Estimating population viability of the northern Great Plains piping plover population considering updated population structure, climate change, and intensive management
- United States Geological Survey, Northern Prairie Wildlife Research Center, Jamestown, ND, United States
One challenge in wildlife conservation is understanding how various threats and management actions may influence long-term population viability. This is particularly evident when there is considerable uncertainty regarding population structure and vital rates. Reassessment of current knowledge and population trends is necessary for listed species to improve management actions that benefit conservation. We present an updated population viability analysis for northern Great Plains piping plovers (Charadrius melodus circumcinctus) based on the latest scientific data on survival, fecundity, and connectivity. Further, we explore the consequences of potential management actions and the stochastic effects of global climate change on population viability through changes in survival and fecundity. Our results predict elevated risks of extinction after 50 years (0.088 – 0.373) compared to previous predictions (0.033) based on assumed conditions of low connectivity among four major breeding groups structured as a metapopulation. We explored eight scenarios based on empirically-derived, higher connectivity rates and found that the northern Great Plains population never had a mean predicted population growth rate greater than one (0.946 – 0.996). Two scenarios that simulated a reduction in adult survival showed higher extinction probabilities (0.267 – 0.373), whereas two other scenarios that simulated an increase in fecundity exhibited lower extinction probabilities (0.088 – 0.103). These results indicate that viability of the northern Great Plains population of piping plovers could be improved with management actions that increase fecundity as long as adult survival is not simultaneously reduced. Lastly, breeding groups appeared to function less independently when connectivity rates were higher, as the breeding population was divided evenly among breeding groups. This indicates that the presumed metapopulation structure of our study system may need to be re-evaluated, and that empirically-based estimates of connectivity are essential to assessing population viability of mobile species that exhibit a spatially structured distribution.
1 Introduction
Increasing rates of widespread species declines are altering biodiversity and ecosystem processes at an unsustainable rate (Hooper et al., 2012; Moritz and Agudo, 2013; Rosenberg et al., 2019). This phenomenon is seemingly caused by multiple overlapping threats from habitat loss and degradation, emerging infectious diseases, novel species interactions, and global climate change (Smith et al., 2009; Maclean and Wilson, 2011; Doherty et al., 2016; Jarzyna et al., 2016). Now, more than ever, implementing effective conservation and management actions will be critical in responding to the current biodiversity crisis (Cahill et al., 2013; Pimm et al., 2014; Ceballos et al., 2020). Conserving small or declining populations requires assessing, updating, and predicting how populations will respond to varying environmental conditions or management actions for complex systems, often with considerable uncertainty (Duarte et al., 2017). One challenging task in wildlife conservation is assessing how different threats or proposed management actions may influence wildlife demography and long-term viability.
Population viability analysis (PVA) is an essential analytical tool in wildlife conservation for assessing long-term population viability, efficacy of potential management actions, and possible impacts of climate change through simulating scenarios to explore the effects of changes to population vital rates (Beissinger and Westphal, 1998; Reed et al., 2002; Radchuk et al., 2016; Chaudhary and Oli, 2020). PVA uses demographic data to simulate future population size and is therefore limited by the quality of available data and its applicability of current estimates to future conditions (Coulson et al., 2001; Chaudhary and Oli, 2020); thus, it benefits from reassessments when updated data become available. One criticism of PVA is often the lack of sufficient demographic data and reliance on expert opinion, which has been shown to reduce predictive accuracy (Pullin et al., 2004; Martin et al., 2012; Cook et al., 2014; Shaffer, 2019). However, PVA can be particularly useful when comparing relative trends and efficacy of multiple management actions (Brook et al., 2000; Ellner et al., 2002; Reed et al., 2002; Milligan et al., 2018; Saunders et al., 2018; Luck et al., 2022). Rather than attempting to provide accurate estimates of population trends, PVA can be instrumental to help identify optimal management decisions and future recovery goals in the context of uncertainty (Coulson et al., 2001; McGowan et al., 2014). In this study, we demonstrate the applicability of PVA to compare the future effects of multiple scenarios as well as the importance of periodic updates of vital rates for a listed species of high conservation interest and uncertain population status.
The ultimate recovery aim for northern Great Plains (NGP) piping plovers (Charadrius melodus circumcinctus; hereafter ‘plovers’) is to maintain a self-sustaining, viable population (USFWS, 1985; COSEWIC, 2001). In the NGP, plovers face numerous threats including channelization and modification of river flows, human development, predation, and climate change (USFWS, 2020). Climate change is considered “a severe threat to the species” but the effects on demography are not well understood (USFWS, 2020). Reproductive success appears limited in portions of the population likely due to high predation levels, such that predation is considered a widespread stressor to the NGP population (USFWS, 2020). In order to remove the NGP population from lists of federally threatened and endangered species these threats must be understood and attempted to be ameliorated and certain criteria must be met (USFWS, 2016; Environment Canada, 2006). In the United States, a viable breeding population must be demonstrated to have an extinction risk <0.05 within the next 50 years (USFWS, 2016; draft recovery plan was used as it is the most recent assessment of the U.S. NGP population), and in Canada, a self-sustaining, viable population that meets a minimum population size (813 pairs) must be maintained for 11 years (Environment Canada, 2006). In the most recent PVA for NGP plovers, expert opinion informed certain demographic rates including balanced, low inter-group connectivity rates of 0.0066, and the extinction probability was 0.033 after 50 years (McGowan et al., 2014). In a post-hoc scenario where connectivity rates were increased to 0.13, extinction risk for the entire NGP population increased to 0.083 – above the proposed delisting criteria (McGowan et al., 2014; USFWS, 2016). A recent large-scale empirical study resighting marked individuals found that dispersal between two spatially distinct breeding groups in the NGP was much higher than those initially simulated connectivity rates and was also unbalanced for both adult (U.S. Alkali Wetlands to Northern Rivers: 0.04, Northern Rivers to U.S. Alkali Wetlands: 0.17) and hatch-year (U.S. Alkali Wetlands to Northern Rivers: 0.33, Northern Rivers to U.S. Alkali Wetlands: 0.17) plovers (Swift et al., 2021a; Swift et al., 2022). This suggests that extinction risk for NGP plovers under high, unbalanced dispersal conditions may not meet the draft recovery criteria (USFWS, 2016) and needs to be reevaluated.
Here, we used eight scenarios to explore the effects of increased connectivity among breeding groups, predation management, and climate change on survival and fecundity to assess population viability for the NGP population of plovers. First, we used two different updated, empirically-derived estimates of demographic rates and connectivity, from a portion of the NGP breeding range (Update: unbalanced connectivity [UU] and Update: balanced connectivity [UB]; Swift et al., 2021a; Swift et al., 2022). Second, we explored how predation management, via the use of nest exclosures to increase nest survival (Anteau et al., 2022), affects population viability with two different scenarios. We tested potential effects of increased fecundity first without an effect on adult survival (Predation: fecundity only [PF]) and secondly increased fecundity with decreased adult survival (Predation: fecundity and survival [PS]). A common concern among managers, although based on anecdotal evidence in the NGP, is that nest exclosures may result in increased adult mortality (see Anteau et al., 2022; this effect has been shown in the other breeding populations Great Lakes: Roche et al., 2010a; and Atlantic Coast: Stantial, 2020). Lastly, our final four scenarios examined the impact of climate change and the associated predicted increase in stochastic weather events may have on fecundity and annual survival (Climate: stochastic fecundity [CF], Climate: fecundity increase [CI], Climate: fecundity decrease [CD], and Climate: survival decrease [CS]). Thus, our results, with updated information on extinction risk and population trends within the next 50 years for the entire NGP population will provide useful support for management decisions with rapid application for the management and conservation of a listed species, as well as inform potential future recovery objectives.
2 Methods
2.1 Study system and model
Piping plovers are a small-bodied shorebird endemic to North America that are federally protected under the U.S. Endangered Species Act (USFWS, 1985) and Canadian Species at Risk Act (COSEWIC, 2001). In part because of their protected status, plovers have been well-studied and vital rates are known for many breeding areas in the NGP. Additionally, PVA has been conducted repeatedly on this population as part of the management process (Ryan et al., 1993; Plissner and Haig, 2000; Larson et al., 2002; McGowan and Ryan, 2009; McGowan et al., 2014). However, a wealth of new data have been published since the most recent PVA (McGowan et al., 2014), and studies focused on connectivity and dispersal (Ellis et al., 2021; Swift et al., 2021a; Swift et al., 2021b; Swift et al., 2022), renesting (Swift et al., 2020), and juvenile survival (fledge to second-year; Catlin et al., 2015; Catlin et al., 2016; Roche et al., 2016a; Hunt et al., 2018; Catlin et al., 2019; Swift et al., 2021a; Swift et al., 2022) have filled critical knowledge gaps that required expert knowledge to inform prior PVAs. This new information allowed us to update critical vital rate estimates such as survival, fecundity, and connectivity and re-evaluate population viability for plovers throughout the NGP breeding range. We maintained the model structure and assumptions of the most recently published PVA (McGowan et al., 2014) for our simulations to rapidly assess population viability for the USFWS and directly compare the effects of new demographic information.
We define three spatial scales for our text: 1) the NGP population, which encompasses all breeding individuals outside of the Great Lakes and Atlantic Coast populations, 2) four breeding groups, a pre-defined metapopulation structure (McGowan et al., 2014; USFWS, 2016; USFWS, 2020), and 3) various breeding areas where research, monitoring, or management have actively occurred by various stakeholders (Figure S1). Each breeding group is composed of multiple breeding areas (i.e., Northern Rivers includes breeding areas along the Missouri River in Montana and North and South Dakota: Fort Peck, Lake Sakakawea, Garrison Reach, and Lake Oahe; Table S1). Our study area included all breeding areas in the NGP of the United States and Canada (i.e., breeding areas in Colorado, Nebraska, South Dakota, North Dakota, Montana, Manitoba, Saskatchewan, and Alberta), and uses the previously defined metapopulation structure composed of four breeding groups (Prairie Canada, U.S. Alkali Wetlands, Northern Rivers, and Southern Rivers; McGowan et al., 2014; USFWS, 2016; USFWS, 2020). Plovers breeding in the NGP use bare to sparsely vegetated areas of sand or gravel substrate found on sandbars of flowing rivers, shorelines of depressional wetlands, lakes, and reservoirs (Anteau et al., 2019; Swift et al., 2021a), and margins of various stages of sand and gravel mines (Jorgensen et al., 2021). Most habitat is primarily created and maintained by water-level fluctuations (Anteau et al., 2014a; McCauley et al., 2016; Anteau et al., 2019). During the nonbreeding season, individuals breeding in the NGP largely overwinter along the Gulf Coast of Texas and Mexico (Ellis et al., 2021).
Here, we outline the most recent assessment of population viability (McGowan et al., 2014). In McGowan et al. (2014), extinction risk was modeled under presumed low probabilities of connectivity between breeding groups, a low density-dependent ceiling, non-varying mean adult and juvenile survival probabilities, and breeding group-specific fecundity estimates (see McGowan et al., 2014 for details). A density-dependent ceiling of 6,000 females was used to reduce fecundity to zero if the population in a breeding group exceeded this threshold as there is some weak evidence for density-dependent reproductive success at local levels in the NGP (Catlin, 2009; Anteau et al., 2014b; and this has been demonstrated in other parts of the breeding range: Cohen et al., 2009; Robinson et al., 2020). Mean annual adult survival was set at 0.78 (SE = 0.03) and mean juvenile survival (fledging to second-year) was set at 0.52 (SE = 0.12; Table 1). Fecundity estimates varied by breeding group: Prairie Canada (0.52 female fledglings per pair, SE = 0.40); U.S. Alkali Wetlands (0.60, SE = 0.47); Northern Rivers (0.32, SE = 0.27); and Southern Rivers (0.77, SE = 0.24) based on the best available data at that time (McGowan et al., 2014, Table 1). Connectivity among breeding groups was assumed by experts to be low and equal among all pairs of breeding groups (rate: 0.0066, SE = 0.02; Table 2). McGowan et al. (2014) used a quasi-extinction probability which assumed extinction if there were fewer than 50 individuals in a breeding group or 100 individuals in the total NGP population, and the model allowed for parametric uncertainty and temporal variability. This customized PVA uses a hierarchical three-loop structure to select initial values in the outer loop, sampling variance in the secondary replicate loop, and temporal variability in the innermost annual loop. Thus, under 1,000 different initial conditions, population viability is simulated 1,000 times in the secondary loop and for 50 years in the annual loop (see McGowan et al., 2014 for additional details and diagrams). Three model outputs are produced: mean population growth rate across the 1,000 outer loop replicates, extinction probability at year 50, and median female abundance at year 50 (see McGowan et al., 2014 for details). Other than the stated updated vital rate estimates or variances, we retained the publicly available model code from McGowan et al. (2014) for analyses. All simulations were conducted in program R v 3.6.3 (R Core Team, 2020), and estimates are reported with 2.5 and 97.5 percentiles (95% credible intervals) from the 1,000 outer loop replicates. For median abundance estimates, 95% quantiles are also presented for the secondary replicates at year 50.
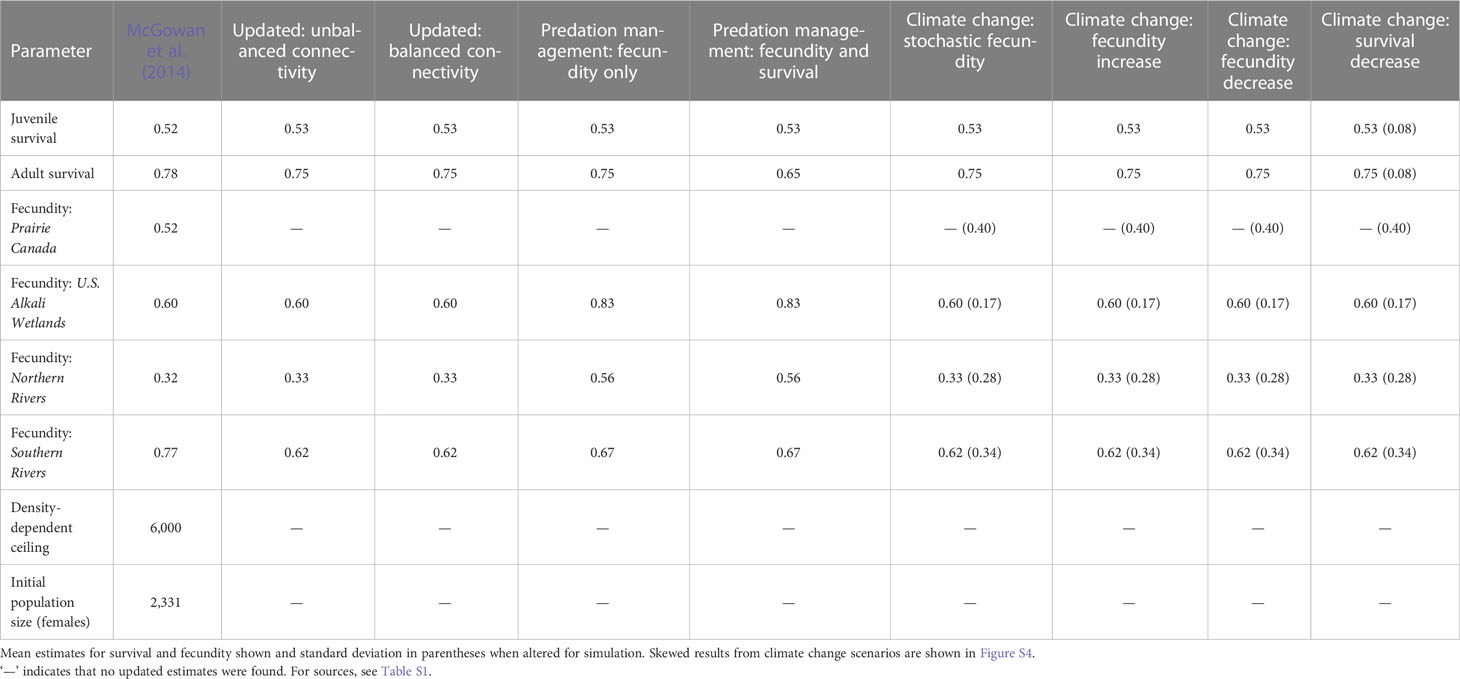
Table 1 Estimates used in the McGowan et al. (2014) model and updated values of population vital rates used for population viability analysis of northern Great Plains piping plovers.
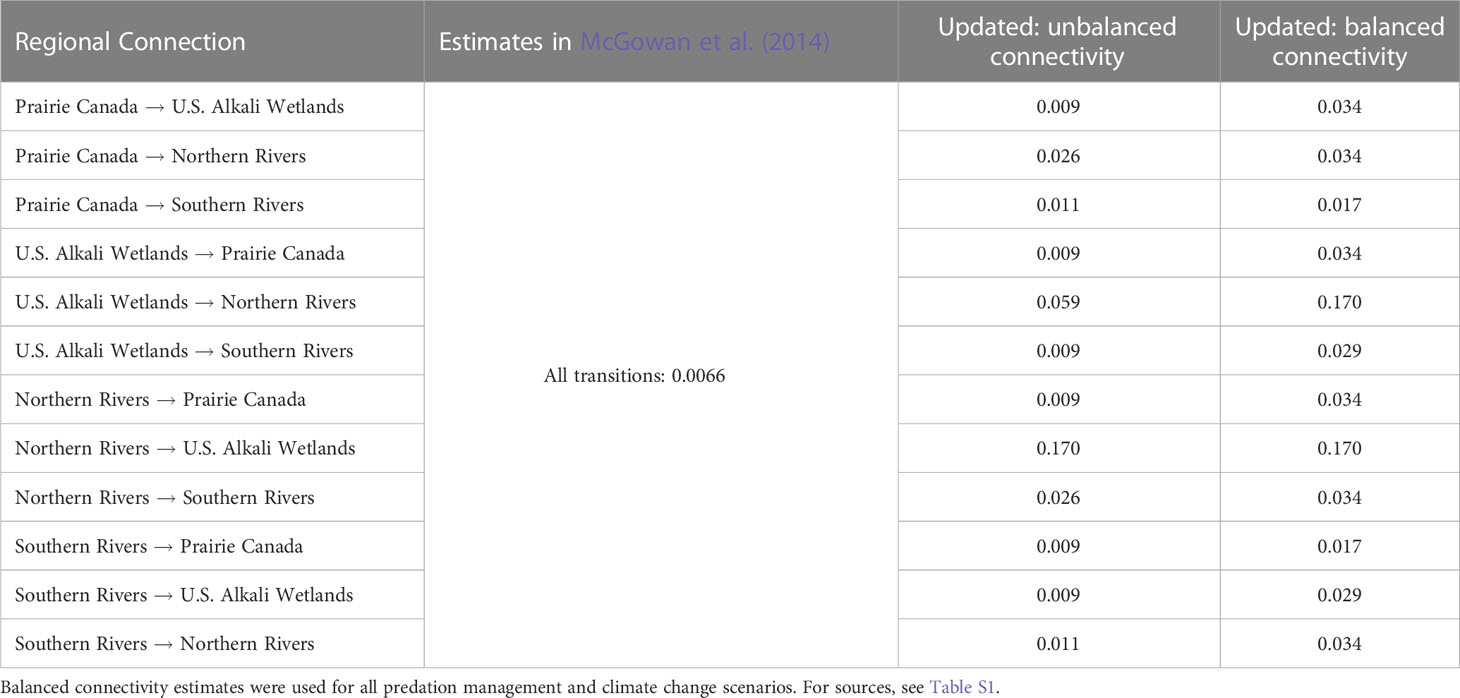
Table 2 Connectivity estimates used in the McGowan et al. (2014) model and updated values used for population viability analysis of northern Great Plains piping plovers.
2.2 Updating vital rate estimates
To reassess population viability, we collected estimates pertaining to survival, fecundity, and connectivity from both published and gray literature focusing on works published since 2014 (Tables 1 and 2, for sources see Table S1). We calculated the mean of published estimates of survival and fecundity for each age class or breeding group as relevant (Table S1). Our updated estimates of survival and fecundity were similar to those used by McGowan et al. (2014), with the exception of a lower estimate of fecundity for the Southern Rivers. We did not change any of the other parameters in the model (i.e., density-dependent ceiling, initial population size) because we found no additional data to inform them. We retained the 2006 International Piping Plover Census data (Elliott-Smith et al., 2009) as the initial population size and proportion of population in each breeding group. Extensive flooding on the Missouri River, high water levels on wetlands, and use of non-traditional nesting habitat (e.g., gravel roads and parking lots) meant that counts in the more recent 2011 International Piping Plover Census (Elliott-Smith et al., 2015) were likely an underestimate of true population size (2,249 individuals counted in 2011 compared to 4,662 in 2006), and minimum breeding population size estimates on the Missouri River recovered to similar 2006 levels after 2011 (Anteau et al., 2019).
Because less data were available to inform estimates of connectivity across the full NGP, we chose to test two different levels of connectivity. Swift et al. (2021a); Swift et al. (2022) provided the first empirical estimates of connectivity among breeding groups in the NGP, focusing on movements between the U.S. Alkali Wetlands and Northern Rivers. Swift et al. (2021a); Swift et al. (2022) documented high, unbalanced natal dispersal (i.e., hatch-year to first breeding attempt) between these two breeding groups. Individuals were more likely to disperse from the U.S. Alkali Wetlands to breed on the Northern Rivers (0.33, SD = 0.23) than vice versa (0.17, SD = 0.04). Swift et al. (2021a) also documented high, unbalanced breeding dispersal (i.e., successive annual breeding attempts by adults) between these two groups and high rates of movements within these breeding areas (Swift et al., 2023). However, adults were more likely to disperse from the Northern Rivers to breed on the U.S. Alkali Wetlands (0.17, SD = 0.01) than vice versa (0.04, SD = 0.006) Swift et al. (2021a). Because this PVA was not structured to account for age-specific dispersal rates, we converted these empirically-derived estimates based on the mean proportion of second-years (completing natal dispersal) to adults (completing breeding dispersal) within the U.S. Alkali Wetlands and Northern Rivers (mean: 0.0983 second-years per adult; 2014 – 2019 annual range: 0.0934 – 0.1110). These proportions combined with our empirical estimates resulted in estimates of 0.073 for dispersal from the U.S. Alkali Wetlands to the Northern Rivers and 0.17 for dispersal from the Northern Rivers to the U.S. Alkali Wetlands. Additionally, we collated all reported movements between breeding groups from Swift et al. (2021a) and Ellis et al. (2021) representing 473 known inter-group movements. The observed movements do not account for heterogeneity in detection probabilities between breeding groups.
For our first estimate of connectivity (UU), we scaled the empirically-derived estimate of connectivity between the U.S. Alkali Wetlands and Northern Rivers based on the observed number of movements of marked individuals among breeding groups resulting in unbalanced rates of connectivity among breeding groups (Table 2). Observed connectivity was the highest between the U.S. Alkali Wetlands and Northern Rivers groups (Northern Rivers to U.S. Alkali Wetlands n > 200 observed movements, connectivity: 0.170; U.S. Alkali Wetlands to Northern Rivers n > 100, 0.059). Dispersal was the next most common from Prairie Canada to the Northern Rivers and from the Northern Rivers to the Southern Rivers (connectivity: 0.026). Observed movements from Prairie Canada to the Southern Rivers and from the Southern Rivers to the Northern Rivers was the next most common (connectivity: 0.011), and all other rates were set to 0.009 as known movements were rarely reported (n < 4). While plovers are monitored throughout their range, capture-mark-resight efforts have varied considerably and were lowest in Prairie Canada during the period when most movement observations occurred, making estimates of movements into and out of Prairie Canada potentially biased low. Additionally, questions remained if unbalanced connectivity throughout the NGP was representative of long-term trends because empirical estimates were largely derived from a six-year study with high water flows on the Missouri River (Swift et al., 2021a; Swift et al., 2022). Plovers have rarely been documented dispersing long distances (>500 km; Haig and Oring, 1988; Hillman et al., 2012; Amirault-Langlais et al., 2014; Catlin et al., 2015; Swift et al., 2021a; Swift et al. 2021b), therefore, we derived a second estimate of connectivity based on the distance between centroid locations of each of the four breeding groups, resulting in balanced connectivity estimates between pairs of breeding groups (UB; Table 2). Individuals were most likely to disperse between the Northern Rivers and the U.S. Alkali Wetlands (connectivity: 0.170; determined from Swift et al., 2021a; Swift et al., 2022) as they were the closest (~118 km). The following estimates of connectivity were then scaled by the distance between centroids. Dispersal between the Southern and Northern Rivers, Prairie Canada and Northern Rivers, and Prairie Canada and the U.S. Alkali Wetlands, was lower (connectivity: 0.034) as they were, on average, ~584 km apart. The Southern Rivers and U.S. Alkali Wetlands were ~702 km apart therefore connectivity rates were set to 0.029. Lastly, the Southern Rivers and Prairie Canada were the farthest apart (~1,176 km), so connectivity was lowest at 0.017. Balanced connectivity estimates were used to test predation management and climate change scenarios (see below) because of the concerns regarding the conditions (i.e., high water flows on the Missouri River) during the six-year study providing the empirical estimates which informed the unbalanced connectivity estimates.
2.3 Testing predation management scenarios
Nest depredation is a leading cause of nest failure in ground nesting birds (Martin, 1993; Anteau et al., 2022). Management to reduce nest loss to predation uses different techniques and tools of varying effectiveness such as removal, exclusion, and hazing (Shivik et al., 2003). One common form of predation management for shorebird nests is predator exclosures (i.e., nest cages; Rimmer and Deblinger, 1990; Melvin et al., 1992), which are nesting-bird-permeable but exclude most avian and mammalian predators from nests (Anteau et al., 2022). Nest exclosures have repeatedly been documented to improve nest success for piping plovers and other ground-nesting species (Rimmer and Deblinger, 1990; Mabee and Estelle, 2000; Murphy et al., 2003a; Isaksson et al., 2007; Maslo and Lockwood, 2009; Dinsmore et al., 2014; Anteau et al., 2022), but concerns remain regarding how effective they may be at increasing population growth rates or if there are any negative impacts, such as a reduction in incubating adult survival (Murphy et al., 2003b; Roche et al., 2010a; Smith et al., 2011; Gaines et al., 2020; Stantial, 2020). A previous PVA on NGP plovers reported that the use of predator exclosures, which would increase nest and fledgling rates (with no effect on adult survival), would lead to a stable population (Larson et al., 2002). Using an experimental design in the wetlands of the Prairie Pothole Region, Anteau et al. (2022) found that nest exclosures successfully increased nest survival without any negative effects on chick survival, within-season adult survival, or annual adult survival. It is still unknown if this pattern holds in other breeding habitat types in the NGP found in other breeding groups, such as riverine sandbars or former sand or gravel mines.
We used two simulations to assess population viability if managers enacted effective predation management to increase population fecundity. To represent a realistic management scenario, we assumed nest exclosures would not be used everywhere plovers breed, but rather would be used in breeding areas where existing monitoring or prior predation management actions have been previously implemented (solely in the U.S. breeding range; U.S. Alkali Wetlands; Northern Rivers: Garrison Reach; Southern Rivers: Central Platte River, Lower Platte, Loup and Elkhorn Rivers, Gavins Point Reach, Lewis and Clark Lake). To adjust fecundity estimates for the U.S. breeding areas, we recalculated fecundity solely for those breeding areas with prior or current monitoring using the nest survival estimates for exclosed nests from the U.S. Alkali Wetlands (Anteau et al., 2022) and the original chick survival estimates from each study specific to that breeding area (Table S1). We then took the mean of all adjusted and unadjusted fecundity estimates for each breeding group, with the exception of the U.S. Alkali Wetlands, where we used the estimate derived from Anteau et al. (2022) for exclosed nests. These adjusted estimates of fecundity would, therefore, suggest an intensive effort to find and exclose nests throughout the NGP. Because of lingering concerns regarding possible adult mortality with predator exclosures, we provide two simulations: one with increased fecundity as a result of predation management (PF; i.e., only positive effects of nest exclosures or alternatives such as predator removal or hazing) and one with both increased fecundity and decreased adult survival as a result of predation management (PS; i.e., if nest exclosure use reduces adult survival). We reduced adult survival to account for concerns due to the potential increased adult mortality associated with nest exclosures if used outside of the U.S. Alkali Wetlands. Annual adult snowy plover (C. nivosus) survival was shown to be reduced with the use of predator exclosures in a study from 1990-2014 in Oregon, USA (Gaines et al., 2020). We used the low estimate of snowy plover adult survival from a year with high predator exclosure effort (87% of nests exclosed) as an example of a worst-case scenario for piping plovers. The predator community in Gaines et al. (2020) was like that in the NGP (corvids, skunks, foxes), and both plover species have a similar migratory distance and annual cycle. In addition, this estimate of annual adult survival (0.65) is similar to earlier estimates of NGP piping plover survival when nest exclosure efforts were widespread. For these simulations, we used the updated estimates of survival and balanced connectivity (Table 1).
2.4 Testing climate change scenarios
We used four simulations focused on the potential effects of future global climate change on breeding plovers in the NGP by increasing stochasticity in the PVA for fecundity and survival estimates. In the NGP, climate change is expected to change precipitation patterns, warm temperatures, and may cause more heavy rainfall events (USGCRP, 2018). Over the next two to three decades, temperatures are expected to increase leading to more summer days over 32°C. While total precipitation and streamflow projections show minimal changes from current conditions, annual variability is expected to increase leading to more extreme events like the flooding that occurred in 2011 (Anteau et al., 2019) and severe droughts. The effects that variable precipitation and temperature may have on habitat availability for nesting plovers or on reproductive success are unknown. Severe storms may lead to adult mortality, abandonment of nests, or direct mortality of chicks or eggs. Extreme floods or droughts may eventually increase habitat availability in later years, and therefore fecundity (Anteau et al., 2019), as sandbars and shorelines are scoured or water levels draw down to the point in which no water remains, respectively. Vegetative invasion of shorelines limits habitat (Anteau et al., 2014a), but the rate of vegetative invasion and effect on fecundity would likely be influenced by timing of precipitation events (e.g., wet vs. dry spring/summers). As such, to explore the potential effects of climate on plover fecundity for three of our four climate simulations, we model increased stochasticity to represent the intra- and inter-annual variability of extreme climatic events: one where future climate maintains similar levels of stochastic plover fecundity to current observed levels by altering the variance around fecundity estimates (CF), one with altered variance and where future climate skews plover fecundity above the current average (CI), and one with altered variance and where future climate skews plover fecundity below the current average (CD). Thus, the estimates in the outer loop are those used in the updated run with balanced connectivity, but the variance around the fecundity parameters (and the skew of the distribution) of the secondary loop has changed based on the observed variance from all published fecundity estimates in each breeding group (Prairie Canada: 0.40, U.S. Alkali Wetlands: 0.17, Northern Rivers: 0.28, Southern Rivers: 0.34).
For our fourth climate change scenario (CS), we simulated stochasticity in both fecundity and survival by also increasing the variance around adult and juvenile survival estimates in addition to the increased variation around fecundity to address potential full annual cycle impacts to the species. Plovers occupy marine shorelines and tidal marshes during the nonbreeding season and face threats such as hurricanes, harmful algal blooms, and oil spills which may reduce survival (Saunders et al., 2014; Ellis et al., 2021). The frequency and severity of hurricanes is predicted to increase (Bender et al., 2010), and harmful algal blooms are expected to increase in frequency and range with continued ocean warming and eutrophication (IPCC, 2021). Both hurricanes and harmful algal blooms negatively affect NGP plover nonbreeding season survival (Ellis et al., 2021). For this final simulation, we increased the variance and skewed the mean estimates (by altering the distribution in the secondary loop) of adult and juvenile survival below the current average to represent an increased occurrence of hurricanes and harmful algal blooms during the nonbreeding season in addition to stochastic fecundity. We informed the increased variance around survival (0.08) based on the mean effects of hurricanes and harmful algal blooms in Ellis et al. (2021).
3 Results
None of our eight simulations had the entire NGP population see population growth rates greater than one (Figure 1) or had any breeding group increase to the density-dependent ceiling (Figure S4). Extinction probabilities for the NGP population varied from 0.088 (PF) to 0.373 (PS; Figures 2, S2). In only one of eight simulations did the NGP population decline below the initial population size (median abundance estimate; PS; Figure 3).
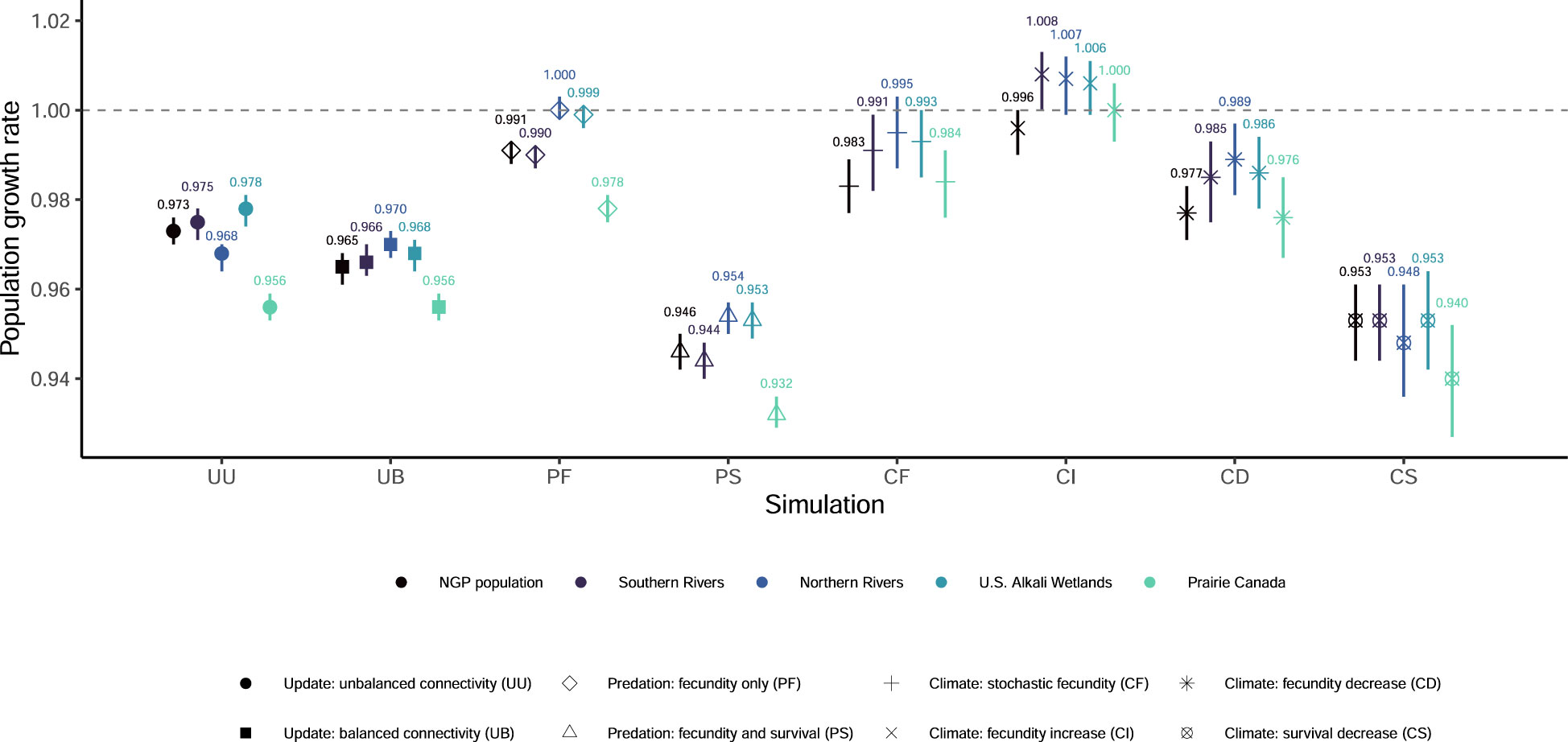
Figure 1 Mean population growth rates and associated 95% quantiles from 1,000 outer-loop replicates for eight updated simulations of population viability for the northern Great Plains (NGP) piping plover (Charadrius melodus circumcinctus) population and four breeding groups. The grey dashed line indicates a population growth rate equal to one.
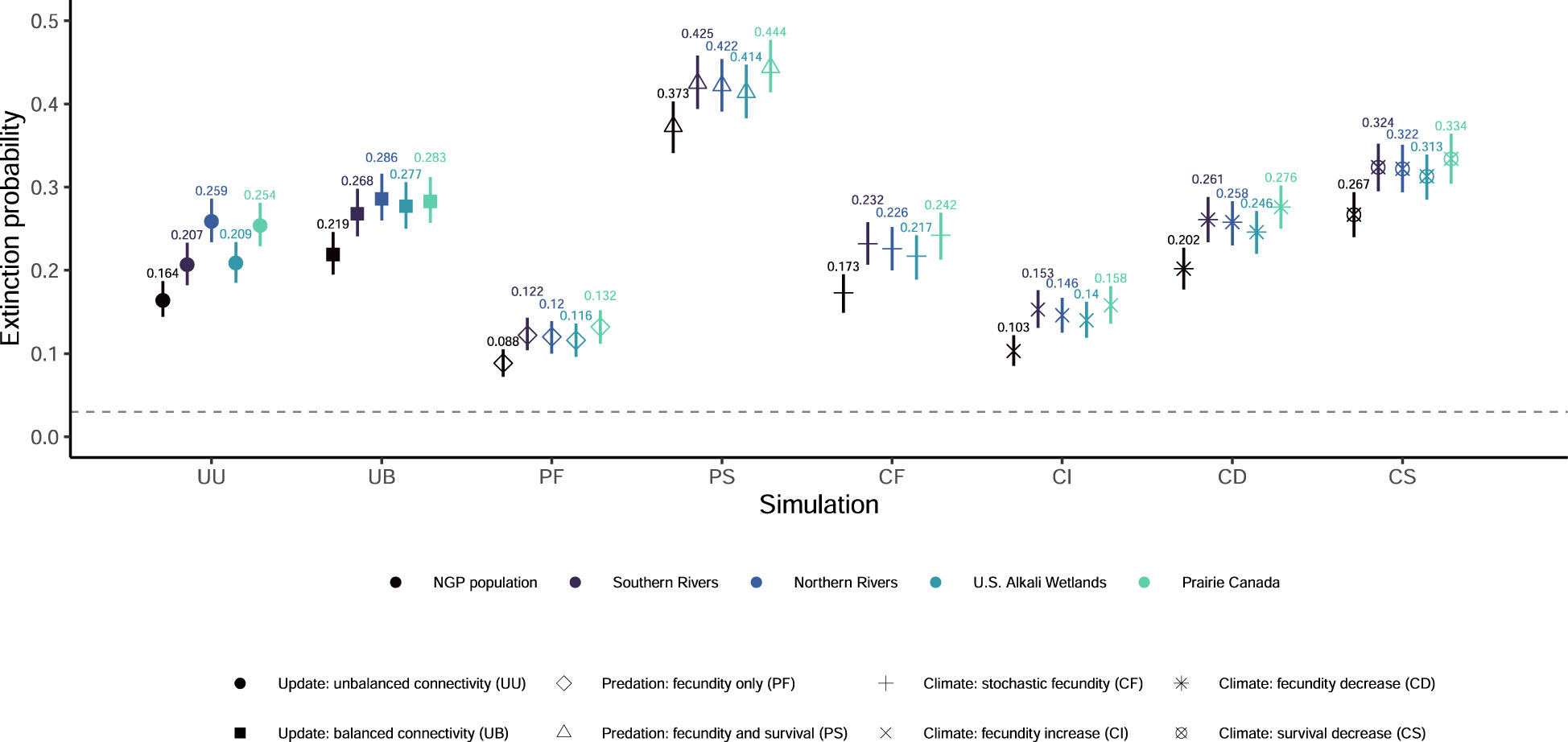
Figure 2 Mean extinction probabilities and associated 95% quantiles from 1,000 outer-loop replicates for eight updated simulations of population viability for the northern Great Plains (NGP) piping plover (Charadrius melodus circumcinctus) population and four breeding groups. The grey dashed line indicates the extinction probability from the most recent population viability analysis (0.033, McGowan et al., 2014).
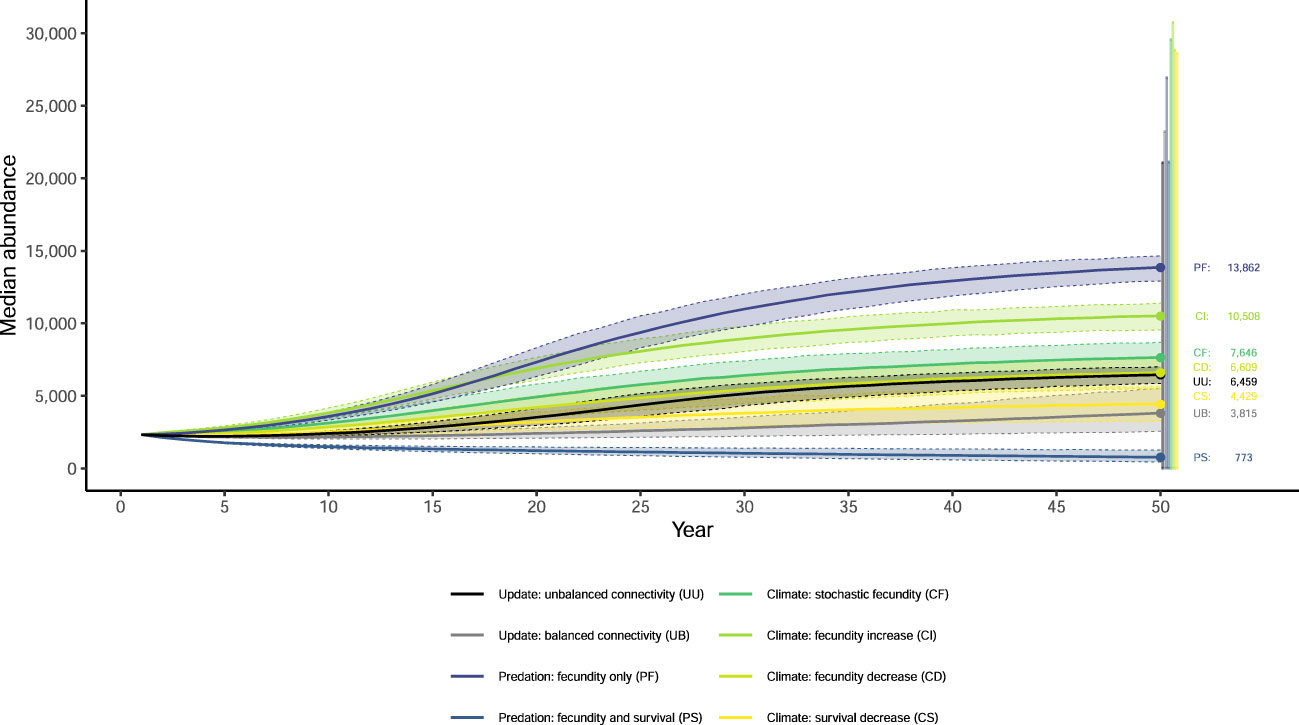
Figure 3 Median abundance of females and associated 95% quantiles from 1,000 outer-loop replicates (dashed lines) after 50 years for eight updated simulations of population viability for the northern Great Plains piping plover (Charadrius melodus circumcinctus) population. Error bars show the 95% quantiles of the secondary replicates at 50 years.
3.1 Updated estimates
Using the unbalanced connectivity estimates informed by empirical rates and the proportion of known movements between breeding groups (UU), the mean population growth rate was 0.973 (0.970, 0.976; Figure 1), extinction probability was 0.164 (0.144, 0.187; Figures 2, S2), and median abundance after 50 years was 6,459 females (secondary replicates 95% quantiles: 7 – 21,082; Figure 3) for the entire NGP population. The Southern Rivers and U.S. Alkali Wetlands breeding groups had the lowest extinction probabilities after 50 years (0.207 and 0.209, respectively; Figures 2, S3). The Southern Rivers was the only breeding group with a median predicted abundance of over 1,000 females after 50 years (Figure S4).
With higher, but balanced, connectivity (UB), the NGP population faced lower population viability (population growth rate: 0.965 [0.961, 0.968], Figure 1; extinction probability: 0.219 [0.195, 0.246], Figure 2; abundance: 3,815 females [6 – 23,217 females], Figure 3). The population was evenly split among the four breeding groups after 50 years (Figure S3; median abundance proportions; Prairie Canada: 17.7%, U.S. Alkali Wetlands: 20.0%, Northern Rivers: 17.8%, and Southern Rivers: 22.3%) with fewer than 900 females predicted in all four breeding groups (Figure S4). Neither the full population nor any of the breeding groups had a population growth rate equal to or greater than one (Figure 1). Lastly, all four breeding groups had mean extinction probabilities over 0.26 (Prairie Canada: 0.283, U.S. Alkali Wetlands: 0.277, Northern Rivers: 0.286, and Southern Rivers: 0.268; Figure 2).
3.2 Predation management
With increased fecundity due to widespread predation management (PF), extinction probability after 50 years for the NGP population (0.088, Figure 2) was at its lowest of all the simulations. The mean population growth rate was near 1.00 for the Northern Rivers breeding group (1.001, Figure 1) and U.S. Alkali Wetlands (population growth rate: 0.999, Figure 1). However, when increased fecundity was simulated with a decrease in adult survival (PS), no breeding groups had a population growth rate over 0.955 (Figure 1). Extinction probabilities were higher for the NGP population (0.373, Figure 2) and all four breeding groups, each of which exceeded 0.414 (Figure 2). There was a broad difference in the projected median abundance of females after 50 years between the two predation management simulations. The NGP population increased to 13,862 females (11 – 26,939 females) with increased fecundity (PF) but declined to only 773 females (4 – 21,125 females) with the addition of a decrease in adult survival (PS; Figure 3). In both predation management simulations, the U.S. Alkali Wetlands had the lowest extinction probability among breeding groups (0.116 and 0.414, without and with effects on adult survival respectively, Figure 2) and greatest proportion of the NGP population (0.27 and 0.26, respectively).
3.3 Climate change
Increasing the variance around fecundity estimates (Figure S5A) to represent stochasticity had little effect on population viability (CF). The mean population growth rate was 0.983 (0.977, 0.989; Figure 1), extinction probability was 0.173 (0.149, 0.195, Figure 2), and median abundance after 50 years was 7,646 females (1 – 29,573 females) for the entire NGP population (Figure 3). The U.S. Alkali Wetlands group had the lowest extinction probability (0.217), and Prairie Canada had the highest (0.242, Figure 2). As predicted, skewing fecundity above average (CI; Figure S5B) improved population viability while skewing fecundity below average (CD; Figure S5C) had the opposite effect.
Simulating stochastic fecundity and stochastic, skewed below average survival (CS; Figure S5D) resulted in reduced population viability compared to the other climate change scenarios. Extinction probability for the NGP population was 0.267 (0.240, 0.294, Figure 2) and mean population growth rate was 0.953 (0.944, 0.962; Figure 1). Of the four breeding groups, Prairie Canada had the highest extinction probability (0.334, Figure 2) and lowest population growth rate (0.940, Figure 1).
4 Discussion
After updating connectivity rates to reflect current knowledge rather than expert opinion, our work indicates that population viability for piping plovers in the northern Great Plains is lower than previously assumed. If there is no change to the current driving forces of vital rates, the extinction probability of the NGP population is between 0.164 and 0.219 (UU and UB, respectively). Additionally, our results provide information on the relative risks and impacts of global climate change as well as potential of certain management actions on population viability. Management efforts to improve fecundity (e.g., nest exclosures; PF) greatly reduced extinction probability (0.088), but only if there was no concurrent reduction in adult survival (PS; extinction probability: 0.373). Overall, relative improvements to population viability occurred when fecundity was increased (either through predation management or as a potential artifact of climate change); whereas population viability was the poorest in scenarios that simulated a reduction in adult survival, which provides guidance for future management decisions.
Management of a listed species relies on the continual reassessment of knowledge and adaption of actions in response. In the most recent PVA for NGP plovers (McGowan et al., 2014), mean population growth rates were higher and extinction probabilities were lower than previous efforts (Ryan et al., 1993; Plissner and Haig, 2000; Larson et al., 2002; McGowan and Ryan, 2009; McGowan et al., 2014). McGowan et al. (2014) hypothesized three possibilities for this estimate of improved population viability, two of which may explain the reduction of population viability observed in our study. First, fecundity estimates were substantially increased in the Southern Rivers breeding group in the original assessment due to intensive habitat and predation management on the Gavins Point Reach and Lewis and Clark Lake areas (Catlin et al., 2015). Our simulation used a lower estimate of fecundity for the Southern Rivers group that incorporated empirically-derived estimates from nearly all breeding areas in this group (including 14 years of monitoring on the central Platte River, 9 years on the lower Platte, Elkhorn, and Loup Rivers, and 8 years on the Gavins Point Reach and Lewis and Clark Lake sections of the Missouri River). Second, McGowan et al. (2014) stated that the metapopulation structure of the four breeding groups likely insulated the overall population from extinction. Our much higher estimates of connectivity are similar to a post-hoc analysis in McGowan et al. (2014) showing higher extinction probability when the metapopulation structure was lessened. By decreasing fecundity estimates in the Southern Rivers and increasing connectivity among the four breeding groups, population viability was reduced and was more in line with earlier assessments of population viability. This current assessment of population viability was able to take advantage of updated information to fill information gaps in these two crucial aspects of the model, yet uncertainty remains for a number of parameters including initial population size, which the model is particularly sensitive towards (McGowan et al., 2014). Additionally, by maintaining the original model structure and assumptions, we were able to directly assess the effects of updated information (including increased connectivity) on population viability through comparisons to earlier results. Updated vital rate estimates therefore indicate that NGP plover population viability is lower than assumed in earlier evaluations.
For listed species, PVA is useful to set and evaluate measurable recovery objectives (Morris et al., 2002; Zeigler et al., 2013; McGowan et al., 2014). The NGP plover population has multiple recovery goals between the United States and Canada (USFWS, 1988; USFWS, 2016 [draft]; Environment Canada, 2006). Under three scenarios (PF after 14 years, CF after 25 years, and CI after 9 years) the median abundance breached the minimum population of 813 pairs for at least 11 years in Prairie Canada (Environment Canada, 2006) – even though we did not simulate an increase in fecundity for this breeding group. This suggests that even moderate connectivity to the U.S. breeding range has the potential to increase the portion of the population breeding in Canada. However, none of our scenarios meet a viable population definition in the U.S. breeding range which is designated by a “less than 5 percent likelihood of extinction in the next 50 years” (USFWS, 2016). Additional U.S. recovery criteria include stable or increasing population growth over 50 years, which was never met for all four breeding groups, and at least 15% of the population in each of the four breeding groups, which was commonly met with high connectivity estimates in our scenarios (USFWS, 2016). This suggests that under the conditions simulated here, NGP piping plovers would require continued management and conservation actions to preserve existing populations, particularly in the U.S. portion of the breeding range.
The comparisons of different future effect scenarios showed that conservation or management actions that improved fecundity reduced extinction probabilities and improved population viability (three of eight scenarios). While a variety of management actions can improve fecundity for piping plovers (e.g., habitat restoration, predation management), we focused on the effects of one widely used tool, nest exclosures. Our results indicate that plover viability may respond positively to predation management actions, such as the widespread use of nest exclosures, to increase fecundity as long as it does not affect other vital rates (i.e., reduced adult survival, changes to chick survival). This highlights the need to fully understand the composite effects of management actions on multiple demographic rates and compare alternative management actions. For snowy plovers, the use of nest exclosures prohibits population recovery because of a reduction adult survival, but lethal predator removal could facilitate population recovery (Watts et al., 2012; Eberhart-Phillips and Colwell, 2014). Other management actions may have a similar effect, but the magnitude of the effect on fecundity would require additional study. The use of nest exclosures on other species where it has been shown to improve fecundity (Rimmer and Deblinger, 1990; Mabee and Estelle, 2000; Isaksson et al., 2007; Dinsmore et al., 2014) may yield similar improved population viability if their use does not affect adult survival as well. Previous simulations indicated that the use of predator exclosures to increase fledging rates could stabilize the NGP population (Larson et al., 2002). A recent experimental study in the U.S. Alkali Wetlands found no detrimental effects and only marginal increases to chick survival with the use of nest exclosures (Anteau et al., 2022). Predation risk for adults using exclosures and the effectiveness of exclosures for protecting nests may vary with predator communities (Anteau et al., 2022). Furthermore, predator communities likely vary among habitat types (e.g., wetland margins, riverine sandbars, reservoir shorelines, and sand and gravel mines) and geography within the NGP (Ivan and Murphy, 2005; Catlin et al., 2011; Andes et al., 2019). It is therefore critical that future research focuses on understanding, via experimental manipulation, the potential ramifications of utilizing predation management, particularly nest exclosures, in habitats outside of the U.S. Alkali Wetlands to determine if nest exclosures may be a viable management tool to reduce extinction probabilities for plovers.
All eight of our scenarios included higher estimates of connectivity than previously assumed for this population. In previous simulations (McGowan et al., 2014), a presumed metapopulation structure that was expressed through a balanced and low rate of connectivity (0.006) insulated the NGP population from extinction. Our simulations were based on updated, empirically-based connectivity rates (lowest rate of 0.009 or 0.017) and resulted in mean extinction probabilities that were 4.9x (0.164; unbalanced connectivity) or 6.6x (0.219; balanced connectivity) higher than derived by McGowan et al. (2014). None of our subsequent simulations that introduced variation due to management actions or climate change resulted in mean population extinction probability as low as the 0.033 estimated by McGowan et al. (2014), and the most favorable scenario (PF) still had a mean extinction probability that was 2.6x higher (0.088; Figure 2). This indicates that the higher connectivity rates underlying our simulations had a very strong negative influence on population viability that was difficult to overcome even with aggressive range-wide management aimed at improving fecundity. Metapopulations are characterized as subpopulations linked by rare dispersal events that can recolonize areas that go extinct (Hanski, 1998; Fronhofer et al., 2012). In fact, when connectivity is high or strongly, positively autocorrelated over time, metapopulations can be more prone to extinction (Perry and Lee, 2019). To meet the classical metapopulation definition, demographically-independent, spatially-segregated, distinct breeding populations must be prone to extinction and recolonization (Hanski, 1998; Fronhofer et al., 2012). Demographic independence allows for strong populations to rescue declining populations (Hanski, 1998). While this model allows for dynamic extinction and recolonization to occur for a breeding group, the high rates of connectivity led to the NGP population being evenly distributed among defined breeding groups, and extinction probabilities increased similarly among the four breeding groups in five of eight scenarios (unless fecundity was increased at different rates). As has been shown previously (Roche et al., 2010b; Roche et al., 2016b; Ellis et al., 2021), this indicates that vital rates for breeding areas in the NGP may be highly synchronous as high connectivity links semi-independent breeding areas and therefore does not fit the classical definition of a metapopulation. Future reassessments of population viability might focus on re-evaluating the defined metapopulation structure to more accurately reflect observed species life history in this region and improve predictive accuracy.
The role of PVA in conservation decision-making has been debated (Wolf et al., 2015; Chaudhary and Oli, 2020). Recovery criteria have at times been criticized for lacking consistency, transparency, objectivity, and scientific justification (Neel et al., 2012; Zeigler et al., 2013; Boor, 2014; Doak et al., 2015). PVAs have been suggested as a rigorous tool that can be utilized, when data to support such analyses are available, to support the recovery planning process (Morris et al., 2002; Boor, 2014; Doak et al., 2015). And while increasing use of PVAs in defining recovery objectives adds scientific rigor to the recovery planning process to address these concerns, others have argued that many studies are poorly executed, not reproducible or replicable, or inconsistent with species’ life histories (Pe’er et al., 2013; Wolf et al., 2015; Chaudhary and Oli, 2020). Updating a PVA with new information, as we have done here, can play an important role at improving the use of PVAs in conservation decision-making. Replacing data in PVA that are informed by expert opinion or that the model is sensitive to can improve the science used to inform conservation decision-making. Here we showed that updating a PVA with more recent data, filling in gaps previously informed by expert opinion, and testing alternative future scenarios can change the outlook of population viability for a listed species. Using periodically updated PVA in this way to re-evaluate relative estimates of population viability by exploring the effects of updated information, testing recovery action alternatives, and addressing uncertainties can be a useful strategy to improve conservation decision-making for listed species.
Quantifying the future effects on a population of a protected species is often challenging due to limitation of knowledge. Vital rate estimates for plovers are available for many breeding areas in the NGP, yet some uncertainties remain (e.g., long-term connectivity rates, directionality of effects of climate change, initial population size, etc.). We addressed many of these concerns by simulating multiple alternatives, providing credible intervals, and comparing relative differences among various scenarios. It is still important to acknowledge that this PVA is a simplification of plover biology and only an approximation of potential futures. Notwithstanding such limitations, PVA allows us to demonstrate that within the plausible range of vital rate estimates used in each of these scenarios, piping plover population viability in the NGP was lower than previously assumed – particularly with high rates of connectivity within the NGP. Our results also highlight the importance of periodic reassessments of knowledge and potentially updating PVA when needed to improve conservation and management actions. Simulating the trending effect (i.e., positive or negative) of global climate change can lead to more informed management decisions in the future, as new information is gained on how climate projections may affect fecundity and survival. These simulations provide important insights into the potential long-term impact of high rates of connectivity, low adult survival as a result of management actions, and potential impacts of climate change.
Data availability statement
Publicly available datasets were analyzed in this study. The names of the repository/repositories and accession number(s) can be found in the article/Supplementary Material.
Ethics statement
Ethical review and approval was not required for the animal study because this study did not involved the capture and handling of animals. Instead, this study utilizes data collected by others.
Author contributions
All authors contributed to the study conception and design. RS led the analysis and the writing of the manuscript. All authors contributed to the article and approved the submitted version.
Funding
We thank the U.S. Fish and Wildlife Service and U.S. Geological Survey – Northern Prairie Wildlife Research Center for financial support to conduct this work.
Acknowledgments
The authors are grateful to Conor McGowan for providing the R code for the simulation model and to our U.S. Fish and Wildlife Service partners for input on future scenarios relevant to managing the species. We also thank Michelle Stantial and Megan Milligan for reviews on an earlier draft.
Conflict of interest
The authors RS and MA declared that they were editorial board members of Frontiers, at the time of submission. This had no impact on the peer review process and the final decision.
The remaining authors declare that the research was conducted in the absence of any commercial or financial relationships that could be construed as a potential conflict of interest.
Publisher’s note
All claims expressed in this article are solely those of the authors from the U.S. Geological Survey and do not represent the views of those of the publisher, the editors, and the reviewers but do represent the views of the U.S. Geological Survey. Any product that may be evaluated in this article, or claim that may be made by its manufacturer, is not guaranteed or endorsed by the publisher.
Author disclaimer
Any use of trade, product, or firm names is for descriptive purposes only and does not imply endorsement by the U.S. Government.
Supplementary material
The Supplementary Material for this article can be found online at: https://www.frontiersin.org/articles/10.3389/fbirs.2023.1157682/full#supplementary-material
References
Amirault-Langlais D. L., Imlay T. L., Boyne A. W. (2014). Dispersal patterns suggest two breeding populations of piping plovers in eastern Canada. Wilson J. @ Ornithol. 126, 352–359. doi: 10.1676/13-056.1
Andes A. K., Shaffer T. L., Sherfy M. H., Hofer C. M., Dovichin C. M., Ellis-Felege S. N. (2019). Accuracy of nest fate classification and predator identification from evidence at nests of least terns and piping plovers. Ibis 161, 286–300. doi: 10.1111/ibi.12629
Anteau M. J., Shaffer T. L., Wiltermuth M. T., Sherfy M. H. (2014b). Landscape selection by piping plovers has implications for measuring habitat and population size. Landsc. Ecol. 29, 1033–1044. doi: 10.1007/s10980-014-0041-z
Anteau M. J., Sherfy M. H., Shaffer T. L., Swift R. J., Toy D. L., Dovichin C. M. (2019). Demographic responses of least terns and piping plovers to the 2011 Missouri river flood–a large-scale case study (US Geological Survey 2018-1176). doi: 10.3133/ofr20181176
Anteau M. J., Swift R. J., Sherfy M. H., Koons D. N., Ellis K. S., Shaffer T. L., et al. (2022). Experimental evaluation of predator exclosures on nest, chick, and adult survival of piping plovers. J. Wildl. Manage. 86, e22139. doi: 10.1002/jwmg.22139
Anteau M. J., Wiltermuth M. T., Sherfy M. H., Shaffer T. L. (2014a). Measuring and predicting abundance and dynamics of habitat for piping plovers on a large reservoir. Ecol. Modell. 272, 16–27. doi: 10.1016/j.ecolmodel.2013.08.020
Beissinger S. R., Westphal M. I. (1998). On the use of demographic models of population viability in endangered species management. J. Wildl. Manage. 62, 821–841. doi: 10.2307/3802534
Bender M. A., Knutson T. R., Tuleya R. E., Sirutis J. J., Vecchi G. A., Garner S. T., et al. (2010). Modeled impact of anthropogenic warming on the frequency of intense Atlantic hurricanes. Science 327, 454–458. doi: 10.1126/science.1180568
Boor G. K. H. (2014). A framework for developing objective and measurable recovery criteria for threatened and endangered species. Conserv. Biol. 28, 33–43. doi: 10.1111/cobi.12155
Brook B. W., O'Grady J. J., Chapman A. P., Burgman M. A., Akcakaya H. R., Frankham R. (2000). Predictive accuracy of population viability analysis in conservation biology. Nature 404, 385–387. doi: 10.1038/35006050
Cahill A. E., Aiello-Lammens M. E., Fisher-Reid M. C., Hua X., Karanewsky C. J., Yeong Ryu H., et al. (2013). How does climate change cause extinction? Proc. R. Soc B Biol. Sci. 280, 20121890. doi: 10.1098/rspb.2012.1890
Catlin D. H. (2009). Population dynamics of piping plovers (Charadrius melodus) on the Missouri river (PhD dissertation, Virginia Tech University). Available at: https://vtechworks.lib.vt.edu/handle/10919/27442.
Catlin D. H., Felio J. H., Fraser J. D. (2011). Effect of great-horned owl trapping on chick survival in piping plovers. J. Wildl. Manage. 75, 458–462. doi: 10.1002/jwmg.56
Catlin D. H., Fraser J. D., Felio J. H. (2015). Demographic responses of piping plovers to habitat creation on the Missouri river. Wildl. Monogr. 192, 1–42. doi: 10.1002/wmon.1016
Catlin D. H., Gibson D., Hunt K. L., Friedrich M. J., Weithman C. E., Karpanty S. M., et al. (2019). Direct and indirect effects of nesting density on survival and breeding propensity of an endangered shorebird. Ecosphere 10, e02740. doi: 10.1002/ecs2.2740
Catlin D. H., Zeigler S. L., Brown M. B., Dinan L. R., Fraser J. D., Hunt K. L., et al. (2016). Metapopulation viability of an endangered shorebird depends on dispersal and human-created habitats: piping plovers (Charadrius melodus) and prairie rivers. Mov. Ecol. 4, 1–15. doi: 10.1186/s40462-016-0072-y
Ceballos G., Ehrlich P. R., Raven P. H. (2020). Vertebrates on the brink as indicators of biological annihilation and the sixth mass extinction. Proc. Natl. Acad. Sci. U.S.A. 117, 13596–13602. doi: 10.1073/pnas.1922686117
Chaudhary V., Oli M. K. (2020). A critical appraisal of population viability analysis. Conserv. Biol. 34, 26–40. doi: 10.1111/cobi.13414
Cohen J. B., Houghton L. M., Fraser J. D. (2009). Nesting density and reproductive success of piping plovers in response to storm-and human-created habitat changes. Wildl. Monogr. 173, 1–24. doi: 10.2193/2007-553
Cook C. N., Carter R. B., Hockings M. (2014). Measuring the accuracy of management effectiveness evaluations of protected areas. J. Environ. Manage. 139, 164–171. doi: 10.1016/j.jenvman.2014.02.023
COSEWIC (2001). COSEWIC assessment and update status report on the Piping Plover circumcinctus subspecies (Charadrius melodus circumcinctus) and the melodus subspecies (Charadrius melodus melodus), in Canada. (Ottawa: Committee on the Status of Endangered Wildlife in Canada) 33.
Coulson T., Mace G. M., Hudson E., Possingham H. (2001). The use and abuse of population viability analysis. Trends Ecol. Evol. 16, 219–221. doi: 10.1016/S0169-5347(01)02137-1
Dinsmore S. J., Lauten D. J., Castelein K. A., Gaines E. P., Stern M. A. (2014). Predator exclosures, predator removal, and habitat improvement increase nest success of snowy plovers in Oregon, USA. Condor 116, 619–628. doi: 10.1650/CONDOR-14-7.1
Doak D. F., Boor G. K. H., Bakker V. J., Morris W. F., Louthan A., Morrison S. A., et al. (2015). Recommendations for improving recovery criteria under the US endangered species act. BioScience 65, 189–199. doi: 10.1093/biosci/biu215
Doherty T. S., Glen A. S., Nimmo D. G., Ritchie E. G., Dickman C. R. (2016). Invasive predators and global biodiversity loss. Proc. Natl. Acad. Sci. U.S.A. 113, 11261–11265. doi: 10.1073/pnas.1602480113
Duarte A., Pearl C. A., Adams M. J., Peterson J. T. (2017). A new parameterization for integrated population models to document amphibian reintroductions. Ecol. Appl. 27, 1761–1775. doi: 10.1002/eap.1564
Eberhart-Phillips L. J., Colwell M. A. (2014). Conservation challenges of a sink: the viability of an isolated population of the snowy plover. Bird Conserv. Int. 24, 327–341. doi: 10.1017/S0959270913000506
Elliott-Smith E., Bidwell M., Holland A. E., Haig S. M. (2015). Data from the 2011 international piping plover census (U.S. Geological Survey Data Series), 922. doi: 10.3133/ds922
Elliott-Smith E., Haig S. M., Powers B. M. (2009). Data from the 2006 international piping plover census (U.S. Geological Survey Data Series), 426. doi: 10.3133/ds426
Ellis K. S., Anteau M. J., Cuthbert F. J., Gratto-Trevor C. L., Jorgensen J. G., Newstead D. J., et al. (2021). Impacts of extreme environmental disturbances on piping plover survival are partially moderated by migratory connectivity. Biol. Cons. 264, 109371. doi: 10.1016/j.biocon.2021.109371
Ellner S. P., Fieberg J., Ludwig D., Wilcox C. (2002). Precision of population viability analysis. Conserv. Bio. 16, 258–261. doi: 10.1046/j.1523-1739.2002.00553.x
Environment Canada (2006). Recovery strategy for the piping plover (Charadrius melodus circumcinctus) in canada. species at risk act recovery strategy series (Ottawa: Environment Canada).
Fronhofer E. A., Kubisch A., Hilker F. M., Hovestadt T., Poethke H. J. (2012). Why are metapopulations so rare? Ecology 93, 1967–1978. doi: 10.1890/11-1814.1
Gaines E. P., Dinsmore S. J., Murphy M. T. (2020). Effects of management for productivity on adult survival of snowy plovers. J. Field Ornithol. 91, 130–141. doi: 10.1111/jofo.12330
Haig S. M., Oring L. W. (1988). Distribution and dispersal in the piping plover. Auk 105, 630–638. doi: 10.1093/auk/105.4.630
Hillman M. D., Karpanty S. M., Fraser J. D., Cuthbert F. J., Altman J. M., Borneman T. E., et al. (2012). Evidence for long-distance dispersal and successful interpopulation breeding of the endangered piping plover. Waterbirds 35, 642–644. doi: 10.1675/063.035.0414
Hooper D. U., Adair E. C., Cardinale B. J., Byrnes J. E., Hungate B. A., Matulich K. L., et al. (2012). A global synthesis reveals biodiversity loss as a major driver of ecosystem change. Nature 486, 105–108. doi: 10.1038/nature11118
Hunt K. L., Fraser J. D., Friedrich M. J., Karpanty S. M., Catlin D. H. (2018). Demographic response of piping plovers suggests that engineered habitat restoration is no match for natural riverine processes. Condor 120, 149–165. doi: 10.1650/CONDOR-17-93.1
IPCC (2021). Climate change 2021: the physical science basis. contribution of working group I to the sixth assessment report of the intergovernmental panel on climate change. Eds. Masson-Delmotte V., Zhai P., Pirani A., Connors S. L., Péan C., Berger S., Caud N., Chen Y., Goldfarb L., Gomis M. I., Huang M., Leitzell K., Lonnoy E., Matthews J. B. R., Maycock T. K., Waterfield T., Yelekçi O., Yu R., Zhou B. (Cambridge, United Kingdom and New York, NY, USA: Cambridge University Press).
Isaksson D., Wallander J., Larsson M. (2007). Managing predation on ground-nesting birds: the effectiveness of nest exclosures. Biol. Conserv. 136, 136–142. doi: 10.1016/j.biocon.2006.11.015
Ivan J. S., Murphy R. K. (2005). What preys on piping plover eggs and chicks? Wildl. Soc Bull. 33, 113–119. doi: 10.2193/0091-7648(2005)33[113:WPOPPE]2.0.CO;2
Jarzyna M. A., Zuckerberg B., Finley A. O., Porter W. F. (2016). Synergistic effects of climate and land cover: grassland birds are more vulnerable to climate change. Landsc. Ecol. 31, 2275–2290. doi: 10.1007/s10980-016-0399-1
Jorgensen J. G., Brenner S. J., Greenwalt L. R., Vrtiska M. P. (2021). Decline of novel ecosystems used by endangered species: the case of piping plovers, least terns, and aggregate mines. Ecosphere 12, e03474. doi: 10.1002/ecs2.3474
Larson M. A., Ryan M. R., Murphy R. K. (2002). Population viability of piping plovers: effects of predator exclusion. J. Wildl. Manage. 66, 361–371. doi: 10.2307/3803169
Luck C., Jessopp M., Cronin M., Rogan E. (2022). Using population viability analysis to examine the potential long-term impact of fisheries bycatch on protected species. J. Nat. Conserv. 67, 126157. doi: 10.1016/j.jnc.2022.126157
Mabee T. J., Estelle V. B. (2000). Assessing the effectiveness of predator exclosures for plovers. Wilson Bull. 112, 14–20. doi: 10.1676/0043-5643(2000)112[0014:ATEOPE]2.0.CO;2
Maclean I. M., Wilson R. J. (2011). Recent ecological responses to climate change support predictions of high extinction risk. Proc. Natl. Acad. Sci. U.S.A. 108, 12337–12342. doi: 10.1073/pnas.1017352108
Martin T. E. (1993). Nest predation and nest sites: new perspectives on old patterns. BioScience 43, 523–532. doi: 10.2307/1311947
Martin T. G., Nally S., Burbidge A. A., Arnall S., Garnett S. T., Hayward M. W., et al. (2012). Acting fast helps avoid extinction. Conserv. Lett. 5, 274–280. doi: 10.1111/j.1755-263X.2012.00239.x
Maslo B., Lockwood J. L. (2009). Evidence-based decisions on the use of predator exclosures in shorebird conservation. Biol. Conserv. 142, 3213–3218. doi: 10.1016/j.biocon.2009.07.034
McCauley L. A., Anteau M. J., Post van der Burg M. (2016). Consolidation drainage and climate change may reduce piping plover habitat in the great plains. J. Fish Wildl. Manage. 7, 4–12. doi: 10.3996/072015-JFWM-068
McGowan C. P., Catlin D. H., Shaffer T. L., Gratto-Trevor C. L., Aron C. (2014). Establishing endangered species recovery criteria using predictive simulation modeling. Biol. Conserv. 177, 220–229. doi: 10.1016/j.biocon.2014.06.018
McGowan C. P., Ryan M. R. (2009). A quantitative framework to evaluate incidental take and endangered species population viability. Biol. Conserv. 142, 3128–3136. doi: 10.1016/j.biocon.2009.08.012
Melvin S. M., Macivor L. H., Griffin C. R. (1992). Predator exclosures: a technique to reduce predation at piping plover nests. Wildl. Soc Bull. 20, 143–148. Available at: https://www.jstor.org/stable/3782887.
Milligan M. C., Wells S. L., McNew L. B. (2018). A population viability analysis for sharp-tailed grouse to inform reintroductions. J. Fish Wildl. Manage. 9, 565–581. doi: 10.3996/112017-JFWM-090
Moritz C., Agudo R. (2013). The future of species under climate change: resilience or decline? Science 341, 504–508. doi: 10.1126/science.1237190
Morris W. F., Bloch P. L., Hudgens B. R., Moyle L. C., Stinchcombe J. R. (2002). Population viability analysis in endangered species recovery plans: past use and future improvements. Ecol. Appl. 12, 708–712. doi: 10.1890/1051-0761(2002)012[0708:PVAIES]2.0.CO;2
Murphy R. K., Greenwood R. G., Ivan J. S., Smith K. A. (2003a). Predator exclusion methods for managing endangered shorebirds: are two barriers better than one? Waterbirds 26, 156–159. doi: 10.1675/1524-4695(2003)026[0156:PEMFME]2.0.CO;2
Murphy R. K., Michaud I. M. G., Prescott D. R. C., Ivan J. S., Anderson B. J., French-Pombier M. L. (2003b). Predation on adult piping plovers at predator exclosure cages. Waterbirds 26, 150–155. doi: 10.1675/1524-4695(2003)026[0150:POAPPA]2.0.CO;2
Neel M. C., Leidner A. K., Haines A., Goble D. D., Scott J. M. (2012). By the numbers: how is recovery defined by the US endangered species act? BioScience 62, 646–657. doi: 10.1525/bio.2012.62.7.7
Pe’er G., Matsinos Y. G., Johst K., Franz K. W., Turlure C., Radchuk V., et al. (2013). A protocol for better design, application, and communication of population viability analyses. Conserv. Biol. 27, 644–656. doi: 10.1111/cobi.12076
Perry G. L., Lee F. (2019). How does temporal variation in habitat connectivity influence metapopulation dynamics? Oikos 128, 1277–1286. doi: 10.1111/oik.06052
Pimm S. L., Jenkins C. N., Abell R., Brooks T. M., Gittleman J. L., Joppa L. N., et al. (2014). The biodiversity of species and their rates of extinction, distribution, and protection. Science 344, 1246752. doi: 10.1126/science.1246752
Plissner J. H., Haig S. M. (2000). Status of a broadly distributed endangered species: results and implications of the second international piping plover census. Can. J. Zool. 78, 128–139. doi: 10.1139/z99-179
Pullin A. S., Knight T. M., Stone D. A., Charman K. (2004). Do conservation managers use scientific evidence to support their decision-making? Biol. Conserv. 119, 245–252. doi: 10.1016/j.biocon.2003.11.007
Radchuk V., Oppel S., Groeneveld J., Grimm V., Schtickzelle N. (2016). Simple or complex: relative impact of data availability and model purpose on the choice of model types for population viability analyses. Ecol. Modell. 323, 87–95. doi: 10.1016/j.ecolmodel.2015.11.022
R Core Team (2020). R: a language and environment for statistical computing (Vienna, Austria: R Foundation for Statistical Computing).
Reed J. M., Mills L. S., Dunning J. B. Jr., Menges E. S., McKelvey K. S., Frye R., et al. (2002). Emerging issues in population viability analysis. Conserv. Biol. 16, 7–19. doi: 10.1046/j.1523-1739.2002.99419.x
Rimmer D. W., Deblinger R. D. (1990). Use of predator exclosures to protect piping plover nests. J. Field Ornithol. 61, 217–223. Available at: https://www.jstor.org/stable/4513532.
Robinson S. G., Gibson D., Riecke T. V., Fraser J. D., Bellman H. A., DeRose-Wilson A., et al. (2020). Piping plover population increase after hurricane sandy mediated by immigration and reproductive output (Condor), 122. doi: 10.1093/condor/duaa041
Roche E. A., Arnold T. W., Cuthbert F. J. (2010a). Apparent nest abandonment as evidence of breeding-season mortality in great lakes piping plovers (Charadrius melodus). Auk 127, 402–410. doi: 10.1525/auk.2009.09034
Roche E. A., Cohen J. B., Catlin D. H., Amirault-Langlais D. L., Cuthbert F. J., Gratto-Trevor C. L., et al. (2010b). Range-wide piping plover survival: correlated patterns and temporal declines. J. Wildl. Manage. 74, 1784–1791. doi: 10.2193/2009-446
Roche E. A., Shaffer T. L., Dovichin C. M., Sherfy M. H., Anteau M. J., Wiltermuth M. T. (2016b). Synchrony of piping plover breeding populations in the U.S. Northern Great Plains. Condor 118, 558–570. doi: 10.1650/CONDOR-15-195.1
Roche E. A., Sherfy M. H., Ring M. M., Shaffer T. L., Anteau M. J., Stucker J. H. (2016a). Demographics and movements of least terns and piping plovers in the central platte river valley, Nebraska (US Geological Survey 2016-1061). doi: 10.3133/ofr20161061
Rosenberg K. V., Dokter A. M., Blancher P. J., Sauer J. R., Smith A. C., Smith P. A., et al. (2019). Decline of the north American avifauna. Science 366, 120–124. doi: 10.1126/science.aaw1313
Ryan M. R., Root B. G., Mayer P. M. (1993). Status of piping plovers in the great plains of north America: a demographic simulation model. Conserv. Biol. 7, 581–585. doi: 10.1046/j.1523-1739.1993.07030581.x
Saunders S. P., Arnold T. W., Roche E. A., Cuthbert F. J. (2014). Age-specific survival and recruitment of piping plovers Charadrius melodus in the great lakes region. J. Avian Biol. 45, 437–449. doi: 10.1111/jav.00319
Saunders S. P., Cuthbert F. J., Zipkin E. F. (2018). Evaluating population viability and efficacy of conservation management using integrated population models. J. Appl. Ecol. 55, 1380–1392. doi: 10.1111/1365-2664.13080
Shaffer M. L. (2019). “Population viability analysis,” in Challenges in the conservation of biological resources (Routledge), 107–118.
Shivik J. A., Treves A., Callahan P. (2003). Nonlethal techniques for managing predation: primary and secondary repellents. Conserv. Biol. 17, 1531–1537. doi: 10.1111/j.1523-1739.2003.00062.x
Smith K. F., Acevedo-Whitehouse K., Pedersen A. B. (2009). The role of infectious diseases in biological conservation. Anim. Conserv. 12, 1–12. doi: 10.1111/j.1469-1795.2008.00228.x
Smith R. K., Pullin A. S., Stewart G. B., Sutherland W. J. (2011). Is nest predator exclusion an effective strategy for enhancing bird populations? Biol. Conserv. 144, 1–10. doi: 10.1016/j.biocon.2010.05.008
Stantial M. L. (2020). Factors limiting abundance and productivity of piping plovers (Charadrius melodus) in new Jersey (State University of New York College of Environmental Science and Forestry: PhD dissertation).
Swift R. J., Anteau M. J., Ellis K. S., Ring M. M., Sherfy M. H., Toy D. L. (2021b). Dispersal distance is driven by habitat availability and reproductive success in northern great plains piping plovers. Mov. Ecol. 9, 1–14. doi: 10.1186/s40462-021-00293-3
Swift R. J., Anteau M. J., Ellis K. S., Ring M. M., Sherfy M. H., Toy D. L., et al. (2021a). Spatial variation in population dynamics of northern great plains piping plovers (U.S. Geological Survey Open-File Report 2020-1152). doi: 10.3133/ofr20201152
Swift R. J., Anteau M. J., Ellis K. S., Ring M. M., Sherfy M. H., Toy D. L., et al. (2022). Implications of habitat-driven survival and dispersal on recruitment in a spatially structured piping plover population. Ecosphere 13, e4190. doi: 10.1002/ecs2.4190
Swift R. J., Anteau M. J., Ring M. M., Toy D. L., Sherfy M. H. (2020). Low renesting propensity and reproductive success make renesting unproductive for the threatened piping plover (Charadrius melodus) (122, duz066: Condor).
Swift R. J., Anteau M. J., Ellis K. S., Ring M. M., Sherfy D. L., Toy M. H. (2023). Conspecific density and habitat quality affect breeding habitat selection: Support for the social attraction hypothesis. Ecosphere 14(5), e4524. doi: 10.1002/ecs2.4524
U.S. Fish and Wildlife Service (USFWS) (1985). Determination of endangered and threatened status for the piping plover (USFWS, Washington, D.C., USA).
U.S. Fish and Wildlife Service (USFWS) (1988). Great lakes and northern great plains piping plover recovery plan (MN, USA: Twin Cities).
U.S. Fish and Wildlife Service (USFWS) (2016). Draft revised recovery plan for the northern great plains piping plover (Charadrius melodus) (CO, USA: Denver).
U.S. Fish and Wildlife Service (USFWS) (2020). Piping plover (Charadrius melodus) 5-year review: summary and evaluation (MI, USA: East Lansing).
USGCRP (2018). Impacts, risks, and adaptation in the united states: fourth national climate assessment, volume II. Eds. Reidmiller D. R., Avery C. W., Easterling D. R., Kunkel K. E., Lewis K. L. M., Maycock T. K., Stewart B. C. (Washington, DC, USA: U.S. Global Change Research Program).
Watts C. M., Cao J., Panza C., Dugaw C., Colwell M., Burroughs E. A. (2012). Modeling the effects of predator exclosures on a western snowy plover population. Nat. Res. Model. 25, 529–547. doi: 10.1111/j.1939-7445.2012.00131.x
Wolf S., Hartl B., Carroll C., Neel M. C., Greenwald D. N. (2015). Beyond PVA: why recovery under the endangered species act is more than population viability. BioScience 65, 200–207. doi: 10.1093/biosci/biu218
Keywords: climate change, conservation scenarios, extinction probability, population viability analyses (PVA), predation management, protected species, adaptive management, metapopulation
Citation: Swift RJ, Anteau MJ, Ellis KS, MacDonald GJ, Ring MM, Sherfy MH and Toy DL (2023) Estimating population viability of the northern Great Plains piping plover population considering updated population structure, climate change, and intensive management. Front. Bird Sci. 2:1157682. doi: 10.3389/fbirs.2023.1157682
Received: 02 February 2023; Accepted: 17 May 2023;
Published: 02 June 2023.
Edited by:
David Koons, Colorado State University, United StatesReviewed by:
Elizabeth Hunter, United States Department of the Interior, United StatesChristy N. Wails, Virginia Tech, United States
Copyright © 2023 Swift, Anteau, Ellis, MacDonald, Ring, Sherfy and Toy. This is an open-access article distributed under the terms of the Creative Commons Attribution License (CC BY). The use, distribution or reproduction in other forums is permitted, provided the original author(s) and the copyright owner(s) are credited and that the original publication in this journal is cited, in accordance with accepted academic practice. No use, distribution or reproduction is permitted which does not comply with these terms.
*Correspondence: Rose J. Swift, rswift@usgs.gov