The Precursor to Glutathione (GSH), γ-Glutamylcysteine (GGC), Can Ameliorate Oxidative Damage and Neuroinflammation Induced by Aβ40 Oligomers in Human Astrocytes
- 1Centre for Healthy Ageing, School of Psychiatry, Faculty of Medicine, University of New South Wales, Sydney, NSW, Australia
- 2School of Biotechnology and Biomolecular Sciences, Faculty of Science, University of New South Wales, Sydney, NSW, Australia
- 3Faculty of Medicine, University of New South Wales, Sydney, NSW, Australia
- 4Department of Aged Care and Rehabilitation, Bankstown Hospital, Bankstown, NSW, Australia
- 5Neuropsychiatric Institute, Euroa Centre, Prince of Wales Hospital, Sydney, NSW, Australia
Glutathione (GSH) is one of the most abundant thiol antioxidants in cells. Many chronic and age-related diseases are associated with a decline in cellular GSH levels or impairment in the catalytic activity of the GSH biosynthetic enzyme glutamate cysteine ligase (GCL). γ-glutamylcysteine (GGC), a precursor to glutathione (GSH), can replenish depleted GSH levels under oxidative stress conditions, by circumventing the regulation of GSH biosynthesis and providing the limiting substrate. Soluble amyloid-β (Aβ) oligomers have been shown to induce oxidative stress, synaptic dysfunction and memory deficits which have been reported in Alzheimer’s disease (AD). Calcium ions, which are increased with age and in AD, have been previously reported to enhance the formation of Aβ40 oligomers, which have been casually associated with the pathogenesis of the underlying neurodegenerative condition. In this study, we examined the potential beneficial effects of GGC against exogenous Aβ40 oligomers on biomarkers of apoptosis and cell death, oxidative stress, and neuroinflammation, in human astrocytes. Treatment with Aβ40 oligomers significantly reduced the cell viability and apoptosis of astrocyte brain cultures and increased oxidative modifications of DNA, lipids, and protein, enhanced pro-inflammatory cytokine release and increased the activity of the proteolytic matrix metalloproteinase enzyme, matric metalloproteinase (MMP)-2 and reduced the activity of MMP-9 after 24 h. Co-treatment of Aβ40 oligomers with GGC at 200 μM increased the activity of the antioxidant enzymes superoxide dismutase (SOD) and glutathione peroxidase (GPx) and led to significant increases in the levels of the total antioxidant capacity (TAC) and GSH and reduced the GSSG/GSH ratio. GGC also upregulated the level of the anti-inflammatory cytokine IL-10 and reduced the levels of the pro-inflammatory cytokines (TNF-α, IL-6, and IL-1β) and attenuated the changes in metalloproteinase activity in oligomeric Aβ40-treated astrocytes. Our data provides renewed insight on the beneficial effects of increased GSH levels by GGC in human astrocytes, and identifies yet another potential therapeutic strategy to attenuate the cytotoxic effects of Aβ oligomers in AD.
Introduction
Alzheimer’s disease (AD) is the most common form of dementia affecting the elderly. Extracellular deposition of β-amyloid (Aβ plaques), intraneuronal tau accumulation, inflammation (activated astrocytes and microglia), and neuronal loss are all consistent pathological features of the disease (Porquet et al., 2015). Unlike Aβ plaques, inflammation correlates with neuronal loss and cognitive decline in AD, suggesting it plays an important role in disease progression (Wang et al., 2015; Wilkins et al., 2015).
It is well established that Aβ peptides existed in both fibrillary and non-fibrillar forms (Poduslo and Howell, 2015; Zhang et al., 2015). Soluble oligomeric Aβ aggregates have been shown to bind specifically to synapses in differentiated hippocampal neuronal cultures. These oligomers are capable of disrupting long-term potentiation, a classic experimental paradigm for memory and synaptic plasticity (Izzo et al., 2014; Nguyen and Derreumaux, 2014). Small soluble Aβ oligomers are now considered the primary neurotoxic entity in AD. Several studies have shown that both oligomeric Aβ42 and Aβ40 are both neurotoxic using both human and murine neuronal cell cultures. Moreover, there is a strong association between the level of soluble oligomeric Aβ, and the severity of synaptic loss and cognitive dysfunction in AD when compared to their fibrillar counterpart (Lesne, 2014; Nguyen et al., 2014; Wang et al., 2015). Similarly, a number of early-onset familial AD mutations display a more aggressive disease course and a greater propensity for Aβ to form soluble oligomeric Aβ aggregates (Ferreira and Klein, 2011; Streltsov et al., 2011; Wilcox et al., 2011). While several studies have examined the neurotoxic potential of Aβ42 in several models, little is known about the effects of Aβ40 oligomers in human astrocytes.
Glutathione (GSH) is an important endogenous antioxidant found in millimolar concentrations in the brain. GSH levels have been shown to decrease with ageing and in several age-related degenerative diseases and AD in particular (Harris et al., 2015; Ilyas and Rehman, 2015; Romero-Haro and Alonso-Alvarez, 2015). Soluble oligomeric Aβ has been shown to induce oxidative stress and has been proposed to play a central role in the oxidative damage detected in AD brain (Xu et al., 2014). It has been shown that administration of γ-glutamylcysteine (GGC) increases cellular levels of GSH, circumventing the regulation of GSH biosynthesis by providing the limiting substrate (Nakamura et al., 2012; Quintana-Cabrera et al., 2012).
Whilst extracellular Aβ plaques, NFT, inflammation in the form or reactive astrocytes and microglia, and neuronal loss are all consistent pathological features of AD, a mechanistic link between these factors is yet to be clarified. Although most of the past research has focused on fibrillar Aβ, soluble oligomeric Aβ species are now considered to be of major pathological importance in AD. GGC is a dipeptide which exhibits potent antioxidant properties in several experimental models (Lai et al., 2008; Quintana-Cabrera et al., 2012). It serves as an essential cofactor for the antioxidant enzyme glutathione peroxidase (GPx) and is a precursor for GSH synthesis (Quintana-Cabrera et al., 2012). The metal-chelating properties of GGC have also been demonstrated previously (Salama et al., 2016).
In this study, we evaluated the protective role of up-regulation of GSH by GGC against biomarkers of apoptosis and cell death, oxidative stress, and neuroinflammation against oligomeric Aβ40 in primary human astrocyte cell cultures.
Materials and Methods
Cell Cultures
Human adult brain tissues were collected following surgical resection with both informed and written consent from patients who underwent surgery at the Centre for Mininally Invasive Neurosurgery, Prince of Wales Private Hospital, NSW, Australia. Healthy brain specimens were also acquired from patients during surgery for their tumor. A portion of the surgically resected brain tumor and healthy tissue sample was snap-frozen in liquid nitrogen immediately and stored at −80°C for prolonged storage for future use. Astrocytes were prepared from the mixed brain cell cultures using a protocol previously described by Guillemin et al. (2001).
Human astrocytes were pre-incubated for 15 min with 200 μM GGC. Afterward, cells were treated with oligomeric Aβ40 (10 nM, see Supplementary File for production and characterization), and biochemical assays were subsequently measured 24 h later. Experiments were performed in quadruplicates using cultures derived from three different human fetal brains.
Extracellular LDH Activity as a Measurement for Cytotoxicity
The release of lactate dehydrogenase (LDH) into culture supernatant correlated with the amount of cell death and membrane damage, providing an accurate measure of cellular toxicity. LDH activity was assayed using a standard spectrophotometric technique described by Koh and Choi (1987).
Caspase-3 Activity as a Measurement for Apoptosis
Caspase-3 activity is a well-established apoptotic biomarker. We quantified caspase-3 activity in brain cells using a commercially available kit (R&D systems) according to the manufacturer’s instructions. Briefly, an aliquot of cell homogenate was incubated with the labeled substrate DEVD-pNA (acetyl-Asp-Glu-Val-Asp p-nitroaniline), which is cleaved by the caspase-3 enzyme and releases the chromophore pNA. The levels of pNA were detected using the BMG Fluostar Optima multimode plate reader (NY, USA), at a wavelength of 405 nm.
7′-Dichlorofluorescin Diacetate (DCFDA) Assay for Production of Reactive Oxygen Species
Cells were incubated in 10 μM DCFDA (Sigma) in PBS at 37°C for 30 min as previously described (Chiu et al., 2013). Treatments were added in PBS. After addition of treatments, Fluorescence was measured at 0 min and 90 min with an excitation wavelength of 485 nm and an emission wavelength of 535 nm. The initial zero-point readings were subtracted from the 90 min readings as background.
Quantification of 8-Hydroxy-2′-Deoxyguanosine (8-OH-dG) as a Marker for Oxidative DNA Damage
We used 8-Hydroxy-2′-Deoxyguanosine (8-OH-dG) as a biomarker for oxidative DNA on brain cells exposed to different treatments using the commercially available HT 8-OH-dG ELISA II kit (R&D Systems) as guided by the manufacturer’s instructions. Briefly, the 8-OH-dG monoclonal antibody binds competitively to the pre-coated 8-OH-dG as well as in the cell homogenate. Antibody bound to 8-OH-dG in the cell homogenate is washed away during washing and only antibody bound to the well was detected using a HRP-conjugate and colorimetric substrate and the BMG Fluostar Optima multimode plate reader (NY, USA).
Measurement of Lipid Peroxidation
Lipid peroxidation level in the brain cell homogenate was quantified using the thiobarbituric acid reactive substances (TBARS) as previously described (Buege and Aust, 1978) and using the BMG Fluostar Optima multimode plate reader (NY, USA).
Measurement of Protein Carbonyl Content
Protein carbonyls were used as a measure of protein oxidation, in brain cell homogenates using DNPH as previously described (Levine et al., 2000) and using the BMG Fluostar Optima multimode plate reader (NY, USA).
Measurement of Superoxide Dismutase (SOD) and Glutathione Peroxidase (GPx) of the Antioxidant Enzymes Activity
Superoxide dismutase (SOD) and GPx activities were quantified using commercially available kits (Cayman chemicals) according to the manufacturer’s guidelines. The SOD assay is based on the formation of a formazan dye following exposure to superoxide anions (generated by hypoxanthine xanthine oxidase system) on a tetrazolium salt. The generated superoxide anions are dismutased by SOD leading to a reduction in the formation of formazan dye. The levels of formazan can be quantified at 450 nm was using the BMG Fluostar Optima multimode plate reader (NY, USA). GPx is based on the reduction of hydrogen peroxide by GPx present in the brain cell homogenate using GSH. Glutathione reductase (GR) and NADPH are then used to regenerate GSH. The assay quantified the levels of NADPH at 340 nm using the BMG Fluostar Optima multimode plate reader (NY, USA).
Measurement of Total Antioxidant Capacity
Total antioxidant capacity (TAC) was assessed in brain cell homogenates using the commercially available kit (Cayman total antioxidant assay) in accordance to the manufacturer’s guide using the BMG Fluostar Optima multimode plate reader (NY, USA).
Determination of Reduced GSH and Oxidized to Reduced GSH Ratio
The GSH assay method used in this study was adapted from Rahman et al. (2006) and all buffer formulations and assay reagent preparations were based on the original protocol. Briefly, neuronal homogenates extracts were diluted in Potassium phosphate-EDTA (KPE) buffer such that the sample values would fall within the standard curve range. Standards were prepared in matching dilutions of the lysis/extraction buffer in KPE buffer. Afterward, 20 μl of KPE buffer, serving as the blank, along with 20 μl of each of the standards, were dispensed into wells of a single row of a 96-well microplate. The assay’s designated standard range was between 0.1 through to 46.0 nM. The plate was loaded into BMG’s Fluostar Optima multimode plate reader (NY, USA), controlled by native software (version 2.10 R2). Both pumps were employed to dispense assay reagents, with Pump 1 being used to deliver a solution of equal parts GR and 5,5′-dithiobis-(2-nitrobenzoic acid; DTNB) in KPE. Pump 2 was used to deliver β-NADPH in KPE. One-hundred and twenty microliter of the combined GR and DTNB reagent and 60 μl of β-NADPH reagent was dispensed per well, into wells containing samples/standards. The rate of chromogenic TNB (5-thio-2-nitrobenzoic acid) formation from the non-chromogenic substrate DTNB [5,5′-dithio-bis (2-nitrobenzoic acid)] is directly proportional to the amount of GSH present and can be measured at 412 nm. Oxidized to reduced GSH ratio was determined using GSSG/GSH detection kit (Enzo Diagnostics, New York, NY, USA) as described in the manufacturer’s guidelines.
Quantification of a Panel of Inflammatory Cytokines
The levels of the anti-inflammatory cytokine IL-10 and the pro-inflammatory cytokines TNF-α, IL-6, and IL-1β in brain cell homogenates were quantified using specific ELISA kits (Ray Biotech, Peachtree Corners, GA, USA) according to the manufacturer’s instructions. These assays use biotinylated antibodies and streptavidin–HRP conjugate and a TMB (3,3′,5,5′-tetramethylbenzidine)-based detection system.
Measurement of Matric Metalloproteinase Activity (MMP-2 and MMP-9)
Matric metalloproteinase (MMP)-2 and MMP-9 activities were quantified using commercially available kits (AnaSpec) according to the manufacturer’s guidelines. The MMP-2 and MMP-9 activity assays use a 5-FAM (fluorophore) and QXL520™ (quencher) labeled FRET peptide substrate to quantify MMP-2 and MMP-9 activity in the sample. Cleavage of the FRET peptide by MMP-2 and MMP-9 alters the fluorescence of 5-FAM which can be quantified at excitation/emission of 490 nm/520 nm using the BMG Fluostar Optima multimode plate reader (NY, USA).
Bradford Protein Assay for the Quantification of Total Protein
LDH, caspase 3, SOD and GPx activities, the levels of DCFDA, 8-OH-dG, TBARS, DNPH, GSSG/GSH and several cytokines, and TAC were adjusted for variations in cell number using the Bradford protein assay described by Bradford (1976).
Data Analysis
The results obtained are presented as the means ± the standard error of measurement (SEM). One-way analysis of variance (ANOVA) and post hoc Tukey’s multiple comparison tests were used to determine statistical significance between treatment groups. Differences between treatment groups were considered significant if p was less than 0.05 (p < 0.05).
Results
Characterization of Recombinant Oligomeric Aβ40
Recombinant preparations of oligomeric Aβ40 were prepared and characterized using atomic force microscopy (AFM). Supplementary Figure S1A shows oligomeric Aβ40 as evidenced by a homogeneous population of spherical particles averaging 3–5 nm in size. No mature amyloid fibril structures were observed in these preparations.
We further characterized our oligomeric Aβ40 preparation using western blotting (Supplementary Figure S1B) using the monoclonal antibody 6E10 recognizing residues 1–17 of Aβ. Recombinant oligomeric Aβ40 preparations contained large amounts of low molecular weight species corresponding to monomer, dimer, trimer and tetramer, with a smear of Aβ species between 16 kDa and 50 kDa. Similar trends in migration patterns for oligomeric Aβ40 have been observed in other laboratories using the same Aβ aggregation method (Dahlgren et al., 2002). However, other laboratories have also reported detection of a larger molecular weight oligomeric Aβ species in their recombinant preparations that range from 40–98 kDa (Garzon and Fahnestock, 2007). Differences in these results may be due to variations in western blotting techniques used from laboratory to laboratory, for example, the specific composition of gels and the running buffer/s used.
Neurotoxic Effect of Oligomeric Aβ40 in Isolated Adult Astrocytes Can be Ameliorated Using GGC
Figure 1A shows the effect on neuronal viability, assessed by release of LDH into the media of human primary astrocytes following treatment with 10 nM oligomeric Aβ40 for 24 h. Oligomeric Aβ40 significantly decreased astrocyte cell viability. Treatment with GGC (200 μM) provided significant protection from exposure to oligomeric Aβ40. Treatment of astrocytes with GGC significantly reduced caspase 3 activity (Figure 1B), supporting the protective effects of GGC against oligomeric Aβ40-mediated apoptosis.
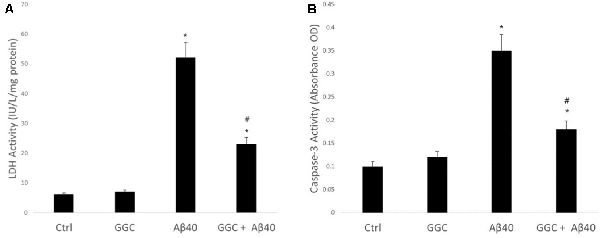
Figure 1. Neurotoxic effect of oligomeric Aβ40 in isolated adult astrocytes can be ameliorated using GGC. (A) Aβ40 significantly decreased astrocyte cell viability. Treatment with GGC (200 mM) provided significant protection from exposure to oligomeric Aβ40. (B) Treatment of astrocytes with GGC significantly reduced caspase 3 activity. Significance *p < 0.05 compared to control non-treated cells. #p < 0.05 compared to control cells treated with oligomeric Aβ40.
Oligomeric Aβ40 Induces Free Radical Damage to DNA, Lipids, and Protein Which Could be Attenuated Using GGC
To confirm that oligomeric Aβ40 is cytotoxic to astrocytes via induction of oxidative stress, we monitored the effect of oligomeric Aβ40 on free radical generation. Figure 2A demonstrates that incubation with oligomeric Aβ40 can increase reactive oxygen species production using the DCFDA Assay, and which was reduced by treatment with GGC. Co-treatment with GGC ameliorated oxidative DNA damage (Figure 2B), lipid peroxidation (Figure 2C), and protein carbonyl formation (Figure 2D).
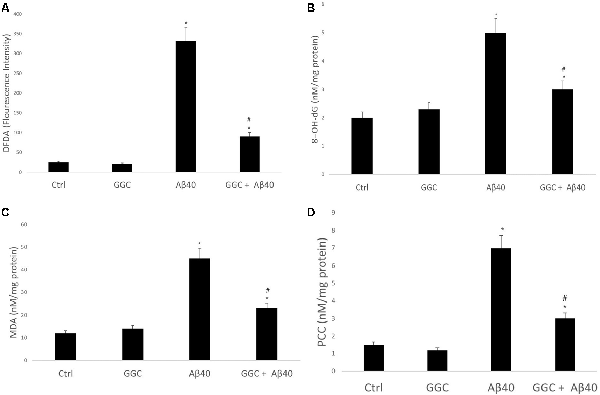
Figure 2. GGC attenuates oligomeric Aβ40-mediated oxidative stress production and oxidative damage to DNA, lipids, and protein in isolated adult astrocytes. (A) Oligomeric Aβ40 increased reactive oxygen species production using the DCFDA Assay, and which was reduced by treatment with GGC. Co-treatment with GGC also ameliorated (B) oxidative DNA damage, (C) lipid peroxidation, and (D) protein carbonyl formation. Significance *p < 0.05 compared to control non-treated cells. #p << 0.05 compared to control cells treated with oligomeric Aβ40.
Oligomeric Aβ40 Depletes Endogenous GSH Levels Which Can be Attenuated Using the GGC
Oligomeric Aβ40 decreased the activity of the antioxidant enzymes SOD (Figure 3A) and GPx (Figure 3B) compared to non-treated cells. Similarly, it reduced the levels of the GSH (Figure 3C) and the TAC (Figure 3D) in brain cell homogenates and increased the ratio GSSG/GSH (Figure 3E). Co-administration with GGC significantly increased the activity of SOD and GPx. Similarly, GGC increased the levels of GSH and TAC and significantly reduced the GSSG/GSH ratio. Therefore, GGC may attenuate oligomeric Aβ40-mediated disruption of endogenous antioxidant enzymes and replenish GSH levels in human brain cells.
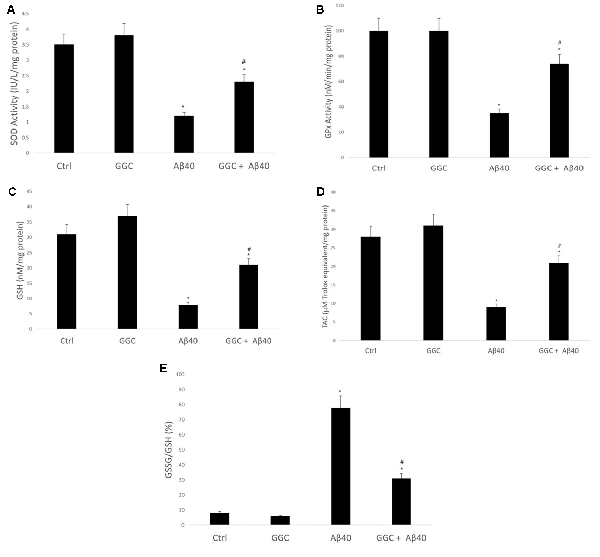
Figure 3. GGC increases (A) SOD and (B) GPx activities and (C) total antioxidant capacity, (D) attenuates oligomeric Aβ40-mediated GSH depletion, and (E) decreases the GSSG/GSH ratio in isolated adult astrocytes. Significance *p < 0.05 compared to control non-treated cells. #p < 0.05 compared to control cells treated with oligomeric Aβ40.
Oligomeric Aβ40 Induces Inflammation Which Is Modulated by GGC
Treating human brain cells with oligomeric Aβ40 significantly decreased the level of the anti-inflammatory cytokine IL-10 (Figure 4A) and increased the levels of the pro-inflammatory cytokines, TNF-α, IL-6 and IL-1β compared to non-treated cells (Figures 4B–D). GGC effectively modulated the observed oligomeric Aβ40-induced changes in the levels of inflammatory cytokines in vitro. We observed a significant upregulation in IL-10 levels and significant downregulation of TNF-α, IL-6, and IL-1β in GGC-treated cells, suggestive of the beneficial effects of GGC against oligomeric Aβ40-induced neuroinflammatory response.
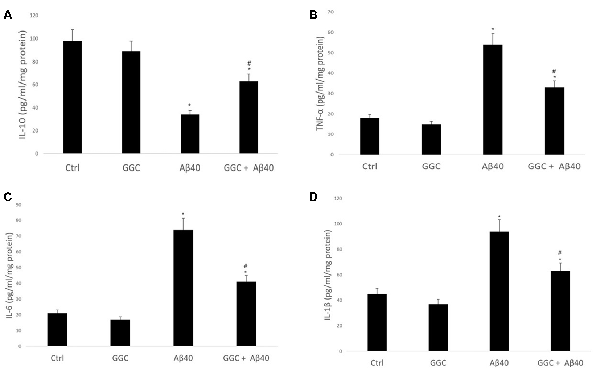
Figure 4. GGC increases (A) the levels of the anti-inflammatory cytokine IL-10 and decreases the levels of pro-inflammatory cytokines, (B) TNF-α, (C) IL-6 and (D) IL-β in isolated adult astrocytes. Significance *p < 0.05 compared to control non-treated cells. #p < 0.05 compared to control cells treated with oligomeric Aβ40.
GGC Supplementation Reduces Metalloproteinase Activity Induced by Oligomeric Aβ40
Treating human brain cells with oligomeric Aβ40 significantly increased the activity of MMP-2 (Figure 5A) and decreased the activity of MMP-9 compared to non-treated cells (Figure 5B). GGC attenuated the effect of oligomeric Aβ40-induced changes in MMP-2 and MMP-9 activities in vitro. We observed a significant decrease in MMP-2 activity and a significant increase in MMP-9 activity in GGC-treated cells, suggestive of the differential effect of GGC and increased GSH on MMPs.
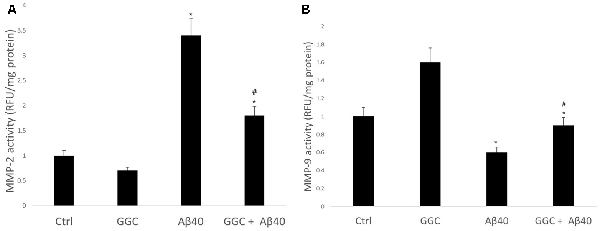
Figure 5. GGC reduces (A) MMP-2 and (B) MMP-9 activities and attenuates oligomeric Aβ40-induction of metalloproteinase activity in isolated adult astrocytes. Significance *p < 0.05 compared to control non-treated cells. #p << 0.05 compared to control cells treated with oligomeric Aβ40.
Discussion
GSH is the most potent antioxidant in the human body. It has multiple antioxidant actions which include direct conjugation with free radicals, enzyme-mediated neutralization of free radicals, and the regeneration of other eminent antioxidants such as Vitamin C. Owing to its potent biological availability, replenishing GSH levels following GSH depletion is an important therapeutic target. In this study, we attempted to evaluate whether the GSH precursor, GGC, could increase GSH levels in primary astrocytes and protect primary astrocytes against biomarkers of apoptosis and cell death, oxidative stress, and neuroinflammation when exposed to pathophysiological concentration of oligomeric Aβ40.
Aβ is a peptide that is formed following the proteolytic cleavage of the amyloid precursor protein (APP; Murphy and LeVine, 2010). It forms the principal component of extracellular amyloid plaques which are one of the main pathological hallmarks of AD. While several Aβ species with variable lengths (38–43 amino acids) have been identified, Aβ40 is thought to be most abundant (80%–90%) followed by Aβ42 (5%–10%; Selkoe, 2001). These peptides have been localized in amyloid plaques and can form oligomers and protofibrils, although Aβ42 is more hydrophobic and fibrillogenic (Selkoe, 2001). Post-mortem analysis of human brains from AD patients showed that that Aβ40 discriminated between AD patients and high pathology controls more readily than Aβ42 (Gao et al., 2010). Aβ40 oligomers have been shown to induce neurotoxicity and disrupt brain lipid bilayers while other amyloid species do not (Bode et al., 2019). Aβ40 is induced by an increase in intracellular Ca2+, and this has led to the hypothesis that the accumulation of intracellular Aβ may occur as an early event in the pathogenesis of AD (Itkin et al., 2011). Therefore, aggregation of intracellular Aβ may be an adaptive response to increases in [Ca2+]i and may enhance Ca2+-mediated excitotoxicity, which may induce progressive memory loss and cognitive deficits and enhance neuronal cell apoptosis. This can explain why ageing is a major risk factor for AD, since when calcium imbalance is more pronounced with advanced age.
Our data show that addition of Aβ40 oligomers increased cytotoxicity in astrocytes after a 24-h incubation. On the contrary, treatment of astrocytes with GGC attenuated cell viability and decreased oligomeric Aβ40-mediated oxidative stress and release of pro-inflammatory cytokines in vitro after 24 h. Our results suggest that GGC can enter astrocytes and increase GSH levels and reduce the GSSG/GSH ratio, which in turn, attenuates intracellular oxidative stress and protect astrocytes from oligomeric Aβ40-mediated oxidative stress and inflammatory response.
MMPs are important proteolytic enzymes necessary for the maintenance of the integrity of the extracellular matrix. Under normal physiological conditions, MMPs are secreted as inactive zymogens. However, under pathological conditions, activated MMPs are associated with increased degradation of ECM components and are involved in ECM re-modeling (Dollery et al., 1995). MMP2 has been reported to be a key player in the disruption of tight junction proteins and enhance blood brain barrier (BBB) permeability (Yang et al., 2007). Consistent with these findings, our data shows that Aβ40 oligomers can increase MMP-2 activity in stimulated astrocytes and correlated with increased oxidative stress, inflammation and reduced cell viability. MMP-9 has been shown to degrade fibrillar deposits and is co-localized with vascular amyloid deposits and neurofibrillary tangles (Alvarez-Sabín et al., 2004). However, since only the latent form of MMP-9 has been shown to accumulate in the AD brain, it has been hypothesized that impaired MMP-9 activity may lead to accumulation of abnormal Aβ peptides in amyloid plaques (Lim et al., 1996). With regards to this hypothesis, our data shows that Aβ40 oligomers reduce MMP-9 activity in human astrocytes and MMP-9 activity is increased following treatment with GGC. Our results are in line with previous findings showing the direct inhibitory effect of GSH on MMP-2 gelatinolytic capacity, and GSH-mediated increases in MMP-9 gelatinolytic activity in vitro (Bogani et al., 2007). The same study reported upregulation of MMP-9 transcription and activity following treatment with GSH (Bogani et al., 2007), and we observed a similar finding in GGC treated cells alone. Therefore, the differential effect of GGC on MMPs is likely to reduce ECM breakdown and promote degradation of Aβ peptide and may be dependent on GSH status.
We additionally found that GGC can increase intracellular GSH levels and lower the GSSG/GSH ratio in astrocytes. Alterations in the levels of either reduced (GSH) or oxidized (GSSG) GSH may affect the function of insulin-degrading enzyme (IDE) which is involved in mediating insulin degradation (Kulas et al., 2019). IDE is also a major regulator of Aβ peptides and reduced IDE activity can reduce Aβ degradation by more than 50% (Farris et al., 2003). One study demonstrated the inhibitory effects of GSSG on IDE activity, potentially due to antagonism of substrate binding. However, GSH enhanced IDE activity non-enzymatically by reducing exposed disulfide bonds and stimulating insulin breakdown (Cordes et al., 2011). Therefore, the intracellular GSH/GSSG ratio may affect the processing and degradation of both insulin and Aβ. Further studies are necessary to confirm whether GGC can increase IDE activity due to increased GSH levels, and may represent an additional mechanism to explain the potential neuroprotective effects of GGC in AD.
GGC is a dipeptide containing cysteine and glutamic acid. Apart from the metal chelating activity of cysteine residue that has been demonstrated in N-acetylcysteine (NAC; Sevgiler et al., 2011), glutamic acid has been reported to chelate redox-active metals. Therefore, the presence of both cysteine and glutamic acid residues in GGC may be likely responsible for its potent antioxidant and anti-inflammatory effects in brain cells against insult oligomeric Aβ40. In general, dipeptides have been considered unlikely to be effective as oral drug candidates, since they are prone to hydrolysis by digestive or serum proteases. However, GGC consists of a gamma-glutamyl bond which makes it resistant to hydrolysis by endoproteases (Anderson and Meister, 1983). As well, the importance of GGC as a cofactor for GPx to increase GSH levels is also another mechanism for the protective effects of GGC in human brain cells. The observed immunomodulatory effects of GGC on oligomeric Aβ40-induced inflammation may, thus, be also due to chelation of redox-active metals and additional antioxidant mechanisms (Figure 6).
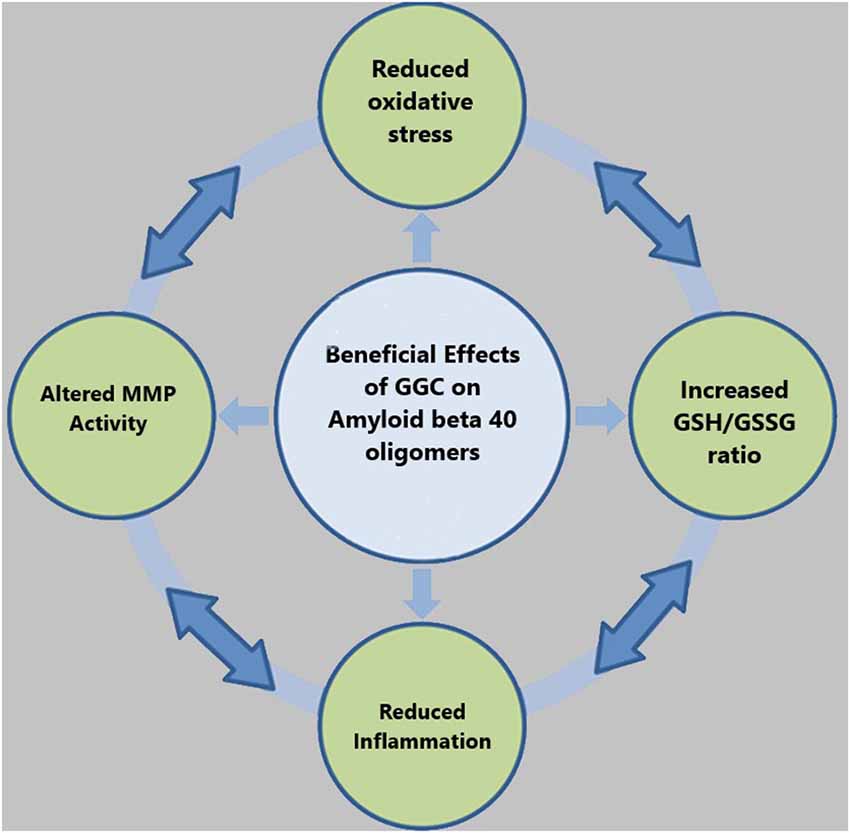
Figure 6. GGC can protect against oligomeric Aβ40 toxicity by reducing oxidative stress and increasing GSH levels.
The effects of GGC in human astrocytes is relevant for neuroprotection in AD and other neurodegenerative diseases since astrocytes represent the supporting cells in the brain and protect neurons against oxidative stress and enhance neuronal survival during cytotoxic conditions. It has been demonstrated that GSH depleted astrocytes lose their neuroprotective roles, leading to neuronal toxicity and a consequent reduction in cell viability (Pizzurro et al., 2014a,b). Depletion of GSH in astrocytes is also deleterious to astrocytes themselves (Im et al., 2006; Oz et al., 2006). Astrocytes are responsible for the clearance of debris in extracellular space and are required to maintain blood-brain barrier stability (He et al., 2008). The loss of astrocyte function can compromise the function and structure of neurons, culminating in neurodegeneration, and overall brain dysfunction (Schilling et al., 2006). Thus, maintenance of optimal GSH levels is necessary for neuroprotection against oxidative stress and neuroinflammation in astrocytes by maintaining a healthy environment in the CNS.
Strategies aimed at elevating GSH levels have been met with mixed success. The limiting factors include: (1) the inability of GSH to cross the cell membrane via direct absorption; (2) feedback inhibitory effects of GSH on glutamate cysteine ligase (GCL) activity (the first biosynthetic enzyme), which is regulated by the availability of cysteine (Jones, 2011); and (3) reduced activity of GCL with advanced age (Liu and Dickinson, 2003). Moreover, several cysteine-based antioxidant trials on AD patients have been unsuccessful (Berk et al., 2013). The therapeutic potential for GGC to increase GSH is related to the second GSH biosynthetic enzyme, glutathione synthetase (GS) which is not regulated by non-allosteric feedback inhibition by GSH. GS catalyzes the addition of glycine to GGC to produce GSH (Zarka and Bridge, 2017). This provides support for the benefits of GGC as an immediate precursor to GSH.
Direct delivery of GCC to the brain has been previously reported to protect against cellular depletion of GSH in the brain using the Buthionine sulfoximine (BSO) model of depletion and increase GSH levels in the pre-perturbed model (Pileblad and Magnusson, 1992; Dringen et al., 1997). It has been reported that the cytosolic concentrations of GGC are close to 7 μM, and passive uptake of intact GGC can be directed into cells. Reduced GSH levels due to impaired GCL activity is unlikely to be replenished by other GSH precursors such as NAC or other cysteine prodrugs. Supplementation with GGC is likely to increase GS activity and attenuate GSH depletion.
Previous studies such as those by Dringen et al. (1997) relied on limiting or sub-physiological conditions to examine the effects of GGC on increases in GS activity in astrocyte-rich primary cultures. However, these do not accurately represent healthy pre-pathological conditions and are unlikely to add support to the ability of GGC to bypass feedback inhibition of GCL. Le et al. (2011) showed that 2-h GGC treatment at 100 or 500 μM, following 4-h exposure to H2O2, could not significantly replenish GSH levels or increase GSH levels above homeostatic levels in murine neurons and astrocyte cell cultures. However, in that study, the neuronal and glial cells were co-treated with cytotoxic concentrations of H2O2, and therefore it is unlikely that GGC could produce beneficial effects on GSH in this condition. As well, that study could be improved by adding an additional control condition which was exposed to GGC in the absence of H2O2 insult.
Recently, our group showed that orally dosed GGC (2 and 4 g) is bioavailable and can increase intracellular GSH levels above homeostasis in lymphocytes of healthy, non-fasting human subjects with no adverse effects (Zarka and Bridge, 2017). This suggests that GGC may increase GSH levels in the clinic with potential as adjunct therapy for the treatment of disorders associated with acute and/or chronic GSH depletion. Previous preclinical studies have demonstrated little or no toxicity following administration of GGC (Joshi et al., 2007; Espinosa-Diez et al., 2015; Zhang et al., 2015; Ding et al., 2016; Henderson et al., 2016; Salama et al., 2016). The present study set out for the first time to evaluate GGC as a GSH-elevating strategy in primary human astrocytes, starting from homeostatic levels, with promising data.
In conclusion, this study has shown that GGC can decrease apoptosis, oxidative stress and inflammation in human astrocytes and protect astrocytes from oligomeric Aβ40-mediated cytotoxicity cellular GSH depletion. The beneficial effects of GGC on human brain cells may prevent against neurodegeneration by increasing the availability of GSH precursors, which would contribute to the neuroprotective effects of GGC in AD.
Data Availability
All datasets generated for this study are included in the manuscript and/or the Supplementary Files.
Ethics Statement
The approval for this study was obtained from the Human Research Ethics Committee of the University of New South Wales (human brain tissue reference number HC12563).
Author Contributions
NB, DC, and PS formulated the present hypothesis. NB was responsible for writing the report. NB, MZ, B-EJ, JW, TJ, and WB performed the experiments.
Funding
This work was supported by a National Health and Medical Research Council of Australia Capacity Building Grant and a UNSW Faculty of Medicine Research Grant. NB is the recipient of an Alzheimer’s Australia Viertel Foundation Postdoctoral Research Fellowship at the University of New South Wales.
Conflict of Interest Statement
The authors declare that the research was conducted in the absence of any commercial or financial relationships that could be construed as a potential conflict of interest.
Supplementary Material
The Supplementary Material for this article can be found online at: https://www.frontiersin.org/articles/10.3389/fnagi.2019.00177/full#supplementary-material
References
Alvarez-Sabín, J., Delgado, P., Abilleira, S., Molina, C. A., Arenillas, J., Ribó, M., et al. (2004). Temporal profile of matrix metalloproteinases and their inhibitors after spontaneous intracerebral hemorrhage: relationship to clinical and radiological outcome. Stroke 35, 1316–1322. doi: 10.1161/01.str.0000126827.69286.90
Anderson, M. E., and Meister, A. (1983). Transport and direct utilization of γ-glutamylcyst(e)ine for glutathione synthesis. Proc. Natl. Acad. Sci. U S A 80, 707–711. doi: 10.1073/pnas.80.3.707
Berk, M., Malhi, G. S., Gray, L. J., and Dean, O. M. (2013). The promise of N-acetylcysteine in neuropsychiatry. Trends Pharmacol. Sci. 34, 167–177. doi: 10.1016/j.tips.2013.01.001
Bode, D. C., Freeley, M., Nield, J., Palma, M., and Viles, J. H. (2019). Amyloid-β oligomers have a profound detergent-like effect on lipid membrane bilayers, imaged by atomic force and electron microscopy. J. Biol. Chem. 294, 7566–7572. doi: 10.1074/jbc.ac118.007195
Bogani, P., Canavesi, M., Hagen, T. M., Visioli, F., and Bellosta, S. (2007). Thiol supplementation inhibits metalloproteinase activity independent of glutathione status. Biochem. Biophys. Res. Commun. 363, 651–655. doi: 10.1016/j.bbrc.2007.09.018
Bradford, M. M. (1976). A rapid and sensitive method for quantitation of microgram quantities of protein utilising the principle of protein-dye binding. Anal. Biochem. 53, 452–458. doi: 10.1006/abio.1976.9999
Buege, J. A., and Aust, S. D. (1978). Microsomal lipid peroxidation. Meth. Enzymol. 52, 302–310. doi: 10.1016/S0076-6879(78)52032-6
Chiu, A. S., Gehringer, M. M., Braidy, N., Guillemin, G. J., Welch, J. H., and Neilan, B. A. (2013). Gliotoxicity of the cyanotoxin, β-methyl-amino-L-alanine (BMAA). Sci. Rep. 3:1482. doi: 10.1038/srep01482
Cordes, C. M., Bennett, R. G., Siford, G. L., and Hamel, F. G. (2011). Redox regulation of insulin degradation by insulin-degrading enzyme. PLoS One 6:e18138. doi: 10.1371/journal.pone.0018138
Dahlgren, K. N., Manelli, A. M., Stine, W. B. Jr., Baker, L. K., Krafft, G. A., and LaDu, M. J. (2002). Oligomeric and fibrillar species of amyloid-β peptides differentially affect neuronal viability. J. Biol. Chem. 277, 32046–32053. doi: 10.1074/jbc.M201750200
Ding, D., Jiang, H., Chen, G. D., Longo-Guess, C., Muthaiah, V. P., Tian, C., et al. (2016). N-acetyl-cysteine prevents age-related hearing loss and the progressive loss of inner hair cells in γ-glutamyl transferase 1 deficient mice. Aging 8, 730–750. doi: 10.18632/aging.100927
Dollery, C. M., McEwan, J. R., and Henney, A. M. (1995). Matrix metalloproteinases and cardiovascular disease. Circ. Res. 77, 863–868. doi: 10.1161/01.RES.77.5.863
Dringen, R., Kranich, O., Löschmann, P.-A., and Hamprecht, B. (1997). Use of dipeptides for the synthesis of glutathione by astroglia-rich primary cultures. J. Neurochem. 69, 868–874. doi: 10.1046/j.1471-4159.1997.69020868.x
Espinosa-Diez, C., Fierro-Fernández, M., Sánchez-Gómez, F., Rodríguez-Pascual, F., Alique, M., Ruiz-Ortega, M., et al. (2015). Targeting of γ-glutamyl-cysteine ligase by miR-433 reduces glutathione biosynthesis and promotes TGF-β-dependent fibrogenesis. Antioxid. Redox Signal. 23, 1092–1105. doi: 10.1089/ars.2014.6025
Farris, W., Mansourian, S., Chang, Y., Lindsley, L., Eckman, E. A., Frosch, M. P., et al. (2003). Insulin-degrading enzyme regulates the levels of insulin, amyloid β-protein and the β-amyloid precursor protein intracellular domain in vivo. Proc. Natl. Acad. Sci. U S A 100, 4162–4167. doi: 10.1073/pnas.0230450100
Ferreira, S. T., and Klein, W. L. (2011). The Aβ oligomer hypothesis for synapse failure and memory loss in Alzheimer’s disease. Neurobiol. Learn. Mem. 96, 529–543. doi: 10.1016/j.nlm.2011.08.003
Gao, C. M., Yam, A. Y., Wang, X. M., Magdangal, E., Salisbury, C., Peretz, D., et al. (2010). Aβ 40 oligomers identified as a potential biomarker for the diagnosis of Alzheimer’s disease. PLoS One 5:e15725. doi: 10.1371/journal.pone.0015725
Garzon, D. J., and Fahnestock, M. (2007). Oligomeric amyloid decreases basal levels of brain-derived neurotrophic factor (BDNF) mRNA via specific downregulation of BDNF transcripts IV and V in differentiated human neuroblastoma cells. J. Neurosci. 27, 2628–2635. doi: 10.1523/JNEUROSCI.5053-06.2007
Guillemin, G. J., Kerr, S. J., Smythe, G. A., Smith, D. G., Kapoor, V., Armati, P. J., et al. (2001). Kynurenine pathway metabolism in human astrocytes: a paradox for neuronal protection. J. Neurochem. 78, 842–853. doi: 10.1046/j.1471-4159.2001.00498.x
Harris, I. S., Treloar, A. E., Inoue, S., Sasaki, M., Gorrini, C., Lee, K. C., et al. (2015). Glutathione and thioredoxin antioxidant pathways synergize to drive cancer initiation and progression. Cancer Cell 27, 211–222. doi: 10.1016/j.ccell.2014.11.019
He, Z., Li, B., Yu, L., Liu, Q., Zhong, N., and Ran, P. (2008). Suppression of oxidant-induced glutathione synthesis by erythromycin in human bronchial epithelial cells. Respiration 75, 202–209. doi: 10.1159/000111569
Henderson, M., Rice, B., Sebastian, A., Sullivan, P. G., King, C., Robinson, R. A., et al. (2016). Neuroproteomic study of nitrated proteins in moderate traumatic brain injured rats treated with γ glutamyl cysteine ethyl ester administration post injury: insight into the role of glutathione elevation in nitrosative stress. Proteomics Clin. Appl. 10, 1218–1224. doi: 10.1002/prca.201600004
Ilyas, S., and Rehman, A. (2015). Oxidative stress, glutathione level and antioxidant response to heavy metals in multi-resistant pathogen, Candida tropicalis. Environ. Monit. Assess. 187:4115. doi: 10.1007/s10661-014-4115-9
Im, J. Y., Paik, S. G., and Han, P. L. (2006). Cadmium-induced astroglial death proceeds via glutathione depletion. J. Neurosci. Res. 83, 301–308. doi: 10.1002/jnr.20722
Itkin, A., Dupres, V., Dufrêne, Y. F., Bechinger, B., Ruysschaert, J. M., and Raussens, V. (2011). Calcium ions promote formation of amyloid β-peptide (1–40) oligomers causally implicated in neuronal toxicity of Alzheimer’s disease. PLoS One 6:e18250. doi: 10.1371/journal.pone.0018250
Izzo, N. J., Xu, J., Zeng, C., Kirk, M. J., Mozzoni, K., Silky, C., et al. (2014). Alzheimer’s therapeutics targeting amyloid β 1–42 oligomers II: Sigma-2/PGRMC1 receptors mediate Aβ 42 oligomer binding and synaptotoxicity. PLoS One 9:e111899. doi: 10.1371/journal.pone.0111899
Joshi, G., Hardas, S., Sultana, R., St Clair, D. K., Vore, M., and Butterfield, D. A. (2007). Glutathione elevation by γ-glutamyl cysteine ethyl ester as a potential therapeutic strategy for preventing oxidative stress in brain mediated by in vivo administration of adriamycin: implication for chemobrain. J. Neurosci. Res. 85, 497–503. doi: 10.1002/jnr.21158
Koh, J. Y., and Choi, D. W. (1987). Quantitative determination of glutamate mediated cortical neuronal injury in cell culture by lactate dehydrogenase efflux assay. J. Neurosci. Methods 20, 83–90. doi: 10.1016/0165-0270(87)90041-0
Kulas, J. A., Franklin, W. F., Smith, N. A., Manocha, G. D., Puig, K. L., Nagamoto-Combs, K., et al. (2019). Ablation of amyloid precursor protein increases insulin-degrading enzyme levels and activity in brain and peripheral tissues. Am. J. Physiol. Endocrinol. Metab. 316, E106–E120. doi: 10.1152/ajpendo.00279.2018
Lai, Y. C., Hickey, R. W., Chen, Y. M., Bayir, H., Sullivan, M. L., Chu, C. T., et al. (2008). Autophagy is increased after traumatic brain injury in mice and is partially inhibited by the antioxidant γ-glutamylcysteinyl ethyl ester. J. Cereb. Blood Flow Metab. 28, 540–550. doi: 10.1038/sj.jcbfm.9600551
Le, T. M., Jiang, H., Cunningham, G. R., Magarik, J. A., Barge, W. S., Cato, M. C., et al. (2011). γ-glutamylcysteine ameliorates oxidative injury in neurons and astrocytes in vitro and increases brain glutathione in vivo. Neurotoxicology 32, 518–525. doi: 10.1016/j.neuro.2010.11.008
Lesne, S. E. (2014). Toxic oligomer species of amyloid-β in Alzheimer’s disease, a timing issue. Swiss Med. Wkly. 144:w14021. doi: 10.4414/smw.2014.14021
Levine, R. L., Wehr, N., Williams, J. A., Stadtman, E. R., and Shacter, E. (2000). Determination of carbonyl groups in oxidized proteins. Methods Mol. Biol. 99, 15–24. doi: 10.1385/1-59259-054-3:15
Lim, G. P., Backstrom, J. R., Cullen, M. J., Miller, C. A., Atkinson, R. D., and Tökés, Z. A. (1996). Matrix metalloproteinases in the neocortex and spinal cord of amyotrophic lateral sclerosis patients. J. Neurochem. 67, 251–259. doi: 10.1046/j.1471-4159.1996.67010251.x
Liu, R. M., and Dickinson, D. A. (2003). Decreased synthetic capacity underlies the age-associated decline in glutathione content in Fisher 344 rats. Antioxid. Redox Signal. 5, 529–536. doi: 10.1089/152308603770310176
Murphy, M. P., and LeVine, H. III. (2010). Alzheimer’s disease and the amyloid-β peptide. J. Alzheimers Dis. 19, 311–323. doi: 10.3233/JAD-2010-1221
Nakamura, Y. K., Dubick, M. A., and Omaye, S. T. (2012). γ-Glutamylcysteine inhibits oxidative stress in human endothelial cells. Life Sci. 90, 116–121. doi: 10.1016/j.lfs.2011.10.016
Nguyen, P., and Derreumaux, P. (2014). Understanding amyloid fibril nucleation and Aβ oligomer/drug interactions from computer simulations. Acc. Chem. Res. 47, 603–611. doi: 10.1021/ar4002075
Nguyen, P. H., Li, M. S., and Derreumaux, P. (2014). Amyloid oligomer structure characterization from simulations: a general method. J. Chem. Phys. 140:094105. doi: 10.1063/1.4866902
Oz, H. S., Im, H. J., Chen, T. S., de Villiers, W. J., and McClain, C. J. (2006). Glutathione-enhancing agents protect against steatohepatitis in a dietary model. J. Biochem. Mol. Toxicol. 20, 39–47. doi: 10.1002/jbt.20109
Pileblad, E., and Magnusson, T. (1992). Increase in rat brain glutathione following intracerebroventricular administration of γ-glutamylcysteine. Biochem. Pharmacol. 44, 895–903. doi: 10.1016/0006-2952(92)90121-x
Pizzurro, D. M., Dao, K., and Costa, L. G. (2014a). Astrocytes protect against diazinon- and diazoxon-induced inhibition of neurite outgrowth by regulating neuronal glutathione. Toxicology 318, 59–68. doi: 10.1016/j.tox.2014.01.010
Pizzurro, D. M., Dao, K., and Costa, L. G. (2014b). Diazinon and diazoxon impair the ability of astrocytes to foster neurite outgrowth in primary hippocampal neurons. Toxicol. Appl. Pharmacol. 274, 372–382. doi: 10.1016/j.taap.2013.11.023
Poduslo, J. F., and Howell, K. G. (2015). Unique molecular signatures of Alzheimer’s disease amyloid β peptide mutations and deletion during aggregate/oligomer/fibril formation. J. Neurosci. Res. 93, 410–423. doi: 10.1002/jnr.23507
Porquet, D., Andrés-Benito, P., Griñán-Ferré, C., Camins, A., Ferrer, I., Canudas, A. M., et al. (2015). Amyloid and tau pathology of familial Alzheimer’s disease APP/PS1 mouse model in a senescence phenotype background (SAMP8). Age 37:9747. doi: 10.1007/s11357-015-9747-3
Quintana-Cabrera, R., Fernandez-Fernandez, S., Bobo-Jimenez, V., Escobar, J., Sastre, J., Almeida, A., et al. (2012). γ-Glutamylcysteine detoxifies reactive oxygen species by acting as glutathione peroxidase-1 cofactor. Nat. Commun. 3:718. doi: 10.1038/ncomms1722
Rahman, I., Kode, A., and Biswas, S. K. (2006). Assay for quantitative determination of glutathione and glutathione disulfide levels using enzymatic recycling method. Nat. Protoc. 1, 3159–3165. doi: 10.1038/nprot.2006.378
Romero-Haro, A. A., and Alonso-Alvarez, C. (2015). The level of an intracellular antioxidant during development determines the adult phenotype in a bird species: a potential organizer role for glutathione. Am. Nat. 185, 390–405. doi: 10.1086/679613
Salama, S. A., Arab, H. H., Maghrabi, I. A., Hassan, M. H., and AlSaeed, M. S. (2016). γ-glutamyl cysteine attenuates tissue damage and enhances tissue regeneration in a rat model of lead-induced nephrotoxicity. Biol. Trace Elem. Res. 173, 96–107. doi: 10.1007/s12011-016-0624-4
Schilling, S., Lauber, T., Schaupp, M., Manhart, S., Scheel, E., Böhm, G., et al. (2006). On the seeding and oligomerization of pGlu-amyloid peptides (in vitro). Biochemistry 45, 12393–12399. doi: 10.1021/bi0612667
Selkoe, D. J. (2001). Alzheimer’s disease: genes, proteins and therapy. Physiol. Rev. 81, 741–766. doi: 10.1152/physrev.2001.81.2.741
Sevgiler, Y., Karaytug, S., and Karayakar, F. (2011). Antioxidative effects of N-acetylcysteine, lipoic acid, taurine and curcumin in the muscle of Cyprinus carpio L. exposed to cadmium. Arh. Hig. Rada Toksikol. 62, 1–9. doi: 10.2478/10004-1254-62-2011-2082
Streltsov, V. A., Varghese, J. N., Masters, C. L., and Nuttall, S. D. (2011). Crystal structure of the amyloid-β p3 fragment provides a model for oligomer formation in Alzheimer’s disease. J. Neurosci. 31, 1419–1426. doi: 10.1523/JNEUROSCI.4259-10.2011
Wang, Q., Ning, L., Niu, Y., Liu, H., and Yao, X. (2015). Molecular mechanism of the inhibition and remodeling of human islet amyloid polypeptide (hIAPP1–37) oligomer by resveratrol from molecular dynamics simulation. J. Phys. Chem. B 119, 15–24. doi: 10.1021/jp507529f
Wang, X., Zhu, M., Hjorth, E., Cortés-Toro, V., Eyjolfsdottir, H., Graff, C., et al. (2015). Resolution of inflammation is altered in Alzheimer’s disease. Alzheimers Dement. 11, 40.e2–50.e2. doi: 10.1016/j.jalz.2013.12.024
Wilcox, K. C., Lacor, P. N., Pitt, J., and Klein, W. L. (2011). Aβ oligomer-induced synapse degeneration in Alzheimer’s disease. Cell. Mol. Neurobiol. 31, 939–948. doi: 10.1007/s10571-011-9691-4
Wilkins, H. M., Carl, S. M., Weber, S. G., Ramanujan, S. A., Festoff, B. W., Linseman, D. A., et al. (2015). Mitochondrial lysates induce inflammation and Alzheimer’s disease-relevant changes in microglial and neuronal cells. J. Alzheimers Dis. 45, 305–318. doi: 10.3233/JAD-142334
Xu, P. X., Wang, S. W., Yu, X. L., Su, Y. J., Wang, T., Zhou, W. W., et al. (2014). Rutin improves spatial memory in Alzheimer’s disease transgenic mice by reducing Aβ oligomer level and attenuating oxidative stress and neuroinflammation. Behav. Brain Res. 264, 173–180. doi: 10.1016/j.bbr.2014.02.002
Yang, Y., Estrada, E. Y., Thompson, J. F., Liu, W., and Rosenberg, G. A. (2007). Matrix metalloproteinase-mediated disruption of tight junction proteins in cerebral vessels is reversed by synthetic matrix metalloproteinase inhibitor in focal ischemia in rat. J. Cereb. Blood Flow Metab. 27, 697–709. doi: 10.1038/sj.jcbfm.9600375
Zarka, M. H., and Bridge, W. J. (2017). Oral administration of γ-glutamylcysteine increases intracellular glutathione levels above homeostasis in a randomised human trial pilot study. Redox Biol. 11, 631–636. doi: 10.1016/j.redox.2017.01.014
Zhang, Q., Guo, S., Zhang, X., Tang, S., Wang, L., Han, X., et al. (2015). Amyloid β oligomer-induced ERK1/2-dependent serine 636/639 phosphorylation of insulin receptor substrate-1 impairs insulin signaling and glycogen storage in human astrocytes. Gene 561, 76–81. doi: 10.1016/j.gene.2015.02.011
Zhang, H., Kovacs-Nolan, J., Kodera, T., Eto, Y., and Mine, Y. (2015). γ-Glutamyl cysteine and γ-glutamyl valine inhibit TNF-α signaling in intestinal epithelial cells and reduce inflammation in a mouse model of colitis via allosteric activation of the calcium-sensing receptor. Biochim. Biophys. Acta 1852, 792–804. doi: 10.1016/j.bbadis.2014.12.023
Keywords: Alzheimer’s disease, glutathione, oxidative stress, antioxidants, dementia
Citation: Braidy N, Zarka M, Jugder B-E, Welch J, Jayasena T, Chan DKY, Sachdev P and Bridge W (2019) The Precursor to Glutathione (GSH), γ-Glutamylcysteine (GGC), Can Ameliorate Oxidative Damage and Neuroinflammation Induced by Aβ40 Oligomers in Human Astrocytes. Front. Aging Neurosci. 11:177. doi: 10.3389/fnagi.2019.00177
Received: 28 February 2019; Accepted: 02 July 2019;
Published: 08 August 2019.
Edited by:
Anne Eckert, University Psychiatric Clinic Basel, SwitzerlandReviewed by:
Patrizia Giannoni, University of Nîmes, FranceAmandine Grimm, University of Basel, Switzerland
Copyright © 2019 Braidy, Zarka, Jugder, Welch, Jayasena, Chan, Sachdev and Bridge. This is an open-access article distributed under the terms of the Creative Commons Attribution License (CC BY). The use, distribution or reproduction in other forums is permitted, provided the original author(s) and the copyright owner(s) are credited and that the original publication in this journal is cited, in accordance with accepted academic practice. No use, distribution or reproduction is permitted which does not comply with these terms.
*Correspondence: Nady Braidy, n.braidy@unsw.edu.au