (2012). S-nitrosylation of EGFR and Src activates an oncogenic signaling network in human basal-like breast cancer. Mol. cancer Res. MCR 10 (9), 1203–1215. doi:10.1158/1541-7786.MCR-12-0124
The Methodist Hospital Research Institute (2023). Phase II trial of alpelisib with iNOS inhibitor and nab-paclitaxel in patients with HER2 negative metastatic or locally advanced metaplastic breast cancer (MpBC). Clinical trial registration NCT05660083. clinicaltrials.gov. Available at: https://clinicaltrials.gov/study/NCT05660083 (Accessed January 1, 2023).
Thomas D. D., Ridnour L. A., Isenberg J. S., Flores-Santana W., Switzer C. H., Donzelli S., et al. (2008). The chemical biology of nitric oxide. Implications in cellular signaling. Free Radic. Biol. Med. 45 (1), 18–31. doi:10.1016/j.freeradbiomed.2008.03.020
Tidcombe H., Jackson-Fisher A., Mathers K., Stern D. F., Gassmann M., Golding J. P. (2003). Neural and mammary gland defects in ErbB4 knockout mice genetically rescued from embryonic lethality. Proc. Natl. Acad. Sci. U. S. A. 100 (14), 8281–8286. doi:10.1073/pnas.1436402100
Tovey S. M., Witton C. J., Bartlett J. M. S., Stanton P. D., Reeves J. R., Cooke T. G. (2004). Outcome and human epidermal growth factor receptor (HER) 1-4 status in invasive breast carcinomas with proliferation indices evaluated by bromodeoxyuridine labelling. Breast Cancer Res. 6 (3), R246–R251. doi:10.1186/bcr783
Toyoda H., Komurasaki T., Uchida D., Takayama Y., Isobe T., Okuyama T., et al. (1995). Epiregulin. A novel epidermal growth factor with mitogenic activity for rat primary hepatocytes. J. Biol. Chem. 270 (13), 7495–7500. doi:10.1074/jbc.270.13.7495
TRIUMPH Investigators Alexander J. H., Reynolds H. R., Stebbins A. L., Dzavik V., Harrington R. A., Van de Werf F., et al. (2007). Effect of tilarginine acetate in patients with acute myocardial infarction and cardiogenic shock: the TRIUMPH randomized controlled trial. JAMA 297 (15), 1657–1666. doi:10.1001/jama.297.15.joc70035
Tzahar E., Waterman H., Chen X., Levkowitz G., Karunagaran D., Lavi S., et al. (1996). A hierarchical network of interreceptor interactions determines signal transduction by Neu differentiation factor/neuregulin and epidermal growth factor. Mol. Cell. Biol. 16 (10), 5276–5287. doi:10.1128/MCB.16.10.5276
Ubersax J. A., Ferrell J. E. (2007). Mechanisms of specificity in protein phosphorylation. Nat. Rev. Mol. Cell Biol. 8 (7), 530–541. doi:10.1038/nrm2203
Ueda S., Ogata S., Tsuda H., Kawarabayashi N., Kimura M., Sugiura Y., et al. (2004). The correlation between cytoplasmic overexpression of epidermal growth factor receptor and tumor aggressiveness: poor prognosis in patients with pancreatic ductal adenocarcinoma. Pancreas 29 (1), e1–e8. doi:10.1097/00006676-200407000-00061
Ullrich A., Coussens L., Hayflick J. S., Dull T. J., Gray A., Tam A. W., et al. (1984). Human epidermal growth factor receptor cDNA sequence and aberrant expression of the amplified gene in A431 epidermoid carcinoma cells. Nature 309 (5967), 418–425. doi:10.1038/309418a0
Van de Wouwer M., André S., Gabius H. J., Villalobo A. (2011). Nitric oxide changes distinct aspects of the glycophenotype of human neuroblastoma NB69 cells. Nitric Oxide Biol. Chem. 24 (2), 91–101. doi:10.1016/j.niox.2010.12.007
Vannini F., Kashfi K., Nath N. (2015). The dual role of iNOS in cancer. Redox Biol. 6, 334–343. doi:10.1016/j.redox.2015.08.009
Verma S., Miles D., Gianni L., Krop I. E., Welslau M., Baselga J., et al. (2012). Trastuzumab emtansine for HER2-positive advanced breast cancer. N. Engl. J. Med. 367 (19), 1783–1791. doi:10.1056/NEJMoa1209124
Villegas S. N., Gombos R., García-López L., Gutiérrez-Pérez I., García-Castillo J., Vallejo D. M., et al. (2018). PI3K/Akt cooperates with oncogenic notch by inducing nitric oxide-dependent inflammation. Cell Rep. 22 (10), 2541–2549. doi:10.1016/j.celrep.2018.02.049
von Lintig F. C., Dreilinger A. D., Varki N. M., Wallace A. M., Casteel D. E., Boss G. R. (2000). Ras activation in human breast cancer. Breast Cancer Res. Treat. 62 (1), 51–62. doi:10.1023/a:1006491619920
von Minckwitz G., Huang C. S., Mano M. S., Loibl S., Mamounas E. P., Untch M., et al. (2019). Trastuzumab emtansine for residual invasive HER2-positive breast cancer. N. Engl. J. Med. 380 (7), 617–628. doi:10.1056/NEJMoa1814017
Wada T., Qian X. L., Greene M. I. (1990). Intermolecular association of the p185neu protein and EGF receptor modulates EGF receptor function. Cell 61 (7), 1339–1347. doi:10.1016/0092-8674(90)90697-d
Wahl M. I., Nishibe S., Kim J. W., Kim H., Rhee S. G., Carpenter G. (1990). Identification of two epidermal growth factor-sensitive tyrosine phosphorylation sites of phospholipase C-gamma in intact HSC-1 cells. J. Biol. Chem. 265 (7), 3944–3948. doi:10.1016/s0021-9258(19)39685-1
Walsh C. T., Garneau-Tsodikova S., Gatto G. J. (2005). Protein posttranslational modifications: the chemistry of proteome diversifications. Angew. Chem. Int. Ed. 44 (45), 7342–7372. doi:10.1002/anie.200501023
Walton G. M., Chen W. S., Rosenfeld M. G., Gill G. N. (1990). Analysis of deletions of the carboxyl terminus of the epidermal growth factor receptor reveals self-phosphorylation at tyrosine 992 and enhanced in vivo tyrosine phosphorylation of cell substrates. J. Biol. Chem. 265 (3), 1750–1754. doi:10.1016/S0021-9258(19)40080-X
Wang D., Liu D., Yuchi J., He F., Jiang Y., Cai S., et al. (2020). MusiteDeep: a deep-learning based webserver for protein post-translational modification site prediction and visualization. Nucleic Acids Res. 48 (W1), W140–W146. doi:10.1093/nar/gkaa275
Wang H.-M., Xu Y. F., Ning S. L., Yang D. X., Li Y., Du Y. J., et al. (2014). The catalytic region and PEST domain of PTPN18 distinctly regulate the HER2 phosphorylation and ubiquitination barcodes. Cell Res. 24 (9), 1067–1090. doi:10.1038/cr.2014.99
Wang J., He P., Gaida M., Yang S., Schetter A. J., Gaedcke J., et al. (2016). Inducible nitric oxide synthase enhances disease aggressiveness in pancreatic cancer. Oncotarget 7 (33), 52993–53004. doi:10.18632/oncotarget.10323
Waterman H., Katz M., Rubin C., Shtiegman K., Lavi S., Elson A., et al. (2002). A mutant EGF-receptor defective in ubiquitylation and endocytosis unveils a role for Grb2 in negative signaling. EMBO J. 21 (3), 303–313. doi:10.1093/emboj/21.3.303
Waterman H., Sabanai I., Geiger B., Yarden Y. (1998). Alternative intracellular routing of ErbB receptors may determine signaling potency. J. Biol. Chem. 273 (22), 13819–13827. doi:10.1074/jbc.273.22.13819
Wikstrand C. J., Reist C. J., Archer G. E., Zalutsky M. R., Bigner D. D. (1998). The class III variant of the epidermal growth factor receptor (EGFRvIII): characterization and utilization as an immunotherapeutic target. J. Neurovirology 4 (2), 148–158. doi:10.3109/13550289809114515
Wong A. J., Ruppert J. M., Bigner S. H., Grzeschik C. H., Humphrey P. A., Bigner D. S., et al. (1992). Structural alterations of the epidermal growth factor receptor gene in human gliomas. Proc. Natl. Acad. Sci. U. S. A. 89 (7), 2965–2969. doi:10.1073/pnas.89.7.2965
Wu Y.-L., Cheng Y., Zhou X., Lee K. H., Nakagawa K., Niho S., et al. (2017). Dacomitinib versus gefitinib as first-line treatment for patients with EGFR-mutation-positive non-small-cell lung cancer (ARCHER 1050): a randomised, open-label, phase 3 trial. Lancet. Oncol. 18 (11), 1454–1466. doi:10.1016/S1470-2045(17)30608-3
Xie H., Pallero M. A., Gupta K., Chang P., Ware M. F., Witke W., et al. (1998). EGF receptor regulation of cell motility: EGF induces disassembly of focal adhesions independently of the motility-associated PLCgamma signaling pathway. J. Cell Sci. 111 (5), 615–624. doi:10.1242/jcs.111.5.615
Xie W., Paterson A. J., Chin E., Nabell L. M., Kudlow J. E. (1997). Targeted expression of a dominant negative epidermal growth factor receptor in the mammary gland of transgenic mice inhibits pubertal mammary duct development. Mol. Endocrinol. 11 (12), 1766–1781. doi:10.1210/mend.11.12.0019
Yamamoto T., Ebisuya M., Ashida F., Okamoto K., Yonehara S., Nishida E. (2006). Continuous ERK activation downregulates antiproliferative genes throughout G1 phase to allow cell-cycle progression. Curr. Biol. CB 16 (12), 1171–1182. doi:10.1016/j.cub.2006.04.044
Yamauchi T., Ueki K., Tobe K., Tamemoto H., Sekine N., Wada M., et al. (1997). Tyrosine phosphorylation of the EGF receptor by the kinase Jak2 is induced by growth hormone. Nature 390 (6655), 91–96. doi:10.1038/36369
Yao X., Wu Y., Zhu M., Qian H., Chen Y. (2015). Nitric oxide/cyclic guanosine monophosphate inducers sodium nitroprusside and L-arginine inhibit the proliferation of gastric cancer cells via the activation of type II cyclic guanosine monophosphate-dependent protein kinase. Oncol. Lett. 10 (1), 479–484. doi:10.3892/ol.2015.3229
Yarden Y., Sliwkowski M. X. (2001). Untangling the ErbB signalling network. Nat. Rev. Mol. Cell Biol. 2 (2), 127–137. doi:10.1038/35052073
Yi E. S., Harclerode D., Gondo M., Stephenson M., Brown R. W., Younes M., et al. (1997). High c-erbB-3 protein expression is associated with shorter survival in advanced non-small cell lung carcinomas. Mod. Pathology An Official J. U. S. Can. Acad. Pathology, Inc 10 (2), 142–148.
Yoo Y.-M., Jung E. M., Ahn C., Jeung E. B. (2018). Nitric oxide prevents H2O2-induced apoptosis in SK-N-MC human neuroblastoma cells. Int. J. Biol. Sci. 14 (14), 1974–1984. doi:10.7150/ijbs.28050
Yoshikawa T., Phan K. Q., Tagawa H., Sasaki K., Feng H., Kishimura A., et al. (2020). Modification of nitric oxide donors onto a monoclonal antibody boosts accumulation in solid tumors. Int. J. Pharm. 583, 119352. doi:10.1016/j.ijpharm.2020.119352
Yoshioka H., Shimokawa M., Seto T., Morita S., Yatabe Y., Okamoto I., et al. (2019). Final overall survival results of WJTOG3405, a randomized phase III trial comparing gefitinib versus cisplatin with docetaxel as the first-line treatment for patients with stage IIIB/IV or postoperative recurrent EGFR mutation-positive non-small-cell lung cancer. Ann. Oncol. Official J. Eur. Soc. Med. Oncol. 30 (12), 1978–1984. doi:10.1093/annonc/mdz399
Yoshioka Y., Suzuki T., Matsuo Y., Tsurita G., Watanabe T., Dohmae N., et al. (2017). Protein lysine methyltransferase SMYD3 is involved in tumorigenesis through regulation of HER2 homodimerization. Cancer Med. 6 (7), 1665–1672. doi:10.1002/cam4.1099
Zaraei S.-O., Sbenati R. M., Alach N. N., Anbar H. S., El-Gamal R., Tarazi H., et al. (2021). Discovery of first-in-class imidazothiazole-based potent and selective ErbB4 (HER4) kinase inhibitors. Eur. J. Med. Chem. 224, 113674. doi:10.1016/j.ejmech.2021.113674
Zhang D., Sliwkowski M. X., Mark M., Frantz G., Akita R., Sun Y., et al. (1997). Neuregulin-3 (NRG3): a novel neural tissue-enriched protein that binds and activates ErbB4. Proc. Natl. Acad. Sci. U. S. A. 94 (18), 9562–9567. doi:10.1073/pnas.94.18.9562
Zhang J., Cao J., Li J., Zhang Y., Chen Z., Peng W., et al. (2014). A phase I study of AST1306, a novel irreversible EGFR and HER2 kinase inhibitor, in patients with advanced solid tumors. J. Hematol. Oncol. 7, 22. doi:10.1186/1756-8722-7-22
Zhang J., Saba N. F., Chen G. Z., Shin D. M. (2015). Targeting HER (ERBB) signaling in head and neck cancer: an essential update. Mol. Aspects Med. 45, 74–86. doi:10.1016/j.mam.2015.07.001
Zhang Y., Zhang L., Li R., Chang D. W., Ye Y., Minna J. D., et al. (2017). Genetic variations in cancer-related significantly mutated genes and lung cancer susceptibility. Ann. Oncol. Official J. Eur. Soc. Med. Oncol. 28 (7), 1625–1630. doi:10.1093/annonc/mdx161
Zhou C., Qiu L., Sun Y., Healey S., Wanebo H., Kouttab N., et al. (2006). Inhibition of EGFR/PI3K/AKT cell survival pathway promotes TSA’s effect on cell death and migration in human ovarian cancer cells. Int. J. Oncol. 29 (1), 269–278. doi:10.3892/ijo.29.1.269
Zhu L., Zhang C., Liu Q. (2019). PTEN S-nitrosylation by NOS1 inhibits autophagy in NPC cells. Cell Death Dis. 10 (4), 306–313. doi:10.1038/s41419-019-1542-0
Zrihan-Licht S., Deng B., Yarden Y., McShan G., Keydar I., Avraham H. (1998). Csk homologous kinase, a novel signaling molecule, directly associates with the activated ErbB-2 receptor in breast cancer cells and inhibits their proliferation. J. Biol. Chem. 273 (7), 4065–4072. doi:10.1074/jbc.273.7.4065
Keywords: nitric oxide, cancer, human epidermal growth factor receptor 2, epidermal growth factor receptor, post-translational modification, nitrosation
Citation: O’Neill CE, Sun K, Sundararaman S, Chang JC and Glynn SA (2024) The impact of nitric oxide on HER family post-translational modification and downstream signaling in cancer. Front. Physiol. 15:1358850. doi: 10.3389/fphys.2024.1358850
Received: 20 December 2023; Accepted: 16 February 2024;
Published: 27 March 2024.
Edited by:
Erika M. Palmieri, National Cancer Institute at Frederick (NIH), United StatesReviewed by:
Wei Shi, University of North Carolina at Chapel Hill, United StatesGuowei Yin, Columbia University, United States
Linda Julian, Yale University, United States
Copyright © 2024 O’Neill, Sun, Sundararaman, Chang and Glynn. This is an open-access article distributed under the terms of the Creative Commons Attribution License (CC BY). The use, distribution or reproduction in other forums is permitted, provided the original author(s) and the copyright owner(s) are credited and that the original publication in this journal is cited, in accordance with accepted academic practice. No use, distribution or reproduction is permitted which does not comply with these terms.
*Correspondence: Sharon A. Glynn, U2hhcm9uLkdseW5uQHVuaXZlcnNpdHlvZmdhbHdheS5pZQ==