Temporal attention
- Evolutionary Cognition, Department of Psychology, University of Tübingen, Tübingen, Germany
Attention, that is, the ability to focus processing resources on a specific part of sensory input, is often thought of as being mainly allocated in space, toward specific objects or modalities. However, attention can also be allocated within time. Temporal attention can be induced implicitly, that is, through learning of temporal regularities between at least two events, or explicitly, by the active instruction to attend to a specific time point. Moreover, temporal attention can be induced via external rhythmic stimulation. Many studies throughout the last 120 years have shown that, irrespective of the way temporal attention is induced, it facilitates processing of upcoming events. Based on early findings measuring reaction time, researchers initially assumed that temporal attention primarily accelerates motor processing. Within the last 20 years, however, more and more evidence has emerged supporting the view that temporal attention facilitates perceptual processing. Moreover, temporal attention may even interact with other attentional domains such as spatial attention or feature-based attention. In the present article, we summarize classical as well as current findings and theoretical accounts on how temporal attention affects perceptual processing. Furthermore, we sketch current challenges and open questions in research on temporal attention.
Time as attention domain
Attention has the basic function of focusing processing resources on a subset of sensory input (e.g., Johnston and Dark, 1986; Desimone and Duncan, 1995; Summerfield and Egner, 2009; Carrasco, 2011). It can be assumed that without that selective mechanism, humans would be overloaded by sensory input and would therefore be unable to interpret the world in a meaningful way or act in a goal-directed manner. A large body of experimental research has shown that attention can operate in different domains such as space, sensory modalities or sensory features (for reviews, see, e.g., Johnston and Dark, 1986; Carrasco, 2011; Klein and Lawrence, 2012), and on different levels of representations, ranging from low-level sensory representations such as orientation to higher-level object-like representations (e.g., Johnston and Dark, 1986; Chen, 2012). One of the most fundamental attention domains is that of time (see also Lawrence and Klein, 2013), though it has been much less studied than others. Allocating attention in time, typically referred to as temporal attention, can be broadly defined as the allocation of processing resources toward a specific time point (e.g., Lawrence and Klein, 2013; Nobre and Rohenkohl, 2014). This process was described as early as 1874 in pioneering work by Wundt as “vorbereitende Spannung der Aufmerksamkeit” (preparatory tension of attention) (Wundt, 1874, p. 737). This allocation of attention within time is typically based on some type of implicit or explicit temporal contingency between successive sensory stimuli, with one stimulus allowing anticipation of the subsequent one(s). Like the allocation of attention to locations in space, temporal attention leads to a processing benefit for stimuli presented at the attended moment in time (e.g., Lange et al., 2003; Correa et al., 2006a; Bausenhart et al., 2007; Rolke, 2008; Jepma et al., 2012; Rohenkohl et al., 2012).
Temporal attention's mechanisms and beneficial effects have already been summarized in several reviews published over the last decade (e.g., Nobre and Rohenkohl, 2014; Nobre and Van Ede, 2018). The goal of the present article is not to provide a fully comprehensive overview of all facets of temporal attention research;1 instead, we will focus on the effect(s) of temporal attention on stimulus processing. Herein, we will put particular emphasis on perceptual processing and the main lines of research in this subfield of temporal attention research: In the first two sections, we will provide a summary of the most common experimental paradigms as well as a recap of the history of temporal attention research. In doing so, we will also point out some of the methodological differences among experimental paradigms. In the third and the fourth sections, we will focus on the facilitatory effects of temporal attention on perceptual processing, and we will discuss both, studies examining effects of temporal attention within and across modalities and studies examining its interactions with other attention domains. In the fifth section, we will then summarize current theories on the mechanisms by which temporal attention specifically facilitates perceptual processing. We will conclude our review by outlining some unresolved issues and providing an outlook on possible future directions in temporal attention research.
Experimental paradigms in temporal attention
Several experimental approaches or paradigms have been developed throughout the history of temporal attention research (see Figures 1A–C; for an overview see Rolke and Ulrich, 2010; Lawrence and Klein, 2013; Nobre and Rohenkohl, 2014). Even though these paradigms differ in several aspects and may even involve distinct mechanisms, all of them rely on the (explicit or implicit) temporal contingency between two or more successive sensory stimuli. The common observation in all these paradigms is that the processing of a stimulus presented at a temporally attended moment in time is facilitated relative to the processing of a stimulus presented at a temporally unattended or less-attended moment in time (see Figure 1D).
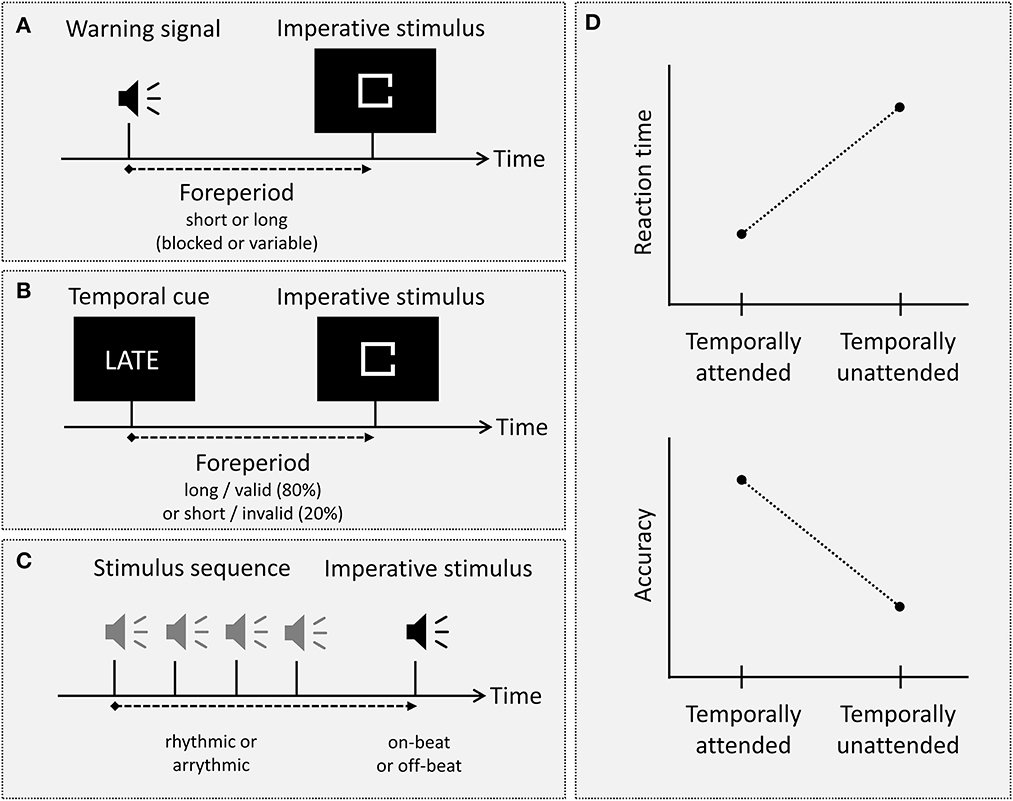
Figure 1. (A–C) Illustration of three major types of experimental paradigms in temporal attention research, that is, the foreperiod paradigm, probabilistic temporal cueing, and a typical rhythmic temporal attention paradigm. In the foreperiod paradigm (A), a warning signal precedes the imperative stimulus by either a (blocked or variable) short or long foreperiod. In probabilistic temporal cueing (B), an explicit temporal cue either validly or invalidly announces the occurrence of the imperative stimulus after a short or long foreperiod. In a typical rhythmic temporal attention paradigm (C), the imperative stimulus is preceded by a rhythmic (or an arrhythmic) stimulus sequence and/or is presented either in accordance with the rhythm (“on-beat”) or not (“off-beat”). (D) The typical beneficial effect of temporal attention, exemplified for the dependent variables reaction time and accuracy: Responses are faster and more accurate for temporally attended as compared to temporally unattended stimuli.
The oldest and most basic type of experimental paradigm in the study of temporal attention is the foreperiod paradigm: In this paradigm, a warning signal is presented before an imperative stimulus which typically requires some kind of overt response (typically a speeded keypress). The crucial manipulation is the length of the temporal interval between warning signal and imperative stimulus, which is called the foreperiod. In the blocked (or constant) foreperiod paradigm, the foreperiod remains constant within a block of trials but varies across blocks; in the variable foreperiod paradigm, the foreperiod varies randomly from trial to trial. In both variants, the effect of temporal attention on processing of the imperative stimulus is quantified as the difference between at least two different foreperiod lengths, that is a “short” foreperiod, which is often around or <1 s long, and a “long” foreperiod between 1 and 4 s (e.g., Rolke and Hofmann, 2007; Steinborn et al., 2010; for studies including wider foreperiod ranges see, e.g., Klemmer, 1956; Bertelson and Tisseyre, 1969; Müller-Gethmann et al., 2003), with reaction time (RT) to the imperative stimulus being the most common dependent variable. Interestingly, the result pattern observed when measuring RT differs strikingly between blocked and variable foreperiod paradigms (for a review, see, e.g., Niemi and Näätänen, 1981). In the blocked foreperiod paradigm, an increase in foreperiod length typically leads to an increase in RT, except for very short foreperiods between 50 and 150 ms (e.g., Klemmer, 1956; Karlin, 1959; Bertelson and Tisseyre, 1969; Müller-Gethmann et al., 2003). In contrast, in the variable foreperiod paradigm, RT typically decreases with increasing foreperiod length (e.g., Drazin, 1961; Los and Van den Heuvel, 2001; Los et al., 2001; Los and Heslenfeld, 2005; Steinborn et al., 2008; see also Niemi and Näätänen, 1981). Furthermore, this descending foreperiod-RT function in the variable foreperiod paradigm is qualified by sequential effects (e.g., Los and Van den Heuvel, 2001; Los et al., 2001; Van der Lubbe et al., 2004; Steinborn et al., 2008; for a review see, e.g., Los, 2010). Specifically, when analyzing the variable foreperiod effect not only as a function of the current foreperiod N, but also as a function of the foreperiod in the previous trial N−1, it has been shown that RT for a short-foreperiod trial N is longer if the foreperiod in trial N−1 was long than if it was short (e.g., Los et al., 2001; see also Los et al., 2014). Furthermore, it has been shown that the slope of the foreperiod-RT function in the variable foreperiod paradigm depends on the type of foreperiod distribution: Specifically, a descending foreperiod-RT function is typically observed if foreperiods are sampled with equal probability (so-called aging foreperiod distribution). In contrast, if the probability of short foreperiods is increased (so-called non-aging foreperiod distribution) so that the subjective overall probability of the different foreperiods is equalized, the foreperiod effect on RT is strongly attenuated or even eliminated (e.g., Baumeister and Joubert, 1969; Nickerson and Burnham, 1969; Näätänen, 1971).
The discrepancy in results across blocked and variable foreperiod paradigm, that is, an ascending as opposed to a descending foreperiod-RT function, has been attributed to different underlying mechanisms: Specifically, the RT increase in the blocked foreperiod paradigm is assumed to be the consequence of an imperfect time-keeping ability (Treisman, 1964; Gottsdanker, 1975; Näätänen and Merisalo, 1977) in the sense that the estimation of longer foreperiods tends to be less accurate (Treisman, 1964; Gibbon, 1977). Therefore, even though the temporal contingency between warning signal and imperative stimulus remains constant in each block of trials, long foreperiod blocks nonetheless come with a higher degree of temporal uncertainty because the exact moment in time the imperative stimulus will be presented can be estimated less accurately. This results in less efficient focusing attention to that moment in time in blocks with a (rather) long foreperiod than in blocks with a (rather) short foreperiod.2 In the variable foreperiod paradigm, participants experience a different type of temporal uncertainty because of the foreperiod's variation from trial to trial (see also Klemmer, 1956). Here, the exact moment of occurrence of an imperative stimulus in a current trial is uncertain because it cannot be predicted directly on grounds of the previous trial. Nonetheless, the temporal onset of the imperative stimulus can still be anticipated using the passage of time during the foreperiod. Specifically, the more time that has elapsed since the presentation of the warning signal without the imperative stimulus being presented, the higher the probability that the imperative stimulus will be presented at the next moment in time (also referred to as hazard function). As a result of this increasing stimulus occurrence probability, more and more resources will be allocated toward the next possible moment in time, leading to faster responses with increasing foreperiod length (see also Niemi and Näätänen, 1981). Although the hazard function can account for the classic variable foreperiod effect, it does not provide a straightforward explanation for the above-described sequential effect because it is agnostic to the contribution of previous trials to the foreperiod effect. Consequently, some authors have advocated the ideas that different, and potentially independent, mechanisms might be involved in the two effects. For instance, in a developmental study, Vallesi and Shallice (2007) showed that sequential effects were present in participants of a young age (4–5 years old), whereas the variable foreperiod effect itself appeared some years later. Moreover, Mento and Tarantino (2015) reported that children were able to combine different hints to focus attention in the variable foreperiod paradigm only at an age above 8 years. These studies suggest that the sequential effect—in contrast to other temporal attention mechanisms—might reflect rather implicit or automatic mechanisms, which might be available at an early age. Further support for this assumption came from electrophysiological studies (e.g., Mento, 2017) and studies comparing temporal attention effects between healthy and atypical populations (e.g., Mento et al., 2019). In contrast to a dual-process account, other authors have advocated the idea of a single mechanism accounting for the classic variable foreperiod effect and the sequential effect. For instance, Los and Van den Heuvel (2001) assumed that both effects are caused by trace conditioning mechanisms (see also Los and Heslenfeld, 2005). Furthermore, Los et al. (2014, 2017); see also Mattiesing et al., 2017) more recently proposed that both effects are signatures of memory traces stored in long-term memory, which are formed and continuously updated on grounds of past experiences. Importantly, and irrespective of the difference in the assumed mechanisms within the variable foreperiod paradigm and the difference between variable and blocked foreperiod paradigms, both paradigms share the common feature that they entail what might be called a “gradual” manipulation of temporal attention: Due to the temporal contingency between warning signal and imperative stimulus, the presentation of the warning signal initiates an intentional (or unintentional) process of resource allocation toward the temporal onset of the imperative stimulus, but the degree of this allocation will depend on the precision of the temporal estimation process as well as the degree of temporal uncertainty.
A second type of paradigm temporal attention research, which has become increasingly popular over the last 20 years, is the temporal orienting paradigm. In this type of paradigm, participants are provided with explicit information about which foreperiod to expect or which interval to attend. The most widely used variant is the (probabilistic) temporal cueing paradigm, which can be characterized as a temporal analog of the spatial cueing paradigm (e.g., Posner et al., 1973). In this paradigm, a temporal cue provides explicit information about when to expect the imperative stimulus. Like in the spatial cueing paradigm, the crucial manipulation in temporal cueing paradigms is the validity of the cue: Specifically, the temporal information provided by the cue is correct (valid) in about 75–80% of the trials, whereas it is incorrect (invalid) in the remaining trials. Furthermore, in some studies, valid temporal cues have been contrasted against neutral cues, that is, cues that do not indicate a specific foreperiod (e.g., Coull et al., 2016; Korolczuk et al., 2018). For instance, Coull et al. (2016) compared blocks in which the cue indicated one of four foreperiods with 100% validity with blocks in which the cue did not provide any information about the foreperiod. Typically, the temporal information provided by the cue is symbolic (e.g., one of two colors or forms, with one indicating a short and the other one indicating a long foreperiod; Miniussi et al., 1999; Jepma et al., 2012; Rohenkohl et al., 2014), but verbal information has also been used (e.g., Correa et al., 2006a,b). Furthermore, the cued temporal information (i.e., whether the cue indicates a short or a long foreperiod) can been presented in a way that the information changes from trial to trial (e.g., Miniussi et al., 1999; Correa et al., 2004) or remains constant for a given block of trials (e.g., Correa et al., 2004, Experiment 2; Correa et al., 2006a). Using a slightly different approach, some researchers have employed a temporal Hillyard paradigm (e.g., Lange et al., 2003; Lange, 2009; see also Lange and Röder, 2010), which, like temporal cueing, can be characterized as an analog to selective attention paradigms being used in other attentional domains (e.g., Hillyard et al., 1973). In this paradigm, participants are presented with either a short or long temporal interval, each being marked by an onset and offset marker. In separate blocks, participants are instructed to attend to offset markers following one temporal interval (e.g., only the short interval), and to respond to those offset markers that deviate in a basic feature (e.g., their pitch) from the other offset markers. Accordingly, the critical manipulation in this paradigm is not stimulus occurrence probability (i.e., the probability that the imperative stimulus will appear after a short or long foreperiod), but the task-relevance of a specific time point (i.e., whether the offset marker is presented at the to-be-attended time point or at an unattended time point).
Like the foreperiod paradigms, temporal orienting paradigms typically lead to a processing benefit: For instance, RT to imperative stimuli following valid temporal cues is faster than RT to imperative stimuli following invalid temporal cues (e.g., Correa et al., 2010).3 This temporal cueing effect has been observed irrespective of whether the cued temporal information varies from trial to trial (e.g., Griffin et al., 2002; Correa et al., 2004, Experiment 2) or remains constant within a block (e.g., Correa et al., 2004; Experiment 2; Correa et al., 2006a), although there is some evidence that block-wise cueing effects might be larger for some tasks (Correa et al., 2004). Similarly, in the Hillyard paradigm, a processing benefit is observed for stimuli presented at the to-be-attended time point relative to those presented at an unattended time point (e.g., Lange et al., 2003). One crucial difference between temporal orienting paradigms and foreperiod paradigms is that in the former paradigms an imperative stimulus can occur at an unattended moment in time, either because participants attend to a different interval or because it is presented earlier than indicated by the temporal cue; in contrast, in the latter paradigms a rather gradual allocation of attention can be assumed and attention is not explicitly oriented away from any time point. Furthermore, probabilistic temporal cueing differs from foreperiod paradigms as explicit temporal information is conveyed by the cue, whereas in the foreperiod paradigms the temporal information is conveyed rather implicitly, that is, through the temporal regularity that exists between the warning signal and the imperative signal.
Another experimental paradigm that has become increasingly popular over the last few years is the rhythmic temporal attention paradigm. The key principle of this paradigm is to present a rhythmic sequence of signals rather than a single warning signal (or cue) before an imperative stimulus. The imperative stimulus is then presented in temporal accordance with the rhythm (“on-beat”) or at a different time point (“off-beat”). Furthermore, depending on the specific study, it is either presented as part of the rhythmic sequence (e.g., Lange, 2009; Rohenkohl et al., 2012) or at its end, with an additional variable foreperiod being interspersed between the rhythmic sequence and the imperative stimulus (e.g., Sanabria et al., 2011; Triviño et al., 2011; Breska and Deouell, 2014, 2017). In this type of paradigm, it has been shown that processing of stimuli presented in accordance with the rhythm is facilitated (e.g., Sanabria et al., 2011; Bolger et al., 2013; but see Elbaz and Yeshurun, 2020). Like temporal orienting paradigms, rhythmic temporal attention paradigms thus entail a comparison of conditions in which an imperative is either presented at an attended moment in time or at an unattended moment in time. However, deviating from all other temporal attention paradigms, the anticipatory process that drives attention toward a specific time point is not based on a discrete preceding event (a cue or warning signal), but on a repetitive sequence of stimuli. Consequently, rhythmic paradigms have been given a special status in temporal attention research, and some studies suggest that they may involve mechanisms that are distinct from discrete temporal attention paradigms such as the foreperiod or the temporal cueing paradigm (e.g., Capizzi et al., 2012; De la Rosa et al., 2012; Lakatos et al., 2013; Samaha et al., 2015; but see Correa and Nobre, 2008, on potentially shared mechanisms; see also Breska and Deouell, 2017).
Finally, although not in the focus of the present overview, there are paradigms in which temporal information is not provided in isolation but is correlated with stimulus-specific information. This specific temporal expectancy (Thomaschke and Dreisbach, 2013), also referred to as time-based event-related expectation (e.g., Ball et al., 2022) is typically studied using a variant of the variable foreperiod paradigm. In this variant, each foreperiod is correlated with a specific type of stimulus (or response) so that the foreperiod predicts not only the temporal onset of a stimulus but also what kind of stimulus will be presented or which response it will require (e.g., Wagener and Hoffmann, 2010; Thomaschke and Dreisbach, 2015; Thomaschke et al., 2018; Ball et al., 2022). The typical result is that performance is better for frequent time-stimulus combinations than for infrequent ones (for an overview see Thomaschke and Dreisbach, 2015).
In sum, different experimental paradigms have been developed to investigate the influence of temporal attention on stimulus processing, and these paradigms may also come along with different processing requirements and effects on the processing of temporally attended stimuli. The next sections provide an overview of the results obtained in temporal attention research.
A recap of the history: From motor preparation to temporal attention
The experimental investigation of temporal attention dates back to the end of the 19th century and the beginning of the 20th century, when experimental psychologists first investigated how the presentation of a warning signal influences responses to a subsequent imperative stimulus (e.g., Wundt, 1874; Woodrow, 1914; Klemmer, 1956; Bevan et al., 1965; for an excellent summary of these early studies see Niemi and Näätänen, 1981). For instance, in a seminal series of experiments, Wundt (1874) observed that the time needed to report the impact of a falling bullet was substantially reduced when the impact was preceded by a warning signal and that this effect was larger for larger drop heights. Following this first observation, various studies showed that properties of the foreperiod such as its length, the employed range and its variability across trials affect performance in response to the imperative stimulus. Thereby, these studies demonstrated the important role that temporal uncertainty plays in the allocation of temporal attention (for a summary see Niemi and Näätänen, 1981).
Apart from attempts to further characterize the factors that influence (the size of) the foreperiod effect, many subsequent studies focused on the theoretically important question of which mental processes within the stimulus-response processing chain are affected by variations in the foreperiod length, and temporal attention in general (for overviews see, e.g., Hackley and Valle-Inclán, 2003; Müller-Gethmann et al., 2003; Rolke and Ulrich, 2010; see also Correa, 2010). Originally, the predominant view was that variations in foreperiod length affect relatively late mental processes such as response preparation and response execution (e.g., Näätänen, 1971; Sanders, 1980; see also Teichner, 1954)—a view that was tightly linked to the idea that a warning signal mainly reduces the time needed for the execution of an action but does not influence stimulus processing itself. This idea was, for instance, advocated by Näätänen (1971) in the so-called motor readiness model. Some evidence for this motoric view comes from studies including measures of motor processes such as response force, which has been shown to be sensitive to foreperiod length (e.g., Mattes and Ulrich, 1997). Furthermore, indirect support for a motoric locus comes from studies that show that the effect of foreperiod on RT interacted neither with the effects of stimulus degradation (Frowein and Sanders, 1978), nor with those of visual stimulus intensity (Raab et al., 1961), nor those of stimulus-response compatibility (e.g., Posner et al., 1973; Frowein and Sanders, 1978). Since these latter variables are assumed to affect perceptual processing and response selection, respectively, the lack of an interaction with foreperiod length was interpreted as evidence that foreperiod length does not operate on either of these processes, but rather on late, motoric processes (e.g., Sanders, 1980).
Challenging the view that variations in foreperiod length exclusively operate on late, motoric processes, subsequent studies have provided evidence in favor of a pre-motor locus (Posner, 1978; Hackley and Valle-Inclán, 2003; Müller-Gethmann et al., 2003; Bausenhart et al., 2006; Hackley et al., 2007). Originally, the notion of a pre-motor locus was advocated on grounds of the observation that the foreperiod effect in choice RT tasks can come along with a speed-accuracy tradeoff (SAT), that is, a reduction in RT is accompanied by an increase in error rate (e.g., Posner et al., 1973). Since SAT effects are assumed to reflect an adjustment of the response criterion rather than a change in the speed of information processing, this finding was interpreted as evidence for a central locus of the foreperiod effect. However, the observation of a SAT has not been replicated in other RT studies (e.g., Müller-Gethmann et al., 2003; Los and Schut, 2008), and more recent research suggests that it seems to be confined to rather short foreperiods and/or situations in which participants receive trial-by-trial RT feedback (Han and Proctor, 2022; see also Lawrence and Klein, 2013). More direct evidence for a pre-motor locus came from psychophysiological studies examining temporal attention effects on the latency of the lateralized readiness potential (LRP) in the event-related potential (ERP). These studies have revealed that temporal attention reduces the latency of the stimulus-locked LRP as an index of the duration of pre-motor processes but has no or little effect on the response-locked LRP as an index of the duration of motor processes (e.g., Müller-Gethmann et al., 2003; Hackley et al., 2007; Seibold and Rolke, 2014b). Furthermore, consistent with a pre-motor locus, several studies also hint at temporal attention effects on central functions such as memory (e.g., Jones and Ward, 2019; but see Kulkarni and Hannula, 2021). For instance, Jones and Ward (2019) observed better recognition memory for items presented with a fixed temporal spacing during the retention interval than for items presented with a variable temporal spacing, suggesting that temporal attention may lead to an encoding benefit or to a more stable representation of encoded items.
Following up the notion of a pre-motoric influence, other researchers have advocated the idea that temporal attention may influence even early, perceptual processing (e.g., Bausenhart et al., 2007; Rolke and Hofmann, 2007; Rolke and Ulrich, 2010), taking up Wundt's original idea that warning signals serve the preparation of attention (Wundt, 1874). Some early, preliminary evidence in this regard was already provided by Niemi and Lehtonen (1982; Experiments 1 and 2) who observed that foreperiod effects on RT were stronger for low-intensity visual stimuli than for high-intensity stimuli (but see Niemi and Lehtonen, 1982, Experiment 3), a finding that was later replicated by Jepma et al. (2012) in the temporal cueing paradigm. Since stimulus intensity affects already early sensory processing (e.g., Kaskey et al., 1980; for an overview see also Nissen, 1977), these results hint that temporal attention may operate on early, perceptual processing. More direct evidence came later from studies employing tasks that put high demands on perceptual processing such as discrimination of (masked) stimuli (Rolke and Hofmann, 2007; Rolke, 2008; Seifried et al., 2010; Rohenkohl et al., 2012; Vangkilde et al., 2012; Cravo et al., 2013), temporal order judgment (Correa et al., 2006b; Bausenhart et al., 2008), and rapid serial stimulus presentation (Martens and Johnson, 2005; Nieuwenstein et al., 2005; Shen and Alain, 2011). Furthermore, evidence for an effect on perceptual processing came from studies employing more direct measures of perceptual processing such as d-prime (Rolke and Hofmann, 2007; Cravo et al., 2013), early components within the ERP (e.g., Lange et al., 2003; Doherty et al., 2005; Correa et al., 2006a; Rolke et al., 2016; Seibold et al., 2020; Balke et al., 2022), or the BOLD responses measured over sensory areas in functional imaging studies (e.g., Hackley et al., 2009; Bueti et al., 2010).
Despite the accumulating evidence that temporal attention can affect perceptual processing, it should also be noted that some studies did not reveal such an early effect (e.g., Griffin et al., 2002; Elbaz and Yeshurun, 2020, Experiment 2; Miniussi et al., 1999; Rudell and Hu, 2001; Wilsch et al., 2020). For instance, Miniussi et al. (1999), who employed trial-by-trial cueing of the temporal onset of a visual target in a simple detection task, did not observe any effect of temporal attention on sensory ERPs such as the P1 and N1; instead, the earliest effect of temporal attention in that study was on the P300 and thus rather late within visual processing (see also Rudell and Hu, 2001; Hackley et al., 2007). Furthermore, several recent studies did not provide evidence for an effect of rhythmic temporal attention on perception (e.g., Elbaz and Yeshurun, 2020; Wilsch et al., 2020). For instance, Elbaz and Yeshurun (2020) asked participants to give a non-speeded judgment about the orientation of a masked visual target presented at the end of a rhythmic or arrhythmic auditory sequence. At variance with a perceptual benefit reported in other rhythmic temporal attention studies (e.g., Rohenkohl et al., 2012; Breska and Deouell, 2017), these authors did not observe higher accuracy in reporting targets presented in-phase with the rhythm as compared to targets presented out of phase. Instead, they observed a lower guessing rate in the rhythmic as compared to the arrhythmic condition, but irrespective of whether the target was presented in-phase or out of phase. Furthermore, Wilsch et al. (2020) measured the combined effect of spatial and rhythmic temporal cueing in a cross-modal setting and did not observe higher discrimination accuracy for targets following a rhythmic context as compared to targets following a random context.
The exact reasons for why perceptual effects of temporal attention are observed in some studies but not in others, are not entirely clear so far. In principle, several factors might play a role: First, the effect of temporal attention may depend on the type of task that has to be performed on the imperative stimulus (see, e.g., Los and Horoufchin, 2011, for such a suggestion; see also Davranche et al., 2011, for neurophysiological evidence) and, specifically with respect to perceptual processing, the effect of temporal attention may become apparent only in perceptually demanding tasks (see also Correa et al., 2006a, for this suggestion). As noted above, most studies providing evidence for an influence of temporal attention on perceptual processing entailed manipulations or tasks that render perceptual processing of the imperative stimulus rather difficult—such as presenting the imperative stimulus only very briefly and/or superimposing it with a mask. Furthermore, some studies showed that the effect of temporal attention was most pronounced in those conditions that were especially difficult (e.g., Rolke, 2008; Seifried et al., 2010; see also Balke et al., 2022). For instance, Rolke (2008) observed the largest temporal attention effect on discrimination of masked visual stimuli in the condition with the shortest target presentation time before masking (or the lowest target contrast). This result indicates that temporal attention facilitates perceptual processing specifically (or even only) in suboptimal sensory conditions. Second, apart from task properties, another factor that might play a role is whether temporal attention is investigated in isolation or jointly with other attention domains such as spatial attention. In the latter situation, it could be possible that the potential of temporal attention in facilitating perceptual processing is masked by the influence of the other attention domain, especially if this domain is more informative or easier to use for attentional orienting (see also Seibold et al., 2019). Interestingly, some evidence that the effect of one attention domain can depend on how easy it can be processed and/or used relative to another domain has been observed for the comparison of spatial and feature-based attention in vision: Whereas it was originally argued that selective effects of feature-based attention arise later than those of spatial attention (e.g., Hillyard and Münte, 1984), more recent research has shown that feature-based attention can also precede or overcome effects of spatial attention, for instance in visual search tasks in which stimuli cannot be selected solely on grounds of location (e.g., Hopf et al., 2004; Seiss et al., 2009). Finally, and specifically with respect to rhythmic temporal attention, it has been suggested that specific methodological factors may play a role in observing a specific benefit for in-phase targets—such as the degree of temporal uncertainty or whether rhythm and target are of the same modality (see Elbaz and Yeshurun, 2020, for a discussion of these methodological factors). Hence, the lack of a temporal attention effect on measures of perceptual processing does not per se invalidate the view that temporal attention affects perception but rather indicates that effects of temporal attention may vary depending on the specific (task) context (see also Correa et al., 2005).
Finally, and at variance with the prevailing notion that temporal attention primarily leads to processing benefits, some studies have shown that temporal attention can also impair stimulus processing (e.g., Correa et al., 2010; Korolczuk et al., 2018; Menceloglu et al., 2021). An illustrative example in that respect is a study by Correa et al. (2010), who investigated the effect of temporal cueing on congruency effects in an arrow flanker task (Experiment 1) and in a setup combining the Simon task with a Spatial Stroop task (Experiment 2). In line with the notion that temporal attention affects perceptual processing, Correa et al. (2010) observed that the size of the Spatial Stroop effect, indicative of perceptual conflict, was smaller in the valid than in the invalid temporal cueing condition. However, and most importantly, the opposite result pattern emerged in the arrow flanker task and the Simon task: Here, the size of the congruency effect, indicative of response conflict, was larger in the valid in comparison to the invalid temporal cueing condition. This basic finding of a larger congruency effect in the Simon and the arrow flanker task has been replicated in subsequent studies (Korolczuk et al., 2018; Menceloglu et al., 2021; but see Menceloglu et al., 2017, for no such effect in a letter flanker task). Furthermore, Korolczuk et al. (2018) reported that the time required to stop an already planned response to an imperative stimulus was longer when the temporal onset of the imperative stimulus was predictable than when it was unpredictable. From a theoretical point of view, these findings, in particular those of Correa et al. (2010), are interesting because they do not only show that temporal attention may directly affects aspects of response selection such as response activation (see Korolczuk et al., 2018), but also suggest that temporal attention can operate on stimulus processing via distinct, parallel mechanisms rather than a single mechanism.
Taken together, the available empirical evidence argues against the traditional view assuming a sole motoric influence and instead supports the view that temporal attention can have multi-faceted effects on the stimulus-response processing chain, including higher-level, cognitive, and late motor processing, but also early, perceptual processing.
Temporal attention effects in different modalities and across modalities
As in research on spatial attention (e.g., Driver and Spence, 1998), the question of how temporal attention affects stimulus processing, and in particular perceptual processing, has been investigated in different modalities and, to some extent, also in multi-modal settings (Ball et al., 2018a,b) as well as in cross-modal settings (Lange and Röder, 2006; Bolger et al., 2013; Mühlberg et al., 2014; Mühlberg and Soto-Faraco, 2019).
In general, unimodal studies have revealed benefits of temporal attention on perceptual processing not only in the visual modality (e.g., Doherty et al., 2005; Correa et al., 2006a; Rohenkohl et al., 2012; Seibold and Rolke, 2014b), but also in the auditory (Lange et al., 2003, 2006; Lange and Röder, 2006; Rimmele et al., 2011), and tactile modalities (Lange and Röder, 2006; Van Ede et al., 2011). These perceptual effects of temporal attention in different modalities have been particularly clearly demonstrated in studies including the measurement of early components of the ERP such as the visual P1, the visual and auditory N1, and the visual N2posterior-contralateral (N2pc), which are directly linked to perceptual processing. For instance, Seibold and Rolke (2014b) showed that targets in a visual search task elicited a more pronounced and earlier arising N2pc in a blocked foreperiod context when the search display appeared after a short foreperiod compared with a long one. Furthermore, Lange et al. (2003) showed that temporally attended auditory stimuli in a temporal Hillyard paradigm elicited a more pronounced auditory N1 than temporally unattended ones. Complementing these findings, studies in which a time-frequency decomposition was applied to the electroencephalogram have shown that temporal attention is accompanied by changes in the amplitude or phase of pre-stimulus neural oscillations in different modalities (e.g., Rohenkohl and Nobre, 2011; Van Ede et al., 2011; Cravo et al., 2013; Todorovic et al., 2015). For example, Van Ede et al. (2011) observed a modulation of the amplitude of neural oscillations within somatosensory areas shortly before the expected onset of the imperative stimulus, and this modulation was spatially specific, that is, contralateral to the expected location of the stimulus. Importantly, these changes in pre-stimulus neural oscillations have been shown to correlate with the amplitude of post-stimulus neural activity in some studies (e.g., Cravo et al., 2013; Todorovic et al., 2015), indicating that they may form the basis for the perceptual processing benefit observed in ERP studies.
Despite the clear evidence for a beneficial effect of temporal attention in different modalities, the results from unimodal studies nonetheless hint to modality-specific differences in the locus of these effects, which becomes evident for the comparison of vision and audition. Specifically, early perceptual effects of temporal attention on auditory processing have been consistently reported in most studies (Lange et al., 2003, 2006; Lange and Röder, 2006; Lange, 2009, 2012; Rimmele et al., 2011; Herbst and Obleser, 2019; but see Lampar and Lange, 2011, Experiment 1) including those using a wide variety of temporal attention manipulations such as temporal orienting (Lampar and Lange, 2011, Experiment 2; Lange et al., 2003; Lange and Röder, 2006), rhythms (Sanders and Astheimer, 2008; Rimmele et al., 2011), and blocked foreperiods (Seibold et al., 2011b), and even when temporal information is conveyed only implicitly by stimulus features (Herbst and Obleser, 2019).4 In contrast, temporal attention effects on early visual processing have been observed less consistently. For instance, as mentioned above, an ERP study by Miniussi et al. (1999) aimed at investigating the effect of temporal cueing in a simple detection task did not reveal an effect of temporal attention before rather late visual processing (i.e., on the P300; see also Griffin et al., 2002, Experiment 2). In contrast, subsequent studies using other types of tasks and temporal attention manipulations did reveal temporal attention effects on early visual processing (e.g., Correa et al., 2006a; Rohenkohl and Nobre, 2011; Seibold and Rolke, 2014b; Balke et al., 2022). For instance, Correa et al. (2006a), who employed blocked temporal cueing in a visual discrimination task, observed a larger P1 and a shorter-latenced N2 for temporally attended stimuli in comparison to temporally unattended ones. Furthermore, Seibold and Rolke (2014b) as well as Balke et al. (2022), both of whom used a blocked foreperiod manipulation in combination with a visual search task, observed amplitude enhancements and latency reductions for both the visual N1 and the N2pc, showing that temporal attention can affect early visual processing.
Apart from methodological differences, one potential reason for these discrepant results could be that the visual and auditory modality are differentially sensitive to temporal attention effects (see also Ball et al., 2022). This view is consistent with the general idea that the auditory modality may be more sensitive to temporal information (e.g., Repp and Penel, 2002; Bratzke et al., 2012), whereas the visual modality may be more sensitive to spatial information (e.g., Bertelson and Aschersleben, 1998; Park et al., 2003). Due to this potential differential sensitivity, temporal attention may affect early auditory processing by default and independent of other factors such as the type of task participants have to perform or the way temporal attention is induced; in contrast, and as already noted above, temporal attention may affect early visual processing only when demands on perceptual processing are high enough (see also Correa et al., 2006a), meaning that early visual processing might be already less optimal. However, given that the above-described comparison is indirect (i.e., across studies), further systematic research is necessary to unravel differential influences of temporal attention on perceptual processing in different modalities.
Apart from unimodal contexts, some recent studies (e.g., Ball et al., 2018a,b) have also addressed multimodal contexts, focusing on the question of whether temporal attention effects differ between unimodal and multimodal stimuli. In these studies, near-threshold (uni- or multimodal) targets were embedded within sequences of audio-visual, visual, or auditory distractors, with the target appearing either early (short foreperiod) or late (late foreperiod) in the sequence. The effect of temporal attention was examined by varying the foreperiod probability across blocks, with either the short or the long foreperiod being more likely in each block. In this context, the effect of temporal attention on discrimination accuracy was larger for multi-modal (audio-visual) stimuli than for unimodal (visual or auditory) stimuli. Importantly, this larger temporal attention effect was observed not only on a group-level (Ball et al., 2018b), but also when comparing the multi-modal condition with the best unimodal conditions for each participant (Ball et al., 2018a). Although multi-modal superiority in temporal attention can be explained in several ways (Ball et al., 2018a; see also Ball et al., 2021), one possible reason for multi-modal superiority would be that temporal information from different modalities can be combined to boost perceptual processing (see also Ten Oever et al., 2014).
Finally, going beyond the question whether temporal attention facilitates perceptual processing in different modalities, some studies have even addressed the possibility of cross-modal transfer, that is, that temporal attention effects can transfer from one (attended) modality to another (unattended) modality (e.g., Lange and Röder, 2006; Bolger et al., 2013; Mühlberg et al., 2014; Menceloglu et al., 2019; Mühlberg and Soto-Faraco, 2019). One example for cross-modal transfer in temporal attention is provided by Lange and Röder (2006) who presented auditory or tactile stimuli being separated either by a short or long foreperiod. The authors instructed participants to attend to one modality and, within that modality, only to stimuli presented after a specific (e.g., short) foreperiod. They observed an enhanced auditory N1 for temporally attended stimuli irrespective of the modality to which participants attended. In contrast to these results, two subsequent studies employing an audio-visual context (Mühlberg et al., 2014) or a visuo-tactile context (Mühlberg and Soto-Faraco, 2019) did not reveal evidence for cross-modal transfer of temporal attention effects. In line with these latter findings, Menceloglu et al. (2019) observed no interaction of temporal attention with modality-specific attention in a study employing auditory and visual stimuli. Temporal attention in this study was tested by presenting blocks with different probabilities for short and long foreperiods and asking participants to attend only to one foreperiod per block. Modality-specific attention was tested by varying the probability for stimulus occurrence in each modality, thereby biasing attention toward the more probable modality. Although beneficial effects of both temporal attention and modality-specific attention were observed, their effects did not interact, arguing for independent influences of temporal attention and modality-specific attention on stimulus processing.
The reasons for these discrepant findings regarding cross-modal effects in temporal attention have yet to be clarified. However, one factor that could play a role is the way in which modality-specific attention was induced in different studies: For instance, in the Menceloglu et al. (2019) study, the primary (more frequent) modality was kept constant for each participant, and modality-specific attention was induced implicitly, that is, by presenting stimuli in one modality in most trials. In contrast, in the study of Lange and Röder (2006), the to-be-attended modality alternated between blocks, and participants were explicitly instructed to focus on one single modality. Accordingly, for cross-modal transfer to occur, modality-specific attention may have to be explicitly task-relevant.
In sum, there is clear evidence that temporal attention facilitates perceptual processing in different modalities (e.g., Lange et al., 2003; Van Ede et al., 2011; Seibold and Rolke, 2014b). Furthermore, perception of multimodal stimuli seems to benefit to a stronger extent from temporal attention than unimodal stimuli (e.g., Ball et al., 2018a), which indicates that processing of temporal regularities may be combined across different sensory modalities. Finally, there is some evidence for cross-modal transfer in temporal attention (Lange and Röder, 2006; but see Menceloglu et al., 2019). Yet, whether these findings on temporal attention within and across modalities reflect modality-specific mechanisms or a supramodal mechanism underlying temporal attention, as it has been discussed in the context of spatial attention (see, e.g., Driver and Spence, 1998), remains a matter of debate.
Temporal attention in combination with other attention domains
Not surprisingly, the discovery that temporal attention affects perceptual processing of the imperative stimulus has also fueled research addressing the interplay of temporal attention with other attention domains. Most of the evidence for this interplay comes from studies investigating temporal attention in combination with spatial attention (e.g., Griffin et al., 2002; Doherty et al., 2005; Lamy, 2005; Rohenkohl and Nobre, 2011; Seibold et al., 2020). In contrast, much less research has been conducted on the interplay of temporal and feature-based attention (but see Kingstone, 1992; Warren et al., 2014; Rolke et al., 2016; Grubert and Eimer, 2018).
Studies combining temporal and spatial attention in the visual modality have revealed mixed results. One set of studies suggests that temporal and spatial attention may exert independent effects on stimulus processing (Coull and Nobre, 1998; Griffin et al., 2002; Lange et al., 2006; MacKay and Juola, 2007; see also Tal-Perry and Yuval-Greenberg, 2022). For instance, Coull and Nobre (1998) employed probabilistic cueing of either the temporal onset or the spatial location of a visual stimulus and measured PET and fMRI to compare the activation of brain areas involved in temporal and spatial attention. These authors observed overlapping neural signatures of the two attention domains, but also a clear differential lateralization of these signatures, indicating that temporal and spatial attention may affect stimulus processing in distinct ways. Even more direct evidence for the idea of independent effects was observed by Griffin et al. (2002, Experiment 1) in an ERP study with a similar experimental setup. Replicating previous findings on spatial attention, they observed effects of spatial attention appearing within the first 100 ms, whereas the effect of temporal attention arose later and was less focused on visual areas. These results led the authors to conclude that spatial and temporal attention modulate visual processing in distinct ways.
In contrast to this conclusion, however, other studies have provided evidence for interactions between temporal and spatial attention (e.g., Milliken et al., 2003; Doherty et al., 2005; Rohenkohl and Nobre, 2011; Seibold et al., 2020). For instance, Doherty et al. (2005) asked participants to monitor a moving disc that disappeared behind an occluder at some point during the trial, and participants had to respond to the presence of a dot upon the re-appearance of the disc. Temporal and spatial attention were varied by making the trajectory of the disc either spatially predictable, temporally predictable, spatio-temporally predictable, or unpredictable in both domains. The main finding of that study was that spatially predictable disks evoked a more pronounced P1 as an index of early visual processing, and this effect was amplified by additional temporal predictability (see also Rohenkohl and Nobre, 2011, for a similar finding). Based on these observations, Doherty et al. (2005) proposed that temporal attention boosts facilitatory (or selective) effects of other attention domains (i.e., spatial attention).
In contrast to the vast array of studies in the visual modality, relatively few studies exist addressing the interplay of temporal and spatial attention in the auditory modality (e.g., Lange et al., 2006; Rimmele et al., 2011; Lange, 2012). For instance, Lange et al. (2006) combined manipulations of temporal and spatial attention in a temporal Hillyard paradigm in which the offset marker of either a short or long interval was presented either to the left or right ear and participants were asked to attend only to stimuli presented within one ear and after one temporal interval. Analogous to previous studies, Lange et al. (2006) observed an enhanced auditory N1 for both temporally attended stimuli and spatially attended stimuli. Crucially, the effects of both domains were additive, indicating that temporal and spatial attention modulate early auditory processing in an independent manner. Moreover, Rimmele et al. (2011) showed that not only did temporal attention and spatial attention exert independent effects on auditory processing, but the effects of temporal attention also preceded those of spatial attention. Hence, the existing studies conducted in the auditory modality suggest that temporal attention and spatial attention are uncoupled.
Although the exact conditions required for observing interactive effects of temporal and spatial attention have yet to be clarified, several factors other than stimulus modality may play a role: One important factor may be the type of manipulation that is used to induce spatial attention and/or temporal attention. For instance, Olk (2014) contrasted arrow cues with more abstract symbolic cues and showed that only the former ones, most probably eliciting involuntary spatial attention (e.g., Ristic and Kingstone, 2006), interacted in their effect with temporal cues, but this was not the case for the latter, abstract ones, which probably elicited only voluntary spatial attention. Furthermore, a recent modeling study by Tal-Perry and Yuval-Greenberg (2022) did not reveal an interaction of spatial cueing with the variable foreperiod effect. Consequently, observing interactions between time and space may also depend on the way spatial and/or temporal attention is manipulated. Another factor that may play a role in whether temporal and spatial attention are combined to facilitate stimulus processing might be the nature of the target object. In the study of Doherty et al. (2005), temporal and spatial attention were not induced by single cues but instead by the trajectory of a single moving object. Since the processing of a movement requires integration of spatial locations across time, it may well be that processing of temporal and spatial information in this type of setup is strongly bound. One final important factor for combining spatial and temporal attention may be the (relative) informativeness of a specific attention domain with respect to the upcoming stimulus and/or how difficult it is to extract and use the information in this domain for attentional preparation. This idea is rooted in the observation that for multi-dimensional stimuli (i.e., stimuli that have to be selected on grounds of several attention domains such as location and color), selection does not seem to follow a fixed temporal order (i.e., selection of location before selection of color), but instead seems to depend on how easy discrimination is in each domain (e.g., Hansen and Hillyard, 1983; for a discussion of this possibility in the context of temporal attention see Seibold et al., 2019). Accordingly, in a case where spatial information is the more informative cue for attentional preparation, this information would be weighted stronger and thereby dominate the effect of the cue in another (i.e., temporal) domain. From a theoretical point of view, this latter possibility is interesting because it would suggest that different types of attentional cues can be used in a flexible manner for preparation, depending on which information is most useful.
Though less often examined than the interaction between temporal and spatial attention, there do exist several studies addressing potential interactions of temporal and feature-based attention. One pioneering study in this respect was conducted by Kingstone (1992) who combined probabilistic temporal cueing with feature-based cueing. Specifically, participants were presented with a combined cue that contained information about both the likely temporal onset of a subsequent imperative stimulus and the type of stimulus. Kingstone (1992) observed that the RT to the imperative stimulus was fastest when both the temporal and the feature cue were valid and slowest when one cue was valid but the other one was invalid, indicating that participants formed combined expectations about the “what” and the “when” of stimulus occurrence.
Further available evidence shows that temporal attention can indeed support selective feature-based processing (e.g., Lakatos et al., 2013; Warren et al., 2014; Grubert and Eimer, 2018; Seibold et al., 2020), which becomes particular clear in the following exemplary studies: First, in a functional imaging study by Warren et al. (2014), participants were presented a Gabor grating that regularly changed its orientation in a clockwise rotation, and they had to detect a change in the Gabor's frequency. To measure the effect of temporal attention, the authors varied the temporal predictability of the particular Gabor orientation at which the frequency change could occur. Warren et al. (2014) showed that, in the temporally predictable condition, orientation-sensitive voxels in the primary visual cortex were tuned toward the orientation at which the frequency change was expected to occur, and this tuning occurred shortly before the actual Gabor grating was presented. Thus, temporal attention was used to tune the neuronal system toward specific stimulus attributes which were important for the expected perceptual task. The second study to show temporal attention supporting feature-based processing was an ERP study on visual search. Grubert and Eimer (2018) showed that the pre-activation of target templates can be temporally aligned with the expected onset of the target. Specifically, these authors observed that probe stimuli that (1) were interspersed between subsequent visual search episodes and (2) shared the target's color elicited an N2pc, but this was only the case if they were presented shortly before the expected onset of the next search display. Finally, Seibold et al. (2020) measured the conjoint effect of temporal, spatial and feature-based attention on early visual processing. In that study, temporal attention was varied by means of probabilistic temporal cueing, whereas spatial and feature-based attention were varied by instructing participants to attend and respond to stimuli at a specific location and in a specific color. Replicating early effects of spatial and feature-based attention, Seibold et al. (2020) observed that both spatial and feature-based attention led to an enhanced visual N1. Importantly, this enhancement was observed only in temporally valid trials, that is, when the stimulus was presented at the expected moment in time. Hence, the results of these three studies show that temporal attention can tune selective effects of other attention domains in the sense that spatially selective and feature-selective processing can start before the actual onset of a stimulus.
Perceptual mechanisms of temporal attention
In this section we will discuss three prominent mechanisms that have been proposed to explain how perceptual processing may benefit from temporal attention (see also Nobre and Van Ede, 2018).
The presumably least disputed and—given attention mechanisms in general—most natural account of the mechanisms underlying temporal attention effects on perceptual processing is sensory enhancement. Sensory enhancement can be roughly described as an improvement in the quality of neural signatures of incoming sensory information (e.g., Downing, 1988; Chun and Wolfe, 2001; Carrasco et al., 2002). According to this account, knowing the temporal onset of a stimulus temporally increases neural responses to incoming sensory signals and thereby facilitates perceptual processing of stimuli presented within the attended time window (e.g., Correa et al., 2006a). Support for this assumption comes from several lines of evidence: First, the early effects of temporal attention in most ERP studies demonstrating these effects appeared as ERP amplitude enhancements, and this pattern emerged across different paradigms, including blocked foreperiods (e.g., Seibold and Rolke, 2014b), different types of temporal orienting (e.g., Correa et al., 2006a; Lange et al., 2006), and rhythmic temporal attention paradigms (e.g., Doherty et al., 2005; Rimmele et al., 2011). Such ERP amplitude enhancements have frequently been linked to an amplification of the incoming sensory signal, similar to what has been proposed in the context of other attention domains such as spatial and feature-based attention (e.g., Hillyard and Anllo-Vento, 1998; Ling et al., 2009; Hopf et al., 2012; Mishra et al., 2012; but see Makeig et al., 2002, for other potential mechanisms that may give rise to ERP amplitude enhancements). A second line of evidence for sensory enhancement by temporal attention comes from the observation that temporal attention leads to a temporary upregulation of neural activity in sensory areas, as revealed in functional imaging studies (e.g., Coull and Nobre, 1998; Hackley et al., 2009). Finally, even though it is indirect, evidence for signal enhancement also comes from studies showing that temporal attention may increase the signal-to-noise ratio (e.g., Bausenhart et al., 2010; Rohenkohl et al., 2012; Balke et al., 2022). For instance, Balke et al. (2022) measured the effect of temporal attention on spatial selection of a target in pop-out visual search under different target salience conditions. They observed that, not only did temporal attention lead to an earlier-occurring N2pc as index of target selection, but this latency reduction was also more pronounced when the target was less salient. On grounds of the assumption that the signal-to-noise ratio is lower for low-salience targets, the stronger temporal attention effect in the low-salience condition could be explained by an increase in the signal-to-noise ratio, and this increase may be caused by an enhancement of the signal.
Directly related to signal enhancement and most probably a mechanism that can be considered a subordinate one is a mechanism that we will refer to as pre-activation of selective processing (e.g., Correa et al., 2010; Warren et al., 2014; Grubert and Eimer, 2018). This mechanism has been proposed particularly in the context of the interplay of temporal attention with other attention domains. Specifically, extending the idea of signal enhancement, the core assumption here is that temporal attention not only facilitates neural processing in sensory areas in general but may also lead to a selective pre-activation or tuning of areas that decode specific, task-relevant features. Thus far, the strongest empirical evidence for this approach has been provided by the studies discussed above showing that temporal attention is already inducing (or enhancing) spatially selective and feature-selective processing in sensory areas shortly before the expected onset of an imperative stimulus (e.g., Van Ede et al., 2011; Warren et al., 2014; Grubert and Eimer, 2018).
Second, several authors have also proposed that temporal attention affects the speed of early perceptual processing (e.g., Rolke, 2008; Seifried et al., 2010; Seibold et al., 2011a,b). This sensory acceleration mechanism is rooted in the so-called law of prior entry (Titchener, 1908), a general mechanism of attention that has also been investigated in the context of other attention domains (e.g., Scharlau, 2004; Vibell et al., 2007; Weiß and Scharlau, 2012; for a review see, e.g., Spence and Parise, 2010). According to this principle, attention increases the speed of neural processing within modality-specific sensory areas, and this leads to an earlier (conscious) perception of a stimulus. In the context of temporal attention, the existence of such a mechanism has been supported by research showing a reduction of perceptual latency for stimuli presented at temporally-attended moment in time (Seifried et al., 2010) as well as by research showing a reduction in latency for early ERPs such as the N1 (e.g., Hackley et al., 2007; Seibold and Rolke, 2014b), the visual N2 and the auditory mismatch negativity (e.g., Correa et al., 2006a; Seibold et al., 2011b), and the N2pc (Seibold and Rolke, 2014b; Rolke et al., 2016; Balke et al., 2021, 2022). The questions of whether and how sensory acceleration might cause signal enhancement (or vice versa), what the connection between these possible mechanisms is, and whether these mechanisms come into play specifically in temporal attention have yet to be answered.
Finally, a third mechanism which has become especially prominent in temporal attention research over the past years concerns the changes in the dynamics of ongoing neural oscillations, in particular the power and the phase of these neural oscillations (see Nobre and Van Ede, 2018; see also Herbst et al., 2022). A power change caused by temporal attention refers to a change in the overall amplitude of ongoing oscillations shortly before the expected onset of a stimulus (see, e.g., Rohenkohl and Nobre, 2011). This amplitude change is typically explained in terms of how synchronized the neural activity is. Specifically, increases (or decreases) in the power of ongoing oscillation are assumed to be the consequence of an increase (or decrease) of neural synchronization. Although changes in oscillatory power have been observed across different frequency bands, oscillations in the range of the alpha-band are particularly frequently cited in the context of temporal attention (e.g., Rohenkohl and Nobre, 2011; Herbst and Obleser, 2017; for an overview, see also Van Diepen et al., 2019). For instance, Herbst and Obleser (2017) who varied the variability of the foreperiod distributions in a probabilistic temporal cueing task observed that the condition with lowest temporal uncertainty (i.e., the smallest range of possible foreperiods) was associated with an increase in alpha power shortly before the expected moment of stimulus onset. Importantly, the reported power changes went along with a processing benefit. A change of the phase of neural oscillation, which has become popular under the term entrainment, refers to the alignment of the phase of neural oscillations (i.e., the maximum amplitude of oscillations in either direction) to an externally induced stimulation, which leads to optimized stimulus processing (e.g., Schroeder and Lakatos, 2009; Mathewson et al., 2010; Stefanics et al., 2010). Empirical research on entrainment has provided evidence for temporal attention-induced entrainment of slow oscillatory activity in the delta range (e.g., Lakatos et al., 2008; Cravo et al., 2013; Breska and Ivry, 2020; Daume et al., 2021; see also Herbst et al., 2022), as well as some evidence for entrainment in higher frequency bands (e.g., Besle et al., 2011; Spaak et al., 2014; Samaha et al., 2015; Solís-Vivanco et al., 2018).
Given that most studies that provided evidence for entrainment in the context of temporal attention used rhythmic stimulation, the question arises: is entrainment a mechanism that is specific to rhythmic temporal attention, or is it a more general mechanism that also plays a role in settings in which temporal attention is induced by a single stimulus, for instance a warning signal or a temporal cue? Even though this question has been addressed in few studies (e.g., Breska and Deouell, 2017; Herbst and Obleser, 2019; Daume et al., 2021), the results obtained so far suggest that entrainment may indeed be a general mechanism of temporal attention. For instance, Daume et al. (2021) recorded the magnetoencephalogram in a task in which a visual disc moved continuously at constant speed, disappeared, and then reappeared again from behind an occluder. The timing of the disc's re-appearance was jittered around the interval that was required to pass the occluder based on its length and the disc's speed. To assess the effect of temporal attention, the authors compared neural activity in two tasks, a temporal task, in which participants had to judge whether the disc had appeared too early or too late, and a luminance task, in which participants had to judge whether the disc was brighter or darker than before disappearing. Despite using a non-rhythmic stimulation, Daume et al. (2021) observed a higher phase-reset of low-frequency oscillations in accordance with the expected temporal onset of reappearance of the disc in the temporal task as compared to the luminance task. This finding of phase-reset in a non-rhythmic stimulation context shows that entrainment is not a mechanism that is necessarily specific to rhythmic temporal attention but may instead reflect a general mechanism that also underlies beneficial effects of temporal attention in non-rhythmic contexts.
Summary and future directions
In sum, the research on temporal attention discussed up to this point in the review clearly shows that temporal attention is a core domain of attention that not only affects stimulus processing across sensory modalities but also interacts with other domains. Furthermore, like other attention domains (see, e.g., Spence and Parise, 2010; Carrasco, 2011), temporal attention may facilitate stimulus processing via several mechanisms, depending on factors such as the specific stimulus, task context, and the specific way temporal attention is induced. Yet, even though steady progress has been made in the last decade of research on temporal attention, particularly on the subjects of rhythmic temporal attention and the role of neural oscillations in temporal attention (e.g., Breska and Deouell, 2017; Herbst et al., 2022), several questions remain unanswered, and new questions have arisen. In the remainder of this review, we will highlight some of the most pressing questions.
First, as became evident reviewing the research across the different sections, temporal attention (like other attention domains) has been studied in different paradigms, with some of them being rooted in the historical tradition of temporal attention research (i.e., foreperiod paradigms) and others being adopted from other attention domains (i.e., temporal orienting paradigms). On the one hand, this paradigmatic diversification has resulted in a wide body of research on temporal attention, contributing to its establishment in the broader landscape of attention. On the other hand, as illustrated for example by the case of the interaction between temporal and spatial attention in vision, this paradigmatic diversification has also led to partially incompatible results, which remain difficult to interpret. The incompatibility of these results may be due in part to the unintentional measurement of partially distinct types of temporal attention in different paradigms (see also Nobre et al., 2007; Lawrence and Klein, 2013). Some empirical evidence for this claim is provided by a direct comparison of the results from studies that used closely similar tasks but different temporal attention manipulations (e.g., De la Rosa et al., 2012, in comparison to Capizzi et al., 2012) or from those studies directly comparing different types of temporal attention manipulations (e.g., Olk, 2014), and also from studies examining the brain structures involved in different temporal attention paradigms (e.g., Coull et al., 2000; Triviño et al., 2016; see also Coull and Nobre, 2008). For instance, De la Rosa et al. (2012) showed that probabilistic temporal cueing effects in a primary task were strongly reduced if participants had to perform a concurrent working memory task, whereas Capizzi et al. (2012) showed that effects of rhythmic temporal cueing remained intact when adding a secondary task. These results as well as those from other studies (e.g., Rohenkohl et al., 2011; Triviño et al., 2011) have led some researchers to draw a distinction between automatic temporal attention, which is assumed to be induced by rhythmic temporal cueing, and controlled temporal attention, which is assumed to be induced in probabilistic temporal cueing (see also Nobre et al., 2007; for another classification scheme see, e.g., Schroeder and Lakatos, 2009). Similar distinctions between unintentional and intentional processes or bottom-up and top-down processes have also been made in the context of the variable foreperiod paradigm (see Los and Van den Heuvel, 2001; Los and Heslenfeld, 2005; Vallesi and Shallice, 2007; Mento and Tarantino, 2015). Here, dissociative result patterns arguing for the contribution of either one or the other process were obtained when comparing different participant populations (Mento et al., 2019; Mento and Granziol, 2020; Duma et al., 2021) or when considering neurophysiological indicators (Mento, 2017; Duma et al., 2020). Classifications like this could serve as a starting point for a more systematic comparison of different temporal attention manipulations, which may in turn help to gain a better understanding of some of the discrepant results that have been reported in the temporal attention literature.
A second, somewhat related research opportunity for future research would be a further systematic investigation of the mechanisms that underlie temporal attention. In particular, it remains unclear whether some of the mechanisms outlined above such as sensory acceleration are distinct mechanisms that can occur independently of other mechanisms or whether they are simply the consequence of other mechanisms, such as entrainment or signal enhancement. Furthermore, as already noted above, it is also still unclear to what extent (some of) these mechanisms operate only under very specific task contexts and/or temporal attention conditions. Here, more systematic research is needed to gain a better understanding of how general these specific mechanisms may be. As mentioned above, some progress has been made with respect to entrainment, which has been demonstrated not only in rhythmic temporal attention tasks but also in non-rhythmic tasks (e.g., Daume et al., 2021), indicating that this mechanism may be more general than originally thought.
In addition to a further examination of experimental paradigms and underlying mechanisms, future research could also put a stronger focus on how temporal attention affects perceptual processing in different unimodal and cross-modal contexts. As described in the previous section, research on temporal attention has been predominantly focused on vision at the expense of other modalities. Consequently, future research could include a systematic investigation of temporal attention effects in other modalities, in particular the tactile modality, as well as different cross-modal and multisensory settings. Another pressing question in this field is the existence of modality-specific differences in the effects of temporal attention, particularly with respect to the comparison of audition and vision. Here, future research should also include a direct comparison of temporal attention effects in different modalities within the same study.
Finally, another promising avenue for future studies could be to investigate how specific temporal attention might aid perceptual processing. For example, one important mechanism in the context of voluntary (or top-down) attention is suppression (or inhibition) of irrelevant stimuli, features, or locations (see, e.g., Geng, 2014). Whereas, suppression has become a major topic in research on spatial attention, and in particular in the context of visual search (see, e.g., Cosman et al., 2018; Gaspelin and Luck, 2018; Feldmann-Wüstefeld et al., 2021), this mechanism has been covered by few studies in the context of temporal attention (but see Los, 2004; Seibold and Rolke, 2014a; Amit et al., 2019; Balke et al., 2021; Gresch et al., 2021; Xu et al., 2021, 2022). For instance, Balke et al. (2021), who examined the effect of a blocked foreperiod manipulation on spatial selection and suppression of a salient distractor in visual search did not observe evidence for an influence of temporal attention on the distractor positivity (or PD), an ERP assumed to index distractor suppression. In contrast, Xu et al. (2021), observed reduced attentional capture if a salient distractor was presented at a location that was frequently occupied by a distractor, and this reduction was stronger if the location was presented more frequently after a specific foreperiod. This result suggests that suppression of stimuli at particular locations can become more effective if the location can be predicted on grounds of temporal information. Following these results, it may be interesting to investigate more systematically the conditions under which temporal attention may support suppression in general and whether temporal attention may (also) serve suppression of specific features.
Taken together, research from the last few years has substantiated the idea that temporal attention, like spatial and feature-based attention, influences perceptual processing of stimuli in different modalities. Furthermore, there is growing evidence of interactions across attention domains: temporal attention can influence the beneficial effects of other attention domains on perceptual processing. Of course, there are still many open questions, such as whether temporal attention is effective in the same way in different modalities and in which conditions temporal attention interacts with other attention domains. Nonetheless, the current state of research clearly shows that the temporal domain is an essential factor in understanding attention in general and must be taken into account in the saddling and interpretation of studies.
Author contributions
VS wrote the first draft of the manuscript. BR and JB wrote sections of the manuscript. All authors reviewed and edited the manuscript and they read and approved the submitted version.
Funding
This research has been funded by the Deutsche Forschungsgemeinschaft (DFG, German Research Foundation) — project number 391742416 (reference number SE 2576/1-1). We furthermore acknowledge support by the Open Access Publishing Fund of the University of Tübingen.
Conflict of interest
The authors declare that the research was conducted in the absence of any commercial or financial relationships that could be construed as a potential conflict of interest.
Publisher's note
All claims expressed in this article are solely those of the authors and do not necessarily represent those of their affiliated organizations, or those of the publisher, the editors and the reviewers. Any product that may be evaluated in this article, or claim that may be made by its manufacturer, is not guaranteed or endorsed by the publisher.
Footnotes
1. ^We will not cover functional imaging and electrophysiological studies focusing on the process of orienting attention in time itself and its associated neural correlates. In that respect, interested readers are referred, for instance, to Coull (2004) and Mento et al. (2015; for a summary, see also Nobre and Van Ede, 2018). Furthermore, we will also not further discuss recent theoretical and computational approaches that link temporal attention to learning mechanisms; in that respect, we refer to the insightful work of Los et al. (2017; see also Salet et al., 2022).
2. ^In the context of the foreperiod paradigm, it is important to consider that warning signals do not only trigger temporal attention, but also lead to a temporary increase in response readiness, most often termed as (phasic) alertness (e.g., Posner and Petersen, 1990; Weinbach and Henik, 2012; but see Lawrence and Klein, 2013, for a different terminology). Although alertness has been shown to lead to similar effects on perception (e.g., Matthias et al., 2010) and response selection (e.g., Weinbach and Henik, 2012) as those reported for temporal attention, both concepts are nonetheless dissociable (for a systematic discussion see Weinbach and Henik, 2012): For instance, functional imaging evidence suggests that temporal attention and alertness are associated with differential brain activation patterns (Hackley et al., 2009). Furthermore, it has been shown that one diagnostic effect of alertness, that is, an increase in congruency effects in response-conflict tasks, is mainly restricted to short FPs (e.g., Weinbach and Henik, 2013), and that alerting effects are observed even when the temporal contingency between a warning signal and the imperative signal required for temporal attention to emerge is eliminated (Weinbach and Henik, 2013; see also Lawrence and Klein, 2013).
3. ^It should be noted that, in temporal cueing, the performance benefit for imperative stimuli following valid temporal cues is not necessarily symmetric across foreperiods. Instead, it is typically weaker (or even absent) for the (relative) longer of two foreperiods (see, e.g., Coull and Nobre, 1998; Correa et al., 2006a). This asymmetry in temporal cueing has been explained in terms of a re-orienting of attention in the invalid long foreperiod condition (e.g., Coull and Nobre, 1998): If the cue invalidly announces a short foreperiod, but the imperative stimulus does not occur after that foreperiod, participants may strategically re-orient attention toward the next possible moment of stimulus presentation, that is the long foreperiod. Consequently, an imperative stimulus in the invalid long foreperiod condition might be attended to a similar degree as one in the valid long foreperiod condition.
4. ^It should be noted that, although these studies have typically revealed amplitude enhancements for early ERPs, some studies (e.g., Lange, 2009) also revealed amplitude reductions. Lange (2013) suggested that the observation of amplitude enhancements as opposed to amplitude reductions may depend on the specific type of manipulation that is used: Specifically, she suggested that varying task-relevance (i.e., instructing participants to attend to a specific interval) as it is done in the temporal Hillyard paradigm is a more direct manipulation of attention and will be reflected amplitude enhancements, whereas varying the probability that a stimulus will appear at all as it is done when comparing rhythmic and arrhythmic temporal sequences induces stimulus expectations, and these expectations will lead to amplitude reductions (see also Summerfield and Egner, 2009, for an insightful review on attention vs. stimulus expectations). However, given that other studies investigating effects of rhythmic temporal attention have shown amplitude enhancement for early ERPs (e.g., Doherty et al., 2005; Rimmele et al., 2011), and given that both amplitude reductions and enhancements were observed at a similar processing level (i.e., on the auditory N1), we do not further differentiate between expectation-based and task-relevance based effects in temporal attention at this point.
References
Amit, R., Abeles, D., Carrasco, M., Yuval-Greenberg, S. (2019). Oculomotor inhibition reflects temporal expectations. Neuroimage 184, 279–292. doi: 10.1016/j.neuroimage.2018.09.026
Balke, J., Rolke, B., Seibold, V. C. (2021). Reduction of temporal uncertainty facilitates stimulus-driven processes in spatial selection. Biol. Psychol. 159, 108028. doi: 10.1016/j.biopsycho.2021.108028
Balke, J., Rolke, B., Seibold, V. C. (2022). Temporal preparation accelerates spatial selection by facilitating bottom-up processing. Brain Res. 1777, 147765. doi: 10.1016/j.brainres.2021.147765
Ball, F., Andreca, J., Noesselt, T. (2022). Context dependency of time-based event-related expectations for different modalities. Psychol. Res. 86, 1239–1251. doi: 10.1007/s00426-021-01564-9
Ball, F., Fuehrmann, F., Stratil, F., Noesselt, T. (2018a). Phasic and sustained interactions of multisensory interplay and temporal expectation. Sci. Rep. 8, 10208. doi: 10.1038/s41598-018-28495-7
Ball, F., Michels, L. E., Thiele, C., Noesselt, T. (2018b). The role of multisensory interplay in enabling temporal expectations. Cognition 170, 130–146. doi: 10.1016/j.cognition.2017.09.015
Ball, F., Spuerck, I., Noesslet, T. (2021). Minimal interplay between explicit knowledge, dynamics of learning and temporal expectations in different, complex uni- and multisensory contexts. Atten. Percept. Psychophys. 83, 2551–2573. doi: 10.3758/s13414-021-02313-1
Baumeister, A. A., Joubert, C. E. (1969). Interactive effects on reaction time of preparatory interval length and preparatory interval frequency. J. Exp. Psychol. 82, 393–395. doi: 10.1037/h0028119
Bausenhart, K. M., Rolke, B., Hackley, S. A., Ulrich, R. (2006). The locus of temporal preparation effects: evidence from the psychological refractory period paradigm. Psychon. Bull. Rev. 13, 536–542. doi: 10.3758/BF03193882
Bausenhart, K. M., Rolke, B., Seibold, V. C., Ulrich, R. (2010). Temporal preparation influences the dynamics of information processing: evidence for early onset of information accumulation. Vis. Res. 50, 1025–1034. doi: 10.1016/j.visres.2010.03.011
Bausenhart, K. M., Rolke, B., Ulrich, R. (2007). Knowing when to hear aids what to hear. Quart. J. Exp. Psychol. 60, 1610–1615. doi: 10.1080/17470210701536419
Bausenhart, K. M., Rolke, B., Ulrich, R. (2008). Temporal preparation improves temporal resolution: evidence from constant foreperiods. Percept. Psychophys. 70, 1504–1514. doi: 10.3758/PP.70.8.1504
Bertelson, P., Aschersleben, G. (1998). Automatic visual bias of perceived auditory location. Psychon. Bull. Rev. 5, 482–489. doi: 10.3758/BF03208826
Bertelson, P., Tisseyre, F. (1969). The time-course of preparation: confirmatory results with visual and auditory warning signals. Acta Psychol. 30, 145–154. doi: 10.1016/0001-6918(69)90047-X
Besle, J., Schevon, C. A., Mehta, A. D., Lakatos, P., Goodman, R. R., McKhann, G. M., et al. (2011). Tuning of the human neocortex to the temporal dynamics of attended events. J. Neurosci. 31, 3176–3185. doi: 10.1523/JNEUROSCI.4518-10.2011
Bevan, W., Hardesty, D. L., Avant, L. L. (1965). Response latency with constant and variable interval schedules. Percept. Mot. Ski. 20, 969–972. doi: 10.2466/pms.1965.20.3.969
Bolger, D., Trost, W., Schön, D. (2013). Rhythm implicitly affects temporal orienting of attention across modalities. Acta Psychol. 142, 238–244. doi: 10.1016/j.actpsy.2012.11.012
Bratzke, D., Seifried, T., Ulrich, R. (2012). Perceptual learning in temporal discrimination: asymmetric cross-modal transfer from audition to vision. Exp. Brain Res. 221, 205–210. doi: 10.1007/s00221-012-3162-0
Breska, A., Deouell, L. Y. (2014). Automatic bias of temporal expectations following temporally regular input independently of high-level temporal expectation. J. Cogn. Neurosci. 26, 1555–1571. doi: 10.1162/jocn_a_00564
Breska, A., Deouell, L. Y. (2017). Neural mechanisms of rhythm-based temporal prediction: delta phase-locking reflects temporal predictability but not rhythmic entrainment. PLoS Biol. 15:e2001665. doi: 10.1371/journal.pbio.2001665
Breska, A., Ivry, R. B. (2020). Context-specific control over the neural dynamics of temporal attention by the human cerebellum. Sci. Adv. 6, eabb1141. doi: 10.1126/sciadv.abb1141
Bueti, D., Bahrami, B., Walsh, V., Rees, G. (2010). Encoding of temporal probabilities in the human brain. J. Neurosci. 30, 4343–4352. doi: 10.1523/JNEUROSCI.2254-09.2010
Capizzi, M., Sanabria, D., Correa, Á. (2012). Dissociating controlled from automatic processing in temporal preparation. Cognition 123, 293–302. doi: 10.1016/j.cognition.2012.02.005
Carrasco, M. (2011). Visual attention: the past 25 years. Vis. Res. 51, 1484–1525. doi: 10.1016/j.visres.2011.04.012
Carrasco, M., Williams, P. E., Yeshurun, Y. (2002). Covert attention increases spatial resolution with or without masks: support for signal enhancement. J. Vis. 2, 467–479. doi: 10.1167/2.6.4
Chen, Z. (2012). Object-based attention: a tutorial review. Atten. Percept. Psychophys. 74, 784–802. doi: 10.3758/s13414-012-0322-z
Chun, M. M., Wolfe, J. M. (2001). “Visual attention,” in Blackwell Handbook of Sensation and Perception, ed E. B. Goldstein (Oxford: Blackwell Publishing), 272–310.
Correa, Á. (2010). “Enhancing behavioural performance by visual temporal orienting,” in Attention and time, eds A. C. Nobre, and J. T. Coull (New York, NY: Oxford University Press), 359–370.
Correa, Á., Cappucci, P., Nobre, A. C., Lupiáñez, J. (2010). The two sides of temporal orienting: facilitating perceptual selection, disrupting response selection. Exp. Psychol. 57, 142–148. doi: 10.1027/1618-3169/a000018
Correa, Á., Lupiáñez, J., Madrid, E., Tudela, P. (2006a). Temporal attention enhances early visual processing: a review and new evidence from event-related potentials. Brain Res. 1076, 116–128. doi: 10.1016/j.brainres.2005.11.074
Correa, Á., Lupiáñez, J., Milliken, B., Tudela, P. (2004). Endogenous temporal orienting of attention in detection and discrimination tasks. Percept. Psychophys. 66, 264–278. doi: 10.3758/BF03194878
Correa, Á., Lupiáñez, J., Tudela, P. (2005). Attentional preparation based on temporal expectancy modulates processing at the perceptual level. Psychon. Bull. Rev. 12, 328–334. doi: 10.3758/BF03196380
Correa, Á., Nobre, A. C. (2008). Neural modulation by regularity and passage of time. J. Neurophysiol. 100, 1649–1655. doi: 10.1152/jn.90656.2008
Correa, Á., Sanabria, D., Spence, C., Tudela, P., Lupiáñez, J. (2006b). Selective temporal attention enhances the temporal resolution of visual perception: evidence from a temporal order judgment task. Brain Res. 1070, 202–205. doi: 10.1016/j.brainres.2005.11.094
Cosman, J. D., Lowe, K. A., Zinke, W., Woodman, G. F., Schall, J. D. (2018). Prefrontal control of visual distraction. Curr. Biol. 28, 414–420.e3. doi: 10.1016/j.cub.2017.12.023
Coull, J. T. (2004). fMRI studies of temporal attention: allocating attention within, or towards, time. Cogn. Brain Res. 21, 216–226. doi: 10.1016/j.cogbrainres.2004.02.011
Coull, J. T., Cotti, J., Vidal, F. (2016). Differential roles for parietal and frontal cortices in fixed versus evolving temporal expectations: dissociating prior from posterior temporal probabilities with fMRI. Neuroimage 141, 40–51. doi: 10.1016/j.neuroimage.2016.07.036
Coull, J. T., Frith, C. D., Buchel, C., Nobre, A. C. (2000). Orienting attention in time: behavioural and neuroanatomical distinction between exogenous and endogenous shifts. Neuropsychologia 38, 808–819. doi: 10.1016/S0028-3932(99)00132-3
Coull, J. T., Nobre, A. C. (1998). Where and when to pay attention: the neural systems for directing attention to spatial locations and to time intervals as revealed by both PET and fMRI. J. Neurosci. 18, 7426–7435. doi: 10.1523/JNEUROSCI.18-18-07426.1998
Coull, J. T., Nobre, A. C. (2008). Dissociating explicit timing from temporal expectation with fMRI. Curr. Opin. Neurobiol. 18, 1–8. doi: 10.1016/j.conb.2008.07.011
Cravo, A. M., Rohenkohl, G., Wyart, V., Nobre, A. C. (2013). Temporal expectation enhances contrast sensitivity by phase entrainment of low-frequency oscillations in visual cortex. J. Neurosci. 33, 4002–4010. doi: 10.1523/JNEUROSCI.4675-12.2013
Daume, J., Wang, P., Maye, A., Zhang, D., Engel, A. K. (2021). Non-rhythmic temporal prediction involves phase resets of low-frequency delta oscillations. Neuroimage 224, 117376. doi: 10.1016/j.neuroimage.2020.117376
Davranche, K., Nazarian, B., Vidal, F., Coull, J. T. (2011). Orienting attention in time activates left intraparietal sulcus for both perceptual and motor task goals. J. Cogn. Neurosci. 23, 3318–3330.doi: 10.1162/jocn_a_00030
De la Rosa, M. D., Sanabria, D., Capizzi, M., Correa, Á. (2012). Temporal preparation driven by rhythms is resistant to working memory interference. Front. Psychol. 3, 308. doi: 10.3389/fpsyg.2012.00308
Desimone, R., Duncan, J. (1995). Neural mechanisms of selective visual attention. Ann. Rev. Neurosci. 18, 193–222. doi: 10.1146/annurev.ne.18.030195.001205
Doherty, J., Rao, A., Mesulam, M., Nobre, A. C. (2005). Synergistic effect of combined temporal and spatial expectations on visual attention. J. Neurosci. 25, 8259–8266. doi: 10.1523/JNEUROSCI.1821-05.2005
Downing, C. J. (1988). Expectancy and visual-spatial attention: effects on perceptual quality. J. Exp. Psychol. Hum. Percept. Perform. 14, 188–202. doi: 10.1037/0096-1523.14.2.188
Drazin, D. H. (1961). Effects of foreperiod, foreperiod variability, and probability of stimulus occurrence on simple reaction time. J. Exp. Psychol. 62, 43–50. doi: 10.1037/h0046860
Driver, J., Spence, C. (1998). Crossmodal attention. Curr. Opin. Neurobiol. 8, 245–253. doi: 10.1016/S0959-4388(98)80147-5
Duma, G. M., Granziol, U., Mento, G. (2020). Should I stay or should I go? How local-global implicit temporal expectancy shapes proactive motor control: an hdEEG study. Neuroimage 220, 117071. doi: 10.1016/j.neuroimage.2020.117071
Duma, M., Danieli, A., Morao, V., Da Rold, M., Baggio, M., Toffoli, L., et al. (2021). Implicit cognitive flexibility in self-limited focal epilepsy of childhood: An HD-EEG study. Epilepsy Behav. 116, 107747. doi: 10.1016/j.yebeh.2020.107747
Elbaz, A., Yeshurun, Y. (2020). Can rhythm-induced attention improve the perceptual representation? PLoS ONE 15, e0231200. doi: 10.1371/journal.pone.0231200
Feldmann-Wüstefeld, T., Weinberger, M., Awh, E. (2021). Spatially guided distractor suppression during visual search. J. Neurosci. 41, 3180–3191. doi: 10.1523/JNEUROSCI.2418-20.2021
Frowein, H. W., Sanders, F. (1978). Effects of visual stimulus degradation, S-R cpompatibility, and foreperiod duration on choice reaction time and movement time. Bull. Psychon. Soc. 12, 106–108. doi: 10.3758/BF03329641
Gaspelin, N., Luck, S. J. (2018). Combined electrophysiological and behavioral evidence for the suppression of salient distractors. J. Cogn. Neurosci. 30, 1265–1280. doi: 10.1162/jocn_a_01279
Geng J. J. (2014). Attentional mechanisms of distractor suppression. Curr Dir Psychol Sci. 23, 147–153. doi: 10.1177/0963721414525780
Gibbon, J. (1977). Scalar expectancy theory and Weber's law in animal timing. Psychol. Rev. 84, 279–325. doi: 10.1037/0033-295X.84.3.279
Gottsdanker, R. (1975). “The attaining and maintaining of preparation,” in Attention and Performance, eds P. M. Rabbit, and S. Dornic (Oxford: Academic Press), 33–49.
Gresch, D., Boettcher, S. E., van Ede, F., Nobre, A. C. (2021). Shielding working-memory representations from temporally predictable external interference. Cognition 217, 104915. doi: 10.1016/j.cognition.2021.104915
Griffin, I., Miniussi, C., Nobre, A. C. (2002). Multiple mechanisms of selective attention: differential modulation of stimulus processing by attention to space or time. Neuropsychologia 40, 2325–2340. doi: 10.1016/S0028-3932(02)00087-8
Grubert, A., Eimer, M. (2018). The time course of target template activation processes during preparation for visual search. J. Neurosci. 38, 9527–9538. doi: 10.1523/JNEUROSCI.0409-18.2018
Hackley, S. A., Langner, R., Rolke, B., Erb, M., Grodd, W., Ulrich, R. (2009). Separation of phasic arousal and expectancy effects in a speeded reaction time task via fMRI. Psychophysiology 46, 163–171. doi: 10.1111/j.1469-8986.2008.00722.x
Hackley, S. A., Schankin, A., Wohlschläger, A., Wascher, E. (2007). Localization of temporal preparation effects via trisected reaction time. Psychophysiology 44, 334–338. doi: 10.1111/j.1469-8986.2007.00500.x
Hackley, S. A., Valle-Inclán, F. (2003). Which stages of processing are speeded by a warning signal? Biol. Psychol. 64, 27–45. doi: 10.1016/S0301-0511(03)00101-7
Han, T., Proctor, R. W. (2022). Revisiting variable-foreperiod effects: evaluating the repetition priming account. Atten. Percept. Psychophys. 84, 1193–1207. doi: 10.3758/s13414-022-02476-5
Hansen, J. C., Hillyard, S. A. (1983). Selective attention to multidimensional auditory stimuli. J. Exp. Psychol. Hum. Percept. Perform. 9, 1–19. doi: 10.1037/0096-1523.9.1.1
Herbst, S. K., Obleser, J. (2017). Implicit variations of temporal predictability: shaping the neural oscillatory and behavioural response. Neuropsychologia 101, 141–152. doi: 10.1016/j.neuropsychologia.2017.05.019
Herbst, S. K., Obleser, J. (2019). Implicit temporal predictability enhances pitch discrimination sensitivity and biases the phase of delta oscillations in auditory cortex. Neuroimage 203, 116198. doi: 10.1016/j.neuroimage.2019.116198
Herbst, S. K., Stefanics, G., Obleser, J. (2022). Endogenous modulation of delta phase by expectation–A replication of Stefanics et al., 2010. Cortex 149, 226–245. doi: 10.1016/j.cortex.2022.02.001
Hillyard, S. A., Anllo-Vento, L. (1998). Event-related brain potentials in the study of visual selective attention. Proc. Nat. Acad. Sci. U. S. A 95, 781–787. doi: 10.1073/pnas.95.3.781
Hillyard, S. A., Hink, R. F., Schwent, V. L., Picton, T. W. (1973). Electrical signs of selective attention in the human brain. Science 182, 177–180. doi: 10.1126/science.182.4108.177
Hillyard, S. A., Münte, T. F. (1984). Selective attention to color and location: an analysis with event-related brain potentials. Percept. Psychophys. 36, 185–198. doi: 10.3758/BF03202679
Hopf, J.-M., Boehler, C. N., Schoenfeld, M. A., Mangun, G. R., Heinze, H.-J. (2012). “Attentional selection for locations, features, and objects in vision,” in The Neuroscience of Attention, ed G. R. Mangun (Oxford: Oxford University Press), 3–29.
Hopf, J. M., Boelmans, K., Schoenfeld, M. A., Luck, S. J., Heinze, H. J. (2004). Attention to features precedes attention to locations in visual search: evidence from electromagnetic brain responses in humans. J. Neurosci. 24, 1822–1832. doi: 10.1523/JNEUROSCI.3564-03.2004
Jepma, M., Wagenmakers, E.-J., Nieuwenhuis, S. (2012). Temporal expectation and information processing: a model-based analysis. Cognition 122, 426–441. doi: 10.1016/j.cognition.2011.11.014
Johnston, W. A., Dark, V. J. (1986). Selective attention. Annu. Rev. Psychol. 37, 43–75. doi: 10.1146/annurev.ps.37.020186.000355
Jones, A., Ward, E. V. (2019). Rhythmic temporal structure at encoding enhances recognition memory. J. Cognit. Neurosci. 31, 1549–1562. doi: 10.1162/jocn_a_01431
Karlin, L. (1959). Reaction time as a function of foreperiod duration and variability. J. Exp. Psychol. 58, 185–191. doi: 10.1037/h0049152
Kaskey, G. B., Salzman, L. F., Klorman, R., Pass, H. L. (1980). Relationships between stimulus intensity and amplitude of visual and auditory event related potentials. Biol. Psychol. 10, 115–125. doi: 10.1016/0301-0511(80)90032-0
Kingstone, A. (1992). Combining expectancies. Quart. J. Exp. Psychol. 44A, 69–104. doi: 10.1080/14640749208401284
Klein, R. M., Lawrence, M. A. (2012). “On the modes and domains of attention,” in Cognitive Neuroscience of Attention, 2nd Edn, ed M. Posner (New York, NY: Guilford Press), 11–28.
Klemmer, E. T. (1956). Time uncertainty in simple reaction time. J. Exp. Psychol. 51, 179–184. doi: 10.1037/h0042317
Korolczuk, I., Burle, B., Coull, J. T. (2018). The costs and benefits of temporal predictability: impaired inhibition of prepotent responses accompanies increased activation of task-relevant responses. Cognition 179, 102–110. doi: 10.1016/j.cognition.2018.06.006
Kulkarni, M., Hannula, D. E. (2021). Temporal regularity may not improve memory for item-specific detail. Front. Psychol. 662, 623402. doi: 10.3389/fpsyg.2021.623402
Lakatos, P., Karmos, G., Mehta, A. D., Ulbert, I., Schroeder, C. E. (2008). Entrainment of neuronal oscillations as a mechanism of attentional selection. Science 320, 110–113. doi: 10.1126/science.1154735
Lakatos, P., Musacchia, G., O'Connel, M. N., Falchier, A. Y., Javitt, D. C., Schroeder, C. E. (2013). The spectrotemporal filter mechanism of auditory selective attention. Neuron 77, 750–761. doi: 10.1016/j.neuron.2012.11.034
Lampar, A., Lange, K. (2011). Effects of temporal trial-by-trial cuing on early and late stages of auditory processing: evidence from event-related potentials. Atten. Percept. Psychophys. 73, 1916–1933. doi: 10.3758/s13414-011-0149-z
Lamy, D. (2005). Temporal expectations modulate attentional capture. Psychon. Bull. Rev. 12, 1112–1119. doi: 10.3758/BF03206452
Lange, K. (2009). Brain correlates of early auditory processing are attenuated by expectations for time and pitch. Brain Cogn. 69, 127–137. doi: 10.1016/j.bandc.2008.06.004
Lange, K. (2012). The N1 effect of temporal attention is independent of sound location and intensity: implications for possible mechanisms of temporal attention. Psychophysiology 49, 1468–1480. doi: 10.1111/j.1469-8986.2012.01460.x
Lange, K. (2013). The ups and downs of temporal orienting: a review of auditory temporal orienting studies and a model associating the heterogeneous findings on the auditory N1 with opposite effects of attention and prediction. Front. Hum. Neurosci. 7, 1–14. doi: 10.3389/fnhum.2013.00263
Lange, K., Krämer, U. M., Röder, B. (2006). Attending points in time and space. Exp. Brain Res. 173, 130–140. doi: 10.1007/s00221-006-0372-3
Lange, K., Röder, B. (2006). Orienting attention to points in time improves stimulus processing both within and across modalities. J. Cogn. Neurosci. 18, 715–729. doi: 10.1162/jocn.2006.18.5.715
Lange, K., Röder, B. (2010). “Orienting attention in audition, touch, and across modalities,” in Attention and Time, eds A. C. Nobre, and J. T. Coull (New York, NY: Oxford University Press), 393–406.
Lange, K., Rösler, F., Röder, B. (2003). Early processing stages are modulated when auditory stimuli are presented at an attended moment in time: an event-related potential study. Psychophysiology 40, 806–817. doi: 10.1111/1469-8986.00081
Lawrence, M., Klein, R. M. (2013). Isolating exogenous and endogenous modes of temporal attention. J. Exp. Psychol. Gen. 142, 560–572. doi: 10.1037/a0029023
Ling, S., Liu, T., Carrasco, M. (2009). How spatial and feature-based attention affect the gain and tuning of population responses. Vis. Res. 49, 1194–1204. doi: 10.1016/j.visres.2008.05.025
Los S. A. (2004). Inhibition of return and nonspecific preparation: Separable inhibitory control mechanisms in space and time. Percept Psychophys. 66, 119–130. doi: 10.3758/BF03194866
Los, S. A. (2010). “Foreperiod and the sequential effect: theory and data,” in Attention and Time, eds A. C. Nobre, and J. T. Coull (Oxford: Oxford University Press), 289–302.
Los, S. A., Heslenfeld, D. J. (2005). Intentional and unintentional contributions to nonspecific preparation: electrophysiological evidence J. Exp. Psychol. Gen. 134, 52–72. doi: 10.1037/0096-3445.134.1.52
Los, S. A., Horoufchin, H. (2011). Dissociative patterns of foreperiod effects in temporal discrimination and reaction time tasks. Quart. J. Exp. Psychol. 64, 1009–1020. doi: 10.1080/17470218.2010.532225
Los, S. A., Knot, D. L., Boers, R. M. (2001). The foreperiod effect revisited: conditioning as a basis for nonspecific preparation. Acta Psychol. 106, 121–145. doi: 10.1016/S0001-6918(00)00029-9
Los, S. A., Kruijne, W., Meeter, M. (2014). Outlines of a multiple trace theory of temporal preparation. Front. Psychol. 5, 1058. doi: 10.3389/fpsyg.2014.01058
Los, S. A., Kruijne, W., Meeter, M. (2017). Hazard versus history: temporal preparation is driven by past experience. J. Exp. Psychol. Hum. Percept. Perform. 43, 78–88. doi: 10.1037/xhp0000279
Los, S. A., Schut, M. L. (2008). The effective time course of preparation. Cogn. Psychol. 57, 20–55. doi: 10.1016/j.cogpsych.2007.11.001
Los, S. A., Van den Heuvel, C. E. (2001). Intentional and unintentional contributions to nonspecific preparation during reaction time foreperiods. J. Exp. Psychol. Hum. Percept. Perform. 27, 370–386. doi: 10.1037/0096-1523.27.2.370
MacKay, A., Juola, J. F. (2007). Are spatial and temporal attention independent? Percept. Psychophys. 69, 972–979. doi: 10.3758/BF03193935
Makeig, S., Westerfield, M., Jung, T. P., Enghoff, S., Townsend, J., Courchesne, E., et al. (2002). Dynamic brain sources of visual evoked responses. Science 295, 690–694. doi: 10.1126/science.1066168
Martens, S., Johnson, A. (2005). Timing attention: cueing target onset interval attenuates the attentional blink. Mem. Cogn. 33, 234–240. doi: 10.3758/BF03195312
Mathewson, K. E., Fabiani, M., Gratton, G., Beck, D. M., Lleras, A. (2010). Rescuing stimuli from invisibility: inducing a momentary release from visual masking with pre-target Entrainment. Cognition 115, 186–191. doi: 10.1016/j.cognition.2009.11.010
Mattes, S., Ulrich, R. (1997). Response force is sensitive to the temporal uncertainty of response stimuli. Percept. Psychophys. 59, 1089–1097. doi: 10.3758/BF03205523
Matthias, E., Bublak, P., Müller, H. J., Schneider, W. X., Krummenacher, J., Finke, K. (2010). The influence of alertness on spatial and nonspatial components of visual attention. J. Exp. Psychol. Hum. Percept. Perform. 36, 38–56. doi: 10.1037/a0017602
Mattiesing, R. M., Kruijne, W., Meeter, M., Los, S. A. (2017). Timing a week later: the role of long-term memory in temporal preparation. Psychon. Bull. Rev. 24, 1900–1905. doi: 10.3758/s13423-017-1270-3
Menceloglu, M., Grabowecky, M., Suzuki, S. (2017). Comparing the effects of implicit and explicit temporal expectation on choice response time and response conflict. Atten. Percept. Psychophys. 79, 169–179. doi: 10.3758/s13414-016-1230-4
Menceloglu, M., Grabowecky, M., Suzuki, S. (2019). Probability-driven and stimulus-driven orienting of attention to time and sensory modality. Atten. Percept. Psychophys. 81, 2732–2744. doi: 10.3758/s13414-019-01798-1
Menceloglu, M., Grabowecky, M., Suzuki, S. (2021). Revealing the effects of temporal orienting of attention on response conflict using continuous movements. Atten. Percept. Psychophys. 83, 1463–1478. doi: 10.3758/s13414-020-02235-4
Mento, G. (2017). The role of the P3 and CNV components in voluntary and automatic temporal orienting: a high spatial-resolution ERP study. Neuropsychologia 107, 31–40. doi: 10.1016/j.neuropsychologia.2017.10.037
Mento, G., Granziol, U. (2020). The developing predictive brain: how implicit temporal expectancy induced by local and global prediction shapes action preparation across development. Dev. Sci. 23, e12954. doi: 10.1111/desc.12954
Mento, G., Scerif, G., Granziol, U., Franzoi, M., Lanfranchi, S. (2019). Dissociating top-down and bottom-up temporal attention in Down syndrome: a neuroconstructive perspective. Cogn. Dev. 49, 81–93. doi: 10.1016/j.cogdev.2018.12.004
Mento, G., Tarantino, V. (2015). Developmental trajectories of internally and externally driven temporal prediction. PLoS ONE 10, e0135098. doi: 10.1371/journal.pone.0135098
Mento, G., Tarantino, V., Vallesi, A., Bisiacchi, P. S. (2015). Spatiotemporal neurodynamics underlying internally and externally driven temporal prediction: a high spatial resolution ERP study. J. Cogn. Neurosci. 27, 425–439. doi: 10.1162/jocn_a_00715
Milliken, B., Lupiáñez, J., Roberts, M., Stevanovski, B. (2003). Orienting in space and time: Joint contributions to exogenous spatial cuing effects. Psychon. Bull. Rev. 10, 877–883. doi: 10.3758/BF03196547
Miniussi, C., Wilding, E. L., Coull, J. T., Nobre, A. C. (1999). Orienting attention in time: Modulation of brain potentials. Brain 122, 1507–1518. doi: 10.1093/brain/122.8.1507
Mishra, J., Martínez, A., Schroeder, C. E., Hillyard, S. A. (2012). Spatial attention boosts short-latency neural responses in human visual cortex. Neuroimage 59, 1968–1978. doi: 10.1016/j.neuroimage.2011.09.028
Mühlberg, S., Oriolo, G., Soto-Faraco, S. (2014). Cross-modal decoupling in temporal attention. Eur. J. Neurosci. 39, 2089–2097. doi: 10.1111/ejn.12563
Mühlberg, S., Soto-Faraco, S. (2019). Cross-modal decoupling in temporal attention between audition and touch. Psychol. Res. 83, 1626–1639. doi: 10.1007/s00426-018-1023-6
Müller-Gethmann, H., Ulrich, R., Rinkenauer, G. (2003). Locus of the effect of temporal preparation: evidence from the lateralized readiness potential. Psychophysiology 40, 597–611. doi: 10.1111/1469-8986.00061
Näätänen, R. (1971). Non-aging foreperiods and simple reaction time. Acta Psychol. 35, 316–327. doi: 10.1016/0001-6918(71)90040-0
Näätänen, R., Merisalo, A. (1977). “Expectancy and preparation in simple reaction time,” in Attention and Performance VI, ed S. Dornic (Hillsdale, NJ: Lawrence Erlbaum), 115–138.
Nickerson, R. S., Burnham, D. W. (1969). Response times with nonaging foreperiods. J. Exp. Psychol. 79, 452–457. doi: 10.1037/h0026889
Niemi, P., Lehtonen, R. (1982). Foreperiod and visual stimulus intensity: a reappraisal. Acta Psychol. 50, 73–82. doi: 10.1016/0001-6918(82)90052-X
Niemi, P., Näätänen, R. (1981). Foreperiod and simple reaction time. Psychol. Bull. 89, 133–162. doi: 10.1037/0033-2909.89.1.133
Nieuwenstein, M. R., Chun, M. M., Van der Lubbe, R. H. J., Hooge, I. T. C. (2005). Delayed attentional engagement in the attentional blink. J. Exp. Psychol. Hum. Percept. Perform. 31, 1463–1475. doi: 10.1037/0096-1523.31.6.1463
Nissen, M. J. (1977). Stimulus intensity and information processing. Percept. Psychophys. 22, 338–352. doi: 10.3758/BF03199699
Nobre, A. C., Correa, A., Coull, J. T. (2007). The hazards of time. Curr. Opin. Neurobiol. 17, 465–470. doi: 10.1016/j.conb.2007.07.006
Nobre, A. C., Rohenkohl, G. (2014). “Time for the fourth dimension in attention,” in The Oxford Handbook of Attention, eds A. C. Nobre, and S. Kastner (Oxford: Oxford University Press), 676–724.
Nobre, A. C., Van Ede, F. (2018). Anticipated moments: temporal structure in attention. Nat. Rev. Neurosci. 19, 34–48. doi: 10.1038/nrn.2017.141
Olk, B. (2014). Effects of spatial, temporal and spatiotemporal cueing are alike when attention is directed voluntarily. Exp. Brain Res. 232, 3623–3633. doi: 10.1007/s00221-014-4033-7
Park, J., Schlag-Rey, M., Schlag, J. (2003) Spatial localization precedes temporal determination in visual perception. Vis Res. 43, 1667–1674. doi: 10.1016/S0042-6989(03)00217-7
Posner, M. I., Klein, R., Summers, J., Buggies, S. (1973). On the selection of signals. Mem. Cogn. 1, 2–12. doi: 10.3758/BF03198062
Posner, M. I., Petersen, S. E. (1990). The attention system of the human brain. Ann. Rev. Neurosci. 13, 25–42. doi: 10.1146/annurev.ne.13.030190.000325
Raab, D., Fehrer, E., Hershenson, M. (1961). Visual reaction time and the Broca-Sulzer phenomenon. J. Exp. Psychol. 6, 193–199. doi: 10.1037/h0044854
Repp, B. H., Penel, A. (2002). Auditory dominance in temporal processing: new evidence from synchronization with simultaneous visual and auditory sequences. J. Exp. Psychol. Hum. Percept. Perform. 28, 1085–1099. doi: 10.1037/0096-1523.28.5.1085
Rimmele, J., Jolsvai, H., Sussman, E. (2011). Auditory target detection is affected by implicit temporal and spatial expectations. J. Cogn. Neurosci. 23, 1136–1147. doi: 10.1162/jocn.2010.21437
Ristic, J., Kingstone, A. (2006). Attention to arrows: pointing to a new direction. Quart. J. Exp. Psychol. 59, 1921–1930. doi: 10.1080/17470210500416367
Rohenkohl, G., Coull, J. T., Nobre, A. C. (2011). Behavioural dissociation between exogenous and endogenous temporal orienting of attention. PLoS ONE 6, e14620. doi: 10.1371/journal.pone.0014620
Rohenkohl, G., Cravo, A. M., Wyart, V., Nobre, A. C. (2012). Temporal expectation improves the quality of sensory information. J. Neurosci. 32, 8424–8428. doi: 10.1523/JNEUROSCI.0804-12.2012
Rohenkohl, G., Gould, I. C., Pessoa, J., Nobre, A. C. (2014). Combining spatial and temporal expectations to improve visual perception. J. Vis. 14, 8. doi: 10.1167/14.4.8
Rohenkohl, G., Nobre, A. C. (2011). Alpha oscillations related to anticipatory attention follow temporal expectations. J. Neurosci. 31, 14076–14084. doi: 10.1523/JNEUROSCI.3387-11.2011
Rolke, B. (2008). Temporal preparation facilitates perceptual identification of letters. Percept. Psychophys. 70, 1305–1313. doi: 10.3758/PP.70.7.1305
Rolke, B., Festl, F., Seibold, V. C. (2016). Toward the influence of temporal attention on the selection of targets in a visual search task: an ERP study. Psychophysiology 53, 1690–1701. doi: 10.1111/psyp.12734
Rolke, B., Hofmann, P. (2007). Temporal uncertainty degrades perceptual processing. Psychon. Bull. Rev. 14, 522–526. doi: 10.3758/BF03194101
Rolke, B., Ulrich, R. (2010). “On the locus of temporal preparation: enhancement of pre-motor processes?,” in Attention and Time, eds A. C. Nobre, and J. T. Coull (Oxford: University Press), 227–241.
Rudell, A. P., Hu, B. (2001). Does a warning signal accelerate the processing of sensory information? Evidence from recognition potential responses to high and low frequency words. Int. J. Psychophysiol. 41, 31–42. doi: 10.1016/S0167-8760(00)00174-4
Salet, J. M., Kruijne, W., van Rijn, H., Los, S. A. (2022). fMTP: a unifying computational framework of temporal preparation across time scales. Psychol. Rev. 129, 911–948. doi: 10.1037/rev0000356
Samaha, J., Bauer, P., Cimaroli, S., Postle, B. R. (2015). Top-down control of the phase of alpha-band oscillations as a mechanism for temporal prediction. Proc. Natl. Acad. Sci. U. S. A. 112, 8439–8444. doi: 10.1073/pnas.1503686112
Sanabria, D., Capizzi, M., Correa, Á. (2011). Rhythms that speed you up. J. Exp. Psychol. Hum. Percept. Perform. 37, 236–244. doi: 10.1037/a0019956
Sanders, A. F. (1980). “Some effects of instructed muscle tension on choice reaction time and movement time,” in Attention and Performance VIII, ed R. Nickerson (Hillsdale, NJ: Lawrence Erlbaum), 59–74.
Sanders, L. D., Astheimer, L. B. (2008). Temporally selective attention modulates early perceptual processing: event-related potential evidence. Percept. Psychophys. 70, 732–742. doi: 10.3758/PP.70.4.732
Scharlau, I. (2004). Evidence against response bias in temporal order tasks with attention manipulation by masked primes. Psychol. Res. 68, 224–236. doi: 10.1007/s00426-003-0135-8
Schroeder, C. E., Lakatos, P. (2009). Low-frequency neuronal oscillations as instruments of sensory selection. Trends Neurosci. 32, 9–18. doi: 10.1016/j.tins.2008.09.012
Seibold, V. C., Bausenhart, K. M., Rolke, B., Ulrich, R. (2011a). Does temporal preparation increase the rate of sensory information accumulation? Acta Psychol. 137, 56–64. doi: 10.1016/j.actpsy.2011.02.006
Seibold, V. C., Dietrich, S., Rolke, B. (2019). Multidimensional selection by temporal attention and feature-based attention: evidence from event-related potentials. Brain Res. 1722, 146340. doi: 10.1016/j.brainres.2019.146340
Seibold, V. C., Fiedler, A., Rolke, B. (2011b). Temporal attention shortens perceptual latency: a temporal prior entry effect. Psychophysiology 48, 708–717. doi: 10.1111/j.1469-8986.2010.01135.x
Seibold, V. C., Rolke, B. (2014a). Does temporal preparation facilitate visual processing in a selective manner? Evidence from attentional capture. Acta Psychol. 151, 51–61. doi: 10.1016/j.actpsy.2014.05.012
Seibold, V. C., Rolke, B. (2014b). Does temporal preparation speed up visual processing? Evidence from the N2pc. Psychophysiology 51, 529–538. doi: 10.1111/psyp.12196
Seibold, V. C., Stepper, M. Y., Rolke, B. (2020). Temporal attention boosts perceptual effects of spatial attention and feature-based attention. Brain Cogn. 142, 105570. doi: 10.1016/j.bandc.2020.105570
Seifried, T., Ulrich, R., Bausenhart, K. M., Rolke, B., Osman, A. (2010). Temporal preparation decreases perceptual latency: evidence from a clock paradigm. Quart. J. Exp. Psychol. 63, 2432–2451. doi: 10.1080/17470218.2010.485354
Seiss, E., Kiss, M., Eimer, M. (2009). Does focused endogenous attention prevent attentional capture in pop-out visual search? Psychophysiology 46, 703–717. doi: 10.1111/j.1469-8986.2009.00827.x
Shen, D., Alain, C. (2011). Temporal attention facilitates short-term consolidation during a rapid serial auditory presentation task. Exp. Brain Res. 215, 285–292. doi: 10.1007/s00221-011-2897-3
Solís-Vivanco, R., Jensen, O., Bonnefond, M. (2018). Top–down control of alpha phase adjustment in anticipation of temporally predictable visual stimuli. J. Cogn. Neurosci. 30, 1157–1169. doi: 10.1162/jocn_a_01280
Spaak, E., de Lange, F. P., Jensen, O. (2014). Local entrainment of alpha oscillations by visual stimuli causes cyclic modulation of perception. J. Neurosci. 34, 3536–3544. doi: 10.1523/JNEUROSCI.4385-13.2014
Spence, C., Parise, C. (2010). Prior entry: a review. Conscious. Cogn. 19, 364–379. doi: 10.1016/j.concog.2009.12.001
Stefanics, G., Hangya, B., Hernadi, I., Winkler, I., Lakatos, P., Ulbert, I. (2010). Phase entrainment of human Delta oscillations can mediate the effects of expectation on reaction speed. J. Neurosci. 30, 13578–13585. doi: 10.1523/JNEUROSCI.0703-10.2010
Steinborn, M., Rolke, B., Bratzke, D., Ulrich, R. (2008). Sequential effects within a short foreperiod context: further support for the conditioning account of temporal preparation. Acta Psychol. 129, 297–307. doi: 10.1016/j.actpsy.2008.08.005
Steinborn, M. B., Rolke, B., Bratzke, D., Ulrich, R. (2010). The effect of a cross-trial shift of auditory warning signals on the sequential foreperiod effect. Acta Psychol. 134, 94–104. doi: 10.1016/j.actpsy.2009.12.011
Summerfield, C., Egner, T. (2009). Expectation (and attention) in visual cognition. Trends Cogn. Sci. 13, 403–409. doi: 10.1016/j.tics.2009.06.003
Tal-Perry, N., Yuval-Greenberg, S. (2022). The spatiotemporal link of temporal expectations: contextual temporal expectation is independent of spatial attention. J. Neurosci. 42, 2516–2523. doi: 10.1523/JNEUROSCI.1555-21.2022
Teichner, W. H. (1954). Recent studies of simple reaction time. Psychol. Bull. 51, 128–149. doi: 10.1037/h0060900
Ten Oever, S., Schroeder, C. E., Poeppel, D., Van Atteveldt, N., Zion-Golumbic, E. (2014). Rhythmicity and cross-modal temporal cues facilitate detection. Neuropsychologia 63, 43–50. doi: 10.1016/j.neuropsychologia.2014.08.008
Thomaschke, R., Bogon, J., Dreisbach, G. (2018). Timing affect: dimension-specific time-based expectancy for affect. Emotion 18, 646–669. doi: 10.1037/emo0000380
Thomaschke, R., Dreisbach, G. (2013). Temporal predictability facilitates action, not perception. Psychol. Sci. 24, 1335–1340. doi: 10.1177/0956797612469411
Thomaschke, R., Dreisbach, G. (2015). The time-event correlation effect is due to temporal expectancy, not to partial transition costs. J. Exp. Psychol. Hum. Percept. Perform. 41, 196–218. doi: 10.1037/a0038328
Titchener, E. B. (1908). Lectures on the Elementary Psychology of Feeling and Attention. New York, NY: Macmillan.
Todorovic, A., Schoffelen, J. M., Van Ede, F., Maris, E., De Lange, F. P. (2015). Temporal expectation and attention jointly modulate auditory oscillatory activity in the beta band. PLoS ONE 10, e0120288. doi: 10.1371/journal.pone.0120288
Treisman, M. (1964). The effect of one stimulus on the threshold for another: an application of signal detectability theory. Br. J. Stat. Psychol. 17, 15–35. doi: 10.1111/j.2044-8317.1964.tb00241.x
Triviño, M., Arnedo, M., Lupiánez, J., Chirivella, J., Correa, Á. (2011). Rhythms can overcome temporal orienting deficit after right frontal damage. Neuropsychologia 49, 3917–3930. doi: 10.1016/j.neuropsychologia.2011.10.009
Triviño, M., Correa, Á., Lupiáñez, J., Funes, M. J., Catena, A., He, X., et al. (2016). Brain networks of temporal preparation: a multiple regression analysis of neuropsychological data. Neuroimage 142, 489–497. doi: 10.1016/j.neuroimage.2016.08.017
Vallesi, A., Shallice, T. (2007). Developmental dissociations of preparation over time: deconstructing the variable foreperiod phenomena. J. Exp. Psychol. Hum. Percept. Perform. 33, 1377–1388. doi: 10.1037/0096-1523.33.6.1377
Van der Lubbe, R. H., Los, S. A., Jaśkowski, P., Verleger, R. (2004). Being prepared on time: on the importance of the previous foreperiod to current preparation, as reflected in speed, force and preparation-related brain potentials. Acta Psychol. 116, 245–262. doi: 10.1016/j.actpsy.2004.03.003
Van Diepen, R. M., Foxe, J. J., Mazaheri, A. (2019). The functional role of alpha-band activity in attentional processing: the current zeitgeist and future outlook. Curr. Opin. Psychol. 29, 229–238. doi: 10.1016/j.copsyc.2019.03.015
Van Ede, F., De Lange, F., Jensen, O., Maris, E. (2011). Orienting attention to an upcoming tactile event involves a spatially and temporally specific modulation of sensorimotor alpha-and beta-band oscillations. J. Neurosci. 31, 2016–2024. doi: 10.1523/JNEUROSCI.5630-10.2011
Vangkilde, S., Coull, J. T., Bundesen, C. (2012). Great expectations: temporal expectation modulates perceptual processing speed. J. Exp. Psychol. Hum. Percept. Perform. 38, 1183–1191. doi: 10.1037/a0026343
Vibell, J., Klinge, C., Zampini, M., Spence, C., Nobre, A. C. (2007). Temporal order is coded temporally in the brain: early event-related potential latency shifts underlying prior entry in a cross-modal temporal order judgment task. J. Cogn. Neurosci. 19, 109–120. doi: 10.1162/jocn.2007.19.1.109
Wagener, A., Hoffmann, J. (2010). Temporal cueing of target-identity and target-location. Exp. Psychol. 57, 436–445. doi: 10.1027/1618-3169/a000054
Warren, S., Yacoub, E., Ghose, G. (2014). Featural and temporal attention selectively enhance task-appropriate representations in human primary visual cortex. Nat. Commun. 5, 5643. doi: 10.1038/ncomms6643
Weinbach, N., Henik, A. (2012). Temporal orienting and alerting – the same or different? Front. Psychol. 3, 236. doi: 10.3389/fpsyg.2012.00236
Weinbach, N., Henik, A. (2013). The interaction between alerting and executive control: dissociating phasic arousal and temporal expectancy. Atten. Percept. Psychophys. 75, 1374–1381. doi: 10.3758/s13414-013-0501-6
Weiß, K., Scharlau, I. (2012). At the mercy of prior entry: prior entry induced by invisible primes is not susceptible to current intentions. Acta Psychol. 139, 54–64. doi: 10.1016/j.actpsy.2011.10.007
Wilsch, A., Mercier, M. R., Obleser, J., Schroeder, C. E., Haegens, S. (2020). Spatial attention and temporal expectation exert differential effects on visual and auditory discrimination. J. Cogn. Neurosci. 32, 1562–1576. doi: 10.1162/jocn_a_01567
Xu, Z., Los, S. A., Theeuwes, J. (2021). Attentional suppression in time and space. J. Exp. Psychol. Hum. Percept. Perform. 47, 1056–1062. doi: 10.1037/xhp0000925
Keywords: temporal attention, foreperiod, perceptual processing, temporal cueing, entrainment, cross-modal processing, spatial attention, feature-based attention
Citation: Seibold VC, Balke J and Rolke B (2023) Temporal attention. Front. Cognit. 2:1168320. doi: 10.3389/fcogn.2023.1168320
Received: 17 February 2023; Accepted: 24 March 2023;
Published: 17 April 2023.
Edited by:
Dariusz Asanowicz, Jagiellonian University, PolandCopyright © 2023 Seibold, Balke and Rolke. This is an open-access article distributed under the terms of the Creative Commons Attribution License (CC BY). The use, distribution or reproduction in other forums is permitted, provided the original author(s) and the copyright owner(s) are credited and that the original publication in this journal is cited, in accordance with accepted academic practice. No use, distribution or reproduction is permitted which does not comply with these terms.
*Correspondence: Verena C. Seibold, verena.seibold@uni-tuebingen.de