- 1College of Architecture and Design, Effat University, Jeddah, Saudi Arabia
- 2Faculty of Engineering, October University for Modern Sciences and Arts (MSA University), Cairo, Egypt
This paper investigates the environmental benefits of adopting hybrid vernacular-modern building technologies through a detailed life-cycle assessment (LCA) of a residential prototype known as the “Ecofordable House” (EH). The EH integrates hybrid techniques, including partially reinforced interlocking compressed stabilized earth brick walls (ICSEB), jack arch and funicular shell roofing systems, and date palm midrib components. Its environmental impacts are compared to those of a reinforced concrete house (CH) use as the baseline conventionally adopted in the Middle East. The LCA follows a cradle-to-grave scenario, covering stages A1–A4, B1–B5, and C1–C4, with additional reference to stage D. The results showed that the CH has a value of 698.22 kg CO2e/m2, while the EH has a Global Warming Potential of 368.17 kg CO2e/m2, which represents a reduction of approximately 47% in global warming potential (GWP). Fossil-based emissions in the EH are 46% lower, biogenic emissions are reduced by 91%, and land use and land-use change (LULUC) have an impact reduction of 82%. The acidification potential is 43% lower, while eutrophication across freshwater, marine, and terrestrial resources is 28%–44% lower. The photochemical ozone creation potential (POCP) is reduced by 43%, and the resource depletion impact for elements and for fossil fuels is reduced by 50% and by 43%, respectively. Water use is 18% lower. Material production (A1–A3) is identified as the primary driver of environmental impacts for both prototypes. Fired clay bricks, concrete, and reinforcement steel are the major contributors toward GWP for CH, while Portland cement, concrete, and reinforcement steel dominate the GWP for EH, but with much lower values due to their reduced quantities. For CH, the major building part contributors are the foundation, roofs, and external walls, while in the EH, conventional reinforced concrete (RC) foundations and external walls are the major contributors. These results support the significant environmental benefits of adopting hybrid modern and vernacular building technologies and materials as ways of reducing environmental impacts while ensuring more durable and structurally sound buildings in hot, arid climates.
1 Introduction
1.1 Research background
The construction industry significantly contributes to global environmental impacts. These impacts include 34% of all energy use by building construction and operation, and carbon emissions account for approximately 37% alone (Zhang et al., 2024; Zhong et al., 2021). Increasingly, there is a call for buildings to be designed and constructed in ways that have lower impacts on the environment.
Vernacular architecture, which describes building practices adapted to local conditions, materials, and cultural context, has received renewed interest as a model for sustainable construction. The integration of such approaches with modern sustainability practices has been studied by Salman (2019) and Zong et al. (2024), among others, showing that such approaches might potentially reduce environmental impact while preserving cultural heritage.
The study by Jagatramka et al. (2021) examines vernacular architectural changes caused by globalization, socio-economic transformations, and material accessibility. The changes adhere to hybrid models such as the Ecofordable House (EH) (Abdel and Abo Eldardaa, 2023), using vernacular materials in combination with modern construction to yield a smaller ecological footprint. Traditional materials are often replaced as a result of urbanization, while hybrid models can conform to be more sustainable by enhancing their constituent materials.
In more industrialized countries, vernacular building materials such as earth are being rediscovered because they are both environmentally and economically advantageous. Enhanced vernacular materials, such as rammed and compressed earth, are less costly, and they can serve modern standards. In developing countries, traditional materials were replaced by concrete and burned bricks to make houses more durable (Gado et al., 2010; Un-Habitat, 2009; 2012). Marsh and Kulshreshtha (2022) observe that though earth is increasingly being used in diverse ways presently in some areas, the old figure of approximately 1/3 of the world’s population living in earthen dwellings is outdated, and current figures stand at 8%–10% globally and up to 20%–25% in developing countries. With growing environmental awareness on the part of businesses, governments, and consumption, efforts are focused on industries that offer the greatest scope for reducing such impacts, including construction. The industry is under increasing pressure not only to reduce the causes and results of global warming but also, through circular economy measures, to reduce raw material depletion, particularly a material that cannot be renewed naturally (Ogunmakinde, 2024; Sandanayake, 2022).
Life-cycle assessment (LCA) has been developed as a methodology for determining a building’s environmental impact in different stages of its life cycle, starting from resource extraction and processing to construction, use, and finally demolition (Dsilva et al., 2023). It estimates impact categories, which are quantified environmental burdens used to assess the building’s impact throughout its life cycle, such as global warming potential (GWP), acidification, eutrophication, smog formation, and ozone depletion (Alsaid et al., 2023; Cabeza et al., 2014; Ortiz et al., 2009). Numerous studies have established that LCA is useful in providing quantified data on the environmental performance of buildings, pointing to areas of potential to mitigate carbon footprint, resource use, and other environmental impacts (Cabeza et al., 2014; Nwodo and Anumba, 2019). Of particular interest are the studies by Ortiz et al. (2009) and Thormark (2006) that highlight the need to undertake LCA for various types of buildings and construction methods, emphasizing that detailed, context-specific assessments that account for local material availability and construction practices need to be undertaken. In a life-cycle assessment (LCA), life-cycle impact assessment (LCIA) methods that account for embodied carbon content vary with respect to regional standards. The methodology in Europe generally applies the CML 4.1 calculation method and follows the EN 15804 standard (European Committee for Standardization, 2021), which is applicable worldwide (except North America). Methodologies in North America are based on TRACI 2.1, developed by the United States Environmental Protection Agency (EPA) (Sartori et al., 2021).
Comparative LCAs between conventional and eco-friendly buildings have been conducted in order to understand the environmental benefits of sustainable construction. Ben-Alon et al. (2021) studied the LCA of natural soil and bio-based resources against contemporary materials in several desert, Mediterranean, temperate, and continental zones. Embodied and operational impacts showed that natural resources lower energy by 27%–73%. Carrobé et al. (2024) compared the environmental impact of earthbag construction with regular cement block construction in the context of the Western Sahara. The results indicate that cement blocks are worse, sometimes presenting an impact 70% higher than earthbag technology.
Rinne et al. (2022) performed a whole-building life-cycle assessment of a hybrid five-floor apartment block in Finland with a combination of timber and concrete and compared it to other alternatives. Given the performance of hybrid solutions, integrating wood-based components may suggest, among other benefits, effective utilization of wood, promoting the creation of more efficient structures. Throughout the study, they also reviewed research on LCA applications, including studies that utilized One Click LCA software.
Jayawardana et al. (2023) conducted LCA-based environmental evaluations for in situ and prefabricated construction methods of office buildings in Sri Lanka. The analysis indicated that the prefabricated method reduces greenhouse gases (GHGs) by 8.06%. In addition, the application of green concrete in prefabrication has been found to further reduce GHG emissions by an extra factor of 2.83%–12.05%.
Gazquez et al. (2022) compare a vernacular and a contemporary social house in Catamarca, Argentina, by means of LCA to assess embodied and operational energy and overall CO2 emissions. The findings revealed that the vernacular house consumes 35% of the embodied energy and 62.9% of the baseline’s operational energy.
Fernandes et al. (2019) investigated the impacts of compressed earth blocks and rammed earth using LCA in a Portuguese context. A cradle-to-gate analysis has shown that using earthen building material will potentially reduce the environmental impact by approximately 50% compared to conventional material.
Despite the growing literature on LCA and sustainable construction, there are some research gaps that this study attempts to fill. First, while several studies have focused on the LCA of buildings in temperate climates (Fnais et al., 2022), there is still a significant case for further research on sustainable construction in dry, hot climates in the Middle East and North Africa.
While LCAs of traditional houses are available (Kaoula et al., 2022), their integration with modern sustainable technologies is rarely investigated. The Ecofordable House presented in this study represents an example of hybrid systems that incorporate vernacular and modern technologies to meet standards of sustainability. It can help prepare a way ahead for a more sustainable built environment, especially in arid regions where environmental conditions present unique challenges for building performance. The functional appropriateness of vernacular construction is deeply entrenched in knowledge about the contextual climates and limitations of resources, yielding valued insights into how buildings can be designed to minimize environmental impacts while being internally comfortable and functional. In contrast, these principles find only limited application in contemporary construction, with even less exposure in LCA-based evaluations (Nguyen et al., 2019; Tawayha et al., 2019).
Most studies have not incorporated real data from a fully constructed prototype and instead based their work on simulations, assumptions, or constructed building parts or units (Arduin et al., 2022; Dormohamadi et al., 2024; Fouly and Abdin, 2022; Nouri et al., 2023). This paper contributes toward addressing these gaps by providing a detailed LCA about a fully constructed eco-friendly prototype constructed in an arid climate using real site documentation.
While numerous studies have focused on operational energy use (Tan et al., 2020; Zhong et al., 2021), this study places greater emphasis on embodied impacts by excluding operational phases and focusing on construction and material-related impacts. This provides a far more complete understanding of the environmental benefits that may be derived from hybrid building materials and practices.
1.2 Aim and significance
This paper follows earlier work that investigated the economic and structural viability of hybrid vernacular-modern technologies through a residential prototype, the EH, which integrates enhanced vernacular technologies constructed using locally sourced materials with low embodied carbon (Abdel and Eldardaa, 2023; Abdel Gelil et al., 2024). These include partially reinforced compressed earth bricks made with local sandy soil, a hybrid roof system where the conventional reinforced concrete (RC) volume is reduced by replacing part of it with small-span curved masonry roofs, and date palm midribs as a sustainable alternative to imported wood.
The research extends the previous studies by conducting a detailed comparative LCA to evaluate the GWP and other environmental impacts of the EH and a conventional RC counterpart, using One Click LCA software. Because it was fully constructed on site with real-world data documented, the EH serves as a benchmark regarding how well the integration of vernacular design principles with modern sustainability tools works.
The findings of this study emphasize that there is a need to revise conventional construction methodologies to more sustainable ones, which could combine traditional solutions with modern technologies. This research explores the environmental consequences of hybrid construction technologies applied in the EH by integrating cement and steel with vernacular and traditional approaches for superior durability and structural sustainability.
The EH in the Western suburban area of Giza represents how those hybrid techniques (partially reinforced ICSEB, jack arches and funicular shells, and palm midribs) reduce the environmental footprint while maintaining structural integrity. The paper adds to the previous studies on cost-effectiveness, affordability, and structural performance of the EH (Abdel and Abo Eldardaa, 2023; Abdel Gelil et al., 2024) by quantifying the environmental impacts of these improved vernacular technologies for a more comprehensive analysis of the house. Hybrid construction methods are significant for regions whose climate and resource conditions raise demands for sustainable solutions (Chen et al., 2024; Mohamed, 2022).
2 Methodology and materials
2.1 Case study description
In this study, an LCA was conducted on an experimental residential prototype, the Ecofordable House (EH), which was fully constructed at the MSA University Campus in Cairo, Egypt, to investigate the environmental impacts of integrating modern RC technology and traditional vernacular construction methods. Another LCA was conducted on a non-constructed Conventional House (CH), modeled following standard reference data from typical RC-framed houses in Egypt, to serve as a comparative benchmark. Assessments were based on a 60-year lifespan from cradle to grave.
Among other recognitions, the EH (Figure 1) was awarded first prize at the “4th Cairo International Exhibition of Innovation” (Abdel and Abo Eldardaa, 2023) and was shortlisted for the Times Higher Education MENA Awards 2023 in the category “Outstanding Contribution to Environmental Leadership.” The prototype was followed by a pilot project, the “Rural Green House Prototype,” completed in El Kharga Oasis, New Valley Governorate. The EH thus acts as a prototype of sustainable, low-impact housing adapted to arid climates. The house is 100 m2 in total. The one-floor configuration, with a usable roof, contains a porch, hallway, living room/reception, two bedrooms, one kitchen, one bathroom, a terrace, and a stairway to the roof. It fulfills the requirement of reduced consumption of energy-intensive materials like cement, steel, and fired bricks yet maintains structural integrity. Abdel and Abo Eldardaa (2023) and Abdel Gelil et al. (2024) extensively investigated the house’s compliance with Egyptian codes, structural, thermal, and acoustical performance, construction steps, and material properties.
The main walling system of the house (Figure 2) is hollow interlocking compressed stabilized earth bricks (ICSEB), prepared from local sandy soil stabilized with Portland cement. These are hollow bricks that could be partially reinforced with steel at key structural points to give strength while minimizing material consumption. Moreover, the interlocking features and accurate dimensions minimize the use of mortar, further contributing to reducing the cement content. The bricks were manufactured on site by a small hydraulic press and, therefore, have reduced material transportation costs.
External walls are 25 cm thick, while internal walls are 12.5 cm thick according to Egyptian construction codes, “Design and Construction of Masonry Works” (ECP 204–2005) (Egyptian Code Committee, 2001a) and “Building with stabilized earth–part one – 2016” (EG-SE2016) (Egyptian Code Committee, 2001b). Bricks made of 70%–75% sand and 25%–30% fine particles, of which 10% was clay, were stabilized with 10% cement by weight. High-strength bricks with 15% cement were used at structural locations such as the first three courses and brick beams. These were cured under humid conditions to give full strength; compressive strengths ranged between 5.1 N/mm2 and 11.4 N/mm2. Mortar was laid in a 1:4 cement-sand ratio for the first course and slurry thin soil-cement mortar thereafter. The walls were treated with water-based acrylic varnish, drastically reducing the water absorption and enhancing durability.
The roofing system of the EH is hybrid (Figure 2), combining masonry jack arches and funicular shells supported by reinforced concrete beams. Jack arches were constructed from fired bricks and wooden formwork, while fired bricks arranged on mud molds were used to shape the doubly curved funicular shells. These systems are enhanced versions of traditional vaults and domes that have smaller spans and are supported by RC or steel beams; they provide structural stability and the possibility of adding floors (Jain, 2000; Keswani, 1997; Khan et al., 2013; Kumar and Maheswari, 2019; Leo Samuel et al., 2017; Maheri and Rahmani, 2003; M. Mohamed, 2014; Venkatarama Reddy, 2009). The EH’s roof was finished with ceramic tiles laid over a sand-filled surface and is fully accessible and usable (the previously investigated finite analysis was performed on a two-floor design (Abdel Gelil M. et al., 2024)).
The date palm leaf midribs (DPLMs), a locally abundant, renewable material (El-Mously, 2018; Midani et al., 2020), were used in place of wood for the making of shutters and internal doors, therefore reinstating traditional craftsmanship and reducing reliance on imported wood. DPLMs provided significant cost savings, reducing material expenses by up to 70% of its value. DPLMs were hand-crafted as slats using traditional furniture-making techniques. These slated latticework patterns were installed inside a wooden frame; non-transparent polycarbonate sheets were installed at the back of the internal door’s wider latticework. To control weevils and for better longevity and durability, a pesticide was sprayed on the fronds prior to manufacturing.
The necessary LCA data for a conventional RC house came from the One Click LCA software database, leveraging datasets curated and verified globally representing standard construction practices. In Egypt, 90% of residential buildings predominantly consist of reinforced concrete (RC) frames combined with masonry infill walls. Typically, these buildings are made up of RC skeletons that comprise foundations, beams, columns, and slabs and employ cement or red fired brick or block masonry in partitions and exterior walls (Aly and Abdelaziz, 2024; ElGohary and Khashaba, 2018).
In a previous study conducted by Abdel and Abo Eldardaa (2023), where the cost-effectiveness of the EH was investigated and compared to that of the CH, professional contractors prepared a detailed bill of quantities for conventional construction of a model similar in design to the EH to give accurate material estimates. The Conventional House has RC columns and beams with a 20-cm-thick RC slab for the roof. The walls are constructed with fired clay bricks, plastered, and painted following traditional masonry construction. Other than walls and the roof, standard wooden paneled doors and windows made of glass and imported wood were used (Abdel and Abo Eldardaa, 2023).
2.2 Life-cycle assessment
2.2.1 Framework
The LCA methodology follows ISO 14040 & ISO 14044 (International Organization for Standardization, 2020a; International Organization for Standardization, 2020b), which establish a standardized framework for evaluating the environmental impact of buildings and materials through five primary phases: i) goal and scope, ii) system boundaries, iii) life-cycle inventory, iv) impact assessment, and v) interpretation of results. Additionally, the study adheres to EN 15978 and EN 15804, which categorize environmental impact into modular life-cycle stages: Product Stage (A1–A3), Construction Stage (A4–A5), Use Stage (B1–B7), End-of-Life Stage (C1–C4), and Module D for potential benefits beyond system boundaries.
2.2.2 Goal and scope
The aim of the present paper is to use LCA methodology to investigate the environmental impact of an eco-friendly prototype, the EH, and compare it with a conventional RC-frame house (CH) over a 60-year lifespan. The functional unit for this study is a residential building with a gross internal floor area (GIFA) of 100 m2. The LCA quantifies the EH and CH impacts from cradle to grave, encompassing resource manufacturing, transportation, construction, use, and end of life, as detailed in the following section.
2.2.3 System boundaries
The EH and CH LCA modules assessed in this study are illustrated in Figure 3. The Product Stage (A1–A3) evaluates the impacts of the extraction, transportation, and manufacturing of resources. The Transportation and Construction Stages (A4–A5) evaluate their transportation to the construction location as well as the installation processes. The Use Stage (B1–B5) covers a building’s operating life cycle, with environmental impacts measured using embodied carbon and other impact categories. The End-of-Life Stage (C1-C4) represents emissions from deconstruction, transport, processing, and disposal. Stage D considers potential environmental benefits beyond the system boundary, including material reuse, recovery, and recycling. Operational energy (B6) and water consumption (B7) have been excluded to emphasize the embodied impacts of materials and construction processes throughout the buildings’ life cycles. Previous LCA studies have utilized this approach for their focus on embodied carbon throughout a building’s life cycle (Anand and Amor, 2017; Hu and Esram, 2021; Moncaster and Symons, 2013; Sandanayake, 2022).
2.2.4 Life-cycle inventory
Data about the EH (Table 1) are gathered from real site documentation, with detailed records of the consumed materials and construction processes. This gives a robust basis for the LCA of the EH. Where the conventional RC house is concerned, this LCA takes typical reference materials and construction practices that are locally typical for its basis. For this LCA, data were primarily sourced from environmental product declarations (EPDs) and verified databases, adhering to ISO 14040/44 and EN 15804 standards. EPDs for the materials used in both the EH and the CH were sourced locally in Egypt through One Click LCA software. The available EPDs were used to provide valid environmental impacts on material manufacture, transportation, and end-of-life. In the absence of EPDs, generic data from similar materials according to the standards from EN 15804 and industry averages from reliable databases such as IMPACT and NMD were used. In the EH, this only occurred for the red and black iron oxide pigments used to produce colored compressed stabilized earth blocks (CSEB). Additionally, the date palm midribs used in the EH were modeled using the closest available dataset: high-density laminated bamboo wood. This selection was made due to the absence of specific EPDs for date palm midribs in the One Click LCA database. The other minor materials, such as small fittings not covered by most EPDs, were assumed to have negligible impact and thus were excluded.
Materials inventories of both the EH and its conventional counterpart present a detailed overview of construction materials used in the LCA. Tables 2, 3 record the components by construction categories, including foundation, walls, roof, finishes, and doors and windows, showing material per building component, quantity, and transportation distance. More detailed descriptions of materials, transportation types, local availability, and waste distribution for both houses are provided in Supplementary Tables S1, S2.
2.2.5 Impact assessment
The outcomes of the LCAs are built with a characterization approach, which converts the emissions and resource usage into an equivalent environmental impact. The calculations are based on the CML 4.1 impact assessment methodology, carried out with One Click LCA software, for the categories listed in Table 4. These include global warming potential (GWP), acidification potential, accumulated exceedance (AP), eutrophication freshwater (EP-freshwater), eutrophication aquatic marine (EP-marine), eutrophication terrestrial (EP-terrestrial), ozone depletion potential (ODP), formation potential of tropospheric ozone (POCP), and abiotic depletion potential (ADP).
One Click LCA software is widely used to assess the environmental impacts of buildings and construction materials. It includes a large verified global and regional database that allows for accurate impact quantification (Dalla Mora et al., 2020; Rinne et al., 2022). The software’s adherence to international standards provides a reliable comparison between the environmental impacts of the Ecofordable House and its conventional counterpart.
3 Results and discussion
This section is divided into four parts. The first part presents the LCA results and provides an overview of the environmental impacts of the EH and CH, including comparisons of key impact categories. The second part focuses on GWP, analyzing its distribution across life-cycle stages, resource types, building parts, and GWP categories (LULUC, fossil, and biogenic). The third part examines other impact categories beyond GWP. Finally, the fourth part offers a comparative summary of the LCA results of the EH and CH.
3.1 Overview of LCA results and environmental impacts
Tables 5, 6 detail the environmental impacts of the EH and the CH, with a side-by-side comparison in Table 7. The findings highlight that the EH has a significantly lower impact across categories. The GWP of the EH is 368.17 kg CO2e per square meter, which is 47.3% lower than the CH (698.22 kg CO2e). The EH’s fossil-based emissions are 46% lower, while biogenic and land-use change emissions are 91.1% and 82.4% lower, respectively. The acidification potential is 42.4% lower, and eutrophication impacts are reduced: 28.4% in freshwater, 43.9% in marine environments, and 42.4% in terrestrial ecosystems. The EH’s advantage is emphasized further by resource depletion metrics, which show 42.8% lower fossil fuel consumption and 50% lower element depletion per square meter. The EH reduces water use by 18% per m2.
The “Performance Metric Carbon Heroes Benchmark” chart (Figure 4) evaluates the embodied carbon results for the EH and the CH, excluding operational carbon (Hollberg et al., 2019). When compared to the global benchmark for single dwelling buildings, Global One Dwelling Building 2023 Q3, which reports an average GWP of 414 kg CO2e/m2 for A1-A4, B4-B5, and C1-C4 (Jungclaus et al., 2024), the EH achieves a GWP of 350 kg CO2e/m2, placing it within the “C” performance range. In contrast, the CH records a much higher GWP of 652 kg CO2e/m2, positioning it in the “G” category, indicative of significantly greater environmental impact. The EH demonstrates a 15.4% improvement over the benchmark, while the CH exceeds this global average by 57.5%.
3.2 Global warming potential (GWP)
3.2.1 GWP by life-cycle stage
Both prototypes show a similar distribution of impacts across life-cycle stages in proportion, though the total values of GWP reveal the advantages of the hybrid sustainable approach included in the EH (Figure 5). Material production is the stage with the highest impact for both houses, A1-A3, and contributes about 86.0% for the EH and 84.1% for the CH, with 31,970.17 kg CO2e and 60,041.09 kg CO2e, respectively. The CH is dependent on almost twice as many high-carbon resources such as steel, cement, and fired bricks than the EH, which integrated smaller quantities of these materials.
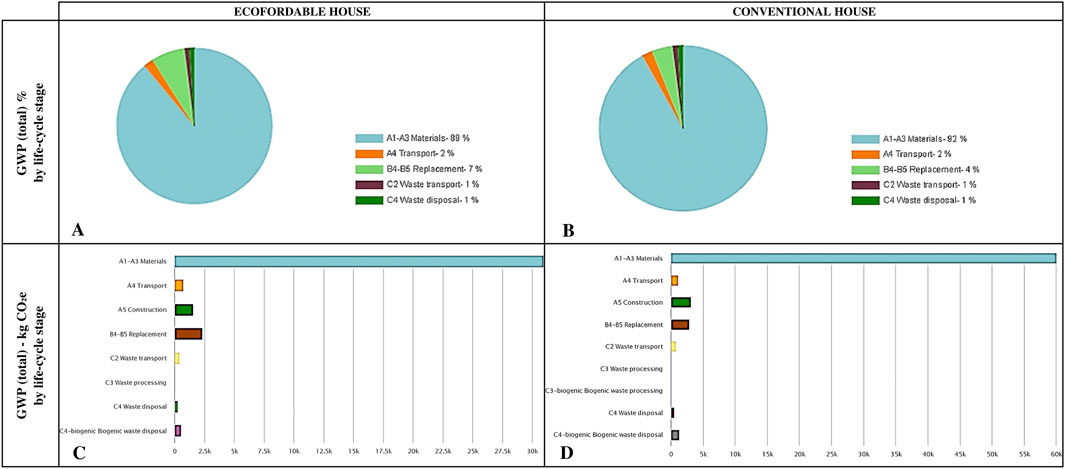
Figure 5. GWP-total by life-cycle stages: (A) percentage for the EH; (B) percentage for the CH; (C) values in kg CO2e for the EH; (D) values in kg CO2e for the CH.
A4 Transportation impacts are low in both, comprising 1.7% in the EH at 715.5 kg CO2e and 1.9% in the CH at 1,167.2 kg CO2e. This is due to the local supply of materials, such as local soil available on campus for producing ICSEB; the CH uses longer transportation distances for conventional material supplies. A5 (Construction) accounts for 4.5% in the EH (1,548.07 kg CO2e) and 4.2% in the CH (3,131.76 kg CO2e). Emissions in the EH are lower because the ICSEB is a mortar-less system and leads to minimal construction waste, but the CH uses more resource-intensive methods.
Material replacement and refurbishment, B4–B5, had a higher percentage in the CH, at 6.4% and 2,823.39 kg CO2e, than in the EH, with 4.0% and 2,342.37 kg CO2e. The EH does not rely on conventional earth construction but adopts reinforced interlocking CSEB walls and does not need plastering or finishing, which typically require refurbishment. The walls were, however, coated with water-based acrylic varnish, which significantly reduced water absorption and enhanced durability, as established in a previous study (Abdel and Abo Eldardaa, 2023; Abdel Gelil M. et al., 2024). Additionally, the EH’s construction consists of small-span fired brick domes and vaults supported on RC ribs, offering durability and structural viability. The interior of the roof is unfinished, bearing only a varnish coat. All these contribute to lower refurbishment needs than conventional construction, as shown in the results of the LCA, in which the CH exhibited slightly higher material substitution emissions than the EH. The previous investigations confirm its durability and support the assumptions of the LCA over a 60-year lifespan.
C1-C4 (end-of-life) impacts are in similar ranges among the EH and the CH: 3.4% (1,240.75 kg CO2e) and 3.9% (2,685.56 kg CO2e), respectively. Nevertheless, the higher emissions within the CH originate from the problems of processing/waste management of conventional materials like reinforced concrete and steel.
3.2.2 GWP by resource type (most contributing materials)
Tables 8, 9 identify the materials that contribute the most to GWP-fossil, and Figure 6 further illustrates the share of the materials in the total GWP. In the CH, materials like high-strength concrete, steel reinforcement, and fired bricks are the primary contributors, while the EH’s GWP is driven by Portland cement and partially reinforced compressed earth bricks. The flow of impacts in the Sankey diagrams (Figure 6) underscores the effectiveness of material substitution and design optimization in reducing emissions for the EH.
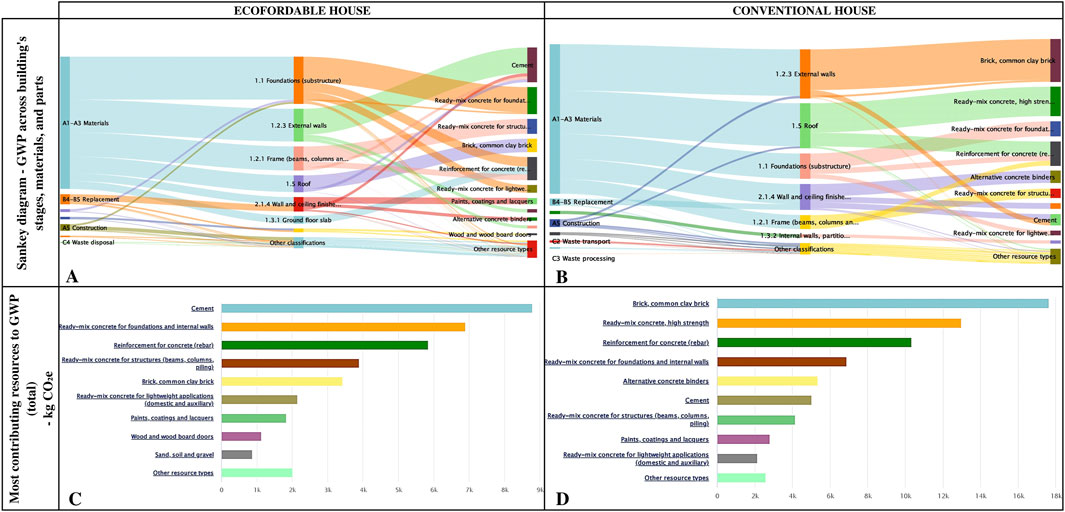
Figure 6. Material contributions to GWP: (A) Sankey diagram for the EH, (B) Sankey diagram for the CH, (C) highest contributing materials for the EH, and (D) highest contributing materials for the CH.
The analysis of the materials contributing to the GWP-fossil of the CH and the EH puts into evidence remarkable differences between the environmental impact of these two types of houses. In the CH, the most relevant contributor to GWP is fired clay bricks, accounting for 27.2% of the total impact. This is followed by ready-mix concrete (19.5%, 12 tons CO2e) and reinforcement steel (16.1%, 9.7 tons CO2e). While these materials give structural integrity, they greatly increase the overall GWP of CHs due to their energy-intensive production methods.
In contrast, the EH uses materials whose GWP contributions are much lower due to reduced quantities: Portland cement at 26.1% (8.2 tons CO2e), ready-mix concrete at 18.8% (5.9 tons CO2e), and reinforcement steel at 17.4% (5.5 tons CO2e). Although the percentage of material contribution is quite similar in both houses, the absolute GWP in the EH is notably lower. Material choices for an EH are thus made in conscious balancing in pursuit of structural performance with environmental sustainability to realize a GWP far below that of a CH.
3.2.3 GWP by building parts
The Spidergram grouped by building parts (Figure 7) highlights the distribution of environmental impacts across different building components. For the CH, external walls, roofs, and foundations dominate the GWP impact, driven by the extensive use of high-carbon resources like reinforced concrete and fired clay bricks. Conversely, the EH achieves a balanced impact distribution, with its walls and foundations contributing the most but at significantly lower levels due to the adoption of ICSEBs and hybrid roofing systems.
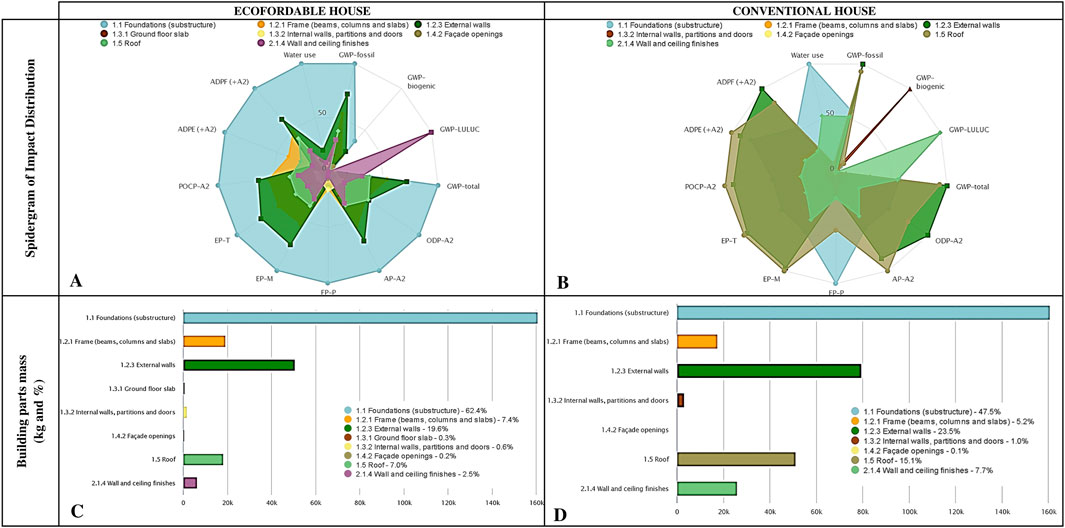
Figure 7. Impact distribution and building parts mass comparison: (A) Spidergram of impact distribution for the EH, (B) Spidergram of impact distribution for the CH, (C) building parts mass (kg and %) for the EH, and (D) building parts mass (kg and %) for the CH.
The conventional strip foundations (62.4% of the total mass) contribute 31.2% to the total GWP of the EH. The emissions are 11,480.84 kg CO2e; in the case of the CH (47.5% of the total mass), the contribution is 16.4% and 11,480.84 kg CO2e. The external walling system of the EH (19.6% of the total mass) accounts for 22.4% of the total GWP at 8,227.13 kg CO2e, while the external fired clay brick walls in the CH (23.5% of the total mass) have the highest percentage share of 28.9%, amounting to 20,144.28 kg CO2e.
The EH roof system (7% of the total mass) contributes approximately 11.7% or 4,327.57 kg CO2e to the total GWP, while in contrast, the CH roof (15.1% of the total mass) accounts for 27.0% or 18,859.22 kg CO2e, representing a wide use of reinforced concrete flat slabs, hence significantly elevating emissions. The jack arch and funicular shell roof system minimizes the use of concrete, reducing its environmental impact. In the case of the EH, the wall and ceiling finishes (2.5% of the total mass) contribute approximately 9.6% to the overall GWP, amounting to 3,527.85 kg CO2e. They include a three-coat water-based acrylic varnish applied on the ICSEB that does not require adding other layers and the plastering of the hybrid roofs. In contrast, the CH walls and ceiling finishes (7.7% of the total mass) contribute 16.1% to the total GWP, amounting to 11,221.28 kg CO2e, including the conventional plastering and painting of the walls and roofs.
3.2.4 GWP breakdown (fossil, biogenic, and LULUC)
GWP breakdown (fossil, biogenic, and LULUC) is shown in Figure 8. The contribution by the fossil GWP dominates the total GWP for both houses. For instance, the EH has a fossil GWP of about 36,545.73 kg CO2e, accounting for approximately 99.3% of its total emissions, representing the application of materials such as cement and concrete, while the CH has a much higher fossil GWP of 67,696.37 kg CO2e contributing to 96.9% of its total. This alone contributes a high percentage due to the extensive utilization of fired clay bricks and reinforced concrete, both highly energy-intensive materials.
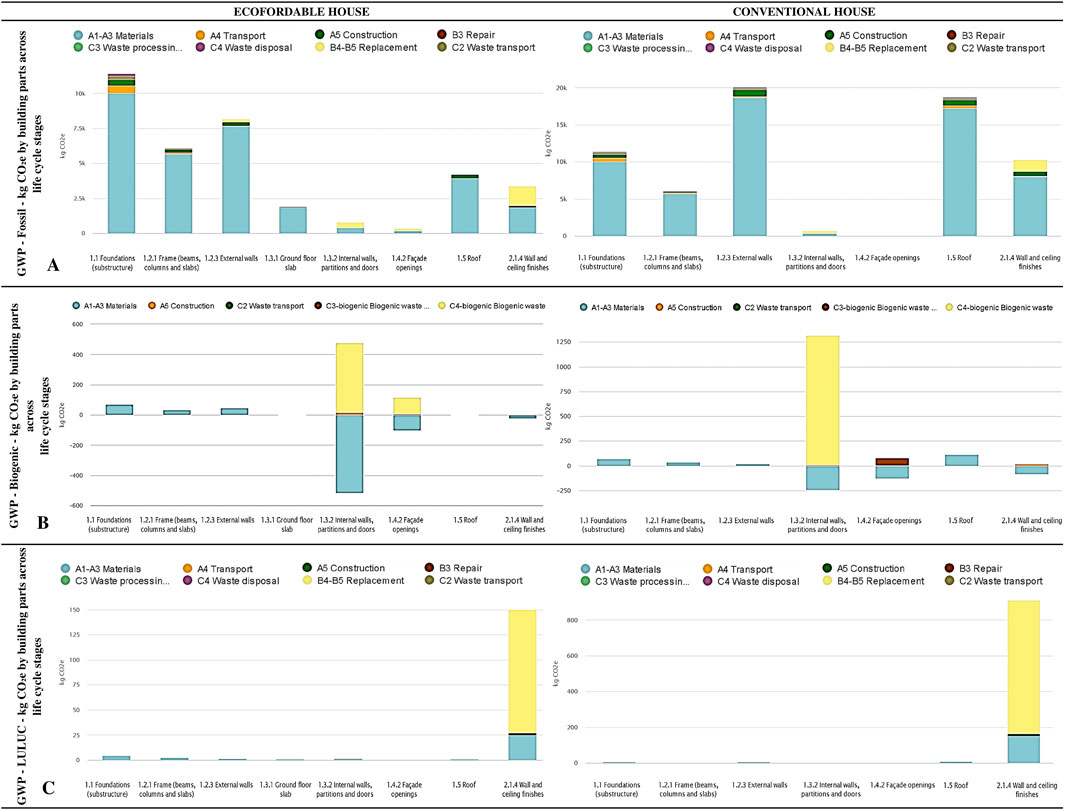
Figure 8. GWP breakdown by building part across life-cycle stages: (A) Fossil GWP (left: EH, right: CH), (B) biogenic GWP (left: EH, right: CH), and (C) LULUC GWP (left: EH, right: CH).
The LULUC GWP, representing all the emissions from land-use changes and extraction of resources, is small in the case of the EH, standing at 165.14 kg CO2e, which constitutes only 0.45% of the total GWP of the EH. This is because the EH is made from locally sourced materials such as ICSEBs and date palm midribs. In contrast, the CH has a very high LULUC GWP of approximately 935.82 kg CO2e, that is, 1.34% of the total amount emitted. This is mainly due to the environmental cost of extracting clay for bricks and sourcing imported wood (Burdova et al., 2023).
The biogenic GWP, which accounts for carbon dioxide absorption and emission from organic materials, was 105.99 kg CO2e for the EH, representing only 0.29% of its total GWP. While the EH contains limited renewable organic materials, primarily date palm midribs for doors and windows and wood frames, the Spidergram (Figure 7) indicates that its biogenic GWP also originates from the foundations and external walls. As mentioned earlier (Section 3.5: Data Sources and Assumptions), the palm midribs were modeled using the closest available dataset: high-density laminated bamboo wood. Given the small quantity of wood-based materials in an EH, differences are assumed to be negligible and do not significantly impact the results.
For the CH, biogenic GWP is significantly higher at 1,189.82 kg CO2e (1.7% of total GWP). While wooden doors are a major contributor, the Spidergram reveals that biogenic emissions are also concentrated in internal walls and partitions. This suggests that additional wood-based components, such as interior finishes or coatings, are influencing the results. Although wood temporarily stores carbon, its long-term impact depends on its end-of-life scenario, whether it is reused, decayed, or incinerated, as discussed by Head et al. (2021). Thus, while the CH exhibits a higher biogenic GWP, the fate of its wood materials in the technosphere could affect long-term emissions.
In summary, the GWP of the EH is far lower than that of the CH, being 46.3% lower in fossil GWP, 91.1% lower in biogenic GWP, and 82.4% lower in LULUC GWP. Overall, such a reduction epitomizes the environmental superiority of locally sourced materials and hybrid technologies.
3.3 Other impact categories
Almost no impact from either house was found on the ODP (Tables 5–7), which reflects the choice of materials and technologies that avoid ozone-depleting substances. The acidification potential in the CH is approximately 1.74 times higher (Figure 9), being 197.95 mol H+ eq., than that in the EH, which was approximately 113.9 mol H+ eq. This is due to the large mass of fired clay bricks and reinforced concrete in the CH, while the use of ICSEBs, locally available materials, and smaller mass of these materials in the EH minimize acidifying emissions (Eštoková et al., 2022). For EP-fresh water, -marine, and -terrestrial, the CH is consistently higher in impact than the EH. Values for the CH are 2.32 kg P eq., 56.8 kg N eq., and 601.94 mol N eq., while the EH recorded lower values of 1.66 kg P eq., 31.95 kg N eq., and 346.71 mol N eq., respectively. The higher eutrophication metrics of the CH are driven by high reliance on fired brick and reinforced concrete. The POCP is higher in the CH at 190.18 kg NMVOC eq. than in the EH, with a contribution of 107.85 kg NMVOC eq. It can be associated with energy-intensive manufacturing emissions in CH materials such as fired bricks and conventional wood. Regarding ADP, potential from the elements and fossil fuels in the CH is more, at 9.99 kg Sb eq. and 543,095.29 MJ, respectively, while the records in the EH are low at 5 kg Sb eq. and 310,703.47 MJ, respectively. Water use in the CH is very high—137,053.72 m3 deprived—compared with the EH, at 112,381.4 m3 deprived. This can be explained by the fact that most of the materials in the CH have large volumes, especially concrete and bricks.
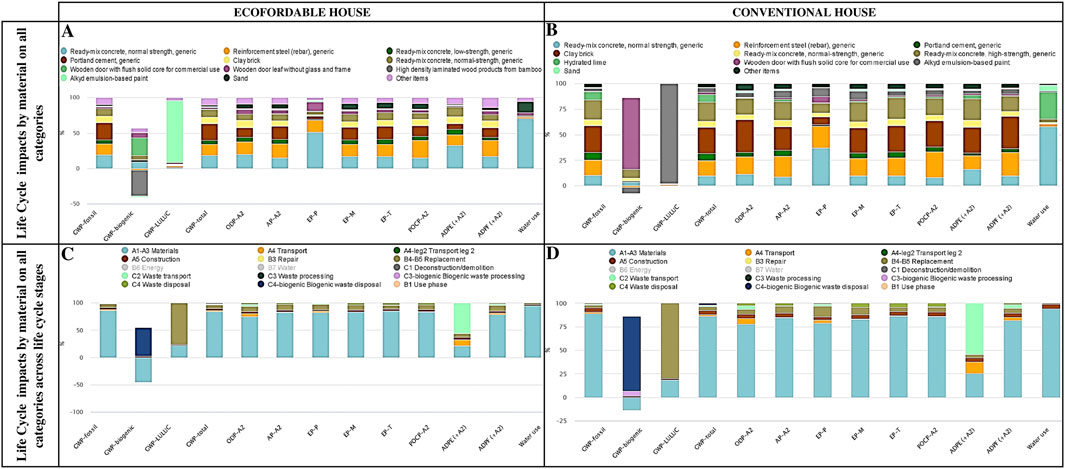
Figure 9. Life-cycle impact distribution by material and stage: (A) Impact contributions by material for the EH, (B) impact contributions by material for the CH, (C) impact contributions by life-cycle stage for the EH, and (D) impact contributions by life-cycle stage for the CH.
3.4 Final comparison between the EH and the CH
The comparative analysis of the EH and CH (Figure 10) highlights the environmental advantages of using hybrid and sustainable construction methods and materials. The EH contributes to a total GWP of 36,816.86 kg CO2e, approximately 47% less than the CH, which has a total GWP of 69,822.00 kg CO2e. Remarkably, the difference is due to the EH using ICSEB for masonry walls (interlocking, hollow, and partially reinforced), roofs constructed with jack arches and funicular shells, and doors and windows made from date palm midribs instead of fired brick walls, RC flat slabs, and commercial wood, respectively, in the construction of the CH.
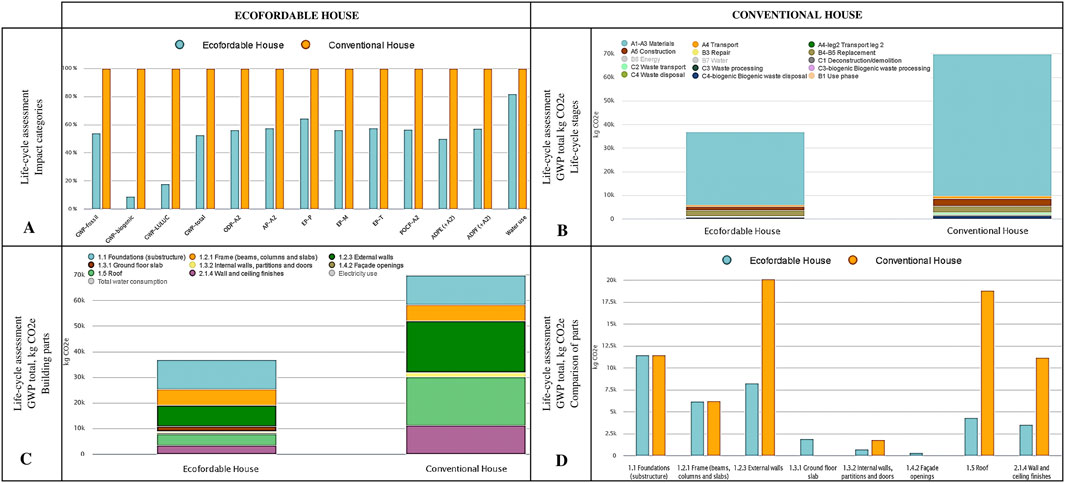
Figure 10. Final comparison between the Ecofordable and Conventional Houses: (A) LCA impact categories, (B) GWP-total across LCA stages, (C) GWP-total by building parts, and (D) side-by-side comparison of GWP-Total by building parts.
Stages A1–A3 dominate the total GWP in both designs, while that of the EH is very low because of dependence on low-carbon materials like earth bricks and renewable palm midribs as well as a lower mass of conventional materials. In contrast, the extensive use of RC and fired clay bricks inflates the material-related emissions for CH.
Both houses present the same GWP contribution of foundation parts; however, the EH presents a higher proportion, 31.2%, due to the smaller overall GWP, although the absolute emissions are the same as CH. External walls made of fired clay bricks have a 20,144.28 kg CO2e GWP, contributing 28.9%, while this is reduced to 8,227.13 kg CO2e for the partially reinforced, interlocking earth brick walls, contributing 22.4% and with a reduction of 59.2%. The application of hybrid, small-span curved masonry roofing systems supported on RC beams reduces roof emissions by more than 77% compared to RC flat slabs. The GWP for the wall and ceiling finishes was reduced by 68.6%.
The EH has a 18% reduction in water use over that of the CH because of the low embodied water in earth bricks compared with fired bricks. The CH has a significantly higher consumption of depletion-fossil fuel energy, totaling 543,095.29 MJ, compared to the EH, which consumes 310,703.47 MJ. This represents a 42.8% reduction in fossil fuel energy consumption for the EH relative to the CH as a result of energy-intensive procedures in the making of RC and fired bricks. The CH is higher in acidification and eutrophication potentials due to the high emission from manufacturing materials and transportation.
4 Conclusion
The Ecofordable House is an experimental residential prototype, totally constructed at the MSA University Campus in Cairo, and represents a hybrid construction system, mixing vernacular techniques with modern technologies. Its life-cycle assessment was compared to the Conventional House, which serves as a benchmark in this work, based on standard reference data from typical RC-framed houses in Egypt.
The integration of cement, concrete, steel, and fired bricks in EH technologies reduced its GWP by 47.3%, fossil-based emissions by 46%, biogenic emissions by 91.1%, and LULUC emissions by 82.4%. The acidification potential is reduced by 42.5%, Eutrophication impacts range between a reduction of 28% to a reduction of 44%, and POCP is reduced by 43.3%. The EH also achieves more efficient use of resources, with reductions of 42.8% in fossil fuel use and 18% in water consumption. The main impacts come from A1–A3 material production stages with reinforced ICSEBs, hybrid funicular shells and Jack-arch roofs, and date palm midribs providing sustainable alternatives to the total reliance on reinforced concrete, fired clay brick materials, and commercial imported wood.
This study demonstrates that hybrid construction systems that integrate modern materials can significantly reduce a building’s environmental footprint without compromising its structural durability and affordability (investigated in earlier studies). It has emphasized a pathway to enhanced vernacular technologies that could contribute toward meeting sustainable construction needs, specifically in regions where climatic and resource conditions demand innovative eco-friendly solutions. While this project focuses on Egypt’s arid-hot climate, its application is transferable to other regions in which material needs and climatic demands necessitate ecological innovations. The hybrid approach is applicable according to local construction traditions, material availability, and structural demands. Being extensively investigated structurally, thermally, and acoustically, the combined solutions adopted in the EH can be applied in different climatic conditions.
Data availability statement
The original contributions presented in the study are included in the article/Supplementary Material, further inquiries can be directed to the corresponding author.
Author contributions
NA: conceptualization, funding acquisition, investigation, project administration, software, writing – original draft, and writing – review and editing.
Funding
The author(s) declare that financial support was received for the research and/or publication of this article. The construction of the experimental residential unit “Ecofordable House” was funded by Dr. Nawal El-Degwi, MSA University head of the board of trustees.
Conflict of interest
The author declares that the research was conducted in the absence of any commercial or financial relationships that could be construed as a potential conflict of interest.
Generative AI statement
The author(s) declare that no Generative AI was used in the creation of this manuscript.
Publisher’s note
All claims expressed in this article are solely those of the authors and do not necessarily represent those of their affiliated organizations, or those of the publisher, the editors and the reviewers. Any product that may be evaluated in this article, or claim that may be made by its manufacturer, is not guaranteed or endorsed by the publisher.
Supplementary material
The Supplementary Material for this article can be found online at: https://www.frontiersin.org/articles/10.3389/fbuil.2025.1568067/full#supplementary-material
References
Abdel, G. M. N., and Abo Eldardaa, M. I. (2023). Cost-effectiveness and affordability evaluation of a residential prototype built with compressed earth bricks, hybrid roofs and palm midribs. Front. Built Environ. 9, 1058782. doi:10.3389/fbuil.2023.1058782
Abdel Gelil, M. N., Moustafa, A., and Darwish, E. A. (2024). Structural, acoustical, and thermal evaluation of an experimental house built with reinforced/hollow interlocking compressed stabilized earth brick-masonry. J. Build. Eng. 86, 108790. doi:10.1016/j.jobe.2024.108790
Alsaid, A. M., Hegazi, Y. S., Shalaby, H. A., and Ahmed, M. A. (2023). Methodology to improve energy efficiency of heritage buildings using HBIM-sabil qaitbay: a case study from Egypt. Civ. Eng. Archit. 11 (1), 425–449. doi:10.13189/cea.2023.110134
Aly, H., and Abdelaziz, O. (2024). Sustainable design trends in the built-environment globally and in Egypt: a literature review. Sustainability 16 (12), 4980. doi:10.3390/su16124980
Anand, C. K., and Amor, B. (2017). Recent developments, future challenges and new research directions in LCA of buildings: a critical review. Renew. Sustain. Energy Rev. 67, 408–416. doi:10.1016/j.rser.2016.09.058
Arduin, D., Caldas, L. R., Paiva, R. D. L. M., and Rocha, F. (2022). Life cycle assessment (LCA) in earth construction: a systematic literature review considering five construction techniques. Sustainability 14 (20), 13228. doi:10.3390/su142013228
Ben-Alon, L., Loftness, V., Harries, K. A., and Cochran Hameen, E. (2021). Life cycle assessment (LCA) of natural vs conventional building assemblies. Renew. Sustain. Energy Rev. 144, 110951. doi:10.1016/j.rser.2021.110951
Burdova, E. K., Budajova, J., Mesaros, P., and Vilcekova, S. (2023). Potential for reducing the carbon footprint of buildings. Eng. Proc. 57 (1), 6. doi:10.3390/engproc2023057006
Cabeza, L. F., Rincón, L., Vilariño, V., Pérez, G., and Castell, A. (2014). Life cycle assessment (LCA) and life cycle energy analysis (LCEA) of buildings and the building sector: a review. Renew. Sustain. Energy Rev. 29, 394–416. doi:10.1016/j.rser.2013.08.037
Carrobé, A., Castell, A., and Martorell, I. (2024). Life cycle assessment comparison between an earthbag building and a conventional sahrawi cement blocks building. Materials 17 (5), 1011. doi:10.3390/ma17051011
Chen, L., Hu, Y., Wang, R., Li, X., Chen, Z., Hua, J., et al. (2024). Green building practices to integrate renewable energy in the construction sector: a review. Environ. Chem. Lett. 22 (2), 751–784. doi:10.1007/s10311-023-01675-2
Dalla Mora, T., Bolzonello, E., Cavalliere, C., and Peron, F. (2020). Key parameters featuring BIM-LCA integration in buildings: a practical review of the current trends. Sustainability 12 (17), 7182. doi:10.3390/su12177182
Dormohamadi, M., Rahimnia, R., and Bunster, V. (2024). Life cycle assessment and life cycle cost analysis of different walling materials with an environmental approach (comparison between earth-based vs. conventional construction techniques in Iran). Int. J. Life Cycle Assess. 29 (3), 355–379. doi:10.1007/s11367-023-02259-6
Dreyfus, G. B., Montzka, S. A., Andersen, S. O., and Ferris, R. (2024). Technical note: a method for calculating offsets to ozone depletion and climate impacts of ozone-depleting substances. Atmos. Chem. Phys. 24 (3), 2023–2032. doi:10.5194/acp-24-2023-2024
Dsilva, J., Zarmukhambetova, S., and Locke, J. (2023). Assessment of building materials in the construction sector: a case study using life cycle assessment approach to achieve the circular economy. Heliyon 9 (10), e20404. doi:10.1016/j.heliyon.2023.e20404
Egyptian Code Committee (2001a). ECP 204-2005, Egyptian code for the design and construction of masonry structures. Cairo, Egypt: HBRC.
Egyptian Code Committee (2001b). EG-SE2016, Building with stabilized earth – part one – 2016. Cairo, Egypt HBRC.
ElGohary, A. S., and Khashaba, S. O. (2018). The challenge of greening the existing residential buildings in the Egyptian market base case. ARCHive-SR 2 (3), 136–152. doi:10.21625/archive.v2i3.355
El-Mously, H. (2018). Innovating green products as a mean to alleviate poverty in Upper Egypt. Ain Shams Eng. J. 9 (4), 2039–2056. doi:10.1016/j.asej.2017.02.001
Elshorbany, Y., Ziemke, J. R., Strode, S., Petetin, H., Miyazaki, K., De Smedt, I., et al. (2024). Tropospheric ozone precursors: global and regional distributions, trends, and variability. Atmos. Chem. Phys. 24 (21), 12225–12257. doi:10.5194/acp-24-12225-2024
Eštoková, A., Wolfová Fabiánová, M., and Ondová, M. (2022). Concrete structures and their impacts on climate change and water and raw material resource depletion. Int. J. Civ. Eng. 20 (6), 735–747. doi:10.1007/s40999-022-00701-8
European Committee for Standardization (2021). “Sustainability of construction works,” in Environmental product declarations. Core rules for the product category of construction products. EN 15804:2012+A2:2019. Available online at: https://www.en-standard.eu/bs-en-15804-2012-a2-2019-sustainability-of-construction-works-environmental-product-declarations-core-rules-for-the-product-category-of-construction-products/.
Fernandes, J., Peixoto, M., Mateus, R., and Gervásio, H. (2019). Life cycle analysis of environmental impacts of earthen materials in the Portuguese context: rammed earth and compressed earth blocks. J. Clean. Prod. 241, 118286. doi:10.1016/j.jclepro.2019.118286
Fnais, A., Rezgui, Y., Petri, I., Beach, T., Yeung, J., Ghoroghi, A., et al. (2022). The application of life cycle assessment in buildings: challenges, and directions for future research. Int. J. Life Cycle Assess. 27 (5), 627–654. doi:10.1007/s11367-022-02058-5
Fouly, S. E.-d. A., and Abdin, A. R. (2022). A methodology towards delivery of net zero carbon building in hot arid climate with reference to low residential buildings — the western desert in Egypt. J. Eng. Appl. Sci. 69 (1), 46. doi:10.1186/s44147-022-00084-6
Gado, T., Mohamed, M., and Osman, M. (2010). Investigating the intelligence of the low-tech earth architecture of the Sahara: a feasibility study from the western desert of Egypt. Intell. Build. Int. 2 (3), 179–197. doi:10.3763/inbi.2010.0038
Gazquez, L. A. M., Hernández, F. F., and López, J. M. C. (2022). A comparison of traditional and contemporary social houses in catarmarca (Argentina). Comfort conditions and life cycle assessment. Sustain. Cities Soc. 82, 103891. doi:10.1016/j.scs.2022.103891
Head, M., Magnan, M., Kurz, W. A., Levasseur, A., Beauregard, R., and Margni, M. (2021). Temporally-differentiated biogenic carbon accounting of wood building product life cycles. SN Appl. Sci. 3 (1), 62. doi:10.1007/s42452-020-03979-2
Hollberg, A., Genova, G., and Habert, G. (2019). Evaluation of parameter uncertainty in environmental impact assessment of buildings. IOP Conf. Ser. Earth Environ. Sci. Paper presented at the. doi:10.1088/1755-1315/323/1/012028
Hu, M., and Esram, N. W. (2021). The status of embodied carbon in building practice and research in the United States: a systematic investigation. Sustainability 13 (23), 12961. doi:10.3390/su132312961
International Organization for Standardization (2020a). Environmental management - life cycle assessment - principles and framework - amendment 1 (ISO 14040:2006/Amd 1:2020). Available online at: https://www.iso.org/standard/76121.html#lifecycle.
International Organization for Standardization (2020b). Environmental management — life cycle assessment — requirements and guidelines - amendment 2. ISO 14044:2006/Amd 2:2020. Available online at: https://www.iso.org/standard/76122.html.
Jagatramka, R., Kumar, A., and Pipralia, S. (2021). Transformations of vernacular architecture of India: problems and prospects. ISVS E-Journal 8 (1), 23–32.
Jain, A. K. (2000). Slum rehabilitation and housing the poor. Soc. Change 30 (1-2), 139–152. doi:10.1177/004908570003000210
Jayawardana, J., Sandanayake, M., Jayasinghe, J. A. S. C., Kulatunga, A. K., and Zhang, G. (2023). A comparative life cycle assessment of prefabricated and traditional construction – a case of a developing country. J. Build. Eng. 72, 106550. doi:10.1016/j.jobe.2023.106550
Jungclaus, M. A., Grant, N., Torres, M. I., Arehart, J. H., and Srubar, W. V. (2024). Embodied carbon benchmarks of single-family residential buildings in the United States. Sustain. Cities Soc. 117, 105975. doi:10.1016/j.scs.2024.105975
Kaoula, D., Rahmani, S., Lemita, I., Ould Zemirli, M. A., and Semahi, S. (2022). Life cycle assessment of traditional Saharan houses: towards a sustainable building? J. Build. Eng. 57, 104782. doi:10.1016/j.jobe.2022.104782
Keswani, K. (1997). The contribution of building centres to low-cost housing in India. Build. Res. and Inf. 25 (1), 50–64. doi:10.1080/096132197370606
Khan, A., Arif, S., Nadeem, O., and Anwer, A. (2013). Jack Arch: the backbone of British colonial residential buildings in India. Pak. J. Sci. 65 (3), 352–356.
Kumar, V. D. S., and Maheswari, A. U. (2019). Analytical study of funicular and flat slabs. Int. J. Sci. Technol. and Eng. 5 (8), 46–50.
Leinonen, I. (2022). A general framework for including biogenic carbon emissions and removals in the life cycle assessments for forestry products. Int. J. Life Cycle Assess. 27 (8), 1038–1043. doi:10.1007/s11367-022-02086-1
Leo Samuel, D. G., Dharmasastha, K., Shiva Nagendra, S. M., and Maiya, M. P. (2017). Thermal comfort in traditional buildings composed of local and modern construction materials. Int. J. Sustain. Built Environ. 6 (2), 463–475. doi:10.1016/j.ijsbe.2017.08.001
Maheri, M. R., and Rahmani, H. (2003). Static and seismic design of one-way and two-way jack arch masonry slabs. Eng. Struct. 25 (13), 1639–1654. doi:10.1016/S0141-0296(03)00143-3
Marsh, A. T. M., and Kulshreshtha, Y. (2022). The state of earthen housing worldwide: how development affects attitudes and adoption. Build. Res. and Inf. 50 (5), 485–501. doi:10.1080/09613218.2021.1953369
M. Midani, N. Saba, and O. Y. Alothman (2020). Date palm fiber composites (Singapore: Springer). doi:10.1007/978-981-15-9339-0
Mohamed, A. S. Y. (2022). “Building technology sustainablism—new vision,” in Paper presented at the proceedings of 2021 4th international conference on civil engineering and architecture (Singapore). doi:10.1007/978-981-16-6932-3_30
Mohamed, M. (2014). Lessons from the past to enhance the environmental performance of primary school classrooms in Egypt. Environ. Ecol. Res. 2 (6), 221–233. doi:10.13189/eer.2014.020601
Moncaster, A. M., and Symons, K. E. (2013). A method and tool for ‘cradle to grave’ embodied carbon and energy impacts of UK buildings in compliance with the new TC350 standards. Energy Build. 66, 514–523. doi:10.1016/j.enbuild.2013.07.046
Nguyen, A. T., Truong, N. S. H., Rockwood, D., and Tran Le, A. D. (2019). Studies on sustainable features of vernacular architecture in different regions across the world: a comprehensive synthesis and evaluation. Front. Archit. Res. 8 (4), 535–548. doi:10.1016/j.foar.2019.07.006
Nouri, H., Safehian, M., and Mir Mohammad Hosseini, S. M. (2023). Life cycle assessment of earthen materials for low-cost housing a comparison between rammed earth and fired clay bricks. Int. J. Build. Pathology Adapt. 41 (2), 364–377. doi:10.1108/IJBPA-02-2021-0021
Nwodo, M. N., and Anumba, C. J. (2019). A review of life cycle assessment of buildings using a systematic approach. Build. Environ. 162, 106290. doi:10.1016/j.buildenv.2019.106290
Ogunmakinde, O. E. (2024). The circular economy in the construction industry: from research to practice. Circ. Econ. 2 (3). doi:10.55845/DHNN3429
Ortiz, O., Castells, F., and Sonnemann, G. (2009). Sustainability in the construction industry: a review of recent developments based on LCA. Constr. Build. Mater. 23 (1), 28–39. doi:10.1016/j.conbuildmat.2007.11.012
Payen, S., Cosme, N., and Elliott, A. H. (2021). Freshwater eutrophication: spatially explicit fate factors for nitrogen and phosphorus emissions at the global scale. Int. J. Life Cycle Assess. 26 (2), 388–401. doi:10.1007/s11367-020-01847-0
Pscherer, T., and Krommes, S. (2024). LCA standards for environmental product assessments in the bioeconomy with a focus on biogenic carbon: a systematic review. Int. J. Life Cycle Assess. 30, 371–393. doi:10.1007/s11367-024-02387-7
Rinne, R., Ilgın, H. E., and Karjalainen, M. (2022). Comparative study on life-cycle assessment and carbon footprint of hybrid, concrete and timber apartment buildings in Finland. Int. J. Environ. Res. Public Health 19 (2), 774. doi:10.3390/ijerph19020774
Salman, M. (2019). “Sustainability and vernacular architecture: rethinking what identity is,” in Urban and architectural heritage Conservation within sustainability: IntechOpen. doi:10.5772/intechopen.82025
Sandanayake, M. S. (2022). Environmental impacts of construction in building industry—a review of knowledge advances, gaps and future directions. Knowledge 2 (1), 139–156. doi:10.3390/knowledge2010008
Sartori, T., Drogemuller, R., Omrani, S., and Lamari, F. (2021). A schematic framework for life cycle assessment (LCA) and green building rating system (GBRS). J. Build. Eng. 38, 102180. doi:10.1016/j.jobe.2021.102180
Seppälä, J., Posch, M., Johansson, M., and Hettelingh, J.-P. (2006). Country-dependent characterisation factors for acidification and terrestrial eutrophication based on accumulated exceedance as an impact category indicator (14 pp). Int. J. Life Cycle Assess. 11 (6), 403–416. doi:10.1065/lca2005.06.215
Tan, Y., Shuai, C., Shen, L., Hou, L., and Zhang, G. (2020). A study of sustainable practices in the sustainability leadership of international contractors. Sustain. Dev. 28 (4), 697–710. doi:10.1002/sd.2020
Tawayha, F. A., Braganca, L., and Mateus, R. (2019). Contribution of the vernacular architecture to the sustainability: a comparative study between the contemporary areas and the old quarter of a mediterranean city. Sustainability 11 (3), 896. doi:10.3390/su11030896
Thormark, C. (2006). The effect of material choice on the total energy need and recycling potential of a building. Build. Environ. 41 (8), 1019–1026. doi:10.1016/j.buildenv.2005.04.026
Un-Habitat (2009). Interlocking stabilised soil blocks: appropriate earth technologies in Uganda. Un-Habitat. Uganda.
Un-Habitat (2012). Going green: a handbook of sustainable housing practices in developing countries. Nairobi: United Nations Human Settlement Programme.
Van Oers, L., and Guinée, J. (2016). The abiotic depletion potential: background, updates, and future. Resources 5 (1), 16. doi:10.3390/resources5010016
van Oers, L., Guinée, J. B., and Heijungs, R. (2020). Abiotic resource depletion potentials (ADPs) for elements revisited—updating ultimate reserve estimates and introducing time series for production data. Int. J. Life Cycle Assess. 25 (2), 294–308. doi:10.1007/s11367-019-01683-x
Vea, E. B., Jwaideh, M., Richardson, K., Ryberg, M., Bjørn, A., and Hauschild, M. (2024). Enabling comprehensive assessment of marine eutrophication impacts and their evaluation against regional safe operating space. Int. J. Life Cycle Assess. 29 (9), 1738–1755. doi:10.1007/s11367-024-02311-z
Venkatarama Reddy, B. V. (2009). Sustainable materials for low carbon buildings. Int. J. Low-Carbon Technol. 4 (3), 175–181. doi:10.1093/ijlct/ctp025
Zhang, S., Ma, M., Zhou, N., and Yan, J. (2024). GLOBUS: global building renovation potential by 2070. doi:10.48550/ARXIV.2406.04133
Zhong, X., Hu, M., Deetman, S., Steubing, B., Lin, H. X., Hernandez, G. A., et al. (2021). Global greenhouse gas emissions from residential and commercial building materials and mitigation strategies to 2060. Nat. Commun. 12 (1), 6126. doi:10.1038/s41467-021-26212-z
Keywords: life cycle assessment and life cycle impact assessment, sustainable construction, hybrid construction systems, global warming potential, house prototype, jack arch and funicular shell roof, date palm leaves midribs, interlocking compressed stabilized earth bricks
Citation: Abdel Gelil Mohamed N (2025) Life-cycle assessment of hybrid vernacular-modern technologies: a comparative study of the ecofordable house and conventional RC structures. Front. Built Environ. 11:1568067. doi: 10.3389/fbuil.2025.1568067
Received: 28 January 2025; Accepted: 10 March 2025;
Published: 08 May 2025.
Edited by:
Castorina Silva Vieira, University of Porto, PortugalReviewed by:
Roberto Alonso González-Lezcano, CEU San Pablo University, SpainDeborah Arduin, University of Aveiro, Portugal
Copyright © 2025 Abdel Gelil Mohamed. This is an open-access article distributed under the terms of the Creative Commons Attribution License (CC BY). The use, distribution or reproduction in other forums is permitted, provided the original author(s) and the copyright owner(s) are credited and that the original publication in this journal is cited, in accordance with accepted academic practice. No use, distribution or reproduction is permitted which does not comply with these terms.
*Correspondence: Nermine Abdel Gelil Mohamed, bmVhYmRlbGhhbGltQGVmZmF0dW5pdmVyc2l0eS5lZHUuc2E=