Dynamic Calcium Release From Endoplasmic Reticulum Mediated by Ryanodine Receptor 3 Is Crucial for Oligodendroglial Differentiation
- Chongqing Key Laboratory of Neurobiology, Department of Histology and Embryology, Third Military Medical University, Chongqing, China
Increased intracellular Ca2+ in oligodendrocyte progenitor cells (OPCs) is important to initiate their differentiation, but the intracellular Ca2+ channel involved in this process remains unclear. As a Ca2+-induced Ca2+ release (CICR) channel that mediates endoplasmic reticulum (ER) Ca2+ release, the role of ryanodine receptors (RyRs) in oligodendroglial development is unexplored. In the present study, we observed that among the three mammalian isoforms, oligodendroglial lineage cells selectively expressed RyR3. Strong RyR3-positive signal was distributed all over the cytoplasm and processes in OPCs and/or immature OLs (imOLs), whereas it gradually decreased and was located mainly around the perinuclear region in mature oligodendrocytes (OLs). In addition, RyR3-mediated intracellular Ca2+ waves following caffeine stimulation were correlated with the expression pattern of RyR3, in which high flat Ca2+ fluctuations and oscillatory Ca2+ waves were more frequently recorded in OPCs and/or imOLs than in OLs. Through further functional exploration, we demonstrated that pretreatment with the RyR antagonist ryanodine could neutralize the increase in intracellular Ca2+ induced by OPC differentiation and reduce the number of mature OLs. Moreover, gene-level knockdown of RyR3 by lentivirus in OPCs resulted in inhibition of OPC differentiation. Taken together, our results provide new insight into the crucial role of RyR3-mediated ER Ca2+ release in the regulation of OPC differentiation and/or myelination.
Introduction
In the CNS, myelinating oligodendrocytes (OLs) originate from oligodendrocyte progenitor cells (OPCs) after passing through a series of distinct developmental stages, i.e., OPCs, immature OLs (imOLs) and mature OLs (Stangel and Hartung, 2002). As impairment of OL differentiation has been considered to be the major cause of remyelination failure, which occurs in numerous demyelination diseases such as multiple sclerosis (MS; Wolswijk, 1998; Chang et al., 2002; Kuhlmann et al., 2008), promoting OPC differentiation into mature OLs becomes a promising approach for myelin repair. However, the mechanism regulating oligodendroglial differentiation remains to be elucidated. As a critical functional pattern of non-excitable glia cells, Ca2+ signaling is essential for oligodendroglial differentiation and myelination (Kirischuk et al., 1995; Cohen et al., 1996; Yoo et al., 1999; Soliven, 2001; Fulton et al., 2010; Cheli et al., 2015; Friess et al., 2016; Baraban et al., 2018; Krasnow et al., 2018). For instance, inhibition of the voltage-operated Ca2+ entry in OPCs repressed their maturation and the myelin forming ability (Cheli et al., 2015). Increasing resting intracellular Ca2+ through membrane depolarization could facilitate MBP synthesis in OPCs (Friess et al., 2016). Newest studies further provided in vivo data showing that the Ca2+ transients in OLs could regulate retraction and elongation of the developing myelin sheath (Baraban et al., 2018; Krasnow et al., 2018). However, the Ca2+ channels involved in oligodendroglial differentiation is believed to be important but remains largely unexplored.
It is known that endoplasmic reticulum (ER) is the major intracellular Ca2+ pool (Meldolesi and Pozzan, 1998) and that ER Ca2+ release is driven mainly by inositol-1,4,5,-trisphosphate receptors (IP3Rs) and ryanodine receptors (RyRs; Koulen and Thrower, 2001). In OPCs, both IP3R2 and ryanodine receptor 3 (RyR3) can mediate highly localized Ca2+ release, of the types called “puffs” and “sparks”, respectively (Haak et al., 2001), but only IP3R2 is able to initiate Ca2+ waves under pharmacological treatments (Haak et al., 2001). However, the functions of those channels during oligodendroglial development remain unclear. Series of studies demonstrate that, compared with IP3Rs, the opening of which requires both Ca2+ and IP3 (Moraru et al., 1999; Foskett et al., 2007), RyRs are Ca2+-induced Ca2+ release (CICR) channels that can be triggered merely by a low concentration of Ca2+ (~1 μM; Meissner et al., 1986, 1997; Bezprozvanny et al., 1991), and this CICR function has been shown to powerfully amplify small inward Ca2+ currents in NG2 glial cells (Haberlandt et al., 2011). Therefore, we propose that RyR3 is likely a critical bridge for the formation of intracellular Ca2+ signaling and thus participates in the regulation of oligodendroglial development.
In the present study, we sought to characterize the expression and function of RyRs during OPC differentiation. Our results showed that RyR3 was selectively expressed and widely distributed in the soma and processes of the oligodendroglial lineage cells and that its expression level was downregulated following OPC differentiation. Using confocal Ca2+ imaging, we found that the ER Ca2+ release after caffeine stimulation was much stronger in OPCs and imOLs than in mature OLs. Moreover, inhibiting the function of RyR3 either pharmacologically or by gene knockdown suppressed the differentiation of OPCs. Our results revealed a critical role for RyR3-mediated Ca2+ signaling in oligodendroglial differentiation that may provide new insight into therapeutic approaches for demyelinating diseases.
Materials and Methods
OPC Culture
Cortical OPCs were purified as previously described (Niu et al., 2012b). Briefly, the mixed glial cells were isolated from cortex of postnatal day 1–3 neonatal Sprague-Dawley (SD) rats and enriched in OPC growth medium followed by two passages to enrich cell numbers. OPC proliferation medium was DMEM/F12 + 1% N2 supplement + PDGFAA. OPCs were induced to differentiate by replacing the medium with OPC differentiation medium: DMEM/F12 + 1% N2 supplement + 5 mg/mL N-acetyl-L-cysteine (Amresco) + 1% fetal bovine serum + 5 mg/mL insulin.
Reagents used were as follows: Dulbecco’s modified Eagle’s medium/F12 (DMEM/F12; Hyclone, SH30023), N2 supplement (Invitrogen, 17502048), fetal bovine serum (FBS; Hyclone, SV30087), insulin (Sigma, I6634), N-acetyl-l-cysteine (NAC; AMRESCO, 0LA0011), PDGFAA (Peprotech, 100-13A). The SD rats related procedures were performed in accordance with the guidelines approved by the Laboratory Animal Welfare and Ethics Committee of the Third Military Medical University (Niu et al., 2016).
Confocal Ca2+ Imaging Measurements
OPCs were grown and differentiated in glass-bottom dish and loaded with the fluorescent Ca2+ sensitive dye Fluo-3AM (5 μM, Invitrogen) for 20 min at 37°C in a modified imaging buffer containing (in mM): NaCl, 135; KCl, 3; MgCl2, 2; Glucose, 8; HEPES, 10 and CaCl2, 2 (pH adjusted to 7.4 with NaOH). The dye-loaded cells were washed twice and maintained for at least 20 min at RT in fresh imaging buffer to allow complete dye de-esterification. Ca2+ wave and fluorescence images were real-time recorded for at least 15 min in all experiments, using a confocal laser-scanning microscope (FluoView FV1000, Olympus, Japan) with the UplanFl40× objective (N.A. 0.95). The image acquisition frequency is 100–180 ms/image. Cell morphology was detected using differential interference contrast (DIC) under confocal microscopy. Ca2+ concentrations were measured by exciting Fluo-3 AM at 488 nm.
Intracellular Ca2+ responses in OPCs, imOLs and mature OLs were recorded with caffeine (20 mM, Sigma, Ca2+ free) stimulation in a Ca2+ free imaging buffer. For spontaneous Ca2+ recordings, OPCs were grown in proliferation medium or differentiation medium for 6 h with or without ryanodine treatment (50 μM, TOCRIS, 1329, 10 min). Fluo-3 loading and cell washing was followed by applying normal proliferating medium (Pro-medium) or differentiating medium (Diff-medium) for the following recording.
Cell Processing and Immunocytochemistry
Cells were grown on coverslips, fixed in cold 4% paraformaldehyde for 20 min, rinsed with 0.01 M PBS, blocked with 1% bovine serum albumin (BSA) and 0.2% Triton-X100 for 30 min and then incubated with primary antibodies diluted in 1% BSA overnight at 4°C and then by fluorophore-conjugated secondary antibodies at room temperature (RT) for 2 h. Cell nuclei were stained with 4′,6-diamidino-2-phenylindole (DAPI, Thermo Fisher) for 10 min.
In this study, the following antibodies were used: mouse polyclonal anti-Olig2 (1:500, Millipore, MABN50), rabbit polyclonal anti-RyR3 (1:200, Millipore, AB9082), and goat anti-myelin basic protein (MBP; 1:500, Santa Cruz, sc13914). The secondary antibodies were as follows: Alexa 568-labeled donkey anti-mouse (1:1000, Invitrogen), Alexa 488-labeled donkey anti-goat (1:1000, Invitrogen), Alexa 568-labeled donkey anti-goat (1:1000, Invitrogen), Alexa 568-labeled donkey anti-rabbit (1:1000, Invitrogen) and Cy5-labeled rabbit anti-mouse (1:500, Jackson ImmunoResearch).
Image Acquisition and Quantification
Fluorescent images were captured using an Axio Imager M2 fluorescence microscope (Zeiss, Oberkochen, Germany) or a confocal laser-scanning microscope (Olympus, IV 1000, Shinjuku, Tokyo) with excitation wavelengths appropriate for Alexa Fluor 488 (488 nm), 596 (568 nm), 647 (628 nm) or DAPI (380 nm). Digital images of the oligodendroglial lineage cells in the supplemental figure were acquired with an Olympus IX51 microscope with an Olympus C-7070 camera (Tokyo). For the statistical analysis, randomly selected images in at least three representative fields were acquired from each sample. Detection and quantification were performed using ImageJ software (National Institutes of Health, NIH).
Western Blot Analysis
The cells were lysed using RIPA lysis buffer (Beyotime, P0013B) with freshly added 1% phenylmethylsulfonyl fluoride (PMSF, Amresco, O754) solution. Protein concentration was determined using Coomassie brilliant Blue G-250. SDS-PAGE and Western blotting were carried out as reported previously (Niu et al., 2012a). Proteins were transferred to polyvinylidene difluoride (PVDF) membranes and visualized by chemiluminescence (ECL Plus, GE Healthcare, Marlborough, MA, USA) after incubation with the antibodies. β-actin was used as the loading control. Quantification of band intensity was performed using ImageJ software. The primary antibodies included the following: rabbit polyclonal anti-RyR3 (1:1000, Millipore), rabbit polyclonal anti-platelet-derived growth factor receptor α (PDGFRα; 1:1000, Santa Cruz, sc-338), mouse anti-2′,3′-cyclic nucleotide-3′-phosphodiesterase (CNPase; 1:1000, Abcam, ab6319), goat anti-MBP (1:1000, Santa Cruz) and mouse anti-β-actin (1:2000, Santa Cruz, sc-47778). The secondary antibodies included the following: goat anti-mouse-HRP (1:2000, Santa Cruz, sc-2094), goat anti-rabbit-HRP (1:2000, Santa Cruz, sc-2313) and rabbit anti-goat-HRP (1:2000, Santa Cruz, sc-2020).
RT-PCR Analysis
Total ribonucleic acid (RNA) was isolated from different stages of OPC cultures (OPC differentiated for 1 day, 2 days, or 4 days using TRIzol (Life Technologies). Real-time polymerase chain reaction (RT-PCR) was performed with the C1000 Touch™ Real-time PCR Detection System (Bio-Rad) and GoTaq® qPCR Master Mix (Promega, Sunnyvale, CA, USA). The amplification procedure and melt curve analysis were performed using three independent replicates for each sample.
The oligonucleotide primers used were as follows:
Lentivirus Mediated shRNA Interference
The shRNA lentivirus was purchased from Obio Technology, Shanghai. Targeted sequence in rat RyR3 gene is: AGATGCTAATTGCATCTC. The primary lentivirus solution was diluted in several gradient concentrations (1:10, 1:100, 1:300, 1:500, 1:1000) to check the best work concentration (normal oligodendroglial viability and good interference efficiency). The lentivirus was diluted 1:300 in the differentiation medium with a primary concentration of 8.67*108 transducing units (TU)/ml for the interference group (shRyR3) and 6.71*108 TU/ml for the control group (shCTL). Lentivirus was removed after transfection for 16 h. OPC were further differentiated for another 32 h and fixed.
Antisense Oligonucleotides
Phosphodiester ODNs protected by terminal phosphorothioate double substitution (capped ODNs) against possible exonuclease-mediated degradation were purchased from Tib-Molbiol (Sigma). The sequences are as follows: anti-RyR3: 5′-A*G*ATGCTAATTGCATC*T*C-3′ (*indicates the phosphorothioate residues) and an 18-mer fully degenerated ODN (dODN), 5′-N*N*NNNNNNNNNNNNNN*N*N-3′ (where N is G, C, A, or T), which was used as a control ODN. ODNs were transported using an artificial cationic lipid (DOTAP; Sigma) to enhance both uptake and stability. Antisense ODNs (aODNs) or dODNs were pre-incubated at 37°C for 30 min. Differentiated OLs were collected on day 3.
Statistics Analysis
All experiments were repeated at least three times. Data are shown as the means ± SEM. Statistical analyses of three groups were performed using one-way analysis of variance (ANOVA) followed by Tukey’s post hoc test. Comparisons between two experimental groups were made using Student’s t-test (GraphPad Prism 6). A probability of p < 0.05 was considered significant.
Results
Oligodendrocytes Selectively Express RyR3, Which Is Downregulated During Differentiation
Similar to our previous studies (Niu et al., 2012b), different stages of oligodendroglial cells in our culture system were identified based on morphological features using DIC and immunostaining for stage-specific markers. Normally, OPCs have small, round cell bodies with a bipolar morphology (Supplementary Figure S1). After 2 days in the differentiation medium, imOLs with 3–5 primary processes and a sparse arborization predominate this stage (Supplementary Figure S1). After 4 days of differentiation, mainly mature OLs characterized by multipolar processes and a rich arborization were observed (Supplementary Figure S1). These features indicate the accuracy of our culture model for studying the developmental schedule of the OL lineage.
To determine the RyR subtypes expressed in OLs, we first analyzed the mRNA levels in OL lineage cells. We found that only RyR3, the known “brain type”, was detected in OL lineage cells. In parallel experiments, RyR3 mRNA was also found in astrocytes, RyR2 mRNA was found in astrocytes and rat brain tissue, and RyR1 mRNA was only found in brain tissue (Figure 1B). Interestingly, the RyR3 mRNA level gradually decreased during OPC differentiation, and this downregulation tendency was further confirmed by western blot analysis (Figures 1C,D).
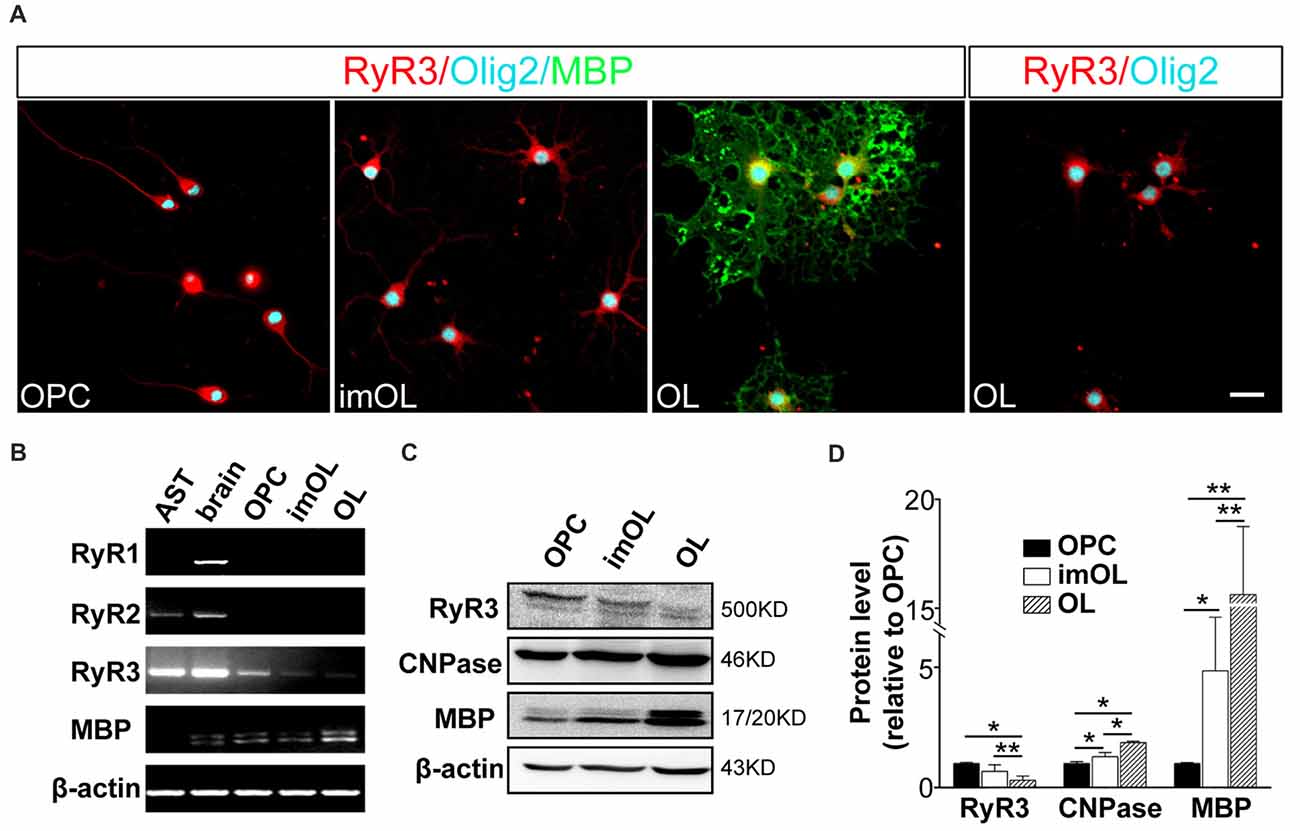
Figure 1. Oligodendroglial cells selectively express ryanodine receptor 3 (RyR3), which is downregulated during differentiation. (A) Immunofluorescence staining of RyR3, Olig2 (oligodendroglial lineage marker) and myelin basic protein (MBP; mature OL marker) in three stages of cultured OL lineage cells. RyR3 was strongly distributed all over the cytoplasm and processes in oligodendrocyte progenitor cells (OPCs) and immature oligodendrocytes (imOLs), while in mature OLs, RyR3 signal was mainly located in the cell body and showed lower expression in the primary processes. Scale bar 20 μm. (B) mRNA levels of RyRs in OL lineage cells, astrocytes (AST) and rat brain tissue. (C,D) Western blot analysis showed increased levels of myelin proteins (CNPase, MBP) and decreased levels of RyR3 protein during OPC development (*p < 0.05, **p < 0.01).
Next, we clarified the RyR3 distribution pattern in OL lineage cells by immunostaining. It has been shown that RyR3 is the subtype of ryanodine receptors expressed in OPCs. Specifically, RyR3 is located throughout the cell body and processes of OPCs, except in the region of the nuclear membrane (Haak et al., 2001). Our present results also revealed the enrichment of RyR3 in the processes and non-nuclear area of OPCs and imOLs, while in mature OLs, RyR3 was mainly located in the cell body and primary processes (Figure 1A).
Taken together, these results reveal a spatiotemporal regulated expression pattern of RyR3 in OL lineage cells, indicating its potential in regulating OL development.
ER Ca2+ Release Following Caffeine Stimulation Is Stage Specific During OPC Differentiation
Although OPCs are reported to have spontaneous oscillatory-like Ca2+ activity with peak and plateau transients, while mature OLs show “flat” Ca2+ signaling (Niu et al., 2016), the ER Ca2+ channels in OPCs (RyR3 and IP3R2) are relatively quiescent in comparison to those in neurons (Haak et al., 2001). To better study the RyR channel function, we took advantage of the classical RyR agonist caffeine (20 mM, 0 Ca2+; Zucchi and Ronca-Testoni, 1997) and real-time recorded the intracellular Ca2+ concentration with confocal microscopy. The Ca2+ release peaks are significantly higher in OPC and imOLs than in mature OLs (Figure 2D). More importantly, three typical Ca2+ responses were recorded in OL lineage cells after caffeine stimulation, showing stage-specific characteristics (Figures 2A,B). The high flat Ca2+ response following caffeine application was mainly found in OPCs (53.7%, n = 65) and imOLs (54.8%, n = 72). Likely, the oscillatory Ca2+ transients were present at a higher ratio in OPCs (35.5%, n = 65) and imOLs (27.9%, n = 72) than in mature OLs (10.5%, n = 76). The low plateaus Ca2+ response was the dominant reaction of mature OLs (76.3%, n = 76) but was barely found in OPCs and imOLs (Figure 2C). Notably, the millimolar concentration of caffeine was previously demonstrated to be an inhibitor of the IP3R channel in glioblastoma (1–10 mM; Kang et al., 2010), cerebellar microsomes (10–50 mM; Brown et al., 1992), B lymphocytes (1–25 mM) and other cells (Sei et al., 2001). In our present study, while the corresponding Ca2+ influx was abolished by using a Ca2+-free extracellular solution, the caffeine (20 mM)-induced Ca2+ transient is likely due to release of Ca2+ from ER mediated mainly by RyR3 channel. We assume that this stage-specific response is probably correlated to the downregulated expression of RyR3 during OL development.
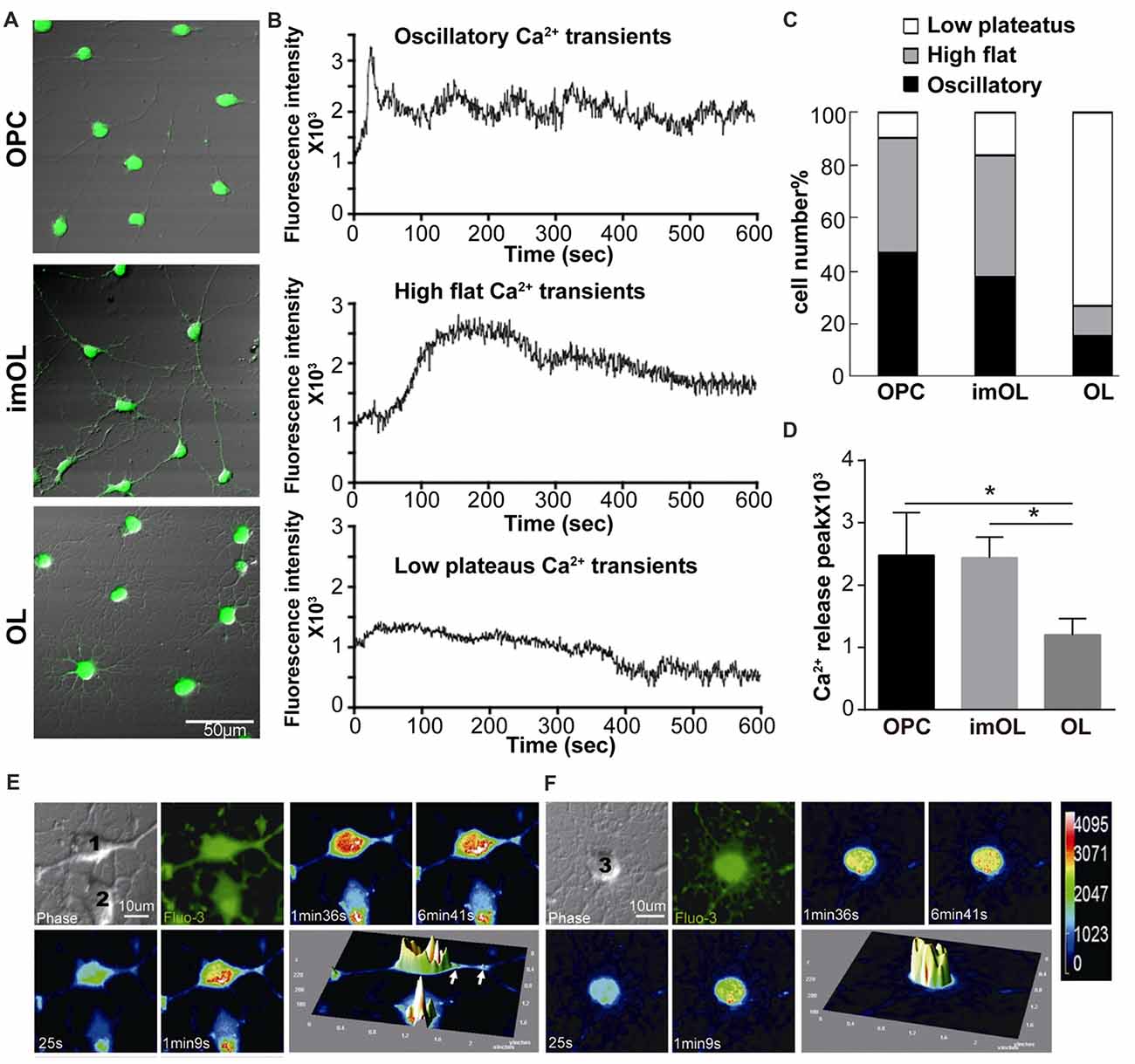
Figure 2. Endoplasmic reticulum (ER) Ca2+ release following caffeine stimulation is stage specific during OPC differentiation. (A) Differential interference contrast (DIC) with Fluo-3 fluorescence of three stages of OL lineage cells, showing typical shape of OPC, imOL and OL. Note that the pictures were acquired at the end point of Ca2+ imaging to ensure the morphology of the oligodendroglial cells, thus Fluo-3 fluorescence was extremely strong. (B) Representative examples of Ca2+ response following caffeine stimulation. Note that the start point in the x-axis is not the beginning point of recording, baseline recording is omitted from the curve. (C) OPCs and imOLs, which tend to respond with oscillatory Ca2+ transients and high flat Ca2+ transients, reacted stronger than OLs, which tend to respond with low plateaus Ca2+ transients. (D) OPC and imOL had significantly higher Ca2+ release peaks after caffeine stimulation compared with mature OLs (*p < 0.05). Time-lapse Ca2+ imaging induced by caffeine (20 mM, 0 Ca2+) in an OPC (1) (E) and a mature OL (F). Four successive scans were selected from a series of images obtained within 10 min. Transient fluorescence fluctuations, representing local Ca2+ release events, were indicated by the pseudocolored peaks in the OPC cell body and processes (arrows), but they occurred only in the soma in mature OLs. The pseudocolor bar in (F) showed the fluorescence intensity of different colors.
Given that RyR3 is not homogeneously distributed in the cell body and cell processes (Haak et al., 2001), especially in mature OLs, as we showed in Figure 1A, we wonder whether there is a regional difference in the Ca2+ response. By transforming the time-lapse Ca2+ imaging into pseudocolor changes and 3D surface plots with Image J software, we found that OPCs respond rapidly and strongly in both the soma and processes (Figure 2E), whereas only somal regions showed a slow and weak Ca2+ response in mature OLs (Figure 2F). Thus, the Ca2+ response pattern is highly correlated with the expression level and distribution of RyR3 in OL lineage cells.
At this point, we clarified the spatiotemporal regulated expression pattern of RyR3 and its expression-correlated functional pattern after caffeine stimulation. Our results indicate that RyR3-mediated Ca2+ signaling actively participates in the developmental regulation of OL lineage cells. The role of RyR3 under physiological conditions requires further exploration.
Inhibition of RyR3 Function by Ryanodine Suppresses OPC Differentiation
As Ca2+ signaling is essential for OPC differentiation and myelination, especially the initiation of OPC differentiation (Cheli et al., 2015; Friess et al., 2016), we next investigated whether there is active Ca2+ signaling at the initial stage of OPC differentiation. Spontaneous Ca2+ signaling was recorded in OPCs cultured in the proliferation medium and in OPCs that had been cultured in differentiation medium for 6 h. Consistent with our previous research (Niu et al., 2016), approximately 38.5% of OPCs presented occasional Ca2+ elevation (n = 18), which was characterized by a gradual increase in the Ca2+ concentration to a peak and a subsequently decreasing Ca2+ signal (Figure 3A). Meanwhile, in OPCs that were induced to initiate differentiation, spontaneous Ca2+ activity was observed in 55.6% of cells (n = 20). Those Ca2+ activities appeared at higher frequency; moreover, the elevation of Ca2+ signaling was more persistent in certain individual cells (Figure 3B). It has been reported that ryanodine, a RyR-specific blocker, locks the RyR channel into a “closed state” at higher concentrations (>50 μM; Meissner, 1986; Lai et al., 1989; McGrew et al., 1989). Thus, we used 50 μM ryanodine as an antagonist to block RyR3. The differentiation-related Ca2+ activity was dramatically inhibited (n = 24; Figures 3C,F). The amount of Ca2+ release reflected as the area under curve was markedly greater in early differentiating OPCs than in proliferating OPCs (Figure 3F). This result indicates that RyR3-mediated Ca2+ release from the ER is a critical process during OPC differentiation. To further verify the function of RyR3 in oligodendroglial differentiation, we treated OPCs with ryanodine (50 μM) and measured the number of mature OLs by immunofluorescence staining after 3 days of differentiation. The number of MBP-positive OLs was significantly decreased in the ryanodine-treated group (Figures 3D,E). Importantly, cell viabilities of OPC cultures were not affected by ryanodine treatment as reflected by the nuclear number, which is consistent with previous works (Matyash et al., 2002; Ruiz et al., 2010). Western blot results showed decreased protein levels of MBP and CNPase, which also reflected the inhibition of oligodendroglial maturation (Figures 3G,H). Thus, our results demonstrate that RyR3-mediated Ca2+ signaling does participate in the differentiation of OPCs.
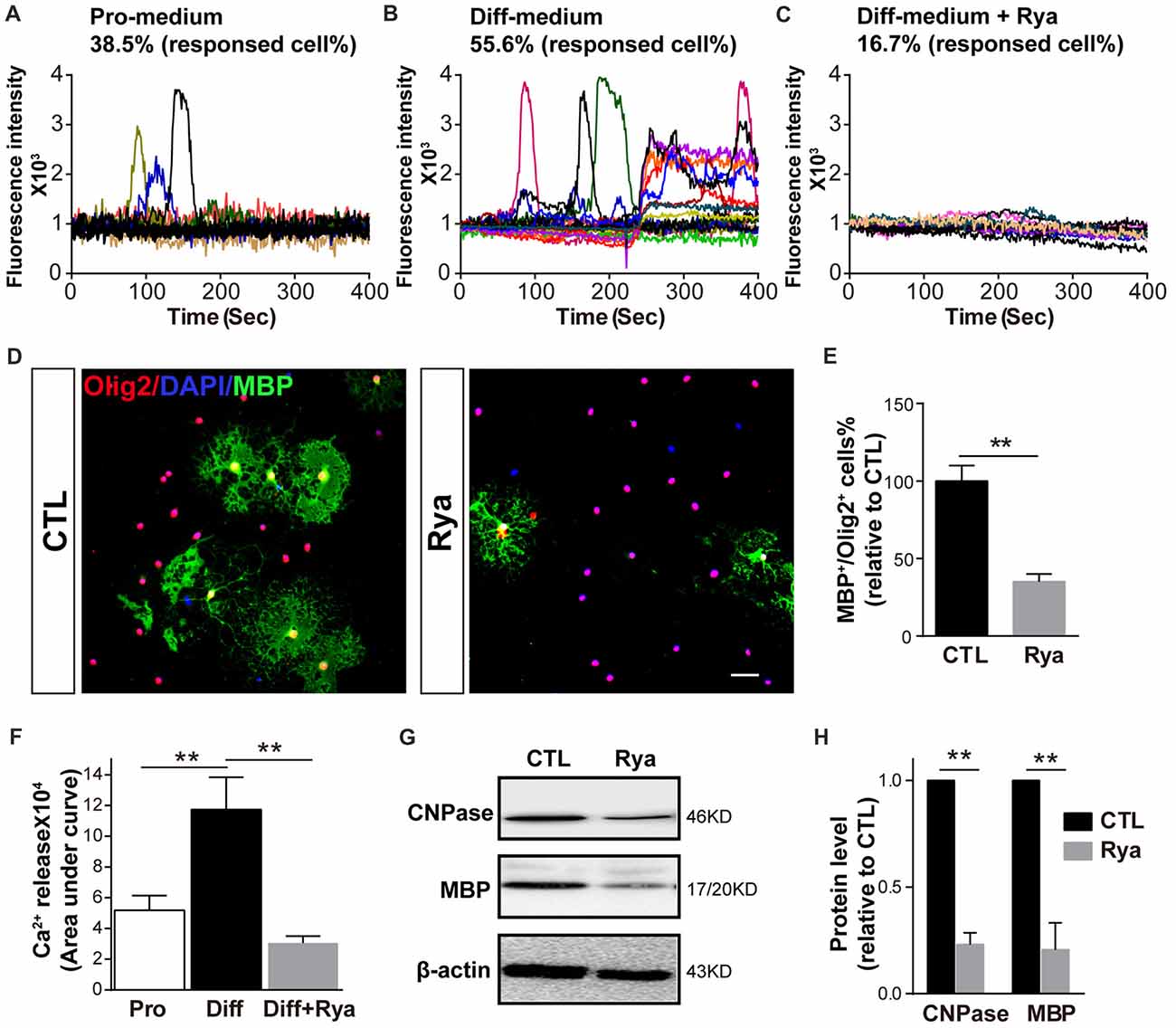
Figure 3. Inhibition of RyR3 by ryanodine suppresses OPC differentiation. (A) Ca2+ recording of OPCs in proliferation medium (Pro-medium) showing random spontaneous waves. (B) OPCs cultured in differentiation medium (Diff-medium) for 6 h showed frequently higher peak waves, which could be inhibited by ryanodine (Rya) (C). (F) The total amount of Ca2+ release, reflected by the area under curve, was greater in early differentiating OPCs than in proliferating OPCs (**p < 0.05), and the release activity in early differentiating OPCs was greatly reduced by ryanodine (**p < 0.05). (D,E) There were fewer MBP-positive mature OLs after treatment with ryanodine (50 μM) for 72 h. Scale bar 50 μm. (G,H) Western blot analysis showed the same inhibitory effect of ryanodine on OPC differentiation (**p < 0.05).
Knockdown of RyR3 in OPCs Results in Inhibition of OPC Differentiation
To further detect the role of RyR3 in OPC differentiation, we performed lentivirus-mediated gene knockdown of RyR3, which more precisely targets RyR3. OPCs were induced to differentiate after infection with an RNA interference lentivirus (shRyR3) or a control lentivirus (shCTL). After differentiation for 48 h, we observed efficient infection of both lentiviruses, visualized as the GFP expression in the cytoplasm (Figure 4A). Immunofluorescence staining for Olig2 (OL lineage marker) and MBP (mature OL marker) showed that the percentage of MBP-positive OLs was significantly decreased and most of the MPB-positive cells showed smaller process areas, and less flat membrane structures after RyR3 knockdown (Figures 4A,B), indicating the blockage of OPC differentiation in the absence of RyR3-mediated Ca2+ signaling. Cell viability after lentivirus treatment was guaranteed by the unchanged Olig2 positive cell number. As antisense oligonucleotides (aODNs) have the ability to selectively reduce the mRNA level of RyR3 (Galeotti et al., 2008), we also applied aODNs in OPC cultures and confirmed that aODNs reduced the protein level of RyR3. Consistent with the shRNA effect, aODN treatment similarly reduced the number of mature OLs (data not shown) and the levels of MBP (Figures 4C,D). Taken together, these observations reveal that gene-level knockdown of RyR3 induces loss of RyR3 channel function in OPCs, finally resulting in inhibition of OPC differentiation, implying that RyR3-mediated Ca2+ signaling plays an essential role during OPC differentiation.
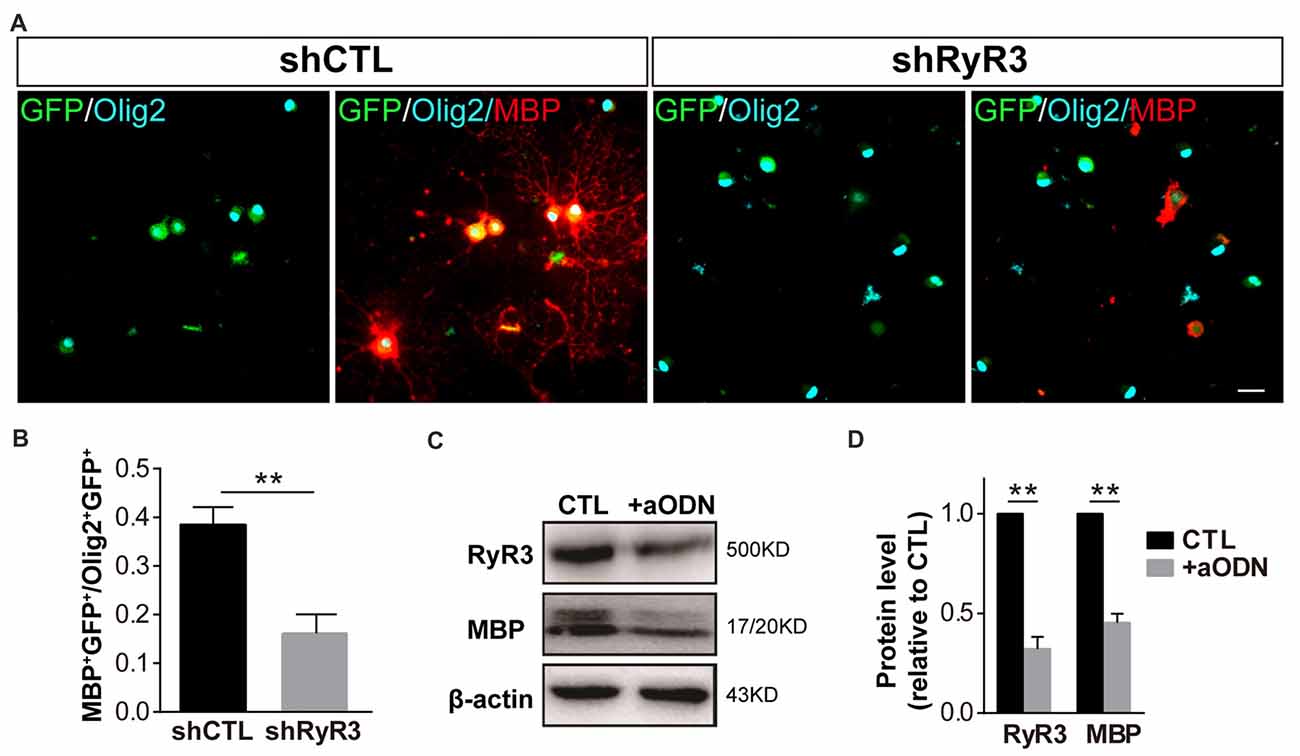
Figure 4. Knockdown of RyR3 in OPCs results in inhibition of OPC differentiation. (A,B) Gene knockdown of RyR3 with shRNA interference mediated by lentivirus infection showed fewer MBP-positive mature OLs after 48 h of differentiation. GFP was expressed in lentivirus-infected cells (**p < 0.05). Scale bar 20 μm. (C,D) Knockdown of RyR3 in OPCs by antisense oligonucleotide (+aODN) resulted in less expression of myelin basic protein (MBP; **p < 0.05).
Discussion
In non-excitable oligodendroglial cells, how intracellular Ca2+ signaling is regulated and how it contributes to oligodendroglial differentiation remains unclear. In our present study, we systematically analyzed the expression and function of the ER Ca2+ release channel—RyR3. We found that RyR3 is the only RyR expressed in oligodendroglial cells and dynamically regulated during OPC differentiation. Importantly, inhibition of RyR3 resulted in blockage of OPC differentiation (Figure 5). Our results not only demonstrate the essential role of RyR3-mediated Ca2+ signaling for oligodendroglial differentiation but also improve our understanding of the intracellular Ca2+ channel function in oligodendroglial lineage cells, which has barely been studied before.
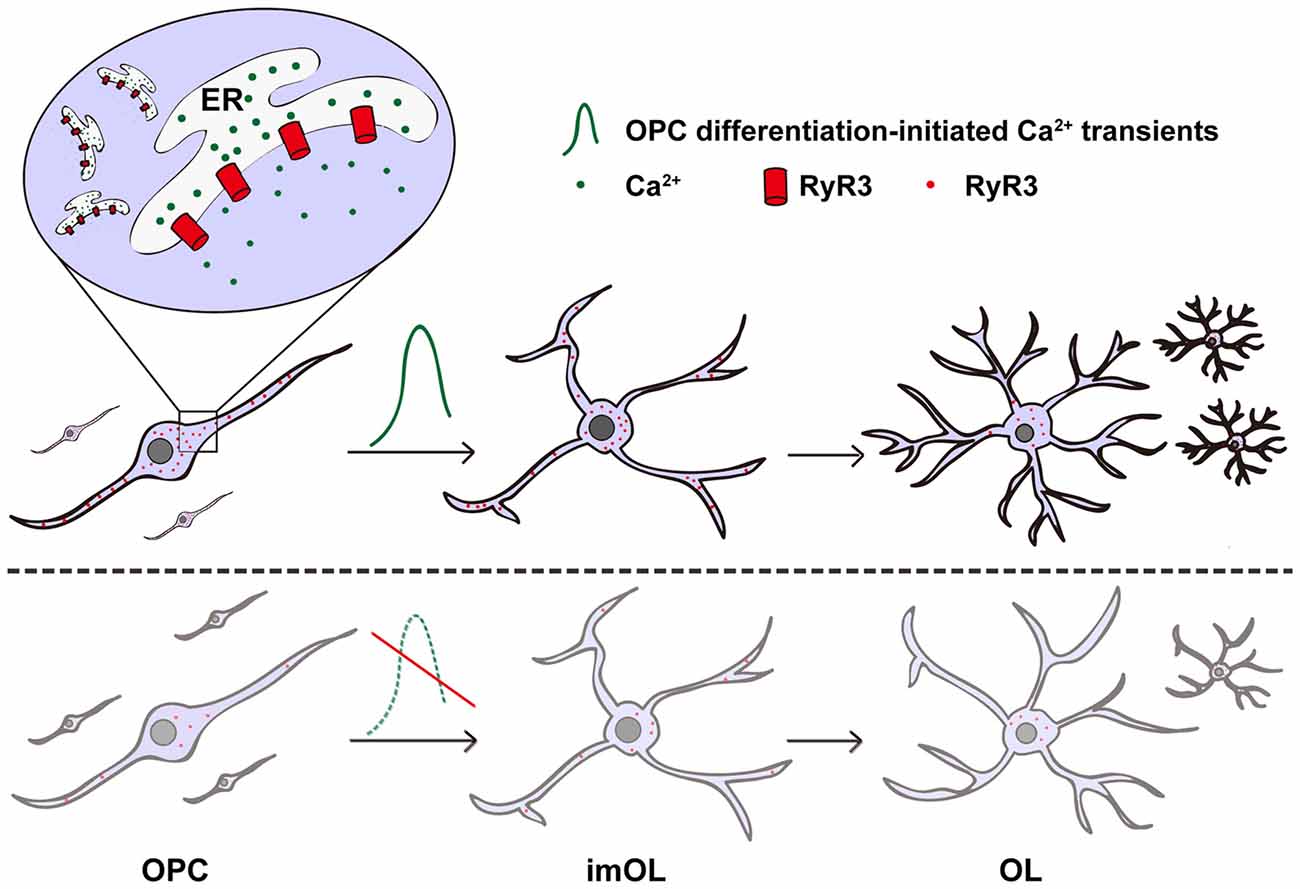
Figure 5. Scheme illustrating the contribution of RyR3-mediated Ca2+ signaling during oligodendroglial development. RyR3, a Ca2+-induced Ca2+ release (CICR) channel located on the ER membrane during oligodendroglial differentiation, note that it is widely distributed in the cell body and processes of OPC/imOLs but down-regulated and restricted in the cell body of mature OLs. The initiation of OPC differentiation requires hyperactive Ca2+ signaling mediated by RyR3. RyR3 knockdown or blockage of RyR3-mediated Ca2+ signaling in OPCs results in inhibition of OPC differentiation.
RyRs (RyR1–3) are major Ca2+ channels responsible for ER Ca2+ release, and RyR-mediated transient increase and oscillations of intracellular Ca2+ has been considered to be particularly important for cell functions (Zalk et al., 2007; Fulton et al., 2010; Suzuki et al., 2012). In oligodendroglial lineage cells, the RyR functionality was suggested to correlate with their developmental stages both in vivo and in vitro (Simpson et al., 1998), but the isoform of RyRs involved in this process has not been identified. Among RyRs, RyR3 is abundantly distributed in the CNS, and it functions in the activation and migration of astrocytes (Fill and Copello, 2002; Matyash et al., 2002; Galeotti et al., 2008; Lanner et al., 2010). In oligodendroglial lineage cells, only RyR3 was selectively expressed in cultured rat cortex OPCs (Haak et al., 2001), while another study demonstrated that three isoforms of RyRs (RyR1–3) were expressed in cultured rat optic nerve-derived mature OLs (Ruiz et al., 2010). Here, using rat cortex-derived OPC cultures, we confirmed the selective expression of RyR3 in oligodendroglial lineage cells and further determined the spatiotemporal expression pattern of RyR3 during oligodendroglial differentiation. Additionally, we found that enrichment of RyR3 in OPCs and imOLs corresponded to the stronger Ca2+ responses in those cell types than in mature OLs following caffeine stimulation. Interestingly, a previous study in myotubes has revealed that embryonic myotubes, which express RyR3, have considerably more variability in the size and kinetics of their Ca2+ sparks than do adult cells, which lose RyR3 expression (Ward et al., 2000). Therefore, expression of RyR3 in OPCs and imOLs likely enabled them to be more hyperactive in terms of Ca2+ transients and thus program them into the differentiating state.
Previous studies showed that Ca2+ responses to depolarization in OPCs were more active than those in mature OLs, and this phenomenon was mainly explained by the downregulation of L-type voltage-operated Ca2+ channels (VOCCs) following OPC maturation (Berger et al., 1992; Takeda et al., 1995; Paez et al., 2010; Zhang et al., 2014). Considering that RyR-mediated CICR is a critical amplification point for depolarization-induced intracellular Ca2+ elevation (Verkhratsky and Petersen, 2002; Pouvreau et al., 2007; Haberlandt et al., 2011), our results suggested that RyR3-mediated Ca2+ release from the ER is also an important contributor to the dynamic intracellular responses during OPC differentiation.
Consistent with a previous study (Simpson et al., 1998), we found that RyR3 was enriched in the entire processes of OPCs and imOLs but was absent in the leading processes of mature OLs, indicating that RyR3-mediated local Ca2+ signaling may contribute to enabling the process elongation and/or movements of OPCs (Paez et al., 2007) and to initiating cell differentiation (Friess et al., 2016). In agreement with this, we provide evidence showing that RyR3 knockdown results in failure of oligodendroglial differentiation, indicating that CICR may critically contribute to OPC differentiation.
Even if it has not been studied in this work, we speculate that in physiological in vivo condition, the Ca2+ signals triggering the opening of RyR3 are likely originated from the nearby neurons. Large amount of evidences have shown that neuronal action potentials could regulate myelin development, the candidate mediators are the neurotransmitters (glutamate, ATP, adenosine…) which could trigger Ca2+ influx in oligodendroglial cells and possibly evoke the following CICR through RyR3 (Butt, 2006; Spitzer et al., 2016; Sun et al., 2016; Krasnow et al., 2018). Moreover, axonal activity-induced increases in extracellular K+ are sufficient to depolarize VOCCs in nearby oligodendroglial cells to produce a significant rise in intracellular Ca2+ which also need CICR to form the final Ca2+ signal (Cheli et al., 2015). Importantly, RyR3 and IP3R2 are usually co-localized in OPCs, and interactions between them determine the spatial and temporal characteristics of Ca2+ signaling (Haak et al., 2001). Although a relative higher concentration of caffeine that was applied in our current study has been shown to stimulate RyR but inhibit the IP3R Ca2+ channel in several cell types (Brown et al., 1992; Sei et al., 2001; Kang et al., 2010), we could not exclude the role of IP3R2 in the Ca2+ signaling formation in oligodendroglial differentiation. Channel-specific antagonists or a genetic approach may be needed in our future studies.
In summary, we provide direct evidence showing that RyR3-mediated ER Ca2+ release is dynamically regulated and plays an essential role in initiating OPC differentiation (Figure 5). Our results supplement the current understanding of the function of intracellular Ca2+ channels in oligodendroglia and may provide valuable insights into therapeutic strategies for demyelinating diseases.
Author Contributions
LX, HL and TL designed experiments and wrote the manuscript. TL, LW, TM and SW conducted the experiments. TL, LW and JN collected and analyzed the data.
Funding
This work was supported by National Natural Science Foundation of China (31271467, 81471297), Natural Science Foundation of Chongqing (CSTCKJCXLJRC07) to LX and China Scholarship Council (3022) to TL.
Conflict of Interest Statement
The authors declare that the research was conducted in the absence of any commercial or financial relationships that could be construed as a potential conflict of interest.
Abbreviations
CICR, Ca2+-induced Ca2+ release; CNPase, 2′,3′-cyclic nucleotide-3′-phosphodiesterase; ER, endoplasmic reticulum; imOL, immature oligodendrocyte; MBP, myelin basic protein; OL, oligodendrocyte; OPC, oligodendrocyte progenitor cell; PDGFRα, platelet-derived growth factor receptor α; RyR, ryanodine receptor; VOCC, voltage-operated Ca2+ channel.
Supplementary Material
The Supplementary Material for this article can be found online at: https://www.frontiersin.org/articles/10.3389/fnmol.2018.00162/full#supplementary-material
References
Baraban, M., Koudelka, S., and Lyons, D. A. (2018). Ca2+ activity signatures of myelin sheath formation and growth in vivo. Nat. Neurosci. 21, 19–23. doi: 10.1038/s41593-017-0040-x
Berger, T., Schnitzer, J., Orkand, P. M., and Kettenmann, H. (1992). Sodium and calcium currents in glial cells of the mouse corpus callosum slice. Eur. J. Neurosci. 4, 1271–1284. doi: 10.1111/j.1460-9568.1992.tb00153.x
Bezprozvanny, I., Watras, J., and Ehrlich, B. E. (1991). Bell-shaped calcium-response curves of Ins(1,4,5)P3- and calcium-gated channels from endoplasmic reticulum of cerebellum. Nature 351, 751–754. doi: 10.1038/351751a0
Brown, G. R., Sayers, L. G., Kirk, C. J., Michell, R. H., and Michelangeli, F. (1992). The opening of the inositol 1,4,5-trisphosphate-sensitive Ca2+ channel in rat cerebellum is inhibited by caffeine. Biochem. J. 282, 309–312. doi: 10.1042/bj2820309
Butt, A. M. (2006). Neurotransmitter-mediated calcium signalling in oligodendrocyte physiology and pathology. Glia 54, 666–675. doi: 10.1002/glia.20424
Chang, A., Tourtellotte, W. W., Rudick, R., and Trapp, B. D. (2002). Premyelinating oligodendrocytes in chronic lesions of multiple sclerosis. N. Engl. J. Med. 346, 165–173. doi: 10.1056/nejmoa010994
Cheli, V. T., Santiago González, D. A., Spreuer, V., and Paez, P. M. (2015). Voltage-gated Ca2+ entry promotes oligodendrocyte progenitor cell maturation and myelination in vitro. Exp. Neurol. 265, 69–83. doi: 10.1016/j.expneurol.2014.12.012
Cohen, R. I., Molina-Holgado, E., and Almazan, G. (1996). Carbachol stimulates c-fos expression and proliferation in oligodendrocyte progenitors. Brain Res. Mol. Brain Res. 43, 193–201. doi: 10.1016/s0169-328x(96)00176-3
Fill, M., and Copello, J. A. (2002). Ryanodine receptor calcium release channels. Physiol. Rev. 82, 893–922. doi: 10.1152/physrev.00013.2002
Foskett, J. K., White, C., Cheung, K. H., and Mak, D. O. (2007). Inositol trisphosphate receptor Ca2+ release channels. Physiol. Rev. 87, 593–658. doi: 10.1152/physrev.00035.2006
Friess, M., Hammann, J., Unichenko, P., Luhmann, H. J., White, R., and Kirischuk, S. (2016). Intracellular ion signaling influences myelin basic protein synthesis in oligodendrocyte precursor cells. Cell Calcium 60, 322–330. doi: 10.1016/j.ceca.2016.06.009
Fulton, D., Paez, P. M., Fisher, R., Handley, V., Colwell, C. S., and Campagnoni, A. T. (2010). Regulation of L-type Ca++ currents and process morphology in white matter oligodendrocyte precursor cells by golli-myelin proteins. Glia 58, 1292–1303. doi: 10.1002/glia.21008
Galeotti, N., Vivoli, E., Bartolini, A., and Ghelardini, C. (2008). A gene-specific cerebral types 1, 2, and 3 RyR protein knockdown induces an antidepressant-like effect in mice. J. Neurochem. 106, 2385–2394. doi: 10.1111/j.1471-4159.2008.05581.x
Haak, L. L., Song, L. S., Molinski, T. F., Pessah, I. N., Cheng, H., and Russell, J. T. (2001). Sparks and puffs in oligodendrocyte progenitors: cross talk between ryanodine receptors and inositol trisphosphate receptors. J. Neurosci. 21, 3860–3870. doi: 10.1523/JNEUROSCI.21-11-03860.2001
Haberlandt, C., Derouiche, A., Wyczynski, A., Haseleu, J., Pohle, J., Karram, K., et al. (2011). Gray matter NG2 cells display multiple Ca2+-signaling pathways and highly motile processes. PLoS One 6:e17575. doi: 10.1371/journal.pone.0017575
Kang, S. S., Han, K. S., Ku, B. M., Lee, Y. K., Hong, J., Shin, H. Y., et al. (2010). Caffeine-mediated inhibition of calcium release channel inositol 1,4,5-trisphosphate receptor subtype 3 blocks glioblastoma invasion and extends survival. Cancer Res. 70, 1173–1183. doi: 10.1158/0008-5472.CAN-09-2886
Kirischuk, S., Scherer, J., Möller, T., Verkhratsky, A., and Kettenmann, H. (1995). Subcellular heterogeneity of voltage-gated Ca2+ channels in cells of the oligodendrocyte lineage. Glia 13, 1–12. doi: 10.1002/glia.440130102
Koulen, P., and Thrower, E. C. (2001). Pharmacological modulation of intracellular Ca2+ channels at the single-channel level. Mol. Neurobiol. 24, 65–86. doi: 10.1385/mn:24:1-3:065
Krasnow, A. M., Ford, M. C., Valdivia, L. E., Wilson, S. W., and Attwell, D. (2018). Regulation of developing myelin sheath elongation by oligodendrocyte calcium transients in vivo. Nat. Neurosci. 21, 24–28. doi: 10.1038/s41593-017-0031-y
Kuhlmann, T., Miron, V., Cui, Q., Wegner, C., Antel, J., and Brück, W. (2008). Differentiation block of oligodendroglial progenitor cells as a cause for remyelination failure in chronic multiple sclerosis. Brain 131, 1749–1758. doi: 10.1093/brain/awn096
Lai, F. A., Misra, M., Xu, L., Smith, H. A., and Meissner, G. (1989). The ryanodine receptor-Ca2+ release channel complex of skeletal muscle sarcoplasmic reticulum. Evidence for a cooperatively coupled, negatively charged homotetramer. J. Biol. Chem. 264, 16776–16785.
Lanner, J. T., Georgiou, D. K., Joshi, A. D., and Hamilton, S. L. (2010). Ryanodine receptors: structure, expression, molecular details, and function in calcium release. Cold Spring Harb. Perspect. Biol. 2:a003996. doi: 10.1101/cshperspect.a003996
Matyash, M., Matyash, V., Nolte, C., Sorrentino, V., and Kettenmann, H. (2002). Requirement of functional ryanodine receptor type 3 for astrocyte migration. FASEB J. 16, 84–86. doi: 10.1096/fj.01-0380fje
McGrew, S. G., Wolleben, C., Siegl, P., Inui, M., and Fleischer, S. (1989). Positive cooperativity of ryanodine binding to the calcium release channel of sarcoplasmic reticulum from heart and skeletal muscle. Biochemistry 28, 1686–1691. doi: 10.1021/bi00430a039
Meissner, G. (1986). Ryanodine activation and inhibition of the Ca2+ release channel of sarcoplasmic reticulum. J. Biol. Chem. 261, 6300–6306.
Meissner, G., Darling, E., and Eveleth, J. (1986). Kinetics of rapid Ca2+ release by sarcoplasmic reticulum. Effects of Ca2+, Mg2+, and adenine nucleotides. Biochemistry 25, 236–244. doi: 10.1021/bi00349a033
Meissner, G., Rios, E., Tripathy, A., and Pasek, D. A. (1997). Regulation of skeletal muscle Ca2+ release channel (ryanodine receptor) by Ca2+ and monovalent cations and anions. J. Biol. Chem. 272, 1628–1638. doi: 10.1074/jbc.272.3.1628
Meldolesi, J., and Pozzan, T. (1998). The endoplasmic reticulum Ca2+ store: a view from the lumen. Trends Biochem. Sci. 23, 10–14. doi: 10.1016/s0968-0004(97)01143-2
Moraru, I. I., Kaftan, E. J., Ehrlich, B. E., and Watras, J. (1999). Regulation of type 1 inositol 1,4,5-trisphosphate-gated calcium channels by InsP3 and calcium: simulation of single channel kinetics based on ligand binding and electrophysiological analysis. J. Gen. Physiol. 113, 837–849. doi: 10.1085/jgp.113.6.837
Niu, J., Li, T., Yi, C., Huang, N., Koulakoff, A., Weng, C., et al. (2016). Connexin-based channels contribute to metabolic pathways in the oligodendroglial lineage. J. Cell Sci. 129, 1902–1914. doi: 10.1242/jcs.178731
Niu, J., Mei, F., Wang, L., Liu, S., Tian, Y., Mo, W., et al. (2012a). Phosphorylated olig1 localizes to the cytosol of oligodendrocytes and promotes membrane expansion and maturation. Glia 60, 1427–1436. doi: 10.1002/glia.22364
Niu, J., Wang, L., Liu, S., Li, C., Kong, J., Shen, H. Y., et al. (2012b). An efficient and economical culture approach for the enrichment of purified oligodendrocyte progenitor cells. J. Neurosci. Methods 209, 241–249. doi: 10.1016/j.jneumeth.2012.05.032
Paez, P. M., Fulton, D. J., Spreur, V., Handley, V., and Campagnoni, A. T. (2010). Multiple kinase pathways regulate voltage-dependent Ca2+ influx and migration in oligodendrocyte precursor cells. J. Neurosci. 30, 6422–6433. doi: 10.1523/JNEUROSCI.5086-09.2010
Paez, P. M., Spreuer, V., Handley, V., Feng, J. M., Campagnoni, C., and Campagnoni, A. T. (2007). Increased expression of golli myelin basic proteins enhances calcium influx into oligodendroglial cells. J. Neurosci. 27, 12690–12699. doi: 10.1523/JNEUROSCI.2381-07.2007
Pouvreau, S., Royer, L., Yi, J., Brum, G., Meissner, G., Rios, E., et al. (2007). Ca2+ sparks operated by membrane depolarization require isoform 3 ryanodine receptor channels in skeletal muscle. Proc. Natl. Acad. Sci. U S A 104, 5235–5240. doi: 10.1073/pnas.0706530104
Ruiz, A., Matute, C., and Alberdi, E. (2010). Intracellular Ca2+ release through ryanodine receptors contributes to AMPA receptor-mediated mitochondrial dysfunction and ER stress in oligodendrocytes. Cell Death Dis. 1:e54. doi: 10.1038/cddis.2010.31
Sei, Y., Gallagher, K. L., and Daly, J. W. (2001). Multiple effects of caffeine on Ca2+ release and influx in human B lymphocytes. Cell Calcium 29, 149–160. doi: 10.1054/ceca.2000.0175
Simpson, P. B., Holtzclaw, L. A., Langley, D. B., and Russell, J. T. (1998). Characterization of ryanodine receptors in oligodendrocytes, type 2 astrocytes, and O-2A progenitors. J. Neurosci. Res. 52, 468–482. doi: 10.1002/(sici)1097-4547(19980515)52:4<468::aid-jnr11>3.0.co;2-#
Soliven, B. (2001). Calcium signalling in cells of oligodendroglial lineage. Microsc. Res. Tech. 52, 672–679. doi: 10.1002/jemt.1051
Spitzer, S., Volbracht, K., Lundgaard, I., and Káradóttir, R. T. (2016). Glutamate signalling: a multifaceted modulator of oligodendrocyte lineage cells in health and disease. Neuropharmacology 110, 574–585. doi: 10.1016/j.neuropharm.2016.06.014
Stangel, M., and Hartung, H. P. (2002). Remyelinating strategies for the treatment of multiple sclerosis. Prog. Neurobiol. 68, 361–376. doi: 10.1016/s0301-0082(02)00105-3
Sun, W., Matthews, E. A., Nicolas, V., Schoch, S., and Dietrich, D. (2016). NG2 glial cells integrate synaptic input in global and dendritic calcium signals. Elife 5:e16262. doi: 10.7554/elife.16262
Suzuki, M., Nagai, Y., Wada, K., and Koike, T. (2012). Calcium leak through ryanodine receptor is involved in neuronal death induced by mutant huntingtin. Biochem. Biophys. Res. Commun. 429, 18–23. doi: 10.1016/j.bbrc.2012.10.107
Takeda, M., Nelson, D. J., and Soliven, B. (1995). Calcium signaling in cultured rat oligodendrocytes. Glia 14, 225–236. doi: 10.1002/glia.440140308
Verkhratsky, A., and Petersen, O. H. (2002). The endoplasmic reticulum as an integrating signalling organelle: from neuronal signalling to neuronal death. Eur. J. Pharmacol. 447, 141–154. doi: 10.1016/s0014-2999(02)01838-1
Ward, C. W., Schneider, M. F., Castillo, D., Protasi, F., Wang, Y., Chen, S. R., et al. (2000). Expression of ryanodine receptor RyR3 produces Ca2+ sparks in dyspedic myotubes. J. Physiol. 525, 91–103. doi: 10.1111/j.1469-7793.2000.t01-2-00091.x
Wolswijk, G. (1998). Chronic stage multiple sclerosis lesions contain a relatively quiescent population of oligodendrocyte precursor cells. J. Neurosci. 18, 601–609. doi: 10.1523/JNEUROSCI.18-02-00601.1998
Yoo, A. S., Krieger, C., and Kim, S. U. (1999). Process extension and intracellular Ca2+ in cultured murine oligodendrocytes. Brain Res. 827, 19–27. doi: 10.1016/s0006-8993(99)01282-2
Zalk, R., Lehnart, S. E., and Marks, A. R. (2007). Modulation of the ryanodine receptor and intracellular calcium. Annu. Rev. Biochem. 76, 367–385. doi: 10.1146/annurev.biochem.76.053105.094237
Zhang, Y., Chen, K., Sloan, S. A., Bennett, M. L., Scholze, A. R., O’Keeffe, S., et al. (2014). An RNA-sequencing transcriptome and splicing database of glia, neurons, and vascular cells of the cerebral cortex. J. Neurosci. 34, 11929–11947. doi: 10.1523/JNEUROSCI.1860-14.2014
Keywords: oligodendrocyte progenitor cells (OPCs), oligodendrocytes (OLs), ryanodine receptor 3 (RyR3), Ca2+ release, differentiation, caffeine
Citation: Li T, Wang L, Ma T, Wang S, Niu J, Li H and Xiao L (2018) Dynamic Calcium Release From Endoplasmic Reticulum Mediated by Ryanodine Receptor 3 Is Crucial for Oligodendroglial Differentiation. Front. Mol. Neurosci. 11:162. doi: 10.3389/fnmol.2018.00162
Received: 30 November 2017; Accepted: 30 April 2018;
Published: 18 May 2018.
Edited by:
Alexej Verkhratsky, University of Manchester, United KingdomReviewed by:
Carlos Matute, University of the Basque Country (UPV/EHU), SpainAlla B. Salmina, Krasnoyarsk State Medical University named after Prof. V.F.Voino-Yasenetski, Russia
Copyright © 2018 Li, Wang, Ma, Wang, Niu, Li and Xiao. This is an open-access article distributed under the terms of the Creative Commons Attribution License (CC BY). The use, distribution or reproduction in other forums is permitted, provided the original author(s) and the copyright owner are credited and that the original publication in this journal is cited, in accordance with accepted academic practice. No use, distribution or reproduction is permitted which does not comply with these terms.
*Correspondence: Hongli Li, lihongli@tmmu.edu.cn
Lan Xiao, xiaolan35@hotmail.com