- Department of Neuroscience, Mortimer B. Zuckerman Mind Brain Behavior Institute, Kavli Institute for Brain Science, Columbia University Medical Center, New York, NY, USA
Calcium (Ca2+) plays innumerable critical functions in neurons ranging from regulation of neurotransmitter release and synaptic plasticity to activity-dependent transcription. Therefore, more than any other cell types, neurons are critically dependent on spatially and temporally controlled Ca2+ dynamics. This is achieved through an exquisite level of compartmentalization of Ca2+ storage and release from various organelles. The function of these organelles in the regulation of Ca2+ dynamics has been studied for decades using electrophysiological and optical methods combined with pharmacological and genetic alterations. Mitochondria and the endoplasmic reticulum (ER) are among the organelles playing the most critical roles in Ca2+ dynamics in neurons. At presynaptic boutons, Ca2+ triggers neurotransmitter release and synaptic plasticity, and postsynaptically, Ca2+ mobilization mediates long-term synaptic plasticity. To explore Ca2+ dynamics in live cells and intact animals, various synthetic and genetically encoded fluorescent Ca2+ sensors were developed, and recently, many groups actively increased the sensitivity and diversity of genetically encoded Ca2+ indicators (GECIs). Following conjugation with various signal peptides, these improved GECIs can be targeted to specific subcellular compartments, allowing monitoring of organelle-specific Ca2+ dynamics. Here, we review recent findings unraveling novel roles for mitochondria- and ER-dependent Ca2+ dynamics in neurons and at synapses.
Introduction
Calcium (Ca2+) ions govern prevalent physiological processes in various cell types (Rizzuto and Pozzan, 2006; Clapham, 2007). This is especially prominent in excitable cells like neurons where Ca2+ influx through the plasma membrane and release of Ca2+ from internal stores transduce the effects of changes in membrane polarization and therefore mediate faithful transfer or storage of information over various timescales (milliseconds to minutes/hours). Therefore, regulation of intracellular Ca2+ homeostasis is central to the proper function of neuronal circuits. The maintenance of baseline levels of intracellular Ca2+ levels is regulated in part through exchangers and pumps such as the plasma membrane Ca2+-ATPase (PMCA pump), the Na+/Ca2+ exchanger (NCX), and the Na+/Ca2+-K+ exchanger (NCKX) which extrude Ca2+ through the plasma membrane into the extracellular space. In addition to these mechanisms, intracellular organelles, such as mitochondria and endoplasmic reticulum (ER), are able to regulate cytoplasmic Ca2+ ([Ca2+]c) through mitochondrial calcium uniporter (MCU) and smooth endoplasmic reticulum Ca2+-ATPase (SERCA), respectively.
In neurons, mitochondria and ER play important physiological roles via [Ca2+]c regulation, thereby diverse synaptic functions including basal synaptic transmission, presynaptic short-term plasticity, and long-term plasticity can be regulated by these organelles (Verkhratsky, 2005; Bardo et al., 2006; Mattson et al., 2008; Vos et al., 2010). In addition, impaired Ca2+ homeostasis in the nervous system has been proposed to play an important function in the physio-pathological mechanisms underlying Alzheimer’s disease, Parkinson’s disease, and spinocerebellar ataxia (Verkhratsky, 2005; Mattson et al., 2008; Schon and Przedborski, 2011).
To monitor Ca2+ dynamics, various fluorescent Ca2+ dyes and genetically encoded Ca2+ indicators (GECIs) were developed and applied both in vitro and in vivo. Also, GECIs tagged with target peptide sequences have allowed imaging of Ca2+ dynamics in specific organelles (Rizzuto et al., 1992; Palmer et al., 2004; Palmer and Tsien, 2006).
Previously published reviews have already summarized the usefulness and limitations of various Ca2+ sensors and GECIs applied to neuronal and non-neuronal cells (Palmer and Tsien, 2006; Knopfel, 2012; Tian et al., 2012; Rose et al., 2014). In this review, we only describe recently uncovered insights about Ca2+ dynamics and its regulation by mitochondria and ER, and we discuss how these organelle-specific Ca2+ sensors have been used for the exploration of the role of these subcellular compartments in the regulation of Ca2+ homeostasis and synaptic function in neurons.
Unveiled Synaptic Functions of Mitochondria-Dependent Ca2+ Homeostasis
Mitochondrial Ca2+ uptake has been studied since the 1950s from studies of rat heart muscle and kidney (Slater and Cleland, 1953; Deluca and Engstrom, 1961). In the nervous system, mitochondria were described at presynaptic terminals and dendrites of various neuronal subtypes using the light and electron microscope (EM) several decades ago (Bartelmez and Hoerr, 1933; Palay, 1956; Gray, 1963; Shepherd and Harris, 1998; Rowland et al., 2000). In axons, mitochondria are short and sparsely distributed, and interestingly, several studies showed that half of presynaptic boutons are occupied by mitochondria (Shepherd and Harris, 1998; Kang et al., 2008). In contrast, dendritic mitochondria have tubular shapes and they are rarely observed in postsynaptic spines in the excitatory neurons (Sheng and Hoogenraad, 2007; Kasthuri et al., 2015).
At presynaptic boutons and terminals, synaptic vesicle (SV) fusion with the plasma membrane occurs following increase of [Ca2+]c following opening of voltage-sensitive Ca2+ channels (VSCC) followed by Ca2+ binding to sensors like synaptotagmins (Schneggenburger and Neher, 2005; Neher and Sakaba, 2008; Jahn and Fasshauer, 2012; Südhof, 2012). The ability of mitochondria to import Ca2+ into the mitochondrial matrix ([Ca2+]m) plays a role in regulating presynaptic [Ca2+]c. This has been characterized in various species, neuronal cell types and circuits (Figure 1A). At the Drosophila neuromuscular junction (NMJ), the GTPase dMiro mutant lacks presynaptic mitochondria through impaired axonal transport (Guo et al., 2005; Wang and Schwarz, 2009). During prolonged stimulation, these mutants lacking presynaptic mitochondria displayed subtle, but significantly increased presynaptic Ca2+ accumulation and display decrease forms of sustained synaptic transmission or synaptic “fatigue” (Guo et al., 2005). Drosophila Drp1 mutants also deplete presynaptic mitochondria at NMJ and exhibit elevated presynaptic Ca2+ levels in resting and evoked states. However, spontaneous release (mini Excitatory junctional potential, mEJP) was not altered, but the evoked synaptic transmission was impaired during high frequency stimulation, and this defect was partially rescued by ATP (Verstreken et al., 2005) suggesting that mitochondria plays a role in synaptic transmission through their ability to generate ATP through oxidative phosphorylation. Although mitochondrial Ca2+ uptake has limited effects on Drosophila NMJ neurons, in mammalian NMJ terminals, acute inhibition of mitochondrial Ca2+ uptake causes rapid depression of the endplate potential (EPP) and increased asynchronous release (David and Barrett, 2003). Furthermore, in synapses of the mammalian central nervous system (CNS), mitochondria-dependent Ca2+ uptake accelerates the recovery from synaptic depression in the calyx of Held (Billups and Forsythe, 2002). Other studies in mammalian hippocampal neurons claimed that impaired mitochondrial anchoring at presynaptic sites increases presynaptic Ca2+ during repetitive stimulation and produces short-term facilitation (STF), and insulin-like growth factor-1 receptor (IGF-1R) signaling regulates resting mitochondrial Ca2+ level and spontaneous transmission (Kang et al., 2008; Gazit et al., 2016). Although most pharmacological studies employed uncoupling agents as mitochondrial Ca2+ influx blocker, which may affect ATP production, these reports support presynaptic control via mitochondrial Ca2+ import (Ly and Verstreken, 2006). A recent study demonstrates that presynaptic boutons associated with mitochondria display lower levels of [Ca2+]c accumulation than presynaptic boutons not associated with mitochondria (Kwon et al., 2016). Furthermore, acute inhibition of mitochondria calcium import increased [Ca2+]c accumulation at presynaptic boutons occupied by mitochondria. In the same study, we demonstrate that this mitochondria-dependent regulation of [Ca2+]c plays an important role in regulating presynaptic release properties including spontaneous release, asynchronous release and short-term synaptic plasticity.
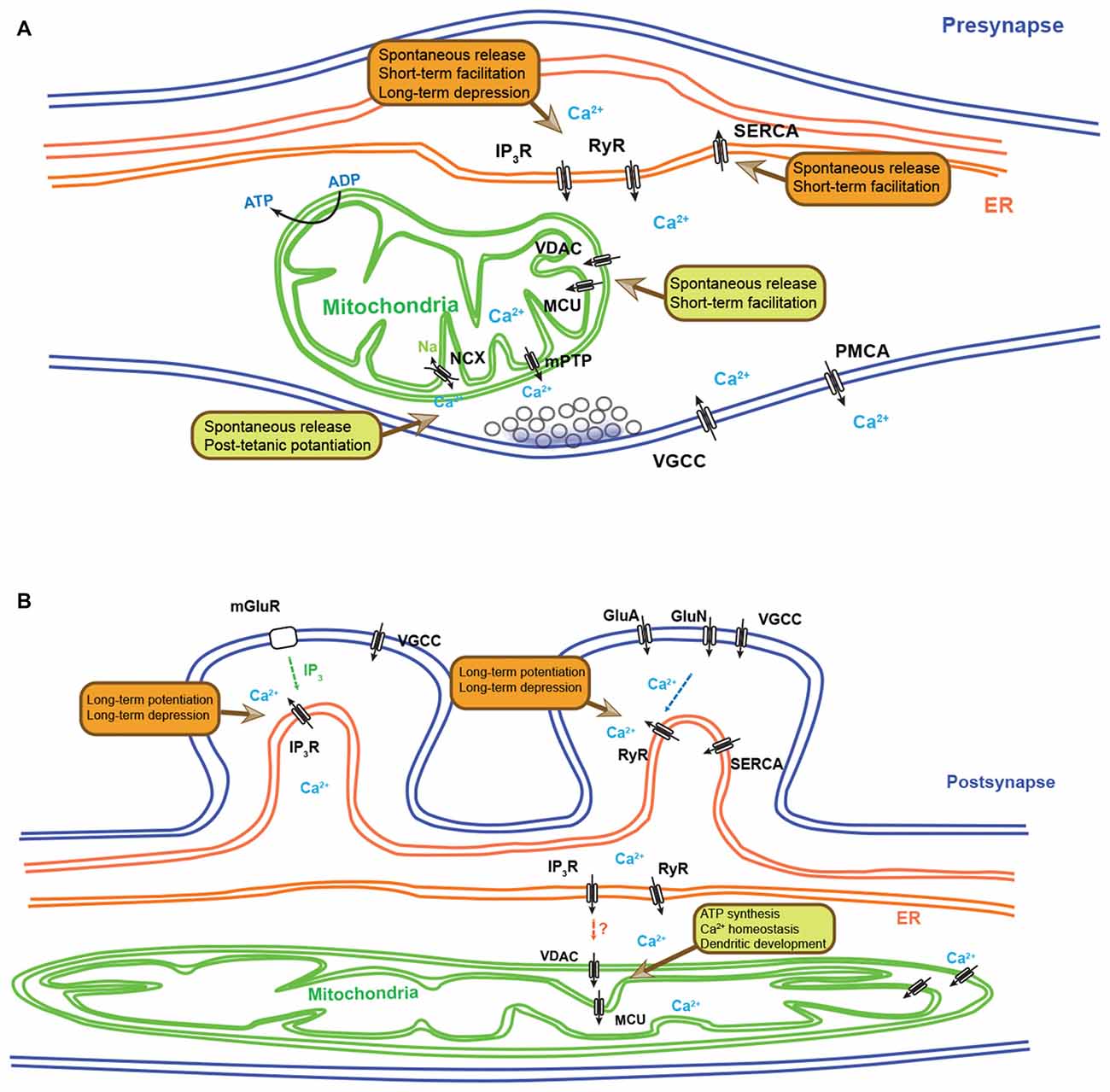
Figure 1. Synaptic functions regulated by endoplasmic reticulum (ER) and mitochondria-dependent Ca2+ homeostasis. (A) Schematic diagram depicting the presynaptic functions regulated by ER- and mitochondria-dependent Ca2+ dynamics. Ca2+ release from ER can modulate spontaneous neurotransmitter release, short-term facilitation (STF) and long-term depression (LTD). Ca2+ re-uptake by the ER controls the spontaneous release and STF. Presynaptic mitochondria also play important roles in regulating spontaneous neurotransmitter release, STF and post-tetanic potentiation (PTP) through their ability to regulate Ca2+ clearance. (B) A simplified schematic diagram depicting the postsynaptic functions regulated by ER- and mitochondria-dependent Ca2+ dynamics. Ca2+ release from ER via IP3-induced Ca2+ release (IICR) and Ca2+-induced Ca2+ release (CICR) controls long-term potentiation (LTP) and LTD. In fact, depending on neuronal and synaptic subtypes, IP3R and RyR show differential distribution and distinct synaptic functions. Dendritic mitochondrial Ca2+ influx can regulate ATP synthesis, Ca2+ homeostasis and dendritic development. In non-neuronal cell types, direct Ca2+ exchange between ER and mitochondria have been described, but their role in neurons has not yet been documented. IP3R, IP3 receptor; RyR, ryanodine receptor; SERCA, smooth endoplasmic reticulum Ca2+-ATPase; VGCC, voltage-gated Ca2+ channel; PMCA, plasma membrane Ca2+-ATPase; NCX: the Na+/Ca2+ exchanger; mPTP, mitochondrial permeability transition pore; MCU, mitochondrial calcium uniporter; VDAC, voltage-dependent anion channel; mGluR, metabotropic glutamate receptor; GluN, NMDA receptor.
In addition to regulation of [Ca2+]c clearance, Ca2+ release from mitochondria plays important roles at presynaptic sites (Figure 1A). Following the sustained high frequency stimulation, an enhancement of synaptic transmission lasting tens of seconds to minutes is observed and which is called post-tetanic potentiation (PTP; Zucker, 1989). Mitochondrial Ca2+ release is suggested as one of the underlying mechanisms for this prolonged enhancement of synaptic transmission. Pharmacological inhibition of mitochondrial Ca2+ uptake and release at the crayfish NMJ impaired PTP (Tang and Zucker, 1997; Zhong et al., 2001). Furthermore, similar phenotypes were observed at mouse NMJ and hippocampal mossy fiber synapses with blocking the mitochondrial NCX, which mediates mitochondrial Ca2+ release (García-Chacón et al., 2006; Lee et al., 2007).
In contrast to presynaptic boutons and terminals, the postsynaptic function of mitochondrial Ca2+ regulation is less well-documented. In mouse hippocampal pyramidal neurons (Li et al., 2004), a minority (<5%) of dendritic spines contains mitochondria. Also, large branched spines in hippocampal CA3 contain mitochondria (Chicurel and Harris, 1992). However, a physiological role of these postsynaptic mitochondria is largely unknown. In general, mitochondria are distributed primarily in dendrite shaft and therefore localized microns away from the postsynaptic density, but might still be able to buffer [Ca2+]c mobilized through Ca2+-channels and glutamate receptors (Thayer and Miller, 1990; White and Reynolds, 1995; Wang and Thayer, 2002). This mitochondrial calcium import can stimulate tricarboxylic acid (TCA) cycle and might increase ATP production (Kann and Kovács, 2007) and may also regulate other ATP-dependent Ca2+ pumps like PMCA and SERCA. While it is still unclear whether or not mitochondria play significant roles in regulating postsynaptic [Ca2+]c under physiological conditions of neurotransmission, they might play a role in pathophysiological contexts. For example, neurons lacking LRRK2, a protein associated with Parkinson’s disease, show impaired dendritic Ca2+ homeostasis through mitochondrial defects and thought to cause defective mitochondrial depolarization and reduction in dendritic complexity (Figure 1B; Cherra et al., 2013).
Overall, mitochondria-dependent Ca2+ clearance and release in neurons plays important physiological and developmental roles pre- and post-synaptically but their functional importance seems to depend on the neuronal subtypes and the structure/size of the pre- and postsynaptic compartments.
Mitochondrial Ca2+-Imaging in Neurons and at Synapses
To investigate organelle-specific Ca2+ dynamics, various Ca2+ sensors are developed (Table 1). One of the first method developed to monitor mitochondrial Ca2+ dynamics was established using rhod-2, a cationic chemical Ca2+-binding fluorophore preferentially accumulating in the mitochondrial matrix presumably because of the highly negative membrane potential across the mitochondrial inner membrane (Minta et al., 1989). Then, in the calyx of Held, rhod-2 and rhod-FF (low affinity version) were used to visualize presynaptic mitochondrial Ca2+ transient (Billups and Forsythe, 2002). However, these dyes cannot be precisely targeted to these organelles. Therefore, GECIs have recently become the preferred method to image Ca2+ in specific organelles including mitochondria. For mitochondrial matrix localization, the targeting presequence of subunit VIII of human cytochrome c oxidase (COXVIII) was tagged to GECIs (Rizzuto et al., 1992). Mitochondria-targeted aequorin (mt-AEQ), a luminescent Ca2+ indicator, was first employed to monitor the neuronal mitochondrial Ca2+, and this probe showed NMDA-induced mitochondrial Ca2+ increase in hippocampal neurons (Baron et al., 2003). However, this probe needs a chemical reaction characterized by a modest turnover rate and has very limited dynamic range (Palmer and Tsien, 2006). Other GECIs have been developed and tested in various neuronal subtypes with the same targeting sequence. Mitochondrial-targeted ratiometric pericam (2mtRP) consists of circularly permutated Enhanced yellow fluorescent protein (cpEFYP) conjugated with Ca2+-responsive calmodulin (CaM) and its binding peptide (Nagai et al., 2001; Robert et al., 2001). This probe has a bimodal excitation spectrum and the relative emission intensity is dependent on Ca2+-binding. In hippocampal neurons, the use of 2mtRP described mitochondrial Ca2+ uptake and also determined cytosolic Ca2+ rise upon synaptic activation via dual imaging with cytosolic Ca2+ dye (fura-red AM; Young et al., 2008). Other CaM conjugated cpEGFPs called GCaMPs (mito-GCaMP2, 2mtGCaMP6m, and mito-GCaMP5G) were used to monitor axonal mitochondrial Ca2+ (Gazit et al., 2016; Kwon et al., 2016; Marland et al., 2016). Both sensors displayed action potential (AP)-dependent mitochondrial Ca2+ import. In addition, red fluorescent GECIs by replacing cpEGFP with cpmApple or cpmRuby (mtRCaMP1e and LAR-GECO1.2) revealed mitochondrial Ca2+ import simultaneously with cytosolic Ca2+ (Akerboom et al., 2013; Wu et al., 2014).
However, these fluorescent proteins have some limitations, for example, they are affected by pH and mitochondrial matrix pH (pHm) can be changed by Ca2+ influx (Abad et al., 2004; Poburko et al., 2011; Chouhan et al., 2012; Marland et al., 2016). In addition to this point, [Ca2+]m can span broad ranges (0.05–300 μM) depending on cell types and stimulation protocol (Arnaudeau et al., 2001; Palmer and Tsien, 2006). Thus, Kd value for Ca2+ of mitochondrial GECI should be considered for experimental purposes because high affinity (low Kd) sensors can be easily saturated by high [Ca2+]m and low affinity (high Kd) sensors may not be sensitive enough to detect small [Ca2+]m changes. Several studies reported low affinity mitochondrial Ca2+ probes for avoiding saturation (Arnaudeau et al., 2001; Suzuki et al., 2014).
In conclusion, these mitochondria-targeted GECIs allow imaging of mitochondria Ca2+ dynamics in neurons and have revealed interesting, synapse-specific properties of mitochondria in the regulation of [Ca2+]c and neurotransmitter release properties.
Regulation of Synaptic Ca2+ Dynamics by the Endoplasmic Reticulum
Neurons are among the most polarized cell types in our body and consists of a soma, relatively short dendrites and long axons. ER is found throughout the entire length of neuronal processes, and usually rough ER is prominent in the cell body and proximal dendrites, whereas smooth ER is dominant in distal dendrites, spines and axons (Spacek and Harris, 1997; Verkhratsky, 2005). ER imports and sequesters large amount of Ca2+ ([Ca2+]er ~500 μM) through SERCA and store-operated Ca2+ entry (SOCE) mechanism (Verkhratsky, 2005; Bardo et al., 2006). Ca2+ release from ER is mediated by two major mechanisms, called Ca2+-induced Ca2+ release (CICR) and IP3-induced Ca2+ release (IICR; Verkhratsky, 2005; Bardo et al., 2006). CICR is caused by the cytosolic Ca2+ increase through N-Methyl-D-Aspartate receptors (NMDAR, GluN receptors) and voltage-gated Ca2+ channels (VGCCs), whereas IICR is triggered by IP3, which is generated via activation of phospholipase C (PLC) depending on metabotropic glutamate receptors (mGluRs) or other receptors like receptor tyrosine kinases (Figure 1).
Ryanodine receptors (RyRs) are involved in CICR, and they have three major subtypes; RyR1, RyR2, and RyR3. All of these isoforms are detected in the brain, and show region-specific expression (Sharp et al., 1993; Furuichi et al., 1994; Giannini et al., 1995; Verkhratsky, 2005; Bardo et al., 2006; Baker et al., 2013). Similar to RyRs, IP3 receptor (IP3R), which mediate IICR, consist of three isoforms, IP3R1, IP3R2, and IP3R3, but IP3R1 is the dominant form in the brain (Sharp et al., 1993, 1999; Verkhratsky, 2005; Bardo et al., 2006; Baker et al., 2013).
Long-term synaptic plasticity is regulated by Ca2+-dependent signaling mechanisms such as Ca2+/calmodulin-dependent kinase II (CaMKII), calcineurin (a Ca2+-dependent phosphatase), protein phosphatase 1 (PP1) and protein kinase C (PKC; Malenka and Nicoll, 1999; Yang et al., 1999; Lüscher and Malenka, 2012). Therefore, Ca2+ release from intracellular stores like the ER regulates long-term synaptic plasticity in specific circuits.
In cerebellar Purkinje cell dendrites, mGluR-IP3-dependent Ca2+ increase is observed during parallel fiber (PF) stimulation and this mediates long-term depression (LTD) of PF-Purkinje cell pathway (Finch and Augustine, 1998; Takechi et al., 1998; Miyata et al., 2000; Wang et al., 2000). At synapses made by hippocampal Schaffer collateral (SC) onto CA1 pyramidal neurons, both long-term potentiation (LTP) and LTD are linked to IP3-dependent signaling (Oliet et al., 1997; Nishiyama et al., 2000; Raymond and Redman, 2002; Nagase et al., 2003). In addition, CICR is also observed in CA1 pyramidal neuronal spines, and LTD is abolished in RyR3-deficient mice and following application of RyR inhibitor (ryanodine) although the connection between CICR and LTP is controversial in SC-CA1 pathway (Reyes and Stanton, 1996; Emptage et al., 1999; Futatsugi et al., 1999; Sandler and Barbara, 1999; Kovalchuk et al., 2000; Nishiyama et al., 2000; Raymond and Redman, 2002). Hippocampal mossy fiber pathway (MF, dentate gyrus to CA3) shows IICR- and CICR-dependent LTP and LTD, however, there are conflicting results regarding the underlying mechanisms (Figure 1B; Yeckel et al., 1999; Itoh et al., 2001; Kapur et al., 2001; Mellor and Nicoll, 2001; Lauri et al., 2003; Lei et al., 2003).
Presynaptic ER-dependent Ca2+ release is also detected and contributes to changes in neurotransmitter release properties and short-term synaptic plasticity at various inhibitory and excitatory synapses including basket cell to Purkinje cell synapses, hippocampal MF pathway, SC-CA1 and CA3-CA3 pyramidal neuron synapses (Figure 1A; Llano et al., 2000; Emptage et al., 2001; Liang et al., 2002; Galante and Marty, 2003; Lauri et al., 2003; Sharma and Vijayaraghavan, 2003; Unni et al., 2004; Mathew and Hablitz, 2008).
In addition to Ca2+ efflux, Ca2+ uptake by ER via SERCA pump affects STF at SC-CA1 presynapses and NMJ (Figure 1A; Castonguay and Robitaille, 2001; Scullin and Partridge, 2010; Scullin et al., 2010). Stromal interaction molecules (STIMs) and Orai1, which allow SOCE, are localized to neuronal compartment including dendritic spines, and impaired SOCE alters α-amino-3-hydroxy-5-methyl-4-isoxazolepropionic acid receptor (AMPAR) trafficking, neuronal Ca2+ signaling and LTP in CA1 pyramidal neuron and cerebellar Purkinje neurons (Baba et al., 2003; Hartmann et al., 2014; Korkotian et al., 2014; Garcia-Alvarez et al., 2015; Segal and Korkotian, 2015).
Imaging Neuronal ER Ca2+ Dynamics
As mentioned above, ER contains high levels of Ca2+, therefore, in order to monitor Ca2+ dynamics in the ER lumen, low affinity sensors were employed. Mag-Fura-2, a low affinity membrane-permeable dye (Kd = 53 μM), has been the first applied for neuronal ER Ca2+ measurement, and after loading this dye in the cytoplasm and organelles, cytosolic dye was removed by perfusion with dye-free pipette solution (Solovyova et al., 2002). This allowed for the first time the visualization of caffeine-induced Ca2+ release and reuptake in the ER of dorsal root ganglia (DRG) neurons (Table 1).
However, this method can non-specifically label internal compartments, therefore, genetically targeted sensors have been developed more recently for the visualization of ER-derived Ca2+ dynamics (Table 1). The signal sequence of calreticulin, a Ca2+-binding protein in ER, and an ER retention sequence, KDEL, lead GECIs into the ER lumen, and to optimize for measuring a massive amount of [Ca2+]er, various mutations were applied to the CaM domain or EF-hand motif of existing GECIs for reducing their Ca2+ affinity. A fluorescence resonance energy transfer (FRET)-based Ca2+ sensor, D1ER, showed altered ER Ca2+ leak function in hippocampal neurons of presenilin double knockout and Alzheimer’s disease model mice (Zhang et al., 2010). Also, bioluminescence-based sensor, GFP-Aequorin protein (GAP), was modified and targeted to ER of DRG and hippocampal neurons, and showed 3- to 4-fold larger ratio change than D1ER (Rodriguez-Garcia et al., 2014). In addition, recently established GCaMP variants for ER Ca2+ detection, calcium-measuring organelle-entrapped protein indicator one in the ER (CEPIA1er) and GCaMPer (10.19), characterized ER Ca2+ uptake and release in cortical neurons and cerebellar Purkinje cells (Suzuki et al., 2014; Henderson et al., 2015). Interestingly, cerebellar Purkinje cells displayed differential ER Ca2+ dynamics in postsynaptic compartment depending on the nature of synaptic inputs (Okubo et al., 2015). These lumenal ER Ca2+ indicators also revealed interesting dynamics in dendritic spines, which suggest that [Ca2+]er and therefore [Ca2+]c can undergo synapse-specific regulation (Suzuki et al., 2014; Henderson et al., 2015).
Future Perspectives
Recent studies characterized the roles of presynaptic mitochondria and circuit-specific ER Ca2+ mobility in dendrites directly via live imaging (Okubo et al., 2015; Kwon et al., 2016), however, organelle-specific Ca2+ dynamics at local synapses is only beginning to be explored. Genetically-encoded Ca2+ sensors targeted to intracellular organelle and/or to specific synapses as well as functional indicators (like pHluorin-tagged synaptophysin or GluRs) will lead to the identification of synapse- and circuit-specific roles of mitochondria and ER Ca2+ in neurons.
MCU has been recently shown to be associated with multiple regulatory proteins, which seems to modify or gate its gating properties and can prevent or enhance mitochondrial Ca2+ uptake upon changes in cytosolic Ca2+ dynamics (Perocchi et al., 2010; Mallilankaraman et al., 2012; Csordás et al., 2013; Plovanich et al., 2013; Raffaello et al., 2013; Sancak et al., 2013; De Stefani et al., 2015). In addition, MCU activity can be differentially controlled in different tissues (Fieni et al., 2012). Therefore, future investigations should probe the function of this MCU-regulatory complex in neurons and test if MCU and/or MCU-associated proteins can act as neuronal subtype-specific and/or synapse-specific functional modifiers.
In non-neuronal cells, ER and mitochondria establish focal connections which play a key role in Ca2+ transfer from ER to mitochondria which has been characterized via intra- and inter-organelle Ca2+ imaging (Rizzuto et al., 1993, 2012; Csordás et al., 2010; Kornmann, 2013). This transfer modulates ATP production in mitochondria and may also affect lipid exchange between these two organelles (Voelker, 1990; Cárdenas et al., 2010; Fujimoto and Hayashi, 2011). At present, in neurons, the role of Ca2+ translocation between ER and mitochondria is largely unknown. Although immuno-EM images in vivo and Ca2+ imaging with dyes in respiratory motor neurons suggested ER-mitochondria Ca2+ crosstalk, future work will need to establish the context in which ER-mitochondria interface regulates Ca2+ dynamics and synaptic function (Takei et al., 1992; Shoshan-Barmatz et al., 2004; Mironov and Symonchuk, 2006).
Author Contributions
All three authors co-wrote the manuscript.
Funding
This work was partially funded by support from the NIH-NINDS (R01NS067557, FP), Japan society for the promotion of science fellowship for research abroad and The Uehara Memorial Foundation (YH), and a grant from the Human Frontier Science Program long-term fellowship (S-KK).
Conflict of Interest Statement
The authors declare that the research was conducted in the absence of any commercial or financial relationships that could be construed as a potential conflict of interest.
References
Abad, M. F., Di Benedetto, G., Magalhães, P. J., Filippin, L., and Pozzan, T. (2004). Mitochondrial pH monitored by a new engineered green fluorescent protein mutant. J. Biol. Chem. 279, 11521–11529. doi: 10.1074/jbc.m306766200
Akerboom, J., Carreras Calderón, N., Tian, L., Wabnig, S., Prigge, M., Tolö, J., et al. (2013). Genetically encoded calcium indicators for multi-color neural activity imaging and combination with optogenetics. Front. Mol. Neurosci. 6:2. doi: 10.3389/fnmol.2013.00002
Arnaudeau, S., Kelley, W. L., Walsh, J. V. Jr., and Demaurex, N. (2001). Mitochondria recycle Ca2+ to the endoplasmic reticulum and prevent the depletion of neighboring endoplasmic reticulum regions. J. Biol. Chem. 276, 29430–29439. doi: 10.1074/jbc.m103274200
Baba, A., Yasui, T., Fujisawa, S., Yamada, R. X., Yamada, M. K., Nishiyama, N., et al. (2003). Activity-evoked capacitative Ca2+ entry: implications in synaptic plasticity. J. Neurosci. 23, 7737–7741.
Baker, K. D., Edwards, T. M., and Rickard, N. S. (2013). The role of intracellular calcium stores in synaptic plasticity and memory consolidation. Neurosci. Biobehav. Rev. 37, 1211–1239. doi: 10.1016/j.neubiorev.2013.04.011
Bardo, S., Cavazzini, M. G., and Emptage, N. (2006). The role of the endoplasmic reticulum Ca2+ store in the plasticity of central neurons. Trends Pharmacol. Sci. 27, 78–84. doi: 10.1016/j.tips.2005.12.008
Baron, K. T., Wang, G. J., Padua, R. A., Campbell, C., and Thayer, S. A. (2003). NMDA-evoked consumption and recovery of mitochondrially targeted aequorin suggests increased Ca2+ uptake by a subset of mitochondria in hippocampal neurons. Brain Res. 993, 124–132. doi: 10.1016/j.brainres.2003.09.022
Bartelmez, G. W., and Hoerr, N. L. (1933). The vestibular club endings in ameiurus. Further evidence on the morphology of the synapse. J. Comp. Neurol. 57, 401–428. doi: 10.1002/cne.900570303
Billups, B., and Forsythe, I. D. (2002). Presynaptic mitochondrial calcium sequestration influences transmission at mammalian central synapses. J. Neurosci. 22, 5840–5847.
Cárdenas, C., Miller, R. A., Smith, I., Bui, T., Molgo, J., Muller, M., et al. (2010). Essential regulation of cell bioenergetics by constitutive InsP3 receptor Ca2+ transfer to mitochondria. Cell 142, 270–283. doi: 10.1016/j.cell.2010.06.007
Castonguay, A., and Robitaille, R. (2001). Differential regulation of transmitter release by presynaptic and glial Ca2+ internal stores at the neuromuscular synapse. J. Neurosci. 21, 1911–1922.
Cherra, S. J. III, Steer, E., Gusdon, A. M., Kiselyov, K., and Chu, C. T. (2013). Mutant LRRK2 elicits calcium imbalance and depletion of dendritic mitochondria in neurons. Am. J. Pathol. 182, 474–484. doi: 10.1016/j.ajpath.2012.10.027
Chicurel, M. E., and Harris, K. M. (1992). Three-dimensional analysis of the structure and composition of CA3 branched dendritic spines and their synaptic relationships with mossy fiber boutons in the rat hippocampus. J. Comp. Neurol. 325, 169–182. doi: 10.1002/cne.903250204
Chouhan, A. K., Ivannikov, M. V., Lu, Z., Sugimori, M., Llinas, R. R., and Macleod, G. T. (2012). Cytosolic calcium coordinates mitochondrial energy metabolism with presynaptic activity. J. Neurosci. 32, 1233–1243. doi: 10.1523/jneurosci.1301-11.2012
Csordás, G., Golenár, T., Seifert, E. L., Kamer, K. J., Sancak, Y., Perocchi, F., et al. (2013). MICU1 controls both the threshold and cooperative activation of the mitochondrial Ca2+ uniporter. Cell Metab. 17, 976–987. doi: 10.1016/j.cmet.2013.04.020
Csordás, G., Várnai, P., Golenár, T., Roy, S., Purkins, G., Schneider, T. G., et al. (2010). Imaging interorganelle contacts and local calcium dynamics at the ER-mitochondrial interface. Mol. Cell 39, 121–132. doi: 10.1016/j.molcel.2010.06.029
David, G., and Barrett, E. F. (2003). Mitochondrial Ca2+ uptake prevents desynchronization of quantal release and minimizes depletion during repetitive stimulation of mouse motor nerve terminals. J. Physiol. 548, 425–438. doi: 10.1113/jphysiol.2002.035196
De Stefani, D., Patron, M., and Rizzuto, R. (2015). Structure and function of the mitochondrial calcium uniporter complex. Biochim. Biophys. Acta 1853, 2006–2011. doi: 10.1016/j.bbamcr.2015.04.008
Deluca, H. F., and Engstrom, G. W. (1961). Calcium uptake by rat kidney mitochondria. Proc. Natl. Acad. Sci. U S A 47, 1744–1750. doi: 10.1073/pnas.47.11.1744
Emptage, N., Bliss, T. V., and Fine, A. (1999). Single synaptic events evoke NMDA receptor-mediated release of calcium from internal stores in hippocampal dendritic spines. Neuron 22, 115–124. doi: 10.1016/s0896-6273(00)80683-2
Emptage, N. J., Reid, C. A., and Fine, A. (2001). Calcium stores in hippocampal synaptic boutons mediate short-term plasticity, store-operated Ca2+ entry and spontaneous transmitter release. Neuron 29, 197–208. doi: 10.1016/s0896-6273(01)00190-8
Fieni, F., Lee, S. B., Jan, Y. N., and Kirichok, Y. (2012). Activity of the mitochondrial calcium uniporter varies greatly between tissues. Nat. Commun. 3:1317. doi: 10.1038/ncomms2325
Finch, E. A., and Augustine, G. J. (1998). Local calcium signalling by inositol-1,4,5-trisphosphate in Purkinje cell dendrites. Nature 396, 753–756. doi: 10.1038/25541
Fujimoto, M., and Hayashi, T. (2011). New insights into the role of mitochondria-associated endoplasmic reticulum membrane. Int. Rev. Cell Mol. Biol. 292, 73–117. doi: 10.1016/B978-0-12-386033-0.00002-5
Furuichi, T., Furutama, D., Hakamata, Y., Nakai, J., Takeshima, H., and Mikoshiba, K. (1994). Multiple types of ryanodine receptor/Ca2+ release channels are differentially expressed in rabbit brain. J. Neurosci. 14, 4794–4805.
Futatsugi, A., Kato, K., Ogura, H., Li, S. T., Nagata, E., Kuwajima, G., et al. (1999). Facilitation of NMDAR-independent LTP and spatial learning in mutant mice lacking ryanodine receptor type 3. Neuron 24, 701–713. doi: 10.1016/s0896-6273(00)81123-x
Galante, M., and Marty, A. (2003). Presynaptic ryanodine-sensitive calcium stores contribute to evoked neurotransmitter release at the basket cell-Purkinje cell synapse. J. Neurosci. 23, 11229–11234.
Garcia-Alvarez, G., Lu, B., Yap, K. A., Wong, L. C., Thevathasan, J. V., Lim, L., et al. (2015). STIM2 regulates PKA-dependent phosphorylation and trafficking of AMPARs. Mol. Biol. Cell 26, 1141–1159. doi: 10.1091/mbc.e14-07-1222
García-Chacón, L. E., Nguyen, K. T., David, G., and Barrett, E. F. (2006). Extrusion of Ca2+ from mouse motor terminal mitochondria via a Na+-Ca2+ exchanger increases post-tetanic evoked release. J. Physiol. 574, 663–675. doi: 10.1113/jphysiol.2006.110841
Gazit, N., Vertkin, I., Shapira, I., Helm, M., Slomowitz, E., Sheiba, M., et al. (2016). IGF-1 receptor differentially regulates spontaneous and evoked transmission via mitochondria at hippocampal synapses. Neuron 89, 583–597. doi: 10.1016/j.neuron.2015.12.034
Giannini, G., Conti, A., Mammarella, S., Scrobogna, M., and Sorrentino, V. (1995). The ryanodine receptor/calcium channel genes are widely and differentially expressed in murine brain and peripheral tissues. J. Cell Biol. 128, 893–904. doi: 10.1083/jcb.128.5.893
Gray, E. G. (1963). Electron microscopy of presynaptic organelles of the spinal cord. J. Anat. 97, 101–106.
Guo, X., Macleod, G. T., Wellington, A., Hu, F., Panchumarthi, S., Schoenfield, M., et al. (2005). The GTPase dMiro is required for axonal transport of mitochondria to Drosophila synapses. Neuron 47, 379–393. doi: 10.1016/j.neuron.2005.06.027
Hartmann, J., Karl, R. M., Alexander, R. P., Adelsberger, H., Brill, M. S., Rühlmann, C., et al. (2014). STIM1 controls neuronal Ca2+ signaling, mGluR1-dependent synaptic transmission and cerebellar motor behavior. Neuron 82, 635–644. doi: 10.1016/j.neuron.2014.03.027
Henderson, M. J., Baldwin, H. A., Werley, C. A., Boccardo, S., Whitaker, L. R., Yan, X., et al. (2015). A low affinity GCaMP3 variant (GCaMPer) for imaging the endoplasmic reticulum calcium store. PLoS One 10:e0139273. doi: 10.1371/journal.pone.0139273
Itoh, S., Ito, K., Fujii, S., Kaneko, K., Kato, K., Mikoshiba, K., et al. (2001). Neuronal plasticity in hippocampal mossy fiber-CA3 synapses of mice lacking the inositol-1,4,5-trisphosphate type 1 receptor. Brain Res. 901, 237–246. doi: 10.1016/s0006-8993(01)02373-3
Jahn, R., and Fasshauer, D. (2012). Molecular machines governing exocytosis of synaptic vesicles. Nature 490, 201–207. doi: 10.1038/nature11320
Kang, J. S., Tian, J. H., Pan, P. Y., Zald, P., Li, C., Deng, C., et al. (2008). Docking of axonal mitochondria by syntaphilin controls their mobility and affects short-term facilitation. Cell 132, 137–148. doi: 10.1016/j.cell.2007.11.024
Kann, O., and Kovács, R. (2007). Mitochondria and neuronal activity. Am. J. Physiol. Cell Physiol. 292, C641–C657. doi: 10.1152/ajpcell.00222.2006
Kapur, A., Yeckel, M., and Johnston, D. (2001). Hippocampal mossy fiber activity evokes Ca2+ release in CA3 pyramidal neurons via a metabotropic glutamate receptor pathway. Neuroscience 107, 59–69. doi: 10.1016/s0306-4522(01)00293-7
Kasthuri, N., Hayworth, K. J., Berger, D. R., Schalek, R. L., Conchello, J. A., Knowles-Barley, S., et al. (2015). Saturated reconstruction of a volume of neocortex. Cell 162, 648–661. doi: 10.1016/j.cell.2015.06.054
Knopfel, T. (2012). Genetically encoded optical indicators for the analysis of neuronal circuits. Nat. Rev. Neurosci. 13, 687–700. doi: 10.1523/JNEUROSCI.0381-14.2014
Korkotian, E., Frotscher, M., and Segal, M. (2014). Synaptopodin regulates spine plasticity: mediation by calcium stores. J. Neurosci. 34, 11641–11651. doi: 10.1523/JNEUROSCI.0381-14.2014
Kornmann, B. (2013). The molecular hug between the ER and the mitochondria. Curr. Opin. Cell Biol. 25, 443–448. doi: 10.1016/j.ceb.2013.02.010
Kovalchuk, Y., Eilers, J., Lisman, J., and Konnerth, A. (2000). NMDA receptor-mediated subthreshold Ca2+ signals in spines of hippocampal neurons. J. Neurosci. 20, 1791–1799.
Kwon, S., Sando, R. III, Lewis, T. L., Hirabayashi, Y., Maximov, A., and Polleux, F. (2016). LKB1 regulates mitochondria-dependent presynaptic calcium clearance and neurotransmitter release properties at excitatory synapses along cortical axons. PLoS Biol. 14:e1002516. doi: 10.1371/journal.pbio.1002516
Lauri, S. E., Bortolotto, Z. A., Nistico, R., Bleakman, D., Ornstein, P. L., Lodge, D., et al. (2003). A role for Ca2+ stores in kainate receptor-dependent synaptic facilitation and LTP at mossy fiber synapses in the hippocampus. Neuron 39, 327–341. doi: 10.1016/s0896-6273(03)00369-6
Lee, D., Lee, K. H., Ho, W. K., and Lee, S. H. (2007). Target cell-specific involvement of presynaptic mitochondria in post-tetanic potentiation at hippocampal mossy fiber synapses. J. Neurosci. 27, 13603–13613. doi: 10.1523/JNEUROSCI.3985-07.2007
Lei, S., Pelkey, K. A., Topolnik, L., Congar, P., Lacaille, J. C., and McBain, C. J. (2003). Depolarization-induced long-term depression at hippocampal mossy fiber-CA3 pyramidal neuron synapses. J. Neurosci. 23, 9786–9795.
Li, Z., Okamoto, K., Hayashi, Y., and Sheng, M. (2004). The importance of dendritic mitochondria in the morphogenesis and plasticity of spines and synapses. Cell 119, 873–887. doi: 10.1016/j.cell.2004.11.003
Liang, Y., Yuan, L. L., Johnston, D., and Gray, R. (2002). Calcium signaling at single mossy fiber presynaptic terminals in the rat hippocampus. J. Neurophysiol. 87, 1132–1137.
Llano, I., Gonzalez, J., Caputo, C., Lai, F. A., Blayney, L. M., Tan, Y. P., et al. (2000). Presynaptic calcium stores underlie large-amplitude miniature IPSCs and spontaneous calcium transients. Nat. Neurosci. 3, 1256–1265. doi: 10.1038/81781
Lüscher, C., and Malenka, R. C. (2012). NMDA receptor-dependent long-term potentiation and long-term depression (LTP/LTD). Cold Spring Harb Perspect. Biol. 4:a005710. doi: 10.1101/cshperspect.a005710
Ly, C. V., and Verstreken, P. (2006). Mitochondria at the synapse. Neuroscientist 12, 291–299. doi: 10.1177/1073858406287661
Malenka, R. C., and Nicoll, R. A. (1999). Long-term potentiation–a decade of progress? Science 285, 1870–1874. doi: 10.1126/science.285.5435.1870
Mallilankaraman, K., Doonan, P., Cárdenas, C., Chandramoorthy, H. C., Müller, M., Miller, R., et al. (2012). MICU1 is an essential gatekeeper for MCU-mediated mitochondrial Ca2+ uptake that regulates cell survival. Cell 151, 630–644. doi: 10.1016/j.cell.2012.10.011
Marland, J. R., Hasel, P., Bonnycastle, K., and Cousin, M. A. (2016). Mitochondrial calcium uptake modulates synaptic vesicle endocytosis in central nerve terminals. J. Biol. Chem. 291, 2080–2086. doi: 10.1074/jbc.M115.686956
Mathew, S. S., and Hablitz, J. J. (2008). Calcium release via activation of presynaptic IP3 receptors contributes to kainate-induced IPSC facilitation in rat neocortex. Neuropharmacology 55, 106–116. doi: 10.1016/j.neuropharm.2008.05.005
Mattson, M. P., Gleichmann, M., and Cheng, A. (2008). Mitochondria in neuroplasticity and neurological disorders. Neuron 60, 748–766. doi: 10.1016/j.neuron.2008.10.010
Mellor, J., and Nicoll, R. A. (2001). Hippocampal mossy fiber LTP is independent of postsynaptic calcium. Nat. Neurosci. 4, 125–126. doi: 10.1038/83941
Minta, A., Kao, J. P., and Tsien, R. Y. (1989). Fluorescent indicators for cytosolic calcium based on rhodamine and fluorescein chromophores. J. Biol. Chem. 264, 8171–8178.
Mironov, S. L., and Symonchuk, N. (2006). ER vesicles and mitochondria move and communicate at synapses. J. Cell Sci. 119, 4926–4934. doi: 10.1242/jcs.03254
Miyata, M., Finch, E. A., Khiroug, L., Hashimoto, K., Hayasaka, S., Oda, S. I., et al. (2000). Local calcium release in dendritic spines required for long-term synaptic depression. Neuron 28, 233–244. doi: 10.1016/s0896-6273(00)00099-4
Nagai, T., Sawano, A., Park, E. S., and Miyawaki, A. (2001). Circularly permuted green fluorescent proteins engineered to sense Ca2+. Proc. Natl. Acad. Sci. U S A 98, 3197–3202. doi: 10.1073/pnas.051636098
Nagase, T., Ito, K. I., Kato, K., Kaneko, K., Kohda, K., Matsumoto, M., et al. (2003). Long-term potentiation and long-term depression in hippocampal CA1 neurons of mice lacking the IP(3) type 1 receptor. Neuroscience 117, 821–830. doi: 10.1016/s0306-4522(02)00803-5
Neher, E., and Sakaba, T. (2008). Multiple roles of calcium ions in the regulation of neurotransmitter release. Neuron 59, 861–872. doi: 10.1016/j.neuron.2008.08.019
Nishiyama, M., Hong, K., Mikoshiba, K., Poo, M. M., and Kato, K. (2000). Calcium stores regulate the polarity and input specificity of synaptic modification. Nature 408, 584–588. doi: 10.1038/35046067
Okubo, Y., Suzuki, J., Kanemaru, K., Nakamura, N., Shibata, T., and Iino, M. (2015). Visualization of Ca2+ filling mechanisms upon synaptic inputs in the endoplasmic reticulum of cerebellar purkinje cells. J. Neurosci. 35, 15837–15846. doi: 10.1523/JNEUROSCI.3487-15.2015
Oliet, S. H., Malenka, R. C., and Nicoll, R. A. (1997). Two distinct forms of long-term depression coexist in CA1 hippocampal pyramidal cells. Neuron 18, 969–982. doi: 10.1016/s0896-6273(00)80336-0
Palay, S. L. (1956). Synapses in the central nervous system. J. Biophys. Biochem. Cytol. 2, 193–202. doi: 10.1083/jcb.2.4.193
Palmer, A. E., Jin, C., Reed, J. C., and Tsien, R. Y. (2004). Bcl-2-mediated alterations in endoplasmic reticulum Ca2+ analyzed with an improved genetically encoded fluorescent sensor. Proc. Natl. Acad. Sci. U S A 101, 17404–17409. doi: 10.1073/pnas.0408030101
Palmer, A. E., and Tsien, R. Y. (2006). Measuring calcium signaling using genetically targetable fluorescent indicators. Nat. Protoc. 1, 1057–1065. doi: 10.1038/nprot.2006.172
Patron, M., Checchetto, V., Raffaello, A., Teardo, E., Vecellio Reane, D., Mantoan, M., et al. (2014). MICU1 and MICU2 finely tune the mitochondrial Ca2+ uniporter by exerting opposite effects on MCU activity. Mol. Cell 53, 726–737. doi: 10.1016/j.molcel.2014.01.013
Perocchi, F., Gohil, V. M., Girgis, H. S., Bao, X. R., McCombs, J. E., Palmer, A. E., et al. (2010). MICU1 encodes a mitochondrial EF hand protein required for Ca2+ uptake. Nature 467, 291–296. doi: 10.1038/nature09358
Plovanich, M., Bogorad, R. L., Sancak, Y., Kamer, K. J., Strittmatter, L., Li, A. A., et al. (2013). MICU2, a paralog of MICU1, resides within the mitochondrial uniporter complex to regulate calcium handling. PLoS One 8:e55785. doi: 10.1371/journal.pone.0055785
Poburko, D., Santo-Domingo, J., and Demaurex, N. (2011). Dynamic regulation of the mitochondrial proton gradient during cytosolic calcium elevations. J. Biol. Chem. 286, 11672–11684. doi: 10.1074/jbc.M110.159962
Raffaello, A., De Stefani, D., Sabbadin, D., Teardo, E., Merli, G., Picard, A., et al. (2013). The mitochondrial calcium uniporter is a multimer that can include a dominant-negative pore-forming subunit. EMBO J. 32, 2362–2376. doi: 10.1038/emboj.2013.157
Raymond, C. R., and Redman, S. J. (2002). Different calcium sources are narrowly tuned to the induction of different forms of LTP. J. Neurophysiol. 88, 249–255.
Reyes, M., and Stanton, P. K. (1996). Induction of hippocampal long-term depression requires release of Ca2+ from separate presynaptic and postsynaptic intracellular stores. J. Neurosci. 16, 5951–5960.
Rizzuto, R., Brini, M., Murgia, M., and Pozzan, T. (1993). Microdomains with high Ca2+ close to IP3-sensitive channels that are sensed by neighboring mitochondria. Science 262, 744–747. doi: 10.1126/science.8235595
Rizzuto, R., De Stefani, D., Raffaello, A., and Mammucari, C. (2012). Mitochondria as sensors and regulators of calcium signalling. Nat. Rev. Mol. Cell Biol. 13, 566–578. doi: 10.1038/nrm3412
Rizzuto, R., and Pozzan, T. (2006). Microdomains of intracellular Ca2+: molecular determinants and functional consequences. Physiol. Rev. 86, 369–408. doi: 10.1152/physrev.00004.2005
Rizzuto, R., Simpson, A. W., Brini, M., and Pozzan, T. (1992). Rapid changes of mitochondrial Ca2+ revealed by specifically targeted recombinant aequorin. Nature 358, 325–327. doi: 10.1038/358325a0
Robert, V., Gurlini, P., Tosello, V., Nagai, T., Miyawaki, A., Di Lisa, F., et al. (2001). Beat-to-beat oscillations of mitochondrial [Ca2+] in cardiac cells. EMBO J. 20, 4998–5007. doi: 10.1093/emboj/20.17.4998
Rodriguez-Garcia, A., Rojo-Ruiz, J., Navas-Navarro, P., Aulestia, F. J., Gallego-Sandin, S., Garcia-Sancho, J., et al. (2014). GAP, an aequorin-based fluorescent indicator for imaging Ca2+ in organelles. Proc. Natl. Acad. Sci. U S A 111, 2584–2589. doi: 10.1073/pnas.1316539111
Rose, T., Goltstein, P. M., Portugues, R., and Griesbeck, O. (2014). Putting a finishing touch on GECIs. Front. Mol. Neurosci. 7:88. doi: 10.3389/fnmol.2014.00088
Rowland, K. C., Irby, N. K., and Spirou, G. A. (2000). Specialized synapse-associated structures within the calyx of Held. J. Neurosci. 20, 9135–9144.
Sancak, Y., Markhard, A. L., Kitami, T., Kovács-Bogdán, E., Kamer, K. J., Udeshi, N. D., et al. (2013). EMRE is an essential component of the mitochondrial calcium uniporter complex. Science 342, 1379–1382. doi: 10.1126/science.1242993
Sandler, V. M., and Barbara, J. G. (1999). Calcium-induced calcium release contributes to action potential-evoked calcium transients in hippocampal CA1 pyramidal neurons. J. Neurosci. 19, 4325–4336.
Schneggenburger, R., and Neher, E. (2005). Presynaptic calcium and control of vesicle fusion. Curr. Opin. Neurobiol. 15, 266–274. doi: 10.1016/j.conb.2005.05.006
Schon, E. A., and Przedborski, S. (2011). Mitochondria: the next (neurode)generation. Neuron 70, 1033–1053. doi: 10.1016/j.neuron.2011.06.003
Scullin, C. S., and Partridge, L. D. (2010). Contributions of SERCA pump and ryanodine-sensitive stores to presynaptic residual Ca2+. Cell Calcium 47, 326–338. doi: 10.1016/j.ceca.2010.01.004
Scullin, C. S., Wilson, M. C., and Partridge, L. D. (2010). Developmental changes in presynaptic Ca2+ clearance kinetics and synaptic plasticity in mouse Schaffer collateral terminals. Eur. J. Neurosci. 31, 817–826. doi: 10.1111/j.1460-9568.2010.07137.x
Segal, M., and Korkotian, E. (2015). Roles of calcium stores and store-operated channels in plasticity of dendritic spines. Neuroscientist doi: 10.1177/1073858415613277 [Epub ahead of print].
Sharma, G., and Vijayaraghavan, S. (2003). Modulation of presynaptic store calcium induces release of glutamate and postsynaptic firing. Neuron 38, 929–939. doi: 10.1016/s0896-6273(03)00322-2
Sharp, A. H., McPherson, P. S., Dawson, T. M., Aoki, C., Campbell, K. P., and Snyder, S. H. (1993). Differential immunohistochemical localization of inositol 1,4,5-trisphosphate- and ryanodine-sensitive Ca2+ release channels in rat brain. J. Neurosci. 13, 3051–3063.
Sharp, A. H., Nucifora, F. C. Jr., Blondel, O., Sheppard, C. A., Zhang, C., Snyder, S. H., et al. (1999). Differential cellular expression of isoforms of inositol 1,4,5-triphosphate receptors in neurons and glia in brain. J. Comp. Neurol. 406, 207–220. doi: 10.1002/(SICI)1096-9861(19990405)406:2<207::AID-CNE6>3.0.CO;2-7
Sheng, M., and Hoogenraad, C. C. (2007). The postsynaptic architecture of excitatory synapses: a more quantitative view. Annu. Rev. Biochem. 76, 823–847. doi: 10.1146/annurev.biochem.76.060805.160029
Shepherd, G. M., and Harris, K. M. (1998). Three-dimensional structure and composition of CA3→CA1 axons in rat hippocampal slices: implications for presynaptic connectivity and compartmentalization. J. Neurosci. 18, 8300–8310.
Shoshan-Barmatz, V., Zalk, R., Gincel, D., and Vardi, N. (2004). Subcellular localization of VDAC in mitochondria and ER in the cerebellum. Biochim. Biophys. Acta 1657, 105–114. doi: 10.1016/j.bbabio.2004.02.009
Slater, E. C., and Cleland, K. W. (1953). The effect of calcium on the respiratory and phosphorylative activities of heart-muscle sarcosomes. Biochem. J. 55, 566–590. doi: 10.1042/bj0550566
Solovyova, N., Veselovsky, N., Toescu, E. C., and Verkhratsky, A. (2002). Ca2+ dynamics in the lumen of the endoplasmic reticulum in sensory neurons: direct visualization of Ca2+-induced Ca2+ release triggered by physiological Ca2+ entry. EMBO J. 21, 622–630. doi: 10.1093/emboj/21.4.622
Spacek, J., and Harris, K. M. (1997). Three-dimensional organization of smooth endoplasmic reticulum in hippocampal CA1 dendrites and dendritic spines of the immature and mature rat. J. Neurosci. 17, 190–203. doi: 10.1002/hipo.20238
Südhof, T. C. (2012). The presynaptic active zone. Neuron 75, 11–25. doi: 10.1016/j.neuron.2012.06.012
Suzuki, J., Kanemaru, K., Ishii, K., Ohkura, M., Okubo, Y., and Iino, M. (2014). Imaging intraorganellar Ca2+ at subcellular resolution using CEPIA. Nat. Commun. 5:4153. doi: 10.1038/ncomms5153
Takechi, H., Eilers, J., and Konnerth, A. (1998). A new class of synaptic response involving calcium release in dendritic spines. Nature 396, 757–760. doi: 10.1038/25547
Takei, K., Stukenbrok, H., Metcalf, A., Mignery, G. A., Südhof, T. C., Volpe, P., et al. (1992). Ca2+ stores in Purkinje neurons: endoplasmic reticulum subcompartments demonstrated by the heterogeneous distribution of the InsP3 receptor, Ca2+-ATPase and calsequestrin. J. Neurosci. 12, 489–505.
Tang, Y., and Zucker, R. S. (1997). Mitochondrial involvement in post-tetanic potentiation of synaptic transmission. Neuron 18, 483–491. doi: 10.1016/s0896-6273(00)81248-9
Thayer, S. A., and Miller, R. J. (1990). Regulation of the intracellular free calcium concentration in single rat dorsal root ganglion neurones in vitro. J. Physiol. 425, 85–115. doi: 10.1113/jphysiol.1990.sp018094
Tian, L., Hires, S. A., and Looger, L. L. (2012). Imaging neuronal activity with genetically encoded calcium indicators. Cold Spring Harb. Protoc. 2012, 647–656. doi: 10.1101/pdb.top069609
Unni, V. K., Zakharenko, S. S., Zablow, L., DeCostanzo, A. J., and Siegelbaum, S. A. (2004). Calcium release from presynaptic ryanodine-sensitive stores is required for long-term depression at hippocampal CA3-CA3 pyramidal neuron synapses. J. Neurosci. 24, 9612–9622. doi: 10.1523/JNEUROSCI.5583-03.2004
Verkhratsky, A. (2005). Physiology and pathophysiology of the calcium store in the endoplasmic reticulum of neurons. Physiol. Rev. 85, 201–279. doi: 10.1152/physrev.00004.2004
Verstreken, P., Ly, C. V., Venken, K. J., Koh, T. W., Zhou, Y., and Bellen, H. J. (2005). Synaptic mitochondria are critical for mobilization of reserve pool vesicles at Drosophila neuromuscular junctions. Neuron 47, 365–378. doi: 10.1016/j.neuron.2005.06.018
Voelker, D. R. (1990). Characterization of phosphatidylserine synthesis and translocation in permeabilized animal cells. J. Biol. Chem. 265, 14340–14346.
Vos, M., Lauwers, E., and Verstreken, P. (2010). Synaptic mitochondria in synaptic transmission and organization of vesicle pools in health and disease. Front. Synaptic Neurosci. 2:139. doi: 10.3389/fnsyn.2010.00139
Wang, S. S., Denk, W., and Häusser, M. (2000). Coincidence detection in single dendritic spines mediated by calcium release. Nat. Neurosci. 3, 1266–1273. doi: 10.1038/81792
Wang, X., and Schwarz, T. L. (2009). The mechanism of Ca2+ -dependent regulation of kinesin-mediated mitochondrial motility. Cell 136, 163–174. doi: 10.1016/j.cell.2008.11.046
Wang, G. J., and Thayer, S. A. (2002). NMDA-induced calcium loads recycle across the mitochondrial inner membrane of hippocampal neurons in culture. J. Neurophysiol. 87, 740–749.
White, R. J., and Reynolds, I. J. (1995). Mitochondria and Na+/Ca2+ exchange buffer glutamate-induced calcium loads in cultured cortical neurons. J. Neurosci. 15, 1318–1328.
Wu, J., Prole, D. L., Shen, Y., Lin, Z., Gnanasekaran, A., Liu, Y., et al. (2014). Red fluorescent genetically encoded Ca2+ indicators for use in mitochondria and endoplasmic reticulum. Biochem. J. 464, 13–22. doi: 10.1042/bj20140931
Yang, S. N., Tang, Y. G., and Zucker, R. S. (1999). Selective induction of LTP and LTD by postsynaptic [Ca2+]i elevation. J. Neurophysiol. 81, 781–787.
Yeckel, M. F., Kapur, A., and Johnston, D. (1999). Multiple forms of LTP in hippocampal CA3 neurons use a common postsynaptic mechanism. Nat. Neurosci. 2, 625–633. doi: 10.1038/10180
Young, K. W., Bampton, E. T., Pinòn, L., Bano, D., and Nicotera, P. (2008). Mitochondrial Ca2+ signalling in hippocampal neurons. Cell Calcium 43, 296–306. doi: 10.1016/j.ceca.2007.06.007
Zhong, N., Beaumont, V., and Zucker, R. S. (2001). Roles for mitochondrial and reverse mode Na+/Ca2+ exchange and the plasmalemma Ca2+ ATPase in post-tetanic potentiation at crayfish neuromuscular junctions. J. Neurosci. 21, 9598–9607.
Zhang, H., Sun, S., Herreman, A., De Strooper, B., and Bezprozvanny, I. (2010). Role of presenilins in neuronal calcium homeostasis. J. Neurosci. 30, 8566–8580. doi: 10.1523/JNEUROSCI.1554-10.2010
Keywords: synapse, mitochondria, endoplasmic reticulum, circuit function, calcium dynamics
Citation: Kwon S-K, Hirabayashi Y and Polleux F (2016) Organelle-Specific Sensors for Monitoring Ca2+ Dynamics in Neurons. Front. Synaptic Neurosci. 8:29. doi: 10.3389/fnsyn.2016.00029
Received: 13 June 2016; Accepted: 30 August 2016;
Published: 16 September 2016.
Edited by:
Marc Fivaz, Duke NUS Graduate Medical School, SingaporeReviewed by:
Lucas Pozzo-Miller, University of Alabama at Birmingham, USAWilliam N. Green, University of Chicago, USA
Copyright © 2016 Kwon, Hirabayashi and Polleux. This is an open-access article distributed under the terms of the Creative Commons Attribution License (CC BY). The use, distribution and reproduction in other forums is permitted, provided the original author(s) or licensor are credited and that the original publication in this journal is cited, in accordance with accepted academic practice. No use, distribution or reproduction is permitted which does not comply with these terms.
*Correspondence: Franck Polleux, ZnAyMzA0QGN1bWMuY29sdW1iaWEuZWR1