- Institute of Coaching and Performance, University of Central Lancashire, Preston, United Kingdom
The majority of “specialised” exercise configurations (e.g., supersets, drop sets, blood flow restriction) are being assessed as “shortcuts” to hypertrophy and strength improvements. However, these advanced training techniques may also offer significant benefits for systemic health and functional outcomes across recreational and clinical populations via locally induced metabolic responses. Stress-regulating mechanisms are known to enhance the body’s resilience by facilitating allostasis, the process of coordinating adaptive processes in reaction to stressors such as physical training. Yet, the role of the local metabolic stress provoked by resistance exercise has not gained much research attention despite its wide potential. Positive effects are not only linked to improved muscular endurance, hypertrophy and strength via primary and secondary mechanisms, but also to the release of myokines, hormones, microRNAs, immune factors, inflammatory substances and other endocrine molecules that initiate numerous health-promoting modifications on a systemic level. Resistance exercise strategies that maximise the local accumulation of metabolites are not well defined, although high volume, close proximity to failure and shorter rests seem to be a necessity. Additionally, blood flow restriction training provides a potent alternative for inducing local acidosis, thereby triggering several pathways associated with improved immunity and physical function even in remote muscle tissues. Future research is warranted to further explore advanced resistance training techniques, as these approaches may offer comparable benefits for physical and mental health to those seen with other forms of exercise such as high-intensity interval training and heavy resistance training.
Highlights
•Resistance exercise-induced local metabolic stress facilitates improvements in strength, hypertrophy and muscular endurance with the light-to-moderate loads. Some of these adaptations also occur in muscles trained for strength separately within the same session.
•Resistance exercise-induced local metabolic stress stimulates a comprehensive release of various myokines and cytokines associated with multiple health benefits on a systemic level. These benefits include blood pressure reductions, metabolic health and potential enhancement of cognitive function.
•The evidenced benefits are realised directly (via metabolites) and indirectly (via mechanical tension). In both cases, local metabolic stress is the key factor of influence in the described protocols.
•Local metabolic stress appears to be maximised by high volume of fatiguing sets. Blood flow restriction exercise augments anaerobic conditions, thereby allowing for a very light resistance to be applied.
1 Introduction
Physical exercise of any type is associated with improved health and life expectancy (Pedersen, 2019; Carapeto and Aguayo-Mazzucato, 2021; Kraschnewski et al., 2016). While moderate aerobic activities demonstrate the highest dose-dependent effect (O’Keefe et al., 2023), vigorous forms of exercise such as high-intensity interval training (HIIT) offer augmented health and functional benefits for the organism through enhanced aerobic and anaerobic adaptations (Atakan et al., 2021). High frequency of intense whole-body endurance exercise forms, however, may not be the best option for life-extending practices among sedentary and older individuals (O’Keefe et al., 2023; Franklin et al., 2020) due to the potential cardiac overuse injury risk (Franklin et al., 2020). On the other hand, regular resistance training has been increasingly associated with improved longevity (Kraschnewski et al., 2016; Shailendra et al., 2022; Momma et al., 2022) and physical function (Pedersen, 2019; Valenzuela et al., 2023) as well as with reduced risk of metabolic and musculoskeletal diseases (Kraschnewski et al., 2016; Shailendra et al., 2022; Momma et al., 2022; Westcott, 2012). While exercise strategies targeting maximal strength capacity dominate research, low-load training’s significance is comparatively underappreciated (Weakley et al., 2023). Yet, training with lighter resistances performed to fatigue could offer significant benefits for recreational population, including those at risk of chronic diseases (Weakley et al., 2023; Yang et al., 2019). These benefits stem from numerous adaptations commonly associated with both traditional strength training and aerobic endurance exercise (Weakley et al., 2023).
Metabolic stress by exercise is characterised by the accumulation of metabolites like lactate, inorganic phosphate and hydrogen ions within muscle cells, resulting from the low energy availability caused by high-intensity demands on energy systems (de Freitas et al., 2017). It can be systemically provoked with strenuous forms of whole-body movements (Atakan et al., 2021), or locally when there is a sustained muscular contraction or restricted blood flow in the targeted muscles (Lawson et al., 2022). While the former exercise modality places significant demands on the cardiovascular system (O’Keefe et al., 2023), the latter focuses on specific muscle tissues thereby maximising the anaerobic conditions regionally (Lawson et al., 2022). This local metabolic stress (LMS) is associated with the augmented production of lactate (Robergs et al., 2004), reactive oxygen species (ROS) (de Freitas et al., 2017) and serum hormones (Goto et al., 2005), contributing to the generation of myokines (Laurens et al., 2020), exerkines (Saheli et al., 2024) and microRNA (Domańska-Senderowska et al., 2019). All these molecules are released into systemic circulation by the exercising muscles and organs, leading to multiple health benefits (Saheli et al., 2024; So et al., 2014; Brooks et al., 2023). Unfortunately, muscular endurance training type, which embodies the principles of LMS (Goto et al., 2005), has primarily been investigated in relation to the protocols examining strength and hypertrophy outcomes (Hickmott et al., 2022; Haugen et al., 2023; Lacio et al., 2021; Warneke et al., 2024; Sødal et al., 2023; Ozaki et al., 2016), while the associations to health and longevity been rarely distinguished between different resistance training modalities (Kraschnewski et al., 2016; Shailendra et al., 2022; Momma et al., 2022).
Local anaerobic stimulus in exercised muscles is likely to be behind the reported significant improvements in blood pressure from isometric and blood flow resistance (BFR) training in recreational populations (Devereux, 2010; Feng et al., 2024). Furthermore, the capacity for local fatigue resistance serves as a practical measure of neuromuscular function, offering valuable insights into the ability to sustain normal activities, particularly in older adults (Devereux, 2010; Bemben, 1998; Kahraman and Varol, 2024; Bemben et al., 1996). Interestingly, both moderate-load exercise and light-load exercise with and without BFR lead to hypertrophy comparable with traditional strength training only if exercises are performed to near the failure points (Weakley et al., 2023; Lixandrão et al., 2018; Keskin et al., 2025; Schoenfeld et al., 2017; Grgic et al., 2018; Robinson et al., 2024), displaying less reliable results for strength increases (Weakley et al., 2023; Lixandrão et al., 2018; Schoenfeld et al., 2017). A recent systematic review and meta-analysis suggested that LMS might be behind the strength, hypertrophy, and endurance improvements observed with superset protocols as evidenced by higher blood lactate levels and energy expenditure (Zhang et al., 2025). General resistance training has demonstrated positive influence on oxidative stress and the liver (Bucarey et al., 2024), inflammation (Kwon et al., 2020; Brandt and Pedersen, 2010), immune system (Kwon et al., 2020; Chow et al., 2022; Zunner et al., 2022), cognition (Saheli et al., 2024; Bu et al., 2025; Huang et al., 2021), metabolic health (Bucarey et al., 2024; Kwon et al., 2020; Chow et al., 2022), cardiovascular system (Devereux, 2010; Chow et al., 2022; Wang et al., 2018; Owen et al., 2010), and has exerted a reduced risk of infections and chronic diseases, including diabetes, cancer, atherosclerosis and neurodegeneration (Brandt and Pedersen, 2010; Zunner et al., 2022; Saliminejad et al., 2019). Whether local metabolic responses in isolated muscle groups contribute to these outcomes remains underexamined, but plausible (Weakley et al., 2023; Goto et al., 2005; Devereux, 2010; Zhang et al., 2025; May et al., 2018; Farrell et al., 2018; Coffey and Hawley, 2007; Spiering et al., 2009; Kraemer and Ratamess, 2005; Fiorenza et al., 2018; Liu et al., 2024). This is particularly highlighted by studies with BFR exercise protocols, which show a remarkable potential to influence a plethora of positive adaptations on a whole-body level instigated by induced local acidosis (Feng et al., 2024; Lixandrão et al., 2018; Martin et al., 2022; Saatmann et al., 2021; Hedt et al., 2022). For example, there is evidence that exercise-provoked LMS could facilitate morphological and functional adaptations in remote muscle tissues (Spiering et al., 2009; Curovic et al., 2024; Cook et al., 2014; Rønnestad et al., 2011; Spillane et al., 2015), and offer a potential for therapeutic influence, significantly reducing pain and functional disability in athletes with chronic non-specific low back pain (Liu et al., 2024).
Exercise focused on specific muscle groups in close proximity to each other produce hypoxia-induced LMS without overburdening the cardiovascular system (Goto et al., 2005; Feng et al., 2024; Martin et al., 2022), potentially making it accessible to recreational individuals. Moreover, unlike athletes who aim to optimise maximal strength and power gains (Van Hooren et al., 2024), recreational people and older adults may benefit from lighter loads to minimise the risk of injury with the reduced strain on connective tissues (Weakley et al., 2023; Bemben, 1998). While low-load training to failure may cause more discomfort than training with heavy loads (Fisher and Steele, 2017), stopping just short of failure may be better tolerated with light loads (de Lemos Muller et al., 2019), and might be more sustainable compared to HIIT protocols (Roy et al., 2018). Therefore, the purpose of this narrative review is to explore the role of resistance exercise-induced LMS in promoting systemic health and functional adaptations and evaluate the usefulness of resistance training modalities to maximise local-to-systemic endocrine responses.
2 Resistance exercise, metabolic stress and secretory factors
As mentioned above, LMS arises when oxygen availability is insufficient in the exercised muscles, leading to a greater reliance on glycolysis for ATP production (de Freitas et al., 2017; Hwang and Willoughby, 2019). In this scenario, sustained contractions create a localised shift from aerobic to anaerobic conditions, resulting in the accumulation of metabolites that exert influence on both local and distant muscle cells, neurons, and organs (Brooks et al., 2023; Huang et al., 2021; Rønnestad et al., 2011; Godfrey et al., 2009; Madarame et al., 2008). Therefore, to elicit a metabolic response in targeted muscles, resistance exercise must induce anaerobic conditions (de Freitas et al., 2017; Lawson et al., 2022; Robergs et al., 2004; Goto et al., 2005). However, heavy loads do not place significant demands on the glycolytic system because they primarily rely on phosphocreatine for maximal, short-duration contractions (de Poli et al., 2019; Hargreaves and Spriet, 2020; Krisanda et al., 1988). This is due to the faster onset of volitional failure caused by the inability to sustain high levels of type 2 fibre recruitment necessary for intense contractions (Krisanda et al., 1988; Hultman and Greenhaff, 1991). In contrast, when a muscle is actively performing against lighter loads, the primary physiological mechanisms for energy requirements arise from oxidative and glycolytic pathways (Lawson et al., 2022; Krisanda et al., 1988). Indeed, according to Henneman’s size principle, motor units are activated in a sequential order starting from smaller, oxygen-dependent type 1 fibres, followed by the larger glycolytic type 2 fibres that are progressively engaged to compensate for the declining force output with fatigue advancement (Wernbom and Aagaard, 2020). Alternatively, when exercise is performed with high velocity against light resistance, the central nervous system can prime the muscle with maximal intent to achieve the greatest possible contraction speed, overriding the typical size principle recruitment sequence (Sale, 1988; Maffiuletti et al., 2016). This process involves a high neural discharge rate, which allows for the rapid activation of type 2 fibres (Maffiuletti et al., 2016; Aagaard et al., 2002), where velocity is prioritised over force and contractions slow down relatively quickly (Boccia et al., 2024). Despite the differences in these recruitment dynamics, the end result of using light resistance remains similar if repetitions are performed until near the failure point – elevated LMS facilitating a complete muscle fibre activation (Lawson et al., 2022; Morton et al., 2019).
In simplified physiological terms, as exercise duration extends, anaerobic glycolysis becomes increasingly engaged to meet energy demands, outpacing oxidative phosphorylation and phosphagen system (Krisanda et al., 1988; Koopman et al., 2006; Koh et al., 2022). This shift results in a transient mismatch between the rate of pyruvate production and its utilisation by aerobic mechanisms (Melkonian and Schury, 2024). During anaerobic glycolysis, pyruvate is converted into lactate which is then used to regenerate nicotinamide adenine dinucleotide (NAD) from its reduced form (NADH) to the oxidised form (NAD+), thereby allowing the energy production process to continue (Melkonian and Schury, 2024). This regeneration is vital for sustaining glycolysis during high-intensity activity (Melkonian and Schury, 2024). However, as muscle contractions persist, NADH levels increase, eventually overwhelming the capacity of lactate to remove hydrogen ions (Melkonian and Schury, 2024). Consequently, the disruption in the pH balance (i.e., muscle acidosis) becomes one of the main limiting factor of muscular contractions (Melkonian and Schury, 2024; Theofilidis et al., 2018). The local hypoxic conditions achieved initiate a whole cascade of events, including the release of various molecules and anabolic hormones (Goto et al., 2005; Hwang and Willoughby, 2019; Hjortshoej et al., 2023), intracellular signalling pathways (Lixandrão et al., 2018), and altered gene expression with satellite cell activity (Goto et al., 2005; Hwang and Willoughby, 2019; Hjortshoej et al., 2023). Notably, these pathways are not only facilitated by LMS in isolation, but also by the mechanical tension inflicted on type II fibres in the anaerobic environment (Lawson et al., 2022). Indeed, if a muscle group is exercised to fatigue, the former mechanism (i.e., metabolic stress) eventually makes the latter mechanism possible (i.e., mechanical tension) (Lawson et al., 2022; Wernbom and Aagaard, 2020), providing a tandem for maximising endocrine responses (Lawson et al., 2022; Cook et al., 2014; Madarame et al., 2008; Jakobsson et al., 2021; Bartolomei et al., 2018; Rahimi et al., 2010). This is supported by findings showing that fatigue-provoking low-load exercise effectively replicates the endogenous production of key anabolic and stress-related factors seen with heavier loads, including the vascular endothelial growth factor and nitric oxide (Hjortshoej et al., 2023). On the other hand, high resistance exercise with longer breaks is limited to the mechanical tension and accompanying molecules (Linnamo et al., 2005), while it fails to perturb the intracellular environment (Hultman and Greenhaff, 1991). Thus, muscle satellite cells, pro-inflammatory cytokines, and immune cells are stimulated to a greater extent by light-to-moderate resistance training in close proximity to failure compared to traditional strength training with heavy loads (Weakley et al., 2023; Rossi et al., 2018). As a result, multiple remodelling factors are released into the bloodstream including ROS, inflammatory markers, microRNAs, and other myokines (Zunner et al., 2022; Wang et al., 2018; Ferraro et al., 2014; Patterson et al., 2013) (Table 1).
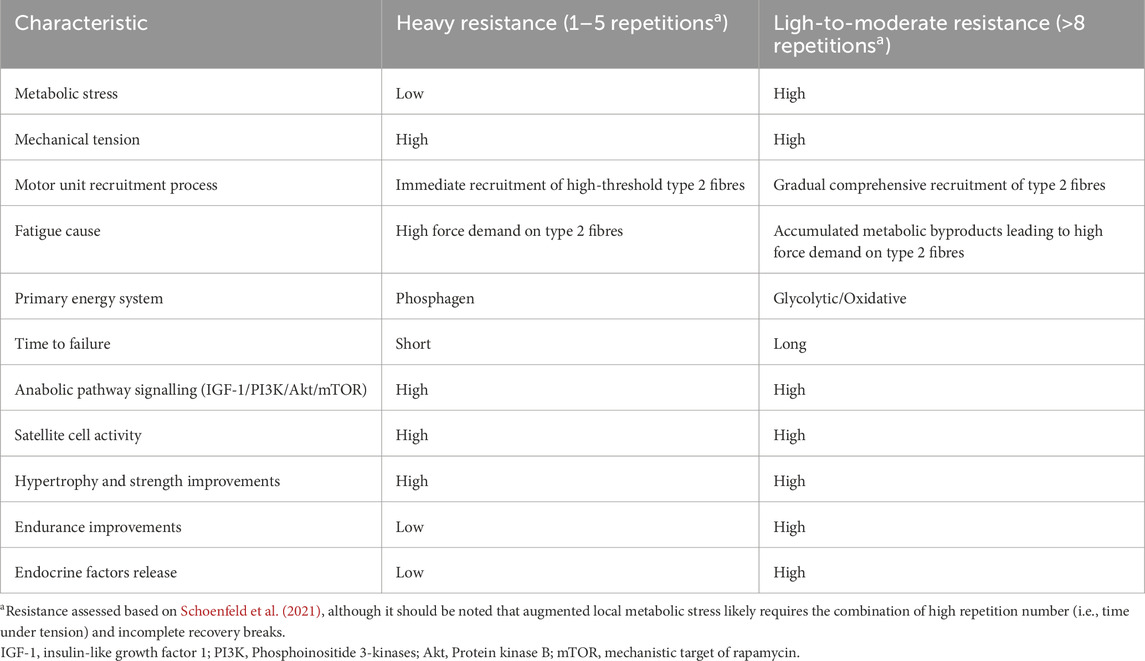
Table 1. Comparison of two different resistance exercise modalities taken to (near) the failure points.
One important molecule associated with metabolic demands but not with mechanical tension per se is lactate, a myokine with autocrine, paracrine and endocrine functions (Lawson et al., 2022; Brooks et al., 2023; Brooks et al., 2022a). Once the lactate production surpasses cellular capacity, it is shuttled into the bloodstream from where it affects multiple tissues and hormones (Brooks et al., 2023; Huang et al., 2021; Nalbandian and Takeda, 2016). Systemic blood lactate levels consistently rise following BFR resistance training with low loads (Fujita et al., 2007; Li et al., 2023), and the associated drop in pH stimulates sympathetic nerve activity, enabling the secretion of growth hormone (Godfrey et al., 2009). Although the LMS triggers a significant release of other anabolic hormones as well (Kraemer and Ratamess, 2005; Fujita et al., 2007; Seo et al., 2016; Manini et al., 2012), the association between testosterone and lactate accumulation is unclear (Walker et al., 2011; Weakley et al., 2017). This could be due to the higher intensity thresholds needed for testosterone [(≥75% of one repetition maximum (1-RM)] (Rahimi et al., 2010; Linnamo et al., 2005) compared to lower resistances (∼60% of 1-RM) (Weakley et al., 2017). In the absence of BFR, regional metabolic perturbations could be provoked by the activation of large muscle groups in close proximity to each other, utilising light-to-moderate loads (Kelleher et al., 2010; Realzola et al., 2022) and short breaks (Kraemer and Ratamess, 2005; Senna et al., 2022; Takarada and Ishii, 2002; Schoenfeld, 2011; Krzysztofik et al., 2019). For example, reciprocal supersets (i.e., paired exercises for agonist and antagonist muscles performed without rest in between) have demonstrated greater effects on energy expenditure, blood lactate levels, and post-exercise oxygen consumption compared to traditional sets with longer rest periods (Zhang et al., 2025; Kelleher et al., 2010; Realzola et al., 2022). In these studies, exercises were paired for the muscles from the opposite side (e.g., leg extension and leg curl) with the equated volume between the training groups (Kelleher et al., 2010; Realzola et al., 2022). Notably, a study that compared supersets involving three consecutive exercises, two consecutive exercises, and a traditional exercise protocol resulted in significantly higher lactate concentrations for the superset groups, particularly for those with more exercises in a row (Weakley et al., 2017). Moreover, supersets involving exercises for the same muscle group (i.e., flat bench press immediately followed by the incline bench press), have been shown to exhibit similar lactate concentration as observed in “pre-exhaustion” and “forced repetitions” groups despite lower total training volume (Wallace et al., 2019). In contrast, a study that used elastic bands to train elbow flexors and extensors found no significant differences in metabolic and mechanical stress markers between reciprocal supersets and traditional 1-min rest intervals (Fink et al., 2021). This discrepancy was likely due to insufficient exercise intensity and the absence of local fatigue in the trained participants (Kraemer and Ratamess, 2005). Therefore, high volume of fatiguing exercises seems necessary to elicit substantial metabolic and hormonal systemic responses (Curovic et al., 2024).
In summary, it appears that LMS can be induced in two ways – either via high volume of fatiguing exercises using low-to-moderate loads and short breaks (Schoenfeld et al., 2017; Fink et al., 2021; Lasevicius et al., 2022; Lacerda et al., 2016), or by BFR applied to the limbs allowing for lighter loads to efficiently facilitate the hypoxic environment (Lixandrão et al., 2018; Hwang and Willoughby, 2019; Krzysztofik et al., 2019). These anaerobic conditions are crucial for the production of metabolic byproducts that drive the adaptations leading to improved strength and hypertrophy (Sødal et al., 2023; Keskin et al., 2025; Kurobe et al., 2015), muscular endurance (Goto et al., 2005; Keskin et al., 2025; Aguiar et al., 2015), cardiometabolic health (Feng et al., 2024; Martin et al., 2022; Saatmann et al., 2021; Hjortshoej et al., 2023; Acosta-Manzano et al., 2020; Zhou et al., 2024; Edwards et al., 2022) and systemic fat tissue reduction (Ramírez-Campillo et al., 2013).
3 Local metabolic stress and functional adaptations
3.1 Local metabolic stress and endurance
Endurance can be defined as an ability to sustain physical exertion over time (Hughes et al., 2018; Schoenfeld et al., 2021). Aerobic endurance exercise relies on oxygen utilisation for energy production, typically involving whole-body movements like running (Patel et al., 2017). Anaerobic (lactic) endurance, on the other hand, occurs when exercise is performed in the absence of sufficient oxygen supply (Patel et al., 2017; Poole et al., 2021), training the body to withstand the metabolic stress (Fiorenza et al., 2018; Patel et al., 2017; Poole et al., 2021; Gastin, 2001; Haugen et al., 2021). This type of endurance can be trained via high-intensity whole-body movements (Poole et al., 2021; Haugen et al., 2021), or via fatiguing muscle contractions imposed on a targeted muscle group (i.e., muscular endurance) (Fisher J et al., 2020; Hackett et al., 2022). Crucially, from the perspective of systemic versus local adjustments, both aerobic and anaerobic whole-body trainings predominantly drive cardiorespiratory adaptations (Farrell et al., 2018; Patel et al., 2017; Poole et al., 2021; Haugen et al., 2021), whereas local anaerobic (i.e., muscular) endurance training primarily imposes muscular adaptations (Farrell et al., 2018; Acosta-Manzano et al., 2020; Fisher J et al., 2020; Hackett et al., 2022). Hence, to differentiate between these categories, they can be referred to as either “systemic aerobic endurance,” “systemic anaerobic endurance” or “local anaerobic (i.e., muscular) endurance.” A challenge emerges, however, if an individual trains multiple upper and lower body muscle groups within the same session following the principles of muscular endurance training guidelines (Triplett and Haff, 2015). In this case, the session could become reliant on the cardiorespiratory system to deal with the energy needs and limit the capacity of separate muscles to perform due to the development of supraspinal fatigue (Taylor et al., 2016; Tanaka and Watanabe, 2012). In fact, it can be proposed that LMS could be strategically manipulated to either optimise local anabolic pathways, enhancing the local muscle growth, strength and muscular endurance (Schoenfeld, 2013), or to facilitate systemic aerobic adaptations by being provoked in separate muscle regions interchangeably, thereby requiring notable post-exercise oxygen consumption (Greer et al., 2021). A meta-analysis comparing HIIT protocols with and without BFR showed that BFR augmented both peripheral and central physiological adaptations, thereby enhancing not only muscular endurance but also the aerobic capacity (Yin et al., 2025). However, while these improvements were likely driven by the elevation of LMS facilitating increases in capillarization, mitochondrial density, cardiac output, and acid-buffering capacity (Yin et al., 2025), the included studies primarily employed intensive running or cycling configurations rather than resistance-based protocols that are less likely to elicit substantial heart rate alterations.
Although vague in its definition, muscular endurance is inherently anaerobic and focuses on repetitive contractions against resistance in hypoxic environment associated with the LMS (de Freitas et al., 2017; Kahraman and Varol, 2024; Schoenfeld et al., 2021; Hackett et al., 2022; Deschenes and Kraemer, 2002). In this context, it must be acknowledged that this type of local endurance encompasses not only the capacity to sustain prolonged efforts against light loads (Schoenfeld et al., 2021) (i.e., “muscular endurance”), but also against moderate loads (i.e., “strength-endurance”) (Fisher J et al., 2020), including the ability to perform with incomplete recovery between sets as well as to complete multiple high-volume sets (Zhang et al., 2025; Fink et al., 2021; Triplett and Haff, 2015). It is, therefore, nearly impossible to establish universal guidelines for training this ability based solely on the number of repetitions or rest durations. That said, standard recommendations differentiate between protocols for muscular endurance and hypertrophy, with the former involving shorter rest intervals (30–60 s), and higher repetition ranges (10–25), and the latter involving longer rest intervals (1–3 min) and fewer repetitions (8–12) (American College of Sports Medicine, 2021). However, hypertrophy can occur equally well with “muscular endurance training” (Schoenfeld et al., 2017; Lasevicius et al., 2022; Fink et al., 2018; Fink et al., 2017), suggesting that muscular endurance could also benefit from “hypertrophy training” (Aguiar et al., 2015). For instance, performing a single exhaustive set at 20% of 1-RM prior to traditional hypertrophy sessions has been shown to significantly enhance both muscular endurance and muscle growth (Aguiar et al., 2015). Furthermore, a systematic review on advanced resistance training techniques indicated that establishing specific guidelines for volume, intensity of effort, and frequency is challenging, as each of these methods appears to maximise muscle responses through the combined effects of mechanical and metabolic stress (Krzysztofik et al., 2019).
It would be unrealistic to expect significant aerobic benefits from local anaerobic conditions due to the cardiorespiratory system not being a primary limiting factor following this type of training (Acosta-Manzano et al., 2020). Notably, however, there are some findings suggesting that an adequate local anaerobic stimulus could simulate exercise modalities that lead to aerobic improvements by imposing significant demands on the cardiorespiratory system (Zhou et al., 2024; Hong et al., 2024). This was illustrated in a study by Hong et al. (2024) which showed that resistance exercise involving large muscle groups such as squats could promote aerobic gains through increased oxygen demands when multiple sets of 10 repetitions were performed (Hong et al., 2024). Similarly, greater metabolic demands in superset configurations have been linked to the elevated post-exercise oxygen consumption (Kelleher et al., 2010; Realzola et al., 2022), potentially triggering aerobic advancements. In agreement, a systematic review with meta-analysis revealed that both muscular endurance and hypertrophy-focused training led to improved cardiorespiratory fitness (Acosta-Manzano et al., 2020). These findings collectively show that when large muscle groups are exercised with a LMS approach, they can contribute to systemic aerobic adaptations, although the extent and consistency of those effects may be limited (Farrell et al., 2018). For example, complementing an aerobic endurance programme with local muscular endurance exercise did not enhance VO2 max in active individuals, though it did increase VO2 max at the onset of blood lactate accumulation (Farrell et al., 2018).
In summary, repeatedly imposed LMS can lead to improved muscular endurance, a term that should be used to describe resistance to fatigue not only with light loads, but also with moderate loads (i.e., strength-endurance) concerning both the contractions to failure and the ability to perform with incomplete breaks. Its role in promoting systemic (aerobic) endurance may be less impactful, but not without positive effects for recreational populations, particularly if different body regions are simultaneously trained.
3.2 Local metabolic stress indirectly leads to hypertrophy and strength with decreasing loads
Complex mechanisms are being increasingly acknowledged as important mediators of hypertrophy, including mTORC1 signaling, myonuclear accretion (i.e., fusion of satellite cells with the existing muscle fibre), microRNA stimulation, enhanced angiogenesis (i.e., formation of new blood vessels), gene expression, mitochondrial biogenesis and similar (Roberts et al., 2023). Unfortunately, the extent to which these mechanisms can be triggered directly by the LMS is unclear. As previously noted, when resistance exercise with light and moderate loads is performed with the close proximity to failure, it can lead to equal hypertrophy gains as exercise with heavier loads (Lixandrão et al., 2018; Keskin et al., 2025; Schoenfeld et al., 2017; Zhang et al., 2025; Farup et al., 2015; Lim et al., 2019) and comparable strength gains as seen with traditional strength training (Weakley et al., 2023; Lim et al., 2019; Grønfeldt et al., 2020). The consistent finding that BFR training with very light loads (∼30% of 1-RM) achieves similar results (Lixandrão et al., 2018; Farup et al., 2015), jointly underlines the contribution of metabolite accumulation to those outcomes (Pearson and Hussain, 2015). The role of the LMS for hypertrophy and strength adaptations may be quite simply explained via increased neural drive to the exercising muscle’s type 2 fibres (Conwit et al., 1999; Morton et al., 2016). As the fatigue develops in oxidative fibres, maintaining the same effort requires recruiting additional motor units thereby activating high-threshold fibres (Sundstrup et al., 2012). This expanded recruitment allows more fibres to experience mechanical tension necessary for hypertrophy (Lawson et al., 2022; Wackerhage et al., 2019) under the condition that repetitions are performed until near the failure points (Schoenfeld et al., 2017; Robinson et al., 2024; Lasevicius et al., 2022). Hence, significant hypertrophy gains with light loads would be impossible to occur if there was no metabolic stress to facilitate this process (Hwang and Willoughby, 2019; Wernbom and Aagaard, 2020; Krzysztofik et al., 2019). On the other hand, strength, being primarily a neuromuscular quality (Triplett and Haff, 2015), may depend more on the intensified voluntary activation with high-force, low-fatigue contractions compared to the high-volume of fatiguing sets (Robinson et al., 2024), as the latter may reduce the efficiency of rapid motor unit recruitment in the subsequent sets (Suchomel et al., 2018).
It should be noted that the occurrence of fatigue aligned with an emphasised LMS may diminish the additional hypertrophic stimuli in the later sets (Nordlund et al., 2004). The main critics of this training modality point out that shorter rest periods and exercise to failure might reduce the total training volume, which is a valid concern. However, since volume is defined as the number of sets multiplied by the number of repetitions, fatigue in the exercising muscles can be manipulated by using lighter loads later in the session (Iversen et al., 2021). In fact, repetitions with submaximal loads performed under the hypoxic local environment yield greater hypertrophic response compared to those performed short of inducing fatigue despite the same total volume (Karsten et al., 2021). Thus, shortening rest periods can be an effective method for creating and maintaining local anaerobic conditions, allowing for more stimulating repetitions. In agreement, time-efficient training strategies with lighter resistance and shorter breaks lead to gains equal to traditional strength training (Zhang et al., 2025; Krzysztofik et al., 2019; Iversen et al., 2021). For example, Farup et al. (2015) examined muscular adaptations following resistance programme with loads around 40% of 1-RM involving 4 sets of unilateral dumbbell curls to failure with the only difference between arms being BFR applied to one limb. Despite the BFR arm performing less repetitions per set (resulting in lower total volume and training time), muscle size increased similarly in both limbs after the programme (Farup et al., 2015). That said, it should be noted that resistance below 20% of 1-RM may be suboptimal (Ozaki et al., 2016; Lasevicius et al., 2018) due to the inefficiency of the muscle to trap metabolites inside the cell (de Freitas et al., 2017; Lasevicius et al., 2018). In this scenario, employing a superset configuration may be helpful to limit the recovery of oxygen availability in the exercising muscles (Weakley et al., 2017; Kelleher et al., 2010; Realzola et al., 2022; Fink et al., 2018). It is also important to highlight that exercising to failure does not appear superior to stopping just short of failure (Grgic et al., 2022), though close proximity looks essential with low loads (Lasevicius et al., 2022). It would be interesting to examine whether the combination of the reciprocal superset and drop set concept (e.g., fatiguing repetitions including a moderate load for agonist followed by the moderate load for antagonist + light load for the same agonist + light load for the same antagonist), followed by longer inter-superset periods, could augment the hypertrophic stimulus in the selected body region and result in the completed session within a shorter timeframe. Notably, tolerance to this method could vary based on the training experience, making it more suitable for highly trained individuals.
3.2.1 Local metabolic stress facilitates vertical strength transfer
Although endogenous hormonal elevations may play a less significant role for the facilitation of strength and hypertrophy than previously believed (Loenneke et al., 2011; West et al., 2010), the role of metabolites should not be dismissed (Lawson et al., 2022; Curovic et al., 2024). Indeed, studies indicate that intramuscular hypoxia manifested by elevated lactate concentrations, oxygenated hemoglobin (Goto et al., 2005) and growth hormone (Kurobe et al., 2015) directly stimulates muscular hypertrophy (Goto et al., 2005; Kurobe et al., 2015; Aguiar et al., 2015), and that external infusion of lactate in mice results in molecular signaling linked to hypertrophy independent of an exercise stimulus (Cerda-Kohler et al., 2018). Interestingly, locally produced endocrine factors associated with metabolic stress may enter the circulation and contribute to the whole-body anabolic adaptations via neurophysiological mechanisms (Brooks et al., 2023; Curovic et al., 2024; Nalbandian and Takeda, 2016; Quistorff et al., 2008). These circulating exerkines and exosomes include lactate (Brooks et al., 2023; Nalbandian and Takeda, 2016; Quistorff et al., 2008), which can promote neuroplasticity and reduce intracortical inhibition, thereby increasing the firing rate of neurons (Huang et al., 2021; Xue et al., 2022; Andrews et al., 2020), followed by ROS (Centner et al., 2018), microRNA (Domańska-Senderowska et al., 2019), and follistatin (Bagheri et al., 2019; Tortoriello et al., 2001), a glycoprotein that suppresses muscle-wasting myostatin (Laurentino et al., 2012). For example, when legs are exercised with the goal of provoking high metabolic response sufficient to perturb systemic circulation, upper body muscles trained separately in the session experience amplified strength and hypertrophy adaptations (May et al., 2018; Cook et al., 2014; Rønnestad et al., 2011; Madarame et al., 2008; Bartolomei et al., 2018; Hansen et al., 2001), though not in all instances (Jakobsson et al., 2021; West et al., 2010). While findings on strength augmentation in the muscle trained before resistance exercise-induced LMS from another body region are conflicting (May et al., 2018; West et al., 2010; Hansen et al., 2001), other studies consistently suggest a strength-enhancing effect when a targeted muscle is trained subsequently to the elevated LMS (Spiering et al., 2009; Rønnestad et al., 2011; Spillane et al., 2015). For example, it has been demonstrated that upper body resistance training session with high metabolic and hormonal perturbations effectively potentiated androgen receptors in the subsequently exercised lower body (Spiering et al., 2009; Spillane et al., 2015), implying a strength-augmenting effect for the leg muscle (Kraemer and Ratamess, 2005). Remarkably, this type of metabolically challenging upper body resistance training could offer protection for the leg muscle morphology and cycling power when positioned after exhaustive endurance running sessions on the same day (Kraemer et al., 1995). This phenomenon, recently named as “vertical strength transfer” (Curovic et al., 2024), explains that strength-trained muscles (i.e., high resistance, low volume) from one body region (e.g., upper body) may be positively influenced by high-volume metabolic exercise from remote body regions (e.g., lower body) through the triggered differential intramuscular signaling, leading to greater hypertrophy and muscle remodeling (Kraemer et al., 1995; Moberg et al., 2021; Verney et al., 2008; Verney et al., 2006; Abaïdia et al., 2017). These neurophysiological effects are proposed to be enabled by the endocrine factors associated with the LMS (Spiering et al., 2009; Cook et al., 2014; Spillane et al., 2015; Madarame et al., 2008; Hansen et al., 2001; Kraemer et al., 1995) (Table 2). One possible explanation for the centrally mediated benefits of increased metabolic stress on strength and hypertrophy adaptations may involve a shift in neuronal firing towards preferential activation of type 2 muscle fibres even in remote muscle groups. This is supported by the study showing enhanced strength and muscular endurance in the leg muscles trained under systemically induced anaerobic conditions by normobaric hypoxic exposure (Manimmanakorn et al., 2013). This study revealed that resistance training with light loads under BFR was not superior compared to the group with systemically deprived oxygen availability because both led to substantial improvements compared to control (Manimmanakorn et al., 2013).
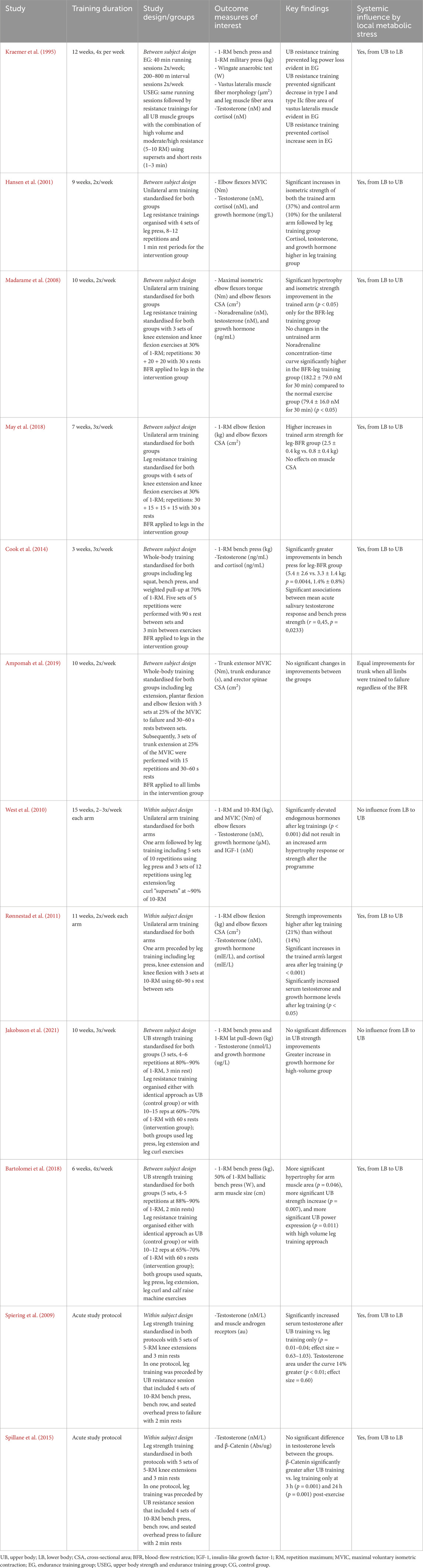
Table 2. Local metabolic stress and circulating endocrine factors influencing remote muscles via “vertical strength transfer” [table adapted by Curovic et al. (2024)]
In summary, training volumes concerning stimulating repetitions with the close proximity to failure may be the most important variable for hypertrophy and strength improvements with decreasing loads (Schoenfeld et al., 2017; Lasevicius et al., 2022; Lim et al., 2019; Morton et al., 2016; Karsten et al., 2021; Dankel et al., 2017; Ampomah et al., 2019), although reaching an absolute failure does not seem to be necessary (Grgic et al., 2022). Short rest periods, especially when using a very light resistance, or the strategic use of supersets or BFR, may be utilised to magnify the hypoxic local conditions that lead to greater levels of mechanical tension and local muscle growth (Weakley et al., 2017; Realzola et al., 2022; Fink et al., 2018; Fink et al., 2017). Strikingly, these conditions also contribute to anabolic and anti-catabolic adaptations in distant muscle cells, suggesting beneficial systemic influence on a whole-body level (Curovic et al., 2024). LMS-mediated strength and hypertrophy adaptations are likely explained via well-established physiological and biomechanical models such as the size principle and force-velocity relationship (i.e., slowing down of contractions near the failure point with high forces generated by high-threshold fibres) (Lawson et al., 2022; Wernbom and Aagaard, 2020; Schoenfeld, 2013; Myrholt et al., 2023). Nevertheless, maximal strength improvements still need to primarily rely on heavier loads (Schoenfeld et al., 2017), although untrained and recreationally active individuals could experience similar strength gains with lighter resistance under the aforementioned conditions (Grgic et al., 2018; Grønfeldt et al., 2020).
4 Local metabolic stress, immune system and health
Life-extending practices often involve controlled stressors that enhance resilience and adaptability through a process known as allostasis (Calabrese et al., 2024; Zhu et al., 2022). For instance, when the body is exposed to extreme cold or heat, it triggers the production of shock proteins and other molecules that protect cells and enhance their survival mechanisms (Patrick and Johnson, 2021; Kunutsor et al., 2024). Exercise-induced allostasis highlights that beneficial adaptations occur within a threshold of stress tolerance (Pearson and Hussain, 2015), explaining why moderate endurance activities tend to have linear associations with lifespan, while excessive number of long and intense whole-body endurance sessions does not always lead to better outcomes (O’Keefe et al., 2023; Franklin et al., 2020). Local anaerobic endurance training, however, may serve as a potent, yet manageable stimulus for several benefits associated with exercise stress. As suggested in previous sections, it could trigger both the mechanical stress pathways (associated with traditional strength training) and metabolic stress pathways (associated with endurance training) (Martin et al., 2022) that jointly lead to local and systemic endurance (Fink et al., 2021; Acosta-Manzano et al., 2020), strength and muscle growth (Lixandrão et al., 2018; Schoenfeld et al., 2017; Martin et al., 2022; Grønfeldt et al., 2020), and preserved health (Feng et al., 2024; Feng et al., 2024; Bemben, 1998; Chow et al., 2022; Saatmann et al., 2021; Zhu et al., 2022) (Figure 1). Indeed, this type of exercise may potently stimulate a comprehensive release of various myokines and exosomes (Goto et al., 2005; Coffey and Hawley, 2007; Hjortshoej et al., 2023; Huang et al., 2024; Guo et al., 2023) that have wide-ranging effects on immune system regulation (Afzali et al., 2018). Hence, it is somewhat surprising that, while strength (García-Hermoso et al., 2018; Li et al., 2018) and aerobic capacity (Strasser and Burtscher, 2018; Leslie et al., 2023) are well researched contributors to longevity, the associations concerning the local muscular (anaerobic) endurance capacity remain largely underexplored (Bemben, 1998; Acosta-Manzano et al., 2020) despite the noted potential (Goto et al., 2005; Kahraman and Varol, 2024; Farrell et al., 2018; Acosta-Manzano et al., 2020; Ramírez-Campillo et al., 2013; Wang et al., 2023).
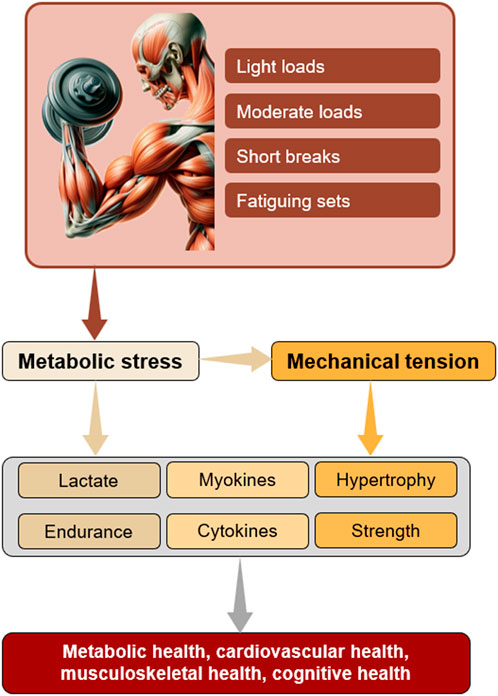
Figure 1. Proposed mechanisms through which locally induced metabolic stress promotes a broad spectrum of beneficial adaptations. Note that blood flow restriction enhances metabolic conditions, enabling the use of lighter loads.
4.1 Reactive oxygen species, cytokines and myokines
LMS-inducing exercise such as seen with BFR training, triggers adaptive mechanisms linked to various physiological effects (Hjortshoej et al., 2023). These involve enhanced muscle fibre recruitment, proliferation of myogenic satellite cells and angiogenesis, as well as acute hormonal, immune, and oxidative stress responses (Goto et al., 2005; Hjortshoej et al., 2023). All those effects synergistically contribute to exercise’s various benefits, including the mitigation of post-traumatic muscle atrophy (van Melick et al., 2016; Lepley et al., 2020), enhancement of neuromuscular activity (Curovic et al., 2024; Bartolomei et al., 2018; Cook et al., 2013), pain reduction (Liu et al., 2024; Hughes and Patterson, 2020; Korakakis et al., 2018), and increased bone mineral density (Jack et al., 2023). Several therapeutic myokines and exerkines are identified as a response to resistance training stimuli (Coffey and Hawley, 2007; Hjortshoej et al., 2023). Amongst them, ROS and cytokines stand out for their immune-regulating characteristics (Ferraro et al., 2014; Nelke et al., 2019). Indeed, findings suggest that exercise-provoked ROS and proinflammatory cytokines, otherwise damaging molecules, can actually promote positive tissue remodeling, supporting the overall immunity (Ferraro et al., 2014; Nelke et al., 2019). For example, taking antioxidant supplements post-training diminishes the beneficial effects of ROS-mediated cell signaling (Merry and Ristow, 2016), highlighting their contribution to improved endogenous antioxidant defenses. The importance of LMS for ROS production has been proposed by findings that highlight low-load BFR training superiority in elevating systemic ROS levels compared to traditional low-load or high-load exercise routines (Martin et al., 2022; Hjortshoej et al., 2023; Centner et al., 2018). Nonetheless, it appears that moderate resistance exercise, which generates greater mechanical stress, stimulates ROS production more effectively than light resistance without BFR (Goldfarb et al., 2008), likely via inflicted muscle damage (Owens et al., 2019; Powers et al., 2011). Although muscle damage and metabolic stress differ in their mechanisms (Martin et al., 2022), training to failure with minimal rest periods could be accompanied with some degree of microtears in the exercising fibres (Martin et al., 2022; Senna et al., 2022). Depending on the severity of this, the muscle’s recovery capacity could be impaired, though some portion of damage can actually benefit the body’s adaptive mechanisms (de Lemos Muller et al., 2019; Owens et al., 2019; Tee et al., 2007; Leal et al., 2018; Armstrong et al., 2012).
Metabolic stress may be one of the key factors behind the production of cytokines, the inflammatory markers associated with exercise’s health effects (Nelke et al., 2019). Interleukin (IL)-6 is released from muscles in response to an energy deficit (Kistner et al., 2022), with its levels rising in proportion to the exercise intensity and duration (Raschke and Eckel, 2013). As an energy sensor (Docherty et al., 2022), IL-6 is upregulated by low glycogen levels (Keller et al., 2001), playing a key role in the body’s metabolic responses (Docherty et al., 2022). Initially thought to be activated only by muscle damage (Patterson et al., 2013), recent studies show inconsistent findings regarding its stimulation with LMS being a potential candidate (Docherty et al., 2022; Pedersen and Febbraio, 2008). While IL-6 is generally a pro-inflammatory cytokine, it exhibits anti-inflammatory effects when stimulated by physical training (Huh, 2018; Muñoz-Cánoves et al., 2013). In addition to its immune-regulating role, IL-6 can improve systemic glucose and fat metabolism (Steinbacher and Eckl, 2015), restore muscle function, and mobilise satellite cells for reparation (Serrano et al., 2008). In contrast, when the whole body is exposed to a high-intensity training session with prolonged duration, this cytokine can jeopardise the immunity and promote muscle wasting (Muñoz-Cánoves et al., 2013). Thus, a possible alternative to frequent HIIT regimes may be found in exercises that induce stress only locally, helping to preserve IL-6’s immune-protective function. Still, this remains speculative and requires further investigation. Despite other interleukins being less researched (Docherty et al., 2022), a notable potential has also been identified with exercise-provoked IL-7 and IL-15, due to their outstanding role in enhancing the immune system (Nelke et al., 2019; Haugen et al., 2010; Duggal et al., 2018). These cytokines are associated with the restoration of 5′AMP-activated protein kinase (AMPK), a critical regulator of metabolic health (Coffey and Hawley, 2007) that declines with aging (Duggal et al., 2018), as well as with the development and maintenance of immature lymphocytes (Duggal et al., 2018). Further research is required to unveil the extent to which the LMS could affect and promote cytokines’ beneficial influence (Nelke et al., 2019).
Myokines are signaling proteins released by muscle cells in response to exercise (Zunner et al., 2022), playing a crucial role in regulating inflammation, metabolism (Laurens et al., 2020; Huh, 2018), muscle repair (Brandt and Pedersen, 2010), and overall health (Bucarey et al., 2024; Brandt and Pedersen, 2010; Leal et al., 2018; Gomarasca et al., 2020). As discussed in the previous sections, their release may be closely tied to the LMS, stimulated by “hypertrophy training” and “strength-endurance training” (Zunner et al., 2022). However, due to the lack of research on specific resistance exercise differences in augmenting myokine production (Bettariga et al., 2024), it is difficult to assess these associations. One interesting group of myokines modified by resistance exercise involves myostatin, follistatin and decorin (Zunner et al., 2022). Myostatin negatively affects muscle mass (Lee and June 2019), and it seems to be suppressed by resistance training-induced decorin and follistatin (Zunner et al., 2022; Bagheri et al., 2019; Khalafi et al., 2023; Willoughby et al., 2022). A single study that compared low-load BFR exercise to the heavy-load protocol revealed similar downregulation of myostatin in both instances (Laurentino et al., 2012). It may be the case that the suppression of this muscle-wasting signalling factor is aligned with the mechanical tension, and that BFR exercise was simply able to provoke enough (metabolic) stimulus for this to occur. Future research is needed to explore the extent of the regulatory influence exerted by various resistance exercise modes on these myokines, given their significant protective potential. For example, decorin has demonstrated the ability to inhibit angiogenesis and tumorigenesis (Baghy et al., 2020), while follistatin is linked to the healing of muscle injuries (Zhu et al., 2011).
Irisin is a myokine associated with both endurance and resistance exercise (Zunner et al., 2022), strongly corresponding to the AMPK activity and peroxisome proliferator-activated receptor gamma coactivator 1-alpha (PGC-1α) pathways, which are typically activated by endurance training (Zunner et al., 2022; Hjortshoej et al., 2023). Yet, this myokine also correlates with increased muscle mass and strength (Martínez Muñoz et al., 2019; Kim et al., 2016), suggesting a multifaceted beneficial impact. Irisin has demonstrated positive effects on metabolic and musculoskeletal health (Liu et al., 2021), as well as on the enhancement of cognitive function (Islam et al., 2021). To the author’s knowledge, only one study has compared the influence of LMS-inducing exercise (via BFR approach) against traditional strength training, finding greater concentrations in circulating irisin with the former modality (Kraemer et al., 2016). Further research is therefore warranted to explore the mechanisms underlying irisin’s stimulation.
Another myokine associated with both strength and endurance training is a “meteorite-like” molecule that indirectly supports adipocyte browning and mitochondrial health by enhancing immune cell function, while upregulating anti-inflammatory cytokines (Rao et al., 2014). It is closely connected to the PGC1a4, a significant subpart of a large PGC – 1α transcription group that also includes PGC-1α1. Both PGC-1α1 and PGC-1α4 are elevated in the system by the combination of resistance and vigorous aerobic exercise (Moberg et al., 2021; Hansson et al., 2019; Lundberg et al., 2014). While PGC-1α1 responds well to endurance training and improves aerobic adaptations (Agudelo et al., 2019; Mortensen et al., 2006), resistance training yields metabolic benefits via PGC-1α4, a cofactor that enhances glycolysis and ATP production in the muscle (Koh et al., 2022; Neto et al., 2023). PGC-1α group contributes to metabolic health (Neto et al., 2023) and prevention of pathophysiological diseases (Qian et al., 2024), possibly achieved by its overexpression in myotubes promoting fat oxidation and anaerobic glycolysis (Neto et al., 2023). The potential of the LMS to simultaneously target and stimulate the two isoforms of PGC-1α (PGC-1α4 and PGC-1α4) may be related to the dual influence of metabolite accumulation and mechanical tension. This needs to be explored through well-designed training studies targeting the elevation of the LMS and examining its effects on both transcriptional pathways.
Brain-derived neurotrophic factor (BDNF) is produced by various cells in the body (Zunner et al., 2022). When BDNF is stimulated by muscle contractions, it can assist in neuroprotection and prevent neurodegeneration (Palasz et al., 2020). This myokine may be potentiated by the heightened LMS during high-volume resistance exercise protocols. One study has shown that fatigue-provoking training consisting of 3 sets of 10 repetitions with 60-s rest intervals elicited a significantly greater increase (p < 0.01) in peripheral serum compared to 5 sets of 5 repetitions with 180-s rests (Marston et al., 2017). Notably, lactate and BDNF changes were positively correlated only after the local strength-endurance protocol (r = 0.70; p < 0.01), but not following a traditional strength training protocol (r = 0.18; p = 0.56) (Marston et al., 2017). This suggests that LMS could have played a facilitatory role in both direct and indirect stimulation of BDNF via enhanced conditions for mechanical tension (Lawson et al., 2022). In contrast, a separate study found that neither high-load nor moderate-load resistance training twice a week for 12 weeks affected peripheral growth factors (Marston et al., 2019). The authors proposed that higher frequency of high-volume sessions might be necessary for the enhancement of growth factors (Marston et al., 2019), although achieving this balance may be challenging for recreational individuals. It would be interesting to examine whether BFR could augment the changes seen in BDNF and upregulate its expression in response to resistance training stimulus.
4.2 Local metabolic stress and health effects
One of the most significant benefits of LMS may be aligned to improved cardiovascular health (Yang et al., 2019; Devereux, 2010; Zhou et al., 2024; Angelopoulos et al., 2023). Isometric resistance training causes greater blood pressure reductions than any other training modality (Owen et al., 2010; Inder et al., 2016; Edwards et al., 2023), with its intensity and associated local muscle fatigue closely linked to those effects (Devereux, 2010; Owen et al., 2010; Edwards et al., 2022; Inder et al., 2016; Devereux et al., 2012). Ischemic anaerobic conditions during isometric exercises (Robergs et al., 2004) trigger vasodilation and vascular adaptations proposed to enhance blood flow (Edwards et al., 2022). Consequently, the resting systolic blood pressure and mean arterial pressure are significantly reduced over time (Devereux, 2010; Edwards et al., 2022). These reductions are closely connected to the local fatigue measured by peak lactate, highlighting the importance of LMS in promoting hypotensive adaptations (Devereux et al., 2012). Interestingly, a study by Peters et al. (2006) suggested the role of ROS as mediating factors between the therapeutic influence of isometric training intervention for hypertensive adults and the significant blood pressure reductions reported (mean reduction 13 mm Hg, p < 0.05) (Peters et al., 2006). The authors attributed this effect to a decrease in oxidative stress (Peters et al., 2006), enabled by the ROS-dependent intracellular signaling that enhances endogenous antioxidant defenses (Sauer et al., 2001). A promising role of LMS to facilitate hypertension improvements was also suggested in a recent systematic review with meta-analysis (Feng et al., 2024) which showed that BFR training was able to significantly reduce blood pressure in middle-aged and elderly women (Feng et al., 2024). Furthermore, potential of the LMS adaptations to preserve cardiovascular health was observed in a longitudinal cohort study of 1,104 adult men, where a significant negative association was reported between the push-up capacity and cardiovascular disease incidence over 10 years (Yang et al., 2019). It is, therefore, reasonable to suggest that fatiguing low-resistance training does not only offer a safe alternative (Miller et al., 2021) to a heavy-resistance training (Martin et al., 2022) and extreme endurance training (O’Keefe et al., 2023; O’Keefe et al., 2015) for individuals with cardiovascular risks, but could also serve as a therapeutical intervention to target hypertensive conditions (Devereux, 2010; Feng et al., 2024).
Lactate is among the main mediators of health-promoting effects by local resistance exercise that triggers its accumulation (Lawson et al., 2022; Fiorenza et al., 2018; Nalbandian and Takeda, 2016; Fujita et al., 2007; Li et al., 2023; Kelleher et al., 2010; Realzola et al., 2022; Devereux et al., 2012). This metabolite acts as a signaling molecule shuttled to distant tissues (Brooks et al., 2023; Brooks et al., 2022a; Nalbandian and Takeda, 2016; Brooks et al., 2022b), where it exerts paracrine effects linked to the endogenous production of growth hormone, testosterone, cortisol, insulin-like growth factor 1, vascular endothelial growth factor, noradrenaline, and nitric oxide (Lawson et al., 2022; Brooks et al., 2023; Farrell et al., 2018; Rønnestad et al., 2011; Godfrey et al., 2009; Hjortshoej et al., 2023; Jakobsson et al., 2021; Cerda-Kohler et al., 2018). Lactate positively affects glucose metabolism (Saatmann et al., 2021; Acosta-Manzano et al., 2020), bone health (Gomarasca et al., 2020; Liu et al., 2021), and immune cell recruitment (Rossi et al., 2018), potentially even leading to protective effects on cancer occurrence (Zhu et al., 2022). Moreover, lactate is preferentially utilised as an energy resource by brain cells (Quistorff et al., 2008; Xue et al., 2022), where it supports neuroplasticity and cerebrovascular adaptability (Huang et al., 2021; Xue et al., 2022), thereby exerting neuroprotective effects (Bu et al., 2025; Huang et al., 2024; Deng et al., 2024; Vaynman and Gomez-Pinilla, 2006). Emerging evidence suggests that lactate metabolism beneficially links physical activity, gut microbiota and neurodegeneration, highlighting the need for further research to understand those interactions and develop interventions to mitigate the progression of neurological diseases (Rojas-Valverde et al., 2023). An interesting study by Blackmore et al. (2024) found that high-intensity exercise involving anaerobic zones significantly enhanced hippocampal function, preserved cortical volume, and strengthened neural network connectivity in aged individuals (Blackmore et al., 2024). These benefits, which persisted for at least 5 years, were associated with intensity-dependent changes in circulating biochemical markers, highlighting the need to investigate how aerobic-anaerobic transitions contribute to neuroprotection (Blackmore et al., 2024). Furthermore, lactates independently lead to molecular adaptations beneficial for muscle tissues (Cerda-Kohler et al., 2018), and their rise is associated with the downregulation of ghrelin (McCarthy et al., 2023), a finding that carries important implications for weight loss strategies via appetite suppression. Findings also suggest that LMS could enhance angiogenic gene expression (Larkin et al., 2012) and mitochondrial biogenic mRNA responses (Fiorenza et al., 2018), possibly due to the post-exercise elevations in muscle hemoglobin concentrations (Larkin et al., 2012). Future research is, therefore, necessary to further explore the impressive capacity of locally accumulated metabolites to influence a wide range of health benefits. This could be realised by allocating study participants to groups following either a traditional strength training protocol or LMS-inducing training protocol, whilst also factoring in the perceived effort with its possible negative effect on exercise performance in the subsequent days. This could be prevented by separating upper and lower body muscle group sessions (Curovic et al., 2024), thus allowing a longer recovery until progressive adaptations are achieved.
5 Conclusion and future perspectives
Resistance exercise-induced LMS promotes conditions for strength and hypertrophy improvements with light loads by enabling mechanical tension on type 2 fibres in the anaerobic intramuscular environment. The LMS-aligned protocols lead to improved muscular endurance and reductions in blood pressure, while also demonstrating a notable potential for the improvement of metabolic and cognitive health. The most efficient strategy for inducing local metabolic response appears to involve targeted resistance training for a selected body region using light-to-moderate loads with the close proximity to failure achieved by the contraction duration or repetition number and/or shortened rest periods. Additionally, BFR resistance training could serve as a potent alternative for the mentioned physiological responses due to accelerated metabolite accumulation (i.e., lactate) in muscles after the onset of contractions. Furthermore, the regional metabolic stress obtained by high-volume exercise of one body segment (e.g., lower body) could facilitate adaptations from isolate strength-trained muscles in remote body segments (e.g., upper extremities) via stimulated neurophysiological mechanisms (Curovic et al., 2024). Therefore, it may be the time to shift the focus from comparing “advanced training techniques” to traditional routines solely for (local) strength and hypertrophy improvements (Weakley et al., 2023; Curovic et al., 2024; Grønfeldt et al., 2020), and instead start evaluating their impact on a broader range of outcomes (Hjortshoej et al., 2023; Angelopoulos et al., 2023; Miller et al., 2021). Randomised controlled trials comparing LMS protocols with traditional strength training interventions could provide valuable insights into the magnitude of those effects via incorporating wide range of functional assessments (e.g., strength qualities, muscular endurance, aerobic power, cognitive processing tests) and biochemical circulating factors (e.g., lactates, PGC – 1α group, BDNF, irisin, decorin, folistatin, myostatin, interleukins) with their influence toward cardiometabolic adaptations (e.g., insulin sensitivity, lipid profile, blood pressure).
Author contributions
IC: Conceptualization, Data curation, Formal Analysis, Investigation, Methodology, Resources, Visualization, Writing – original draft, Writing – review and editing.
Funding
The author(s) declare that no financial support was received for the research and/or publication of this article.
Conflict of interest
The author declares that the research was conducted in the absence of any commercial or financial relationships that could be construed as a potential conflict of interest.
Generative AI statement
The authors declare that no Generative AI was used in the creation of this manuscript.
Publisher’s note
All claims expressed in this article are solely those of the authors and do not necessarily represent those of their affiliated organizations, or those of the publisher, the editors and the reviewers. Any product that may be evaluated in this article, or claim that may be made by its manufacturer, is not guaranteed or endorsed by the publisher.
References
Aagaard P., Simonsen E. B., Andersen J. L., Magnusson P., Dyhre-Poulsen P. (2002). Increased rate of force development and neural drive of human skeletal muscle following resistance training. J. Appl. Physiology 93, 1318–1326. doi:10.1152/japplphysiol.00283.2002
Abaïdia A.-E., Delecroix B., Leduc C., Lamblin J., McCall A., Baquet G., et al. (2017). Effects of a strength training session after an exercise inducing muscle damage on recovery Kinetics. J. Strength Cond. Res. 31, 115–125. doi:10.1519/JSC.0000000000001479
Acosta-Manzano P., Rodriguez-Ayllon M., Acosta F. M., Niederseer D., Niebauer J. (2020). Beyond general resistance training. Hypertrophy versus muscular endurance training as therapeutic interventions in adults with type 2 diabetes mellitus: a systematic review and meta-analysis. Obes. Rev. 21, e13007. doi:10.1111/obr.13007
Afzali A. M., Müntefering T., Wiendl H., Meuth S. G., Ruck T. (2018). Skeletal muscle cells actively shape (auto)immune responses. Autoimmun. Rev. 17, 518–529. doi:10.1016/j.autrev.2017.12.005
Agudelo L. Z., Ferreira D. M. S., Dadvar S., Cervenka I., Ketscher L., Izadi M., et al. (2019). Skeletal muscle PGC-1α1 reroutes kynurenine metabolism to increase energy efficiency and fatigue-resistance. Nat. Commun. 10, 2767. doi:10.1038/s41467-019-10712-0
Aguiar A. F., Buzzachera C. F., Pereira R. M., Sanches V. C., Januário R. B., da Silva R. A., et al. (2015). A single set of exhaustive exercise before resistance training improves muscular performance in young men. Eur. J. Appl. Physiol. 115, 1589–1599. doi:10.1007/s00421-015-3150-8
American College of Sports Medicine (2021). ACSM’s guidelines for exercise testing and Prescription. (12th ed). Philadelphia, PA: Wolters Kluwer.
Ampomah K., Amano S., Wages N. P., Volz L., Clift R., Ludin A. F. M., et al. (2019). Blood flow–restricted exercise does not induce a cross-transfer of effect: a randomized controlled trial. Med. and Sci. Sports and Exerc. 51, 1817–1827. doi:10.1249/MSS.0000000000001984
Andrews S. C., Curtin D., Hawi Z., Wongtrakun J., Stout J. C., Coxon J. P. (2020). Intensity Matters: high-intensity interval exercise enhances motor Cortex plasticity more than moderate exercise. Cereb. Cortex 30, 101–112. doi:10.1093/cercor/bhz075
Angelopoulos P., Tsekoura M., Mylonas K., Tsigkas G., Billis E., Tsepis E., et al. (2023). The effectiveness of blood flow restriction training in cardiovascular disease patients: a scoping review. J. Frailty Sarcopenia Falls 8, 107–117. doi:10.22540/JFSF-08-107
Armstrong R. B., Warren G. L., Warren J. A. (2012). Mechanisms of exercise-induced muscle fibre injury. Sports Med. 12, 184–207. doi:10.2165/00007256-199112030-00004
Atakan M. M., Li Y., Koşar Ş. N., Turnagöl H. H., Yan X. (2021). Evidence-based effects of high-intensity interval training on exercise capacity and health: a review with Historical perspective. Int. J. Environ. Res. Public Health 18, 7201. doi:10.3390/ijerph18137201
Bagheri R., Rashidlamir A., Motevalli M. S., Elliott B. T., Mehrabani J., Wong A. (2019). Effects of upper-body, lower-body, or combined resistance training on the ratio of follistatin and myostatin in middle-aged men. Eur. J. Appl. Physiol. 119, 1921–1931. doi:10.1007/s00421-019-04180-z
Baghy K., Reszegi A., Tátrai P., Kovalszky I. (2020). Decorin in the tumor Microenvironment. Adv. Exp. Med. Biol. 1272, 17–38. doi:10.1007/978-3-030-48457-6_2
Bartolomei S., Hoffman J. R., Stout J. R., Merni F. (2018). Effect of lower-body resistance training on upper-body strength adaptation in trained men. J. Strength Cond. Res. 32, 13–18. doi:10.1519/JSC.0000000000001639
Bemben M. G. (1998). Age-related alterations in muscular endurance. Sports Med. 25, 259–269. doi:10.2165/00007256-199825040-00004
Bemben M. G., Massey B. H., Bemben D. A., Misner J. E., Boileau R. A. (1996). Isometric intermittent endurance of four muscle groups in men aged 20-74 yr. Med. Sci. Sports Exerc 28, 145–154. doi:10.1097/00005768-199601000-00026
Bettariga F., Taaffe D. R., Galvão D. A., Lopez P., Bishop C., Markarian A. M., et al. (2024). Exercise training mode effects on myokine expression in healthy adults: a systematic review with meta-analysis. J. Sport Health Sci. 13, 764–779. doi:10.1016/j.jshs.2024.04.005
Blackmore D. G., Schaumberg M. A., Ziaei M., Belford S., To X. V., O'Keeffe I., et al. (2024). Long-term improvement in hippocampal-dependent Learning ability in healthy, aged individuals following high intensity interval training. Aging Dis. doi:10.14336/AD.2024.0642
Boccia G., D’Emanuele S., Brustio P. R., Rainoldi A., Schena F., Tarperi C. (2024). Decreased neural drive affects the early rate of force development after repeated burst-like isometric contractions. Scand. J. Med. Sci. Sports 34, e14528. doi:10.1111/sms.14528
Brandt C., Pedersen B. K. (2010). The role of exercise-induced myokines in muscle Homeostasis and the defense against chronic diseases. J. Biomed. Biotechnol. 2010, 520258. doi:10.1155/2010/520258
Brooks G. A., Arevalo J. A., Osmond A. D., Leija R. G., Curl C. C., Tovar A. P. (2022a). Lactate in contemporary biology: a phoenix risen. J. physiology 600, 1229–1251. doi:10.1113/JP280955
Brooks G. A., Curl C. C., Leija R. G., Osmond A. D., Duong J. J., Arevalo J. A. (2022b). Tracing the lactate shuttle to the mitochondrial reticulum. Exp. and Mol. Med. 54, 1332–1347. doi:10.1038/s12276-022-00802-3
Brooks G. A., Osmond A. D., Arevalo J. A., Duong J. J., Curl C. C., Moreno-Santillan D. D., et al. (2023). Lactate as a myokine and exerkine: drivers and signals of physiology and metabolism. J. Appl. Physiology 134, 529–548. doi:10.1152/japplphysiol.00497.2022
Bu A., Afghah F., Castro N., Bawa M., Kohli S., Shah K., et al. (2025). Actuating Extracellular Matrices Decouple the mechanical and biochemical effects of muscle contraction on motor neurons. Adv. Healthc. Mater. 14, 2403712. doi:10.1002/adhm.202403712
Bucarey J. L., Trujillo-González I., Paules E. M., Espinosa A. (2024). Myokines and their potential protective role against oxidative stress in metabolic Dysfunction-associated Steatotic liver disease (MASLD). Antioxidants 13, 1363. doi:10.3390/antiox13111363
Calabrese E. J., Nascarella M., Pressman P., Hayes A. W., Dhawan G., Kapoor R., et al. (2024). Hormesis determines lifespan. Ageing Res. Rev. 94, 102181. doi:10.1016/j.arr.2023.102181
Carapeto P. V., Aguayo-Mazzucato C. (2021). Effects of exercise on cellular and tissue aging. Aging (Albany NY) 13, 14522–14543. doi:10.18632/aging.203051
Centner C., Zdzieblik D., Dressler P., Fink B., Gollhofer A., König D. (2018). Acute effects of blood flow restriction on exercise-induced free radical production in young and healthy subjects. Free Radic. Res. 52, 446–454. doi:10.1080/10715762.2018.1440293
Cerda-Kohler H., Henríquez-Olguín C., Casas M., Jensen T. E., Llanos P., Jaimovich E. (2018). Lactate administration activates the ERK1/2, mTORC1, and AMPK pathways differentially according to skeletal muscle type in mouse. Physiol. Rep. 6, e13800. doi:10.14814/phy2.13800
Chow L. S., Gerszten R. E., Taylor J. M., Pedersen B. K., van Praag H., Trappe S., et al. (2022). Exerkines in health, resilience and disease. Nat. Rev. Endocrinol. 18, 273–289. doi:10.1038/s41574-022-00641-2
Coffey V. G., Hawley J. A. (2007). The molecular bases of training adaptation. Sports Med. 37, 737–763. doi:10.2165/00007256-200737090-00001
Conwit R. A., Stashuk D., Tracy B., McHugh M., Brown W. F., Metter E. J. (1999). The relationship of motor unit size, firing rate and force. Clin. Neurophysiol. 110, 1270–1275. doi:10.1016/s1388-2457(99)00054-1
Cook C. J., Kilduff L. P., Beaven C. M. (2014). Improving strength and power in trained athletes with 3 weeks of occlusion training. Int. J. sports physiology Perform. 9, 166–172. doi:10.1123/ijspp.2013-0018
Cook S. B., Murphy B. G., Labarbera K. E. (2013). Neuromuscular function after a bout of low-load blood flow-restricted exercise. Med. Sci. Sports Exerc 45, 67–74. doi:10.1249/MSS.0b013e31826c6fa8
Curovic I., Rhodes D., Alexander J., Harper D. J. (2024). Vertical strength transfer phenomenon between upper body and lower body exercise: systematic scoping review. Sports Med. 54, 2109–2139. doi:10.1007/s40279-024-02039-8
Dankel S. J., Jessee M. B., Mattocks K. T., Mouser J. G., Counts B. R., Buckner S. L., et al. (2017). Training to fatigue: the Answer for Standardization when assessing muscle hypertrophy? Sports Med. 47, 1021–1027. doi:10.1007/s40279-016-0633-7
de Freitas M. C., Gerosa-Neto J., Zanchi N. E., Lira F. S., Rossi F. E. (2017). Role of metabolic stress for enhancing muscle adaptations: practical applications. World J. Methodol. 7, 46–54. doi:10.5662/wjm.v7.i2.46
de Lemos Muller C. H., Ramis T. R., Ribeiro J. L. (2019). Effects of low-load resistance training with blood flow restriction on the perceived exertion, muscular resistance and endurance in healthy young adults. Sport Sci. Health 15, 503–510. doi:10.1007/s11332-019-00536-2
Deng T., Yu W., Lü Y. (2024). Different physical exercise in the treatment of Alzheimer’s disease. Psychogeriatrics 25, e13207. doi:10.1111/psyg.13207
de Poli R. de A. B., Roncada L. H., Malta E. de S., Artioli G. G., Bertuzzi R., Zagatto A. M. (2019). Creatine Supplementation improves phosphagen energy pathway during Supramaximal effort, but does not improve anaerobic capacity or performance. Front. Physiol. 10, 352. doi:10.3389/fphys.2019.00352
Deschenes M. R., Kraemer W. J. (2002). Performance and physiologic adaptations to resistance training. Am. J. Phys. Med. Rehabil. 81, S3–S16. doi:10.1097/00002060-200211001-00003
Devereux G. (2010) The effects of isometric exercise training on resting blood pressure with specific reference to selected cardiovascular, neuromuscular, and metabolic variables. PhD thesis, Kent, United Kingdom: Canterbury Christ Church University.
Devereux G. R., Coleman D., Wiles J. D., Swaine I. (2012). Lactate accumulation following isometric exercise training and its relationship with reduced resting blood pressure. J. Sports Sci. 30, 1141–1148. doi:10.1080/02640414.2012.692482
Docherty S., Harley R., McAuley J. J., Crowe L. A. N., Pedret C., Kirwan P. D., et al. (2022). The effect of exercise on cytokines: implications for musculoskeletal health: a narrative review. BMC Sports Sci. Med. Rehabilitation 14, 5. doi:10.1186/s13102-022-00397-2
Domańska-Senderowska D., Laguette M. J. N., Jegier A., Cięszczyk P., September A. V., Brzeziańska-Lasota E. (2019). MicroRNA profile and adaptive response to exercise training: a review. Int. J. sports Med. 40 (4), 227–235. doi:10.1055/a-0824-4813
Duggal N. A., Pollock R. D., Lazarus N. R., Harridge S., Lord J. M. (2018). Major features of immunesenescence, including reduced thymic output, are ameliorated by high levels of physical activity in adulthood. Aging Cell 17, e12750. doi:10.1111/acel.12750
Edwards J. J., Deenmamode A. H. P., Griffiths M., Arnold O., Cooper N. J., Wiles J. D., et al. (2023). Exercise training and resting blood pressure: a large-scale pairwise and network meta-analysis of randomised controlled trials. Br. J. Sports Med. 57, 1317–1326. doi:10.1136/bjsports-2022-106503
Edwards J. J., Wiles J., O’Driscoll J. (2022). Mechanisms for blood pressure reduction following isometric exercise training: a systematic review and meta-analysis. J. Hypertens. 40, 2299–2306. doi:10.1097/HJH.0000000000003261
Farrell J. W. I., Lantis D. J., Ade C. J., Cantrell G. S., Larson R. D. (2018). Aerobic exercise supplemented with muscular endurance training improves onset of blood lactate accumulation. J. Strength and Cond. Res. 32, 1376–1382. doi:10.1519/JSC.0000000000001981
Farup J., de Paoli F., Bjerg K., Riis S., Ringgard S., Vissing K. (2015). Blood flow restricted and traditional resistance training performed to fatigue produce equal muscle hypertrophy. Scand. J. Med. Sci. Sports 25, 754–763. doi:10.1111/sms.12396
Feng M., Li J., Zhao J., Pan X., Wang M., Han Q. (2024). Effect of blood flow restriction training on health promotion in middle-aged and elderly women: a systematic review and meta-analysis. Front. Physiol. 15, 1392483. doi:10.3389/fphys.2024.1392483
Ferraro E., Giammarioli A. M., Chiandotto S., Spoletini I., Rosano G. (2014). Exercise-induced skeletal muscle remodeling and metabolic adaptation: Redox signaling and role of Autophagy. Antioxid. Redox Signal 21, 154–176. doi:10.1089/ars.2013.5773
Fink J., Kikuchi N., Nakazato K. (2018). Effects of rest intervals and training loads on metabolic stress and muscle hypertrophy. Clin. Physiol. Funct. Imaging 38, 261–268. doi:10.1111/cpf.12409
Fink J., Schoenfeld B. J., Sakamaki-Sunaga M., Nakazato K. (2021). Physiological responses to agonist–antagonist superset resistance training. J SCI SPORT Exerc. 3, 355–363. doi:10.1007/s42978-020-00092-z
Fink J. E., Schoenfeld B. J., Kikuchi N., Nakazato K. (2017). Acute and long-term responses to different rest intervals in low-load resistance training. Int. J. Sports Med. 38, 118–124. doi:10.1055/s-0042-119204
Fiorenza M., Gunnarsson T. P., Hostrup M., Iaia F. M., Schena F., Pilegaard H., et al. (2018). Metabolic stress-dependent regulation of the mitochondrial biogenic molecular response to high-intensity exercise in human skeletal muscle. J. Physiol. 596, 2823–2840. doi:10.1113/JP275972
Fisher J. P., Steele J. (2017). Heavier and lighter load resistance training to momentary failure produce similar increases in strength with differing degrees of discomfort. Muscle Nerve 56, 797–803. doi:10.1002/mus.25537
Fisher J P., Steele J., Androulakis-Korakakis P., Smith D., Gentil P., Giessing J. (2020). The strength-endurance continuum revisited:a critical commentary of the recommendation ofdifferent loading ranges for different muscular adaptations. J. Trainology 9, 1–8. doi:10.17338/trainology.9.1_1
Franklin B. A., Thompson P. D., Al-Zaiti S. S., Albert C. M., Hivert M. F., Levine B. D., et al. (2020). Exercise-related acute cardiovascular events and potential Deleterious adaptations following long-term exercise training: Placing the risks into perspective–an update: a Scientific Statement from the American heart association. Circulation 141, e705–e736. doi:10.1161/CIR.0000000000000749
Fujita S., Abe T., Drummond M. J., Cadenas J. G., Dreyer H. C., Sato Y., et al. (2007). Blood flow restriction during low-intensity resistance exercise increases S6K1 phosphorylation and muscle protein synthesis. J. Appl. Physiol. 103, 903–910. doi:10.1152/japplphysiol.00195.2007
García-Hermoso A., Cavero-Redondo I., Ramírez-Vélez R., Ruiz J. R., Ortega F. B., Lee D. C., et al. (2018). Muscular strength as a predictor of all-cause mortality in an Apparently healthy population: a systematic review and meta-analysis of Data from Approximately 2 Million men and women. Arch. Phys. Med. Rehabil. 99, 2100–2113.e5. doi:10.1016/j.apmr.2018.01.008
Gastin P. B. (2001). Energy system interaction and relative contribution during maximal exercise. Sports Med. 31, 725–741. doi:10.2165/00007256-200131100-00003
Godfrey R. J., Whyte G. P., Buckley J., Quinlivan R. (2009). The role of lactate in the exercise-induced human growth hormone response: evidence from McArdle disease. Br. J. Sports Med. 43, 521–525. doi:10.1136/bjsm.2007.041970
Goldfarb A. H., Garten R. S., Chee P. D. M., Cho C., Reeves G. V., Hollander D. B., et al. (2008). Resistance exercise effects on blood glutathione status and plasma protein carbonyls: influence of partial vascular occlusion. Eur. J. Appl. Physiol. 104, 813–819. doi:10.1007/s00421-008-0836-1
Gomarasca M., Banfi G., Lombardi G. (2020). Myokines: the endocrine coupling of skeletal muscle and bone. Adv. Clin. Chem. 94, 155–218. doi:10.1016/bs.acc.2019.07.010
Goto K., Ishii N., Kizuka T., Takamatsu K. (2005). The impact of metabolic stress on hormonal responses and muscular adaptations. Med. Sci. Sports Exerc 37, 955–963. doi:10.1249/01.mss.0000170470.98084.39
Greer B. K., O’Brien J., Hornbuckle L. M., Panton L. B. (2021). EPOC Comparison between resistance training and high-intensity interval training in Aerobically Fit women. Int. J. Exerc Sci. 14, 1027–1035. doi:10.70252/ODIN6912
Grgic J., Schoenfeld B. J., Orazem J., Sabol F. (2022). Effects of resistance training performed to repetition failure or non-failure on muscular strength and hypertrophy: a systematic review and meta-analysis. J. Sport Health Sci. 11, 202–211. doi:10.1016/j.jshs.2021.01.007
Grgic J., Schoenfeld B. J., Skrepnik M., Davies T. B., Mikulic P. (2018). Effects of rest interval duration in resistance training on measures of muscular strength: a systematic review. Sports Med. 48, 137–151. doi:10.1007/s40279-017-0788-x
Grønfeldt B. M., Lindberg Nielsen J., Mieritz R. M., Lund H., Aagaard P. (2020). Effect of blood-flow restricted vs heavy-load strength training on muscle strength: systematic review and meta-analysis. Scand. J. Med. and Sci. Sports 30, 837–848. doi:10.1111/sms.13632
Guo L., Quan M., Pang W., Yin Y., Li F. (2023). Cytokines and exosomal miRNAs in skeletal Muscle–adipose crosstalk. Trends Endocrinol. and Metabolism 34, 666–681. doi:10.1016/j.tem.2023.07.006
Hackett D., Ghayomzadeh M., Farrell S., Davies T., Sabag A. (2022). Influence of total repetitions per set on local muscular endurance: a systematic review with meta-analysis and meta-regression. Sci. and Sports 37, 405–420. doi:10.1016/j.scispo.2021.11.002
Hansen S., Kvorning T., Kjaer M., Sjøgaard G. (2001). The effect of short-term strength training on human skeletal muscle: the importance of physiologically elevated hormone levels. Scand. J. Med. and Sci. sports 11, 347–354. doi:10.1034/j.1600-0838.2001.110606.x
Hansson B., Olsen L. A., Nicoll J. X., von Walden F., Melin M., Strömberg A., et al. (2019). Skeletal muscle signaling responses to resistance exercise of the elbow extensors are not compromised by a preceding bout of aerobic exercise. Am. J. physiology Regul. Integr. Comp. physiology 317, R83-R92–R92. doi:10.1152/ajpregu.00022.2019
Hargreaves M., Spriet L. L. (2020). Skeletal muscle energy metabolism during exercise. Nat. Metab. 2, 817–828. doi:10.1038/s42255-020-0251-4
Haugen F., Norheim F., Lian H., Wensaas A. J., Dueland S., Berg O., et al. (2010). IL-7 is expressed and secreted by human skeletal muscle cells. Am. J. Physiol. Cell Physiol. 298, C807–C816. doi:10.1152/ajpcell.00094.2009
Haugen M. E., Vårvik F. T., Larsen S., Haugen A. S., van den Tillaar R., Bjørnsen T. (2023). Effect of free-weight vs. machine-based strength training on maximal strength, hypertrophy and jump performance – a systematic review and meta-analysis. BMC Sports Sci. Med. Rehabil. 15, 103. doi:10.1186/s13102-023-00713-4
Haugen T., Sandbakk Ø., Enoksen E., Seiler S., Tønnessen E. (2021). Crossing the Golden training Divide: the Science and practice of training World-Class 800- and 1500-m Runners. Sports Med. 51, 1835–1854. doi:10.1007/s40279-021-01481-2
Hedt C., McCulloch P. C., Harris J. D., Lambert B. S. (2022). Blood flow restriction enhances rehabilitation and Return to Sport: the paradox of proximal performance. Arthrosc. Sports Med. Rehabil. 4, e51–e63. doi:10.1016/j.asmr.2021.09.024
Hickmott L. M., Chilibeck P. D., Shaw K. A., Butcher S. J. (2022). The effect of load and volume Autoregulation on muscular strength and hypertrophy: a systematic review and meta-analysis. Sports Med. - Open 8, 9. doi:10.1186/s40798-021-00404-9
Hjortshoej M. H., Aagaard P., Storgaard C. D., Juneja H., Lundbye-Jensen J., Magnusson S. P., et al. (2023). Hormonal, immune, and oxidative stress responses to blood flow-restricted exercise. Acta Physiol. 239, e14030. doi:10.1111/apha.14030
Hong S., Oh M., Oh C.-G., Lee H. D., Suh S. H., Park H., et al. (2024). Cardiorespiratory and aerobic demands of squat exercise. Sci. Rep. 14, 18383. doi:10.1038/s41598-024-68187-z
Huang K.-Y., Upadhyay G., Ahn Y., Sakakura M., Pagan-Diaz G. J., Cho Y., et al. (2024). Neuronal innervation regulates the secretion of neurotrophic myokines and exosomes from skeletal muscle. Proc. Natl. Acad. Sci. U. S. A. 121, e2313590121. doi:10.1073/pnas.2313590121
Huang Z., Zhang Y., Zhou R., Yang L., Pan H. (2021). Lactate as potential mediators for exercise-induced positive effects on neuroplasticity and cerebrovascular plasticity. Front. physiology 12, 656455. doi:10.3389/fphys.2021.656455
Hughes D. C., Ellefsen S., Baar K. (2018). Adaptations to endurance and strength training. Cold Spring Harb. Perspect. Med. 8, a029769. doi:10.1101/cshperspect.a029769
Hughes L., Patterson S. D. (2020). The effect of blood flow restriction exercise on exercise-induced hypoalgesia and endogenous opioid and endocannabinoid mechanisms of pain modulation. J. Appl. Physiol. 128, 914–924. doi:10.1152/japplphysiol.00768.2019
Huh J. Y. (2018). The role of exercise-induced myokines in regulating metabolism. Arch. Pharm. Res. 41, 14–29. doi:10.1007/s12272-017-0994-y
Hultman E., Greenhaff P. L. (1991). Skeletal muscle energy metabolism and fatigue during intense exercise in man. Sci. Prog. 75, 361–370.
Hwang P. S., Willoughby D. S. (2019). Mechanisms behind blood flow-restricted training and its effect toward muscle growth. J. strength Cond. Res. 33 (Suppl. 1), S167-S179–S179. doi:10.1519/JSC.0000000000002384
Inder J. D., Carlson D. J., Dieberg G., McFarlane J. R., Hess N. C., Smart N. A. (2016). Isometric exercise training for blood pressure management: a systematic review and meta-analysis to optimize benefit. Hypertens. Res. 39, 88–94. doi:10.1038/hr.2015.111
Islam M. R., Valaris S., Young M. F., Haley E. B., Luo R., Bond S. F., et al. (2021). Exercise hormone irisin is a critical regulator of cognitive function. Nat. Metab. 3, 1058–1070. doi:10.1038/s42255-021-00438-z
Iversen V. M., Norum M., Schoenfeld B. J., Fimland M. S. (2021). No time to Lift? Designing time-efficient training Programs for strength and hypertrophy: a narrative review. Sports Med. 51, 2079–2095. doi:10.1007/s40279-021-01490-1
Jack R. A., Lambert B. S., Hedt C. A., Delgado D., Goble H., McCulloch P. C. (2023). Blood flow restriction therapy preserves lower extremity bone and muscle mass after ACL Reconstruction. Sports Health 15, 361–371. doi:10.1177/19417381221101006
Jakobsson J., Theos A., Malm C. (2021). Effects of different types of lower body resistance exercise on upper-body strength in men and women, with special reference to anabolic hormones. Int. J. Exerc. Sci. 14 (3), 1052–1069. doi:10.70252/DYRH9028
Kahraman Y., Varol İ. (2024). Resistance training status detection via local muscular endurance adaptation maximum repetition strategy: Brief review. Sci. J. Sport Perform. 3, 160–169. doi:10.55860/swdb1533
Karsten B., Fu Y. L., Larumbe-Zabala E., Seijo M., Naclerio F. (2021). Impact of two high-volume set configuration Workouts on resistance training outcomes in recreationally trained men. J. Strength Cond. Res. 35, S136–S143. doi:10.1519/JSC.0000000000003163
Kelleher A. R., Hackney K. J., Fairchild T. J., Keslacy S., Ploutz-Snyder L. L. (2010). The metabolic costs of reciprocal supersets vs. traditional resistance exercise in young recreationally active adults. J. Strength Cond. Res. 24, 1043–1051. doi:10.1519/JSC.0b013e3181d3e993
Keller C., Steensberg A., Pilegaard H., Osada T., Saltin B., Pedersen B. K., et al. (2001). Transcriptional activation of the IL-6 gene in human contracting skeletal muscle: influence of muscle glycogen content. FASEB J. 15, 2748–2750. doi:10.1096/fj.01-0507fje
Keskin K., Gogus F. N., Gunay M., Fujita R. A. (2025). Equated volume load: similar improvements in muscle strength, endurance, and hypertrophy for traditional, pre-exhaustion, and drop sets in resistance training. Sport Sci. Health 21, 495–504. doi:10.1007/s11332-024-01281-x
Khalafi M., Aria B., Symonds M. E., Rosenkranz S. K. (2023). The effects of resistance training on myostatin and follistatin in adults: a systematic review and meta-analysis. Physiol. Behav. 269, 114272. doi:10.1016/j.physbeh.2023.114272
Kim H.-J., Lee H.-J., So B., Son J. S., Yoon D., Song W. (2016). Effect of aerobic training and resistance training on circulating irisin level and their association with change of body composition in overweight/obese adults: a pilot study. Physiol. Res. 65, 271–279. doi:10.33549/physiolres.932997
Kistner T. M., Pedersen B. K., Lieberman D. E. (2022). Interleukin 6 as an energy allocator in muscle tissue. Nat. Metab. 4, 170–179. doi:10.1038/s42255-022-00538-4
Koh J. H., Pataky M. W., Dasari S., Klaus K. A., Vuckovic I., Ruegsegger G. N., et al. (2022). Enhancement of anaerobic glycolysis - a role of PGC-1α4 in resistance exercise. Nat. Commun. 13, 2324. doi:10.1038/s41467-022-30056-6
Koopman R., Manders R. J. F., Jonkers R. A. M., Hul G. B. J., Kuipers H., van Loon L. J. C. (2006). Intramyocellular lipid and glycogen content are reduced following resistance exercise in untrained healthy males. Eur. J. Appl. Physiol. 96, 525–534. doi:10.1007/s00421-005-0118-0
Korakakis V., Whiteley R., Epameinontidis K. (2018). Blood Flow Restriction induces hypoalgesia in recreationally active adult male anterior knee pain patients allowing therapeutic exercise loading. Phys. Ther. Sport 32, 235–243. doi:10.1016/j.ptsp.2018.05.021
Kraemer R. R., Goldfarb A. H., Reeves G. V., Meachum W. A., Castracane V. D. (2016). Effects of partial vascular occlusion on irisin responses to loaded muscle contractions. Appl. Physiol. Nutr. Metab. 41, 332–334. doi:10.1139/apnm-2015-0464
Kraemer W., Patton J., Gordon S., Harman E. A., Deschenes M. R., Reynolds K., et al. (1995). Compatibility of high-intensity strength and endurance training on hormonal and skeletal muscle adaptations. J. Appl. Physiology 78, 976–989. doi:10.1152/jappl.1995.78.3.976
Kraemer W. J., Ratamess N. A. (2005). Hormonal responses and adaptations to resistance exercise and training. Sports Med. 35, 339–361. doi:10.2165/00007256-200535040-00004
Kraschnewski J. L., Sciamanna C. N., Poger J. M., Rovniak L. S., Lehman E. B., Cooper A. B., et al. (2016). Is strength training associated with mortality benefits? A 15year cohort study of US older adults. Prev. Med. 87, 121–127. doi:10.1016/j.ypmed.2016.02.038
Krisanda J., Moreland T., Kushmerick M. (1988). “ATP supply and demand during exercise,” in Exercise, nutrition, energy and metabolism (New York: Mc Millan), 27–44.
Krzysztofik M., Wilk M., Wojdała G., Gołaś A. (2019). Maximizing muscle hypertrophy: a systematic review of advanced resistance training techniques and methods. Int. J. Environ. Res. Public Health 16, 4897. doi:10.3390/ijerph16244897
Kunutsor S. K., Lehoczki A., Laukkanen J. A. (2024). The untapped potential of cold water therapy as part of a lifestyle intervention for promoting healthy aging. GeroScience 47, 387–407. doi:10.1007/s11357-024-01295-w
Kurobe K., Huang Z., Nishiwaki M., Yamamoto M., Kanehisa H., Ogita F. (2015). Effects of resistance training under hypoxic conditions on muscle hypertrophy and strength. Clin. Physiol. Funct. Imaging 35, 197–202. doi:10.1111/cpf.12147
Kwon J. H., Moon K. M., Min K.-W. (2020). Exercise-induced myokines can explain the importance of physical activity in the elderly: an overview. Healthc. (Basel) 8, 378. doi:10.3390/healthcare8040378
Lacerda L. T., Martins-Costa H. C., Diniz R. C. R., Lima F. V., Andrade A. G. P., Tourino F. D., et al. (2016). Variations in repetition duration and repetition numbers influence muscular activation and blood lactate response in protocols Equalized by time under tension. J. Strength Cond. Res. 30, 251–258. doi:10.1519/JSC.0000000000001044
Lacio M., Vieira J. G., Trybulski R., Campos Y., Santana D., Filho J. E., et al. (2021). Effects of resistance training performed with different loads in untrained and trained male adult individuals on maximal strength and muscle hypertrophy: a systematic review. Int. J. Environ. Res. Public Health 18, 11237. doi:10.3390/ijerph182111237
Larkin K. A., Macneil R. G., Dirain M., Sandesara B., Manini T. M., Buford T. W. (2012). Blood flow restriction enhances post-resistance exercise angiogenic gene expression. Med. Sci. Sports Exerc 44, 2077–2083. doi:10.1249/MSS.0b013e3182625928
Lasevicius T., Schoenfeld B. J., Silva-Batista C., Barros T. d. S., Aihara A. Y., Brendon H., et al. (2022). Muscle failure promotes greater muscle hypertrophy in low-load but not in high-load resistance training. J. Strength Cond. Res. 36, 346–351. doi:10.1519/JSC.0000000000003454
Lasevicius T., Ugrinowitsch C., Schoenfeld B. J., Roschel H., Tavares L. D., De Souza E. O., et al. (2018). Effects of different intensities of resistance training with equated volume load on muscle strength and hypertrophy. Eur. J. Sport Sci. 18, 772–780. doi:10.1080/17461391.2018.1450898
Laurens C., Bergouignan A., Moro C. (2020). Exercise-released myokines in the control of energy metabolism. Front. Physiol. 11, 91. doi:10.3389/fphys.2020.00091
Laurentino G. C., Ugrinowitsch C., Roschel H., Aoki M. S., Soares A. G., Neves M., et al. (2012). Strength training with blood flow restriction diminishes myostatin gene expression. Med. Sci. Sports Exerc 44, 406–412. doi:10.1249/MSS.0b013e318233b4bc
Lawson D., Vann C., Schoenfeld B. J., Haun C. (2022). Beyond mechanical tension: a review of resistance exercise-induced lactate responses and muscle hypertrophy. J. Funct. Morphol. Kinesiol. 7, 81. doi:10.3390/jfmk7040081
Leal L. G., Lopes M. A., Batista M. L. (2018). Physical exercise-induced myokines and muscle-adipose tissue crosstalk: a review of current knowledge and the implications for health and metabolic diseases. Front. Physiol. 9, 1307. doi:10.3389/fphys.2018.01307
Lee J. H., Jun H.-S. (2019). Role of myokines in regulating skeletal muscle mass and function. Front. Physiol. 10, 42. doi:10.3389/fphys.2019.00042
Lepley L. K., Davi S. M., Burland J. P., Lepley A. S. (2020). Muscle atrophy after ACL injury: implications for clinical practice. Sports Health 12, 579–586. doi:10.1177/1941738120944256
Leslie E., Luna V., Gibson A. L. (2023). Older adult aerobic capacity, muscular strength, fitness and body composition after 20+ Years of exercise training: a systematic review and meta-analysis. Int. J. Exerc Sci. 16, 620–637. doi:10.70252/HSVD9987
Li R., Xia J., Zhang X. I., Gathirua-Mwangi W. G., Guo J., Li Y., et al. (2018). Associations of muscle mass and strength with all-cause mortality among US older adults. Med. Sci. Sports Exerc 50, 458–467. doi:10.1249/MSS.0000000000001448
Li S., Guo R., Wang J., Zheng X., Zhao S., Zhang Z., et al. (2023). The effect of blood flow restriction exercise on N-lactoylphenylalanine and appetite regulation in obese adults: a cross-design study. Front. Endocrinol. (Lausanne) 14, 1289574. doi:10.3389/fendo.2023.1289574
Lim C., Kim H. J., Morton R. W., Harris R., Phillips S. M., Jeong T. S., et al. (2019). Resistance exercise-induced changes in muscle phenotype are load dependent. Med. Sci. Sports Exerc 51, 2578–2585. doi:10.1249/MSS.0000000000002088
Linnamo V., Pakarinen A., Komi P. V., Kraemer W. J., Häkkinen K. (2005). Acute hormonal responses to submaximal and maximal heavy resistance and Explosive exercises in men and women. J. Strength Cond. Res. 19, 566–571. doi:10.1519/R-15404.1
Liu L., Guo J., Chen X., Tong X., Xu J., Zou J. (2021). The role of irisin in exercise-mediated bone health. Front. Cell Dev. Biol. 9, 668759. doi:10.3389/fcell.2021.668759
Liu Y., Liu J., Liu M., Wang M. (2024). The effect of blood flow restriction training on core muscle strength and pain in male collegiate athletes with chronic non-specific low back pain. Front. Public Health 12, 1496482. doi:10.3389/fpubh.2024.1496482
Lixandrão M. E., Ugrinowitsch C., Berton R., Vechin F. C., Conceição M. S., Damas F., et al. (2018). Magnitude of muscle strength and mass adaptations between high-load resistance training versus low-load resistance training associated with blood-flow restriction: a systematic review and meta-analysis. Sports Med. 48, 361–378. doi:10.1007/s40279-017-0795-y
Loenneke J. P., Fahs C. A., Wilson J. M., Bemben M. G. (2011). Blood flow restriction: the metabolite/volume threshold theory. Med. Hypotheses 77, 748–752. doi:10.1016/j.mehy.2011.07.029
Lundberg T. R., Fernandez-Gonzalo R., Tesch P. A. (2014). Exercise-induced AMPK activation does not interfere with muscle hypertrophy in response to resistance training in men. J. Appl. physiology Bethesda 116, 611–620. doi:10.1152/japplphysiol.01082.2013
Madarame H., Neya M., Ochi E., Nakazato K., Sato Y., Ishii N. (2008). Cross-transfer effects of resistance training with blood flow restriction. Med. Sci. sports Exerc. 40, 258–263. doi:10.1249/mss.0b013e31815c6d7e
Maffiuletti N. A., Aagaard P., Blazevich A. J., Folland J., Tillin N., Duchateau J. (2016). Rate of force development: physiological and methodological considerations. Eur. J. Appl. Physiol. 116, 1091–1116. doi:10.1007/s00421-016-3346-6
Manimmanakorn A., Hamlin M. J., Ross J. J., Taylor R., Manimmanakorn N. (2013). Effects of low-load resistance training combined with blood flow restriction or hypoxia on muscle function and performance in netball athletes. J. Sci. Med. Sport 16, 337–342. doi:10.1016/j.jsams.2012.08.009
Manini T. M., Yarrow J. F., Buford T. W., Clark B. C., Conover C. F., Borst S. E. (2012). Growth hormone responses to acute resistance exercise with vascular restriction in young and old men. Growth Horm. IGF Res. 22, 167–172. doi:10.1016/j.ghir.2012.05.002
Marston K. J., Brown B. M., Rainey-Smith S. R., Bird S., Wijaya L., Teo S. Y. M., et al. (2019). Twelve weeks of resistance training does not influence peripheral levels of neurotrophic growth factors or homocysteine in healthy adults: a randomized-controlled trial. Eur. J. Appl. Physiol. 119, 2167–2176. doi:10.1007/s00421-019-04202-w
Marston K. J., Newton M. J., Brown B. M., Rainey-Smith S. R., Bird S., Martins R. N., et al. (2017). Intense resistance exercise increases peripheral brain-derived neurotrophic factor. J. Sci. Med. Sport 20, 899–903. doi:10.1016/j.jsams.2017.03.015
Martin P. M., Bart R. M., Ashley R. L., Velasco T., Wise S. R. (2022). An overview of blood flow restriction physiology and clinical considerations. Curr. Sports Med. Rep. 21, 123–128. doi:10.1249/JSR.0000000000000948
Martínez Muñoz I. Y., Camarillo R. E. del S., Correa Padilla T., Santillán Benítez J. G., Camarillo Romero M. D. S., Montenegro Morales L. P., et al. (2019). Association of irisin serum concentration and muscle strength in normal-weight and overweight young women. Front. Endocrinol. (Lausanne) 10, 621. doi:10.3389/fendo.2019.00621
May A. K., Russell A. P., Warmington S. A. (2018). Lower body blood flow restriction training may induce remote muscle strength adaptations in an active unrestricted arm. Eur. J. Appl. physiology 118, 617–627. doi:10.1007/s00421-018-3806-2
McCarthy S. F., Bornath D. P. D., Jarosz C., Tucker J. A. L., Medeiros P. J., Kenno K. A., et al. (2023). Intense interval exercise induces lactate accumulation and a greater suppression of acylated ghrelin compared with submaximal exercise in middle-aged adults. J. Appl. Physiol. 134, 1177–1187. doi:10.1152/japplphysiol.00709.2022
Melkonian E. A., Schury M. P. (2024). “Biochemistry, anaerobic glycolysis,” in StatPearls (Treasure Island, FL: StatPearls Publishing). Available online at: http://www.ncbi.nlm.nih.gov/books/NBK546695/(Accessed December 3, 2024).
Merry T. L., Ristow M. (2016). Do antioxidant supplements interfere with skeletal muscle adaptation to exercise training? J. Physiol. 594, 5135–5147. doi:10.1113/JP270654
Miller B. C., Tirko A. W., Shipe J. M., Sumeriski O. R., Moran K. (2021). The systemic effects of blood flow restriction training: a systematic review. Int. J. Sports Phys. Ther. 16, 978–990. doi:10.26603/001c.25791
Moberg M., Apró W., Cervenka I., Ekblom B., van Hall G., Holmberg H. C., et al. (2021). High-intensity leg cycling alters the molecular response to resistance exercise in the arm muscles. Sci. Rep. 11, 6453. doi:10.1038/s41598-021-85733-1
Momma H., Kawakami R., Honda T., Sawada S. S. (2022). Muscle-strengthening activities are associated with lower risk and mortality in major non-communicable diseases: a systematic review and meta-analysis of cohort studies. Br. J. Sports Med. 56, 755–763. doi:10.1136/bjsports-2021-105061
Mortensen O. H., Frandsen L., Schjerling P., Nishimura E., Grunnet N. (2006). PGC-1alpha and PGC-1beta have both similar and distinct effects on myofiber switching toward an oxidative phenotype. Am. J. Physiol. Endocrinol. Metab. 291, E807–E816. doi:10.1152/ajpendo.00591.2005
Morton R. W., Oikawa S. Y., Wavell C. G., Mazara N., McGlory C., Quadrilatero J., et al. (2016). Neither load nor systemic hormones determine resistance training-mediated hypertrophy or strength gains in resistance-trained young men. J. Appl. physiology Bethesda 121, 129–138. doi:10.1152/japplphysiol.00154.2016
Morton R. W., Sonne M. W., Farias Zuniga A., Mohammad I. Y. Z., Jones A., McGlory C., et al. (2019). Muscle fibre activation is unaffected by load and repetition duration when resistance exercise is performed to task failure. J. Physiol. 597, 4601–4613. doi:10.1113/JP278056
Muñoz-Cánoves P., Scheele C., Pedersen B. K., Serrano A. L. (2013). Interleukin-6 myokine signaling in skeletal muscle: a double-edged sword? FEBS J. 280, 4131–4148. doi:10.1111/febs.12338
Myrholt R. B., Solberg P., Pettersen H., Seynnes O., Paulsen G. (2023). Effects of low- versus high-velocity-loss thresholds with similar training volume on maximal strength and hypertrophy in highly trained individuals. Int. J. Sports Physiol. Perform. 18, 368–377. doi:10.1123/ijspp.2022-0161
Nalbandian M., Takeda M. (2016). Lactate as a signaling molecule that regulates exercise-induced adaptations. Biology 5, 38. doi:10.3390/biology5040038
Nelke C., Dziewas R., Minnerup J., Meuth S. G., Ruck T. (2019). Skeletal muscle as potential central link between sarcopenia and immune senescence. EBioMedicine 49, 381–388. doi:10.1016/j.ebiom.2019.10.034
Neto I. V. de S., Pinto A. P., Muñoz V. R., de Cássia Marqueti R., Pauli J. R., Ropelle E. R., et al. (2023). Pleiotropic and multi-systemic actions of physical exercise on PGC-1α signaling during the aging process. Ageing Res. Rev. 87, 101935. doi:10.1016/j.arr.2023.101935
Nordlund M. M., Thorstensson A., Cresswell A. G. (2004). Central and peripheral contributions to fatigue in relation to level of activation during repeated maximal voluntary isometric plantar flexions. J. Appl. Physiol. 96, 218–225. doi:10.1152/japplphysiol.00650.2003
O’Keefe J. H., Lavie C. J., Guazzi M. (2015). Part 1: potential dangers of extreme endurance exercise: how much is too much? Part 2: screening of school-age athletes. Prog. Cardiovasc Dis. 57, 396–405. doi:10.1016/j.pcad.2014.11.004
O’Keefe J. H., O’Keefe E. L., Eckert R., Lavie C. J. (2023). Training strategies to optimize cardiovascular Durability and life expectancy. Mo Med. 120, 155–162.
Owen A., Wiles J., Swaine I. (2010). Effect of isometric exercise on resting blood pressure: a meta analysis. J. Hum. Hypertens. 24, 796–800. doi:10.1038/jhh.2010.13
Owens D. J., Twist C., Cobley J. N., Howatson G., Close G. L. (2019). Exercise-induced muscle damage: what is it, what causes it and what are the nutritional solutions? Eur. J. Sport Sci. 19, 71–85. doi:10.1080/17461391.2018.1505957
Ozaki H., Loenneke J. P., Buckner S. L., Abe T. (2016). Muscle growth across a variety of exercise modalities and intensities: contributions of mechanical and metabolic stimuli. Med. Hypotheses 88, 22–26. doi:10.1016/j.mehy.2015.12.026
Palasz E., Wysocka A., Gasiorowska A., Chalimoniuk M., Niewiadomski W., Niewiadomska G. (2020). BDNF as a promising therapeutic agent in Parkinson’s disease. Int. J. Mol. Sci. 21, 1170. doi:10.3390/ijms21031170
Patel H., Alkhawam H., Madanieh R., Shah N., Kosmas C. E., Vittorio T. J. (2017). Aerobic vs anaerobic exercise training effects on the cardiovascular system. World J. Cardiol. 9, 134–138. doi:10.4330/wjc.v9.i2.134
Patrick R. P., Johnson T. L. (2021). Sauna use as a lifestyle practice to extend healthspan. Exp. Gerontol. 154, 111509. doi:10.1016/j.exger.2021.111509
Patterson S. D., Leggate M., Nimmo M. A., Ferguson R. A. (2013). Circulating hormone and cytokine response to low-load resistance training with blood flow restriction in older men. Eur. J. Appl. Physiol. 113, 713–719. doi:10.1007/s00421-012-2479-5
Pearson S. J., Hussain S. R. (2015). A review on the mechanisms of blood-flow restriction resistance training-induced muscle hypertrophy. Sports Med. 45, 187–200. doi:10.1007/s40279-014-0264-9
Pedersen B. K. (2019). Which type of exercise keeps you young? Curr. Opin. Clin. Nutr. and Metabolic Care 22, 167–173. doi:10.1097/MCO.0000000000000546
Pedersen B. K., Febbraio M. A. (2008). Muscle as an endocrine organ: focus on muscle-derived interleukin-6. Physiol. Rev. 88, 1379–1406. doi:10.1152/physrev.90100.2007
Peters P. G., Alessio H. M., Hagerman A. E., Ashton T., Nagy S., Wiley R. L. (2006). Short-term isometric exercise reduces systolic blood pressure in hypertensive adults: possible role of reactive oxygen species. Int. J. Cardiol. 110, 199–205. doi:10.1016/j.ijcard.2005.07.035
Poole D. C., Rossiter H. B., Brooks G. A., Gladden L. B. (2021). The anaerobic threshold: 50+ years of controversy. J. Physiol. 599, 737–767. doi:10.1113/JP279963
Powers S. K., Ji L. L., Kavazis A. N., Jackson M. J. (2011). Reactive oxygen species: impact on skeletal muscle. Compr. Physiol. 1, 941–969. doi:10.1002/cphy.c100054
Qian L., Zhu Y., Deng C., Liang Z., Chen J., Chen Y., et al. (2024). Peroxisome proliferator-activated receptor gamma coactivator-1 (PGC-1) family in physiological and pathophysiological process and diseases. Signal Transduct. Target Ther. 9, 50. doi:10.1038/s41392-024-01756-w
Quistorff B., Secher N. H., Lieshout J. J. (2008). Lactate fuels the human brain during exercise. FASEB J. 22, 3443–3449. doi:10.1096/fj.08-106104
Rahimi R., Qaderi M., Faraji H., Boroujerdi S. S. (2010). Effects of very short rest periods on hormonal responses to resistance exercise in men. J. Strength and Cond. Res. 24, 1851–1859. doi:10.1519/JSC.0b013e3181ddb265
Ramírez-Campillo R., Andrade D. C., Campos-Jara C., Henríquez-Olguín C., Alvarez-Lepín C., Izquierdo M. (2013). Regional fat changes induced by localized muscle endurance resistance training. J. Strength Cond. Res. 27, 2219–2224. doi:10.1519/JSC.0b013e31827e8681
Rao R. R., Long J. Z., White J. P., Svensson K. J., Lou J., Lokurkar I., et al. (2014). Meteorin-like is a hormone that regulates immune-adipose interactions to increase beige fat thermogenesis. Cell 157, 1279–1291. doi:10.1016/j.cell.2014.03.065
Raschke S., Eckel J. (2013). Adipo-myokines: two sides of the same coin--mediators of inflammation and mediators of exercise. Mediat. Inflamm. 2013, 320724. doi:10.1155/2013/320724
Realzola R. A., Mang Z. A., Millender D. J., Beam J. R., Bellovary B. N., Wells A. D., et al. (2022). Metabolic profile of reciprocal supersets in young, recreationally active women and men. J. Strength and Cond. Res. 36, 2709–2716. doi:10.1519/JSC.0000000000003920
Robergs R. A., Ghiasvand F., Parker D. (2004). Biochemistry of exercise-induced metabolic acidosis. Am. J. Physiol. Regul. Integr. Comp. Physiol. 287, R502–R516. doi:10.1152/ajpregu.00114.2004
Roberts M. D., McCarthy J. J., Hornberger T. A., Phillips S. M., Mackey A. L., Nader G. A., et al. (2023). Mechanisms of mechanical overload-induced skeletal muscle hypertrophy: current understanding and future directions. Physiol. Rev. 103, 2679–2757. doi:10.1152/physrev.00039.2022
Robinson Z. P., Pelland J. C., Remmert J. F., Refalo M. C., Jukic I., Steele J., et al. (2024). Exploring the dose–response relationship between Estimated resistance training proximity to failure, strength gain, and muscle hypertrophy: a Series of meta-Regressions. Sports Med. 54, 2209–2231. doi:10.1007/s40279-024-02069-2
Rojas-Valverde D., Bonilla D. A., Gómez-Miranda L. M., Calleja-Núñez J. J., Arias N., Martínez-Guardado I. (2023). Examining the interaction between exercise, gut microbiota, and neurodegeneration: future research directions. Biomedicines 11, 2267. doi:10.3390/biomedicines11082267
Rønnestad B. R., Nygaard H., Raastad T. (2011). Physiological elevation of endogenous hormones results in superior strength training adaptation. Eur. J. Appl. physiology 111, 2249–2259. doi:10.1007/s00421-011-1860-0
Rossi F. E., de Freitas M. C., Zanchi N. E., Lira F. S., Cholewa J. M. (2018). The role of inflammation and immune cells in blood flow restriction training adaptation: a review. Front. Physiol. 9, 1376. doi:10.3389/fphys.2018.01376
Roy M., Williams S. M., Brown R. C., Meredith-Jones K. A., Osborne H., Jospe M., et al. (2018). High-intensity interval training in the real World: outcomes from a 12-Month intervention in overweight adults. Med. Sci. Sports Exerc 50, 1818–1826. doi:10.1249/MSS.0000000000001642
Saatmann N., Zaharia O.-P., Loenneke J. P., Roden M., Pesta D. H. (2021). Effects of blood flow restriction exercise and possible applications in type 2 diabetes. Trends Endocrinol. and Metabolism 32, 106–117. doi:10.1016/j.tem.2020.11.010
Saheli M., Moshrefi M., Baghalishahi M., Mohkami A., Firouzi Y., Suzuki K., et al. (2024). Cognitive fitness: Harnessing the strength of exerkines for aging and metabolic challenges. Sports (Basel) 12, 57. doi:10.3390/sports12020057
Sale D. G. (1988). Neural adaptation to resistance training. Med. Sci. sports Exerc. 20, S135–S145. doi:10.1249/00005768-198810001-00009
Saliminejad K., Khorram Khorshid H. R., Soleymani F. S., Ghaffari S. H. (2019). An overview of microRNAs: biology, functions, therapeutics, and analysis methods. J. Cell Physiol. 234, 5451–5465. doi:10.1002/jcp.27486
Sauer H., Wartenberg M., Hescheler J. (2001). Reactive oxygen species as intracellular messengers during cell growth and differentiation. Cell Physiol. Biochem. 11, 173–186. doi:10.1159/000047804
Schoenfeld B. (2011). The Use of Specialized training techniques to maximize muscle hypertrophy. Strength and Cond. J. 33, 60–65. doi:10.1519/ssc.0b013e3182221ec2
Schoenfeld B. J. (2013). Potential mechanisms for a role of metabolic stress in hypertrophic adaptations to resistance training. Sports Med. 43, 179–194. doi:10.1007/s40279-013-0017-1
Schoenfeld B. J., Grgic J., Ogborn D., Krieger J. W. (2017). Strength and hypertrophy adaptations between low-vs. High-load resistance training: a systematic review and meta-analysis. J. Strength Cond. Res. 31, 3508–3523. doi:10.1519/JSC.0000000000002200
Schoenfeld B. J., Grgic J., Van Every D. W., Plotkin D. L. (2021). Loading recommendations for muscle strength, hypertrophy, and local endurance: a Re-Examination of the repetition continuum. Sports (Basel) 9, 32. doi:10.3390/sports9020032
Senna G. W., Dantas E. H. M., Scudese E., Brandão P. P., Lira V. A., Baffi M., et al. (2022). Higher muscle damage triggered by shorter inter-set rest periods in volume-equated resistance exercise. Front. Physiol. 13, 827847. doi:10.3389/fphys.2022.827847
Seo D.-I., So W.-Y., Sung D. J. (2016). Effect of a low-intensity resistance exercise programme with blood flow restriction on growth hormone and insulin-like growth factor-1 levels in middle-aged women. South Afr. J. Res. Sport, Phys. Educ. Recreat. 38, 167–177.
Serrano A. L., Baeza-Raja B., Perdiguero E., Jardí M., Muñoz-Cánoves P. (2008). Interleukin-6 is an essential regulator of satellite cell-mediated skeletal muscle hypertrophy. Cell Metab. 7, 33–44. doi:10.1016/j.cmet.2007.11.011
Shailendra P., Baldock K. L., Li L. S. K., Bennie J. A., Boyle T. (2022). Resistance training and mortality risk: a systematic review and meta-analysis. Am. J. Prev. Med. 63, 277–285. doi:10.1016/j.amepre.2022.03.020
So B., Kim H. J., Kim J., Song W. (2014). Exercise-induced myokines in health and metabolic diseases. Integr. Med. Res. 3 (4), 172–179. doi:10.1016/j.imr.2014.09.007
Sødal L. K., Kristiansen E., Larsen S., van den Tillaar R. (2023). Effects of drop sets on skeletal muscle hypertrophy: a systematic review and meta-analysis. Sports Med. Open 9, 66. doi:10.1186/s40798-023-00620-5
Spiering B. A., Kraemer W. J., Vingren J. L., Ratamess N. A., Anderson J. M., Armstrong L. E., et al. (2009). Elevated endogenous testosterone concentrations potentiate muscle androgen receptor responses to resistance exercise. J. steroid Biochem. Mol. Biol. 114, 195–199. doi:10.1016/j.jsbmb.2009.02.005
Spillane M., Schwarz N., Willoughby D. S. (2015). Upper-body resistance exercise augments vastus lateralis androgen receptor–DNA binding and canonical Wnt/β-catenin signaling compared to lower-body resistance exercise in resistance-trained men without an acute increase in serum testosterone. Steroids 98, 63–71. doi:10.1016/j.steroids.2015.02.019
Steinbacher P., Eckl P. (2015). Impact of oxidative stress on exercising skeletal muscle. Biomolecules 5, 356–377. doi:10.3390/biom5020356
Strasser B., Burtscher M. (2018). Survival of the fittest: VO2max, a key predictor of longevity? Front. Biosci. Landmark Ed. 23, 1505–1516. doi:10.2741/4657
Suchomel T. J., Nimphius S., Bellon C. R., Stone M. H. (2018). The importance of muscular strength: training considerations. Sports Med. 48, 765–785. doi:10.1007/s40279-018-0862-z
Sundstrup E., Jakobsen M. D., Andersen C. H., Zebis M. K., Mortensen O. S., Andersen L. L. (2012). Muscle activation strategies during strength training with heavy loading vs. repetitions to failure. J. Strength Cond. Res. 26, 1897–1903. doi:10.1519/JSC.0b013e318239c38e
Takarada Y., Ishii N. (2002). Effects of low-intensity resistance exercise with short Interset rest period on muscular function in middle-aged women. J. Strength and Cond. Res. 16, 123–128. doi:10.1519/1533-4287(2002)016<0123:eolire>2.0.co;2
Tanaka M., Watanabe Y. (2012). Supraspinal regulation of physical fatigue. Neurosci. Biobehav Rev. 36, 727–734. doi:10.1016/j.neubiorev.2011.10.004
Taylor J. L., Amann M., Duchateau J., Meeusen R., Rice C. L. (2016). Neural contributions to muscle fatigue: from the brain to the muscle and back Again. Med. Sci. Sports Exerc 48, 2294–2306. doi:10.1249/MSS.0000000000000923
Tee J. C., Bosch A. N., Lambert M. I. (2007). Metabolic consequences of exercise-induced muscle damage. Sports Med. 37, 827–836. doi:10.2165/00007256-200737100-00001
Theofilidis G., Bogdanis G. C., Koutedakis Y., Karatzaferi C. (2018). Monitoring exercise-induced muscle fatigue and adaptations: making Sense of popular or emerging Indices and Biomarkers. Sports (Basel) 6, 153. doi:10.3390/sports6040153
Tortoriello D. V., Sidis Y., Holtzman D. A., Holmes W. E., Schneyer A. L. (2001). Human follistatin-related protein: a structural homologue of follistatin with nuclear localization. Endocrinology 142, 3426–3434. doi:10.1210/endo.142.8.8319
Triplett N. T., Haff G. G. (2015). Essentials of Strength training and conditioning. Champaign, IL: Human Kinetics.
Valenzuela P. L., Saco-Ledo G., Morales J. S., Gallardo-Gómez D., Morales-Palomo F., López-Ortiz S., et al. (2023). Effects of physical exercise on physical function in older adults in residential care: a systematic review and network meta-analysis of randomised controlled trials. Lancet Healthy Longev. 4, e247–e256. doi:10.1016/S2666-7568(23)00057-0
Van Hooren B., Aagaard P., Blazevich A. J. (2024). Optimizing resistance training for Sprint and endurance athletes: Balancing positive and negative adaptations. Sports Med. 54, 3019–3050. doi:10.1007/s40279-024-02110-4
van Melick N., van Cingel R. E. H., Brooijmans F., Neeter C., van Tienen T., Hullegie W., et al. (2016). Evidence-based clinical practice update: practice guidelines for anterior cruciate ligament rehabilitation based on a systematic review and multidisciplinary consensus. Br. J. Sports Med. 50, 1506–1515. doi:10.1136/bjsports-2015-095898
Vaynman S., Gomez-Pinilla F. (2006). Revenge of the ‘sit’: how lifestyle impacts neuronal and cognitive health through molecular systems that interface energy metabolism with neuronal plasticity. J. Neurosci. Res. 84, 699–715. doi:10.1002/jnr.20979
Verney J., Kadi F., Charifi N., Féasson L., Saafi M. A., Castells J., et al. (2008). Effects of combined lower body endurance and upper body resistance training on the satellite cell pool in elderly subjects. Muscle and Nerve 38, 1147–1154. doi:10.1002/mus.21054
Verney J., Kadi F., Saafi M. A., Piehl-Aulin K., Denis C. (2006). Combined lower body endurance and upper body resistance training improves performance and health parameters in healthy active elderly. Eur. J. Appl. Physiol. 97, 288–297. doi:10.1007/s00421-006-0175-z
Wackerhage H., Schoenfeld B. J., Hamilton D. L., Lehti M., Hulmi J. J. (2019). Stimuli and sensors that initiate skeletal muscle hypertrophy following resistance exercise. J. Appl. Physiology 126, 30–43. doi:10.1152/japplphysiol.00685.2018
Walker S., Taipale R. S., Nyman K., Kraemer W. J., Häkkinen K. (2011). Neuromuscular and hormonal responses to constant and variable resistance loadings. Med. Sci. Sports Exerc 43, 26–33. doi:10.1249/MSS.0b013e3181e71bcb
Wallace W., Ugrinowitsch C., Stefan M., Rauch J., Barakat C., Shields K., et al. (2019). Repeated Bouts of advanced strength training techniques: effects on volume load, metabolic responses, and muscle activation in trained individuals. Sports (Basel) 7, 14. doi:10.3390/sports7010014
Wang B., Davies T. B., Way K. L., Tran D. L., Davis G. M., Singh M. F., et al. (2023). Effect of resistance training on local muscle endurance in middle-aged and older adults: a systematic review with meta-analysis and meta-regression. Arch. Gerontol. Geriatr. 109, 104954. doi:10.1016/j.archger.2023.104954
Wang L., Lv Y., Li G., Xiao J. (2018). MicroRNAs in heart and circulation during physical exercise. J. Sport Health Sci. 7, 433–441. doi:10.1016/j.jshs.2018.09.008
Warneke K., Lohmann L. H., Behm D. G., Wirth K., Keiner M., Schiemann S., et al. (2024). Effects of chronic Static Stretching on maximal strength and muscle hypertrophy: a systematic review and meta-analysis with meta-regression. Sports Med. - Open 10, 45. doi:10.1186/s40798-024-00706-8
Weakley J., Schoenfeld B., Ljungberg J., Halson S. L., Phillips S. M. (2023). Physiological responses and adaptations to lower load resistance training: implications for health and performance. Sports Med. - Open 9, 28. doi:10.1186/s40798-023-00578-4
Weakley J. J. S., Till K., Read D. B., Roe G. A. B., Darrall-Jones J., Phibbs P. J., et al. (2017). The effects of traditional, superset, and tri-set resistance training structures on perceived intensity and physiological responses. Eur. J. Appl. Physiol. 117, 1877–1889. doi:10.1007/s00421-017-3680-3
Wernbom M., Aagaard P. (2020). Muscle fibre activation and fatigue with low-load blood flow restricted resistance exercise-An integrative physiology review. Acta Physiol. (Oxf) 228, e13302. doi:10.1111/apha.13302
West D. W., Burd N. A., Tang J. E., Moore D. R., Staples A. W., Holwerda A. M., et al. (2010). Elevations in ostensibly anabolic hormones with resistance exercise enhance neither training-induced muscle hypertrophy nor strength of the elbow flexors. J. Appl. physiology Bethesda 108, 60–67. doi:10.1152/japplphysiol.01147.2009
Westcott W. L. (2012). Resistance training is medicine: effects of strength training on health. Curr. Sports Med. Rep. 11, 209–216. doi:10.1249/JSR.0b013e31825dabb8
Willoughby D. S., Cardaci T. D., Machek S. B., Wilburn D. T., Heileson J. L. (2022). Resistance exercise-induced increases in muscle myostatin mRNA and protein expression are subsequently decreased in circulation in the presence of increased levels of the Extracellular Matrix Stabilizing protein decorin. J. Sports Sci. Med. 21, 616–624. doi:10.52082/jssm.2022.616
Xue X., Liu B., Hu J., Bian X., Lou S. (2022). The potential mechanisms of lactate in mediating exercise-enhanced cognitive function: a dual role as an energy supply substrate and a signaling molecule. Nutr. and metabolism 19, 52. doi:10.1186/s12986-022-00687-z
Yang J., Christophi C. A., Farioli A., Baur D. M., Moffatt S., Zollinger T. W., et al. (2019). Association between push-up exercise capacity and future cardiovascular events among active adult men. JAMA Netw. Open 2, e188341. doi:10.1001/jamanetworkopen.2018.8341
Yin M., Deng S., Deng J., Xu K., Nassis G. P., Girard O., et al. (2025). Physiological adaptations and performance enhancement with combined blood flow restricted and interval training: a systematic review with meta-analysis. J. Sport Health Sci., 101030.
Zhang X., Weakley J., Li H., Li Z., García-Ramos A. (2025). Superset versus traditional resistance training Prescriptions—a systematic review and meta-analysis exploring acute and chronic effects on mechanical, metabolic, and Perceptual variables. Sports Med. doi:10.1007/s40279-025-02176-8
Zhou J., Guo R., Ma J., Cu Z., Guo L., Yu W. (2024). The effect of low-intensity interval exercise with blood flow restriction on plasma cardiac Troponin: a cross-design trial. Anatol. J. Cardiol. 28, 542–549. doi:10.14744/AnatolJCardiol.2024.4458
Zhu C., Ma H., He A., Li Y., He C., Xia Y. (2022). Exercise in cancer prevention and anticancer therapy: Efficacy, molecular mechanisms and clinical information. Cancer Lett. 544, 215814. doi:10.1016/j.canlet.2022.215814
Zhu J., Li Y., Lu A., Gharaibeh B., Ma J., Kobayashi T., et al. (2011). Follistatin improves skeletal muscle healing after injury and disease through an interaction with muscle regeneration, angiogenesis, and fibrosis. Am. J. pathology 179 (2), 915–930. doi:10.1016/j.ajpath.2011.04.008
Keywords: hypertrophy, strength, muscular endurance, superset, blood flow restriction, anaerobic, lactate
Citation: Curovic I (2025) The role of resistance exercise-induced local metabolic stress in mediating systemic health and functional adaptations: could condensed training volume unlock greater benefits beyond time efficiency?. Front. Physiol. 16:1549609. doi: 10.3389/fphys.2025.1549609
Received: 21 December 2024; Accepted: 07 April 2025;
Published: 17 April 2025.
Edited by:
Dawid Koźlenia, Wroclaw University of Health and Sport Sciences, PolandReviewed by:
Erich Hohenauer, University of Applied Sciences and Arts of Southern Switzerland, SwitzerlandCristina Benavente, University of Granada, Spain
Copyright © 2025 Curovic. This is an open-access article distributed under the terms of the Creative Commons Attribution License (CC BY). The use, distribution or reproduction in other forums is permitted, provided the original author(s) and the copyright owner(s) are credited and that the original publication in this journal is cited, in accordance with accepted academic practice. No use, distribution or reproduction is permitted which does not comply with these terms.
*Correspondence: Ivan Curovic, aWN1cm92aWNAdWNsYW4uYWMudWs=