- 1Department of Pharmacology, School of Medicine, Tulane University, New Orleans, LA, United States
- 2Department of Medical Pharmacology and Physiology, School of Medicine, University of Missouri, Columbia, MO, United States
Introduction: Chronic metabolic stress is a common underlying factor of multiple diseases, including obesity, type II diabetes, and metabolic syndrome. Lymphatic dysfunction, including valve defects, impaired contractile activity, and hyperpermeability, is also associated with these same diseases. We recently reported that acute metabolic stress leads to activation of KATP channels in lymphatic muscle cells, resulting in impairment of the intrinsic lymphatic pacemaker that drives their spontaneous contractions and active lymphatic pumping.
Methods: In the present study, we tested whether lymphatic contractile dysfunction occurs in the db/db mouse, a model of metabolic syndrome, and, if so, to what extent dysfunction might be mediated by KATP channel activation. Contractile function was assessed ex vivo in cannulated and pressurized popliteal collecting lymphatics from age-matched db/db mice or their BKS controls (from males and females at 18–20 weeks of age).
Results: Vessels from db/db mice exhibited pressure-dependent spontaneous contractions that were significantly reduced in amplitude, frequency, and calculated fractional pump flow at all tested pressures in the range 0.5 to 5 cmH2O, compared to BKS controls. The impaired contractile function of lymphatic vessels from db/db mice was improved by the KATP channel inhibitor glibenclamide (GLIB) at a concentration (1 mM) previously shown to have little or no off-target effects on lymphatic function. Because db/db mice are both obese and have elevated blood glucose levels, we tested whether elevated glucose per se altered contractile function. In glucose levels characteristic of diabetic animals (23 mM), the contraction frequency and fractional pump flow of lymphatic vessels from WT mice were significantly decreased compared to those observed in normal (5 mM) glucose concentrations. The equivalent concentration of mannitol, an osmotic control, did not result in any significant changes in lymphatic contractile function. Lymphatic dysfunction induced by high glucose was rescued by GLIB (1 mM), and lymphatic vessels from Kir6.1−/− mice were largely resistant to the inhibitory effects of high glucose.
Discussion: Our results suggest that a substantial fraction of lymphatic contractile impairment in db/db mice is mediated by the activation of KATP channels in lymphatic muscle cells, in part due to chronic metabolic stress associated with elevated glucose.
1 Introduction
The lymphatic system plays fundamental roles in the reabsorption and transport of excess interstitial fluid back into the central veins, the coordination of efficient and effective immune responses, the absorption and transport of lipids, as well as tissue-specific roles that contribute to the normal functioning of tissues and organs (Liao and Padera, 2013; Randolph et al., 2017; Petrova and Koh, 2018; 2020; Stritt et al., 2021; Angeli and Lim, 2023; Davis et al., 2024). It is now recognized that dysfunction of the lymphatic system is not only associated with various diseases, but it can also be a key determining factor in their development and progression. Relevant to the central topic of this study, obesity, metabolic syndrome, and type-2 diabetes have been bidirectionally associated with secondary lymphedema (Greene et al., 2012; Mehrara and Greene, 2014; Greene, 2016; Ashing et al., 2020; Figenshau and Lindquist, 2020; Kataru et al., 2020; de Sire et al., 2021; Rockson, 2021; Sudduth and Greene, 2022b; a).
Secondary lymphedema is more prevalent in breast-cancer patients following surgical (i.e., lymph node dissection), chemo- and radio-therapeutic intervention (Rockson, 2023; Siotos et al., 2024); and obesity, type-2 diabetes, and metabolic syndrome are established risk factors (Helyer et al., 2010; Tsai et al., 2018; Duyur Cakit et al., 2019; Rockson, 2020; Siotos et al., 2024). Studies conducted using various animal models of obesity, metabolic syndrome, and type-2 diabetes have provided important insights into some of the mechanisms leading to lymphatic dysfunction in association with these diseases. For instance, we recently demonstrated that lymphatic valve deficiency results from diet-induced obesity in WT mice; and diet-induced obese ApoE-KO mice display an even more severe dysfunctional phenotype including hyperpermeability and contractile impairment, in addition to profound valve dysfunction (Castorena-Gonzalez, 2022; Davis et al., 2022b). Previously, in vivo studies on diet-induced obese WT mice uncovered a reduced contractility of collecting lymphatics (i.e., decreased frequency) and impaired response to mechanostimulation (Blum et al., 2014), as well as impaired lymphatic fluid transport and impaired dendritic cell migration (Weitman et al., 2013). Increased lymphatic vascular permeability was demonstrated in obese and type-2 diabetic mice (Scallan et al., 2015). In a rat model of obesity and metabolic syndrome, studies have demonstrated an association with various aspects of dysfunction in collecting lymphatic vessels, including impairment of their intrinsic contractility, inward remodeling, and blunted flow-mediated responses. These animals were also characterized by increased expression of inflammatory genes (i.e., TNFα, SREBP1C), decreased expression of nitric oxide synthase (eNOS), decreased expression of the sarcoplasmic reticulum Ca2+ ATPase pump (i.e., SERCA2a), and increased accumulation of CD163+ MHCII + macrophages (Chakraborty et al., 2010; Zawieja et al., 2012; Zawieja et al., 2016a; Zawieja et al., 2016b; Lee et al., 2020; Lee et al., 2022).
Although such studies have provided important insights into some of the underlying mechanisms of lymphatic dysfunction in obesity, metabolic syndrome, and type-2 diabetes, much remains to be fully elucidated. The significance of exploring novel mechanisms of lymphatic system dysfunction in these metabolic diseases is highlighted by the increasing number of patients afflicted with secondary lymphedema, in association with the global obesity epidemic, and the lack of pharmacological therapies to reverse or ameliorate lymphatic dysfunction.
In a recent study, we demonstrated that acute metabolic stress leads to lymphatic dysfunction mediated by reduced mitochondrial ATP production and increased ROS production, which inhibit the ionic pacemaking mechanisms that control the spontaneous contractility of lymphatic muscle cells (Kim et al., 2024). Interestingly, gain of function mutations in ATP-sensitive potassium channels (i.e., KATP channels) are known to cause Cantú syndrome and a consistent phenotype of Cantú patients is the development of primary lymphedema resulting from contractile impairment of collecting lymphatic vessels (Davis et al., 2020; Davis et al., 2022a; Davis et al., 2023). Altogether, these findings prompted us to test the hypothesis that in metabolic diseases, including obesity, metabolic syndrome, and type-2 diabetes, chronic metabolic stress results in activation of KATP channels leading to lymphatic dysfunction.
Therefore, in this study, we utilized db/db mice, a well-known and established model of type-2 diabetes, obesity, and metabolic syndrome, to determine if contractile dysregulation is present, and if so, determine the extent to which activation of KATP channels contributes. Furthermore, since high blood glucose levels are an important characteristic of these mice, we assessed high glucose as a potential significant mediator of the underlying metabolic stress in this process.
2 Materials and methods
2.1 Animals
WT (C57BL/6J, Cat.No. 000664), db/db (BKS.Cg-Dock7m+/+Leprdb/J, Cat.No. 000642), and BKS (C57BLKS/J, Cat.No. 000662) male and female mice were purchased at 6 weeks of age from the Jackson Laboratory–Bar Harbor, MA, USA (JAX). Kir6.1−/− mice were a gift from Susumu Seino (Kobe University, Kobe, Japan). Mice were housed at a temperature of 22–25oC under a 12-h light/dark cycle with uninterrupted access to food (regular chow) and water until terminal tissue collection and ex vivo experimentation were performed. In preparation for terminal dissection, mice were anesthetized by means of intraperitoneal injection of Ketamine/Xylazine (100/10 mg/kg) and placed on a heated pad for blood collection and tissue dissection. Adequate levels of anesthesia were ensured by continuous monitoring for indicators of pain and by assessing loss of pedal and pinna reflexes. Following dissection, animals were euthanized via overdose of Ketamine/Xylazine and subsequent cervical dislocation.
Age-matched db/db and BKS mice were utilized at 18–20 weeks of age. At this age, db/db mice display continued hyperinsulinemia, in addition to sustained hyperglycemia. Kir6.1−/− showed a high mortality rate beyond 3–4 months of age, and therefore, experiments on Kir6.1−/− and their corresponding WT controls were performed at 6–10 weeks of age. Experiments were conducted in male and female mice in equal ratios. Initially, the data were analyzed separately; however, once we determined that the contractile function was similar in both sexes for the different animal groups, the data were combined.
2.2 Assessment of blood glucose levels
With a mouse under anesthesia, unfasted blood glucose concentration was assessed from a droplet of blood collected after a small incision of the tail dorsal caudal vein. Glucose concentration was determined using a glucose meter.
2.3 Vessel isolation, pressure myography, and data acquisition
Popliteal afferent collecting lymphatic vessels were isolated as previously described (Scallan and Davis, 2013; Scallan et al., 2015; Castorena-Gonzalez et al., 2018; Zawieja et al., 2018). Briefly, starting with the mouse in the prone position laying on a heated tissue dissection/isolation pad, the superficial saphenous vein was exposed by making a proximal-to-distal incision along the calf area beginning at the ankle and the popliteal afferent lymphatic vessels that run along this major vein were isolated. Then, each popliteal lymphatic tissue was pinned down onto the Sylgard-coated surface of a dissection chamber filled with Krebs-BSA where most of the adipose and connective tissues surrounding the vessel were micro-dissected. Subsequently, a partially clean vessel segment was transferred to an observation chamber filled with Krebs-BSA solution. The vessel was cannulated and pressurized to three cmH2O, under no-flow conditions, using two glass micropipettes (inside diameter was ∼40–50 µm). To ensure accurate diameter tracking, the cannulated vessel segment was further cleared of remaining connective and adipose tissue. The observation chamber with cannulated lymphatic vessel segment was transferred onto the XY-stage of an inverted microscope for experimentation. Polyethylene tubing (PE-190) was attached to the back of each glass micropipette and then connected to a microfluidic flow control system (Elveflow OB1 MK3, Paris) with attached low-pressure transducers. Consistent with previous studies (Davis et al., 2011; Lapinski et al., 2017; Castorena-Gonzalez et al., 2018; Castorena-Gonzalez et al., 2020), to minimize longitudinal bowing and associated diameter-tracking artifacts at higher intraluminal pressures, input (Pinput) and output (Poutput) pressures were briefly set to the maximum pressure to be included in the experimental protocol, and the vessel segment was stretched axially to remove longitudinal slack. Before starting an experimental protocol, the vessel was then allowed to equilibrate at 37°C for 30–60 min at Pinput = Poutput = 3 cmH2O while constantly superfused with Krebs buffer (without BSA) using a peristaltic pump at a rate of 0.5 mL/min. Lymphatic vessels usually began displaying spontaneous contractions within 15–30 min during warm up. Custom-written LabVIEW programs (National Instruments; Austin, TX) were employed for pressure control, as well as real-time vessel diameter tracking and acquisition of video (Davis, 2005). Videos were recorded in brightfield mode at 30 fps using a Basler A641fm camera.
2.4 Solutions and chemicals
During microdissection and cannulation of lymphatic segments, a Krebs-BSA buffer was utilized. It contained: 146.9 mM NaCl, 4.7 mM KCl, 2 mM CaCl2·2H2O, 1.2 mM MgSO4, 1.2 mM NaH2PO4·H2O, 3 mM NaHCO3, 1.5 mM Na-HEPES, 5 mM D (+)-glucose, and 0.5% BSA (pH = 7.4). A similar Krebs buffer without BSA was utilized for constant superfusion of the lymphatic vessels during warm up and experimental protocols. To determine the maximum passive diameter of a vessel as a function of intraluminal pressure, a Ca2+-free Krebs buffer (where 3 mM EGTA replaced calcium) was used. All reagents in the different solutions/buffers, as well as D (+)-glucose (Cat.No.: G5767), and D-mannitol (Cat.No.: M4125) were purchased from Sigma-Aldrich (St. Louis, MO). Glibenclamide (Cat.No.: 0911) was purchased from Tocris Bioscience.
2.5 Assessment of lymphatic contractile function
The contractile activity of lymphatic vessels was assessed as a function of intraluminal pressure (i.e., Pinput = Poutput = 3, 2, 1, 0.5, 3, and 5 cmH2O). For each vessel, the functional response to changes in intraluminal pressure was determined under control conditions and subsequently in the presence of glibenclamide (GLIB, 1 µM), a concentration previously shown to have little or no off-target effects (Kim et al., 2021). The functional responses to different concentrations of glucose and mannitol were assessed in lymphatic vessels from WT animals under no-flow conditions at three cmH2O. Following each experimental protocol, the maximum passive diameter of a given vessel at the different tested levels of intraluminal pressure was determined after superfusing the vessel with a Ca2+-free Krebs buffer for 30 min. Following experimentation, custom-written analysis programs were used to detect peak end-diastolic diameter (EDD), end-systolic diameter (ESD), contraction amplitude (AMP), and contraction frequency (FREQ) on a contraction-by-contraction basis. In cases when FREQ was zero, no value of AMP was recorded. These parameters were average over a 5-min period and then used to calculate other parameters commonly employed to characterize the contractile function of lymphatic vessels as follows:
2.6 Statistical analyses
The number N refers to the number of animals per group that were included in this study, while n refers to the total number of experiments (i.e., lymphatic segments tested independently). In most cases, more than one lymphatic segment from the same mouse was successfully studied. A description of the specific statistical tests implemented for each data set is included in each figure caption. Normality of the data distributions was assessed using the Shapiro-Wilk and Kolmogorov-Smirnov tests. Results are reported as mean ± SEM with significance set at p < 0.05. All statistical analyzes were performed using GraphPad Prism 10.4.1.
3 Results
3.1 Activation of KATP channels contributes to the contractile impairment of collecting lymphatic vessels in db/db mice
To determine whether db/db mice display lymphatic contractile deficiencies, we characterized the contractile function of isolated, cannulated, and pressurized popliteal afferent collecting lymphatic vessels from db/db mice (N = 17) and BKS controls (N = 13). Consistent with many other studies, db/db mice displayed a significantly increased body weight (53.2 ± 0.6 g) versus that of BKS mice (24.4 ± 1.4 g, Figure 1A), and displayed significantly higher blood glucose levels, i.e., 519.9 ± 14.25 mg/dL and 157.7 ± 13.0 mg/dL, respectively (Figure 1B). The contractile activity of lymphatic vessels from these mice was assessed in the absence of intraluminal flow, and as a function of intraluminal pressure (i.e., PInput = POutput = 3, 2, 1, 0.5, 3, and 5 cmH2O, Figure 1C) in control conditions, as well as in the presence of the KATP channel inhibitor Glibenclamide (GLIB, 1 µM). A representative example of a vessel from a BKS animal is shown in Figure 1D. At the end of each experimental protocol, the maximum passive diameter of each vessel was determined in a Ca2+-free Krebs buffer at the different levels of intraluminal pressure. Although not statistically significant, lymphatic vessels from db/db mice were 7.6% ± 0.7% smaller in diameter than those in the BKS group. Consistent with our previously published data using popliteal lymphatic vessels from WT mice, lymphatic vessels from BKS mice displayed rhythmic and robust contractions and high sensitivity to changes in intraluminal pressure, with lower frequency and larger amplitude contractions at low levels of pressure, and higher frequency and lower amplitude contractions as pressure increased (Figure 2A). In contrast, lymphatic vessels from db/db mice displayed significantly impaired contractility as evident by relatively low amplitude, low frequency, erratic contractions (Figure 2C). Inhibition of KATP channels with GLIB (1 µM) further induced significant increases in the contractile frequency and calculated fractional pump flow (FPF) in lymphatic vessels from BKS mice (Figures 2B, 3A–F); and importantly, GLIB partially restored lymphatic contractions of vessels from db/db mice (Figures 2D, 3A–C,G–I), as indicated by the significantly increased frequency and partially enhanced fractional pump flow. GLIB did not induce any significant changes in mean normalized contraction amplitude in either genotype.
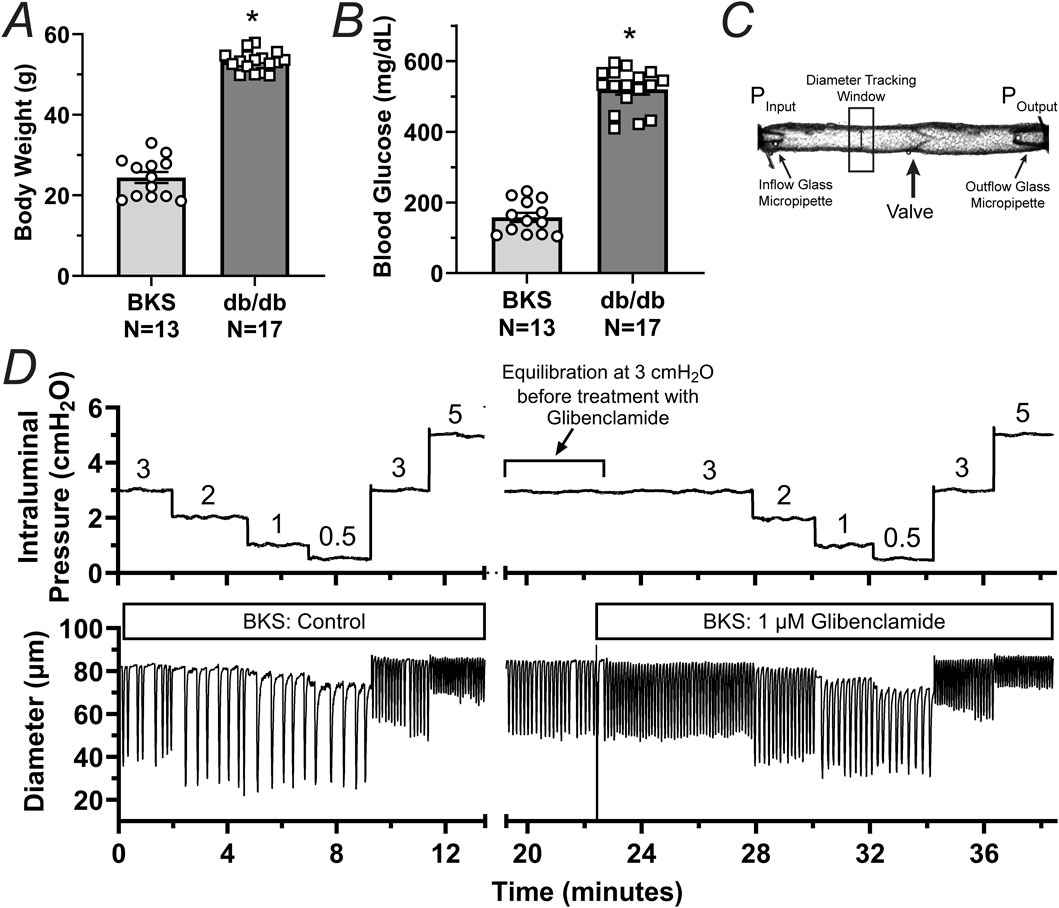
Figure 1. Phenotypic characterization and experimental protocol for the assessment of the contractile function of lymphatic vessels from BKS (control) and db/db mice. (A, B) Body weight and unfasted blood glucose levels in db/db (N = 17) and BKS (N = 13) mice respectively. (C) Diagram depicting a single-valve isolated, cannulated, and pressurized popliteal afferent collecting lymphatic vessel from a BKS mouse. This panel highlights the inflow and outflow glass micropipettes and their corresponding pressures. (D) Representative trace of internal diameter displaying the contractile activity of a lymphatic vessel from a BKS mouse under control conditions and in the presence of glibenclamide (GLIB, 1 μM). Data in panels A and B are presented as mean ± SEM. Statistical differences were determined using parametric t tests with significance defined at *p < 0.05.
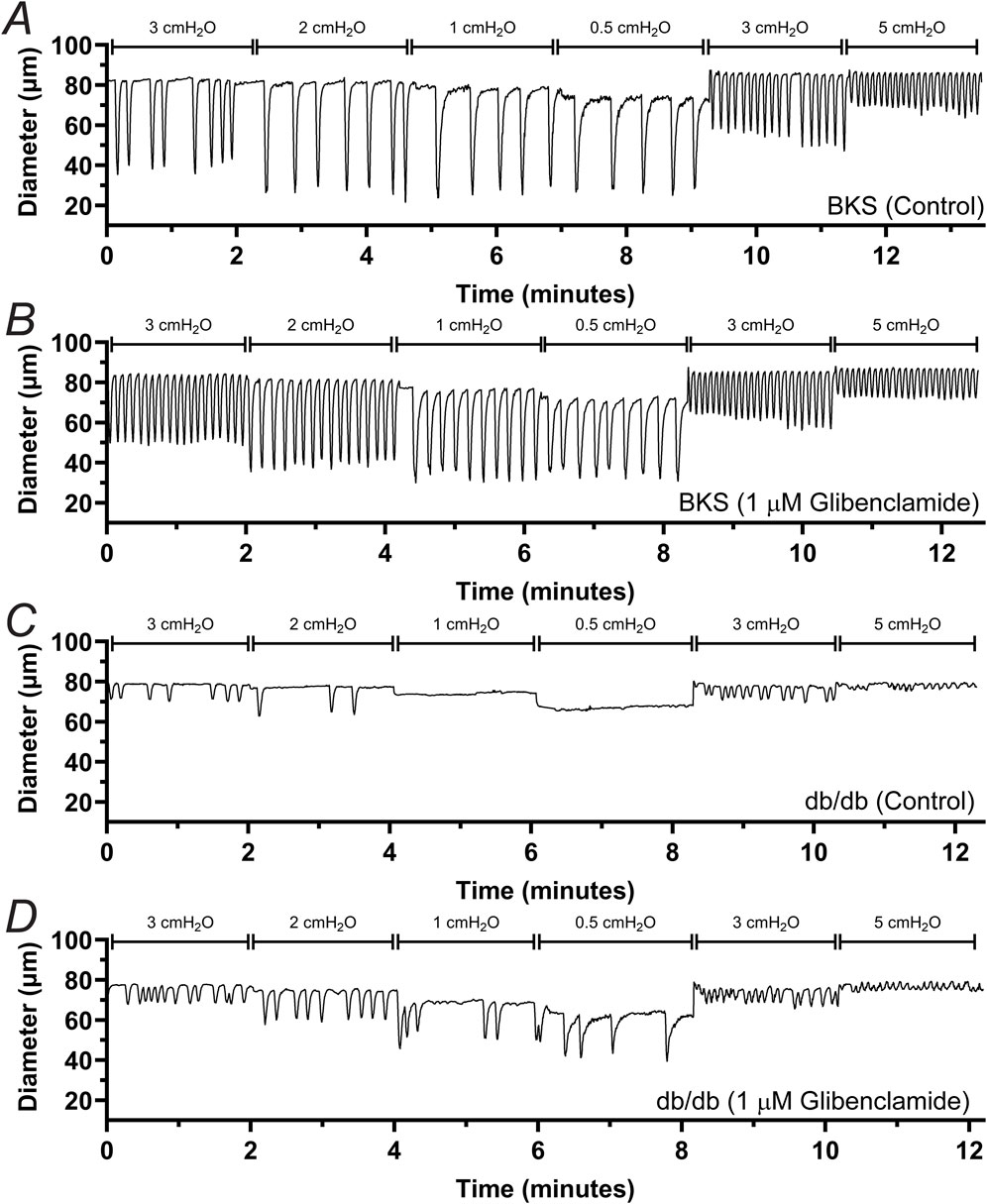
Figure 2. Effect of KATP channel inhibition by GLIB on the contractile activity of lymphatic vessels from BKS and db/db mice. Representative traces depicting the contractile response to different levels of intraluminal pressure for lymphatic vessels from BKS (A, B) and db/db mice (C, D). Functional response was assessed under control conditions (A, C) and in the presence of GLIB (B, D).
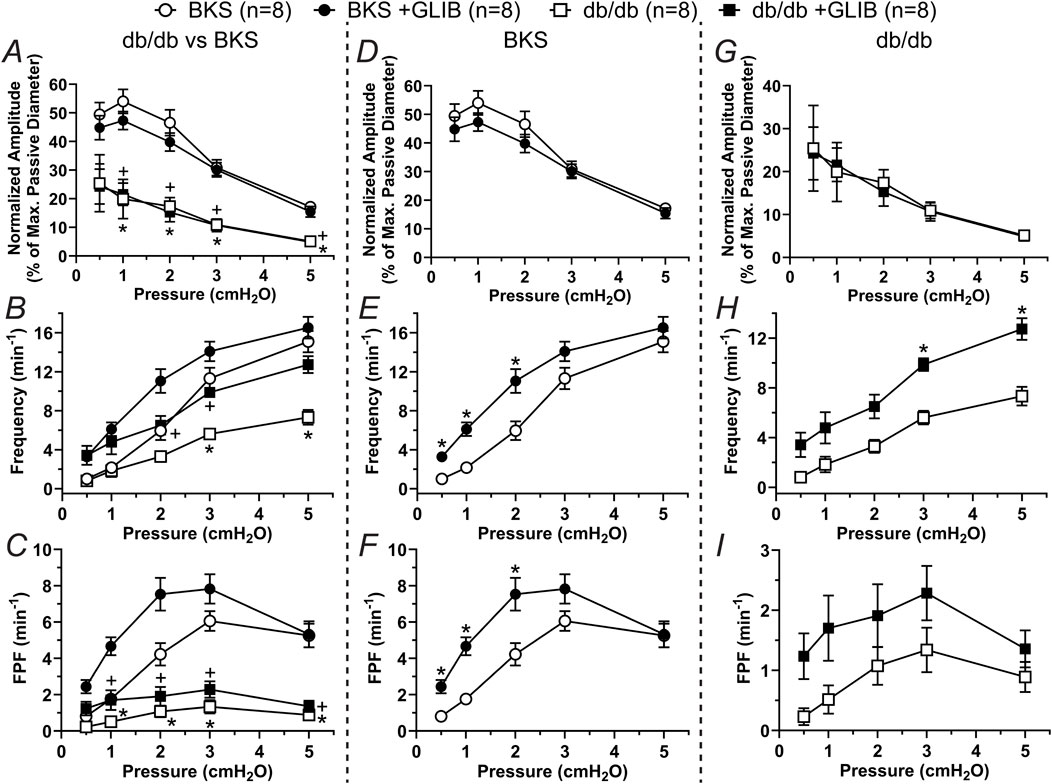
Figure 3. KATP channel inhibition by GLIB enhances the contractility of lymphatic vessels from BKS mice and partially rescues the lymphatic contractile impairment of lymphatic vessels from db/db mice. Summary data of contractile parameters as a function of intraluminal pressure. (A–C) Normalized amplitude, frequency, and FPF comparing all groups. In these panels, the symbols indicate significant difference between db/db and BKS in control conditions (*) and in the presence of GLIB (+). (D–F) Contractile parameters for lymphatic vessels from BKS mice in control conditions and with GLIB. (G–I) Contractile parameters for lymphatic vessels from db/db mice in control conditions and with GLIB. Data are presented as mean ± SEM. Statistical significance was determined at p < 0.05 using two-way ANOVA followed by Tukey post hoc test for multiple comparisons for data in panels (A–C) or Šídák for data in panels (D, I).
In addition to an increase in contraction frequency, lymphatic contractions, in both the BKS and db/db groups, appeared more rhythmic. To assess lymphatic contractile rhythmicity, we measured the intercontraction interval (i.e., time between successive contractions) for ∼15–30 contractions and then utilized the calculated Standard Deviation (STDEV) as a measure of intercontraction interval variability. For simplicity, these additional analyses were conducted only at pressure 3 cmH2O. Representative traces of the contractile activity of lymphatic vessels from BKS and db/db mice in control and in the presence of GLIB are shown in Figures 4A, B, D, E. In control conditions, the STDEV of the intercontraction intervals of vessels from the BKS group were significantly smaller than that of vessels from db/db mice, which was indicative of the rhythmic contractions observed in vessels from BKS mice, and the erratic lymphatic contractions in db/db mice. Interestingly, treatment with GLIB resulted in a significant increase in lymphatic vessel rhythmicity in both BKS and db/db groups, as indicated by the paired analyses showing consistent decreases in the STDEV of intercontraction intervals (Figures 4C, F).
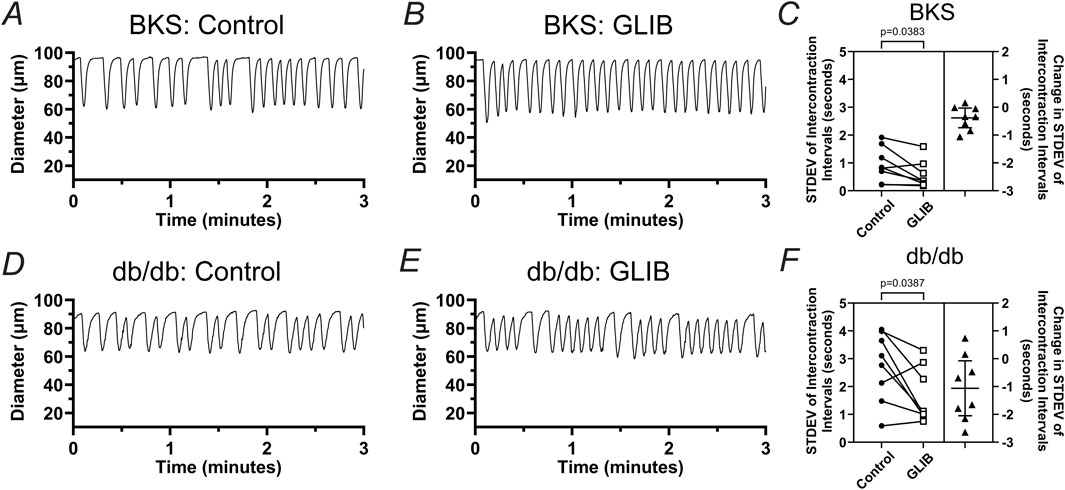
Figure 4. Inhibition of KATP channels by GLIB increases the rhythmicity of lymphatic contractions in vessels from BKS and db/db mice. Representative traces of the contractile activity (at 3 cmH2O of intraluminal pressure) of lymphatic vessels from (A, B) BKS or (D, E) db/db mice under control conditions, and upon treatment with GLIB. (C, F) Standard deviation (STDEV) of the intercontraction intervals in control conditions and in the presence of GLIB for lymphatic vessels from BKS and db/db groups respectively. STDEV is used as a measurement of the variability of the intercontraction intervals, and therefore, an indicator of contractile rhythmicity, i.e., lower STDEV values indicative of higher rhythmicity, while higher STDEV values are indicative of erratic non-rhythmic contractions. Data is presented as paired STDEV values per vessel in control conditions and with GLIB, as well as the change in STDEV. Statistical significance was determined using a paired t-test with significance defined at *p < 0.05.
3.2 High concentrations of glucose induce lymphatic contractile impairment independently of osmotic stress
In previous studies using db/db mice or diet-induced obese mice, which display obesity and hyperglycemia, we showed that unfasted blood glucose levels reached ∼23 mM (Scallan et al., 2015; Castorena-Gonzalez, 2022). To determine whether higher concentrations of glucose, including concentrations characteristic of diabetic mice, induce contractile dysfunction, we characterized the contractile activity of popliteal lymphatic vessels from WT mice pressurized to 1 cmH2O under no-flow conditions after acute exposure to 5 mM (control), 10 mM, 15 mM, and 23 mM of glucose. Glucose concentration did not affect the normalized amplitude of contractions; however, normalized frequency and calculated fractional pump flow (FPF) were significantly decreased (i.e., >40%) in 23 mM glucose (Figures 5A–C). Contractile activity at the lower pressures was particularly affected, consistent with observations made in previous studies (Davis et al., 2020; Davis et al., 2023). To determine if the contractile dysfunction induced by high glucose was merely associated with induced osmotic stress, we tested the functional responses of popliteal lymphatic vessels to equivalent concentrations of the osmotic diuretic, mannitol. The osmotic stress effects of mannitol did not result in any significant changes in the contractile function of these collecting lymphatics, and even led to a slight (22%) increase in contraction frequency (Figures 5A–C).
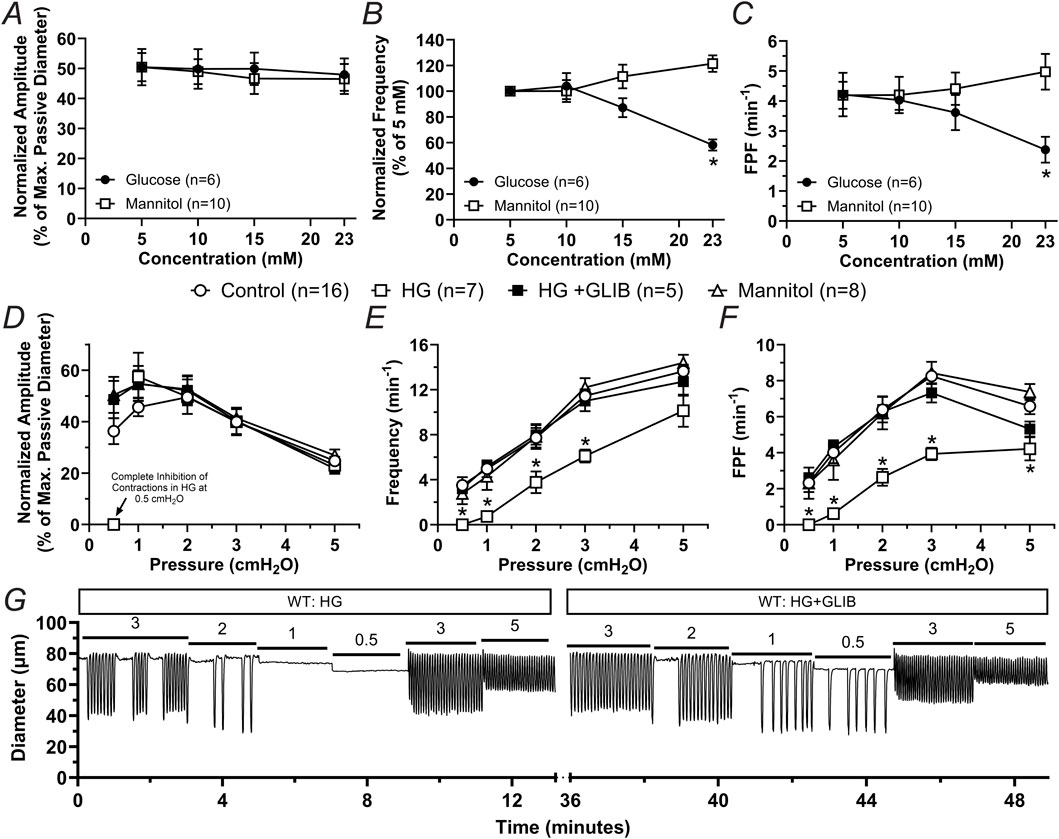
Figure 5. High glucose induces contractile dysfunction mediated by KATP channel activation but independent of osmotic stress in lymphatic vessels from WT mice. (A–C) Mean normalized amplitude, normalized frequency, and FPF as a function of increasing concentrations of glucose or mannitol, respectively. Data are presented as mean ± SEM. Statistical significance was determined at p < 0.05 using two-way ANOVA followed by Šídák post hoc test for multiple comparisons. (D–F) Mean normalized amplitude, frequency, and FPF as a function of intraluminal pressure in control conditions, or in the presence of high glucose (HG, 23 mM), HG + GLIB (1 µM), or mannitol (23 mM). Data are presented as mean ± SEM. Statistical significance was determined at p < 0.05 comparing all groups using two-way ANOVA followed by Tukey post hoc test for multiple comparisons. (G) Representative trace of the contractile activity of a lymphatic vessel from a WT mouse as a function of intraluminal pressure in HG, as well as in HG and in the presence of GLIB.
3.3 Lymphatic vessels from KATP deficient animals are largely resistant to contractile dysfunction induced by high glucose
Since inhibition of KATP channels with GLIB partially rescued the contractile dysfunction of lymphatic vessels from db/db mice and significantly increased lymphatic contractile rhythmicity in both BKS and db/db groups (Figures 2–4), we decided to test the extent to which activation of KATP channels plays a role in the lymphatic contractile impairment induced by high glucose (HG, i.e., 23 mM). Consistent with the experiments performed in vessels from db/db mice, the contractile function of lymphatic vessels from WT mice was assessed at different levels of intraluminal pressure under control conditions, HG (23 mM), HG + GLIB (1 µM), or mannitol (23 mM). The contractile function of lymphatic vessels in the presence of mannitol was similar to that of vessels in control conditions. In contrast, HG induced robust contractile impairment (i.e., significantly decreased frequency and FPF). Furthermore, GLIB completely rescued the lymphatic contractile dysfunction induced by HG (Figures 5D–F) even at low pressure levels where HG resulted in complete inhibition of contractions. Representative traces of the contractile activity of a lymphatic vessel from a WT mouse in HG, as well as in HG in the presence of GLIB, are shown in Figure 5G.
To further confirm the involvement of KATP channels in the contractile dysfunction induced by HG, we assessed the effects of HG in regulating the contractile function of lymphatic vessels from mice globally deficient in Kir6.1 (i.e., Kir6.1−/− mice lacking KATP channels). The contractile frequency and FPF observed in lymphatic vessels from Kir6.1−/− remained largely unaffected in HG. Interestingly, the normalized contraction amplitude of lymphatic vessels from these KATP deficient mice was significantly increased in the presence of HG in the lower pressures (i.e., 0.5 cmH2O and one cmH2O), but remained unchanged at higher pressures (i.e., >1 cmH2O) (Figures 6A–C). Representative traces of the contractile activity of a lymphatic vessel from a Kir6.1−/− mouse in control conditions and in the presence of HG are shown in Figures 6D, E.
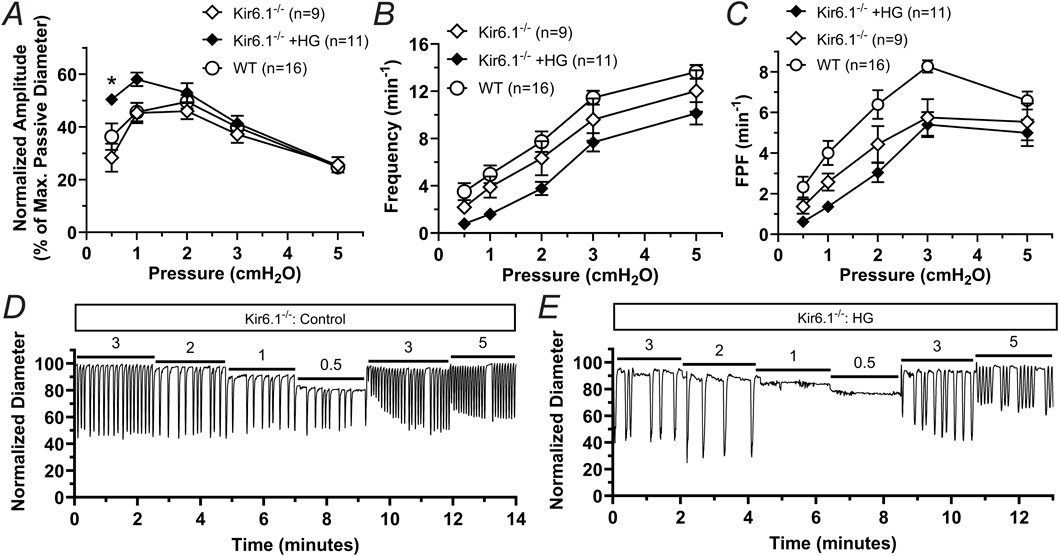
Figure 6. Lymphatic vessels from Kir6.1-deficient mice are largely resistant to lymphatic dysfunction induced by HG. (A) Normalized amplitude, (B) frequency, and (C) FPF as a function of intraluminal pressure for lymphatic vessels from mice lacking Kir6.1 in control conditions or in the presence of high glucose (HG, 23 mM). Data from WT vessels under control conditions, initially presented in Figures 5D–F, is here included again for comparison. Data are presented as mean ± SEM. Statistical significance was determined at p < 0.05 comparing all groups using two-way ANOVA followed by Šídák post hoc test for multiple comparisons. Representative traces of the contractile activity as a function of intraluminal pressure of a lymphatic vessel from a Kir6.1−/− mouse under (D) control conditions and (E) while in the presence of HG.
4 Discussion
In the present study, we employed db/db mice as a model of type-2 diabetes, obesity, and metabolic syndrome to first determine the extent of lymphatic contractile dysfunction in collecting lymphatic vessels, and then to investigate and elucidate the underlying mechanisms. We found that popliteal lymphatic vessels from db/db mice displayed robust contractile impairment, which could be partially rescued by inhibition of KATP channels by GLIB. In healthy lymphatics from WT mice, metabolic stress induced by HG (similar to those observed in db/db mice) resulted in contractile dysfunction mediated by KATP activation, as it was reversed by GLIB. These observations were finally validated using a global Kir6.1-deficient mouse model. The contractile function of lymphatic vessels lacking KATP channels was highly resistant to the detrimental effects of HG.
Initially, we characterized the contractile function of popliteal afferent collecting lymphatic vessels from db/db mice and their matching BKS controls and demonstrated that profound contractile dysfunction is associated with this model, as evident by an erratic contraction pattern, low amplitude and low frequency contractions. The contractile activity of these vessels was assessed as a function of intraluminal pressure, in a range of pressures spanning the previously determined physiological levels (Czepielewski et al., 2021). While lymphatic vessels from db/db mice still displayed a functional response to increasing pressure, the largely impaired contractions are likely incapable of generating enough propulsive pressure for efficient transport of lymph (evident by the significantly reduced FPF), especially in the presence of any adverse pressure gradient.
Since our previous studies demonstrated that acute metabolic stress is associated with lymphatic dysfunction mediated by activation of KATP channels (Kim et al., 2024), we tested the effects of GLIB and demonstrated that pharmacological inhibition of KATP channels significantly enhanced the otherwise poor contractility of lymphatic vessels from db/db mice. These findings suggest that a component of lymphatic dysfunction in db/db mice is associated with activation of KATP channels.
Furthermore, approximately 37% and 25% of the vessels from db/db mice did not display any contractile activity at the lower range of intraluminal pressures, 0.5 and 1 cmH2O respectively; while in the BKS group, only ∼12% of the vessels did not display contractile activity at 0.5 cmH2O, but all vessels displayed robust rhythmic contractions at 1 cmH2O. Interestingly, inhibition of KATP channels by GLIB induced a significant increase in contractile function in BKS and db/db mice even at these low levels of intraluminal pressure. These observations suggest that the mechanisms underlying lymphatic pacemaking dysfunction in db/db mice not only are dependent on KATP channel activation but also are particularly vulnerable at lower levels of intraluminal pressure.
Results from our previous studies also showed that impaired contractility of LMCs in acute metabolic stress resulted in increased production of reactive oxygen species (ROS) and/or reduced mitochondrial ATP (Kim et al., 2024). Therefore, we hypothesized that a similar mechanism operated in animal models of chronic metabolic stress. The db/db mouse model recapitulates many of the characteristics observed in obesity, type-2 diabetes, and metabolic syndrome, including high blood glucose. We explored the potential role of high glucose as the main driver of lymphatic dysfunction in chronic metabolic stress in db/db mice. In isolated lymphatic vessels from WT mice, high glucose induced significant reduction in contractile frequency, while contraction amplitude remained unchanged, strikingly similar to the effects of acute metabolic stress induced by pharmacological inhibition of the mitochondrial electron transport chain (Kim et al., 2024). In the present study, lymphatic contractile function in lymphatics from WT mice was only significantly impaired at a high glucose level of 23 mM (HG), a level of unfasted glucose displayed in WT mice fed a western diet or in db/db mice (Scallan et al., 2015; Castorena-Gonzalez, 2022). An equivalent concentration of the osmotic diuretic mannitol did not induce any significant changes in lymphatic contractility, suggesting that the lymphatic contractile dysfunction induced by HG is not mediated by osmotic stress. HG completely or nearly-completely inhibited the contractile function of popliteal lymphatic vessels in the pressure range <2 cmH2O. This was fully reversed by GLIB, suggesting an important role for KATP channel activation in HG-induced metabolic stress leading to hyperpolarization of the cell membrane of LMCs and subsequent inhibition of the pacemaking activity that regulates the intrinsic lymphatic pumping.
To confirm the direct involvement of KATP channels in acute and chronic metabolic stress induced by HG, as it occurs in db/db mice, we then performed experiments in lymphatic vessels from mice globally deficient in Kir6.1 (i.e., Kir6.1−/−). Upon acute stimulation with HG, vessels from these Kir6.1 deficient mice were largely protected against the detrimental effects of HG previously observed in vessels from WT mice. Our previous studies have demonstrated that KATP channel activation occurs only in the LMC layer because 1) mouse LECs do not express functional KATP channels (Davis et al., 2020); and 2) lymphatic vessels from mice with SM-specific inactivation of KATP channels are equally resistant to acute metabolic stress as vessels from Kir6.1−/− mice (Kim et al., 2024). These findings suggest that LMC KATP channels can potentially be targeted therapeutically by GLIB to improve lymphatic contractile function in animal models of chronic metabolic stress, e.g., db/db, diet-induced obesity, and ApoE-KO among others, as well as in mice displaying a gain of function in KATP channels, as happens in Cantú syndrome patients. Future studies are necessary to address these important questions.
Data availability statement
The original contributions presented in the study are included in the article; further inquiries can be directed to the corresponding author.
Ethics statement
The animal study was approved by the Institutional Animal Care and Use Committee of the University of Missouri. The study was conducted in accordance with the local legislation and institutional requirements.
Author contributions
JC-G: Data curation, Formal Analysis, Funding acquisition, Investigation, Methodology, Writing – original draft, Writing – review and editing. HK: Data curation, Formal Analysis, Funding acquisition, Investigation, Methodology, Writing – original draft, Writing – review and editing. MD: Conceptualization, Data curation, Formal Analysis, Funding acquisition, Investigation, Methodology, Project administration, Software, Supervision, Writing – original draft, Writing – review and editing.
Funding
The author(s) declare that financial support was received for the research and/or publication of this article. Research reported in this publication was supported by the National Institutes of Health (NIH) under award numbers: NIH R01 HL-122578 to MJD and R01-HL168568 to JAC-G. HJK was supported in part by grant NRF-2020R1A6A3A0303715 from the National Research Foundation of Korea.
Acknowledgments
The authors are grateful for the expert assistance of Min Li and Shanyu Ho (University of Missouri).
Conflict of interest
The authors declare that the research was conducted in the absence of any commercial or financial relationships that could be construed as a potential conflict of interest.
Generative AI statement
The author(s) declare that no Generative AI was used in the creation of this manuscript.
Publisher’s note
All claims expressed in this article are solely those of the authors and do not necessarily represent those of their affiliated organizations, or those of the publisher, the editors and the reviewers. Any product that may be evaluated in this article, or claim that may be made by its manufacturer, is not guaranteed or endorsed by the publisher.
References
Angeli V., Lim H. Y. (2023). Biomechanical control of lymphatic vessel physiology and functions. Cell Mol. Immunol. 20 (9), 1051–1062. doi:10.1038/s41423-023-01042-9
Ashing K. T., Lai L., Meyers E., Serrano M., George M. (2020). Exploring the association between diabetes and breast cancer morbidity: considerations for quality care improvements among Latinas. Int. J. Qual. Health Care 32 (2), 120–125. doi:10.1093/intqhc/mzz130
Blum K. S., Karaman S., Proulx S. T., Ochsenbein A. M., Luciani P., Leroux J. C., et al. (2014). Chronic high-fat diet impairs collecting lymphatic vessel function in mice. PLoS One 9 (4), e94713. doi:10.1371/journal.pone.0094713
Castorena-Gonzalez J. A. (2022). Lymphatic valve dysfunction in western diet-fed mice: new insights into obesity-induced lymphedema. Front. Pharmacol. 13, 823266. doi:10.3389/fphar.2022.823266
Castorena-Gonzalez J. A., Srinivasan R. S., King P. D., Simon A. M., Davis M. J. (2020). Simplified method to quantify valve back-leak uncovers severe mesenteric lymphatic valve dysfunction in mice deficient in connexins 43 and 37. J. Physiol. 598 (12), 2297–2310. doi:10.1113/JP279472
Castorena-Gonzalez J. A., Zawieja S. D., Li M., Srinivasan R. S., Simon A. M., de Wit C., et al. (2018). Mechanisms of connexin-related lymphedema: a critical role for Cx45, but not Cx43 or Cx47, in the entrainment of spontaneous lymphatic contractions. Circ. Res. 123 (8), 964–985. doi:10.1161/CIRCRESAHA.117.312576
Chakraborty S., Zawieja S., Wang W., Zawieja D. C., Muthuchamy M. (2010). Lymphatic system: a vital link between metabolic syndrome and inflammation. Ann. N. Y. Acad. Sci. 1207 (Suppl. 1), E94–E102. doi:10.1111/j.1749-6632.2010.05752.x
Czepielewski R. S., Erlich E. C., Onufer E. J., Young S., Saunders B. T., Han Y. H., et al. (2021). Ileitis-associated tertiary lymphoid organs arise at lymphatic valves and impede mesenteric lymph flow in response to tumor necrosis factor. Immunity 54, 2795–2811.e9. doi:10.1016/j.immuni.2021.10.003
Davis M. J. (2005). An improved, computer-based method to automatically track internal and external diameter of isolated microvessels. Microcirculation 12 (4), 361–372. doi:10.1080/10739680590934772
Davis M. J., Castorena-Gonzalez J. A., Kim H. J., Li M., Remedi M., Nichols C. G. (2023). Lymphatic contractile dysfunction in mouse models of Cantú Syndrome with KATP channel gain-of-function. Funct. (Oxf) 4 (3), zqad017. doi:10.1093/function/zqad017
Davis M. J., Kim H. J., Nichols C. G. (2022a). K(ATP) channels in lymphatic function. Am. J. Physiol. Cell Physiol. 323 (4), C1018–C1035. doi:10.1152/ajpcell.00137.2022
Davis M. J., Kim H. J., Zawieja S. D., Castorena-Gonzalez J. A., Gui P., Li M., et al. (2020). Kir6.1-dependent K(ATP) channels in lymphatic smooth muscle and vessel dysfunction in mice with Kir6.1 gain-of-function. J. Physiol. 598 (15), 3107–3127. doi:10.1113/JP279612
Davis M. J., Rahbar E., Gashev A. A., Zawieja D. C., Moore J. E. (2011). Determinants of valve gating in collecting lymphatic vessels from rat mesentery. Am. J. Physiol. Heart Circ. Physiol. 301 (1), H48–H60. doi:10.1152/ajpheart.00133.2011
Davis M. J., Scallan J. P., Castorena-Gonzalez J. A., Kim H. J., Ying L. H., Pin Y. K., et al. (2022b). Multiple aspects of lymphatic dysfunction in an ApoE-/- mouse model of hypercholesterolemia. Front. Physiol. 13, 1098408. doi:10.3389/fphys.2022.1098408
Davis M. J., Zawieja S. D., King P. D. (2024). Transport and immune functions of the lymphatic system. Annu. Rev. Physiol. 87, 151–172. doi:10.1146/annurev-physiol-022724-104908
de Sire A., Inzitari M. T., Moggio L., Pinto M., de Sire G., Supervia M., et al. (2021). Effects of intermittent pneumatic compression on lower limb lymphedema in patients with type 2 diabetes mellitus: a pilot randomized controlled trial. Med. Kaunas. 57 (10), 1018. doi:10.3390/medicina57101018
Duyur Cakit B., Pervane Vural S., Ayhan F. F. (2019). Complex decongestive therapy in breast cancer-related lymphedema: does obesity affect the outcome negatively? Lymphat. Res. Biol. 17 (1), 45–50. doi:10.1089/lrb.2017.0086
Figenshau K. G., Lindquist M. B. (2020). Disease specific to chronic lymphedema and class III obesity. Case Rep. Med. 2020, 9234183. doi:10.1155/2020/9234183
Greene A. K. (2016). Diagnosis and management of obesity-induced lymphedema. Plast. Reconstr. Surg. 138 (1), 111e–118e. doi:10.1097/PRS.0000000000002258
Greene A. K., Grant F. D., Slavin S. A. (2012). Lower-extremity lymphedema and elevated body-mass index. N. Engl. J. Med. 366 (22), 2136–2137. doi:10.1056/NEJMc1201684
Helyer L. K., Varnic M., Le L. W., Leong W., McCready D. (2010). Obesity is a risk factor for developing postoperative lymphedema in breast cancer patients. Breast J. 16 (1), 48–54. doi:10.1111/j.1524-4741.2009.00855.x
Kataru R. P., Park H. J., Baik J. E., Li C., Shin J., Mehrara B. J. (2020). Regulation of lymphatic function in obesity. Front. Physiol. 11, 459. doi:10.3389/fphys.2020.00459
Kim H. J., Li M., Nichols C. G., Davis M. J. (2021). Large-conductance calcium-activated K(+) channels, rather than K(ATP) channels, mediate the inhibitory effects of nitric oxide on mouse lymphatic pumping. Br. J. Pharmacol. 178 (20), 4119–4136. doi:10.1111/bph.15602
Kim H. J., Norton C. E., Zawieja S. D., Castorena-Gonzalez J. A., Davis M. J. (2024). Acute metabolic stress induces lymphatic dysfunction through KATP channel activation. Funct. (Oxf) 5 (5), zqae033. doi:10.1093/function/zqae033
Lapinski P. E., Lubeck B. A., Chen D., Doosti A., Zawieja S. D., Davis M. J., et al. (2017). RASA1 regulates the function of lymphatic vessel valves in mice. J. Clin. Invest 127 (7), 2569–2585. doi:10.1172/JCI89607
Lee Y., Chakraborty S., Muthuchamy M. (2020). Roles of sarcoplasmic reticulum Ca(2+) ATPase pump in the impairments of lymphatic contractile activity in a metabolic syndrome rat model. Sci. Rep. 10 (1), 12320. doi:10.1038/s41598-020-69196-4
Lee Y., Zawieja S. D., Muthuchamy M. (2022). Lymphatic collecting vessel: new perspectives on mechanisms of contractile regulation and potential lymphatic contractile pathways to target in obesity and metabolic diseases. Front. Pharmacol. 13, 848088. doi:10.3389/fphar.2022.848088
Liao S., Padera T. P. (2013). Lymphatic function and immune regulation in health and disease. Lymphat. Res. Biol. 11 (3), 136–143. doi:10.1089/lrb.2013.0012
Mehrara B. J., Greene A. K. (2014). Lymphedema and obesity: is there a link? Plast. Reconstr. Surg. 134 (1), 154e–160e. doi:10.1097/PRS.0000000000000268
Petrova T. V., Koh G. Y. (2018). Organ-specific lymphatic vasculature: from development to pathophysiology. J. Exp. Med. 215 (1), 35–49. doi:10.1084/jem.20171868
Petrova T. V., Koh G. Y. (2020). Biological functions of lymphatic vessels. Science 369 (6500), eaax4063. doi:10.1126/science.aax4063
Randolph G. J., Ivanov S., Zinselmeyer B. H., Scallan J. P. (2017). The lymphatic system: integral roles in immunity. Annu. Rev. Immunol. 35, 31–52. doi:10.1146/annurev-immunol-041015-055354
Rockson S. G. (2020). The role of body mass index in breast cancer-associated lymphedema. Lymphat. Res. Biol. 18 (6), 501. doi:10.1089/lrb.2020.29095.sr
Rockson S. G. (2021). Advances in lymphedema. Circ. Res. 128 (12), 2003–2016. doi:10.1161/CIRCRESAHA.121.318307
Rockson S. G. (2023). Breast cancer-related lymphedema: the primary/secondary conundrum. Lymphat. Res. Biol. 21 (2), 99–100. doi:10.1089/lrb.2023.29141.editorial
Scallan J. P., Davis M. J. (2013). Genetic removal of basal nitric oxide enhances contractile activity in isolated murine collecting lymphatic vessels. J. Physiol. 591 (8), 2139–2156. doi:10.1113/jphysiol.2012.250662
Scallan J. P., Hill M. A., Davis M. J. (2015). Lymphatic vascular integrity is disrupted in type 2 diabetes due to impaired nitric oxide signalling. Cardiovasc Res. 107 (1), 89–97. doi:10.1093/cvr/cvv117
Siotos C., Arnold S. H., Seu M., Lunt L., Ferraro J., Najafali D., et al. (2024). Breast cancer-related lymphedema: a comprehensive analysis of risk factors. J. Surg. Oncol. 130, 1521–1531. doi:10.1002/jso.27841
Stritt S., Koltowska K., Makinen T. (2021). Homeostatic maintenance of the lymphatic vasculature. Trends Mol. Med. 27 (10), 955–970. doi:10.1016/j.molmed.2021.07.003
Sudduth C. L., Greene A. K. (2022a). Current overview of obesity-induced lymphedema. Adv. Wound Care (New Rochelle) 11 (7), 392–398. doi:10.1089/wound.2020.1337
Sudduth C. L., Greene A. K. (2022b). Lymphedema and obesity. Cold Spring Harb. Perspect. Med. 12 (5), a041176. doi:10.1101/cshperspect.a041176
Tsai R. J., Dennis L. K., Lynch C. F., Snetselaar L. G., Zamba G. K. D., Scott-Conner C. (2018). Lymphedema following breast cancer: the importance of surgical methods and obesity. Front. Womens Health 3 (2). doi:10.15761/FWH.1000144
Weitman E. S., Aschen S. Z., Farias-Eisner G., Albano N., Cuzzone D. A., Ghanta S., et al. (2013). Obesity impairs lymphatic fluid transport and dendritic cell migration to lymph nodes. PLoS One 8 (8), e70703. doi:10.1371/journal.pone.0070703
Zawieja S. D., Castorena-Gonzalez J. A., Scallan J. P., Davis M. J. (2018). Differences in L-type Ca(2+) channel activity partially underlie the regional dichotomy in pumping behavior by murine peripheral and visceral lymphatic vessels. Am. J. Physiol. Heart Circ. Physiol. 314 (5), H991–H1010. doi:10.1152/ajpheart.00499.2017
Zawieja S. D., Gasheva O., Zawieja D. C., Muthuchamy M. (2016a). Blunted flow-mediated responses and diminished nitric oxide synthase expression in lymphatic thoracic ducts of a rat model of metabolic syndrome. Am. J. Physiol. Heart Circ. Physiol. 310 (3), H385–H393. doi:10.1152/ajpheart.00664.2015
Zawieja S. D., Wang W., Chakraborty S., Zawieja D. C., Muthuchamy M. (2016b). Macrophage alterations within the mesenteric lymphatic tissue are associated with impairment of lymphatic pump in metabolic syndrome. Microcirculation 23 (7), 558–570. doi:10.1111/micc.12307
Keywords: lymphatic vessel, contractile dysfunction, db/db, lymphedema, KATP
Citation: Castorena-Gonzalez JA, Kim HJ and Davis MJ (2025) Chronic metabolic stress impairs lymphatic contractility via activation of KATP channels in a mouse model of Type-2 diabetes. Front. Physiol. 16:1558763. doi: 10.3389/fphys.2025.1558763
Received: 10 January 2025; Accepted: 14 April 2025;
Published: 30 April 2025.
Edited by:
Irena Levitan, University of Illinois Chicago, United StatesReviewed by:
Al-Shaimaa Faissal Ahmed, Minia University, EgyptIbra S. Fancher, University of Delaware, United States
Copyright © 2025 Castorena-Gonzalez, Kim and Davis. This is an open-access article distributed under the terms of the Creative Commons Attribution License (CC BY). The use, distribution or reproduction in other forums is permitted, provided the original author(s) and the copyright owner(s) are credited and that the original publication in this journal is cited, in accordance with accepted academic practice. No use, distribution or reproduction is permitted which does not comply with these terms.
*Correspondence: Michael J. Davis, ZGF2aXNtakBoZWFsdGgubWlzc291cmkuZWR1
†These authors have contributed equally to this work