- 1Department of Physiology and Biophysics, The State University of New York: The University at Buffalo, Buffalo, NY, United States
- 2Department of Ophthalmology, The State University of New York: The University at Buffalo, Buffalo, NY, United States
SLC4A11 is the most abundant membrane transport protein in corneal endothelial cells. Its functional presence is necessary to support the endothelial fluid pump that draws fluid from the corneal stroma, preventing corneal edema. Several molecular actions have been proposed for SLC4A11 including H2O transport and cell adhesion. One of the most reproduced actions that SLC4A11 mediates is a H+ (or OH−) conductance that is enhanced in the presence of NH4Cl. The mechanism by which this occurs is controversial with some providing evidence in favor of NH3-H+ cotransport and others providing evidence for uncoupled H+ transport that is indirectly stimulated by the effects of NH4Cl upon intracellular pH and membrane potential. In the present study we provide new evidence and revisit previous studies, to support a model in which NH4Cl causes direct allosteric activation of SLC4A11 by means of an acidic shift in the intracellular pK (pKi) that governs the relationship between intracellular pH (pHi) and SLC4A11 H+-conductance. These findings have important implications for the assignment of a physiological role for SLC4A11.
1 Introduction
The members of the SLC4 family of solute carrier proteins are mainly Na+-independent and Na+-dependent Cl−/HCO3− exchangers or Na+-coupled HCO3− (or CO3=) transporters (Parker and Boron, 2013; Lee et al., 2022). SLC4A11 (originally named “BTR1”: Bicarbonate Transporter Related Protein 1) was the last member of the SLC4 family to be cloned (Parker et al., 2001) and is the only member of the family that does not transport HCO3−/CO3= (Jalimarada et al., 2013; Ogando et al., 2013; Loganathan et al., 2016). Instead, SLC4A11 influences intracellular pH (pHi) by conducting H+, or its thermodynamic equivalent OH− (Kao et al., 2015; Myers et al., 2016). For convenience hereafter, and in the absence of any definitive data in favor of one substrate over the other, we will assume that H+ are the transported species. SLC4A11 is expressed at low levels in many tissues, but appears to have most functional impact in the cornea and inner ear as evidenced by the corneal dystrophies and progressive hearing loss that are caused by SLC4A11 mutations in both humans and mice (Vithana et al., 2006; 2008; Desir et al., 2007; Lopez et al., 2009; Gröger et al., 2010; Han et al., 2013).
Most studies of SLC4A11 have focused on its role in the cornea. Here, SLC4A11 is expressed in the basolateral membrane of the corneal endothelial cells that line the posterior (aqueous-humor facing side) of the cornea (Vilas et al., 2013). These cells are responsible for pumping fluid from the corneal stroma to prevent it from swelling and losing its ability to optimally transmit and refract light (Hodson, 1977). SLC4A11 is clearly valuable for endothelial pumping because autosomal recessive inheritance of SLC4A11 mutations cause congenital hereditary endothelial dystrophy (CHED), a non-progressive corneal thickening and opacification (Vithana et al., 2006). Furthermore, an apparently discrete set of SLC4A11 mutations result in autosomal dominant inheritance of a late-onset form of Fuchs endothelial corneal dystrophy (FECD4), which typically manifests from the 4th decade of life, preceded by the appearance of excrescences from the Descemet’s membrane that underlie the endothelial cells, which are known as guttae (Weiss et al., 2024). However, the mechanism by which SLC4A11 loss compromises the endothelial pump remains elusive because there is little consensus about SLC4A11’s molecular action.
The earliest proposal for SLC4A11 action was that it, like plantal Slc4-like proteins, performed borate transport: specifically electrogenic 2Na+-B(OH)4– cotransport (Frommer and von Wirén, 2002; Park et al., 2004). This hypothesis fell out of favor due to an inability of others to find evidence for this mode of action with mammalian SLC4A11 (Jalimarada et al., 2013; Ogando et al., 2013; Vilas et al., 2013; Kao et al., 2015; Loganathan et al., 2016). That original characterization study also attributed an EIPA-insensitive Na+/H+ exchanger-like activity to SLC4A11, but neither the EIPA-sensitivity nor the Na+-dependence of this H+ transport activity has proven to be universally repeatable, leaving open the possibility that such results were influenced by endogenous activities (Park et al., 2004; Ogando et al., 2013; Kao et al., 2015; 2016; Zhang et al., 2015; Myers et al., 2016). Other reported SLC4A11 transport modes include a H2O permeability that is disturbed by disease-causing mutations (Vilas et al., 2013), a role in extracellular matrix adhesion (Malhotra et al., 2020), and a conditional presence in mitochondria (Ogando et al., 2019). Any of these, alone or in combination, remain a feasible explanation for loss of endothelial pump function with SLC4A11 mutation. However, at present, none of these features has been investigated by more than one group.
In this study, we focus on the robust and repeatable Na+-independent H+ transport action of SLC4A11, an action that is stimulated by increases in pHi, extracellular pH (pHe), and NH4Cl (Zhang et al., 2015; Myers et al., 2016; Kao et al., 2020; Quade et al., 2020) and which is also disrupted by disease-causing mutations (Kao et al., 2015; Quade et al., 2022). However, there remains a controversy over whether the NH4Cl-stimulated action of SLC4A11 represents SLC4A11-mediated NH3-H+ cotransport (Zhang et al., 2015; Kao et al., 2020) or an indirect stimulation of SLC4A11-mediated H+ conductance caused by cellular depolarization and sub-membranous alkalinization of pHi due to NH3 movement across the lipid bilayer (Myers et al., 2016). A recent attempt to distinguish NH3-H+ cotransport from NH4+ or H+ transport using the Goldman-Hodgkin-Katz approach led to the conclusion that SLC4A11 operates in competing modes of NH3-H+ cotransport or unaccompanied H+ transport, with the inference that the presence of NH3/NH4+ inhibits unaccompanied H+ conduction (Kao et al., 2020). These are important distinctions as they inform the predicted direction of transport, the interpretation of SLC4A11 structure, and the ultimately the physiological role of SLC4A11. In our previous work, we demonstrated that SLC4A11-mediated H+ transport is governed by an intracellular pK (pKi), the value of which can be modulated by changes in pHe and by disease-causing mutations (Quade et al., 2020; Quade et al., 2022). At pHe = 7.50, pKi is too alkaline to be determined, but can be no more acidic than 7.6 (Quade et al., 2020). However, when we raise pHe, pKi is shifted into measurable range. For example, pKi for human SLC4A11 ∼ 7.04 when pHe = 8.50 (Quade et al., 2022). This acidic-shift in pKi manifests as a rise in SLC4A11 current. We note that no study of SLC4A11 activity has directly measured transmembrane NH3/NH4+ movement, relying instead on proxies such as pH and voltage changes. With that as context, here we revisit the phenomenon of NH4Cl-stimulation of SLC4A11-mediated H+ currents to determine whether it could be explained by a direct allosteric effect of NH3/NH4+ upon SLC4A11 pKi.
2 Results
2.1 Determining pKi for SLC4A11 at pHe = 8.50
In a previous study we determined that pKi for human SLC4A11 at pHe = 8.50 is 7.04 ± 0.01 (Quade et al., 2022). For this study we generated a contemporary set of control data to confirm the pKi of human SLC4A11 at pHe = 8.50 in new experimental hands. As we have previously reported, SLC4A11-expressing oocytes slowly alkalinize upon exposure to pHe = 8.50 solution, and the rate of alkalinization can be enhanced by clamping the membrane potential (Vm) at a value more positive than the predicted reversal potential for H+ (EH). An example of this phenomenon can see seen in the first figure of Quade et al. (2022). As pHi rises, we gather a series of I-V plots such that each I-V plot can be assigned to a value of pHi. Figure 1A shows a selection of these plots gathered from a single SLC4A11-expressing oocyte as pHi is caused to rise under voltage-clamp. Note that the slope of the I-V relationship (i.e., membrane conductance, Gm) rises as pHi increases. The relationship between pHi and Gm is shown for six SLC4A11-expressing cells in Figure 1B, in which the trace marked by black diamonds is the full data set from the cell shown in Figure 1A. The average Gm.max was 90 ± 14 µS. Also shown in Figure 1B are resting pHi/Gm relationships from six H2O-injected cells (crosses) for which the average Gm was 2 ± 1 µS. In order to extract pKi values from the SLC4A11 data, we first normalize Gm data from each cell to its own Gm,max (Figure 1C) and fit those data to the Hill equation, generating the best-fit relationships described by a pKi and an apparent Hill coefficient (Napp) shown as gray lines in Figure 1D. The average of these relationships is represented as a black dotted-line in Figure 1. We calculate an average pKi of 7.08 ± 0.04 for human SLC4A11 at pHe = 8.50, which is not different from the range that we had previously determined in Quade et al. (2022) (p = 0.32, two-tailed, unpaired t-test).
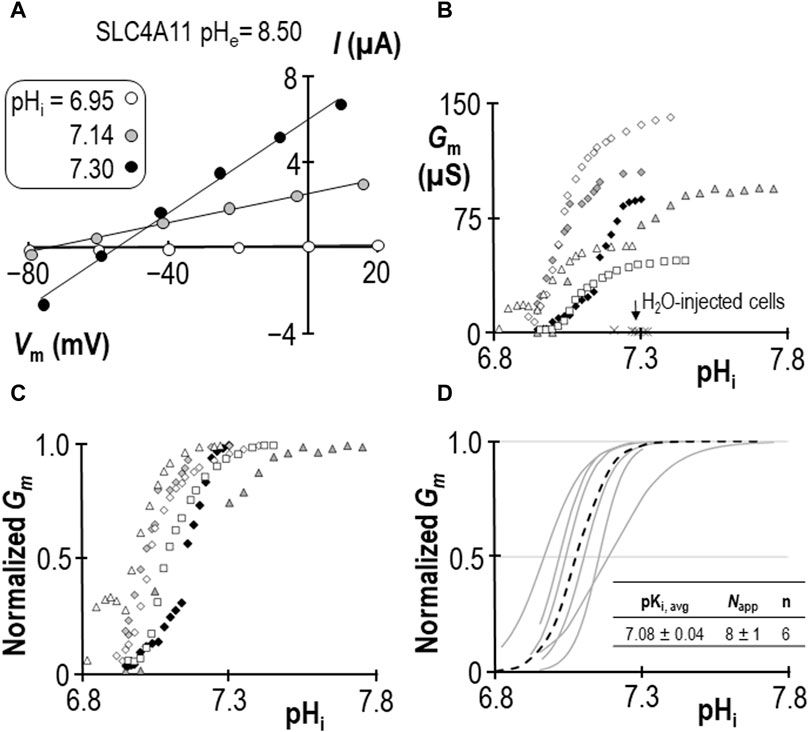
Figure 1. SLC4A11 behavior in the absence of NH4Cl (extracellular pH = 8.50) (A) A representative selection of current-voltage (I-V) relationships gathered from a single SLC4A11-expressing oocyte as intracellular pH (pHi) rises. (B) The relationship between pHi and slope conductance (Gm) from the full-set of I-V relationships gathered from the SLC4A11-expressing cell shown in panel (A) (black diamonds) and from five other SLC4A11-expressing cells (each represented by its own symbol). The pHi versus Gm relationship at resting pHi for five H2O-injected oocytes is represented by crosses. (C) SLC4A11 data from panel (B), normalized to its respective maximum Gm (Gm,max). (D) Best-fit lines for each cell to the Hill equation are shown in gray. The dashed black line represents the Hill equation generated using the average pKi and apparent Hill coefficients (Napp) of the n = 6 replicates, as shown in the inset table.
2.2 Determining pKi for SLC4A11 at pHe = 8.50 in the presence of 1 mM NH4Cl
The response of SLC4A11-expressing oocytes in pHe = 8.50 solution was altered in two ways in the presence of 1 mM NH4Cl. First, as shown in the example in Figure 2A, the cell acidified rather than alkalinized (initial dpHi/dt = 5.1 ± 0.9 × 10−4 pH units/s, n = 6). This was also a feature of H2O-injected cells (initial dpHi/dt = 5.5 ± 0.8 × 10−4 pH units/s, n = 6, Figure 2A inset). Second, unique to SLC4A11-expressing cells, Gm unexpectedly rose to a plateau (over period “a”) and subsequently declined to a value close to its starting value (over period “b”) when pHi was further acidified by clamping Vm at a value more negative than EH. Let us first focus our attention on period “b,” during which acidification causes a familiar decline. Figure 2B shows a selection of responses from the cell represented in Figure 2A. The pHi versus Gm relationships gathered from seven SLC4A11-expressing cells during period “b” are plotted in Figure 2C. The average Gm.max was 89 ± 5 µS, which is not different from that for the group of cells assayed in the absence of NH4Cl (p = 0.94, two-tailed unpaired t-test). Also shown in Figure 2C are resting pHi/Gm relationships from six control cells (originally injected with H2O in place of SLC4A11 cRNA) that were acidified prior to assay by HCl injection (crosses). The average Gm of these cells was 11 ± 2 µS. Best-fit normalized Gm versus pHi data for SLC4A11-expressing cells is shown in Figure 2D. We calculate that the average pKi for SLC4A11-expressing cells at pHe = 8.50 in the presence of 1 mM NH4Cl is 6.28 ± 0.05, which is significantly more acidic than the pKi range determined in the absence of NH4Cl (p < 0.001, one-tailed, unpaired t-test). On the other hand, there was no significant difference in the value of Napp compared to its value in the absence of NH4Cl (P = 0.17, two-tailed unpaired t-test).
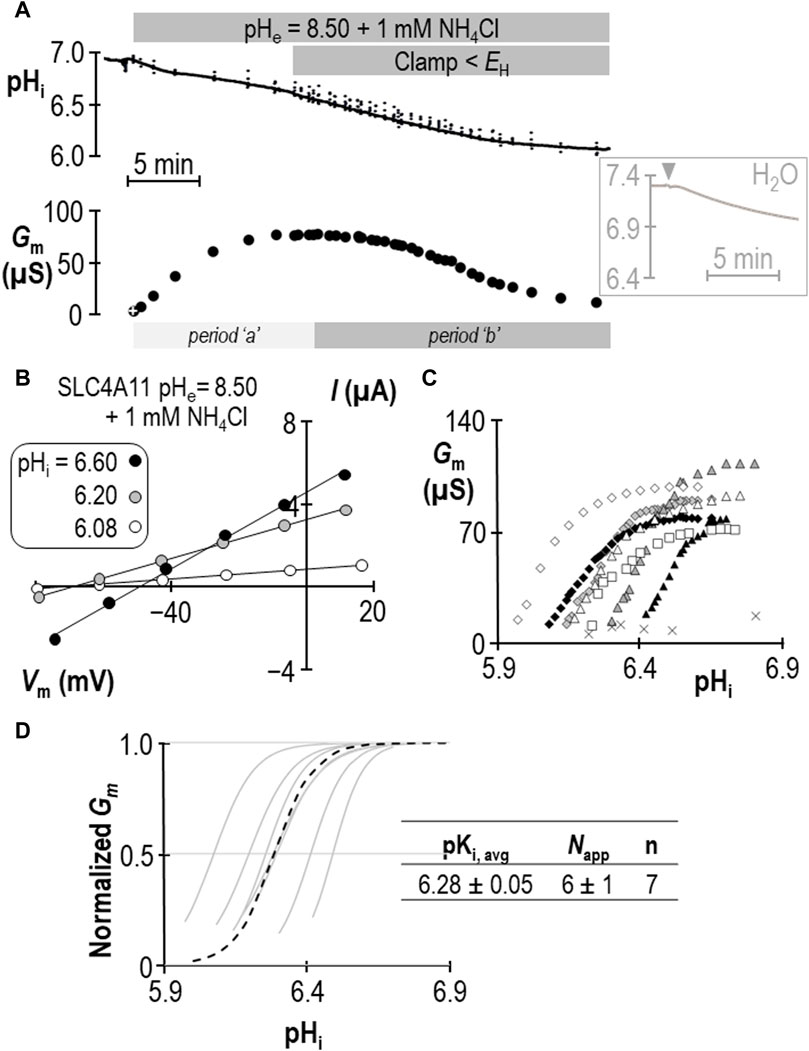
Figure 2. SLC4A11 behavior in the presence of 1 mM NH4Cl (extracellular pH = 8.50). (A) A representative example of the pHi (top) and Gm (bottom) response of an SLC4A11-expressing oocyte to the addition of 1 mM NH4Cl. The first Gm point in the data series (white cross) was gathered prior to the presence of NH4Cl. The inset shows a representative pHi response to the addition of 1 mM NH4Cl (point of solution change indicated by gray triangle) of a H2O-injected cell at pHe = 8.50. (B) A representative selection of I-V relationships gathered from a single SLC4A11-expressing oocyte as pHi falls during period “b”. (C) The relationship between pHi and Gm from the full-set of I-V relationships gathered from the SLC4A11-expressing cell shown in panel (B) (black diamonds) and from six other SLC4A11-expressing cells (each represented by its own symbol). The pHi versus Gm relationship for six H2O-injected oocytes after acidification by HCl injection is represented by crosses. (D) Best-fit lines for each cell to the Hill equation are shown in gray. The dashed black line represents the Hill equation generated using the average pKi and Napp of the n = 7 replicates, as shown in the inset table.
Does the action of SLC4A11 during period “a” represent a second pKi that reports acid-activation? We hypothesized that period “a” represented the time during which pKi was transitioning between its ± NH4Cl values. If we assume that neither Gm,max nor Napp change in a single SLC4A11-expressing oocyte during the course of an experiment such as that in Figure 2A, we can solve the Hill equation to generate an apparent pKi (pKi,app) for each point in the experiment at which we have paired values of Gm and pHi. The results of this approach are shown in Figure 3A and support the hypothesis that period “a” is dynamic time of pKi adjustment, while period “b” represents a time over which SLC4A11 has assumed a new and relatively stable pKi. In three of our seven experiments, we extended the protocol to examine a period “c” (example shown in Figure 3B) during which we could examine the behavior of SLC4A11 in the pH-range of period “a”, but after SLC4A11 has assumed its + NH4Cl pKi. As shown in Figure 3C, SLC4A11 exhibits a similar pKi during period “b” versus period “c” pKi (P = 0.19: two-tailed, paired t-test) and does not revisit the apparent acid-activated behavior exhibited during period “a.” In summary for this section, we find that the presence of 1 mM NH4Cl results in a significant acidic shift in the value of pKi for human SLC4A11 at pHe = 8.50.
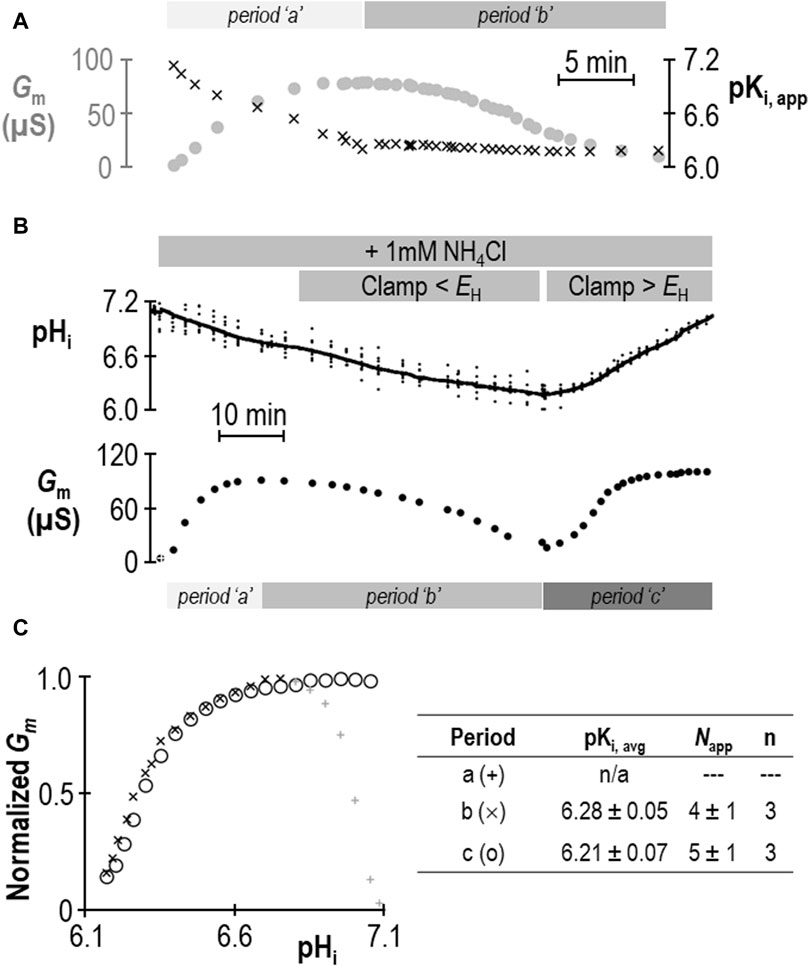
Figure 3. Validation of change in SLC4A11 pKi upon addition of NH4Cl. (A) Gm data from Figure 2A replotted with the apparent pKi (pKi,app) calculated from the pHi at each value of Gm. (B) A representative experiment similar to that shown in Figure 2A, extended into a third experimental period in which Gm is monitored during a return to starting pHi. (C) Gm values from panel (B) plotted for each of the three experimental periods, normalized to Gm,max for each period. The inset table shows pKi and Napp for periods “b” and “c” calculated from best-fit data to the Hill equation for three such experiments.
2.3 Determining the ion-selectivity of SLC4A11 in the presence of 1 mM NH4Cl
In an earlier study we demonstrated that for SLC4A11-expressing oocytes, the relationship between Vm and the transmembrane pH gradient (64 mV/pH-unit) was close to Nernstian with respect to H+ (Myers et al., 2016). Figure 4A shows equivalent data gathered in the presence of 1 mM NH4Cl from experiments such as those shown in Figures 2A, 3B. The average slope of the relationship is 71 ± 5 mV/decade (Figure 4B) with an x-axis intercept of −1.25 ± 0.04 (Figure 4C). Data from one of the three Figure 3B-style experiments is highlighted in Figure 4A, with black data points taken from period “b” and white data points taken from period “c.” In summary for this section, the slope of the relationship between Vm and transmembrane pH gradient does not appear to be greatly disturbed by the presence of 1 mM NH4Cl, except for the unusual observation that the relationship does not intersect with the origin. The meaning of this observation is explored in Section 3.3.
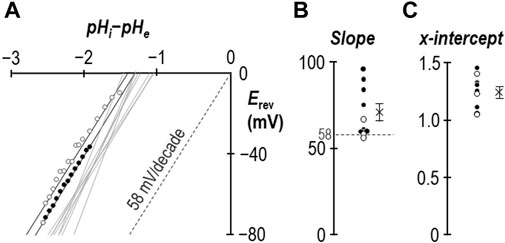
Figure 4. The ion-selectivity of SLC4A11 in the presence of NH4Cl. (A) The relationship between Vm and the transmembrane pH gradient (pHi-pHe), determined from the experiments represented in Figures 2, 3, are shown by solid gray lines. One example data set is shown in full from period “b” (filled circles) and period “c” (open circles). The Nernstian slope of ideal H+ selectivity is shown as a gray dashed line. (B) The average slopes calculated from the data in panel (A) are shown as filled (data gathered during period “b”) and open (data gathered during period “c”) circles. The cross represents the average of these data. (C) The average x-intercepts calculated from the data in panel (A) are shown as filled (data gathered during period “b”) and open (data gathered during period “c”) circles. The cross represents the average of these data.
2.4 Comparing the influence of pHe and [NH3] on SLC4A11 pKi
Using a similar work-flow to that described above, we determined the pKi of SLC4A11 at pHe = 7.50 in the presence of 1 mM NH4Cl (Figures 5A–C). Gm.max in this cohort of cells was 104 ± 7 µS, which is not different from the equivalent range reported from cells assayed at pHe = 8.50 + NH4Cl (p = 0.09: two-tailed, unpaired t-test). Because the ratio of NH3:NH4+ is pH-sensitive, we also determined the pKi of SLC4A11 at pHe = 8.50 in the presence of 0.12 mM NH4Cl (Figures 6A–C). In both conditions, although [NH4Cl] is different, [NH3] is the same (0.017 mM) Figure 6D summarizes the values of pKi that have been determined during this study. We find that pKi at pHe = 7.50 in the presence of 1 mM NH4Cl is 7.06 ± 0.05 (Figure 5C), and the pKi at pHe = 8.50 in the presence of 0.12 mM NH4Cl is 7.04 ± 0.05 (Figure 6C). These values are not significant different from each other (p = 0.68: two-tailed, unpaired t-test).
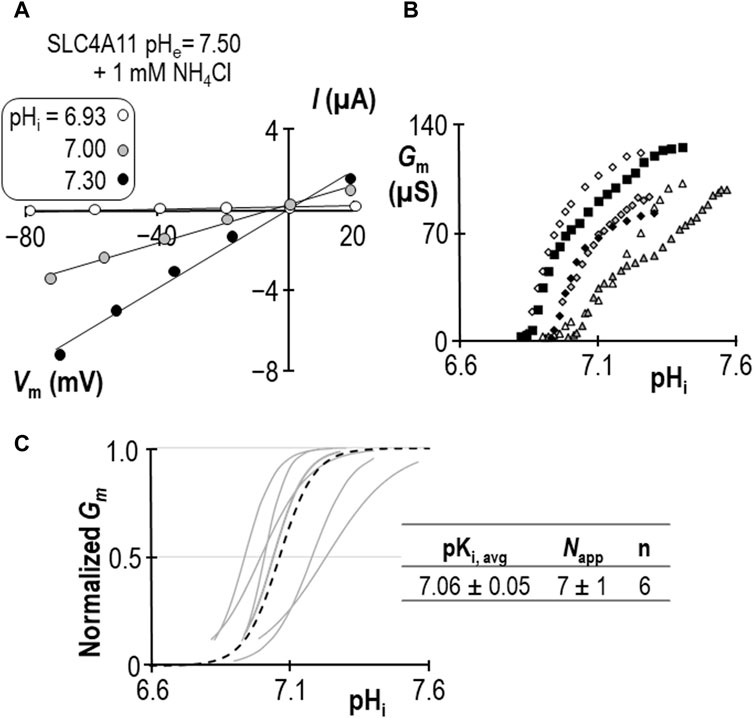
Figure 5. SLC4A11 behavior in the presence of 1 mM NH4Cl (extracellular pH = 7.50). (A) A representative selection of current-voltage (I-V) relationships gathered from a single SLC4A11-expressing oocyte as intracellular pH (pHi) rises. (B) The relationship between pHi and slope conductance (Gm) from the full-set of I-V relationships gathered from the SLC4A11-expressing cell shown in panel (A) (black diamonds) and from five other SLC4A11-expressing cells (each represented by its own symbol). (C) Best-fit lines for each cell to the Hill equation are shown in gray. The dashed black line represents the Hill equation generated using the average pKi and apparent Hill coefficients (Napp) of the n = 6 replicates, as shown in the inset table.
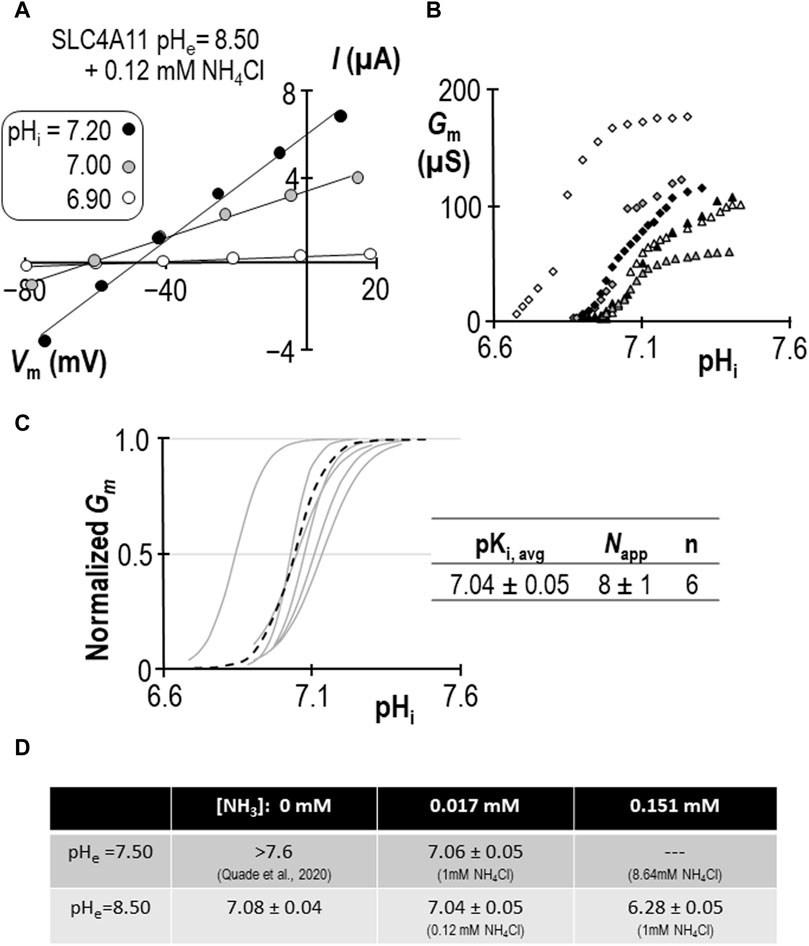
Figure 6. SLC4A11 behavior in the presence of 0.12 mM NH4Cl (extracellular pH = 8.50). (A) A representative selection of current-voltage (I-V) relationships gathered from a single SLC4A11-expressing oocyte as intracellular pH (pHi) rises. (B) The relationship between pHi and slope conductance (Gm) from the full-set of I-V relationships gathered from the SLC4A11-expressing cell shown in panel (A) (black diamonds) and from five other SLC4A11-expressing cells (each represented by its own symbol). (C) Best-fit lines for each cell to the Hill equation are shown in gray. The dashed black line represents the Hill equation generated using the average pKi and apparent Hill coefficients (Napp) of the n = 6 replicates, as shown in the inset table. (D) A summary of the pKi of SLC4A11 detemined under the various conditions tested in this study.
We can make two additional statistical comparisons: [1] at pHe = 8.50, pKi is not significantly different in the presence or absence of 0.12 mM NH4Cl (P = 0.50: two-tailed, unpaired t-test), and [2] in the presence of 1 mM NH4Cl, raising pHe from 7.50 to 8.50 has a significant acidifying effect on pKi (p< 0.01: two-tailed, unpaired t-test). The interpretation of these findings are discussed in Section 3.4.
3 Discussion
3.1 NH4Cl increases SLC4A11 Gm by shifting pKi in the acidic direction
Our data show that, at pHe = 8.50, the extracellular presence of 1 mM NH4Cl results in a significant acidic shift in pKi such that, at almost any value of pHi between 6.0 and 7.3 (i.e., the range bounded by the two ± NH4Cl traces: solid and dashed black-lines in Figure 7A), Gm would be increased. A formal determination of pKi at physiological pHe in the absence of NH4Cl has been precluded by a practical limitation on how high we can raise oocyte pHi, but we have previously estimated that the value must be more alkaline than 7.6 (Quade et al., 2020). The ability of NH4Cl to cause an acidic shift in pKi, enables us to determine a pKi range of 7.06 ± 0.05 at pHe = 7.50 in the present study. Thus, we can imagine that, even with the conservative estimate of the NH4Cl-free pKi, the implication is similar: the presence of NH4Cl would cause an increase in Gm at typical physiological values of pHi (e.g., 7.0–7.3, which is included in the range bounded by the solid and dashed gray-lines in Figure 7A). Critically, this stimulatory effect of NH4Cl represents only an increase in Gm caused by a redefinition of the pHi versus Gm relationship. Neither Gm,max nor Napp are significantly altered by NH4Cl (at least as determined at pHe = 8.50) so the redefinition appears to represent a direct acidic-translation of the relationship. Although we are wary of assigning any meaning to the numerical value of Napp (which is a function of the number of titratable moieties within SLC4A11), the observation of an unchanging Napp at least implies that mechanism by which SLC4A11 responds to pHi is similarly complex in the absence and presence of NH4Cl.
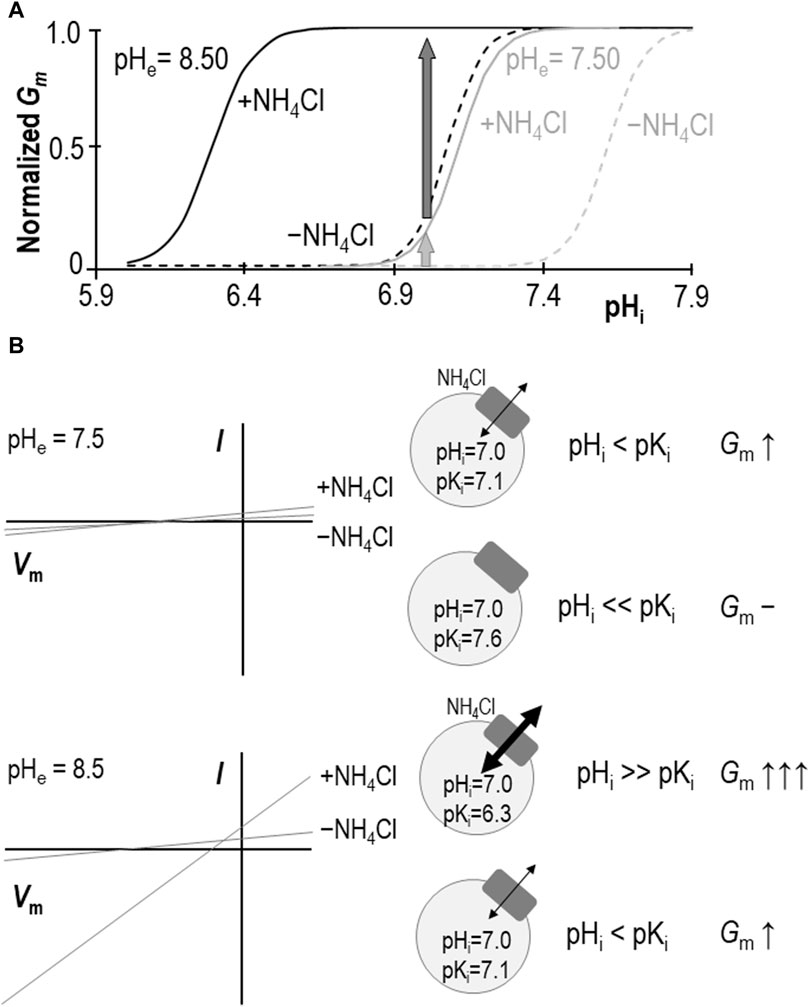
Figure 7. Models of SLC4A11 action in the presence and absence of NH4Cl. (A) Cartoon representing the pHi versus Gm relationships for SLC4A11 at pHe 7.50 (gray lines) and 8.50 (black lines) in the presence (solid lines) and absence (dashed lines) of 1 mM NH4Cl. (B) Cartoon showing how the scheme in panel (A) could explain how NH4Cl can achieve greater potency with respect to enhancing SLC4A11-mediated conductance at pHe = 8.5 (lower panel) versus 7.5 (upper panel), as observed in prior studies such as Zhang et al., 2015 or Myers et al., 2016. I-V plots are cartoons, gray circles represent cells expressing SLC4A11 (gray boxes) with black arrows indicating relative magnitudes of conductance.
We had once before investigated the role of NH4Cl in stimulating SLC4A11 (Myers et al., 2016). We found, as others had before us (Zhang et al., 2015), that the presence of 5 mM NH4Cl causes an increase in SLC4A11 Gm (or Im at fixed Vm in the case of those other studies). However, although we appreciated at that time that SLC4A11 was pHi-dependent, we did not consider the possibility that the relationship between pHi and Gm could be modulated. The addition of 5 mM NH4Cl to (even H2O-injected) oocytes causes a rapid and robust depolarization (Musa-Aziz et al., 2009). This action in itself is sufficient to drive SLC4A11-mediated H+ efflux, resulting in a cellular alkalinization that further activates SLC4A11 (Myers et al., 2016). Thus, in that study, when we increased Gm to Gm.max at pHe = 8.50 by adding 5 mM NH4Cl and saw no change in that value upon NH4Cl removal (maintaining a voltage clamp at 0 mV to mimic the depolarizing effect of NH4Cl presence), we assumed that the presence of NH4Cl was merely causing Gm to rise according to the prescribed pHi vs. Gm relationship. In light of our new data, we reinterpret those data as likely to have been gathered at a pHi value that was greater than the NH4Cl-free pKi, where Gm values for both ± NH4Cl relationships are close to Gm,max. That is to say that we reinterpret our data in favor of a model in which NH4Cl causes a direct rather than indirect allosteric activation of SLC4A11.
3.2 Data do not conclusively support a more important role for NH3 versus NH4+ for SLC4A11 action
Models in which SLC4A11 transports NH3/NH4+ favor a NH3:nH+ cotransport mechanism over NH4+ transport because NH3 increases in concentration with rising pH, thereby providing an explanation for the greater stimulation of SLC4A11 currents/conductance at pHe = 8.50 than pHe = 7.50. We might use the same logic to conclude that NH3 is more likely than NH4+ to be the allosterically activating species. However, our data provide an alternative explanation: as shown in Figures 7A,B, at the typical resting pHi range for SLC4A11-expressing oocytes [6.9–7.0: (Quade et al., 2020)], the shift in pKi makes less difference to Gm at pHe = 7.50 (light gray arrow in Figures 7A,B/upper panel) than at pHe = 8.50 (dark gray arrow in Figures 7A,B/lower panel). In this case it is not necessary to invoke a model in which the abundance of the substrate/activator is pH dependent; NH4+ (whose fractional abundance is relatively unchanged between pHe 7.50 and 8.50) could be considered equally likely to be the species responsible for the observed stimulation.
Our data do not speak definitively to the nature of the activating species. We believe, because the time course of pKi shift (>10 min: Figure 3A) is much slower than the rate of solution turnover in the bath (<1 min) that the allosteric activation likely requires the activating species to accumulate intracellularly in order to exert its effect on SLC4A11. Because the handling of NH3/NH4+ by oocytes is unusually complex (Musa-Aziz et al., 2009), it is difficult to specifically relate this time course of activation to the accumulation of either species. On the one hand NH3 is presumed to be the more membrane permeable of the two species. On the other hand, in contrast to the expected alkalinization observed in mammalian cells exposed to NH4Cl, (even H2O-injected) oocyte pHi paradoxically acidifies as if NH4+ is accumulating faster, perhaps entering via non-selective cation channels as NH3 is sequestered in sub-membranous granules (Burckhardt and Frömter, 1992). For this reason, it is not clear whether there is a diagnostically useful differential in their rate of accumulation that could point to one species over the other.
3.3 SLC4A11 retains H+ selectivity in the presence of NH4Cl
Another critical parameter that is unchanged in the presence of NH4Cl is its H+ selectivity, because the Vm of SLC4A11-expressing cells exhibits a close-to-Nernstian response to changes in the transmembrane pH gradient (Figure 4). This suggests that SLC4A11 remains a selective H+ conductor rather than assuming a novel NH3-coupling mode. Although previous studies have claimed to provide evidence of NH3-coupled H+ transport using a similar approach (Zhang et al., 2015; Kao et al., 2020), we believe that these finding should be interpreted with caution as the approach violates necessary assumptions of reversal potential calculations: chiefly that substrates can only cross the membrane via SLC4A11 (not true for NH3) and that Vm is dominated by the action of SLC4A11 (not true due to the depolarizing action of NH3/NH4+). In the absence of a specific inhibitor for SLC4A11, to distinguish the behavior of the protein from that of the system, the best that such calculations may achieve is a description of the permeability of the system (i.e., SLC4A11 and its membrane environment). One such study concluded that SLC4A11 was capable of both NH3:H+ cotransport and H+ transport (Kao et al., 2020). If so, this is not the typical action of an obligatorily coupled cotransport protein and could equally describe the behavior of a H+ conductor in an NH3-permeable membrane.
There is one unusual aspect to our reversal potential data that requires further explanation. In previous studies we have found that the relationship between the transmembrane gradient and Vm for wild-type SLC4A11 crosses the x-axis at a value close to zero as expected for a H+ conductor. In the present study performed in the presence of NH4Cl, we find that the relationship is substantially offset from the origin and intersects the x-axis at −1.25 pH-units as if we had underestimated pHi by 1.25 units. This is not impossible, as seven of the ten data sets were gathered while SLC4A11 was mediating H+ influx and thus pH immediately below the membrane may have been more acidic than bulk pHi being measured at the tip of our microelectrode, which is impaled deeper into the cell. However, three of these data sets were gathered while SLC4A11 was mediating H+ efflux, and exhibited the same offset, so we do not believe that this can be the correct explanation. An alternate explanation is illustrated in Figure 8. Here we show that, if we consider these data as being representative of the system and interpret them using a modified Goldman-Hodgkin-Katz equation, we can reproduce the offset by implementing a small permeability to a depolarizing cation. Two examples are provided: in Model 1, we add a sodium permeability to the system. Because the abundance of H+ is so small compared to the abundance of Na+, the relative permeability of Na+ to H+ must be very small not to completely overwhelm Vm. In this instance, PNa/PH = 6 × 10−10 provides a good fit to our observations. In Model 2 we add an NH4+ permeability to the system. We assume that [NH4Cl] is 1 mM on both sides of the membrane and calculate [NH4+]i for each value of pHi. In this instance PNH4/PH = 6 × 10−8 provides a good fit for our data. Although the relationship curves off to an asymptote, the initial slope is Nernstian with respect to H+, and its projection (dashed gray line) crosses the x-axis at −1.25 pH units. We note that the x-intercept of our data is also a projection and we do not know whether our data would also curve in a similar way if extended towards the x-axis. In any case this is not a critical issue as the relationship can be made to conform to the dashed line if we lower our estimate of [NH4Cl]i to 0.1 mM. As either permeability is trivial compared to that of H+, it does not appear to be a major confounding factor to our hypothesis. In the absence of a specific blocker, we cannot know whether the additional permeability is intrinsic to SLC4A11 or to the system in general. However, as we have only observed this in the presence of NH4+, and because NH4+ is a depolarizing influence in even H2O-injected cells, we tentatively suggest that the x-axis offset represents the previously described endogenous NH4+ conductance.
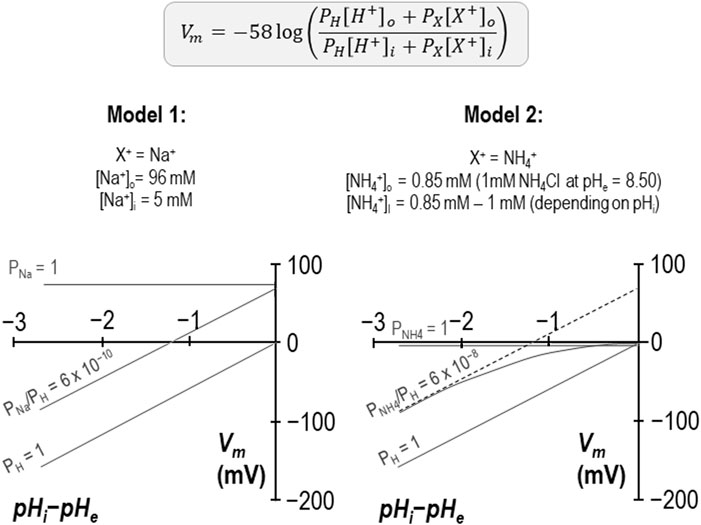
Figure 8. Models of additional, non-H+ permeabilities in SLC4A11-expressing cells in the presence of NH4Cl Two models based on a modified Goldman-Hodgkin-Katz equation in which the implementation of a small cationic permeability (either Na+ in the case of Model 1 or NH4+ in the case of Model 2) causes an offset in the projected x-axis intercept, as observed in our experiments conducted at pHe = 8.50 + 1 mM NH4Cl (per Figure 4).
3.4 The relationship between pKi shifts caused by extracellular alkalinization versus NH4Cl addition
As previously observed, in the absence of NH4Cl, a shift of pHe from 7.50 to 8.50, is itself sufficient to acid-shift SLC4A11 pKi by more than 0.5 pH-units (Quade et al., 2020). If we compare SLC4A11 pKi determined at pHe = 7.50 + 1 mM NH4Cl to pKi determined at pHe = 8.50 + 0.12 mM NH4Cl (conditions in which [NH3] is the same and pKi is not different) we may conclude that, in the presence of NH4Cl, pKi has become pHe-independent and is determined by [NH3] alone. In that case we could consider [NH3] and pHe as activators that share a common mechanism. If SLC4A11 is less pHe dependent in the presence of NH4Cl, that would imply that the stimulatory effect of pHe on SLC4A11 may have limited relevance to its in vivo action.
On the other hand, if we compare SLC4A11 pKi determined at pHe = 7.50 + 1 mM NH4Cl to pKi determined at pHe = 8.50 + 1 mM NH4Cl, values which are ∼0.8 units apart, we may conclude that the phenomenon of pHe-dependence is preserved in the presence of 1 mM NH4Cl and that the actions of NH4Cl and pHe are, at least in part, mechanistically independent and additive. The common ground between these two ways of looking at the data are that the presence of NH4Cl alters the relationship between pHe and pKi such that a more acidic pKi can be achieved at a given pHe. Interestingly, this is the opposite to a phenomenon that we have observed in relation to certain pathological SLC4A11 mutants such as R125H, in which the mutation causes pKi to become more alkaline at a given pHe (Quade et al., 2022). Unfortunately, the technical limitations on how far we can extend pHi and the low resolution of our pKi assay (due to the large standard error intrinsic to the data sets) currently preclude us from more detailed exploration of the relationship between these activating parameters.
3.5 Implications for the physiological role of SLC4A11
The fluid pumping action of corneal endothelial cells requires the action of a basolateral Na/K-ATPase to generate the transmembrane sodium gradient that drives the Na+/CO3= cotransporter, drawing osmolytes from the stromal fluid to discourage fluid accumulation. The pump is energized by glutaminolysis that feeds α-ketoglutarate into the TCA cycle to generate ATP; a side product of this reaction is NH3 (Zhang et al., 2017). We have previously hypothesized that acid-loading by SLC4A11 could be useful to stabilize pHi during robust bicarbonate pump function, responding to Na+/CO3= cotransporter action by sensing a local rise in pHi (Myers et al., 2016; Nehrke, 2016). Our new data indicate that SLC4A11 is a H+ conductor both in the presence and absence of NH4Cl and thus that NH3/NH4+ is an allosteric activator rather than a cotransported substrate of SLC4A11. This action is incompatible with a role of corneal endothelial SLC4A11 in mediating the export of excess NH3 from glutaminolysis, and incompatible with SLC4A11 being able to harness an outwardly-directed NH3 gradient to mediate H+ efflux. Because the electrochemical gradient for H+ is typically inwardly directed, we predict that a rise in intracellular NH3/NH4+ promotes H+ influx independent of any rise in pHi. The ability of NH3/NH4+ to acid shift pKi implies that the ability of SLC4A11 to support pump function is potentiated by NH3/NH4+, the generation of which could be considered to be a proxy for the energetic requirements of the pump.
3.6 Summary
The presence of NH4Cl causes an acidic shift in the pKi of human SLC4A11, which translates to an increase in Gm at physiological values of pHi. The presence of NH4Cl does not affect the H+ selectivity of SLC4A11, thus we conclude that NH4Cl is an allosteric activator of SLC4A11-mediated H+ conductance. The influence of increasing [NH4Cl] upon SLC4A11 activity is reminiscent of the influence of increasing pHe, but further work will be required to determine whether these share a common mechanism.
4 Materials and methods
4.1 Oocyte preparation and culture
Ovaries were harvested from female Xenopus laevis (Xenopus Express, Brooksville, FL) in accordance with the protocol approved by the University at Buffalo Institutional Animal Care and Use Committee. Frogs were anesthetized in 0.2% tricaine solution, ovariectomized, and euthanized by exsanguination. Extracted tissue was cut into ∼1 cm2 pieces and washed in a Ca2+-free solution (82 mM NaCl, 2 mM KCl, 20 mM MgCl2, 5 mM HEPES, pH 7.50). Oocytes were liberated by digestion in 2 mg/mL type 1A collagenase solution, and the isolated cells were washed further in the Ca2+-free solution to remove the collagenase prior to resuspension in a physiological buffer (ND96: 96 mM NaCl, 2 mM KCl, 1.8 mM CaCl2, 1 mM MgCl2, 5 mM HEPES, pH 7.50, 200 mOsmol/kg H2O). Until experimental use, oocytes were cultured at 18°C in OR3 medium (14 g/L Leiboviz’s L-15 medium powder, 5 mM HEPES, 20 mL/L 100x penicillin-streptomycin, pH 7.50, 200 mOsmol/kg H2O).
4.2 cRNA preparation and injection
Our starting material was a clone of human SLC4A11-B in pBSXG4 vector. The construct was linearized with HindIII, which cuts at a site downstream of the open reading frame providing a termination point for transcription. The linearized DNA was purified using a MinElute PCR Purification Kit (QIAgen, Germantown, MD) used as a template for cRNA synthesis using the T7 mMESSAGE mMACHINE kit (Invitrogen, Carlsbad, CA). cRNA was purified using an RNeasy MinElute Cleanup KIt (QIAgen). 25 ng of cRNA or H2O was injected into each oocyte using a Nanoject programmable injector (Drummond Scientific, Broomall, PA).
4.3 Electrophysiology
Oocytes were placed into chamber (RC-3Z: Warner Instruments, Hamden, CT) on an anti-vibration table (Vision IsoStation; Newport Corp., Irvine, CA) and were superfused at 2 mL/min with solutions fed from syringe pumps (Harvard Apparatus, Holliston, MA). Borosilicate glass capillaries (BF200-156-10: Sutter Instrument, Novato, CA) were pulled into microelectrodes (such that they exhibited a tip resistance of 0.1–2 MΩ when filled with saturated KCl solution) using a micropipette puller (P-1000: Sutter Instrument). Oocytes were impaled with two such KCl-filled microelectrodes (one current-passing and one voltage-sensing) connected to an oocyte clamp (OC275: Warner Instruments, Hamden, CT). A bath clamp (725I: Warner Instruments) was used to hold the potential of the chamber fluid at 0 mV. Current-voltage (I-V) plots were gathered in 20 mV, 100 ms steps, returning to the spontaneous membrane potential for 100 ms between each step. H+-selective microelectrodes were pulled in the same manner as voltage electrodes but the tips were filled with hydrogen ionophore I/cocktail B (Sigma Aldrich) and backfilled with a solution composed of 40 mM KH2PO4, 15 mM NaCl, pH 7.0. These electrodes were connected to a dual-channel electrometer (HiZ-223: Warner Instruments). Complete technical details can be found in the 2013 review by Lee, Boron, and Parker (Lee et al., 2013). Signals were digitized via a Digidata 1550 unit and captured using Clampex 10.4 software (Molecular Devices LLC, San Jose) and custom continuous acquisition software (written by Mr. Dale Huffman for Walter Boron’s laboratory at Case Western Reserve University, Cleveland, OH).
4.4 Electrophysiology solutions
pH 7.50 solutions contained 96 mM NaCl, 2 mM KCl, 1.8 mM CaCl2, 1 mM MgCl2, 5 mM HEPES, 200 mOsmol/kg H2O. pH 8.50 solutions had the same composition but were buffered with 5 mM Bicine in place of HEPES. NH4Cl was added to NH4Cl-containing solutions as a powder, and pH was readjusted as necessary.
4.5 Data analysis
Data are presented as means ± standard error of the mean. Slope conductance (Gm) was determined from the slope of a linear trendline fit to I-V data in Microsoft Excel. Normalized Gm data was plotted against pHi (expressed as [OH−]) and fit to the Hill equation using the solver add-in of Excel to determine the values of EC50 and Napp that would result in the minimum root square difference between the observed data and the outcome of the Hill equation:
EC50 was converted into a value of pKi using the following equation:
For Figure 3A, we calculated pKi,app by solving the Hill equation for EC50 at each pair of Gm and [OH−] (i.e., pHi) data points. We assumed that Gm,max and Napp were constants: 79 µS and 7 respectively, corresponding to the data gathered from this cell during period “b.” Calculations of [NH3] at a given pH value and [NH4Cl] assume a pKa for the NH3/NH4+ equilibrium of 9.25.
Statistical analysis was performed in Excel using unpaired t-tests, one- or two-tailed as necessary. For multiple comparison, ANOVA was performed using MiniTab software.
Data availability statement
The datasets presented in this study can be found in online repositories. The names of the repository/repositories and accession number(s) can be found below: https://doi.org/10.6084/m9.figshare.25928233.v1.
Ethics statement
The animal study was approved by IACUC at University at Buffalo Jacobs School of Medicine. The study was conducted in accordance with the local legislation and institutional requirements.
Author contributions
RP: Conceptualization, Data curation, Formal Analysis, Investigation, Methodology, Validation, Visualization, Writing–original draft, Writing–review and editing. BQ: Conceptualization, Investigation, Methodology, Visualization, Writing–review and editing. AM: Methodology, Project administration, Resources, Supervision, Writing–review and editing. MP: Conceptualization, Data curation, Formal Analysis, Funding acquisition, Investigation, Methodology, Project administration, Resources, Supervision, Validation, Visualization, Writing–original draft, Writing–review and editing.
Funding
The author(s) declare that financial support was received for the research, authorship, and/or publication of this article. This work was funded by NIH grant NEI-EY028580 to MP. RP was partially supported by an award from the Stephen Besch Scholarship fund.
Acknowledgments
Thanks to Rossana Occhipinti at Case Western Reserve University for helpful discussions and to Jacob Tondreau for technical assistance.
Conflict of interest
The authors declare that the research was conducted in the absence of any commercial or financial relationships that could be construed as a potential conflict of interest.
Publisher’s note
All claims expressed in this article are solely those of the authors and do not necessarily represent those of their affiliated organizations, or those of the publisher, the editors and the reviewers. Any product that may be evaluated in this article, or claim that may be made by its manufacturer, is not guaranteed or endorsed by the publisher.
References
Burckhardt B. C., Frömter E. (1992). Pathways of NH3/NH4+ permeation across Xenopus laevis oocyte cell membrane. Pflugers Arch. 420, 83–86. doi:10.1007/BF00378645
Desir J., Moya G., Reish O., Van Regemorter N., Deconinck H., David K. L., et al. (2007). Borate transporter SLC4A11 mutations cause both Harboyan syndrome and non-syndromic corneal endothelial dystrophy. J. Med. Genet. 44, 322–326. doi:10.1136/jmg.2006.046904
Frommer W. B., von Wirén N. (2002). Plant biology: ping-pong with boron. Nature 420, 282–283. doi:10.1038/420282a
Gröger N., Fröhlich H., Maier H., Olbrich A., Kostin S., Braun T., et al. (2010). SLC4A11 prevents osmotic imbalance leading to corneal endothelial dystrophy, deafness, and polyuria. J. Biol. Chem. 285, 14467–14474. doi:10.1074/jbc.M109.094680
Han S. B., Ang H.-P., Poh R., Chaurasia S. S., Peh G., Liu J., et al. (2013). Mice with a targeted disruption of Slc4a11 model the progressive corneal changes of congenital hereditary endothelial dystrophy. Invest. Ophthalmol. Vis. Sci. 54, 6179–6189. doi:10.1167/iovs.13-12089
Jalimarada S. S., Ogando D. G., Vithana E. N., Bonanno J. A. (2013). Ion transport function of SLC4A11 in corneal endothelium. Invest. Ophthalmol. Vis. Sci. 54, 4330–4340. doi:10.1167/iovs.13-11929
Kao L., Azimov R., Abuladze N., Newman D., Kurtz I. (2015). Human SLC4A11-C functions as a DIDS-stimulatable H+(OH−) permeation pathway: partial correction of R109H mutant transport. Am. J. Physiol. Cell Physiol. 308, C176–C188. doi:10.1152/ajpcell.00271.2014
Kao L., Azimov R., Shao X. M., Abuladze N., Newman D., Zhekova H., et al. (2020). SLC4A11 function: evidence for H+(OH-) and NH3-H+ transport. Am. J. Physiol. Cell Physiol. 318, C392–C405. doi:10.1152/ajpcell.00425.2019
Kao L., Azimov R., Shao X. M., Frausto R. F., Abuladze N., Newman D., et al. (2016). Multifunctional ion transport properties of human SLC4A11: comparison of the SLC4A11-B and SLC4A11-C variants. Am. J. Physiol. Cell Physiol. 311, C820–C830. doi:10.1152/ajpcell.00233.2016
Lee S.-K., Boron W. F., Parker M. D. (2013). Monitoring ion activities in and around cells using ion-selective liquid-membrane microelectrodes. Sensors (Basel) 13, 984–1003. doi:10.3390/s130100984
Lee S.-K., Occhipinti R., Moss F. J., Parker M. D., Grichtchenko I. I., Boron W. F. (2022). Distinguishing among HCO3–, CO3=, and H+ as substrates of proteins that appear to Be “bicarbonate”. Transp. J. Am. Soc. Nephrol. ASN 2022030289. doi:10.1681/ASN.2022030289
Loganathan S. K., Schneider H.-P., Morgan P. E., Deitmer J. W., Casey J. R. (2016). Functional assessment of SLC4A11, an integral membrane protein mutated in corneal dystrophies. Am. J. Physiol. Cell Physiol. 311, C735–C748. doi:10.1152/ajpcell.00078.2016
Lopez I. A., Rosenblatt M. I., Kim C., Galbraith G. C., Jones S. M., Kao L., et al. (2009). Slc4a11 gene disruption in mice: cellular targets of sensorineuronal abnormalities. J. Biol. Chem. 284, 26882–26896. doi:10.1074/jbc.M109.008102
Malhotra D., Jung M., Fecher-Trost C., Lovatt M., Peh G. S. L., Noskov S., et al. (2020). Defective cell adhesion function of solute transporter, SLC4A11, in endothelial corneal dystrophies. Hum. Mol. Genet. 29, 97–116. doi:10.1093/hmg/ddz259
Musa-Aziz R., Jiang L., Chen L.-M., Behar K. L., Boron W. F. (2009). Concentration-dependent effects on intracellular and surface pH of exposing Xenopus oocytes to solutions containing NH3/NH4+. J. Membr. Biol. 228, 15–31. doi:10.1007/s00232-009-9155-7
Myers E. J., Marshall A., Jennings M. L., Parker M. D. (2016). Mouse Slc4a11 expressed in Xenopus oocytes is an ideally selective H+/OH- conductance pathway that is stimulated by rises in intracellular and extracellular pH. Am. J. Physiol. Cell Physiol. 311, C945–C959. doi:10.1152/ajpcell.00259.2016
Nehrke K. (2016). H(OH), H(OH), H(OH): a holiday perspective. Focus on Mouse Slc4a11 expressed in Xenopus oocytes is an ideally selective H+/OH- conductance pathway that is stimulated by rises in intracellular and extracellular pH. Am. J. Physiol. Cell Physiol. 311, C942–C944. doi:10.1152/ajpcell.00309.2016
Ogando D. G., Choi M., Shyam R., Li S., Bonanno J. A. (2019). Ammonia sensitive SLC4A11 mitochondrial uncoupling reduces glutamine induced oxidative stress. Redox Biol. 26, 101260. doi:10.1016/j.redox.2019.101260
Ogando D. G., Jalimarada S. S., Zhang W., Vithana E. N., Bonanno J. A. (2013). SLC4A11 is an EIPA-sensitive Na+ permeable pHi regulator. Am. J. Physiol. Cell Physiol. 305, C716–C727. doi:10.1152/ajpcell.00056.2013
Park M., Li Q., Shcheynikov N., Zeng W., Muallem S. (2004). NaBC1 is a ubiquitous electrogenic Na+-coupled borate transporter essential for cellular boron homeostasis and cell growth and proliferation. Mol. Cell 16, 331–341. doi:10.1016/j.molcel.2004.09.030
Parker M. D., Boron W. F. (2013). The divergence, actions, roles, and relatives of sodium-coupled bicarbonate transporters. Physiol. Rev. 93, 803–959. doi:10.1152/physrev.00023.2012
Parker M. D., Ourmozdi E. P., Tanner M. J. (2001). Human BTR1, a new bicarbonate transporter superfamily member and human AE4 from kidney. Biochem. Biophys. Res. Commun. 282, 1103–1109. doi:10.1006/bbrc.2001.4692
Quade B. N., Marshall A., Parker M. D. (2020). pH dependence of the Slc4a11-mediated H+ conductance is influenced by intracellular lysine residues and modified by disease-linked mutations. Am. J. Physiol. Cell Physiol. 319, C359–C370. doi:10.1152/ajpcell.00128.2020
Quade B. N., Marshall A., Parker M. D. (2022). Corneal dystrophy mutations R125H and R804H disable SLC4A11 by altering the extracellular pH dependence of the intracellular pK that governs H+(OH-) transport. Am. J. Physiol. Cell Physiol. 323, C990–C1002. doi:10.1152/ajpcell.00221.2022
Vilas G. L., Loganathan S. K., Liu J., Riau A. K., Young J. D., Mehta J. S., et al. (2013). Transmembrane water-flux through SLC4A11: a route defective in genetic corneal diseases. Hum. Mol. Genet. 22, 4579–4590. doi:10.1093/hmg/ddt307
Vithana E. N., Morgan P., Sundaresan P., Ebenezer N. D., Tan D. T. H., Mohamed M. D., et al. (2006). Mutations in sodium-borate cotransporter SLC4A11 cause recessive congenital hereditary endothelial dystrophy (CHED2). Nat. Genet. 38, 755–757. doi:10.1038/ng1824
Vithana E. N., Morgan P. E., Ramprasad V., Tan D. T. H., Yong V. H. K., Venkataraman D., et al. (2008). SLC4A11 mutations in Fuchs endothelial corneal dystrophy. Hum. Mol. Genet. 17, 656–666. doi:10.1093/hmg/ddm337
Weiss J. S., Rapuano C. J., Seitz B., Busin M., Kivelä T. T., Bouheraoua N., et al. (2024). IC3D classification of corneal dystrophies-edition 3. Cornea 43, 466–527. doi:10.1097/ICO.0000000000003420
Zhang W., Li H., Ogando D. G., Li S., Feng M., Price F. W., et al. (2017). Glutaminolysis is essential for energy production and ion transport in human corneal endothelium. EBioMedicine 16, 292–301. doi:10.1016/j.ebiom.2017.01.004
Keywords: acid-base, Btr1, NaBC1, cornea, proton
Citation: Pasternack RA, Quade BN, Marshall A and Parker MD (2024) NH3/NH4+ allosterically activates SLC4A11 by causing an acidic shift in the intracellular pK that governs H+(OH−) conductance. Front. Physiol. 15:1440720. doi: 10.3389/fphys.2024.1440720
Received: 29 May 2024; Accepted: 30 July 2024;
Published: 14 August 2024.
Edited by:
Ian Michael Thornell, The University of Iowa, United StatesReviewed by:
Mark Oliver Bevensee, University of Alabama at Birmingham, United StatesKirk L. Hamilton, University of Otago, New Zealand
Gergely Gyimesi, University of Bern, Switzerland
Copyright © 2024 Pasternack, Quade, Marshall and Parker. This is an open-access article distributed under the terms of the Creative Commons Attribution License (CC BY). The use, distribution or reproduction in other forums is permitted, provided the original author(s) and the copyright owner(s) are credited and that the original publication in this journal is cited, in accordance with accepted academic practice. No use, distribution or reproduction is permitted which does not comply with these terms.
*Correspondence: Mark D. Parker, cGFya2VyMjhAYnVmZmFsby5lZHU=