AMPA Receptor-Mediated Ca2+ Transients in Mouse Olfactory Ensheathing Cells
- Division of Neurophysiology, University of Hamburg, Hamburg, Germany
Ca2+ signaling in glial cells is primarily triggered by metabotropic pathways and the subsequent Ca2+ release from internal Ca2+ stores. However, there is upcoming evidence that various ion channels might also initiate Ca2+ rises in glial cells by Ca2+ influx. We investigated AMPA receptor-mediated inward currents and Ca2+ transients in olfactory ensheathing cells (OECs), a specialized glial cell population in the olfactory bulb (OB), using whole-cell voltage-clamp recordings and confocal Ca2+ imaging. By immunohistochemistry we showed immunoreactivity to the AMPA receptor subunits GluA1, GluA2 and GluA4 in OECs, suggesting the presence of AMPA receptors in OECs. Kainate-induced inward currents were mediated exclusively by AMPA receptors, as they were sensitive to the specific AMPA receptor antagonist, GYKI53655. Moreover, kainate-induced inward currents were reduced by the selective Ca2+-permeable AMPA receptor inhibitor, NASPM, suggesting the presence of functional Ca2+-permeable AMPA receptors in OECs. Additionally, kainate application evoked Ca2+ transients in OECs which were abolished in the absence of extracellular Ca2+, indicating that Ca2+ influx via Ca2+-permeable AMPA receptors contribute to kainate-induced Ca2+ transients. However, kainate-induced Ca2+ transients were partly reduced upon Ca2+ store depletion, leading to the conclusion that Ca2+ influx via AMPA receptor channels is essential to trigger Ca2+ transients in OECs, whereas Ca2+ release from internal stores contributes in part to the kainate-evoked Ca2+ response. Endogenous glutamate release by OSN axons initiated Ca2+ transients in OECs, equally mediated by metabotropic receptors (glutamatergic and purinergic) and AMPA receptors, suggesting a prominent role for AMPA receptor mediated Ca2+ signaling in axon-OEC communication.
Introduction
Ca2+ signaling in glial cells is involved in various intercellular processes such as the release of gliotransmitters, modulation of synaptic transmission, long-range Ca2+ wave propagation, and neurovascular coupling (Haydon, 2001; Carmignoto and Gomez-Gonzalo, 2010). Moreover, intracellular processes such as apoptosis, transcription as well as posttranslational modification are regulated by Ca2+ signaling (McConkey and Orrenius, 1997; Flavell and Greenberg, 2008). In glial cells, rises of intracellular Ca2+ are predominantly triggered by Ca2+ release from internal Ca2+ stores induced by metabotropic pathways (Deitmer et al., 1998; Verkhratsky et al., 1998), while Ca2+ influx was reported in rare cases in specialized astrocyte-like glial cells such as cerebellar Bergmann glia and retinal Müller glia (Burnashev et al., 1992; Muller et al., 1992; Wakakura and Yamamoto, 1994). However, more recent studies suggest that astroglial cells of different regions of the brain also express ligand-gated ion channels that may trigger Ca2+ responses via Ca2+ influx from the extracellular space (Schipke et al., 2001; Lalo et al., 2006; Mishra et al., 2016; Droste et al., 2017). In addition, store-operated channels as well as transient receptor potential (TRP) channels contribute to Ca2+ signaling in astrocytes (Singaravelu et al., 2006; Rungta et al., 2016; Belkacemi et al., 2017; Rakers et al., 2017; Toth et al., 2019). These studies indicate that Ca2+ influx might play a previously underestimated role in glial cell physiology and function. Olfactory ensheathing cells (OECs) represent a specialized population of glial cells, exclusively located in the olfactory nerve layer (ONL) in the olfactory bulb (OB) and the peripheral olfactory mucosa (Su and He, 2010; Lohr et al., 2014). They support growth and guidance of axons of olfactory sensory neurons (OSN) from the olfactory epithelium (OE) into the main OB (Graziadei and Graziadei, 1979; Doucette, 1984; Yang et al., 2015). It is assumed, that OECs generate an environment allowing the lifelong re-growth and re-integration of axons into the cellular network after degeneration by the expression of different neurite growth factors and cell adhesions molecules (Crandall et al., 2000; Woodhall et al., 2001; Cao et al., 2007; Yang et al., 2015). In addition, studies showed that Ca2+ signaling in OECs is a critical regulator for neurite outgrowth in OEC/retinal ganglion cell (RGC) co-cultures, suggesting a prominent role for Ca2+ signaling in OEC-axon interaction (Hayat et al., 2003). Extrasynaptic release of glutamate and ATP by OSN axons initiates Gq-mediated Ca2+ release from internal stores in OECs via mGluR1 and P2Y1 receptors (Rieger et al., 2007; Thyssen et al., 2010). Montague and Greer (1999) investigated the distribution of the ionotropic AMPA receptor subunits, GluA1, GluA2/3 and GluA4 in the ONL and found expression by nerve-associated glial cells. However, whether OECs exhibit functional AMPA receptors, which might conduct membrane currents and mediate Ca2+ influx is not known. We performed whole-cell voltage-clamp recordings, showing that kainate application induced AMPA receptor-mediated inward currents in OECs, which are partly mediated by Ca2+-permeable AMPA receptors. Kainate also induced Ca2+ transients that depended on Ca2+ influx via the receptor channel itself, but additionally comprised Ca2+ release from internal stores. Electrical stimulation of OSN axons evoked Ca2+ transients mediated by metabotropic receptors as well as Ca2+-permeable AMPA receptors, suggesting a role for AMPA receptor-mediated Ca2+ signaling in axon-OEC communication.
Materials and Methods
Animals and Olfactory Bulb Preparation
Mice of the GLAST-CreERT2 × tdTomatofl/fl, PLP-CreERT2 × tdTomatofl/fl (age: p28–60) and NMRI (age: p18–p21; Naval Medical Research Institute) strains (Leone et al., 2003; Mori et al., 2006; Madisen et al., 2010) were kept at the institutional animal facility of the University of Hamburg. These mice expressed Cre recombinase under control of the promoters of the glutamate/aspartate transporter EAAT1 (GLAST; expressed by OECs and astrocytes) and the proteolipid protein (PLP; expressed by OECs and oligodendrocytes), respectively (Droste et al., 2017; Beiersdorfer et al., 2019). Animal rearing and all experimental procedures were performed according to the European Union’s and local animal welfare guidelines (GZ G21305/591-00.33; Behörde für Gesundheit und Verbraucherschutz, Hamburg, Germany). To induce reporter expression in GLAST-CreERT2 × tdTomatofl/fl and PLP-CreERT2 × tdTomatofl/fl mice, tamoxifen (Carbolution Chemicals GmbH, St. Ingbert, Germany) was dissolved in 8% ethanol/92% Mygliol®812 (Sigma Aldrich, Taufkirchen, Germany) and injected intraperitoneally for three consecutive days (starting p21; 100 mg/kg bodyweight). Animals were analyzed 7–12 days after the first injection. OBs were prepared as described previously (Stavermann et al., 2012). Both OBs were removed from the opened head in cool preparation solution (molarities in mM: 83 NaCl, 1 NaH2PO4x2H2O, 26.2 NaHCO3, 2.5 KCl, 70 sucrose, 20 D-(+)-glucose, and 2.5 MgSO4 × 7 H2O). Standard artificial cerebrospinal fluid (ACSF) for experiments and storage of the preparations consisted of (molarities in mM): 120 NaCl, 2.5 KCl, 1 NaH2PO4x2H2O, 26 NaHCO3, 2.8 D-(+)-glucose, 1 MgCl2, and 2 CaCl2). Preparation solution and ACSF were continuously perfused with carbogen (95% O2 and 5% CO2) to maintain the pH of 7.4 and to supply oxygen.
Reagents
The compounds (2S,3S,4S)-3-(Carboxymethyl)-4-(prop- 1-en-2-yl)pyrrolidine-2-carboxylic acid (kainic acid, agonist of AMPA/kainate receptors), (S)-α-Amino-3-hydroxy-5- methylisoxazole-4-propionic acid, 4-(8-Methyl-9H-1,3-dioxolo [4,5-h][2,3]benzodiazepin-5-yl)-benzenamine hydrochloride (GYKI53655, antagonist of AMPA receptors), (E)-ethyl 1,1a,7,7a-tetrahydro-7-(hydroxyimino) cyclopropa[b]chromene-1a-carboxylate (CPCCOEt, antagonist of mGluR1), 2-methyl- 6-(phenylethynyl) pyridine (MPEP, antagonist of mGluR5), 2′-deoxy-N6-methyladenosine 3′,5′-bisphosphate (MRS2179, antagonist of P2Y1), 4-(2-[7-Amino-2-(2-furyl)[1,2,4]triazolo [2,3-α][1,3,5]triazin-5-ylamino]ethyl) pheno (ZM241385, antagonist of A2A receptors), (R)-(+)-7-chloro-8-hydroxy-3-methyl-1-phenyl-2,3,4,5-tetrahydro-1H-3-benzazepine (SCH2 3390, antagonist of D1-like dopamine receptors) and carbenoxolone disodium salt (CBX; inhibiting gap junctions) were obtained from Abcam (Cambridge, United Kingdom). The reagents D-2-amino-5-phosphonovaleric acid (D-APV; antagonist of NMDA receptors), N-[3-[[4-[(3-aminopropyl)amino]butyl] amino]propyl]-1-naphthaleneacetamide trihydrochloride (Naspm trihydrochloride antagonist of Ca2+-permeable AMPA receptors) and tetrodotoxin (TTX; inhibiting voltage-gated sodium channels) were received from Alomone labs (Jerusalem, Israel). All reagents were stored as stock solutions corresponding to the manufacturer’s instructions and added to ACSF directly before the experiment. Drugs were applied via the perfusion system driven by a peristaltic pump (Reglo MS4/12, Ismatec, Wertheim, Germany).
Electrophysiology and Analysis
For electrophysiological experiments, OB slices (220 μm, horizontal) of GLAST-CreERT2 × tdTomatofl/fl mice were prepared using a vibratome (Leica VT1200S, Nußloch, Germany). Slices were transferred into a recording chamber and continuously superfused with ACSF via the perfusion system. The experiments were performed at room temperature. Whole-cell voltage-clamp recordings (Multiclamp 700B, Molecular Devices) were performed on OECs, identified by tdTomato expression (excited at 543 nm, emission 553–618 nm), and the distinct localization in the ONL (Au et al., 2002). Recordings were digitized (Digidata 1440A, Molecular Devices) at 10–20 kHz and filtered (Bessel filter 2 kHz). The holding potential was −80 mV, achieved by current injection of −35.14 ± 13.5 pA on average. Patch pipettes had a resistance of 4–7 MΩ when filled with internal solution containing (mM): 105 K-gluconate, 30 KCl, 10 HEPES, 10 phosphocreatine, 4 Mg-ATP, 0.3 Na-GTP, 0.3 EGTA, and pH = 7.43. For visualization of the recorded OEC, ATTO-488 carboxy (20 μM, ATTO-Tech GmbH, Siegen, Germany) was added to the internal solution before the experiments. Agonists were applied via the perfusion system for 30 s. Antagonists were applied via the perfusion system 10 min prior to agonist application. The flow rate of the perfusion system amounted to approximately 2 mL/min. The experimental bath was oval in shape and had a diameter of 2.1 cm, the bath volume amounted to 1 mL. Therefore, agonist and antagonist concentrations continuously increased in the experimental bath, until the final concentration is reached. Vice versa, agonists were slowly washed out, accounting for long lasting effects. Kainate-induced inward currents in OECs were analyzed by measuring amplitude the currents.
Ca2+ Imaging and Analysis
For Ca2+ imaging experiments, GLAST-CreERT2 × tdTomatofl/fl and NMRI mice were used. Whole OBs were glued onto coverslips, transferred into a recording chamber and the coverslip secured with a U-shaped platinum wire. For multi-cell bolus loading (Stosiek et al., 2003), a glass pipette with a resistance of ∼3.5 MΩ (when filled with ACSF) was filled with 200 μM Fluo-8 AM (Thermo Fisher Scientific, Darmstadt, Germany) in ACSF, made from a 4 mM stock solution (dissolved in DMSO and 20% pluronic acid). After inserting the injection pipette into the ONL, the Ca2+ indicator was pressure-injected with 0.7 bar for 30 s into the tissue (PDES-01 AM, NPI electronic GmbH, Tamm, Germany), followed by an incubation of 20 min. Changes in the cytosolic Ca2+ concentration in OECs were detected by the fluorescence of Fluo-8 (excitation: 488 nm; emission: 500–530 nm) using a confocal microscope (eC1, Nikon, Düsseldorf, Germany). Images were acquired at a time rate of one frame every 3 s. A similar perfusion system and flow rate was used as described above. However, the experimental bath for Ca2+ imaging experiments was differently shaped (diameter 1 cm, volume 1.9 mL) resulting in differences in the kinetics of wash-in and wash-out of drugs between the electrophysiological and Ca2+ imaging experiments. To analyze changes in cytosolic Ca2+ in single cell somata, regions of interest (ROIs) were defined using Nikon EZ-C1 3.90 software. OECs were identified by GLAST promoter-driven tdTomato expression in GLAST-CreERT2 × tdTomatofl/fl mice in most experiments. In wild type mice, OECs were identified by their distinct localization in the ONL. The changes in Ca2+ were recorded throughout the experiments as relative changes in Fluo-8 fluorescence (ΔF) with respect to the resting fluorescence, which was normalized to 100%. Quantification of the Ca2+ transients was achieved by calculating the amplitude of ΔF.
Statistics
All values are stated as mean values ± standard error of the mean. The number of experiments is given as n = x, where x is the number of analyzed cells. At least 3 animals were analyzed for each set of experiments. Statistical significance was estimated by comparing three means using Friedmann ANOVA and the Wilcoxon post hoc test for paired data, and for comparing two means using the Mann–Whitney U-Test, with the error probability p (∗ p ≤ 0.05; ∗∗ p ≤ 0.01; ∗∗∗ p ≤ 0.001).
Immunohistochemistry
Immunohistochemistry on OBs of PLP-CreERT2 × tdTomatofl/fl mice (≥P28) was performed as described before (Droste et al., 2017). After dissection, the OBs were kept for 1 h at room temperature (RT) in formaldehyde (4% in PBS, pH 7.4). Afterward, 100 μm thick sagittal slices were prepared with a vibratome (VT1000S, Leica, Nußloch, Germany) and incubated for 1 h in blocking solution (10% normal goat serum (NGS), 0.5% Triton X-100 in PBS) at RT. Subsequently, the slices were incubated for 48 h at 4°C with the following primary antibodies: Guinea pig anti-GluA1 (Alomone labs; 1:200); rabbit anti-GluA2 (Millipore, 1:200); rabbit anti-GluA4 (Millipore; 1:200). To validate the specificity of the GluA antibodies we used cerebellar slices as control, since the distribution of GluA subunits is well documented in this brain area (Supplementary Figure S1). Moreover, the antibodies against GluA1 and GluA4 have been validated in glia-specific GluA1 and GluA4 double knockout mice before (Saab et al., 2012). In our control experiments, the GluA2 antibody only labeled cells known to express GluA2, but not cells that lack GluA2 such as Bergmann glial cells (Burnashev et al., 1992; Muller et al., 1992; Saab et al., 2012), as shown before for the used antibody (Droste et al., 2017). Hence, we consider the used antibodies as efficient and specific. The antibodies were diluted in 1% NGS, 0.05% TritonX-100 in PBS. Slices were incubated in PBS with the following secondary antibodies for 24 h at 4°C: goat anti-rabbit Alexa Fluor 488 (Thermo Fisher Scientific; 1:1000) or goat anti-guinea pig Alexa Fluor 488 (Thermo Fisher Scientific; 1:1000). Moreover, Hoechst 33342 (5 μM; Molecular Probes, Eugene, OR, United States) was added to stain nuclei. Slices were mounted on slides using self-hardening embedding medium (Immu-Mount, Thermo Fisher Scientific). Immunohistological stainings were analyzed using a confocal microscope (Nikon eC1). Confocal images were adjusted for brightness and contrast using ImageJ and Adobe Photoshop CS6.
Results
Distribution of GluA Subunits in the ONL
AMPA receptors constitute of four subunits (GluA1-GluA4), which form complex heteromeric cation channels, activated by glutamate or selective receptor agonists such as AMPA and kainate (Steinhauser and Gallo, 1996). The composition of the subunits is especially critical to determine the Ca2+ permeability of the channel. Thus, GluA2-lacking AMPA receptors show a high permeability for Ca2+ whereas GluA2-containing AMPA receptors are impermeable for Ca2+ (Hollmann et al., 1991). The OB is divided into several clearly distinguishable layers (Figures 1A,B) and immunoreactivity against GluA1, GluA2/3, and GluA4 demonstrated a wide distribution of these subunits in all layers, while mRNA expression analyses showed low amounts of GluA3 in the OB (Montague and Greer, 1999; Horning et al., 2004). We aimed to analyze the distribution of GluA1, GluA2, and GluA4 specifically in the ONL by immunohistochemistry using PLP-CreERT2 × tdTomatofl/fl mice. The plp gene encodes for the PLP, expressed by oligodendrocytes, and its slice variant DM20, expressed by oligodendrocytes and OECs, enabling the specific identification of oligodendrocytes and OECs by Cre-recombinase-driven tdTomato expression (Griffiths et al., 1995; Dickinson et al., 1997). OECs are present exclusively in the ONL, whereas oligodendrocytes are located in the glomerular layer (GL) and deeper layers but not in the ONL (Figures 1C,D; Doucette, 1990, 1991). Therefore, PLP-CreERT2-driven tdTomato expression in the ONL is assumed to be restricted to OECs (Figures 1C,D; Beiersdorfer et al., 2019; Piantanida et al., 2019). GluA1 immunoreactivity was widely distributed in the entire ONL, colocalizing intensely with tdTomato-expressing OECs (Figures 1E,F). Homogenous GluA2 immunoreactivity was detected in the ONL, with scattered colocalization with tdTomato-positive OECs (Figures 1G,H). In the GL, GluA2-positive juxtaglomerular cells were present (Figure 1G, arrow; Droste et al., 2017). Additionally, GluA4 immunoreactivity was found in the ONL, however, it sparsely colocalized with tdTomato-positive OEC somata (Figures 1I,J). At higher magnification, GluA4 immunoreactivity was found in close approximation of tdTomato-expressing OECs, likely indicating GluA4 located in cell processes of OECs (Figure 1J, see arrowheads). The results suggest that OECs express GluA1 and GluA2, while GluA4 appears not to be present in OEC somata but presumably in cell processes.
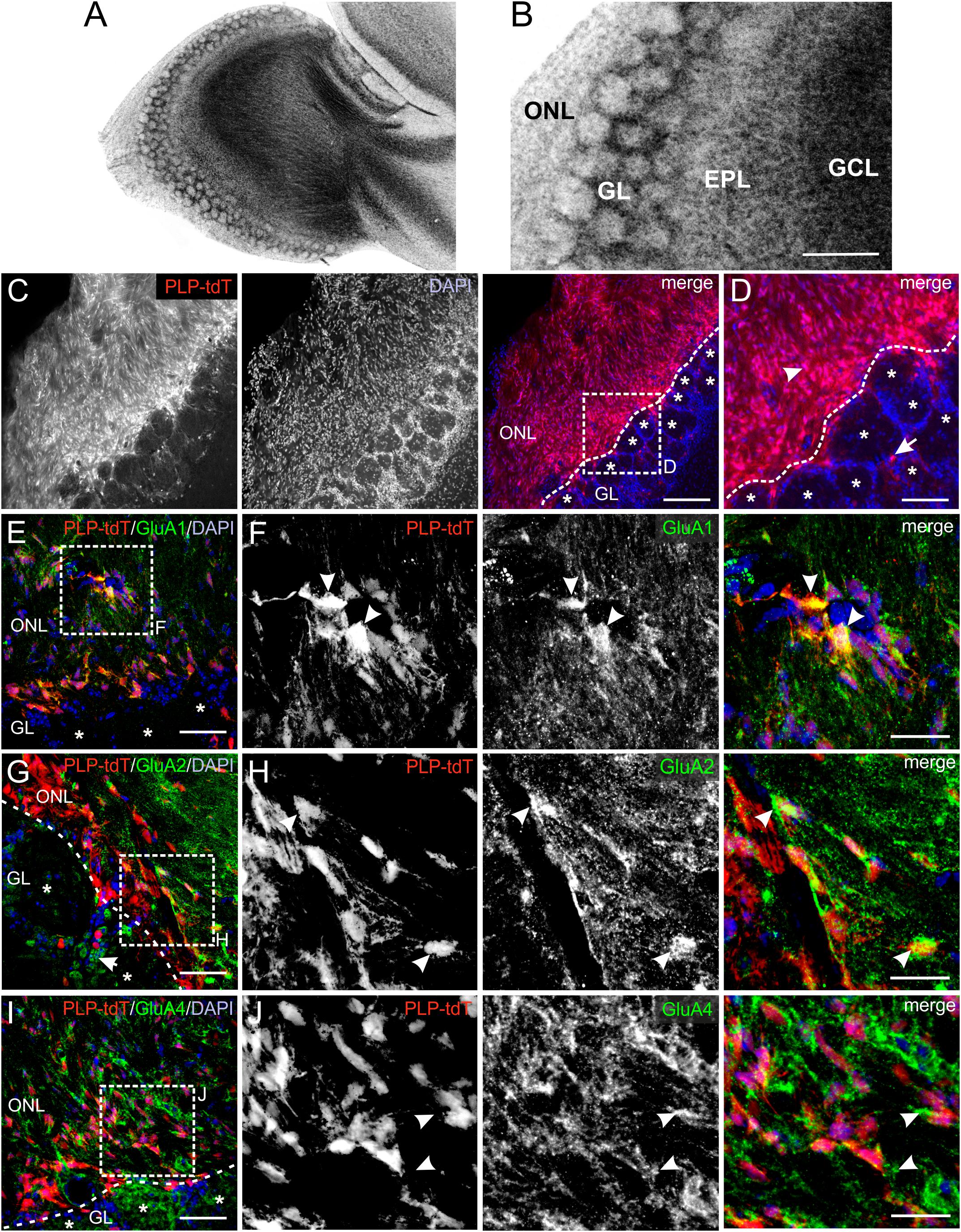
Figure 1. Distribution of AMPA receptor subunits in the olfactory nerve layer (ONL). (A) Laminar organization of the olfactory bulb. Nuclei were stained with Hoechst (5 μM). (B) The ONL, glomerular layer (GL), external plexiform layer (EPL) and granule cell layer (GCL) are clearly distinguishable. Scale bar: 200 μm. (C) Distribution of PLP-Cre-dependent tdTomato expression in the ONL and GL. Olfactory ensheathing cells (OECs) are located in the ONL, whereas tdTomato-expressing oligodendrocytes are located in the GL. Nuclei are stained with Dapi (5 μM; blue). The assumed border between the ONL and GL is indicated by the dotted line. Glomeruli are marked by asterisks. Scale bar: 150 μm. (D) Magnified view from (C). OECs are located in the ONL (arrowhead) and oligodendrocytes (arrow) in the GL visualized by PLP-Cre-dependent tdTomato expression (red). Scale bar: 50 μm. (E) Distribution of GluA1 immunoreactivity (green) in the ONL of PLP-CreERT2 × tdTomatofl/fl mice (red). Nuclei are stained with Dapi (blue). Glomeruli are marked by asterisks. Scale bar: 50 μm. (F) Magnified view from (E). GluA1 immunostaining (green) colocalize with tdTomato-expressing OECs (red) in the ONL (arrowheads). Scale bar: 25 μm. (G) Distribution of GluA2 immunoreactivity (green) in the ONL of PLP-CreERT2 × tdTomatofl/fl mice (red). GluA2 is widely distributed in the ONL, as well as in the GL. Nuclei are stained with Dapi (blue). Glomeruli are marked by asterisks. Scale bar: 50 μm. (H) Magnified view from (G). GluA2 immunoreactivity colocalizes with tdTomato-expressing OECs (red) in the ONL (arrowheads). Scale bar: 25 μm. (I) Distribution of GluA4 immunoreactivity (green) in the ONL of PLP-CreERT2 × tdTomatofl/fl mice (red). Nuclei are stained with Dapi (blue). Glomeruli are marked by asterisks. Scale bar: 50 μm. (J) Magnified view from (I). GluA4 immunoreactivity (green) is sparsely colocalized with tdTomato-positive OEC somata (red), but is localized adjacent to OEC somata (arrowheads). Scale bar: 25 μm.
Kainate Induces AMPA Receptor-Mediated Inward Currents in OECs
Functional AMPA/kainate receptors have been investigated in macroglial cells, namely astrocytes and oligodendrocyte precursor cells in gray and white matter as well as microglial cells (Burnashev et al., 1992; Muller et al., 1992; Jabs et al., 1994; Seifert and Steinhauser, 1995; Noda et al., 2000; Zonouzi et al., 2011; Hoft et al., 2014; Droste et al., 2017). Based on our immunohistochemical data, we suggested that OECs in the ONL might also express functional AMPA receptors. Therefore, we performed electrophysiological recordings in OECs in acute OB slices of GLAST-CreERT2 × tdTomatofl/fl mice. OECs were identified by GLAST promoter-driven tdTomato expression in the ONL (Figure 2A). Additionally, a voltage-step protocol was applied to characterize the I/V relationship of the recorded cell. All OECs analyzed showed a characteristic linear I/V relationship (Figures 2B,C; Rela et al., 2010; Beiersdorfer et al., 2019). We used kainate to agonize AMPA/kainate receptors, since kainate induced a non-desensitizing AMPA receptor-mediated inward current in acutely isolated hippocampal glial cells (Seifert and Steinhauser, 1995). Kainate application (100 μM, 30 s) induced a prominent slowly rising and long lasting inward current with a mean amplitude of 231.5 ± 58.9 pA (at −80 mV holding potential) in OECs (Figure 2D). To specifically isolate kainate-induced responses in OECs, all experiments were performed in the presence of the gap junction inhibitor carbenoxolone (CBX, 100 μM) to avoid intercellular panglial communication to juxtaglomerular astrocytes that respond to kainate and might interfere with recordings in OECs (Droste et al., 2017; Beiersdorfer et al., 2019). Additionally, neuronal activity was suppressed by inhibiting voltage-gated sodium channels by tetrodotoxin (TTX, 1 μM). Application of GYKI53655 (100 μM), a selective AMPA receptor antagonist, significantly reduced kainate-induced inward currents in OECs by 88.8 ± 3.2% of the control (n = 5; p = 0.022), suggesting that the kainate-induced response is mainly mediated by AMPA receptors in OECs (Figures 2D,E). To further characterize the AMPA receptor subtype involved in kainate-induced inward currents, GluA2-lacking Ca2+-permeable AMPA receptors were inhibited by the selective antagonist, NASPM (100 μM) (Koike et al., 1997). NASPM reduced kainate-mediated inward currents in OECs significantly by 63.9 ± 2.7% of the control (n = 6; p = 0.019), suggesting that OECs exhibit functional Ca2+-permeable AMPA receptors (Figures 2F,G).
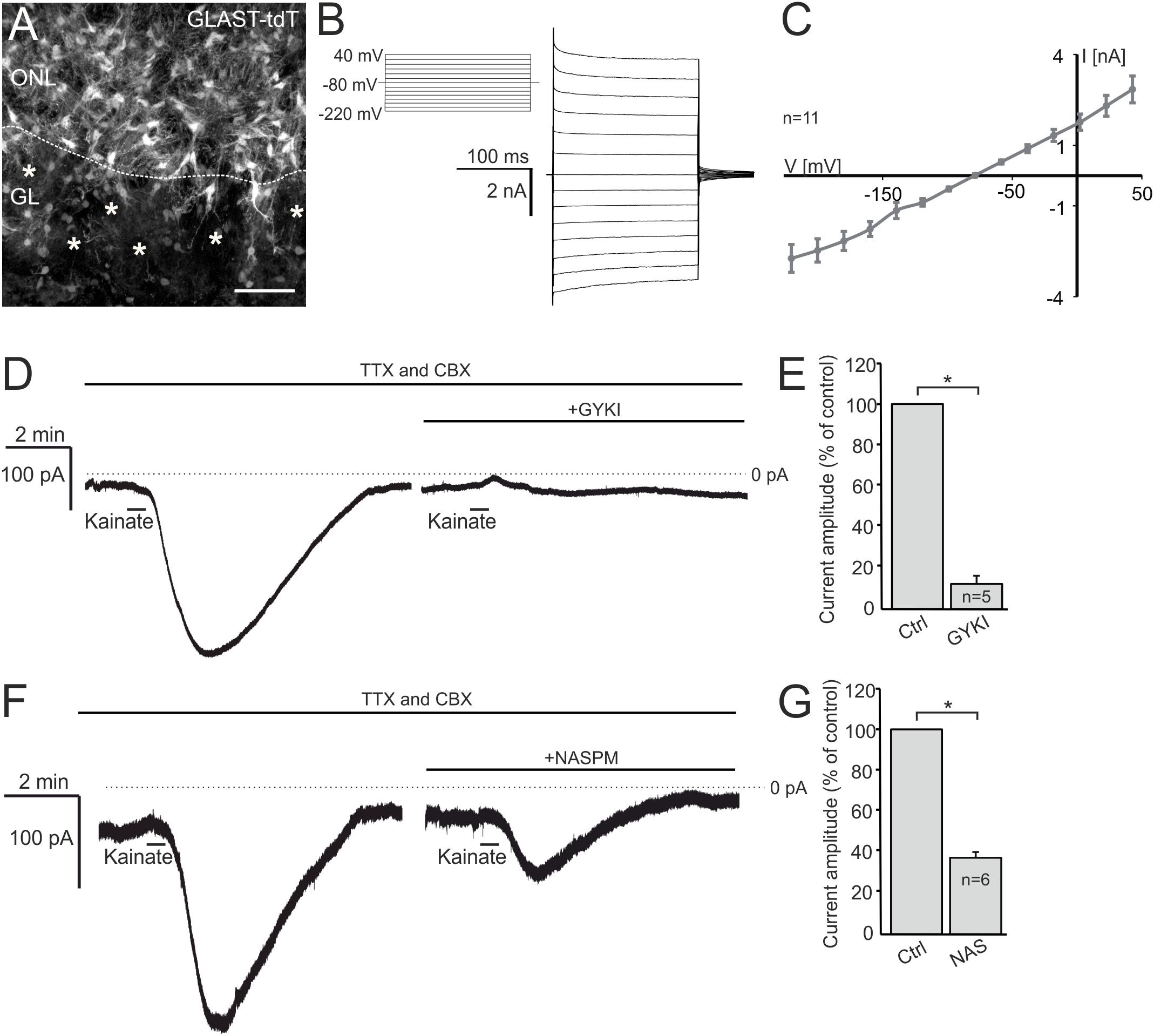
Figure 2. Kainate induces AMPA receptor-mediated inward currents in olfactory ensheathing cells (OECs). (A) Distribution of OECs in the olfactory nerve layer (ONL), identified by GLAST-CreERT2-driven tdTomato expression. Scale bar: 50 μm. (B) Voltage-step protocol applied to an OEC, recorded in whole cell voltage clamp mode. (C) All recorded OECs showed a linear current-voltage relationship. (D) The application of kainate (100 μM) induced an inward current in OECs, that was reduced by the selective AMPA receptor inhibitor GYKI 53655 (100 μM). All experiments were performed in the presence of TTX (1 μM) and CBX (150 μM). (E) Averaged amplitudes and statistical analysis of kainate-induced inward currents in OECs. (F) NASPM (100 μM), a selective inhibitor of Ca2+-permeable AMPA receptors, reduced kainate-induced inward currents significantly. (G) Averaged amplitudes and statistical analysis of kainate-induced inward currents in OECs. ∗p ≤ 0.05.
Kainate-Mediated Ca2+ Transients in OECs Depend on Extracellular Ca2+
Kainate-induced Ca2+ transients in Bergmann glial cells of the cerebellum result from Ca2+ influx directly through the AMPA receptor channel (Burnashev et al., 1992; Muller et al., 1992). However, AMPA/kainate receptors may also activate metabotropic pathways (Wang et al., 1997; Rozas et al., 2003; Takago et al., 2005). We performed confocal Ca2+ imaging experiments to investigate the source of Ca2+ that fuels Ca2+ transients in OECs in response to kainate application. The Ca2+ indicator Fluo-8 AM was pressure injected into OB in-toto preparations of GLAST-CreERT2 × tdTomatofl/fl mice (Figures 3A,B). Experiments were performed in the presence of CBX and TTX to suppress indirect effects. Kainate application induced Ca2+ transients in OECs with a mean amplitude of 107.8 ± 5.3% ΔF. GYKI 53655 (100 μM), an AMPA receptor antagonist, greatly reduced kainate-evoked Ca2+ transients in OECs by 84.0 ± 6.1% of the control (n = 27; p = 5.93 × 10–6), showing that kainate-evoked Ca2+ transients are mediated by AMPA receptors (Figures 3C,D). Removal of extracellular Ca2+ reduced kainate-induced Ca2+ transients by 91.9 ± 2.3% (n = 43; p = 1.16 × 10–8). Kainate-evoked Ca2+ responses recovered after readdition of extracellular Ca2+, the average amplitude reached 122.0 ± 18.3% of the control (p = 0.16) (Figures 3E,F). To estimate the impact of intracellular Ca2+ stores on kainate-induced Ca2+ transients in OECs, we applied cyclopiazonic acid (CPA, 20 μM) to inhibit Ca2+ uptake into Ca2+ stores via SERCA (sarcoplasmic/endoplasmic reticulum calcium ATPase). Kainate-induced Ca2+ transients were significantly reduced by 57.6 ± 4.2% upon Ca2+ store depletion (n = 70; p = 8.18 × 10–8) (Figures 3G,H). Our results suggest that Ca2+ influx via AMPA receptor channels is essential to trigger Ca2+ transients in OECs, whereas Ca2+ release from internal stores is a secondary effect as a result of the kainate-evoked Ca2+ influx. To verify that the AMPA-evoked membrane current and associated Ca2+ influx was not elicited by a preceding Ca2+ increase, we evoked a Ca2+ transient by application of ADP and recorded membrane currents. ADP induced a large Ca2+ increase but failed to elicit membrane currents, demonstrating that Ca2+-dependent membrane conductances are negligible in OECs (Supplementary Figure S2).
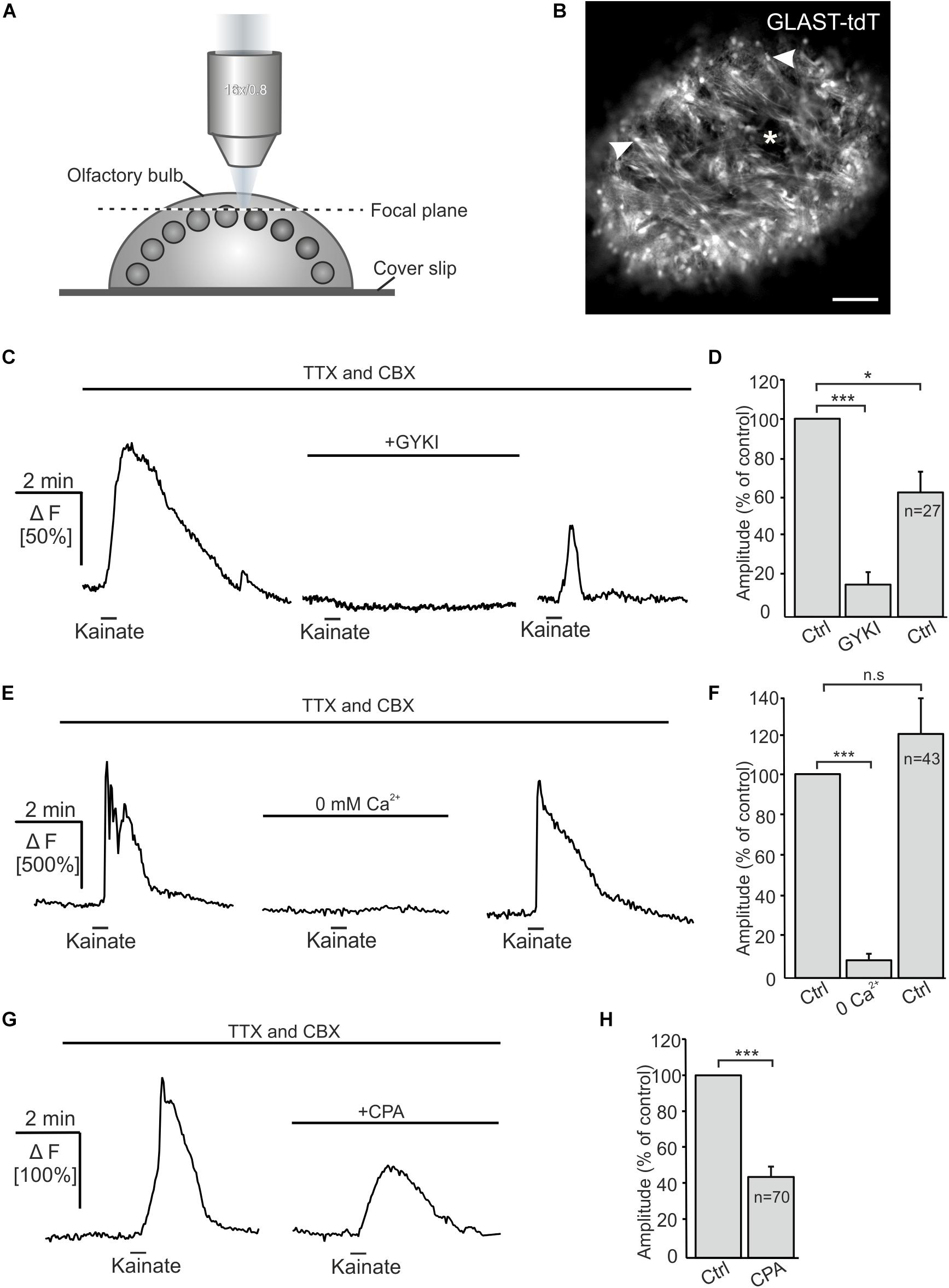
Figure 3. Kainate-induced Ca2+ transients in olfactory ensheathing cells (OECs) depend on extracellular Ca2+ and intracellular Ca2+ stores. (A) Schematic illustration of the experimental set up of Ca2+ imaging experiments. Olfactory bulb (OB) in-toto preparations of GLAST-CreERT2 × tdTomatofl/fl mice were fixed and placed under the confocal microscope. Fluo-8 was pressure injected into the tissue. The focal plane as seen in panel (B) is indicated by the dotted line. (B) Confocal image of tdTomato-expressing OECs in an OB in-toto preparation. Arrowheads highlight OEC somata located in the ONL. One glomerulus is indicated by an asterisk. Scale bar: 100 μm. (C) Kainate-induced Ca2+ transients in OECs in the presence of TTX (1 μM) and CBX (150 μM) were reduced by GYKI 53655 (100 μM). (D) GYKI 53655 significantly reduced kainate-mediated Ca2+ transients. (E) Withdrawal of extracellular Ca2+ abolished kainate-induced Ca2+ transients in OECs completely. Restitution of extracellular Ca2+ caused a recovery of kainate-induced Ca2+ transients in OECs. (F) Averaged amplitudes and statistical analysis of kainate-mediated Ca2+ transients. (G) Upon intracellular Ca2+ store depletion by CPA (20 μM) kainate-induced Ca2+ transients were significantly reduced. (H) Averaged amplitudes and statistical analysis of the effect of CPA on kainate-mediated Ca2+ transients. ∗∗∗p ≤ 0.001; ∗p ≤ 0.05. n.s.: not significant.
Endogenous Glutamate Release Induces AMPA Receptor-Mediated Ca2+ Transients in OECs
Electrical stimulation of OSN axons evokes ectopic release of glutamate and ATP, which initiates mGluR1 and P2Y1 receptor-mediated Ca2+ rises in OECs (Berkowicz et al., 1994; Rieger et al., 2007; Thyssen et al., 2010). Here, we showed that OECs express functional Ca2+-permeable AMPA receptors which may also contribute to Ca2+ transients in response to neuronal activity. We endogenously released glutamate upon electrical stimulation of OSN axons (20 Hz, 2 s) and recorded Ca2+ transients in Fluo-8-loaded OECs in wild type animals. Electrical stimulation of OSN axons induced Ca2+ transients in OECs with an amplitude of 51.8 ± 5.0% ΔF (n = 46). By eliminating purinergic (MRS2179), dopaminergic (SCH23390) and mGluR1-mediated Ca2+ transients (CPCCOEt) as well as possible indirect NMDA-mediated neuronal responses (D-APV), the mean amplitude of stimulation-induced Ca2+ transients in OECs were significantly reduced to 61.0 ± 9.3% of the control (p = 3.68 × 10–4). Additional inhibition of Ca2+-permeable AMPA receptors by the selective antagonist NASPM further reduced the mean amplitude to 30.6 ± 5.8 (p = 1.36 × 10–5) of the control. NBQX almost completely abolished stimulation-induced Ca2+ transients in OECs and the amplitude further decreased significantly to 13.01 ± 3.3% of the control (p = 0.002). Receptor inhibition was reversible and stimulation-induced Ca2+ transients in OECs recovered after wash out (Figure 4). The results indicate that endogenous glutamate release triggers AMPA receptor-mediated Ca2+ responses in OECs, suggesting a possible role for AMPA receptors in axon-OEC communication.
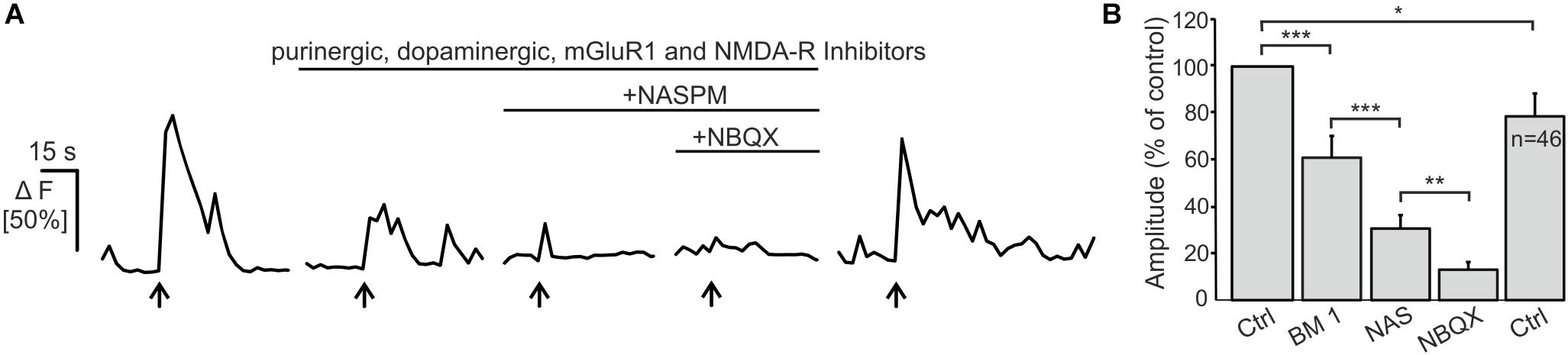
Figure 4. Electrical stimulation of OSN axons evokes AMPA-mediated Ca2+ transients in olfactory ensheathing cells (OECs).(A) Electrical stimulation of OSN axons evoked Ca2+ transients in OECs in control conditions (ACSF), and in the presence of purinergic (MRS2179, 100 μM; ZM241385; 0.5 μM), dopaminergic (SCH23390; 5 μM) an glutamatergic (CPCCOEt, 100 μM; D-APV, 100 μM) receptor antagonists. The additional application of NASPM (100 μM) further reduced Ca2+ transients in OECs. NBQX (20 μM) abolished stimulation-induced Ca2+ transients. (B) Averaged amplitudes of stimulation-evoked Ca2+ transients in OECs. ∗∗∗p < 0.0001; ∗∗p < 0.01; ∗p < 0.05.
Discussion
In the present study, we investigated the role of AMPA receptors in OEC physiology and axon-OEC communication. Kainate induced inward currents and Ca2+ transients in OECs that were partially mediated by the GluA2-lacking Ca2+-permeable AMPA receptor subtype. Endogenous glutamate release initiated AMPA receptor-mediated Ca2+ transients in OECs, indicating the relevance of AMPA receptors for axon-OEC communication.
We showed clear GluA1 and GluA2 immunoreactivity in the ONL colocalized with tdTomato-expressing OECs, while GluA4 immunoreactivity rarely colocalized with tdTomato in OECs. However, in the present study tdTomato was predominantly localized in OEC somata, limiting the detection of OEC processes, in which GluA4 might be enriched, as it has been demonstrated in a study by Montague and Greer (1999), showing strong GluA4 immunoreactivity in cell processes of presumed olfactory nerve-associated glial cells. Therefore, the results of our and other studies suggest the presence of at least three of four GluA subunits in OECs.
While the presence of GluA2 in OECs argues against Ca2+ permeability of the AMPA receptors, our physiological data implies the involvement of Ca2+ influx via Ca2+-permeable AMPA receptors. AMPA receptor-dependent Ca2+ influx could either be conducted by Ca2+-permeable AMPA receptors or by voltage-gated Ca2+ channels activated by AMPA receptor-mediated depolarization (Porter and McCarthy, 1995). However, OECs do not generate voltage-dependent Ca2+ influx, e.g., via voltage-gated Ca2+ channels, ruling out that a depolarization by AMPA receptors is causative for the kainate-evoked Ca2+ signals (Thyssen et al., 2010). In addition, both kainate-evoked membrane currents and Ca2+ transients were largely reduced by NASPM, a selective blocker of Ca2+-permeable AMPA receptors (Koike et al., 1997). Hence, taking into account that on the one hand side OECs show immunoreactivity to the GluA2 subunit and on the other hand side the current and Ca2+ responses are NASPM-sensitive, the results suggest that OECs express both Ca2+-permeable as well as Ca2+-impermeable AMPA receptors. This is confirmed by the NASPM-insensitive but GYKI-sensitive fraction of kainate-evoked inward currents. In addition to Ca2+ influx via Ca2+-permeable AMPA receptors, Ca2+ release from internal stores contributes to AMPA receptor-mediated Ca2+ transients. OECs generate prominent Ca2+-induced Ca2+ release (CICR) and even a small Ca2+ increase generates a fast and large Ca2+ transient by CICR (Stavermann et al., 2012). Thus, Ca2+ influx through AMPA receptors upon kainate application could induce CICR that boosts the Ca2+ increase and accounts for the fast rising kinetics of the Ca2+ signal as compared to the rising phase of the kainate-evoked membrane current. We conclude that Ca2+ influx is the initial Ca2+ signal that triggers subsequent internal Ca2+ release such as CICR, as shown by the entire suppression of the Ca2+ signal by external Ca2+ withdrawal but only partial reduction upon Ca2+ store depletion.
Ca2+ signaling in OECs may serve different functions. In other glial cells such as astrocytes, increases in the cytosolic Ca2+ concentration has been reported to evoke release of so-called gliotransmitters that affect nearby neurons and synapses, but also release of vasoactive substances such as prostaglandins and arachidonic acid (Bazargani and Attwell, 2016; Guerra-Gomes et al., 2017). OECs enwrap blood vessels in the ONL (Herrera et al., 2005) and respond to neurotransmitters extrasynaptically released from axons of OSN with Ca2+ signals (Rieger et al., 2007; Thyssen et al., 2010; this study). Increases in intracellular Ca2+ in OECs results in constriction of adjacent capillaries (Thyssen et al., 2010). Hence, Ca2+ signals evoked by glutamate release from axons in the ONL and subsequent activation of Ca2+-permeable AMPA receptors may lead to vasoresponses that adjust blood flow to the metabolic demand upon action potential firing, a mechanism termed neurovascular coupling.
In summary, our results show that release of glutamate from axons of OSN results in Ca2+ transients in OECs that are partially mediated by AMPA receptors. AMPA receptor-mediated Ca2+ transients were due to Ca2+ influx, most likely through Ca2+-permeable AMPA receptors themselves, and subsequent Ca2+ release from internal Ca2+ stores.
Data Availability Statement
The datasets generated for this study are available on request to the corresponding authors.
Ethics Statement
The animal study was reviewed and approved by GZ G21305/591-00.33; Behörde für Gesundheit und Verbraucherschutz, Hamburg, Germany.
Author Contributions
AB and CL designed the experiments and wrote the manuscript. AB performed the experiments and analyzed the data.
Funding
This work was supported by the Deutsche Forschungsgemeinschaft (LO779/10 and SFB 1328 TP-A07).
Conflict of Interest
The authors declare that the research was conducted in the absence of any commercial or financial relationships that could be construed as a potential conflict of interest.
Acknowledgments
We thank A. C. Rakete and M. Fink for technical assistance. We also thank A. Scheller and F. Kirchhoff for providing transgenic animals.
Supplementary Material
The Supplementary Material for this article can be found online at: https://www.frontiersin.org/articles/10.3389/fncel.2019.00451/full#supplementary-material
FIGURE S1 | Control stainings of the cerebellum to validate the specificity of anti-GluA antibodies. GluA1 was detected in Purkinje neurons (arrowhead) and Bergmann glial cells (arrow), GluA2 in Purkinje neurons and GluA4 in Bergmann glial cells, in line with published data. Scale bars: 50 μm.
FIGURE S2 | ADP evokes Ca2+ transients but not membrane currents. (A) The application of kainate (100 μM) induced an inward current in OECs, whereas the application of ADP (100 μM) did not. (B) Average current amplitudes of kainate and ADP-induced currents in OECs. (C) Application of kainate and ADP induced Ca2+ transients in OECs. (D) Average amplitudes of kainate- and ADP-evoked Ca2+ transients.
References
Au, W. W., Treloar, H. B., and Greer, C. A. (2002). Sublaminar organization of the mouse olfactory bulb nerve layer. J. Comp. Neurol. 446, 68–80. doi: 10.1002/cne.10182
Bazargani, N., and Attwell, D. (2016). Astrocyte calcium signaling: the third wave. Nat. Neurosci. 19, 182–189. doi: 10.1038/nn.4201
Beiersdorfer, A., Scheller, A., Kirchhoff, F., and Lohr, C. (2019). Panglial gap junctions between astrocytes and olfactory ensheathing cells mediate transmission of Ca(2+) transients and neurovascular coupling. Glia 67, 1385–1400. doi: 10.1002/glia.23613
Belkacemi, T., Niermann, A., Hofmann, L., Wissenbach, U., Birnbaumer, L., Leidinger, P., et al. (2017). TRPC1- and TRPC3-dependent Ca(2+) signaling in mouse cortical astrocytes affects injury-evoked astrogliosis in vivo. Glia 65, 1535–1549. doi: 10.1002/glia.23180
Berkowicz, D. A., Trombley, P. Q., and Shepherd, G. M. (1994). Evidence for glutamate as the olfactory receptor cell neurotransmitter. J. Neurophysiol. 71, 2557–2561. doi: 10.1152/jn.1994.71.6.2557
Burnashev, N., Khodorova, A., Jonas, P., Helm, P. J., Wisden, W., Monyer, H., et al. (1992). Calcium-permeable AMPA-kainate receptors in fusiform cerebellar glial cells. Science 256, 1566–1570. doi: 10.1126/science.1317970
Cao, L., Zhu, Y. L., Su, Z., Lv, B., Huang, Z., Mu, L., et al. (2007). Olfactory ensheathing cells promote migration of Schwann cells by secreted nerve growth factor. Glia 55, 897–904. doi: 10.1002/glia.20511
Carmignoto, G., and Gomez-Gonzalo, M. (2010). The contribution of astrocyte signalling to neurovascular coupling. Brain Res. Rev. 63, 138–148. doi: 10.1016/j.brainresrev.2009.11.007
Crandall, J. E., Dibble, C., Butler, D., Pays, L., Ahmad, N., Kostek, C., et al. (2000). Patterning of olfactory sensory connections is mediated by extracellular matrix proteins in the nerve layer of the olfactory bulb. J. Neurobiol. 45, 195–206. doi: 10.1002/1097-4695(200012)45:4<195::aid-neu1>3.0.co;2-y
Deitmer, J. W., Verkhratsky, A. J., and Lohr, C. (1998). Calcium signalling in glial cells. Cell Calcium 24, 405–416. doi: 10.1016/s0143-4160(98)90063-x
Dickinson, P. J., Griffiths, I. R., Barrie, J. M., Kyriakides, E., Pollock, G. F., and Barnett, S. C. (1997). Expression of the dm-20 isoform of the plp gene in olfactory nerve ensheathing cells: evidence from developmental studies. J. Neurocytol. 26, 181–189. doi: 10.1023/a:1018584013739
Doucette, J. R. (1984). The glial cells in the nerve fiber layer of the rat olfactory bulb. Anat. Rec. 210, 385–391. doi: 10.1002/ar.1092100214
Doucette, R. (1990). Glial influences on axonal growth in the primary olfactory system. Glia 3, 433–449. doi: 10.1002/glia.440030602
Doucette, R. (1991). PNS-CNS transitional zone of the first cranial nerve. J. Comp. Neurol. 312, 451–466. doi: 10.1002/cne.903120311
Droste, D., Seifert, G., Seddar, L., Jadtke, O., Steinhauser, C., and Lohr, C. (2017). Ca(2+)-permeable AMPA receptors in mouse olfactory bulb astrocytes. Sci. Rep. 7:44817. doi: 10.1038/srep44817
Flavell, S. W., and Greenberg, M. E. (2008). Signaling mechanisms linking neuronal activity to gene expression and plasticity of the nervous system. Annu. Rev. Neurosci. 31, 563–590. doi: 10.1146/annurev.neuro.31.060407.125631
Graziadei, G. A., and Graziadei, P. P. (1979). Neurogenesis and neuron regeneration in the olfactory system of mammals. II. Degeneration and reconstitution of the olfactory sensory neurons after axotomy. J. Neurocytol. 8, 197–213. doi: 10.1007/bf01175561
Griffiths, I. R., Dickinson, P., and Montague, P. (1995). Expression of the proteolipid protein gene in glial cells of the post-natal peripheral nervous system of rodents. Neuropathol. Appl. Neurobiol. 21, 97–110. doi: 10.1111/j.1365-2990.1995.tb01035.x
Guerra-Gomes, S., Sousa, N., Pinto, L., and Oliveira, J. F. (2017). Functional roles of astrocyte calcium elevations: from synapses to behavior. Front. Cell Neurosci. 11:427. doi: 10.3389/fncel.2017.00427
Hayat, S., Wigley, C. B., and Robbins, J. (2003). Intracellular calcium handling in rat olfactory ensheathing cells and its role in axonal regeneration. Mol. Cell. Neurosci. 22, 259–270. doi: 10.1016/s1044-7431(03)00051-4
Haydon, P. G. (2001). GLIA: listening and talking to the synapse. Nat. Rev. Neurosci. 2, 185–193. doi: 10.1038/35058528
Herrera, L. P., Casas, C. E., Bates, M. L., and Guest, J. D. (2005). Ultrastructural study of the primary olfactory pathway in Macaca fascicularis. J. Comp. Neurol. 488, 427–441. doi: 10.1002/cne.20588
Hoft, S., Griemsmann, S., Seifert, G., and Steinhauser, C. (2014). Heterogeneity in expression of functional ionotropic glutamate and GABA receptors in astrocytes across brain regions: insights from the thalamus. Philos. Trans. R. Soc. Lond. B Biol. Sci. 369, 20130602. doi: 10.1098/rstb.2013.0602
Hollmann, M., Hartley, M., and Heinemann, S. (1991). Ca2+ permeability of KA-AMPA–gated glutamate receptor channels depends on subunit composition. Science 252, 851–853. doi: 10.1126/science.1709304
Horning, M. S., Kwon, B., Blakemore, L. J., Spencer, C. M., Goltz, M., Houpt, T. A., et al. (2004). Alpha-amino-3-hydroxy-5-methyl-4-isoxazolepropionate receptor subunit expression in rat olfactory bulb. Neurosci. Lett. 372, 230–234. doi: 10.1016/j.neulet.2004.09.044
Jabs, R., Kirchhoff, F., Kettenmann, H., and Steinhauser, C. (1994). Kainate activates Ca(2+)-permeable glutamate receptors and blocks voltage-gated K+ currents in glial cells of mouse hippocampal slices. Pflugers. Arch. 426, 310–319. doi: 10.1007/bf00374787
Koike, M., Iino, M., and Ozawa, S. (1997). Blocking effect of 1-naphthyl acetyl spermine on Ca(2+)-permeable AMPA receptors in cultured rat hippocampal neurons. Neurosci. Res. 29, 27–36. doi: 10.1016/s0168-0102(97)00067-9
Lalo, U., Pankratov, Y., Kirchhoff, F., North, R. A., and Verkhratsky, A. (2006). NMDA receptors mediate neuron-to-glia signaling in mouse cortical astrocytes. J. Neurosci. 26, 2673–2683. doi: 10.1523/JNEUROSCI.4689-05.2006
Leone, D. P., Genoud, S., Atanasoski, S., Grausenburger, R., Berger, P., Metzger, D., et al. (2003). Tamoxifen-inducible glia-specific Cre mice for somatic mutagenesis in oligodendrocytes and Schwann cells. Mol. Cell. Neurosci. 22, 430–440. doi: 10.1016/s1044-7431(03)00029-0
Lohr, C., Grosche, A., Reichenbach, A., and Hirnet, D. (2014). Purinergic neuron-glia interactions in sensory systems. Pflugers Arch. 466, 1859–1872. doi: 10.1007/s00424-014-1510-6
Madisen, L., Zwingman, T. A., Sunkin, S. M., Oh, S. W., Zariwala, H. A., Gu, H., et al. (2010). A robust and high-throughput Cre reporting and characterization system for the whole mouse brain. Nat. Neurosci. 13, 133–140. doi: 10.1038/nn.2467
McConkey, D. J., and Orrenius, S. (1997). The role of calcium in the regulation of apoptosis. Biochem. Biophys. Res. Commun. 239, 357–366. doi: 10.1006/bbrc.1997.7409
Mishra, A., Reynolds, J. P., Chen, Y., Gourine, A. V., Rusakov, D. A., and Attwell, D. (2016). Astrocytes mediate neurovascular signaling to capillary pericytes but not to arterioles. Nat. Neurosci. 19, 1619–1627. doi: 10.1038/nn.4428
Montague, A. A., and Greer, C. A. (1999). Differential distribution of ionotropic glutamate receptor subunits in the rat olfactory bulb. J. Comp. Neurol. 405, 233–246. doi: 10.1002/(sici)1096-9861(19990308)405:2<233:aid-cne7<3.0.co;2-a
Mori, T., Tanaka, K., Buffo, A., Wurst, W., Kuhn, R., and Gotz, M. (2006). Inducible gene deletion in astroglia and radial glia–a valuable tool for functional and lineage analysis. Glia 54, 21–34. doi: 10.1002/glia.20350
Muller, T., Moller, T., Berger, T., Schnitzer, J., and Kettenmann, H. (1992). Calcium entry through kainate receptors and resulting potassium-channel blockade in Bergmann glial cells. Science 256, 1563–1566. doi: 10.1126/science.1317969
Noda, M., Nakanishi, H., Nabekura, J., and Akaike, N. (2000). AMPA-kainate subtypes of glutamate receptor in rat cerebral microglia. J. Neurosci. 20, 251–258. doi: 10.1523/jneurosci.20-01-00251.2000
Piantanida, A. P., Acosta, L. E., Brocardo, L., Capurro, C., Greer, C. A., and Rela, L. (2019). Selective Cre-mediated gene deletion identifies connexin 43 as the main connexin channel supporting olfactory ensheathing cell networks. J. Comp. Neurol. 527, 1278–1289. doi: 10.1002/cne.24628
Porter, J. T., and McCarthy, K. D. (1995). GFAP-positive hippocampal astrocytes in situ respond to glutamatergic neuroligands with increases in [Ca2+]i. Glia 13, 101–112. doi: 10.1002/glia.440130204
Rakers, C., Schmid, M., and Petzold, G. C. (2017). TRPV4 channels contribute to calcium transients in astrocytes and neurons during peri-infarct depolarizations in a stroke model. Glia 65, 1550–1561. doi: 10.1002/glia.23183
Rela, L., Bordey, A., and Greer, C. A. (2010). Olfactory ensheathing cell membrane properties are shaped by connectivity. Glia 58, 665–678. doi: 10.1002/glia.20953
Rieger, A., Deitmer, J. W., and Lohr, C. (2007). Axon-glia communication evokes calcium signaling in olfactory ensheathing cells of the developing olfactory bulb. Glia 55, 352–359. doi: 10.1002/glia.20460
Rozas, J. L., Paternain, A. V., and Lerma, J. (2003). Noncanonical signaling by ionotropic kainate receptors. Neuron 39, 543–553. doi: 10.1016/s0896-6273(03)00436-7
Rungta, R. L., Bernier, L. P., Dissing-Olesen, L., Groten, C. J., LeDue, J. M., Ko, R., et al. (2016). Ca(2+) transients in astrocyte fine processes occur via Ca(2+) influx in the adult mouse hippocampus. Glia 64, 2093–2103. doi: 10.1002/glia.23042
Saab, A. S., Neumeyer, A., Jahn, H. M., Cupido, A., Simek, A. A., Boele, H. J., et al. (2012). Bergmann glial AMPA receptors are required for fine motor coordination. Science 337, 749–753. doi: 10.1126/science.1221140
Schipke, C. G., Ohlemeyer, C., Matyash, M., Nolte, C., Kettenmann, H., and Kirchhoff, F. (2001). Astrocytes of the mouse neocortex express functional N-methyl-D-aspartate receptors. FASEB J. 15, 1270–1272. doi: 10.1096/fj.00-0439fje
Seifert, G., and Steinhauser, C. (1995). Glial cells in the mouse hippocampus express AMPA receptors with an intermediate Ca2+ permeability. Eur. J. Neurosci. 7, 1872–1881. doi: 10.1111/j.1460-9568.1995.tb00708.x
Singaravelu, K., Lohr, C., and Deitmer, J. W. (2006). Regulation of store-operated calcium entry by calcium-independent phospholipase A2 in rat cerebellar astrocytes. J. Neurosci. 26, 9579–9592. doi: 10.1523/JNEUROSCI.2604-06.2006
Stavermann, M., Buddrus, K., St John, J. A., Ekberg, J. A., Nilius, B., Deitmer, J. W., et al. (2012). Temperature-dependent calcium-induced calcium release via InsP3 receptors in mouse olfactory ensheathing glial cells. Cell Calcium 52, 113–123. doi: 10.1016/j.ceca.2012.04.017
Steinhauser, C., and Gallo, V. (1996). News on glutamate receptors in glial cells. Trends Neurosci. 19, 339–345. doi: 10.1016/0166-2236(96)10043-6
Stosiek, C., Garaschuk, O., Holthoff, K., and Konnerth, A. (2003). In vivo two-photon calcium imaging of neuronal networks. Proc. Natl. Acad. Sci. U.S.A. 100, 7319–7324. doi: 10.1073/pnas.1232232100
Su, Z., and He, C. (2010). Olfactory ensheathing cells: biology in neural development and regeneration. Prog. Neurobiol. 92, 517–532. doi: 10.1016/j.pneurobio.2010.08.008
Takago, H., Nakamura, Y., and Takahashi, T. (2005). G protein-dependent presynaptic inhibition mediated by AMPA receptors at the calyx of Held. Proc. Natl. Acad. Sci. U.S.A. 102, 7368–7373. doi: 10.1073/pnas.0408514102
Thyssen, A., Hirnet, D., Wolburg, H., Schmalzing, G., Deitmer, J. W., and Lohr, C. (2010). Ectopic vesicular neurotransmitter release along sensory axons mediates neurovascular coupling via glial calcium signaling. Proc. Natl. Acad. Sci. U.S.A. 107, 15258–15263. doi: 10.1073/pnas.1003501107
Toth, A. B., Hori, K., Novakovic, M. M., Bernstein, N. G., Lambot, L., and Prakriya, M. (2019). CRAC channels regulate astrocyte Ca(2+) signaling and gliotransmitter release to modulate hippocampal GABAergic transmission. Sci. Signal. 12:eaaw5450. doi: 10.1126/scisignal.aaw5450
Verkhratsky, A., Orkand, R. K., and Kettenmann, H. (1998). Glial calcium: homeostasis and signaling function. Physiol. Rev. 78, 99–141. doi: 10.1152/physrev.1998.78.1.99
Wakakura, M., and Yamamoto, N. (1994). Cytosolic calcium transient increase through the AMPA/kainate receptor in cultured Muller cells. Vision Res. 34, 1105–1109. doi: 10.1016/0042-6989(94)90293-3
Wang, Y., Small, D. L., Stanimirovic, D. B., Morley, P., and Durkin, J. P. (1997). AMPA receptor-mediated regulation of a Gi-protein in cortical neurons. Nature 389, 502–504. doi: 10.1038/39062
Woodhall, E., West, A. K., and Chuah, M. I. (2001). Cultured olfactory ensheathing cells express nerve growth factor, brain-derived neurotrophic factor, glia cell line-derived neurotrophic factor and their receptors. Brain Res. Mol. Brain Res. 88, 203–213. doi: 10.1016/s0169-328x(01)00044-4
Yang, H., He, B. R., and Hao, D. J. (2015). Biological roles of olfactory ensheathing cells in facilitating neural regeneration: a systematic review. Mol. Neurobiol. 51, 168–179. doi: 10.1007/s12035-014-8664-2
Keywords: olfactory ensheathing cells, AMPA receptor, calcium, patch clamp, GluA2
Citation: Beiersdorfer A and Lohr C (2019) AMPA Receptor-Mediated Ca2+ Transients in Mouse Olfactory Ensheathing Cells. Front. Cell. Neurosci. 13:451. doi: 10.3389/fncel.2019.00451
Received: 08 July 2019; Accepted: 20 September 2019;
Published: 04 October 2019.
Edited by:
Hajime Hirase, University of Copenhagen, DenmarkReviewed by:
Didier De Saint Jan, Centre National de la Recherche Scientifique (CNRS), FranceKoji Shibasaki, Gunma University, Japan
Copyright © 2019 Beiersdorfer and Lohr. This is an open-access article distributed under the terms of the Creative Commons Attribution License (CC BY). The use, distribution or reproduction in other forums is permitted, provided the original author(s) and the copyright owner(s) are credited and that the original publication in this journal is cited, in accordance with accepted academic practice. No use, distribution or reproduction is permitted which does not comply with these terms.
*Correspondence: Antonia Beiersdorfer, antonia.beiersdorfer@uni-hamburg.de; Christian Lohr, christian.lohr@uni-hamburg.de