- Aquatic Global Change Ecology and Conservation Lab, University of Alberta, Department of Biological Sciences, Edmonton, AB, Canada
Identifying features of biogenic (i.e., living) habitat that attract and retain organisms is a key pursuit in ecological habitat selection research. Here we present an integrative method for creating aquatic artificial habitat modules that allow the user to isolate and flexibly manipulate structural and compositional features of replicated biogenic habitats for a range of habitat selection study designs in aquatic environments: This method combines techniques from engineering (3D scanning and printing), paleontology, and visual art (moulding and casting) into a stream-lined work flow that is likely to perform on par with or better than other techniques widely used to create artificial replicas of biogenic habitats in terms of design accessibility (availability and cost of construction materials and equipment, and training requirements), scalability (durability, ease of deployment, and reproducibility), and the ecology of the artificial habitat module (degree to which structural and compositional features of the habitat elicit appropriate visual, chemosensory, and auditory cues, and impact of the structure on the surrounding environment). This method can be flexibly modified to answer a variety of questions regarding habitat selection cues, for a range of aquatic biogenic habitat types, and can be adapted for theoretical and applied contexts including cue studies and restoration planning.
Introduction
Biogenic habitats (made of living organisms) are globally prevalent and provide critical resources for other species in high-biodiversity ecosystems (Loh et al., 2019). A major research theme in ecology is identifying attributes of biogenic habitats that enhance their detection and use by organisms, with the goal of predicting how changes in habitat quantity and quality influence resident communities (Mercader et al., 2019). While habitat ‘quantity’ may be estimated as the size, area, or volume of habitat-forming structures (Agudo-Adriani et al., 2016), indicators of habitat ‘quality’ are more varied and context specific. For example, architectural complexity affects ecological services such as shelter from predation and sites for reproduction and feeding (Cheminée et al., 2016). Species composition of the biogenic habitat also determines the quality of forage resources available to residents (Wilson et al., 2008). Many studies employ artificial habitats (AHs) to isolate and test the role of various structural and compositional features hypothesized to affect biogenic habitat quantity and quality, with responses measured as resident organism habitat selection (attraction) to and use (retention) of structures (Smith et al., 2016; Strain et al., 2018). Resident organisms sense biochemical features of high-value (e.g., high proportion living tissue) substrate via olfactory cues, and are subsequently retained via enhanced foraging resources the substrate provides. They also detect high-value three-dimensional features (e.g., high structural complexity) via visual cues, and are retained at habitats by shelter resources provided by the structure (Figure 1).
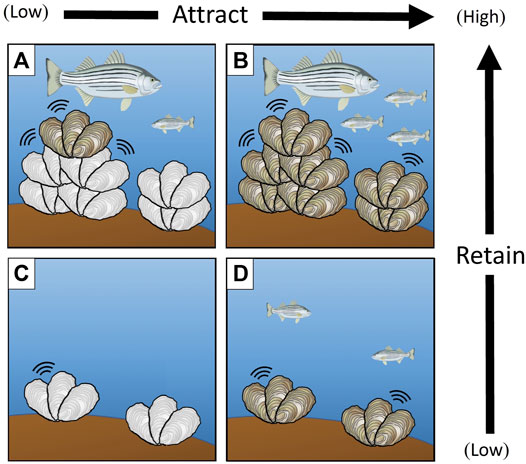
FIGURE 1. The structural complexity and substrate composition of biogenic habitats (e.g., oyster reef, family Ostriedae) host a range of visual, auditory, and chemosensory cues affecting the selection (attraction) and use (retention) of resident biota (e.g., Striped bass, Morone saxatilis). Composition mediates a range of visual, auditory, and chemosensory cues that attract resident species. Structural features influence the amount and type of shelter space and foraging resources available, influencing species retention. Studies integrating ecologically realistic artificial habitats (white oysters) with live biogenic habitat (grey oysters) are a useful tool for disentangling the relative influence of features affecting habitat selection (i.e., attraction) and use (i.e., retention). (A) Complex structural features and limited compositional cues are hypothesized to attract resident organisms at low rates, but retain organisms once attracted. (B) Complex structural features and high compositional cues are hypothesized to attract and retain species at high rates. (C) Few structural features or compositional cues are hypothesized to result in low species attraction and retention. (D) Few structural features but high compositional cues are hypothesized to attract organisms at high rates, but retain few organisms that are attracted.
Extensive background research on existing aquatic AH designs and deployment methods reveals several performance categories that impact their use in studies seeking to disentangle features affecting biogenic habitat selection: 1) accessibility (resource availability, cost, and training required to work with the AH construction materials and equipment), 2) scalability (durability, module ease of deployment including size and modularity, and reproducibility), and 3) ecology (degree of morphological realism, chemosensory stimulation, and environmental impact; Table 1).
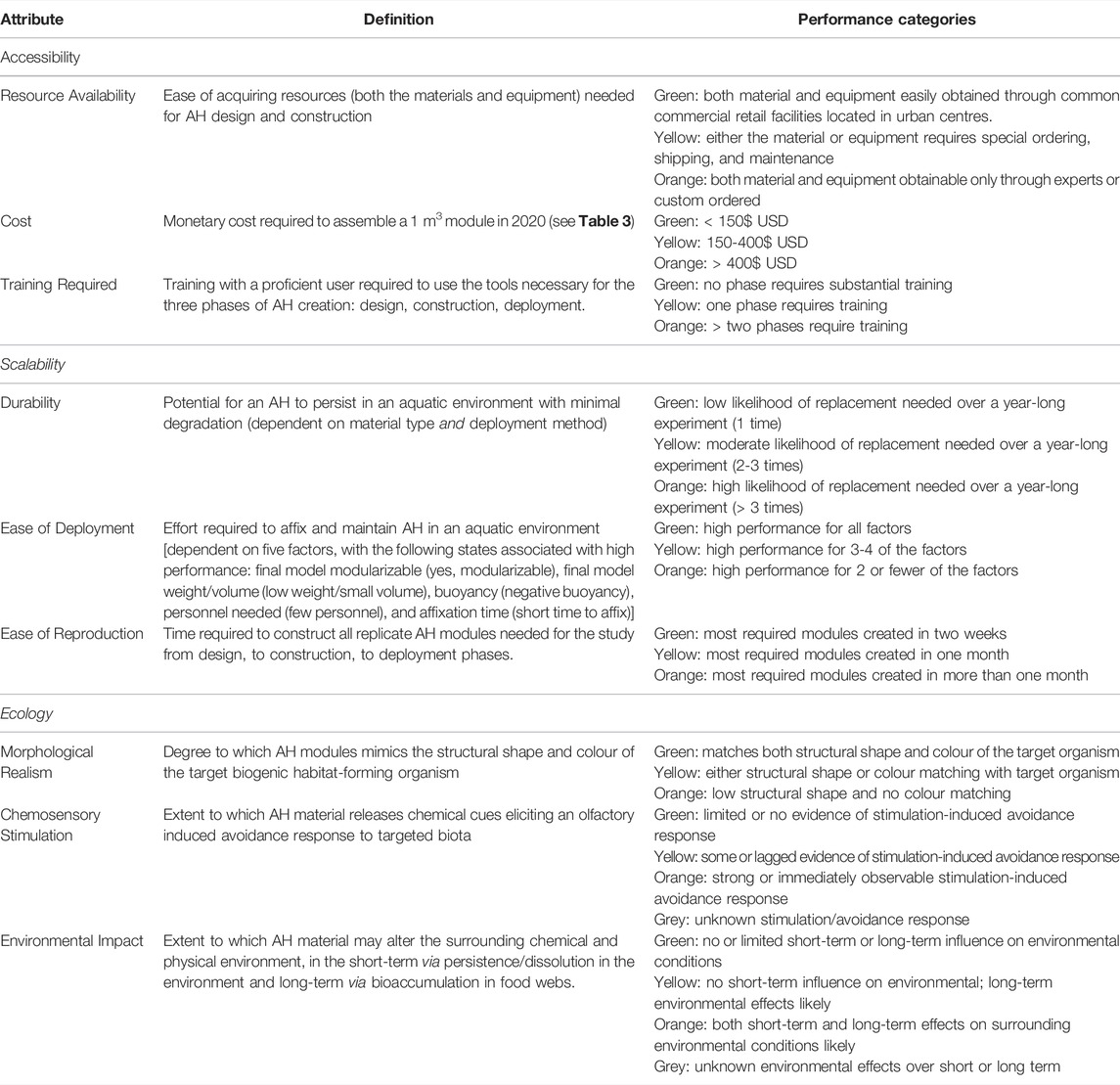
TABLE 1. Descriptions for key attributes affecting the performance of Artificial Habitat (AH) module designs for aquatic research, and associated criteria for high (green), moderate (yellow), low (orange), and unknown response (grey) performance for components of each attribute.
The method presented here stems from experimentation to identify an approach for AH design that would allow us to flexibly manipulate morphological and compositional features hypothesized to affect habitat selection in aquatic ecosystems. Considering existing AH designs and commonly used materials used in aquatic research relative to the attributes described above highlighted key gaps in existing knowledge and opportunities for innovation (Figure 2; Table 2). In particular, some existing AH designs require specialized or expensive materials/equipment, or produce large modules that are challenging to scale ‘up’ or ‘down’ to meet the needs of the research. Crucially, many AH designs fall short in their ability to reproduce morphological realism (e.g., structural configuration, surface texture, and colouration; Figure 2), and perform poorly regarding unintended chemosensory stimulation and environmental impact (Table 2). For example, in Figure 2D, this structure does not well mimic the seagrass Posidonia oceanica (Charbonnel et al., 2011) nor do the stacked ceramic tiles mimic coastal habitat (Brotto and Araujo, 2001).
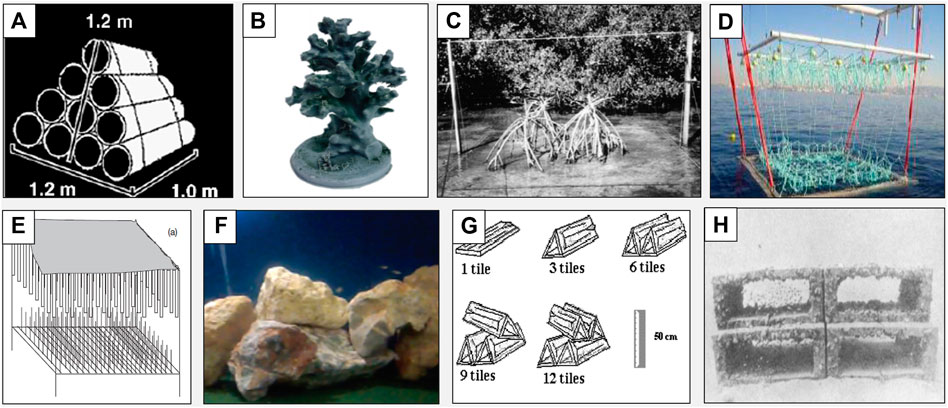
FIGURE 2. A wide variety of materials and configurations have been used to create artificial habitat modules in aquatic environments. Here we illustrate examples of materials and their common designs presented in Table 2. (A) A plastic PVC pyramid simulating freshwater reservoir habitat (Santos et al., 2011), (B) 3D printed plastic module (Plastic polylactic acid filament) simulating coral species (Ruhl and Dixson, 2019) (C) Mangrove prop root bundles simulating complex mangrove habitat (Ellis and Bell, 2004), (D) Line “floating rope” structures simulating restored Posidonia oceanica (Neptune grass) beds (Charbonnel et al., 2011) (E) Plastic PVC pipe and metal iron rods module simulating mangrove roots and seagrass leaves, respectively (Verweij et al., 2006) (F) Rocks/rubble simulating coastal nursery habitat (Mercader et al., 2019) (G) Ceramic tiles simulating coastal habitat (Brotto and Araujo, 2001) (H) Concrete blocks with varying hole sizes simulating coral reefs (Talbot et al., 1978)
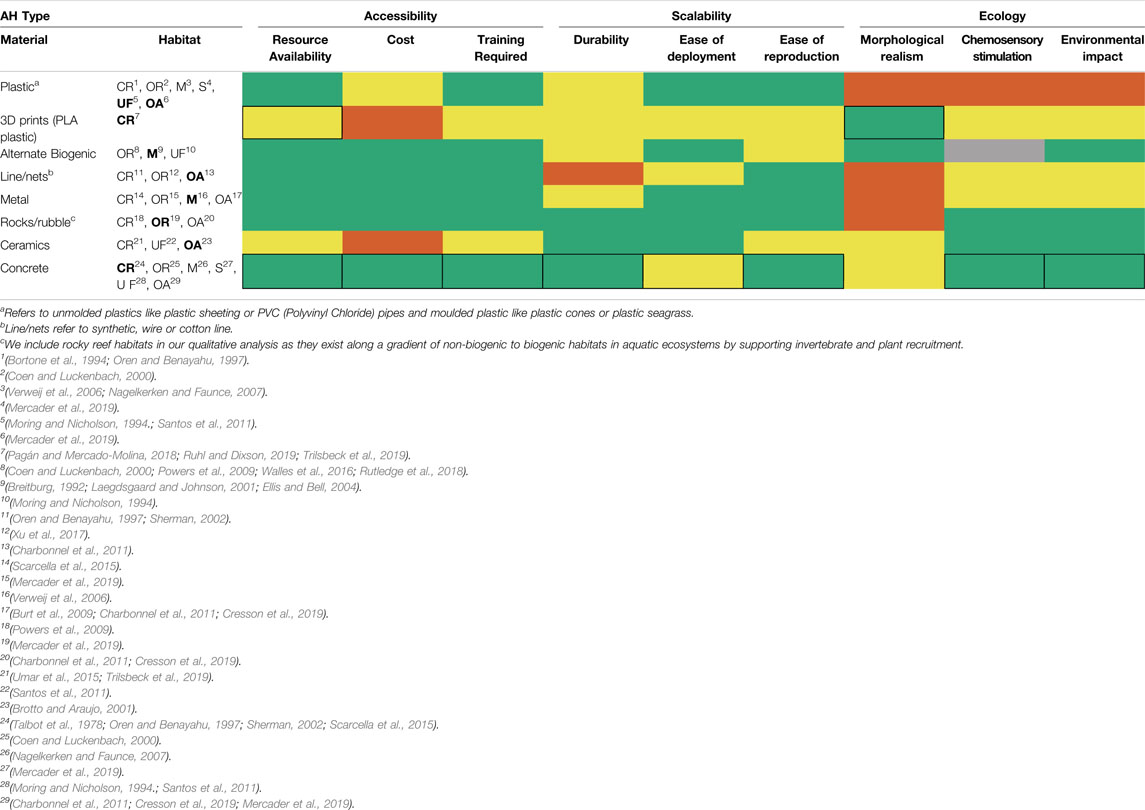
TABLE 2. Performance of common Artificial Habitat (AH) types used in aquatic research, given by their construction materials and the habitats they are designed to mimic, relative to three groups of metrics (accessibility, scalability, and ecology). Green = high performance, yellow = moderate orange = poor, and; grey = unknown. Detailed descriptions of each metric and performance criteria are provided in Table 1. CR = Coral Reef, OR = Oyster Reef, M = Mangrove, S = Seagrass Bed, UF = Unspecified Freshwater habitat, OA = other aquatic habitats. Bold text = example studies highlighted in Figure 2. Thick black borders indicate characteristics of existing methods integrated into 3D-SPMC.
Morphologically realistic habitat structures may be an important feature to habitat selection processes, which in turn could support downstream ecosystem functioning provided by diverse species assemblages using structurally complex habitats. Furthermore, plastic-based materials have the potential to leach chemicals into the surrounding environment, eliciting a range of chemosensory stimulation responses in surrounding organisms (McCormick et al., 2020), and the physical breakdown of other traditional AH materials (including plastics, thin metal sheets, and line) can lead to the contamination of food webs with micro-debris (Fotopoulou and Karapanagioti, 2019). Alternate biogenic materials (e.g., wood or shell) to construct biodegradable AHs may reduce environmental impacts, but confounding chemical cues associated with these materials and the unknown long-term effects of “degrading” habitat on resident biota make them a poor choice for habitat selection studies (Arvedlund and Kavanagh, 2009; Dixson et al., 2014).
Here we describe 3D-SPMC (three-dimensional Scan, Print, Mould, and Cast), an integrative method for AH design that allows users to isolate and flexibly manipulate compositional and structural elements of biogenic habitats to address research questions regarding habitat selection cues. The method was developed to address the opportunities for innovation outlined above and was also motivated by the lack of pragmatic guidance in published AH research for users seeking to isolate and manipulate structural and compositional features of focal biogenic habitat-forming organisms. After describing the 3D-SPMC method, we 1) briefly describe its implementation in a field study of habitat selection cues for habitat-forming coral, 2) outline its performance (in terms of accessibility, scalability, and ecology) compared to other approaches to AH design, and 3) discuss applications of the method to studying habitat selection cues and informing the design of biogenic habitat restoration projects.
Materials and Equipment
Equipment Required:
• 3D scanner (Step 1)
• 3D printer (Step 2)
• Computer (Steps 1-2)
Materials Required:
• sample of biogenic habitat to be replicated
• 3D printing filament (Step 2)
• Plexiglass/hard plastic sheet (Step 3)
• Flexible mould material (Step 3)
• Mould thickener (optional; Step 3)
• Mould release (or light vegetable oil; Step 3)
• Round-tipped paint brush (Step 3)
• Plaster of Paris (Step 4)
• Paper towel (Step 4)
• Concrete (Step 4)
• Aquatic epoxy (Step 5 and module deployment)
Methods
3D-SPMC contains five major steps that draw on techniques from engineering (steps 1 and 2), and paleontology and visual art (steps 3-5; Cheah et al., 2005). 3D-scanning and printing are emerging engineering technologies with diverse applications, and moulding, casting and 3D assembly are techniques used in paleontology and visual art to replicate designs, conserve the integrity of the original object and create complex structures.
Here we use staghorn coral (Acropora cervicornis), a dominant coral species on Caribbean reefs, as a model habitat-forming organism. Corals are the focus of habitat restoration efforts following decades of decline (Young et al., 2012). Understanding compositional and structural features of corals that attract and retain organisms from the water column to the habitat -a colonization process known as ‘recruitment’ or ‘settlement’ (Booth and Beretta, 1994; Nagelkerken et al., 2015) - can inform the design of restoration projects aiming to restore lost ecological function.
Step-By-Step Procedure
1) 3D Scanning and Virtual Augmentation: The technique requires that a sample of the biogenic habitat-forming organism being approximated is accessible through field or archival sampling. We obtained a staghorn coral skeleton fragment from the Coral Restoration Foundation (CRF) in Key Largo FL, United States. We intentionally selected a fragment where majority of the main structural features were in one plane (Figure 3A); although the 3D file can be manipulated to add/remove features (e.g., branches from the coral), this planar form reduced the need for 3D file manipulation. We scanned the fragment using a 2020i Next Engine Desktop 3D Scanner (NextEngine Inc., Santa Monica, United States) to create a 3D mesh file and manipulated the file using 3D Builder (Microsoft© Application, 2013) to remove all irregularities, ensure high-resolution quality, and create a “water-tight” mesh (remove any “holes” in the file created during scanning). Users that require large and complex AH to address their research question can modularize the process in the 3D file manipulation stage by breaking the design down into smaller separate objects that are assembled into a final product. The design can also be adapted to change proportions of individual components, adjust size ratio, and/or create attachment features. We formatted the resulting 3D-file to create an object with a thin, flat plane running laterally along the edge of the module, which provided a smooth surface for attachment during the mould making process (see Step 3; Figure 3B).
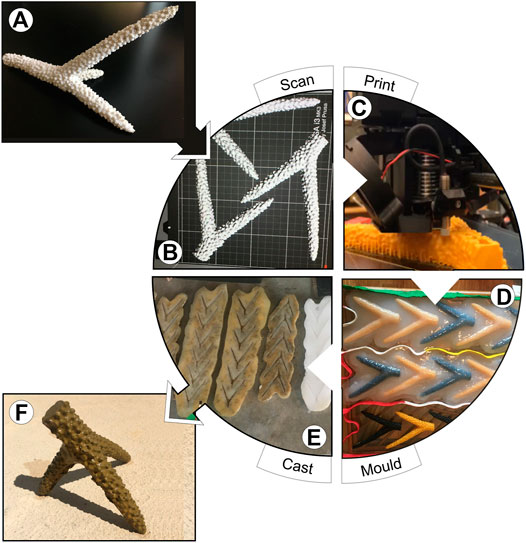
FIGURE 3. (A) Fragment of the focal biogenic habitat-forming organism is selected for 3D-scanning; here a coral skeleton fragment of Acropora cervicornis (staghorn coral) with a flat, Y-shape branch. (B) 3D file created by scanning the fragment from Figure 3A, which can be manipulated further to alter/agument structural features and complexity. (C) Multiple copies of the 3D file are printed using PLA filament and extrusion printers. (D) Consecutive layers of silicone mould material are poured over duplicated 3D prints; plaster casings (not shown) are made for each silicone mould to enhance the mould’s structural stability (E) The resulting silicone moulds are flipped over so the resulting cavity is facing upwards, lined with vegetable oil to act as a mould release and then filled with desired casting material. In this figure, pigmented concrete has been stuffed into the four silicone molds on the left side of the image (where the moulds appear dark grey) and the two silicone moulds on the right side of the image are empty and have not yet been filled with concrete (where the moulds appear white). (F) Concrete casts are assembled into final, more complex artificial habitat modules; here a tri-pod shaped artificial coral for deployment in clusters in a field experiment (see Figure 5).
2) 3D Printing: We printed the resulting 3D-file using two types of extrusion-based printers, the Dremel Digilab 3D45 (Dremel DigiLab, Mt Prospect, United States) and the PRUSA MK 2 and 3 (Prusa Research, Prague, Czech Republic) printers. Both extrude PLA filament (polylactic acid, a common 3D printing filament) by building up consecutive layers of the 3D object, analogous to a hot glue gun extruding liquid plastic that hardens into a firm object. Both of these printers use 1.75 mm PLA filament, with a melting temperature of 175°C, and plate temperature of 60°C. Orienting the object so that the flat plane faced down onto the 3D printer’s build-plate reduced the support material required to hold the structure in place, minimizing print-time (Figure 3C). Print time, or print speed, ranged between 3-5 h per module, depending on the printer and the number of modules loaded onto the build plate (i.e., total print time of 10–12 h if three modules were printed at once). Print set-up was an iterative process involving continual monitoring and adjustment for the first few print layers to ensure an established print base. Design flaws created during scanning and file manipulation (step 1) may only become apparent during the printing process (See Supplementary Information 1.0).
3) Mould Making: To create moulds from which to cast the artificial habitat modules, we first attached the 3D printed corals to a Plexiglass sheet using modeling clay. After coating the entire surface (plexiglass and coral modules) with spray-on Universal Mold Release (Smooth-On Inc., Macungie, United States), we brushed on the first layer of Dragon Skin®10 Medium Series silicone (Smooth-On Inc., Macungie, United States) onto the 3D printed modules using a 1 cm round-tipped paint brush. This first silicone layer was mixed with a few drops of THI-VEX® silicone thickener (Smooth-On Inc., Macungie, United States) to help thicken silicone, ensure adhesion to 3D printed modules, and capture fine details of the organisms’ morphology in the mould (in our case, polyp-level features of the coral; Figure 3D). We poured the next three consecutive layers of silicone onto each mould according to the product’s mixing and pouring directions. The entire mould sat untouched in a cool dry area to set for 24 h. Next, we created a Plaster of Paris (Bondex International, Medina County, United States) casing over top by adding layers of heavy duty paper towel coated in a plaster slurry to give the flexible silicone under-mould structural stability (also called a mother mould/bandage shell mould) The entire mould and plaster casing sat untouched in a cool dry area for an additional 24 h -after which the 3D prints were carefully removed from the silicone layer, resulting in a mould with negative space where the 3D printed corals sat, and a firm support layer.
4) Casting: We used Quikrete® countertop mix (The Quikrete Companies, Atlanta, United States) concrete, water, and yellow pigment (Concrete Concrete, Edmonton, Canada) mixed by hand at a ratio of approximately 50:5:1 to approximate the colour of A. cervicornis. After lightly coating the moulds with vegetable oil (to act as a mould release) using a 1 cm round-tip paint brush, we filled and compressed each mould with the concrete mixture (Figure 3E). Since the silicone mold covered almost the entire 3D-printed coral fragment, the concrete filling is not visualized at the bottom of the mold as most of it is in the empty cavity created when the 3D print was removed. After a 24 h setting period, we carefully removed casts from the moulds and left them to cure further in a flat, dry area for 24 hrs minimum. These flexible and strong silicone moulds can be re-used multiple times for casting.
5) Assembly and Deployment: In our example study region, coral restoration projects typically transplant clusters of ‘tripod’ shaped A. cervicornis to reef environments to enhance coral cover because this shape provides multiple points of attachment to the benthos, increases stability, and exposes the coral to adequate water flow (Hollarsmith et al., 2012). We combined the concrete casts into complex 3D structures that mirrored this tripod shape and size (Figure 3F). Assembly involved carefully breaking some of the coral casts into two pieces to create coral “branches”, which were attached to original casts using Apoxie Sculpt Modeling Compound (Aves, Hudson, United States) and left to set for a minimum of 24 hrs (See Supplementary Information 4.1 for a video of this process). Modules were soaked in sea-water for a minimum of 7 days to leach out concrete-associated chemosensory cues before deployment, consistent with practices in other habitat selection studies using artificial habitat structures (Ruhl and Dixson, 2019). Modules were transported in large totes to the field site by boat and transferred underwater to experimental plots in milk crates by scuba divers (See Supplementary Information 4.2 for a video showing deployment process).
Validation Case Study: Application of 3D-SPMC Habitat Modules to Study Fish Recruitment Cues
We applied artificial corals created via 3D-SPMC in a field experiment to evaluate structural and compositional cues driving juvenile reef fish habitat selection in Key Largo, United States from June-July 2019. Living corals were obtained by collaborators at the Coral Restoration Foundation from their Carysfort Reef offshore coral nursery and were cut to the same dimensions as the artificial coral fragments (12 cm3).The experiment involved placing artificial and living coral modules in replicate 1 m2 clusters at consistent densities (10 corals/cluster) in three treatments representing different percentages of living coral (but equal structural composition) and in two environmental contexts: high complexity seascape (mean relief of the reef framework ≈ 3 m) and low complexity seascape (mean relief of the reef framework ≈ 0.5 m; Ntotal = 48 clusters). Divers attached habitat clusters to the bottom with the same epoxy used for module assembly (see step 5) at sites with no previous living staghorn corals present (Figure 4). Artificial habitat modules were equally easy to deploy compared with living coral fragments and required little additional diver training to affix to the benthos. The modules withstood transportation and under-water handling without damage and remained in place for the entire duration of the 2-month study without maintenance or repair.
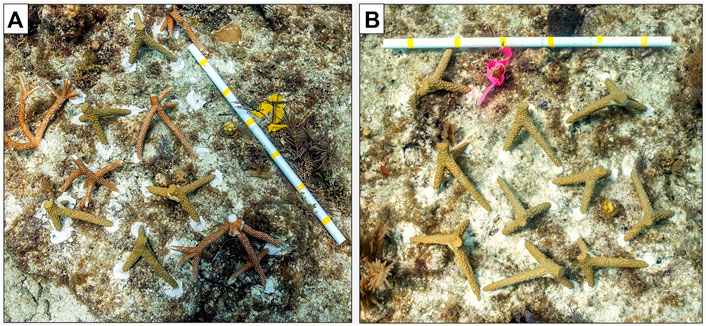
FIGURE 4. (A) Example coral reef habitat patch comprised of 5 artificial coral habitat modules created via 3D-SPMC, and 5 living corals (i.e., 50% living coral in the patch = 50% living coral treatment) deployed in a replicated in situ experiment in FL, United States to test the effect of live coral content (i.e., %) within reef habitat patches, while controlling for structural habitat complexity, on reef fish recruitment in areas of high or low seascape structural complexity. (B) Example coral reef habitat patch comprised of 10 artificial coral habitat modules created via 3D-SPMC, and 0 living corals (i.e., 0% living coral in the patch = 0% living coral treatment).
Validation Case Study: Results
Following deployment, we conducted SCUBA surveys of the abundance of newly recruited fishes (i.e., < 3 cm total length) at each cluster every 1–4 days over 50 days. Preliminary data visualization from our study suggests when structural features (i.e., habitat size and morphology) are held constant, recruiting fish have differing recruitment patterns over the first 50 days after habitat deployment (Figure 5A) and show a strong associations with habitats comprising 0 and 100% proportions of living coral, compared to disassociation with habitats comprising 50% living coral, with higher association to habitats in low complexity environments regardless of habtiat composition treatments. (i.e., low structural complexity, Figure 5B). These initial results suggest an interaction between habitat composition and environmental context, with implications for restoration site selection: increasing coral cover at low complexity sites may yield benefits that are not realized at high complexity locations in the same reefscape and further research may elucidate patterns of use for different species, trophic groups or trait groups during the first 50 days post-outplanting.
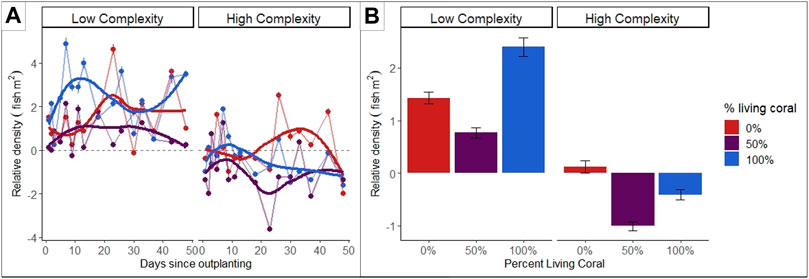
FIGURE 5. (A) Mean fish recruit (⋜ 3 cm) density for each substrate composition treatment (mean ± S.E) with local polynomial regression overlay over the length of the experiment since corals were added to the benthos via “outplanting” (Days 1–50). (B) Mean fish recruit density (± S.E.) of fish recruits (⋜ 3 cm) associated with the habitat patches in three treatments of % living coral (i.e., ratio of artificial coral to live coral modules, ntreatment = 12): 0% = 0 living coral/10 artificial coral, 50% = 5 living coral/5 artificial coral, l, 100% = 10 living coral/0 artificial coral, control = 0 living coral/0 artificial coral. Density was standardized to ambient recruit density measured at control patches within Low and High complexity sites, respectively.
Relative Performance of 3D-SPMC
By combining desirable attributes from multiple methods for artificial habitat design and construction into a streamlined workflow, 3D-SPMC is likely to perform on par with or better than eight other individual AH materials and designs used in habitat selection studies (Tables 2, 3). Accessibility: Most of the materials and equipment required in steps 1 and 2 of 3D-SPMC are easily obtained through retail in urban centres; however the 3D printing components require special maintenance (Table 2). While the baseline costs associated with steps 1 and 2 (3D scanning and printing) of 3D-SPMC are high compared with other approaches (Table 3), the cost per AH module decreases substantially with increased production. For example, incorporating printer purchase and printing costs, ten 10 cm3 modules cost $19.37 each, compared to $0.43 each for 500 modules. Once made, 3D files and prints can be repeatedly edited, re-used, and/or shared, facilitating iterative designs and projects with little financial or time investment. A growing number of online tutorials and training centres offer free training in 3D scanning and printing, making the technology more broadly accessible (See Supplementary Information 1.2). While more costly than other moulding materials, Dragon Skin Series silicone material (step 3) is strong and elastic, meaning it can be used multiple times to create hundreds of replicates (Figure 3D).
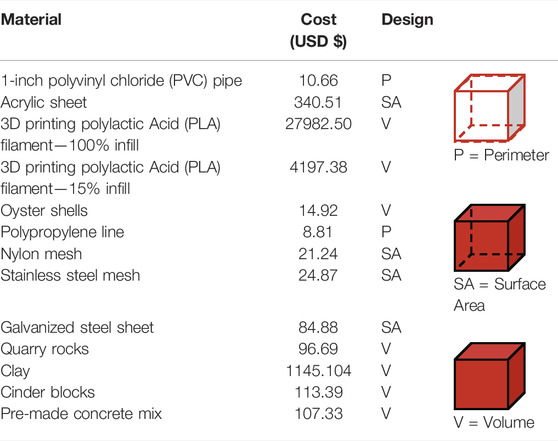
TABLE 3. Cost associated with creating a 1 m3 structure using the 3D-SPMC method and eight other materials commonly used for AH design (cost estimates based on average materials costs in the year 2020). The amount of material required for each design depends on the material type and its method of use, as indicated by red shading in the diagram below (P = Perimeter, SA = Surface Area, V = Volume).
Scalability: Scaling AH production to the research question and study design at hand requires modules that are durable, easy to deploy, and easy to reproduce (Bortone, 2006). We chose concrete for our casting material as it is durable in aquatic environments, including salt water environments, reducing the risk of collapse and potential for short- and long-term changes to the surrounding environment (Table 2). Other applications of concrete in AH construction result in modules that are large and non-modular, often requiring specialized equipment to deploy (e.g., reef balls; Sherman, 2002), or are created with inflexible moulds that are destroyed during the cast-release process, thus not re-useable (Table 2). Modularizing the AH structure into simple planar components (step 1) that can later be assembled into replicate, complex 3-dimensional configurations (step 5) makes the 3D-SPMC method more scalable -a major benefit compared to 3D printing complex modules (Supplementary Figure S1).
Ecology: While existing designs using concrete only score moderately well in terms of structural realism (Figure 2, Table 2), the application of 3D scanning and printing (steps 1 and 2) within 3D-SPMC facilitates the creation of biogenic habitat replicas with a high degree of morphological detail. Once cast, concrete is chemically neutral, and thus less likely to cause unanticipated chemosensory stimulation of target and non-target organisms (and less environmental harm). While some previous 3D printing methods to create artificial habitat modules have used biodegradable PLA filament (Tarazi et al., 2019; Wolfe and Mumby, 2020), 3D-SPMC avoids this potential source of aquatic plastic pollution, and mitigates against unintentional chemical cues leaching into the environment.
Discussion
Application to Aquatic Habitat Restoration Research and Design
Restoration planning has only recently started to include metrics of ecosystem function (Suding and Hobbs, 2009), and/or focus on key species that may accomplish broader co-beneficial goals of restoration (Peterson et al., 2003; Jones and Davidson, 2016; Ladd et al., 2018). Information on key structural and compositional attributes of biogenic habitats that promote habitat selection and use by resident species is essential for conservation and restoration planning; manipulative field and laboratory experiments using this method to create artificial habitats (such as in the experiment described above) can provide insights into the composition and optimal placement of biogenic habitats to bolster ecosystem function and sustainability (Ferrario et al., 2016). As seen in our case study, habitat composition affects fish recruitment, but environmental context also mediates the direction and magnitude of ecological response. Crucially, we only detected this effect by using 3D-SPMC to create modules for an experimental design in which we replicated a high degree of morphological realism across habitat modules varying in material composition (live coral vs concrete). In some aquatic ecosystems, employing artificial structures in restoration itself has resulted in increased fish recruitment (Harding and Mann, 2001; Green et al., 2015) however they are no substitute for the suite of ecosystem services created by living biogenic habitats (e.g., Côté and Darling, 2010; Bruno et al., 2019; Supplementary Information 3.0).
Application to Aquatic Habitat Selection Research
A persistent challenge for testing habitat selection cues has been designing habitat modules that enable researchers to isolate and manipulate structural and compositional attributes, thus disentangling their relative influence on the habitat selection process (Harborne et al., 2011; Coker et al., 2012). 3D-SPMC offers a flexible means to design AH modules that manipulate structure and composition of focal biogenic habitat-forming organisms, and could be employed to provide insights into factors affecting habitat selection by resident biota in environments ranging from coastal oyster reefs and mangroves (Ellis and Bell, 2004; Beck et al., 2011), to woody vegetation in freshwater bodies, to mesophotic glass sponge reefs (Dunham et al., 2018).
Scale is an important consideration when designing AHs for selection cue studies; the spatial and temporal scale of the ecological process being examined and the type(s) of cues to be manipulated influences decisions around study type (i.e., in situ vs. ex situ) and duration, focal habitat size and configuration, ecosystem connectivity, placement and response variable selection (see Supplementary Information 5.0 for a list of key research design questions the researcher should address in selecting appropriate AH configuration). We used coral fragment-sized modules in dense clusters at a spatial scale which fish recruitment is likely to vary, that mimics the design of reef restoration projects, and because recent evidence suggests fine-scale morphology affects larger-scale ecological processes (Urbina-Barreto et al., 2020). We also chose to measure colonization processes (recruitment and retention) that occur at the temporal scale most artificial habitat selection studies typically investigate, however metrics could be expanded to include longer temporal-scale processes like reproduction which could affect the overall abundance of organisms available to be recruited and retained to habitats over longer periods of time. However, 3D-SPMC could be used to create larger and/or more complex habitat patches by generating larger 3D prints (and mould/casts), increasing habitat complexity in the assembly phase by combining multiple casts into a single module, and/or combining multiple habitat modules into a final structure. While the effect of patch size on species colonization and habitat use has been relatively well studied compared to habitat composition (Bohnsack et al., 1994) our method allows researchers to incorporate both aspects into their study design to evaluate habitat composition characteristics.
Examples of Other Applications
By manipulating the composition of casting material (step 4), researchers can use 3D-SPMC to study compositional features hypothesized to affect chemosensory stimulation. For example, studies aiming to study predator, prey, and competitor detection by focal organisms could directly incorporate homogenized tissue, body fluids, or key chemical components (e.g., pheromones, hormones) of con- and hetero-specifics into the casting material. One could also test multiple recruitment cue responses (Huijbers et al., 2012) by deploying AHs in combination with other cues (i.e., acoustic cues).
3D-SPMC could also be used to study epi-biotic habitat colonization by invertebrates like corals, sponge, or oyster spats by altering the configuration and/or casting composition of the AH modules. For example, oyster shells incorporated into structure provided attractant cues to larval oyster and saw higher spat recruitment (Ortego, 2006). Ceramic modules with tighter surface-pore densities may reduce biofouling and/or enhance targeted species-specific settlement (Johari et al., 2010). Companies are already creating “ecologically active” concrete materials that modify composition and surface texture to support specific marine fauna and flora (Perkol-Finkel and Sella, 2014), lowers the carbon footprint of artificial habitat construction (Dennis et al., 2018), and addresses the concern of concrete waste in aquatic ecosytems (Cooke et al., 2020). One could even consider expanding and adapting this method to test biofilm or anti-biofouling coatings that reduce or promote targeted biotic build-up (Tamburri et al., 2008).
This method can also be adapted to evaluate the structural characteristics of soft-bodied biogenic habitat-forming organisms such as sea-fans and seagrasses by using flexible casting material or 3D printing using flexible or biogenic printing material (Yirmibesoglu et al., 2018; Wangpraseurt et al., 2020), with implications to bio-mechanic studies and the contribution of biogenic organisms to shoreline protection (Christianen et al., 2013). Note that specialized printers may be a more expensive option that may limit affordability and accessibility.
Conclusion
Evidence across ecosystems suggest both composition and structural complexity contribute to biogenic habitat quality, impacting the ecological understanding and conservation implications for multiple secondary species (Harborne et al., 2011; Gardiner et al., 2018). Ultimately, more studies in controlled and natural settings are needed to draw conclusions about habitat selection ecology and potential restoration implications; 3D-SPMC is an integrative method which meets this need, is adaptable for use in numerous aquatic ecosystems, and provides a method that is accessible, scalable and considers ecological implications.
Data Availability Statement
The datasets presented in this study can be found in online repositories. The names of the repository/repositories and accession number(s) can be found below: https://github.com/CHANGE-Lab/3D-SPMC_Realistic_Artificial_Habitat_Design.
Ethics Statement
Ethical review and approval was granted by the University of Alberta under Animal Use Protocol #00003176.
Author Contributions
AG and SG conceived the ideas and methodology, AG collected the data, AG and SG led the writing of the manuscript. All authors contributed critically to the drafts and gave final approval for publication.
Funding
This work was funded by the Natural Sciences and Engineering Research Council (NSERC Discovery Grant DGECR-2018-00156; Canada Graduate Scholarship–Master’s Program) and the Alfred P. Sloan Foundation.
Conflict of Interest
The authors declare that the research was conducted in the absence of any commercial or financial relationships that could be construed as a potential conflict of interest.
Publisher’s Note
All claims expressed in this article are solely those of the authors and do not necessarily represent those of their affiliated organizations, or those of the publisher, the editors and the reviewers. Any product that may be evaluated in this article, or claim that may be made by its manufacturer, is not guaranteed or endorsed by the publisher.
Acknowledgments
We thank the Elko Engineering Garage (Erin Whitby and Sun Woo Yu), Marine Paleoecology Lab (Rylan Dievert and Lindsey Leighton), Vertebrate Paleontology Lab (Christina Hatley, Allan Lindoe, Michael Caldwell), Department of Art and Design (Stephanie Jonsson) and Shack Science Hardware Makerspace (Logan Fairgrieve-Park) at the University of Alberta for all their advice and support during the method development process. Additional thanks to the Coral Restoration Foundation (Amelia Moura), Florida Keys National Marine Sanctuary (permit number FKNMS-2019-033), and Aquatic Global Change Ecology and Conservation Lab for their support in method and case study development. Special thanks to Noelle Helder, Kelsey Dougan, and Taylor Restall for research assistance.
Supplementary Material
The Supplementary Material for this article can be found online at: https://www.frontiersin.org/articles/10.3389/fbuil.2022.763315/full#supplementary-material
References
Agudo-Adriani, E. A., Cappelletto, J., Cavada-Blanco, F., and Croquer, A. (2016). Colony Geometry and Structural Complexity of the Endangered speciesAcropora Cervicornispartly Explains the Structure of Their Associated Fish Assemblage. PeerJ 4, e1861. doi:10.7717/peerj.1861
Arvedlund, M., and Kavanagh, K. (2009). “The Senses and Environmental Cues Used by Marine Larvae of Fish and Decapod Crustaceans to Find Tropical Coastal Ecosystems,” in Ecological Connectivity Among Tropical Coastal Ecosystems. Editor I Nagelkerken (Netherlands: Springer), 135–184. doi:10.1007/978-90-481-2406-0_5
Beck, M. W., Brumbaugh, R. D., Airoldi, L., Carranza, A., Coen, L. D., Crawford, C., et al. (2011). Oyster Reefs at Risk and Recommendations for Conservation, Restoration, and Management. BioScience 61 (2), 107–116. doi:10.1525/bio.2011.61.2.5
Bohnsack, J. A., Harper, D. E., McClellan, D. B., and Hulsbeck, M. (1994). Effects of Reef Size on Colonization and Assemblage Structure of Fishes at Artificial Reefs off Southeastern Florida, U.S.A. Bull. Mar. Sci. 55 (2–3), 796–823.
Booth, D. J., and Beretta, G. A. (1994). Seasonal Recruitment, Habitat Associations and Survival of Pomacentrid Reef Fish in the US Virgin Islands. Coral Reefs 13 (2), 81–89. doi:10.1007/BF00300765
Bortone, S. A. (2006). A Perspective of Artificial Reef Research: The Past, Present, and Future. Bull. Mar. Sci. 78 (1), 9.
Bortone, S. A., Van Tassell, J., Brito, A., Falcón, J. M., Mena, J., and Bundrick, C. M. (1994, September). Enhancement of the Nearshore Fish Assemblage in the Canary Islands with Artificial Habitats [Text]. Available at: https://www-ingentaconnect-com.login.ezproxy.library.ualberta.ca/content/umrsmas/bullmar/1994/00000055/f0020002/art00028
Breitburg, D. L. (1992). Episodic Hypoxia in Chesapeake Bay: Interacting Effects of Recruitment, Behavior, and Physical Disturbance. Ecol. Monogr. 62 (4), 525–546. JSTOR. doi:10.2307/2937315
Brotto, D. S., and Araujo, F. G. (2001). Habitat Selection by Fish in an Artificial Reef in Ilha Grande Bay, Brazil. Braz. Arch. Biol. Technol. 44 (3), 319–324. doi:10.1590/S1516-89132001000300015
Bruno, J. F., Côté, I. M., and Toth, L. T. (2019). Climate Change, Coral Loss, and the Curious Case of the Parrotfish Paradigm: Why Don't Marine Protected Areas Improve Reef Resilience? Annu. Rev. Mar. Sci. 11 (1), 307–334. doi:10.1146/annurev-marine-010318-095300
Burt, J., Bartholomew, A., Bauman, A., Saif, A., and Sale, P. F. (2009). Coral Recruitment and Early Benthic Community Development on Several Materials Used in the Construction of Artificial Reefs and Breakwaters. J. Exp. Mar. Biol. Ecol. 373 (1), 72–78. doi:10.1016/j.jembe.2009.03.009
Charbonnel, E., Harmelin, J.-G., Carnus, F., Direac'h, L. L., Ruitton, S., Lenfant, P., et al. (2011). Artificial Reefs in Marseille (France, Mediterranean Sea): From Complex Natural Habitats to Concept of Efficient Artificial Reef Design. Braz. J. Oceanogr. 59, 177–178. doi:10.1590/S1679-87592011000300019
Cheah, C. M., Chua, C. K., Lee, C. W., Feng, C., and Totong, K. (2005). Rapid Prototyping and Tooling Techniques: A Review of Applications for Rapid Investment Casting. Int. J. Adv. Manuf Technol. 25 (3), 308–320. doi:10.1007/s00170-003-1840-6
Cheminée, A., Merigot, B., Vanderklift, M. A., and Francour, P. (2016). Does Habitat Complexity Influence Fish Recruitment? Medit. Mar. Sci. 17 (1), 39–46. Scopus. doi:10.12681/mms.1231
Christianen, M. J. A., van Belzen, J., Herman, P. M. J., van Katwijk, M. M., Lamers, L. P. M., van Leent, P. J. M., et al. (2013). Low-Canopy Seagrass Beds Still Provide Important Coastal Protection Services. PLoS One 8 (5), e62413. doi:10.1371/journal.pone.0062413
Coen, L. D., and Luckenbach, M. W. (2000). Developing success Criteria and Goals for Evaluating Oyster Reef Restoration: Ecological Function or Resource Exploitation? Ecol. Eng. 15 (3), 323–343. doi:10.1016/S0925-8574(00)00084-7
Coker, D. J., Graham, N. A. J., and Pratchett, M. S. (2012). Interactive Effects of Live Coral and Structural Complexity on the Recruitment of Reef Fishes. Coral Reefs 31 (4), 919–927. doi:10.1007/s00338-012-0920-1
Cooke, S. J., Bergman, J. N., Nyboer, E. A., Reid, A. J., Gallagher, A. J., Hammerschlag, N., et al. (2020). Overcoming the concrete Conquest of Aquatic Ecosystems. Biol. Conservation 247, 108589. doi:10.1016/j.biocon.2020.108589
Côté, I. M., and Darling, E. S. (2010). Rethinking Ecosystem Resilience in the Face of Climate Change. Plos Biol. 8 (7), e1000438. doi:10.1371/journal.pbio.1000438
Cresson, P., Le Direach, L., Rouanet, E., Goberville, E., Astruch, P., Ourgaud, M., et al. (2019). Functional Traits Unravel Temporal Changes in Fish Biomass Production on Artificial Reefs. Mar. Environ. Res. 145, 137–146. doi:10.1016/j.marenvres.2019.02.018
Dennis, H. D., Evans, A. J., Banner, A. J., and Moore, P. J. (2018). Reefcrete: Reducing the Environmental Footprint of Concretes for Eco-Engineering marine Structures. Ecol. Eng. 120, 668–678. doi:10.1016/j.ecoleng.2017.05.031
Dixson, D. L., Abrego, D., and Hay, M. E. (2014). Chemically Mediated Behavior of Recruiting Corals and Fishes: A Tipping point that May Limit Reef Recovery. Science 345 (6199), 892–897. doi:10.1126/science.1255057
Dunham, A., Archer, S. K., Davies, S. C., Burke, L. A., Mossman, J., Pegg, J. R., et al. (2018). Assessing Condition and Ecological Role of Deep-Water Biogenic Habitats: Glass Sponge Reefs in the Salish Sea. Mar. Environ. Res. 141, 88–99. doi:10.1016/j.marenvres.2018.08.002
Ellis, W. L., and Bell, S. S. (2004). Conditional Use of Mangrove Habitats by Fishes: Depth as a Cue to Avoid Predators. Estuaries 27 (6), 966–976. doi:10.1007/bf02803423
Ferrario, F., Iveša, L., Jaklin, A., Perkol-Finkel, S., and Airoldi, L. (2016). The Overlooked Role of Biotic Factors in Controlling the Ecological Performance of Artificial marine Habitats. J. Appl. Ecol. 53 (1), 16–24. doi:10.1111/1365-2664.12533
Fotopoulou, K. N., and Karapanagioti, H. K. (2017). “Degradation of Various Plastics in the Environment,” in Hazardous Chemicals Associated with Plastics in the Marine Environment. Editors H. Takada, and H. K. Karapanagioti (Cham: Springer International Publishing), 71–92. doi:10.1007/698_2017_11
Gardiner, R., Bain, G., Hamer, R., Jones, M. E., and Johnson, C. N. (2018). Habitat Amount and Quality, Not Patch Size, Determine Persistence of a woodland-dependent Mammal in an Agricultural Landscape. Landscape Ecol. 33 (11), 1837–1849. doi:10.1007/s10980-018-0722-0
Green, A. L., Maypa, A. P., Almany, G. R., Rhodes, K. L., Weeks, R., Abesamis, R. A., et al. (2015). Larval Dispersal and Movement Patterns of Coral Reef Fishes, and Implications for marine reserve Network Design. Biol. Rev. 90 (4), 1215–1247. doi:10.1111/brv.12155
Harborne, A., Mumby, P., Kennedy, E., and Ferrari, R. (2011). Biotic and Multi-Scale Abiotic Controls of Habitat Quality: Their Effect on Coral-Reef Fishes. Mar. Ecol. Prog. Ser. 437, 201–214. doi:10.3354/meps09280
Harding, J. M., and Mann, R. (2001). Oyster Reefs as Fish Habitat: Opportunistic Use of Restored Reefs by Transient Fishes. J. Shellfish Res. 20 (3), 951–959.
Hollarsmith, J. A., Griffin, S. P., and Moore, T. D. (2012). “Success of Outplanted Acropora Cervicornis Colonies in Reef Restoration,” in Proceedings of the 12th International Coral Reef Symposium, Cairns, Australia, July 9–13, 2012.
Huijbers, C. M., Nagelkerken, I., Lössbroek, P. A. C., Schulten, I. E., Siegenthaler, A., Holderied, M. W., et al. (2012). A Test of the Senses: Fish Select Novel Habitats by Responding to Multiple Cues. Ecology 93 (1), 46–55. doi:10.1890/10-2236.1
Johari, I., Said, S., Hisham, B., Bakar, A., and Ahmad, Z. A. (2010). Effect of the Change of Firing Temperature on Microstructure and Physical Properties of clay Bricks from Beruas (Malaysia). Sci. Sinter 42 (2), 245–254. doi:10.2298/SOS1002245J
Jones, M. E., and Davidson, N. (2016). Applying an Animal‐centric Approach to Improve Ecological Restoration. Restor Ecol. 24 (6), 836–842. doi:10.1111/rec.12447
Ladd, M. C., Miller, M. W., Hunt, J. H., Sharp, W. C., and Burkepile, D. E. (2018). Harnessing Ecological Processes to Facilitate Coral Restoration. Front. Ecol. Environ. 16 (4), 239–247. doi:10.1002/fee.1792
Laegdsgaard, P., and Johnson, C. (2001). Why Do Juvenile Fish Utilise Mangrove Habitats? J. Exp. Mar. Biol. Ecol. 257 (2), 229–253. doi:10.1016/S0022-0981(00)00331-2
Loh, T. L., Archer, S. K., and Dunham, A. (2019). Monitoring Program Design for Data‐limited marine Biogenic Habitats: A Structured Approach. Ecol. Evol. 9 (12), 7346–7359. doi:10.1002/ece3.5261
McCormick, M. I., Chivers, D. P., Ferrari, M. C. O., Blandford, M. I., Nanninga, G. B., Richardson, C., et al. (2020). Microplastic Exposure Interacts with Habitat Degradation to Affect Behaviour and Survival of Juvenile Fish in the Field. Proc. R. Soc. B. 287, 20201947. doi:10.1098/rspb.2020.1947
Mercader, M., Blazy, C., Di Pane, J., Devissi, C., Mercière, A., Cheminée, A., et al. (2019). Is Artificial Habitat Diversity a Key to Restoring Nurseries for Juvenile Coastal Fish? Ex Situ Experiments on Habitat Selection and Survival of Juvenile Seabreams. Restor Ecol. 27 (5), 1155–1165. doi:10.1111/rec.12948
Moring, J. R., and Nicholson, P. H. (1994). Evaluation of Three Types of Artificial Habitats for Fishes in a Freshwater Pond in Maine, USA. Bull. Mar. Sci. 55 (2-3), 1149–1159. 11.
Nagelkerken, I., and Faunce, C. H. (2007). Colonisation of Artificial Mangroves by Reef Fishes in a marine Seascape. Estuarine, Coastal Shelf Sci. 75 (3), 417–422. doi:10.1016/j.ecss.2007.05.030
Nagelkerken, I., Sheaves, M., Baker, R., and Connolly, R. M. (2015). The Seascape nursery: A Novel Spatial Approach to Identify and Manage Nurseries for Coastal marine Fauna. Fish Fish 16 (2), 362–371. doi:10.1111/faf.12057
Oren, U., and Benayahu, Y. (1997). Transplantation of Juvenile Corals: A New Approach for Enhancing Colonization of Artificial Reefs. Mar. Biol. 127 (3), 499–505. doi:10.1007/s002270050038
Ortego, T. R. (2006). Analysis of Bioengineered Concrete for Use in a Submerged Reef Type Breakwater. MSc thesis. Louisiana (LA): Louisiana State University.
Pagán, B. S. P., and Mercado-Molina, A. (2018). Evaluation of the Effectiveness of 3D-Printed Corals to Attract Coral Reef Fish at Tamarindo Reef, Culebra, Puerto Rico. Conservation Evid. 15, 43–47.
Perkol-Finkel, S., and Sella, I. (2014). “Ecologically Active Concrete for Coastal and Marine Infrastructure: Innovative Matrices and Designs,” in Proceeding of the 10th ICE Conference: From Sea to Shore - Meeting the Challenges of the Sea: (Coasts, Marine Structures and Breakwaters 2013), 1139–1149. doi:10.1680/fsts597571139
Peterson, C., Grabowski, J., and Powers, S. (2003). Estimated Enhancement of Fish Production Resulting from Restoring Oyster Reef Habitat: Quantitative Valuation. Mar. Ecol. Prog. Ser. 264, 249–264. doi:10.3354/meps264249
Powers, S., Peterson, C., Grabowski, J., and Lenihan, H. (2009). Success of Constructed Oyster Reefs in No-Harvest Sanctuaries: Implications for Restoration. Mar. Ecol. Prog. Ser. 389, 159–170. doi:10.3354/meps08164
Ruhl, E. J., and Dixson, D. L. (2019). 3D Printed Objects Do Not Impact the Behavior of a Coral-Associated Damselfish or Survival of a Settling Stony Coral. PLoS One 14 (8), e0221157. doi:10.1371/journal.pone.0221157
Rutledge, K. M., Alphin, T., and Posey, M. (2018). Fish Utilization of Created vs. Natural Oyster Reefs (Crassostrea virginica). Estuaries and Coasts 41 (8), 2426–2432. doi:10.1007/s12237-018-0433-4
Santos, L. N., García-Berthou, E., Agostinho, A. A., and Latini, J. D. (2011). Fish Colonization of Artificial Reefs in a Large Neotropical Reservoir: Material Type and Successional Changes. Ecol. Appl. 21 (1), 251–262. doi:10.1890/09-1283.1
Scarcella, G., Grati, F., Bolognini, L., Domenichetti, F., Malaspina, S., Manoukian, S., et al. (2015). Time-series Analyses of Fish Abundance from an Artificial Reef and a Reference Area in the central-Adriatic Sea. J. Appl. Ichthyol. 31 (S3), 74–85. doi:10.1111/jai.12952
Sherman, R. (2002). Artificial Reef Design: Void Space, Complexity, and Attractants. ICES J. Mar. Sci. 59, S196–S200. doi:10.1006/jmsc.2001.1163
Smith, J. A., Lowry, M. B., Champion, C., and Suthers, I. M. (2016). A Designed Artificial Reef Is Among the Most Productive marine Fish Habitats: New Metrics to Address 'production versus Attraction'. Mar. Biol. 163 (9), 188. doi:10.1007/s00227-016-2967-y
Strain, E. M. A., Morris, R. L., Coleman, R. A., Figueira, W. F., Steinberg, P. D., Johnston, E. L., et al. (2018). Increasing Microhabitat Complexity on Seawalls Can Reduce Fish Predation on Native Oysters. Ecol. Eng. 120, 637–644. doi:10.1016/j.ecoleng.2017.05.030
Suding, K. N., and Hobbs, R. J. (2009). Threshold Models in Restoration and Conservation: A Developing Framework. Trends Ecol. Evol. 24 (5), 271–279. doi:10.1016/j.tree.2008.11.012
Talbot, F. H., Russell, B. C., and Anderson, G. R. V. (1978). Coral Reef Fish Communities: Unstable, High‐Diversity Systems? Ecol. Monogr. 48 (4), 425–440. doi:10.2307/2937241
Tamburri, M. N., Luckenbach, M. W., Breitburg, D. L., and Bonniwell, S. M. (2008). Settlement of Crassostrea Ariakensis Larvae: Effects of Substrate, Biofilms, Sediment and Adult Chemical Cues. J. Shellfish Res. 27 (3), 601–608. doi:10.2983/0730-8000(2008)27[601:socale]2.0.co;2
Tarazi, E., Parnas, H., Lotan, O., Zoabi, M., Oren, A., Josef, N., et al. (2019). Nature-Centered Design: How Design Can Support Science to Explore Ways to Restore Coral Reefs. Des. J. 22 (Suppl. 1), 1619–1628. doi:10.1080/14606925.2019.1594995
Trilsbeck, M., Gardner, N., Fabbri, A., Haeusler, M. H., Zavoleas, Y., and Page, M. (2019). Meeting in the Middle: Hybrid clay Three-Dimensional Fabrication Processes for Bio-Reef Structures. Int. J. Architectural Comput. 17 (2), 148–165. doi:10.1177/1478077119849655
Umar, A. N., Zakaria, Z., Anwar, R., and Hassan, O. H. (2015). “Stoneware Clay as a Replacement Material for Artificial Reef Design,” in International Colloquium of Art and Design Education Research (I-CADER 2014). Editors O. H. Hassan, S. Z. Abidin, R. Legino, R. Anwar, and M. F. Kamaruzaman (Singapore: Springer), 145–152. doi:10.1007/978-981-287-332-3_16
Urbina-Barreto, I., Chiroleu, F., Pinel, R., Fréchon, L., Mahamadaly, V., Elise, S., et al. (2021). Quantifying the Shelter Capacity of Coral Reefs Using Photogrammetric 3D Modeling: From Colonies to Reefscapes. Ecol. Indicators 121, 107151. doi:10.1016/j.ecolind.2020.107151
Verweij, M., Nagelkerken, I., de Graaff, D., Peeters, M., Bakker, E., and van der Velde, G. (2006). Structure, Food and Shade Attract Juvenile Coral Reef Fish to Mangrove and Seagrass Habitats: A Field experiment. Mar. Ecol. Prog. Ser. 306, 257–268. doi:10.3354/meps306257
Walles, B., Troost, K., van den Ende, D., Nieuwhof, S., Smaal, A. C., and Ysebaert, T. (2016). From Artificial Structures to Self-Sustaining Oyster Reefs. J. Sea Res. 108, 1–9. doi:10.1016/j.seares.2015.11.007
Wangpraseurt, D., You, S., Azam, F., Jacucci, G., Gaidarenko, O., Hildebrand, M., et al. (2020). Bionic 3D Printed Corals. Nat. Commun. 11 (1), 1748. doi:10.1038/s41467-020-15486-4
Wilson, S. K., Burgess, S. C., Cheal, A. J., Emslie, M., Fisher, R., Miller, I., et al. (2008). Habitat Utilization by Coral Reef Fish: Implications for Specialists vs. Generalists in a Changing Environment. J. Anim. Ecol. 77 (2), 220–228. doi:10.1111/j.1365-2656.2007.01341.x
Wolfe, K., and Mumby, P. J. (2020). RUbble Biodiversity Samplers: 3D‐printed Coral Models to Standardize Biodiversity Censuses. Methods Ecol. Evol. 11 (11), 1395–1400. doi:10.1111/2041-210X.13462
Xu, Q., Zhang, L., Zhang, T., Zhang, X., and Yang, H. (2017). Functional Groupings and Food Web of an Artificial Reef Used for Sea Cucumber Aquaculture in Northern China. J. Sea Res. 119, 1–7. doi:10.1016/j.seares.2016.10.005
Yirmibesoglu, O. D., Morrow, J., Walker, S., Gosrich, W., Cañizares, R., Kim, H., et al. (2018). “Direct 3D Printing of Silicone Elastomer Soft Robots and Their Performance Comparison with Molded Counterparts,” in 2018 IEEE International Conference on Soft Robotics (RoboSoft), Livorno, Italy, April 2018, 295–302. doi:10.1109/ROBOSOFT.2018.8404935
Keywords: artificial habitat, artificial reef, biogenic habitat, structural complexity, habitat selection, restoration ecology, 3D printing
Citation: Garg A and Green SJ (2022) An Integrative Method For Enhancing the Ecological Realism of Aquatic Artificial Habitat Designs Using 3D Scanning, Printing, Moulding and Casting. Front. Built Environ. 8:763315. doi: 10.3389/fbuil.2022.763315
Received: 24 November 2021; Accepted: 08 April 2022;
Published: 03 June 2022.
Edited by:
Rodolfo Silva, National Autonomous University of Mexico, MexicoReviewed by:
Edgar Mendoza, National Autonomous University of Mexico, MexicoJose Manuel Mata-Padilla, CONACYT Center for Research in Applied Chemistry (CIQA), Mexico
Copyright © 2022 Garg and Green. This is an open-access article distributed under the terms of the Creative Commons Attribution License (CC BY). The use, distribution or reproduction in other forums is permitted, provided the original author(s) and the copyright owner(s) are credited and that the original publication in this journal is cited, in accordance with accepted academic practice. No use, distribution or reproduction is permitted which does not comply with these terms.
*Correspondence: Aneri Garg, YW5lcmkuZ2FyZ0BnbWFpbC5jb20=