- 1Centre International de Recherche en Infectiologie, Institut National de la Santé et de la Recherche Médicale, U1111, Université Claude Bernard Lyon 1, Centre National de la Recherche Scientifique, UMR5308, Ecole Normale Supérieure de Lyon, Université de Lyon, Lyon, France
- 2Center for Medical Research, Medical University of Graz, Graz, Austria
- 3Research Unit for Photodermatology, Department of Dermatology, Medical University of Graz, Graz, Austria
- 4Allergy and Clinical Immunology Department, Lyon Sud University Hospital, Pierre-Bénite, France
The human skin is known to be inhabited by diverse microbes, including bacteria, fungi, viruses, archaea, and mites. This microbiome exerts a protective role against infections by promoting immune development and inhibiting pathogenic microbes to colonize skin. One of the factors having an intense effect on the skin and its resident microbes is ultraviolet-radiation (UV-R). UV-R can promote or inhibit the growth of microbes on the skin and modulate the immune system which can be either favorable or harmful. Among potential UV-R targets, skin resident memory T cells (TRM) stand as well positioned immune cells at the forefront within the skin. Both CD4+ or CD8+ αβ TRM cells residing permanently in peripheral tissues have been shown to play prominent roles in providing accelerated and long-lived specific immunity, tissue homeostasis, wound repair. Nevertheless, their response upon UV-R exposure or signals from microbiome are poorly understood compared to resident TCRγδ cells. Skin TRM survive for long periods of time and are exposed to innumerable antigens during lifetime. The interplay of TRM with skin residing microbes may be crucial in pathophysiology of various diseases including psoriasis, atopic dermatitis and polymorphic light eruption. In this article, we share our perspective about how UV-R may directly shape the persistence, phenotype, specificity, and function of skin TRM; and moreover, whether UV-R alters barrier function, leading to microbial-specific skin TRM, disrupting the healthy balance between skin microbiome and skin immune cells, and resulting in chronic inflammation and diseased skin.
Introduction
Skin Microbiome
Human skin with its large surface (1) harbors a wide variety of microbes, which include bacteria, fungi (2), viruses (3, 4), archaea (5, 6) and skin mites (4, 7, 8). These microbes exist in either a mutualistic and/or competitive relationship with each other (microbe-microbe) (9) and the host (10–13). Commensals make up for most of the microbiome followed by opportunistic and/or pathogenic microbes. The diverse physical nature of the skin with its variable water content, pH, lipids and sebum quantity among others crucially influence the diversity of the microbiome. However, it is intriguing that myriads of microbes reside on the skin surface (Figure 1) as well as in sub-epidermal compartments (14), despite the robust nature of the skin's immune system to rapidly detect and neutralize any foreign intruders (15). Many common cutaneous conditions such as atopic dermatitis (AD), psoriasis and rosacea are associated with dysbiosis of skin microbiome, most commonly driven by commensal species. A recent review highlights the latest findings regarding the microbial interactions with the immune system and microbial composition in health and diseases such as AD, acne, chronic wound infections, and primary immunodeficiencies (16).
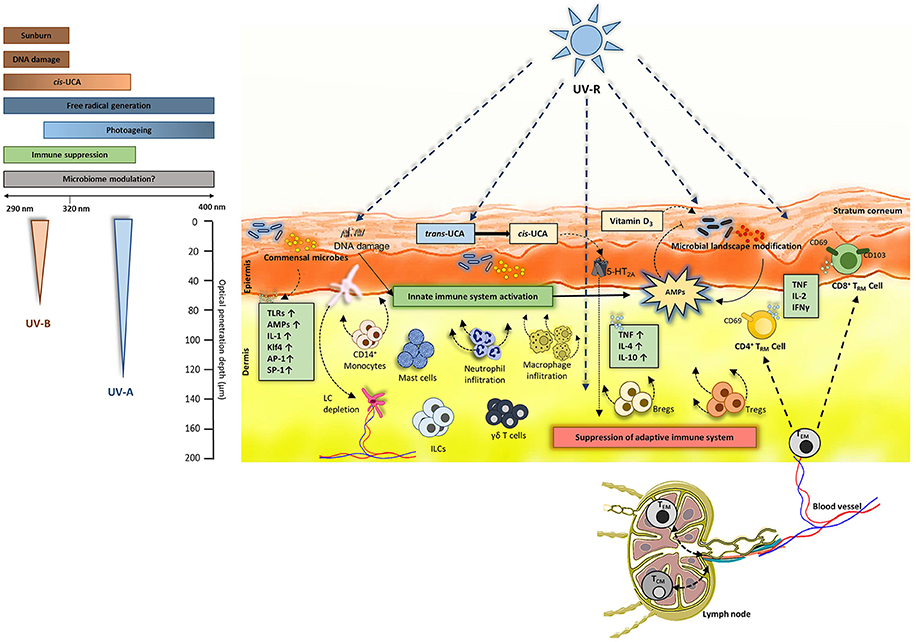
Figure 1. UV-induced events in the skin: Both UV-B (290–320 nm) and UV-A (320–400 nm) penetrate the skin. UV-B causes sunburn and DNA damage and is also known to induce immune suppression. UV-B and UV-A (to some extent) converts trans-UCA to cis-UCA and generates free radicals. Commensal microbiome colonizes the skin and can induce production of various cytokines, antimicrobial peptides (AMPs) and activate toll-like receptors (TLRs). The effects of UV-B and UV-A on skin microbiome is not fully understood. Overall, UV-R is known to activate innate immunity by production of AMPs and by stimulating innate cells like macrophages, mast cells, innate lymphoid cells (ILCs) and skin resident γδ T cells. On the other hand, UV-R induces an immune suppressive environment in the skin by inducing production of TNF, IL-4, IL-10. As overall result, regulatory T cells (Tregs) and B cells (Bregs) are induced leading to functional immune suppression and subsequent inhibition of effector T cells present in the skin. Regarding TCRαβ+ lymphocytes, effector memory T cells (TEM) can circulate between the blood, lymph and skin where they receive environmental signals. Also, the dermis is populated by CD4+ TRM (CD69+ CD103±) whereas the epidermis is composed of CD8+ TRM (CD69+CD103±) in majority. These TRM populations can produce TNF-α, IL-2 and IFN-γ depending on the microenvironment. Nevertheless, the UV effect on these TRM remains to uncovered.
Ultraviolet-Radiation (UV-R)
UV-R is one of the most prominent external factor affecting the skin (17) and the microbiome (8, 18, 19). UV-R mediated immune suppression was first discovered by Kripke et al. (20). This was further confirmed and proved to be T-cell mediated by using contact hypersensitivity (CHS) models in mice (21) and in humans (22–24). The initial key events that are prominently involved in immune suppression after UV-irradiation are DNA damage (25), formation of reactive biophospholipids like platelet activating factor (26) and isomerization of inactive trans- to active cis-urocanic acid (UCA) (27). A study conducted by Kubica et al. (28) used caspase-14 deficient mice which are known to have reduced levels of UCA and observed significant alterations in the skin microbiome. It is intriguing that caspase-14 is involved in proteolysis of filaggrin which is the major source of UCA in the skin and mutations in filaggrin are linked to the development of AD which is in turn linked to an altered microbial landscape (29). Certain skin commensals such as Micrococcus luteus can degrade cis-UCA to its trans isoform (30) and thus potentially diminish immune suppression. An early report from our group suggests that cis-UCA can indeed directly modulate skin microbiome (31). Since UV-R suppresses the immune reaction to antigens of infectious microbes such as M. lepraemurium, bovis BCG, C. albicans, B. burgdorferi, and Schistosoma mansoni (32–34) it can be speculated that exposure to UV-R could enhance susceptibility to infections, however clinical evidence of increased infections after UV-R is very low. This could be due to the fact that UV-R suppresses adaptive immunity but activates innate immunity (35). One of the important innate key players are antimicrobial peptides (AMPs). These are small proteins typically ranging from 10 to 50 amino acid residues that have potential to neutralize invading microorganisms (36) and mediate adaptive immune response (37–39). Dysregulation in AMP expression could be linked to many diseases, including photosensitive conditions like polymorphic light eruption (PLE) (40), where AMPs may be key mediators to maintain homeostasis between host immune system and microbiome. UV-R exposure also leads to infiltration of macrophages and neutrophils (41–43), induces emigration of Langerhans cells (LC) from the skin into the draining lymph nodes (44–46) and affects mast cells. Furthermore, regulatory T cells (Tregs) and B cells (Bregs) are recruited and activated (47, 48). All these cells and UV-induced events are known to be involved in immune suppression (49) (Figure 1). It has been known for a long time that UV-induced immune suppression is mediated by T cells (21, 50), however, the exact role of UV effects on the more recently described TRM and immune function are largely unexplored.
Skin-Resident Memory T Cells (TRM)
Among all the immune cells present in the skin, such as dendritic cells, macrophages, γδ T cells and NK cells, TRM (51) are now considered as key players of immunity (52–54) (Figure 1). They have been described in various tissues such as skin, lung, gut, liver and brain (55–57). TRM, along with effector and central memory T cells (58), are either CD4+ or CD8+ T cells that are derived from naïve specific T cells which were activated upon a previous immune response. Thus, TRM share a common clonal origin with central memory T cells (59) but diverge in terms of dynamics, phenotype, and function. The major characteristics of TRM are their capacity to survive and stay poised in the skin for a long time (60) as well as play a key role for pathogen clearance and immune alert (53). In other words, TRM do not recirculate in the lymph or blood but rather patrol in the skin. CD8+ TRM are more localized in epidermis whereas CD4+ TRM populate preferentially the dermis (61). This non-recirculating pattern is conferred by the expression of CD69 which blocks sphingosine-1-phosphate receptor (S1P1), a receptor normally allowing lymph entrance. Moreover, a significant part of skin TRM express CD103, the α-chain of the integrin αEβ7 which interacts with E-cadherin expressed by keratinocytes. Once arrived in the skin, killer-cell lectin like receptor G1 (KLRG1)-TRM precursors receive key signals for their establishment in the tissue. Among them, TGF-β is a critical signal integrated by TRM via TGF-βRII (52) and required for their residency. TGF-β can notably be produced by keratinocytes which thus play a role on TRM retention (62). TGF-β alone is not sufficient for skin TRM establishment, but rather acts in combination with other cytokines expressed in the skin such as TNF-α and interleukin (IL)-33 (63). Moreover, hair follicles seem to play a role on the recruitment and establishment of skin TRM notably through the production of IL-15 and IL-7 (Figure 2) (64). Apart from cytokines, lipids available in the skin are key for TRM maintenance (65). Functionally, TRM allow a faster immune response upon pathogen entry through the production of alarmins such as IFN-γ and chemokines to recruit neutrophils, monocytes as well as circulating memory T cells on the site. TRM are also able to proliferate locally after a recall response to maintain themselves (66). Finally, TRM are able to be strongly cytotoxic (67).
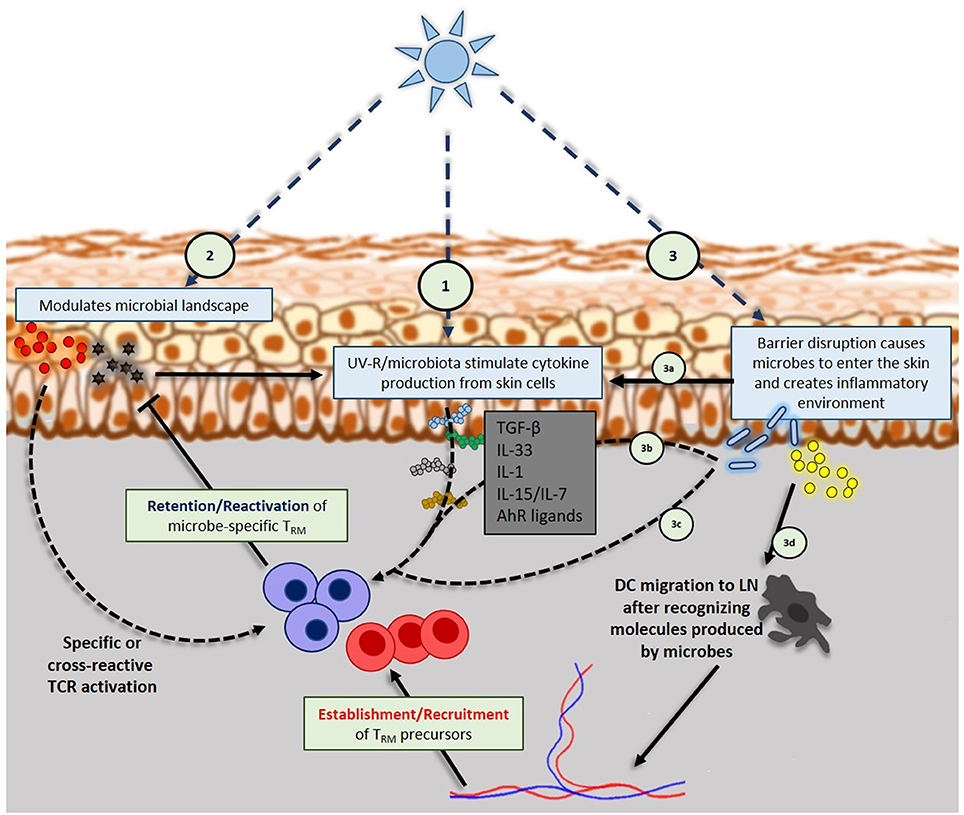
Figure 2. Interplay of UV-R, skin microbiome and skin resident memory TCRαβ+ cells: (1) UV-R induces keratinocytes and other skin cells to produce inflammatory or regulatory cytokines that will influence TRM phenotype, retention and reactivation. (2) UV-R modulates microbial landscape, eventually releasing microbial antigens into the skin that will be up taken by dendritic cells (DC) that will specifically activate TRM (regulatory or effector). Microbial antigens can also trigger the production of inflammatory cytokines by keratinocytes that further activate TRM. (3) High doses of UV-R can cause barrier disruption that will allow skin resident microbes to enter the skin; danger signals from barrier disruption (3a) and microbes entered into the skin (3b) will trigger cytokines production by keratinocytes, DCs, ILCs, NK and TCRγδ cells. Those cytokines will take part in shaping TRM phenotype and activation. Entered microbes can also activate skin TRM in a specific manner (3c) or be uptaken by DCs (3d) in order to activate naïve specific T cells in draining lymph nodes that will be recruited on the site.
UV-Induced Impact on Skin TRM
At least 1–2 × 1010 resident T cells comprising TRM populate the human skin (68, 69), and it is highly logical that they experience similar impacts from UV-R as the other immune cells. These sentinel cells have numerous essential functions within the skin for cutaneous immunity and repair along with wound healing, antimicrobial responses and local tissue inspection (68, 70–72). The impact of UV-R on immune response mediated by T cells such as CD4+, CD8+, and Tregs has been previously described (73–75), however, the effects of UV-R on shaping the persistence, phenotype and specificity of skin TRM are poorly understood. It is therefore important to understand the interaction between the skin TRM and UV-R in mediating UV-induced immune suppression. It is thought that after an acute UV-exposure, the damaged keratinocytes release ATP (76) and ATP-mediated IL-1 (77) in an accelerated way; furthermore, this extracellular ATP is thought to be involved in adaptive immune responses (78, 79). Moreover, UV-R upregulates CD69 expression on TCRγδ cells (77) and could exert a similar effect on skin TRM for which CD69 is crucial for their residency in the tissue. Besides, in the absence of γδ T cells, there was reduced DNA repair of UV-induced lesions in mice, suggesting the role of these γδ T cells in the repair (77). Such a role for TRM has been demonstrated in acute wounds (71) but needs to be addressed in the case of UV-induced damage. TRM may have long been unknown targets of UV-phototherapy in diseases which are now understood as TRM cell-mediated (80). Patients with cutaneous T cell lymphoma (mycosis fungoides) are known to have malignant T cells that lack L-selectin and CCR7 expression, a phenotype that is similar to TRM (81). The common treatment modality for these patients include phototherapy (82) and low-dose radiation. However, the effects of phototherapy on TRM is completely uncharacterized (83).
Influence of Skin Microbiome on Skin TRM
The skin is exposed to a large number of microbes throughout the lifetime, of which only a minor proportion is pathogenic. It has been suggested that the primary purpose of the immune cell memory is to maintain the immune homeostasis with the commensal microbes (84). Recent studies in various mouse models and in humans show that the composition of the skin microbiome is crucial in mediating appropriate immune responses toward a pathogen and in maintaining the normal immune status in the skin (10, 11, 15, 28, 85–87). Whether certain species of commensal microbiome influence the type of TRM within the skin is not known, but a lot can be learnt from the gut. In one of the studies using mice, commensal specific memory T cells were found in the intestines (88) and similar TRM cells could exist in the skin as well. Both memory CD4+ and CD8+ T cells can act against infections with influenza virus (55, 89), lymphocytic choriomeningitis virus (90, 91), herpes simplex virus (92), mycobacterium tuberculosis (93) and parasites (94). Furthermore, microbial and/or antigen-specific memory CD4+ and CD8+ TRM cells produce vast amount of effector cytokines in response to microbes and antigens (95–97) and CD4+ and CD8+ TRM cells can populate and persist in multiple tissue sites long after the microbe or the antigen has been neutralized (98, 99). In the skin, CD8+ TRM can be generated following an infection (92, 100, 101) and CD4+ IL-17-producing TRM cells were identified in the skin of the mice when they were infected by C. albicans (part of skin mycobiome) (102). Besides, another study showed that laboratory SPF (specific-pathogen free) mice had lower non-circulating T cells in the skin and other tissues compared to pet store mice (103). In terms of T-cell memory, SPF-raised mice have a similar adaptive immunity like newborn humans and pet store mice show the profile of memory T cells, similarly observed in adult humans (104). Several studies show a compartmentalization of microbe-specific memory T cells. When humans were injected intradermally with purified protein-derivative from M. tuberculosis, antigen-specific T cells were observed only in the skin but not in the blood (105). HSV2 specific CD8+ T cells were found in genital skin but not at other body sites (106). Variability within the skin microbiome (16) could be a reason for compartmentalization of TRM. Skin TRM persists for long periods of time and are exposed to the microbiome and microbial antigens from the skin during their lifetime. Microbial-specific responses could be a part of the healthy immune balance between the skin microbiome and host immune system and further provide reinforced local immunity. Very interestingly a recent study demonstrated that non-invasive S. epidermidis allows specific CD8+ TRM establishment through non-conventional MHC-Ib H2-M3 peptide presentation. Those H2-M3 restricted CD8+ TRM were shown to play an important role in tissue repair and wound healing (107).
Perspective
Skin microbiome and TRM reside in the upper layers of the skin. Both UV-A and UV-B radiation can penetrate those upper layers (only UV-A particularly reaches the dermis) and imminently impact all the microbes and immune cells (Figure 1).
Does UV-R Directly Shape the Persistence, Phenotype, Specificity and Function of Skin TRM?
UV-R is known to induce production of various cytokines in the skin such as TNF-α (108) or IL-33 (109–111) which are known to be involved in maintaining the phenotype of TRM (52, 64, 112). Besides, a study published in 2016 (62) linked UV-B exposure and TRM retention. Authors demonstrated that UV-B exposure decreased αvβ6 and αvβ8 integrins expression by keratinocytes. Those integrins were required for active TGF-β production which then maintained CD103 expression on TRM allowing their retention in the skin long time after a lymphocytic choriomeningitis viral infection. Hence, the ability of UV-R (notably UV-B) to dose-dependently influence the retention and phenotype of skin TRM by modulating the cutaneous cytokine environment (Figure 2), certainly may at least contribute to the efficacy of suberythemal phototherapy, which has been used for decades to improve pathologies such as psoriasis, atopic dermatitis and other inflammatory diseases (113–116). However, beyond cytokines, it is also possible that TRM persistence depends on TCR-specific signals. The discovery of commensal-specific TRM in the gastrointestinal tract of mice (88) implies that there may be a large number of commensal-specific TRM residing in the skin as well, in addition to γδ T cells, innate lymphoid cells and pathogen-specific TRM. Moreover, the skin microbiome is constantly changing within the individual throughout lifetime (117) and contributes to skin TRM diversity and function (107). Interestingly, UV-R is known to influence the skin microbiome landscape (8, 18, 19, 31). UV may in a dose dependent fashion affect skin microbiome and may shape the repertoire diversity of effector or regulatory TRM. Important remaining questions are the contribution of TRM to the local immune response against (i) non-specific, commensal microbes which could invade the skin upon a skin barrier damage and (ii) invading pathogenic microbes. The first question queries upon their role in chronic pathologies such as psoriasis, atopic dermatitis or PLE. The second question concerns the capacity of TRM to provide a heterologous protection against diverse infections (118) (Figure 2).
Does UV-R Alter Skin Barrier Function, Further Activating Microbe-Specific Skin TRM and Causing Chronic Inflammation?
Commensal microbes are known to improve innate and adaptive responses by producing small molecules which act as mediators between the host and microbes (119). Recently it has been reported that commensal skin microbiome can modulate gene expression of various cytokines, TLRs and AMPs in total skin cells (120). In the skin Staphylococcus aureus is known to promote skin inflammation by producing phenol-soluble modulins (PSMs) (121) which can stimulate IL-1-type (IL-36α and IL-1 α) cytokine production (122) and IL-17 from dermal γδ T cells (123). Moreover, S. aureus secretes proteases which are involved in skin barrier damage, promoting bacterial penetration into the skin which could ultimately generate S. aureus-specific TRM cells. A robust accumulation of commensal-specific T cells under defined conditions may lead to worsening pathogenic conditions such as psoriasis (124, 125). Psoriasis and AD are intriguing examples of possible TRM interplay with commensal microbes. An inflammatory environment exists in these chronic diseases which may lead to severe barrier disruptions through the patient's life. This could eventually lead commensal microbes to penetrate the skin, produce microbial-antigens, and finally lead to specific TRM recruitment and establishment at the inflammatory site. In this context, both allergen-specific TRM and commensal microbe-specific TRM are in place. Whether commensal-specific TRM cells portray a regulatory role or participate in the inflammatory loop is not known. Commensal-specific TRM may also play a role in PLE, an inflammatory skin condition in which itchy skin lesions of diverse morphology occur when the skin is exposed to sunlight. In this disease microbes residing on upper layers may be driven to induce the production of AMPs and express commensal associate molecular patterns (126) which could play a role in pathophysiology of the disease. Furthermore, the capacity of UV-R to cause a barrier defect (127) may contribute to this phenomenon. Patients developing PLE may have skin inhabiting or newly generated commensal-specific TRM that get activated. An inflammatory microenvironment may lead to changes in microbial landscape, further increase specific TRM activation and booster the inflammatory loop.
Conclusion
The specificity of adaptive immune system is complexly linked to the establishment and the persistence of the TRM which recognize previously encountered antigen via specific T cell receptors (TCRs). These specific TRM are generated and kept as a pool of heterogenous population with respect to the numerous microbes and microbe-associated antigens that they encounter during the lifetime of individual. With recent discoveries about potential functions of skin microbiome to educate and modulate host-immune responses, it is important to identify how these microbes influence the skin TRM. Specifically targeting those TRM, directly or via microbiome may allow to develop novel treatment strategies, acting like or even better than phototherapy, but with an improved risk-safety profile.
Author Contributions
VP and LL: conceived the ideas and drafted the manuscript; VP: drafted the figures; J-FN, MV, and PW: corrected and contributed to the draft. All authors revised and approved the final version of the manuscript.
Conflict of Interest Statement
The authors declare that the research was conducted in the absence of any commercial or financial relationships that could be construed as a potential conflict of interest.
Acknowledgments
Ph.D. student VP received funding from the Austrian Science Fund FWF (W1241) and the Medical University of Graz through the Ph.D. Program Molecular Fundamentals of Inflammation (DK-MOLIN). VP was supported by René Touraine Foundation during a research stay in Lyon. LL Ph.D. fellowship is funded by DBV Technologies (Montrouge, France).
References
1. Gallo RL. Human skin is the largest epithelial surface for interaction with microbes. J Invest Dermatol. (2017) 137:1213–1214. doi: 10.1016/j.jid.2016.11.045
2. Findley K, Oh J, Yang J, Conlan S, Deming C, Meyer JA, et al. Topographic diversity of fungal and bacterial communities in human skin. Nature (2013) 498:367–70. doi: 10.1038/nature12171
3. Meyers JM, Munger K. The viral etiology of skin cancer. J Invest Dermatol. (2014) 134:E29–32. doi: 10.1038/skinbio.2014.6
4. Hannigan GD, Meisel JS, Tyldsley AS, Zheng Q, Hodkinson BP, Sanmiguel AJ, et al. The human skin double-stranded DNA virome: topographical and temporal diversity, genetic enrichment, and dynamic associations with the host microbiome. MBio (2015) 6:e01578–01515. doi: 10.1128/mBio.01578-15
5. Horz HP. (2015). Archaeal lineages within the human microbiome: absent, rare or elusive? Life 5:1333–45. doi: 10.3390/life5021333
6. Moissl-Eichinger C, Probst AJ, Birarda G, Auerbach A, Koskinen K, Wolf P, et al. Human age and skin physiology shape diversity and abundance of Archaea on skin. Sci Rep. (2017) 7:4039. doi: 10.1038/s41598-017-04197-4
7. Grice EA, Kong HH, Renaud G, Young AC, Program NCS, Bouffard GG, et al. A diversity profile of the human skin microbiota. Genome Res. (2008) 18:1043–50. doi: 10.1101/gr.075549.107
8. Patra V, Byrne SN, Wolf P. The skin microbiome: is it affected by UV-induced immune suppression? Front Microbiol. (2016) 7:1235. doi: 10.3389/fmicb.2016.01235
9. Martin R, Bermudez-Humaran LG, Langella P. Gnotobiotic rodents: an In Vivo model for the study of microbe-microbe interactions. Front Microbiol. (2016) 7:409. doi: 10.3389/fmicb.2016.00409
10. Naik S, Bouladoux N, Linehan JL, Han SJ, Harrison OJ, Wilhelm C, et al. Commensal-dendritic-cell interaction specifies a unique protective skin immune signature. Nature (2015) 520:104–8. doi: 10.1038/nature14052
11. Nakamizo S, Egawa G, Honda T, Nakajima S, Belkaid Y, Kabashima K. Commensal bacteria and cutaneous immunity. Semin Immunopathol. (2015) 37:73–80. doi: 10.1007/s00281-014-0452-6
12. Grice EA, Dawson TL Jr. Host-microbe interactions: Malassezia and human skin. Curr Opin Microbiol. (2017) 40:81–7. doi: 10.1016/j.mib.2017.10.024
13. Kuhbacher A, Burger-Kentischer A, Rupp S. Interaction of Candida Species with the skin. Microorganisms (2017) 5:32. doi: 10.3390/microorganisms5020032
14. Nakatsuji T, Chiang HI, Jiang SB, Nagarajan H, Zengler K, Gallo RL. The microbiome extends to subepidermal compartments of normal skin. Nat Commun. (2013) 4:1431. doi: 10.1038/ncomms2441
15. Belkaid Y, Segre JA. Dialogue between skin microbiota and immunity. Science (2014) 346:954–959. doi: 10.1126/science.1260144
16. Byrd AL, Belkaid Y, Segre JA. The human skin microbiome. Nat Rev Microbiol. (2018) 16:143–55. doi: 10.1038/nrmicro.2017.157
17. Lee CH, Wu SB, Hong CH, Yu HS, Wei YH. Molecular mechanisms of UV-induced apoptosis and its effects on skin residential cells: the implication in UV-based phototherapy. Int J Mol Sci. (2013) 14:6414–35. doi: 10.3390/ijms14036414
18. Patra V, Halwachs B, Madhusudan N, Wolf P. 523 Ultraviolet-radiation (UV-R) affects the skin microbial load and influences the expression of antimicrobial peptides (AMPs) in mice. J Invest Dermatol. (2016) 136:S249. doi: 10.1016/j.jid.2016.06.546
19. Assarsson M, Duvetorp A, Dienus O, Soderman J, Seifert O. Significant Changes in the Skin Microbiome in Patients with Chronic Plaque Psoriasis after Treatment with Narrowband Ultraviolet B. Acta Derm Venereol. (2017) 98:428–36. doi: 10.2340/00015555-2859
20. Kripke ML, Lofgreen JS, Beard J, Jessup JM, Fisher MS. In vivo immune responses of mice during carcinogenesis by ultraviolet irradiation. J Natl Cancer Inst. (1977) 59:1227–30. doi: 10.1093/jnci/59.4.1227
21. Elmets CA, Bergstresser PR, Tigelaar RE, Wood PJ, Streilein JW. Analysis of the mechanism of unresponsiveness produced by haptens painted on skin exposed to low dose ultraviolet radiation. J Exp Med. (1983) 158:781–94. doi: 10.1084/jem.158.3.781
22. Cooper KD, Oberhelman L, Hamilton TA, Baadsgaard O, Terhune M, Levee G, et al. UV exposure reduces immunization rates and promotes tolerance to epicutaneous antigens in humans: relationship to dose, CD1a-DR+ epidermal macrophage induction, and Langerhans cell depletion. Proc Natl Acad Sci USA. (1992) 89:8497–501. doi: 10.1073/pnas.89.18.8497
23. Kelly DA, Young AR, Mcgregor JM, Seed PT, Potten CS, Walker SL. Sensitivity to sunburn is associated with susceptibility to ultraviolet radiation–induced suppression of cutaneous cell–mediated immunity. J Exp Med. (2000) 191:561–6. doi: 10.1084/jem.191.3.561
24. Wolf P, Hoffmann C, Quehenberger F, Grinschgl S, Kerl H. Immune protection factors of chemical sunscreens measured in the local contact hypersensitivity model in humans. J Invest Dermatol. (2003) 121:1080–7. doi: 10.1046/j.1523-1747.2003.12361.x
25. Applegate LA, Ley RD, Alcalay J, Kripke ML. Identification of the molecular target for the suppression of contact hypersensitivity by ultraviolet radiation. J Exp Med. (1989) 170:1117–31. doi: 10.1084/jem.170.4.1117
26. Wolf P, Nghiem DX, Walterscheid JP, Byrne S, Matsumura Y, Matsumura Y, et al. Platelet-activating factor is crucial in psoralen and ultraviolet A-induced immune suppression, inflammation, and apoptosis. Am J Pathol. (2006) 169:795–805. doi: 10.2353/ajpath.2006.060079
27. De Fabo EC, Noonan FP. Mechanism of immune suppression by ultraviolet irradiation in vivo. I. Evidence for the existence of a unique photoreceptor in skin and its role in photoimmunology. J Exp Med. (1983) 158:84–98. doi: 10.1084/jem.158.1.84
28. Kubica M, Hildebrand F, Brinkman BM, Goossens D, Del Favero J, Vercammen K, et al. The skin microbiome of caspase-14-deficient mice shows mild dysbiosis. Exp Dermatol. (2014) 23:561–7. doi: 10.1111/exd.12458
29. Mcaleer MA, Irvine AD. The multifunctional role of filaggrin in allergic skin disease. J Allergy Clin Immunol. (2013) 131:280–91. doi: 10.1016/j.jaci.2012.12.668
30. Hug DH, Dunkerson DD, Hunter JK. The degradation of L-histidine and trans- and cis-urocanic acid by bacteria from skin and the role of bacterial cis-urocanic acid isomerase. J Photochem Photobiol B (1999) 50:66–73. doi: 10.1016/S1011-1344(99)00072-X
31. Patra V, Bashir M, Somlapura M, Kofeler HC, Peiber T, Wolf P. Isomerization of urocanic acid by ultraviolet radiation and its role in modulation of skin microbiome, antimicrobial peptides, and immune function. J Invest Dermatol. (2017). 137:S261–S261. doi: 10.1016/j.jid.2017.07.595
32. Jeevan A, Evans R, Brown EL, Kripke ML. Effect of local ultraviolet irradiation on infections of mice with Candida albicans, Mycobacterium bovis BCG, and Schistosoma mansoni. J Invest Dermatol. (1992) 99:59–64. doi: 10.1111/1523-1747.ep12611853
33. Cestari TF, Kripke ML, Baptista PL, Bakos L, Bucana CD. Ultraviolet radiation decreases the granulomatous response to lepromin in humans. J Invest Dermatol. (1995) 105:8–13. doi: 10.1111/1523-1747.ep12312309
34. Brown EL, Ullrich SE, Pride M, Kripke ML. The effect of UV irradiation on infection of mice with Borrelia burgdorferi Photochem Photobiol. (2001) 73:537–44. doi: 10.1562/0031-8655(2001)073<0537:TEOUIO>2.0.CO;2
35. Glaser R, Navid F, Schuller W, Jantschitsch C, Harder J, Schroder JM, et al. UV-B radiation induces the expression of antimicrobial peptides in human keratinocytes in vitro and in vivo. J Allergy Clin Immunol. (2009) 123:1117–23. doi: 10.1016/j.jaci.2009.01.043
36. Brandwein M, Bentwich Z, Steinberg D. Endogenous antimicrobial peptide expression in response to bacterial epidermal colonization. Front Immunol. (2017) 8:1637. doi: 10.3389/fimmu.2017.01637
37. Biragyn A, Ruffini PA, Leifer CA, Klyushnenkova E, Shakhov A, Chertov O, et al. Toll-like receptor 4-dependent activation of dendritic cells by beta-defensin 2. Science (2002). 298:1025–29. doi: 10.1126/science.1075565
38. Niyonsaba F, Ushio H, Nakano N, Ng W, Sayama K, Hashimoto K, et al. Antimicrobial peptides human beta-defensins stimulate epidermal keratinocyte migration, proliferation and production of proinflammatory cytokines and chemokines. J Invest Dermatol. (2007) 127:594–604. doi: 10.1038/sj.jid.5700599
39. Navid F, Boniotto M, Walker C, Ahrens K, Proksch E, Sparwasser T, et al. Induction of regulatory T cells by a murine beta-defensin. J Immunol. (2012) 188:735–43. doi: 10.4049/jimmunol.1100452
40. Patra V, Mayer G, Gruber-Wackernagel A, Horn M, Lembo S, Wolf P. Unique profile of antimicrobial peptide expression in polymorphic light eruption lesions compared to healthy skin, atopic dermatitis, and psoriasis. Photodermatol Photoimmunol Photomed. (2017) 34:137–144. doi: 10.1111/phpp.12355
41. Cooper KD, Fox P, Neises G, Katz SI. Effects of ultraviolet radiation on human epidermal cell alloantigen presentation: initial depression of Langerhans cell-dependent function is followed by the appearance of T6- Dr+ cells that enhance epidermal alloantigen presentation. J Immunol. (1985) 134:129–37.
42. Cooper KD, Neises GR, Katz SI. Antigen-presenting OKM5+ melanophages appear in human epidermis after ultraviolet radiation. J Invest Dermatol. (1986) 86:363–70. doi: 10.1111/1523-1747.ep12285600
43. Cooper KD, Duraiswamy N, Hammerberg C, Allen ED, Kimbrough-Green C, Dillon W, et al. Neutrophils, differentiated macrophages, and monocyte/macrophage antigen presenting cells infiltrate murine epidermis after UV injury. J Invest Dermatol. (1993) 101:155–63. doi: 10.1111/1523-1747.ep12363639
44. Toews GB, Bergstresser PR, Streilein JW. Epidermal Langerhans cell density determines whether contact hypersensitivity or unresponsiveness follows skin painting with DNFB. J Immunol. (1980) 124:445–53.
45. Noonan FP, Bucana C, Sauder DN, De Fabo EC. Mechanism of systemic immune suppression by UV irradiation in vivo. II. The UV effects on number and morphology of epidermal Langerhans cells and the UV-induced suppression of contact hypersensitivity have different wavelength dependencies. J Immunol. (1984) 132:2408–16.
46. Achachi A, Vocanson M, Bastien P, Peguet-Navarro J, Grande S, Goujon C, et al. UV radiation induces the epidermal recruitment of dendritic cells that compensate for the depletion of langerhans cells in human skin. J Invest Dermatol. (2015) 135:2058–67. doi: 10.1038/jid.2015.118
47. Schwarz A, Noordegraaf M, Maeda A, Torii K, Clausen BE, Schwarz T. Langerhans cells are required for UVR-induced immunosuppression. J Invest Dermatol. (2010) 130:1419–27. doi: 10.1038/jid.2009.429
48. Liu X, Huang H, Gao H, Wu X, Zhang W, Yu B, et al. Regulatory B cells induced by ultraviolet B through toll-like receptor 4 signalling contribute to the suppression of contact hypersensitivity responses in mice. Contact Dermatitis (2018) 78:117–30. doi: 10.1111/cod.12913
49. Hart PH, Grimbaldeston MA, Finlay-Jones JJ. Sunlight, immunosuppression and skin cancer: role of histamine and mast cells. Clin Exp Pharmacol Physiol. (2001) 28:1–8. doi: 10.1046/j.1440-1681.2001.03392.x
50. Schwarz T. The dark and the sunny sides of UVR-induced immunosuppression: photoimmunology revisited. J Invest Dermatol. (2010) 130:49–54. doi: 10.1038/jid.2009.217
51. Klonowski KD, Williams KJ, Marzo AL, Blair DA, Lingenheld EG, Lefrancois L. Dynamics of blood-borne CD8 memory T cell migration in vivo. Immunity (2004) 20:551–62.
52. Mackay LK, Rahimpour A, Ma JZ, Collins N, Stock AT, Hafon ML, et al. The developmental pathway for CD103(+)CD8+ tissue-resident memory T cells of skin. Nat Immunol. (2013) 14:1294–301. doi: 10.1038/ni.2744
53. Ariotti S, Hogenbirk MA, Dijkgraaf FE, Visser LL, Hoekstra ME, Song JY, et al. T cell memory. Skin-resident memory CD8+ T cells trigger a state of tissue-wide pathogen alert. Science (2014) 346:101–5. doi: 10.1126/science.1254803
54. Schenkel JM, Fraser KA, Beura LK, Pauken KE, Vezys V, Masopust D. T cell memory. Resident memory CD8 T cells trigger protective innate and adaptive immune responses. Science (2014) 346:98–101. doi: 10.1126/science.1254536
55. Teijaro JR, Turner D, Pham Q, Wherry EJ, Lefrancois L, Farber DL. Cutting edge: tissue-retentive lung memory CD4 T cells mediate optimal protection to respiratory virus infection. J Immunol. (2011) 187:5510–4. doi: 10.4049/jimmunol.1102243
56. Wakim LM, Woodward-Davis A, Liu R, Hu Y, Villadangos J, Smyth G, et al. The molecular signature of tissue resident memory CD8 T cells isolated from the brain. J Immunol. (2012) 189:3462–71. doi: 10.4049/jimmunol.1201305
57. Fernandez-Ruiz D, Ng WY, Holz LE, Ma JZ, Zaid A, Wong YC, et al. Liver-resident memory CD8+ T cells form a front-line defense against malaria liver-stage infection. Immunity (2016) 45:889–902. doi: 10.1016/j.immuni.2016.08.011
58. Gehad A, Teague JE, Matos TR, Huang V, Yang C, Watanabe R, et al. A primary role for human central memory cells in tissue immunosurveillance. Blood Adv. (2018) 2:292–8. doi: 10.1182/bloodadvances.2017011346
59. Gaide O, Emerson RO, Jiang X, Gulati N, Nizza S, Desmarais C, et al. Common clonal origin of central and resident memory T cells following skin immunization. Nat Med. (2015) 21:647–53. doi: 10.1038/nm.3860
60. Jiang X, Clark RA, Liu L, Wagers AJ, Fuhlbrigge RC, Kupper TS. Skin infection generates non-migratory memory CD8+ T(RM) cells providing global skin immunity. Nature (2012) 483:227–31. doi: 10.1038/nature10851
61. Gebhardt T, Whitney PG, Zaid A, Mackay LK, Brooks AG, Heath WR, et al. Different patterns of peripheral migration by memory CD4+ and CD8+ T cells. Nature (2011) 477:216–219. doi: 10.1038/nature10339
62. Mohammed J, Beura LK, Bobr A, Astry B, Chicoine B, Kashem SW, et al. Stromal cells control the epithelial residence of DCs and memory T cells by regulated activation of TGF-beta. Nat Immunol. (2016) 17:414–21. doi: 10.1038/ni.3396
63. Skon CN, Lee JY, Anderson KG, Masopust D, Hogquist KA, Jameson SC. Transcriptional downregulation of S1pr1 is required for the establishment of resident memory CD8+ T cells. Nat Immunol. (2013) 14:1285–93. doi: 10.1038/ni.2745
64. Adachi T, Kobayashi T, Sugihara E, Yamada T, Ikuta K, Pittaluga S, et al. Hair follicle-derived IL-7 and IL-15 mediate skin-resident memory T cell homeostasis and lymphoma. Nat Med. (2015) 21:1272–9. doi: 10.1038/nm.3962
65. Pan Y, Tian T, Park CO, Lofftus SY, Mei S, Liu X, et al. Survival of tissue-resident memory T cells requires exogenous lipid uptake and metabolism. Nature (2017) 543:252–6. doi: 10.1038/nature21379
66. Park SL, Zaid A, Hor JL, Christo SN, Prier JE, Davies B, et al. Local proliferation maintains a stable pool of tissue-resident memory T cells after antiviral recall responses. Nat Immunol. (2018) 19:183–91. doi: 10.1038/s41590-017-0027-5
67. Cheuk S, Schlums H, Gallais Serezal I, Martini E, Chiang SC, Marquardt N, et al. CD49a expression defines tissue-resident CD8+ T cells poised for cytotoxic function in human skin. Immunity (2017) 46:287–300. doi: 10.1016/j.immuni.2017.01.009
68. Clark RA, Chong B, Mirchandani N, Brinster NK, Yamanaka K, Dowgiert RK, et al. The vast majority of CLA+ T cells are resident in normal skin. J Immunol. (2006) 176:4431–9. doi: 10.4049/jimmunol.176.7.4431
69. Schaerli P, Ebert LM, Moser B. Comment on The vast majority of CLA+ T cells are resident in normal skin. J Immunol. (2006) 177:1375–6; author reply 1376–7. doi: 10.4049/jimmunol.177.3.1375
70. Girardi M, Oppenheim DE, Steele CR, Lewis JM, Glusac E, Filler R, et al. Regulation of cutaneous malignancy by gammadelta T cells. Science (2001) 294:605–9. doi: 10.1126/science.1063916
71. Toulon A, Breton L, Taylor KR, Tenenhaus M, Bhavsar D, Lanigan C, et al. A role for human skin-resident T cells in wound healing. J Exp Med. (2009) 206:743–50. doi: 10.1084/jem.20081787
72. Macleod AS, Hemmers S, Garijo O, Chabod M, Mowen K, Witherden DA, et al. Dendritic epidermal T cells regulate skin antimicrobial barrier function. J Clin Invest. (2013) 123:4364–74. doi: 10.1172/JCI70064
73. Li-Weber M, Treiber MK, Giaisi M, Palfi K, Stephan N, Parg S, et al. Ultraviolet irradiation suppresses T cell activation via blocking TCR-mediated E. R. K., and NF-κB signaling pathways. J Immunol. (2005) 175:2132–43. doi: 10.4049/jimmunol.175.4.2132
74. Rana S, Byrne SN, Macdonald LJ, Chan CY.-Y., Halliday GM. Ultraviolet B suppresses immunity by inhibiting effector and memory T cells. Am J Pathol. (2008) 172:993–1004. doi: 10.2353/ajpath.2008.070517
75. Schwarz T. 25 years of UV-induced immunosuppression mediated by T cells-from disregarded T suppressor cells to highly respected regulatory T cells. Photochem Photobiol (2008) 84:10–8. doi: 10.1111/j.1751-1097.2007.00223.x
76. Takai E, Tsukimoto M, Harada H, Kojima S. Involvement of P2Y6 receptor in p38 MAPK-mediated COX-2 expression in response to UVB irradiation of human keratinocytes. Radiat Res. (2011) 175:358–66. doi: 10.1667/RR2375.1
77. Macleod AS, Rudolph R, Corriden R, Ye I, Garijo O, Havran WL. Skin-resident T cells sense ultraviolet radiation-induced injury and contribute to DNA repair. J Immunol. (2014) 192:5695–702. doi: 10.4049/jimmunol.1303297
78. Corriden R, Insel PA. Basal release of ATP: an autocrine-paracrine mechanism for cell regulation. Sci Signal. (2010) 3:re1. doi: 10.1126/scisignal.3104re1
79. 78. Skin Cancer Foundation. Actinic keratosis (AK). New York, NY: Skin Cancer Foundation. (2014) Available online at: https://www.skincancer.org/skin-cancer-information/actinic-keratosis (Accessed Jan 23, 2018).
80. Park CO, Kupper TS. The emerging role of resident memory T cells in protective immunity and inflammatory disease. Nat Med. (2015) 21:688–97. doi: 10.1038/nm.3883
81. Campbell JJ, Clark RA, Watanabe R, Kupper TS. Sezary syndrome and mycosis fungoides arise from distinct T-cell subsets: a biologic rationale for their distinct clinical behaviors. Blood (2010) 116:767–71. doi: 10.1182/blood-2009-11-251926
82. Vieyra-Garcia PA, Wei T, Naym DG, Fredholm S, Fink-Puches R, Cerroni L, et al. STAT3/5-dependent IL9 overexpression contributes to neoplastic cell survival in mycosis fungoides. Clin Cancer Res. (2016) 22:3328–39. doi: 10.1158/1078-0432.CCR-15-1784
83. Clark RA. Resident memory T cells in human health and disease. Sci Transl Med. (2015) 7:269rv261. doi: 10.1126/scitranslmed.3010641
84. Mcfall-Ngai M. Adaptive immunity: care for the community. Nature (2007) 445:153. doi: 10.1038/445153a
85. Nakatsuji T, Gallo RL. Dermatological therapy by topical application of non-pathogenic bacteria. J Invest Dermatol. (2014) 134:11–4. doi: 10.1038/jid.2013.379
86. Nakatsuji T, Chen TH, Narala S, Chun KA, Two AM, Yun T, et al. Antimicrobials from human skin commensal bacteria protect against Staphylococcus aureus and are deficient in atopic dermatitis. Sci Transl Med. (2017) 9:eaah4680. doi: 10.1126/scitranslmed.aah4680
87. Schroder T, Ibrahim S. The microbiome and autoimmunity. Internist (2017) 58:449–55. doi: 10.1007/s00108-017-0221-4
88. Hand TW, Dos Santos LM, Bouladoux N, Molloy MJ, Pagan AJ, Pepper M, et al. Acute gastrointestinal infection induces long-lived microbiota-specific T cell responses. Science (2012) 337:1553–6. doi: 10.1126/science.1220961
89. Teijaro JR, Njau MN, Verhoeven D, Chandran S, Nadler SG, Hasday J, et al. Costimulation modulation uncouples protection from immunopathology in memory T cell responses to influenza virus. J Immunol. (2009) 182:6834–43. doi: 10.4049/jimmunol.0803860
90. Wherry EJ, Ahmed R. Memory CD8 T-cell differentiation during viral infection. J Virol. (2004) 78:5535–45. doi: 10.1128/JVI.78.11.5535-5545.2004
91. Remakus S, Sigal LJ. Memory CD8+ T cell protection. Adv Exp Med Biol. (2013) 785:77–86. doi: 10.1007/978-1-4614-6217-0_9
92. Gebhardt T, Wakim LM, Eidsmo L, Reading PC, Heath WR, Carbone FR. Memory T cells in nonlymphoid tissue that provide enhanced local immunity during infection with herpes simplex virus. Nat Immunol. (2009) 10:524–30. doi: 10.1038/ni.1718
93. Khader SA, Bell GK, Pearl JE, Fountain JJ, Rangel-Moreno J, Cilley GE, et al. IL-23 and IL-17 in the establishment of protective pulmonary CD4+ T cell responses after vaccination and during Mycobacterium tuberculosis challenge. Nat Immunol. (2007) 8:369–77. doi: 10.1038/ni1449
94. Anthony RM, Urban JF, Alem F, Hamed HA, Rozo CT, Boucher J-L, et al. Memory T(H)2 cells induce alternatively activated macrophages to mediate protection against nematode parasites. Nat Med. (2006) 12:955–60. doi: 10.1038/nm1451
95. Ellefsen K, Harari A, Champagne P, Bart PA, Sekaly RP, Pantaleo G. Distribution and functional analysis of memory antiviral CD8 T cell responses in HIV-1 and cytomegalovirus infections. Eur J Immunol. (2002) 32:3756–64. doi: 10.1002/1521-4141(200212)32:12<3756::AID-IMMU3756>3.0.CO;2-E
96. Pedron B, Guerin V, Cordeiro DJ, Masmoudi S, Dalle JH, Sterkers G. Development of cytomegalovirus and adenovirus-specific memory CD4 T-cell functions from birth to adulthood. Pediatr Res. (2011) 69:106–11. doi: 10.1203/PDR.0b013e318204e469
97. Wang A, Chandran S, Shah SA, Chiu Y, Paria BC, Aghamolla T, et al. The stoichiometric production of IL-2 and IFN-gamma mRNA defines memory T cells that can self-renew after adoptive transfer in humans. Sci Transl Med. (2012) 4:149ra120. doi: 10.1126/scitranslmed.3004306
98. Masopust D, Vezys V, Marzo AL, Lefrancois L. Preferential localization of effector memory cells in nonlymphoid tissue. Science (2001) 291:2413–7. doi: 10.1126/science.1058867
99. Reinhardt RL, Khoruts A, Merica R, Zell T, Jenkins MK. Visualizing the generation of memory CD4 T cells in the whole body. Nature (2001) 410:101–5. doi: 10.1038/35065111
100. Liu L, Zhong Q, Tian T, Dubin K, Athale SK, Kupper TS. Epidermal injury and infection during poxvirus immunization is crucial for the generation of highly protective T cell-mediated immunity. Nat Med. (2010) 16:224–7. doi: 10.1038/nm.2078
101. Clark RA, Watanabe R, Teague JE, Schlapbach C, Tawa MC, Adams N, et al. Skin effector memory T cells do not recirculate and provide immune protection in alemtuzumab-treated CTCL patients. Sci Transl Med. (2012) 4:117ra117. doi: 10.1126/scitranslmed.3003008
102. Park CO, Fu X, Jiang X, Pan Y, Teague JE, Collins N, et al. Staged development of long-lived T-cell receptor alphabeta TH17 resident memory T-cell population to Candida albicans after skin infection. J Allergy Clin Immunol. (2017) S0091–6749:31737–2. doi: 10.1016/j.jaci.2017.09.042
103. Beura LK, Hamilton SE, Bi K, Schenkel JM, Odumade OA, Casey KA, et al. Normalizing the environment recapitulates adult human immune traits in laboratory mice. Nature (2016) 532:512. doi: 10.1038/nature17655
104. Reese TA, Bi K, Kambal A, Filali-Mouhim A, Beura LK, Burger MC, et al. Sequential infection with common pathogens promotes human-like immune gene expression and altered vaccine response. Cell Host Microbe (2016) 19:713–9. doi: 10.1016/j.chom.2016.04.003
105. Gonzalez PA, Prado CE, Leiva ED, Carreno LJ, Bueno SM, Riedel CA, et al. Respiratory syncytial virus impairs T cell activation by preventing synapse assembly with dendritic cells. Proc Natl Acad Sci USA. (2008) 105:14999–5004. doi: 10.1073/pnas.0802555105
106. Zhu J, Koelle DM, Cao J, Vazquez J, Huang ML, Hladik F, et al. Virus-specific CD8+ T cells accumulate near sensory nerve endings in genital skin during subclinical HSV-2 reactivation. J Exp Med. (2007) 204:595–603. doi: 10.1084/jem.20061792
107. Linehan JL, Harrison OJ, Han SJ, Byrd AL, Vujkovic-Cvijin I, Villarino AV, et al. Non-classical immunity controls microbiota impact on skin immunity and tissue repair. Cell (2018) 172:784–96 e718. doi: 10.1016/j.cell.2017.12.033
108. Wolf P, Maier H, Mullegger RR, Chadwick CA, Hofmann-Wellenhof R, Soyer HP, et al. Topical treatment with liposomes containing T4 endonuclease V protects human skin in vivo from ultraviolet-induced upregulation of interleukin-10 and tumor necrosis factor-alpha. J Invest Dermatol. (2000) 114:149–56. doi: 10.1046/j.1523-1747.2000.00839.x
109. Quan T, He T, Kang S, Voorhees JJ, Fisher GJ. Ultraviolet irradiation alters transforming growth factor beta/smad pathway in human skin in vivo. J Invest Dermatol. (2002) 119:499–506. doi: 10.1046/j.1523-1747.2002.01834.x
110. Byrne SN, Beaugie C, O'sullivan C, Leighton S, Halliday GM. The immune-modulating cytokine and endogenous Alarmin interleukin-33 is upregulated in skin exposed to inflammatory UVB radiation. Am J Pathol. (2011) 179:211–22. doi: 10.1016/j.ajpath.2011.03.010
111. Meephansan J, Komine M, Tsuda H, Tominaga S, Ohtsuki M. Ultraviolet B irradiation induces the expression of IL-33 mRNA and protein in normal human epidermal keratinocytes. J Dermatol Sci. (2012) 65:72–4. doi: 10.1016/j.jdermsci.2011.10.004
112. Kim MT, Harty JT. Impact of inflammatory cytokines on effector and memory CD8+ T cells. Front Immunol. (2014) 5:295. doi: 10.3389/fimmu.2014.00295
113. Vallat VP, Gilleaudeau P, Battat L, Wolfe J, Nabeya R, Heftler N, et al. PUVA bath therapy strongly suppresses immunological and epidermal activation in psoriasis: a possible cellular basis for remittive therapy. J Exp Med. (1994) 180:283–96. doi: 10.1084/jem.180.1.283
114. Krueger JG, Wolfe JT, Nabeya RT, Vallat VP, Gilleaudeau P, Heftler NS, et al. Successful ultraviolet B treatment of psoriasis is accompanied by a reversal of keratinocyte pathology and by selective depletion of intraepidermal T cells. J Exp Med. (1995) 182:2057–68. doi: 10.1084/jem.182.6.2057
115. Ozawa M, Ferenczi K, Kikuchi T, Cardinale I, Austin LM, Coven TR, et al. 312-nanometer ultraviolet B light (narrow-band UVB) induces apoptosis of T cells within psoriatic lesions. J Exp Med. (1999) 189:711–8. doi: 10.1084/jem.189.4.711
116. Patrizi A, Raone B, Ravaioli GM. Management of atopic dermatitis: safety and efficacy of phototherapy. Clin Cosmet Investig Dermatol. (2015) 8:511–20. doi: 10.2147/CCID.S87987
117. Rodriguez JM, Murphy K, Stanton C, Ross RP, Kober OI, Juge N, et al. The composition of the gut microbiota throughout life, with an emphasis on early life. Microb Ecol Health Dis. (2015) 26:26050. doi: 10.3402/mehd.v26.26050
118. Su LF, Kidd BA, Han A, Kotzin JJ, Davis MM. Virus-specific CD4+ memory-phenotype T cells are abundant in unexposed adults. Immunity (2013) 38:373–83. doi: 10.1016/j.immuni.2012.10.021
119. Donia MS, Fischbach MA. Human Microbiota. Small Molecules Human Microbiota Science (2015) 349:1254766. doi: 10.1126/science.1254766
120. Meisel JS, Sfyroera G, Bartow-Mckenney C, Gimblet C, Bugayev J, Horwinski J, et al. Commensal microbiota modulate gene expression in the skin. Microbiome (2018) 6:20. doi: 10.1186/s40168-018-0404-9
121. Nakagawa S, Matsumoto M, Katayama Y, Oguma R, Wakabayashi S, Nygaard T, et al. Staphylococcus aureus virulent psmalpha peptides induce keratinocyte alarmin release to orchestrate IL-17-dependent skin inflammation. Cell Host Microbe (2017) 22:667–77 e665. doi: 10.1016/j.chom.2017.10.008
122. Liu H, Archer NK, Dillen CA, Wang Y, Ashbaugh AG, Ortines RV, et al. Staphylococcus aureus epicutaneous exposure drives skin inflammation via IL-36-mediated T cell responses. Cell Host Microbe (2017) 22:653–66 e655. doi: 10.1016/j.chom.2017.10.006
123. Williams MR, Nakatsuji T, Gallo RL. Staphylococcus aureus: master manipulator of the skin. Cell Host Microbe (2017) 22:579–81. doi: 10.1016/j.chom.2017.10.015
124. Sartor RB. Mechanisms of disease: pathogenesis of Crohn's disease and ulcerative colitis. Nat Clin Pract Gastroenterol Hepatol. (2006) 3:390–407. doi: 10.1038/ncpgasthep0528
125. Belkaid Y, Bouladoux N, Hand TW. Effector and memory T cell responses to commensal bacteria. Trends Immunol. (2013) 34:299–306. doi: 10.1016/j.it.2013.03.003
126. Patra V, Wolf P. Microbial elements as the initial triggers in the pathogenesis of polymorphic light eruption? Exp Dermatol. (2016) 25:999–1001. doi: 10.1111/exd.13162
Keywords: skin microbiome, ultraviolet-radiation, skin resident memory T cells, inflammation, immune suppression, photomedicine, phototherapy
Citation: Patra V, Laoubi L, Nicolas J-F, Vocanson M and Wolf P (2018) A Perspective on the Interplay of Ultraviolet-Radiation, Skin Microbiome and Skin Resident Memory TCRαβ+ Cells. Front. Med. 5:166. doi: 10.3389/fmed.2018.00166
Received: 28 February 2018; Accepted: 11 May 2018;
Published: 30 May 2018.
Edited by:
Günther F.L. Hofbauer, Universität Zürich, SwitzerlandReviewed by:
Oleg E. Akilov, University of Pittsburgh, United StatesSalvador Gonzalez, University of Alcalá, Spain
Copyright © 2018 Patra, Laoubi, Nicolas, Vocanson and Wolf. This is an open-access article distributed under the terms of the Creative Commons Attribution License (CC BY). The use, distribution or reproduction in other forums is permitted, provided the original author(s) and the copyright owner are credited and that the original publication in this journal is cited, in accordance with accepted academic practice. No use, distribution or reproduction is permitted which does not comply with these terms.
*Correspondence: Marc Vocanson, bWFyYy52b2NhbnNvbkBpbnNlcm0uZnI=
Peter Wolf, cGV0ZXIud29sZkBtZWR1bmlncmF6LmF0
†These authors have contributed equally to this work.