Group I mGluR-Mediated Activation of Martinotti Cells Inhibits Local Cortical Circuitry in Human Cortex
- 1Department of Integrative Neurophysiology, Center for Neurogenomics and Cognitive Research, VU University Amsterdam, Amsterdam, Netherlands
- 2Department of Neuropathology, Amsterdam Neuroscience, Amsterdam UMC, University of Amsterdam, Amsterdam, Netherlands
- 3Stichting Epilepsie Instellingen Nederland, Heemstede, Netherlands
- 4Department of Neurosurgery, VU University Medical Center, Amsterdam, Netherlands
Group I metabotropic glutamate receptors (mGluRs) mediate a range of signaling and plasticity processes in the brain and are of growing importance as potential therapeutic targets in clinical trials for neuropsychiatric and neurodevelopmental disorders (NDDs). Fundamental knowledge regarding the functional effects of mGluRs upon pyramidal neurons and interneurons is derived largely from rodent brain, and their effects upon human neurons are predominantly untested. We therefore addressed how group I mGluRs affect microcircuits in human neocortex. We show that activation of group I mGluRs elicits action potential firing in Martinotti cells, which leads to increased synaptic inhibition onto neighboring neurons. Some other interneurons, including fast-spiking interneurons, are depolarized but do not fire action potentials in response to group I mGluR activation. Furthermore, we confirm the existence of group I mGluR-mediated depression of excitatory synapses in human pyramidal neurons. We propose that the strong increase in inhibition and depression of excitatory synapses onto layer 2/3 pyramidal neurons upon group I mGluR activation likely results in a shift in the balance between excitation and inhibition in the human cortical network.
Introduction
Metabotropic glutamate receptors (mGluRs) form a diverse set of G-protein-coupled receptors that are divided into three groups, based on sequence homology, pharmacological properties, and signal transduction (Nakanishi, 1992). The most studied of the three is group I, which comprises mGluR1 and mGluR5, both of which act through Gq proteins. Group I mGluRs are located perisynaptically and are involved in a range of signaling and synaptic plasticity processes (Luján et al., 1996). They are particularly known for inducing a form of long-term depression (LTD) at glutamatergic synapses, which can be mediated by either mGluR1 or mGluR5, depending on brain region, postsynaptic cell type, and specific pathways in which the synapse is involved (Lüscher and Huber, 2010; Sherman, 2014). In addition to their role in LTD, group I mGluR activation potentiates NMDA-receptor-mediated currents (Wang and Daw, 1996; Mannaioni et al., 2001), and can depolarize several types of neurons through activation of a Ca2+-dependent cation conductance and decrease of resting K+ current (Baskys et al., 1990; Crepel et al., 1994; Guérineau et al., 1994, 1995).
While most studies of mGluR function, as well as its therapeutic effects, have centered upon excitatory signaling and pyramidal neurons (Chuang et al., 2000; Bandrowski et al., 2003), mGluRs can induce plasticity at GABAergic synapses through a variety of mechanisms (Galante and Diana, 2004; Valentinova and Mameli, 2016). Furthermore, group I mGluRs are expressed in several types of interneurons in both mouse and human brain (López-Bendito et al., 2002; Boer et al., 2010). Consequently, group I mGluRs depolarize specific types of interneurons (McBain et al., 1994; Van Hooft et al., 2000) and increase synaptic inhibition in rodent brain (Zhou and Hablitz, 1997; Mannaioni et al., 2001). Activation of group I mGluRs can also synchronize network activity by eliciting synchronous spiking in low-threshold spiking interneurons (Beierlein et al., 2000), which include Martinotti cells.
In recent years, group I mGluRs, and mGluR5 in particular, have become of increasing interest as potential therapeutic targets in neuropsychiatric and neurodevelopmental disorders (NDDs) (Barnes et al., 2015), including schizophrenia (Conn et al., 2009), and autistic spectrum disorders (ASDs) (Aguilar-Valles et al., 2015; Wenger et al., 2016). For example, dysregulated group I mGluR-mediated plasticity was proposed to underlie the NDD pathophysiology of fragile X syndrome (FXS) (Bear et al., 2004), since group I mGluR-mediated LTD is exaggerated in hippocampal pyramidal neurons in the FXS mouse model (Huber et al., 2002). Strikingly, mGluR-elicited spiking in Martinotti cells has been shown to be reduced in the Fmr1-KO mouse model for FXS (Paluszkiewicz et al., 2011b). These findings led to clinical trials targeting mGluR5 in adults with FXS (Berry-Kravis, 2014; Jacquemont et al., 2014). Unfortunately, these trials have thus far been unsuccessful, with reasons given ranging from patient age, and drug dosage level, to incomplete knowledge at a brain circuit rather than at a single cell level (Mullard, 2015; Berry-Kravis et al., 2016, 2018). Furthermore, rodent data on mGluR function has rarely been validated in the human brain. New work has started to confirm the existence of some of the effects of mGluRs in human cortex. The influence of group II mGluRs on glutamatergic transmission has recently been shown to be the same in human cortex as it is in rodents (Bocchio et al., 2019), as has mGluR-mediated LTD in fast-spiking interneurons (Szegedi et al., 2016). Given the importance of validation in humans of the basic mechanisms underlying therapies for cognitive disorders, we sought to confirm the effects of group I mGluRs in human cortex. Accordingly, we report that group I mGluRs increase inhibitory transmission onto several types of neurons in human cortex and identify depolarization of Martinotti cells as a potential mechanism. Furthermore, we confirm the existence of mGluR-mediated synaptic depression in human pyramidal neurons. Taken together, these results provide an essential step forward in understanding human mGluR-mediated signaling that may inform our understanding of their therapeutic actions in future clinical trials.
Materials and Methods
Acute Slice Preparation From Human Cortex
All procedures carried out involving patient tissue were approved by the VU University Medical Center Medical Ethical Committee and in accordance with the Dutch law and the declaration of Helsinki. All 40 patients provided written informed consent. The majority of cortical samples were taken from patients that suffered from drug-resistant epilepsy, in most cases due to hippocampal sclerosis (Table 1). During surgery, non-pathological tissue showing no structural abnormalities was resected from anterior and medial temporal cortex (Goriounova et al., 2018) (in this paper Figure 2 shows the exact location and extent of the resection and what tissue block was taken to the lab) in order to reach the pathological focus. Tissue was immediately stored and transported to the physiology laboratory in ice-cold slicing solution containing (in mM) 110 Choline chloride, 26 NaHCO3, 10 D-glucose, 11.6 sodium ascorbate, 7 MgCl2, 3.1 sodium pyruvate, 2.5 KCl, 1.25 NaH2PO4, and 0.5 CaCl2. 350–450 μm thick slices were prepared in the same, carbogenated, solution and were left to recover in aCSF containing (in mM) 125 NaCl, 26 NaHCO3, 10 D-glucose, 3 KCl, 2 CaCl2, 1 MgCl2, and 1.25 NaH2PO4 at 35°C, and then for at least 60 min at room temperature. aCSF in both recovery and recording chambers was continuously bubbled with a mixture of 95% O2 and 5% CO2.
Electrophysiology
Slices in the recording chamber were perfused with aCSF heated to 31–33°C. Recordings were made using borosilicate (GC150-10, Harvard Apparatus, Holliston, MA, United States) glass pipettes with a resistance of 3 – 5 MΩ, pulled on a horizontal puller (P-87, Sutter Instrument Co., Novato, CA, United States). Signals were amplified (Multiclamp 700B, Molecular Devices), digitized (Digidata 1440A, Molecular Devices), and recorded in pCLAMP 10 (Molecular Devices, Sunnyvale, CA, United States). Access resistance was monitored before, during, and after recording. Cells were discarded if the access resistance deviated more than 25% from its value at the start of recording, or if it exceeded 20 MΩ. For current-clamp recordings and voltage-clamp recordings of excitatory postsynaptic current (EPSCs), pipettes contained intracellular solution consisting of (in mM) 148 K-gluconate, 1 KCl, 10 Hepes, 4 Mg-ATP, 4 K2-phosphocreatine, 0.4 GTP and 0.5% biocytin, adjusted with KOH to pH 7.3 (± 290 mOsm). All EPSC recordings except those shown in Figure 5H were performed in the presence of 10 μM Gabazine (Tocris Bioscience, Bristol, United Kingdom). To measure evoked EPSCs (eEPSCs), a pipette filled with aCSF was placed on a stimulation electrode and positioned within 100 μm from the recorded neuron. Current pulses were applied using an ISO-Flex stimulation box, and timed by a Master 9 (A.M.P.I., Jerusalem, Israel). The stimulation pipette was positioned so that a clear postsynaptic response could be observed with a clear separation from the stimulation artifact (Figure 1B). The stimulus intensity was set to evoke a half-maximal current. Pulses were applied every 15 s and a baseline of at least 5 min was recorded after the eEPSC amplitude stabilized. After recording a stable baseline, 25 μM DHPG was perfused into the recording chamber for 5 min. After a 5-min washout period, eEPSCs were measured every 15 s for up to 40 min and responses averaged per 10-min bins. In a subset of experiments, shown in Figure 5H, eEPSCs were recorded during DHPG application. These recordings were performed in the absence of GABAzine, so as not to elicit network events. Spontaneous inhibitory postsynaptic currents (sIPSCs) were measured using an intracellular solution containing (in mM) 70 K-gluconate, 70 KCl, 10 Hepes, 4 Mg-ATP, 4 K2-phosphocreatine, 0.4 GTP and 0.5% biocytin, adjusted with KOH to pH 7.3 (±290 mOsm). IPSC recordings were performed in the presence of 10 μM CNQX (Abcam, Cambridge, United Kingdom) and 50 μM D-APV (Abcam). sIPSCs were recorded from pyramidal neurons located in L2/3 and interneurons located in layer 1.
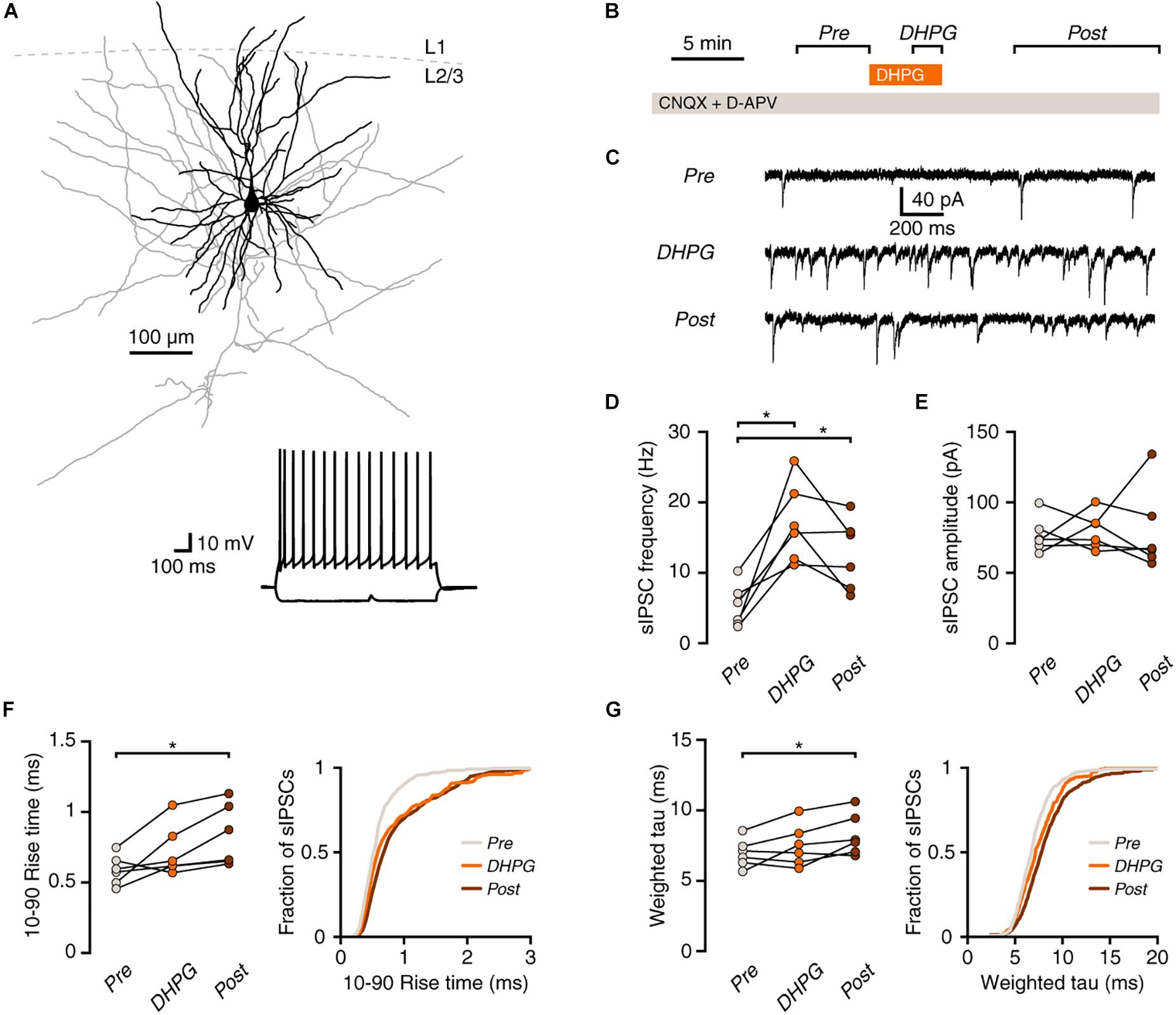
Figure 1. mGluR activation increases synaptic inhibition onto human pyramidal neurons. (A) Example morphological reconstruction of a human pyramidal neuron (350 μm slice; dendrites in black, axon in gray). Inset: electrophysiological response to negative and positive current steps. (B) Experimental protocol. (C) Example traces showing IPSCs before (Pre), during (DHPG) and after (Post) application of DHPG. (D) DHPG elicited a lasting increase in sIPSC frequency in pyramidal neurons (repeated-measures ANOVA: F(2,10) = 16.84, p = 0.003; Tukey’s post hoc test: Pre vs. DHPG *p < 0.05, DHPG vs. Post ns, Pre vs. Post *p < 0.05). (E) sIPSC amplitude was not significantly affected by DHPG [F(2,10) = 0.07, p = 0.929]. (F) Average rise time of sIPSCs in pyramidal neurons was slower after DHPG application [F(2,10) = 7.22, p = 0.011; Tukey’s post hoc test: Pre vs. DHPG ns, DHPG vs. Post ns, Pre vs. Post *p < 0.05]. Right panel, cumulative probability distribution of sIPSC rise times, average of probability distributions calculated for each cell. (G) Decay time of sIPSCs was slower after DHPG application F(2,10) = 5.82, p = 0.021; Tukey’s post hoc test: Pre vs. DHPG ns, DHPG vs. Post ns, Pre vs. Post *p < 0.05]. Right panel, cumulative probability distribution of sIPSC decay times, average of probability distributions calculated for each cell.
Post hoc Morphological Assessment
Slices containing biocytin-filled cells were fixed in 4% paraformaldehyde in PBS for 24 – 48 h at 4°C. Slices were washed at least 3 × 10 min in PBS, and incubated in PBS containing 0.5% Triton X-100 and 1:500 Alexa 488-streptavidin (Invitrogen, Waltham, MA, United States) on a shaker at approximately 18–23°C (room temperature) for 48 h. Slices were then further washed at least 3 × 10 min in PBS and mounted on glass slides in mounting medium containing 0.1M Tris pH 8.5, 25% glycerol, 10% w/v Mowiol (Sigma-Aldrich). The morphology of recorded cells was checked for identification of their cell type (see Ascoli et al., 2008; Tremblay et al., 2016). Selected cells were imaged using an A1 confocal microscope (Nikon, Tokyo, Japan) using a 10×, NA 0.45 objective, scanned at 0.5 μm × 0.5 μm × 1.0 μm (xyz) resolution. Cellular morphology was reconstructed using NeuroMantic software (Myatt et al., 2012).
Immunohistochemistry
To assess the expression of mGluR1α in somatostatin-positive neurons, temporal cortical tissue was used from patients undergoing surgery for mesial temporal lobe epilepsy (MTLE; 1 male, 2 female, 25 – 47 years) and three autopsy controls, displaying a normal cortical structure for the corresponding age and without any significant brain pathology (1 male, 2 female, 25 – 49 years). The control cases included in this study were selected from the databases of the Department of Neuropathology of the Academic Medical Center, University of Amsterdam, Amsterdam, Netherlands. Tissue was obtained during autopsy and used in accordance with the Declaration of Helsinki and the AMC Research Code provided by the Medical Ethics Committee. All autopsies were performed within 24 h after death. Tissue was fixed in 10% buffered formalin and embedded in paraffin. 6 μm sections were incubated overnight at 4°C in primary antibody solution (mGluR1α, 1:100, monoclonal mouse SC-55565, Santa Cruz Biotechnology, Santa Cruz, CA; Somatostatin, 1:300, polyclonal rabbit, AB1595, Chemicon, Temecula, CA, United States). Sections were then incubated for 2 h at room temperature with Alexa Fluor 568-conjugated anti-rabbit and Alexa Fluor 488 anti-mouse immunoglobulin G (IgG, 1:200, Thermo Fisher Scientific, Waltham, MA, United States). Finally, sections were analyzed using a laser scanning confocal microscope (Leica TCS Sp2, Wetzlar, Germany).
Quantification of GRM1 and GRM5 Expression
GRM1 and GRM5 expression levels were quantified using publicly available Allen Institute for Brain Science (AIBS) database on human single-cell transcriptomics at http://celltypes.brain-map.org/, where the detailed methods can be found. The transcriptomic data from Allen Institute comes from human temporal cortical tissue, postmortem or surgically resected, sectioned and dissected per layer (Hodge et al., 2018). The methods include single nuclei fluorescence-activated cells sorting (FACS) isolation based on DAPI and neuronal nuclei staining (NeuN), followed by Smart-seq v4 based library preparation and single-cell deep (2.5 million reads/cell) RNA-Seq.
The data on single nucleus GRM1 and GRM5 mRNA expression in transcriptomic types from AIBS database were pooled to represent higher-order hierarchical clusters (SST, PVALB, PAX6/LAMP5, and excitatory types) from selected cortical layers of interest. Violin plots were made using custom-made Matlab scripts (Mathworks, Natick, MA, United States), the plots represent distribution of mRNA expression on a log scale with counts per million (CPM) value of 4000.
Analysis and Statistics
Electrophysiological data were analyzed using custom scripts in Matlab. All data are represented as mean ± standard error of the mean (SEM). Normal distribution of the data was tested using Shapiro-Wilk tests. Appropriate statistical tests were performed in Prism 7 (Graphpad, La Jolla, CA, United States), and are mentioned in the figure legends.
Results
Group I mGluR Activation Increases Inhibition Onto Human Pyramidal Neurons
Activation of group I mGluRs increases spontaneous inhibition in rodent cortex (Paluszkiewicz et al., 2011b). To test whether this holds true in human cortex, we recorded spontaneous inhibitory postsynaptic currents (sIPSCs) in pyramidal neurons in layer 2/3 of surgically resected human neocortex and activated group I mGluRs by a 5-min bath application of the agonist (S)-3,5-Dihydroxyphenylglycine (DHPG; Figures 1A–C). Application of DHPG led to an increase in the frequency of sIPSCs in pyramidal neurons that lasted after the agonist washout from the bath (Figure 1D). Interestingly, while the amplitude of inhibitory events was unaffected (Figure 1E), both the rise and decay times were increased after washout of the agonist (Figures 1F,G).
Group I mGluRs Strongly Activate Martinotti Cells in Human Cortex
A potential cause of the slower kinetics would be a change in membrane time constant caused by DHPG. However, the membrane time constant after completion of the experiment did not differ from that measured before the start of the experiment [before: 17.7 ± 2.2 ms, after: 15.3 ± 3.0 ms, paired t(5) = 0.931, p = 0.395]. As inputs that are further away from the soma appear to have slower kinetics due to the filtering properties of dendrites (Magee, 2000), we hypothesized that the slower synaptic inputs elicited by DHPG might be onto distal dendrites and were therefore likely coming from Martinotti cells (MCs). We performed current-clamp recordings of putative MCs in layer 2/3 to assess whether group I mGluR activation would elicit a change in membrane potential. Putative MCs were identified by an ovoid-shaped cell body and bitufted proximal dendritic morphology in the DIC microscopic image and by a rebound action potential following a depolarizing current step. Post hoc reconstruction of the morphology of these cells showed that the axon of putative MCs branched out and terminated in layer 1 (Figure 2A; Obermayer et al., 2018). Application of DHPG caused a depolarization of 7.7 ± 1.4 mV before the start of action potential firing (Figure 2B) and led to action potential firing in 6 out of 7 MCs (Figure 2C). In one experiment, a connected pair of MC and pyramidal neuron was recorded (Figure 2D). Upon DHPG application, the MC started firing action potentials, and the pyramidal neuron received an increased number of inhibitory postsynaptic potentials (IPSPs, Figure 2E). Analysis of the pyramidal neuron membrane potential following 50 MC action potentials showed distinct IPSPs (Figure 2F, left panel). Performing the same analysis on randomly generated time points did not show a similar peak (Figure 2F, right panel; p < 0.001). The latency between the peak of the MC action potential and the onset of IPSPs in the pyramidal neuron was 1.75 ms, with a jitter of 396 μs. Thus, action potentials elicited by DHPG in the presynaptic MC generate time-locked inhibitory responses in postsynaptic pyramidal neurons.
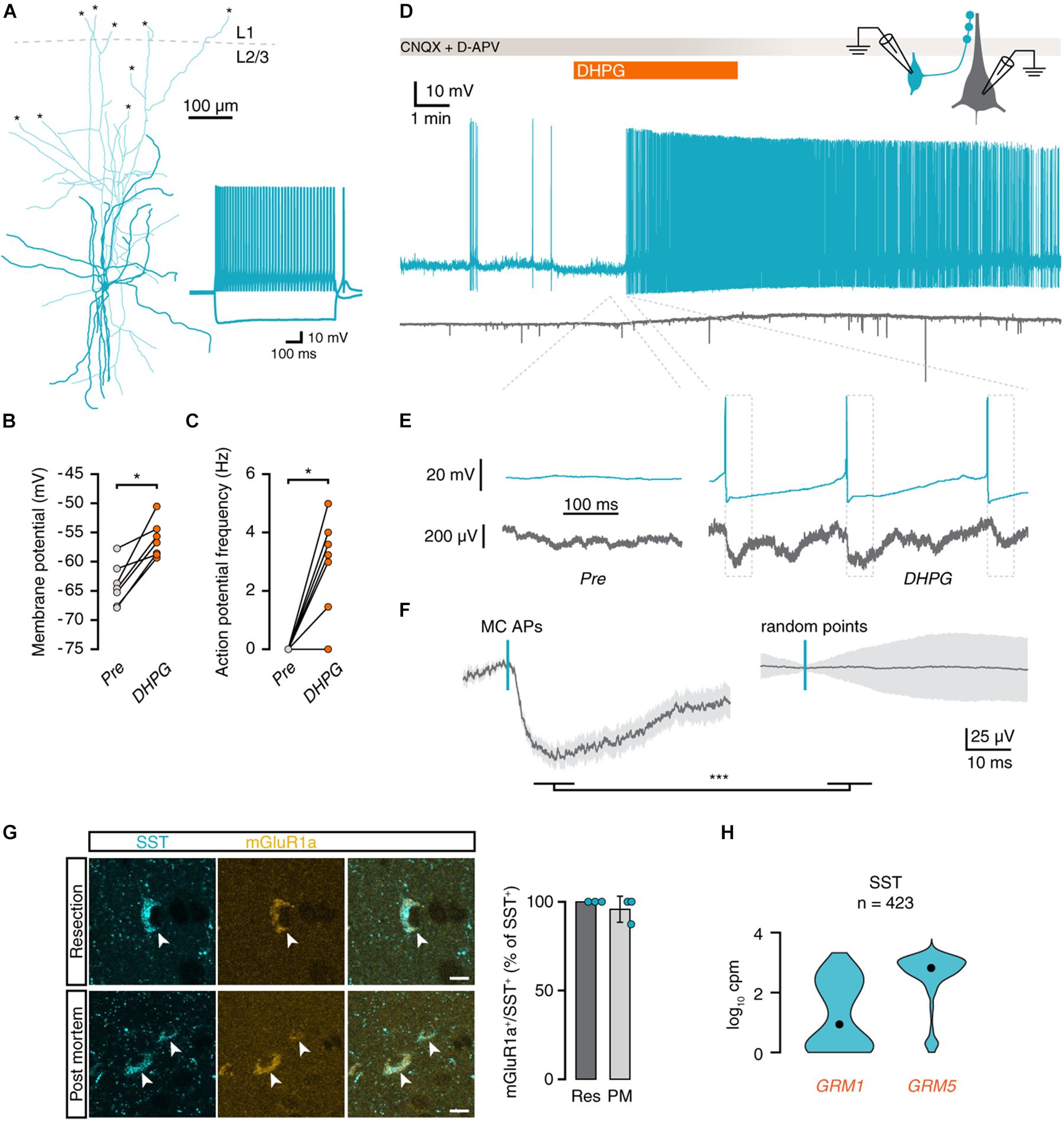
Figure 2. mGluR activation depolarizes Martinotti cells and leads to action potential firing. (A) Morphological reconstruction of an MC in human cortex (350 μm slice). Morphology was recovered post hoc for 5 out of 7 recorded cells. Asterisks denote places where a neurite was cut during slice preparation. Inset: electrophysiological response to negative and positive current steps. (B) Membrane potentials of MCs are depolarized by DHPG (Wilcoxon matched-pairs signed rank test, n = 7, W = 28, p = 0.016). (C) DHPG induced an increase in action potential frequency (Wilcoxon matched-pairs signed rank test, n = 7, W = 21, p = 0.031). (D) Voltage traces of a connected pair consisting of an MC (teal) and a pyramidal neuron (gray). Application of DHPG (orange) induces sustained action potential spiking in the MC. (E) Voltage traces of MC and pyramidal neuron before and during application of DHPG (dashed lines indicate corresponding area of the trace in D). Dashed boxes denote the area used for the analysis in f. (F) Average pyramidal neuron voltage trace (left panel, 50 events, light gray area shows SEM) around MC action potentials (left panel, teal dash) shows an inhibitory response that is absent in voltage traces centered on random time points during the same period (right panel; Mann-Whitney U = 418, p < 0.001). (G) Immunohistochemical staining for somatostatin (cyan) and mGluR1a (yellow) shows that mGluR1a is present in SST+ interneurons (arrowheads) in both resected and post-mortem tissue. Scale bar = 10 μm. Right panel: percentage of SST+ cells positive for mGluR1a per subject. (H) Distribution of GRM1 and GRM5 RNA levels in SST+ cells. Data taken from the Allen Institute human single-cell RNA-seq database. Here and further, black dot shows the median, n number above is the number of cells (nuclei) plotted.
To confirm that DHPG could mediate its effect on local synaptic inhibition directly via Martinotti cells, we performed double-labeling immunohistochemistry for somatostatin and mGluR1a. We observed near-total colocalization of mGluR1a and somatostatin in samples from both surgically resected (22 out of 22 SST+ neurons from 3 samples) and post mortem (22 out of 23 SST+ neurons from 3 samples) human temporal cortex (Figure 2G). In addition, single-cell RNA-sequencing data from the Allen Brain Institute showed strong expression of both GRM1 and GRM5 in human SST+ interneurons (Figure 2H). We therefore conclude that Martinotti cells are equipped with group I mGluRs to directly respond to DHPG and mediate the increase in synaptic inhibition observed in pyramidal neurons in superficial layers of human temporal cortex following group I mGluR activation.
Synaptic Inhibition Onto Layer 1 Interneurons Is Increased by Group I mGluR Activation
Martinotti cells are known to contact most types of interneurons in addition to pyramidal neurons. Therefore, we tested whether interneurons in layer 1 (L1) of the human cortex also receive more inhibitory input upon group I mGluR activation. To this end, we recorded sIPSCs in L1 interneurons (Figures 3A,B). Similar to pyramidal neurons, sIPSC frequency onto L1 interneurons was increased during and after application of DHPG (Figure 3C), without a change in sIPSC amplitude (Figure 3D). In addition to increased sIPSC frequency, 2 out of 12 L1 interneurons showed a small increase in holding current after DHPG application (Figure 3E). This increase in holding current corresponds to a depolarization of 5.4 and 6.7 mV when taking into account the input resistance of the cells. DHPG-induced depolarization in L1 interneurons is therefore unlikely to elicit action potentials. During current-clamp recordings, L1 interneurons exhibited a small depolarization or no response, but did not fire action potentials in response to DHPG (Figure 3F, n = 3). Thus, we did not find any evidence that L1 interneurons contribute to the increase in synaptic inhibition upon group I mGluR activation. In accordance with this, human L1 interneurons express GRM5, but only rarely express GRM1 according to Allen Brain Institute single-cell sequencing data (Figure 3G).
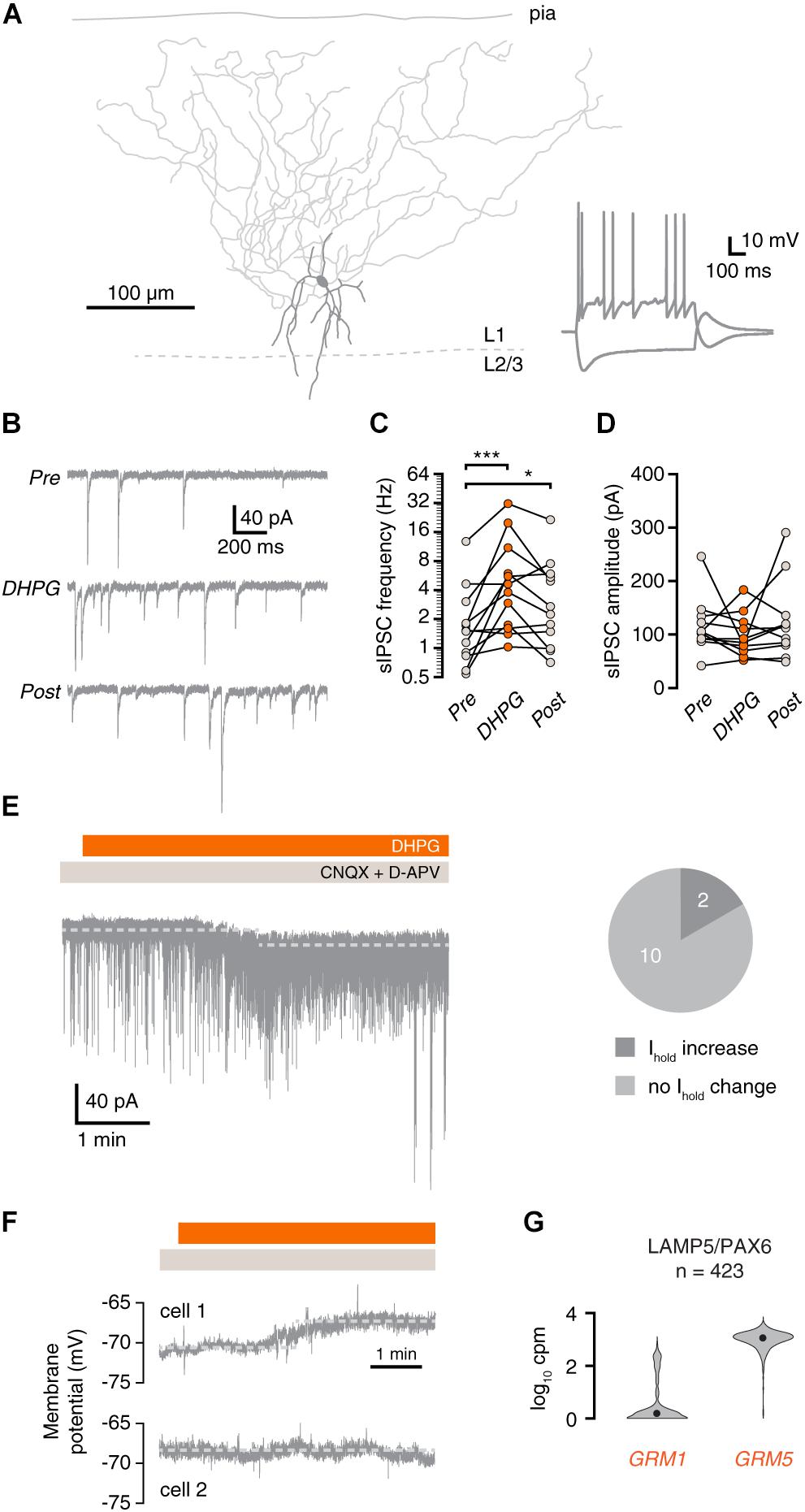
Figure 3. mGluR activation increases synaptic inhibition onto layer 1 interneurons. (A) Morphological reconstruction of a human L1 interneuron (350 μm slice). Morphology was recovered post hoc for 11 out of 15 recorded cells. Inset: electrophysiological response to negative and positive current steps. (B) Example traces showing IPSCs before (Pre), during (DHPG) and after (Post) application of DHPG. (C) DHPG elicited a prolonged increase in sIPSC frequency in L1 interneurons (repeated-measures ANOVA; F(2,22) = 12.09, p < 0.001; Tukey’s post hoc test: Pre vs. DHPG ∗∗∗p < 0.001, DHPG vs. Post ns, Pre vs. Post *p < 0.05]. (D) sIPSC amplitude in L1 interneurons was not significantly affected by DHPG [repeated-measures ANOVA; F(2,20) = 1.16, p = 0.333]. (E) Example current trace showing increased sIPSC frequency and shift in holding current upon DHPG bath application. Right panel, proportion of cells in which the holding current shifted upon DHPG application. (F) L1 interneurons are depolarized (cell 1, upper panel) or were unresponsive (cell 2, lower panel) to DHPG application. (G) GRM1 and GRM5 RNA levels in L1 interneurons. Data taken from the Allen Institute human single-cell RNA-seq database.
Group I mGluRs Depolarize Fast-Spiking Interneurons, but Do Not Elicit Action Potential Firing
In rodents, fast-spiking (FS) interneurons can be depolarized by activation of group I mGluRs. To assess whether FS interneurons contribute to DHPG-induced inhibition in human cortex, we performed current-clamp recordings of FS interneurons (Figures 4A,B). Application of DHPG led to depolarization of all recorded FS interneurons (Figure 4C, n = 7), but did not elicit action potential firing. In accordance with these results, analysis of single-cell sequencing data revealed that, similar to L1 interneurons, human PV+ FS interneurons express GRM5, rather than GRM1 (Figure 4D). DHPG application did lead to an increase in the frequency and amplitude of IPSPs (Figures 4E–G). Although this increase in IPSP frequency is likely due to increased MC activity, it could also be caused by an increase in driving force due to the depolarized membrane potential, which would facilitate detection of events. However, we found no significant correlation between the increase in IPSP frequency and the level of membrane depolarization among FS interneurons (Spearman’s R = −0.26, p = 0.62). Thus, FS interneurons receive increased synaptic inhibition upon group I mGluR activation, but are themselves not likely to contribute to this effect.
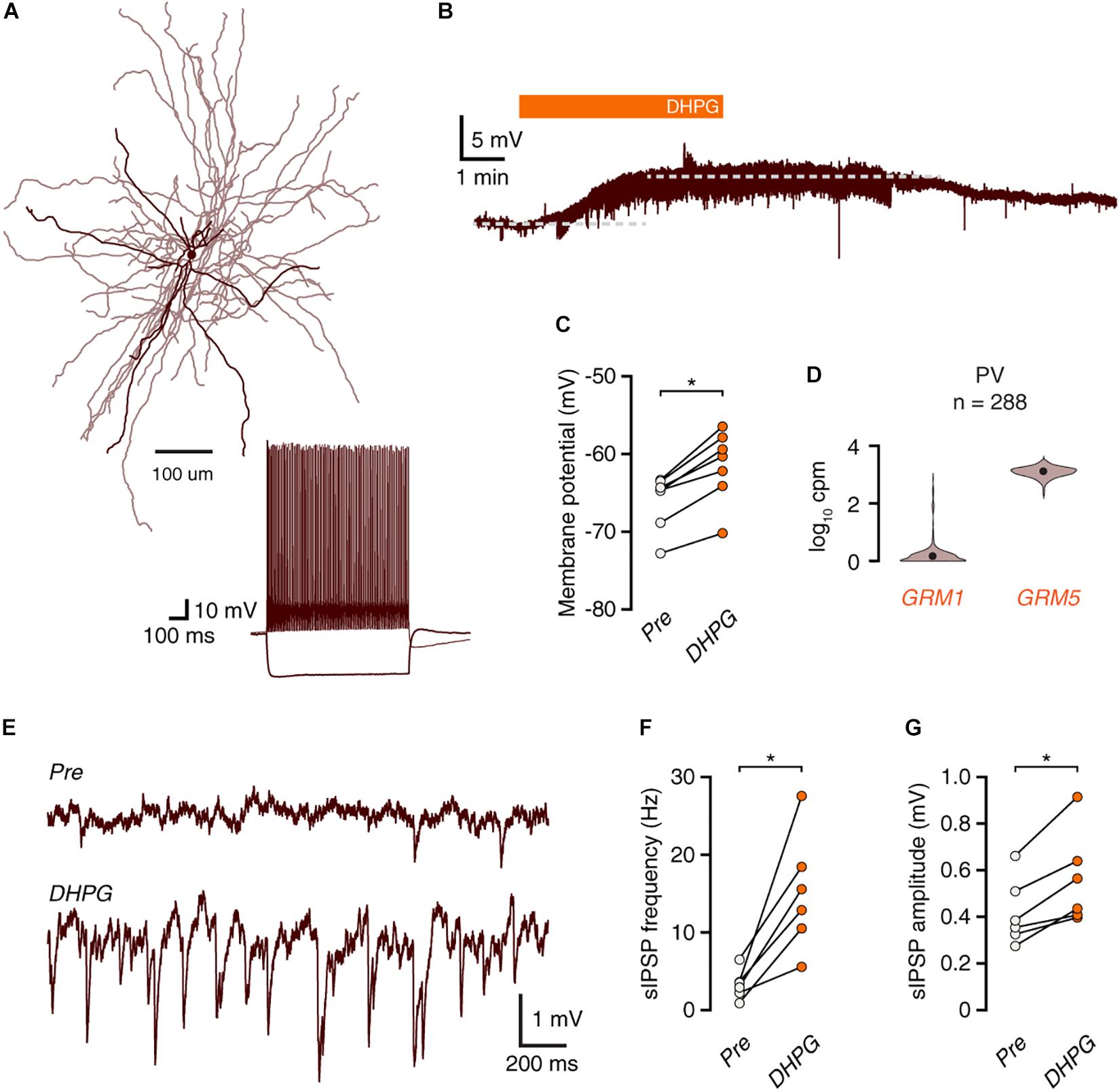
Figure 4. mGluR activation depolarizes FS interneurons without leading to action potential firing. (A) Morphological reconstruction of a human fast-spiking basket cell (350 μm slice). Morphology was recovered post hoc for 4 out of 7 recorded cells. Inset: electrophysiological response to negative and positive current steps. (B) Voltage trace showing depolarization of a fast-spiking interneuron in response to DHPG. (C) Fast-spiking interneurons are depolarized by DHPG (Wilcoxon matched-pairs signed rank test, n = 7, W = –28, *p = 0.016). (D) GRM1 and GRM5 RNA levels in FS parvalbumin+ interneurons. Data taken from the Allen Institute human single-cell RNA-seq database. (E) Representative traces showing an increase in inhibitory synaptic potentials during DHPG bath application compared to baseline. (F) DHPG increased the frequency of spontaneous inhibitory events in fast-spiking interneurons (Wilcoxon matched-pairs signed rank test, n = 6, W = 21, p = 0.031). (G) sIPSP amplitudes are increased by DHPG application (Wilcoxon matched-pairs signed rank test, n = 6, W = 21, p = 0.031).
Excitatory Inputs Onto Human Pyramidal Neurons Exhibit mGluR-Mediated Depression
Finally, we examined whether excitatory inputs were equally affected by group I mGluR activation. In current-clamp, only 2 out of 10 pyramidal neurons responded to DHPG by firing action potentials (Figures 5A,B), although most L2/3 pyramidal neurons express GRM1 and GRM5 (Figure 5C).
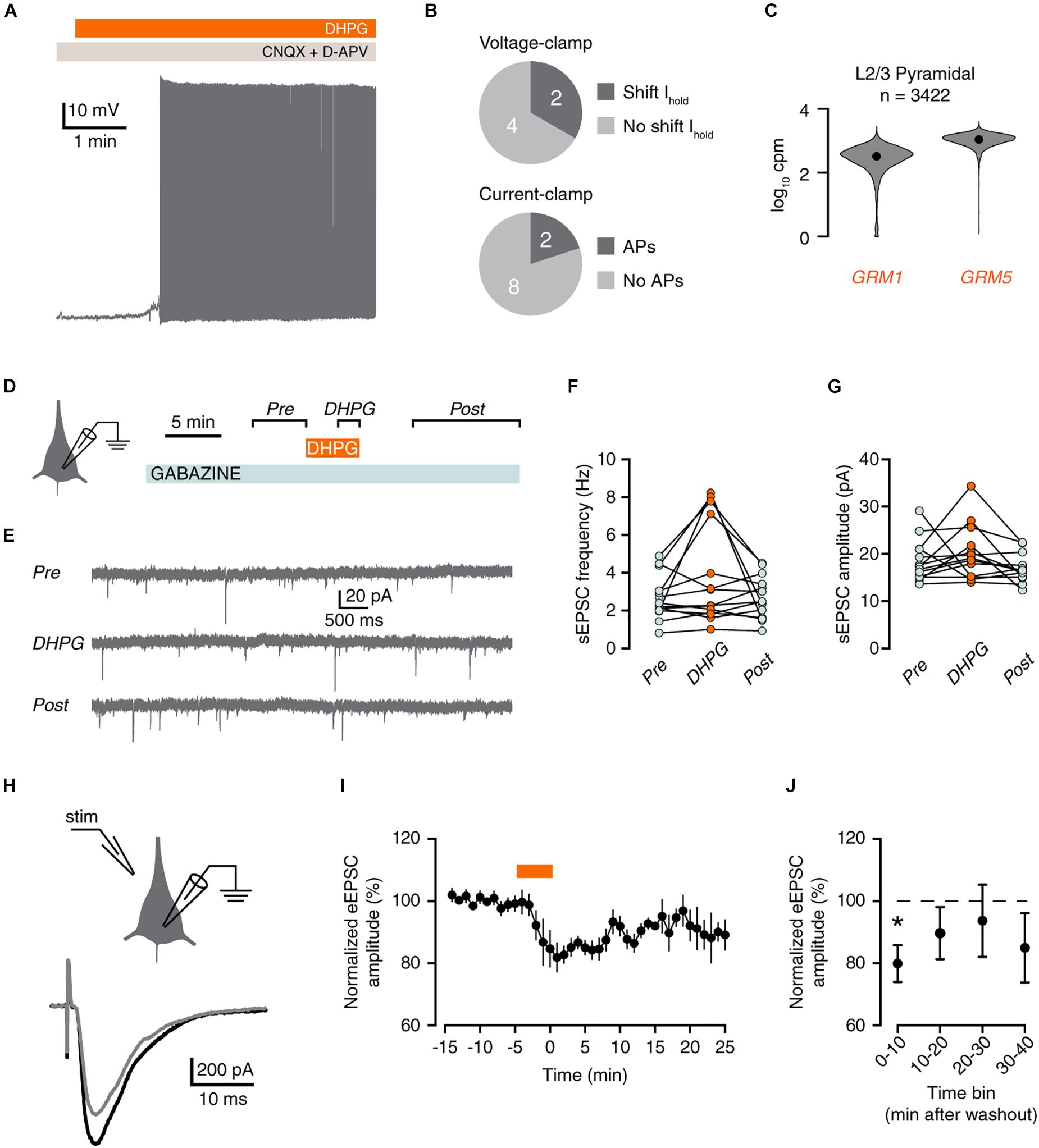
Figure 5. mGluR activation reduces excitatory inputs to pyramidal neurons. (A) Voltage trace showing DHPG-induced action potential firing in a pyramidal neuron. (B) Proportion of pyramidal neurons that displayed a shift in holding potential in voltage-clamp (top panel, neurons from Figure 1). Lower panel, proportion of pyramidal neurons that fired action potentials in response to DHPG in current-clamp. (C) GRM1 and GRM5 RNA levels in L2/3 pyramidal neurons. Data taken from the Allen Institute human single-cell RNA-seq database. (D) Experimental protocol for recording sEPSCs. (E) Example current traces showing sEPSCs. (F) sEPSC frequency was not increased by DHPG [Friedman test, χ2(2) = 3, p = 0.223]. (G) sEPSC frequency did not change significantly upon DHPG application [repeated-measures ANOVA: F(2,24) = 2.55, p = 0.122]. (H) Experimental protocol for recording evoked EPSCs, depicting placement of stimulus pipette (left panel), and example evoked responses (right panel) before (black) and after (gray) DHPG application. (I) Example of eEPSC responses during wash-in of DHPG (orange bar). Mean ± SEM of 4 responses binned per min. (J) DHPG decreased eEPSC amplitude up to 10 min after wash-out of DHPG [Friedman test, χ2(4) = 11.8, p = 0.019. Post hoc: Bonferroni-corrected Wilcoxon matched-pairs signed rank test, 10 min vs. baseline, n = 8, *p < 0.05].
We therefore examined whether DHPG increased excitatory inputs onto pyramidal cells by measuring spontaneous excitatory postsynaptic currents (sEPSCs; Figures 5D,E). Application of DHPG transiently increased sEPSCs by 25% or more in 6 out of 14 pyramidal neurons. However, there was no significant increase in sESPC frequency overall (Figure 5F).
Group I mGluRs are known to induce depression of excitatory synapses. This is mediated by mGluR5, which virtually all L2/3 pyramidal neurons express (Figure 5C). To test whether human pyramidal neuron excitatory synapses undergo mGluR-mediated depression, we evoked EPSCs (eEPSCs) by electrical stimulation (Figure 5G). Indeed, application of DHPG acutely decreased the amplitude of eEPSCs relative to baseline (Figures 5H–J). Therefore, we conclude that pyramidal neurons in human cortex exhibit group I mGluR-mediated depression of excitatory synapses.
Discussion
In this study, we addressed how activation of group I mGluRs affects microcircuits in superficial layers of the human neocortex. Our data demonstrate a cell-type specific recruitment of human cortical interneurons by group I mGluR activation. We find that Martinotti cells are strongly excited by group I mGluR activation, which increases the amount of inhibitory inputs to neighboring L2/3 pyramidal neurons. Somatostatin-positive interneurons in superficial layers of the human neocortex show strong abundance of mRNA for mGluR1 and mGluR5 receptors. Other local interneuron types, including fast spiking interneurons and layer I interneurons are depolarized by group I mGluR activation, but do not fire action potentials in response to this depolarization. Also, these interneuron types show a lower abundance of GRM1 and GRM5 mRNA. Furthermore, excitatory inputs to pyramidal neurons are suppressed by group I mGluR activation. Thus, the large increase in synaptic inhibition across cell types in superficial cortical layers and the depression of excitatory synapses most likely results in a net shift in the balance between excitation and inhibition in the cortical network.
In rodents, layer I interneurons and deep layer fast-spiking interneurons have previously been reported to fire action potentials upon mGluR activation with quisqualic acid (Zhou and Hablitz, 1997). We did not observe induced action potential firing in any human layer I interneuron or fast-spiking interneuron. This discrepancy could be due to the difference in pharmacological ligands used in the earlier study, which also activate ionotropic glutamate receptors in addition to metabotropic receptors. Our data are in agreement with metabotropic-specific ligand effects upon fast-spiking interneurons (Beierlein et al., 2000) and layer 1 cortical interneurons in rodents (Cosgrove and Maccaferri, 2012). Enhanced synaptic inhibition in fast-spiking interneurons and in layer 1 Cajal-Retzius cells is mediated by Martinotti cells in rodents. This effect is mediated by mGluR1a specifically (Beierlein et al., 2000; Cosgrove and Maccaferri, 2012). Therefore, we propose that Martinotti cells mediate enhanced synaptic inhibition in human superficial temporal cortex in response to group I mGluR activation. While we did not see direct action potential firing in any other interneuron types besides putative Martinotti cells, we cannot exclude the possibility that other interneuron types may also be involved in the mGluR-mediated increase in synaptic inhibition we observed.
Our results show that activation of group I mGluRs can directly depolarize both Martinotti cells and fast-spiking interneurons. Since group I mGluRs are located mostly perisynaptically and can therefore likely be activated by spillover of glutamate from the synaptic cleft (Luján et al., 1996), subsequent depolarization of these interneuron types may constitute a mechanism by which inhibition is increased upon a prolonged or very strong initial excitatory drive. Group I mGluR activation can alter neuronal excitability through a variety of differing mechanisms, including protein kinase C-mediated changes upon ion channels, or through calcium-dependent modulation of ion channels (Correa et al., 2017). mGluRs have been proposed to be involved in epileptogenesis (McNamara et al., 2006) and group I mGluRs are upregulated in the hippocampus of patients with temporal lobe epilepsy (Blümcke et al., 2000). In addition, studies have shown that the activation of mGluRs in hippocampal slices can increase epileptiform activity (Merlin and Wong, 1997). However, these studies often block GABAergic signaling in order to induce epileptiform activity, thereby disregarding the strong effect on inhibition we show here, and that is also observed in rodent hippocampus (McBain et al., 1994; Van Hooft et al., 2000). We therefore speculate that increased expression of mGluRs in epilepsy patients could be a homeostatic mechanism, rather than a direct component of the pathophysiology of epileptogenesis. In both cortex and hippocampus, group I mGluR-mediated increase in the frequency of inhibitory events is mediated by mGluR1 (Mannaioni et al., 2001; Sun and Neugebauer, 2011; Cosgrove and Maccaferri, 2012). We observed consistent co-expression of mGluR1a and somatostatin in putative Martinotti cells from both surgically resected tissue and autopsy controls. However, because group I mGluRs have different roles in different populations of neurons (Mannaioni et al., 2001; Volk et al., 2006), it remains to be determined whether mGluR1 or mGluR5 is solely responsible for the functional effects demonstrated here. Specifically, we found that FS and a subset of L1 interneurons are depolarized to some extent by DHPG, an effect that might be due to activation of mGluR5, which both types express.
We found group I mGluR-mediated depression of excitatory synapses received by L2/3 pyramidal neurons, similar to that observed in the rodent brain. Group I mGluR-LTD has previously been shown in human cortex for excitatory synapses onto fast-spiking interneurons (Szegedi et al., 2016). The finding of LTD at excitatory synapses on pyramidal neurons is similar to that in rodent hippocampus (Huber et al., 2000). The LTD we observed is not particularly strong and is shorter in duration than has been found previously (Huber et al., 2000). It is worth mentioning that while other studies in rodents typically use 100 μM DHPG, we only used 25 μM due to its strong acute excitation of network activity. Since the efficacy of DHPG in inducing LTD is dose-dependent (Ayala et al., 2009), this may explain why the LTD we observed was relatively small and short-lived. Overall, however, we demonstrate the occurrence of group I mGluR-induced LTD as a plasticity mechanism conserved across species, which means the aberrant LTD underlying the mGluR theory of FXS (Bear et al., 2004) may also apply to mature human cortex. However, to test mGluR-mediated LTD in FXS patient brain tissue would require using postmortem brain tissue for neurophysiological recordings (Kramvis et al., 2018), since surgically resected tissue as used in this study is not available from FXS patients.
In contrast to evoked excitatory responses, mean amplitudes of spontaneous events were not decreased by mGluR activation in our experiments. Group I mGluRs have been shown to increase the amplitude of excitatory synaptic spontaneous events in rodent somatosensory cortex (Bandrowski et al., 2003) and in rodent hippocampal interneurons (McBain et al., 1994). It is possible that in our recordings, mGluR-induced depression of a subpopulation of synapses is masked by a simultaneous global increase in events of a relatively large amplitude (Bandrowski et al., 2003), and that synaptic depression is visible only during the simultaneous timed activation of multiple synapses that occurs when synaptic events are evoked using extracellular stimulation. Conversely, a depression of excitatory synapses might cause the smaller responses from these synapses to fall below the detection threshold for spontaneous events. This might also explain why we observed no increase in the frequency of sEPSCs in most pyramidal neurons, even though the increase in action potential firing in a subset of pyramidal neurons is quite robust, and we find an increase in sESPC frequency in superficial interneurons. That only a subset of pyramidal neurons responded to mGluR activation may indicate that there are functional subtypes of pyramidal neurons in superficial human cortex that could be distinguished by differential mGluR expression. Indeed, superficial human pyramidal neurons can be divided into two classes based on morphology and electrotonic properties and their somatic location within the cortex corresponds to specific ion channel expression (Deitcher et al., 2017; Kalmbach et al., 2018). It remains to be determined whether these subtypes correspond to pyramidal neurons that do or do not respond to mGluR activation, or whether mGluR responsiveness further subdivides one or both of these classes.
Finally, recent studies using human cortical tissue have shown that there are fundamental differences in how rodent and human neurons function (Verhoog et al., 2013; Testa-Silva et al., 2014; Mohan et al., 2015; Wang et al., 2015; Eyal et al., 2016; Beaulieu-Laroche et al., 2018; Kalmbach et al., 2018). It should be noted that although the human neocortex used shows no structural abnormalities, patients typically had a long history of seizures and had been exposed to a variety of anti-epileptic medications, thus we cannot conclude unequivocally that these factors have not influenced neuronal function in some form. However, specific cholinergic mechanisms and modulation of disynaptic inhibition between cortical pyramidal neurons are conserved between rodents and humans (Obermayer et al., 2018; Poorthuis et al., 2018), as are the action of group II mGluRs (Bocchio et al., 2019), and group I mGluR-dependent LTD of excitatory synapses onto fast-spiking interneurons (Szegedi et al., 2016). We show here that several aspects of group I mGluR activation in the cortex are preserved across these mammalian species. The balance of synaptic excitation to inhibition and the role for aberrant mGluR signaling is of increasing focus for the synaptic, network and behavioral phenotypes related to rodent NDD and neuropsychiatry models (Levenga et al., 2010; Barnes et al., 2015; Nelson and Valakh, 2015; Lee et al., 2017). Notably, the specific aspects of group I mGluR function we validate as occurring in human cortex are also dysregulated in mouse models for FXS, notably enhanced LTD in hippocampal pyramidal neurons (Bear et al., 2004) and altered GABAergic inhibitory function specifically mediated by mGluR1 (Paluszkiewicz et al., 2011a; Cea-Del Rio and Huntsman, 2014). At the start of the 21st century, just over one third of all licensed and approved pharmaceutical drugs directly or indirectly modulated G-protein coupled receptors (Klabunde and Hessler, 2002). However, our fundamental knowledge on the function of G-protein coupled receptors, specifically mGluRs, and their specificity of action upon different neuronal subtypes within the human brain is far from complete. Therefore, we believe that our data have direct implications for interpreting the actions of group I mGluR-mediated signaling not only in human cortical circuits, but for translational approaches when designing clinical models from NDD rodent data to test specific mGluR targets therapeutically.
Data Availability
The raw data supporting the conclusions of this manuscript will be made available by the authors, without undue reservation, to any qualified researcher.
Ethics Statement
This study was carried out in accordance with the recommendations of the VU University Medical Center Medical Ethical Committee and in accordance with the Dutch law and the declaration of Helsinki. All 40 patients provided written informed consent.
Author Contributions
TK, RM, JD, IK, and HM designed the study. TK, JD, IK, JA, JO, MV, and RW performed the experiments. TK, JD, IK, NG, and EA analyzed the data. SI and JB performed neurosurgery. TK, RM, and HM wrote the manuscript.
Funding
HM received funding from Netherlands Organization for Scientific Research (NWO; VICI grant #016.140.610), ERC starting grant “BrainSignals”, and the European Union’s Horizon 2020 Framework Programme for Research and Innovation under the specific grant agreement number 785907 (Human Brain Project SGA2). RM received funding from Netherlands Organization for Scientific Research (NWO VIDI grant #917.10.372) and Fondation Jérôme LeJeune and Hersenstichting NL 2013(1)-229.
Conflict of Interest Statement
The authors declare that the research was conducted in the absence of any commercial or financial relationships that could be construed as a potential conflict of interest.
Acknowledgments
We thank Anton Pieneman and Christiaan de Kock for their assistance in biocytin stainings, and Hans Lodder, Tim Heistek, and Jaap Timmerman for their excellent technical assistance.
References
Aguilar-Valles, A., Matta-Camacho, E., Khoutorsky, A., Gkogkas, C., Nader, K., Lacaille, J.-C., et al. (2015). Inhibition of group I metabotropic glutamate receptors reverses autistic-like phenotypes caused by deficiency of the translation repressor eIF4E binding protein 2. J. Neurosci. 35, 11125–11132. doi: 10.1523/JNEUROSCI.4615-14.2015
Ascoli, G. A., Alonso-Nanclares, L., Anderson, S. A., Barrionuevo, G., Benavides-Piccione, R., Burkhalter, A., et al. (2008). Petilla terminology: nomenclature of features of GABAergic interneurons of the cerebral cortex. Nat. Rev. Neurosci. 9, 557–568. doi: 10.1038/nrn2402
Ayala, J. E., Chen, Y., Banko, J. L., Sheffler, D. J., Williams, R., Telk, A. N., et al. (2009). mGluR5 positive allosteric modulators facilitate both hippocampal LTP and LTD and enhance spatial learning. Neuropsychopharmacology 34, 2057–2071. doi: 10.1038/npp.2009.30
Bandrowski, A. E., Huguenard, J. R., and Prince, D. A. (2003). Baseline glutamate levels affect group I and II mGluRs in layer V pyramidal neurons of rat sensorimotor cortex. J. Neurophysiol. 89, 1308–1316. doi: 10.1152/jn.00644.2002
Barnes, S. A., Pinto-Duarte, A., Kappe, A., Zembrzycki, A., Metzler, A., Mukamel, E. A., et al. (2015). Disruption of mGluR5 in parvalbumin-positive interneurons induces core features of neurodevelopmental disorders. Mol. Psychiatry 20, 1161–1172. doi: 10.1038/mp.2015.113
Baskys, A., Bernstein, N. K., Barolet, A. W., and Carlen, P. L. (1990). NMDA and quisqualate reduce a Ca-dependent K+ current by a protein kinase-mediated mechanism. Neurosci. Lett. 112, 76–81. doi: 10.1016/0304-3940(90)90325-4
Bear, M. F., Huber, K. M., and Warren, S. T. (2004). The mGluR theory of fragile X mental retardation. Trends Neurosci. 27, 370–377. doi: 10.1016/j.tins.2004.04.009
Beaulieu-Laroche, L., Toloza, E. H. S., van der Goes, M.-S., Lafourcade, M., Barnagian, D., Williams, Z. M., et al. (2018). Enhanced dendritic compartmentalization in human cortical neurons. Cell 175, 643.e14–651.e14. doi: 10.1016/j.cell.2018.08.045
Beierlein, M., Gibson, J. R., and Connors, B. W. (2000). A network of electrically coupled interneurons drives synchronized inhibition in neocortex. Nat. Neurosci. 3, 904–910. doi: 10.1038/78809
Berry-Kravis, E. (2014). Mechanism-based treatments in neurodevelopmental disorders: fragile X syndrome. Pediatr. Neurol. 50, 297–302. doi: 10.1016/j.pediatrneurol.2013.12.001
Berry-Kravis, E., Portes, V. D., Hagerman, R., Jacquemont, S., Charles, P., Visootsak, J., et al. (2016). Mavoglurant in fragile X syndrome: results of two randomized, double-blind, placebo-controlled trials. Sci. Transl. Med. 8:321ra5. doi: 10.1126/scitranslmed.aab4109
Berry-Kravis, E. M., Lindemann, L., Jønch, A. E., Apostol, G., Bear, M. F., Carpenter, R. L., et al. (2018). Drug development for neurodevelopmental disorders: lessons learned from fragile X syndrome. Nat. Rev. Drug Discov. 17, 280–299. doi: 10.1038/nrd.2017.221
Blümcke, I., Becker, A. J., Klein, C., Scheiwe, C., Lie, A. A., Beck, H., et al. (2000). Temporal lobe epilepsy associated up-regulation of metabotropic glutamate receptors: correlated changes in mGluR1 mRNA and protein expression in experimental animals and human patients. J. Neuropathol. Exp. Neurol. 59, 1–10. doi: 10.1093/jnen/59.1.1
Bocchio, M., Lukacs, I. P., Stacey, R., Plaha, P., Apostolopoulos, V., Livermore, L., et al. (2019). Group II metabotropic glutamate receptors mediate presynaptic inhibition of excitatory transmission in pyramidal neurons of the human cerebral cortex. Front. Cell. Neurosci. 12:508. doi: 10.3389/fncel.2018.00508
Boer, K., Encha-Razavi, F., Sinico, M., and Aronica, E. (2010). Differential distribution of group I metabotropic glutamate receptors in developing human cortex. Brain Res. 1324, 24–33. doi: 10.1016/j.brainres.2010.02.005
Cea-Del Rio, C. A., and Huntsman, M. M. (2014). The contribution of inhibitory interneurons to circuit dysfunction in Fragile X Syndrome. Front. Cell. Neurosci. 8:245. doi: 10.3389/fncel.2014.00245
Chuang, S.-C., Bianchi, R., and Wong, R. K. S. (2000). Group I mGluR activation turns on a voltage-gated inward current in hippocampal pyramidal cells. J. Neurophysiol. 83, 2844–2853. doi: 10.1152/jn.2000.83.5.2844
Conn, P. J., Lindsley, C. W., and Jones, C. K. (2009). Activation of metabotropic glutamate receptors as a novel approach for the treatment of schizophrenia. Trends Pharmacol. Sci. 30, 25–31. doi: 10.1016/j.tips.2008.10.006
Correa, A. M. B., Guimarães, J. D. S., dos Santos E Alhadas, E., and Kushmerick, C. (2017). Control of neuronal excitability by Group I metabotropic glutamate receptors. Biophys. Rev. 9, 835–845. doi: 10.1007/s12551-017-0301-7
Cosgrove, K. E., and Maccaferri, G. (2012). mGlu1α-dependent recruitment of excitatory GABAergic input to neocortical Cajal-Retzius cells. Neuropharmacology 63, 486–493. doi: 10.1016/j.neuropharm.2012.04.025
Crepel, V., Aniksztejn, L., Ben-Ari, Y., and Hammond, C. (1994). Glutamate metabotropic receptors increase a Ca(2+)-activated nonspecific cationic current in CA1 hippocampal neurons. J. Neurophysiol. 72, 1561–1569. doi: 10.1152/jn.1994.72.4.1561
Deitcher, Y., Eyal, G., Kanari, L., Verhoog, M. B., Antoine Kahou, G. A., Mansvelder, H. D., et al. (2017). Comprehensive morpho-electrotonic analysis shows 2 distinct classes of L2 and L3 pyramidal neurons in human temporal cortex. Cereb. Cortex 27, 5398–5414. doi: 10.1093/cercor/bhx226
Eyal, G., Verhoog, M. B., Testa-Silva, G., Deitcher, Y., Lodder, J. C., Benavides-Piccione, R., et al. (2016). Unique membrane properties and enhanced signal processing in human neocortical neurons. eLife Sci. 5:e16553. doi: 10.7554/eLife.16553
Galante, M., and Diana, M. A. (2004). Group I metabotropic glutamate receptors inhibit GABA release at interneuron-purkinje cell synapses through endocannabinoid production. J. Neurosci. 24, 4865–4874. doi: 10.1523/jneurosci.0403-04.2004
Goriounova, N. A., Heyer, D. B., Wilbers, R., Verhoog, M. B., Giugliano, M., Verbist, C., et al. (2018). Large and fast human pyramidal neurons associate with intelligence. eLife 7:e41714. doi: 10.7554/eLife.41714
Guérineau, N. C., Bossu, J. L., Gahwiler, B. H., and Gerber, U. (1995). Activation of a nonselective cationic conductance by metabotropic glutamatergic and muscarinic agonists in CA3 pyramidal neurons of the rat hippocampus. J. Neurosci. 15, 4395–4407. doi: 10.1523/jneurosci.15-06-04395.1995
Guérineau, N. C., Gähwiler, B. H., and Gerber, U. (1994). Reduction of resting K+ current by metabotropic glutamate and muscarinic receptors in rat CA3 cells: mediation by G-proteins. J. Physiol. 474, 27–33. doi: 10.1113/jphysiol.1994.sp019999
Hodge, R. D., Bakken, T. E., Miller, J. A., Smith, K. A., Barkan, E. R., Graybuck, L. T., et al. (2018). Conserved cell types with divergent features between human and mouse cortex. BioRxiv 384826. doi: 10.1016/bs.ctdb.2018.02.011
Huber, K. M., Gallagher, S. M., Warren, S. T., and Bear, M. F. (2002). Altered synaptic plasticity in a mouse model of fragile X mental retardation. PNAS 99, 7746–7750. doi: 10.1073/pnas.122205699
Huber, K. M., Kayser, M. S., and Bear, M. F. (2000). Role for rapid dendritic protein synthesis in hippocampal mGluR-dependent long-term depression. Science 288, 1254–1256. doi: 10.1126/science.288.5469.1254
Jacquemont, S., Berry-Kravis, E., Hagerman, R., von Raison, F., Gasparini, F., Apostol, G., et al. (2014). The challenges of clinical trials in fragile X syndrome. Psychopharmacology 231, 1237–1250. doi: 10.1007/s00213-013-3289-0
Kalmbach, B. E., Buchin, A., Long, B., Close, J., Nandi, A., Miller, J. A., et al. (2018). h-Channels contribute to divergent intrinsic membrane properties of supragranular pyramidal neurons in human versus mouse cerebral cortex. Neuron 100, 1194.e5–1208.e5. doi: 10.1016/j.neuron.2018.10.012
Klabunde, T., and Hessler, G. (2002). Drug design strategies for targeting g-protein-coupled receptors. Chem. Bio. Chem. 3, 928–944. doi: 10.1002/1439-7633(20021004)3:10<928::aid-cbic928>3.0.co;2-5
Kramvis, I., Mansvelder, H. D., and Meredith, R. M. (2018). Neuronal life after death: electrophysiologic recordings from neurons in adult human brain tissue obtained through surgical resection or postmortem. Handb. Clin. Neurol. 150, 319–333. doi: 10.1016/B978-0-444-63639-3.00022-0
Lee, E., Lee, J., and Kim, E. (2017). Excitation/inhibition imbalance in animal models of autism spectrum disorders. Biol. Psychiatry 81, 838–847. doi: 10.1016/j.biopsych.2016.05.011
Levenga, J., de Vrij, F. M. S., Oostra, B. A., and Willemsen, R. (2010). Potential therapeutic interventions for fragile X syndrome. Trends Mol. Med. 16, 516–527. doi: 10.1016/j.molmed.2010.08.005
López-Bendito, G., Shigemoto, R., Fairén, A., and Luján, R. (2002). Differential distribution of group I metabotropic glutamate receptors during rat cortical development. Cereb. Cortex 12, 625–638. doi: 10.1093/cercor/12.6.625
Luján, R., Nusser, Z., Roberts, J. D. B., Shigemoto, R., and Somogyi, P. (1996). Perisynaptic location of metabotropic glutamate receptors mGluR1 and mGluR5 on dendrites and dendritic spines in the rat hippocampus. Eur. J. Neurosci. 8, 1488–1500. doi: 10.1111/j.1460-9568.1996.tb01611.x
Lüscher, C., and Huber, K. M. (2010). Group 1 mGluR-dependent synaptic long-term depression (mGluR-LTD): mechanisms and implications for circuitry & disease. Neuron 65, 445–459. doi: 10.1016/j.neuron.2010.01.016
Magee, J. C. (2000). Dendritic integration of excitatory synaptic input. Nat. Rev. Neurosci. 1, 181–190. doi: 10.1038/35044552
Mannaioni, G., Marino, M. J., Valenti, O., Traynelis, S. F., and Conn, P. J. (2001). Metabotropic glutamate receptors 1 and 5 differentially regulate CA1 pyramidal cell function. J. Neurosci. 21, 5925–5934. doi: 10.1523/jneurosci.21-16-05925.2001
McBain, C. J., DiChiara, T. J., and Kauer, J. A. (1994). Activation of metabotropic glutamate receptors differentially affects two classes of hippocampal interneurons and potentiates excitatory synaptic transmission. J. Neurosci. 14, 4433–4445. doi: 10.1523/jneurosci.14-07-04433.1994
McNamara, J. O., Huang, Y. Z., and Leonard, A. S. (2006). Molecular signaling mechanisms underlying epileptogenesis. Sci. Signal. 2006:re12. doi: 10.1126/stke.3562006re12
Merlin, L. R., and Wong, R. K. S. (1997). Role of group I metabotropic glutamate receptors in the patterning of epileptiform activities in vitro. J. Neurophysiol. 78, 539–544. doi: 10.1152/jn.1997.78.1.539
Mohan, H., Verhoog, M. B., Doreswamy, K. K., Eyal, G., Aardse, R., Lodder, B. N., et al. (2015). Dendritic and axonal architecture of individual pyramidal neurons across layers of adult human neocortex. Cereb. Cortex 25, 4839–4853. doi: 10.1093/cercor/bhv188
Mullard, A. (2015). Fragile X disappointments upset autism ambitions. Nat. Rev. Drug Discov. 14, 151–153. doi: 10.1038/nrd4555
Myatt, D. R., Hadlington, T., Ascoli, G. A., and Nasuto, S. J. (2012). Neuromantic – from semi-manual to semi-automatic reconstruction of neuron morphology. Front. Neuroinform. 6:4. doi: 10.3389/fninf.2012.00004
Nakanishi, S. (1992). Molecular diversity of glutamate receptors and implications for brain function. Science 258, 597–603. doi: 10.1126/science.1329206
Nelson, S. B., and Valakh, V. (2015). Excitatory/inhibitory balance and circuit homeostasis in autism spectrum disorders. Neuron 87, 684–698. doi: 10.1016/j.neuron.2015.07.033
Obermayer, J., Heistek, T. S., Kerkhofs, A., Goriounova, N. A., Kroon, T., Baayen, J. C., et al. (2018). Lateral inhibition by Martinotti interneurons is facilitated by cholinergic inputs in human and mouse neocortex. Nat. Commun. 9:4101. doi: 10.1038/s41467-018-06628-w
Paluszkiewicz, S. M., Martin, B. S., and Huntsman, M. M. (2011a). Fragile X syndrome: the GABAergic system and circuit dysfunction. Dev. Neurosci. 33, 349–364. doi: 10.1159/000329420
Paluszkiewicz, S. M., Olmos-Serrano, J. L., Corbin, J. G., and Huntsman, M. M. (2011b). Impaired inhibitory control of cortical synchronization in fragile X syndrome. J. Neurophysiol. 106, 2264–2272. doi: 10.1152/jn.00421.2011
Poorthuis, R. B., Muhammad, K., Wang, M., Verhoog, M. B., Junek, S., Wrana, A., et al. (2018). Rapid neuromodulation of layer 1 interneurons in human neocortex. Cell Rep. 23, 951–958. doi: 10.1016/j.celrep.2018.03.111
Sherman, S. M. (2014). The function of metabotropic glutamate receptors in thalamus and cortex. Neuroscientist 20, 136–149. doi: 10.1177/1073858413478490
Sun, H., and Neugebauer, V. (2011). mGluR1, but not mGluR5, activates feed-forward inhibition in the medial prefrontal cortex to impair decision making. J. Neurophysiol. 106, 960–973. doi: 10.1152/jn.00762.2010
Szegedi, V., Paizs, M., Csakvari, E., Molnar, G., Barzo, P., Tamas, G., et al. (2016). Plasticity in single axon glutamatergic connection to GABAergic interneurons regulates complex events in the human neocortex. PLoS Biol. 14:e2000237. doi: 10.1371/journal.pbio.2000237
Testa-Silva, G., Verhoog, M. B., Linaro, D., de Kock, C. P. J., Baayen, J. C., Meredith, R. M., et al. (2014). High bandwidth synaptic communication and frequency tracking in human neocortex. PLoS Biol. 12:e1002007. doi: 10.1371/journal.pbio.1002007
Tremblay, R., Lee, S., and Rudy, B. (2016). GABAergic interneurons in the neocortex: from cellular properties to circuits. Neuron 91, 260–292. doi: 10.1016/j.neuron.2016.06.033
Valentinova, K., and Mameli, M. (2016). mGluR-LTD at excitatory and inhibitory synapses in the lateral habenula tunes neuronal output. Cell Rep. 16, 2298–2307. doi: 10.1016/j.celrep.2016.07.064
Van Hooft, J. A., Giuffrida, R., Blatow, M., and Monyer, H. (2000). Differential expression of group I metabotropic glutamate receptors in functionally distinct hippocampal interneurons. J. Neurosci. 20, 3544–3551. doi: 10.1523/jneurosci.20-10-03544.2000
Verhoog, M. B., Goriounova, N. A., Obermayer, J., Stroeder, J., Hjorth, J. J. J., Testa-Silva, G., et al. (2013). Mechanisms underlying the rules for associative plasticity at adult human neocortical synapses. J. Neurosci. 33, 17197–17208. doi: 10.1523/JNEUROSCI.3158-13.2013
Volk, L. J., Daly, C. A., and Huber, K. M. (2006). Differential roles for group 1 mGluR subtypes in induction and expression of chemically induced hippocampal long-term depression. J. Neurophysiol. 95, 2427–2438. doi: 10.1152/jn.00383.2005
Wang, B., Yin, L., Zou, X., Ye, M., Liu, Y., He, T., et al. (2015). A subtype of inhibitory interneuron with intrinsic persistent activity in human and monkey neocortex. Cell Rep. 10, 1450–1458. doi: 10.1016/j.celrep.2015.02.018
Wang, X. F., and Daw, N. W. (1996). Metabotropic glutamate receptors potentiate responses to NMDA and AMPA from layer V cells in rat visual cortex. J. Neurophysiol. 76, 808–815. doi: 10.1152/jn.1996.76.2.808
Wenger, T. L., Kao, C., McDonald-McGinn, D. M., Zackai, E. H., Bailey, A., Schultz, R. T., et al. (2016). The role of mGluR copy number variation in genetic and environmental forms of syndromic autism spectrum disorder. Sci. Rep. 6:sre19372. doi: 10.1038/srep19372
Keywords: mGluR, human cortex, Martinotti, fast-spiking interneuron, LTD, single-cell RNA-sequencing
Citation: Kroon T, Dawitz J, Kramvis I, Anink J, Obermayer J, Verhoog MB, Wilbers R, Goriounova NA, Idema S, Baayen JC, Aronica E, Mansvelder HD and Meredith RM (2019) Group I mGluR-Mediated Activation of Martinotti Cells Inhibits Local Cortical Circuitry in Human Cortex. Front. Cell. Neurosci. 13:315. doi: 10.3389/fncel.2019.00315
Received: 03 May 2019; Accepted: 27 June 2019;
Published: 11 July 2019.
Edited by:
Annalisa Scimemi, University at Albany, United StatesReviewed by:
Karri P. Lamsa, University of Szeged, HungaryMarco Capogna, Aarhus University, Denmark
Copyright © 2019 Kroon, Dawitz, Kramvis, Anink, Obermayer, Verhoog, Wilbers, Goriounova, Idema, Baayen, Aronica, Mansvelder and Meredith. This is an open-access article distributed under the terms of the Creative Commons Attribution License (CC BY). The use, distribution or reproduction in other forums is permitted, provided the original author(s) and the copyright owner(s) are credited and that the original publication in this journal is cited, in accordance with accepted academic practice. No use, distribution or reproduction is permitted which does not comply with these terms.
*Correspondence: Rhiannon M. Meredith, RMM3r3dith@gmail.com
†Presentaddress: Tim Kroon, MRC Centre for Developmental Neurobiology, Institute of Psychiatry, Psychology and Neuroscience, King’s College London, London, United Kingdom; Matthijs B. Verhoog, Department of Human Biology, Neuroscience Institute, University of Cape Town, Cape Town, South Africa