- 1Negaunee Institute for Plant Conservation Science and Action, Chicago Botanic Garden, Glencoe, IL, United States
- 2Southeastern Grasslands Institute, Clarksville, TN, United States
- 3Plant Biology and Conservation, Northwestern University, Evanston, IL, United States
- 4Ecology Center and Department of Watershed Sciences, Utah State University, Logan, UT, United States
- 5Department of Chemistry, Northwestern University, Evanston, IL, United States
- 6Department of Natural Resource Management, South Dakota State University, Brookings, SD, United States
The U.N. Decade on Ecosystem Restoration aims to accelerate actions to prevent, halt, and reverse the degradation of ecosystems, and re-establish ecosystem functioning and species diversity. The practice of ecological restoration has made great progress in recent decades, as has recognition of the importance of species diversity to maintaining the long-term stability and functioning of restored ecosystems. Restorations may also focus on specific species to fulfill needed functions, such as supporting dependent wildlife or mitigating extinction risk. Yet even in the most carefully planned and managed restoration, target species may fail to germinate, establish, or persist. To support the successful reintroduction of ecologically and culturally important plant species with an emphasis on temperate grasslands, we developed a tool to diagnose common causes of missing species, focusing on four major categories of filters, or factors: genetic, biotic, abiotic, and planning & land management. Through a review of the scientific literature, we propose a series of diagnostic tests to identify potential causes of failure to restore target species, and treatments that could improve future outcomes. This practical diagnostic tool is meant to strengthen collaboration between restoration practitioners and researchers on diagnosing and treating causes of missing species in order to effectively restore them.
1 Introduction
The world is experiencing an unprecedented loss of natural ecosystems (IPBES, 2019). In response, the United Nations proclaimed 2021-2030 the Decade on Ecosystem Restoration, recognizing the key role that ecological restoration plays in reversing the worldwide degradation of ecosystems and maintaining the long-term sustainability of our planet (United Nations, 2019). The practice of ecological restoration requires a high degree of ecological knowledge that can be drawn from weaving together available practitioner experience, Traditional Ecological Knowledge, Local Ecological Knowledge, and scientific discovery (Gann et al., 2019). Spontaneous, or passive, ecological restoration allows degraded ecosystems to recover without intervention following the removal of a disturbance and can be effective in some cases (Jones et al., 2018), but is often insufficient for reestablishing the target community. For example, the restoration site may have a soil bank depleted of propagules, limiting opportunities for a diverse native plant community to regenerate (Lamb et al., 2022), or the level of fragmentation may limit seed dispersal and colonization by desirable species from nearby natural habitats (Prach and Hobbs, 2008). This is the case for the tallgrass prairie ecosystem in North America, where fragmentation is high and regeneration without human assistance is unlikely unless robust remnant populations are adjacent (Kindscher and Tieszen, 1998). In most cases, active restoration of plant diversity is required via the deliberate reintroduction of native species that have been lost (McDonald et al., 2016).
When restoring ecosystems, a reference community with similar environmental (e.g., climate, soil, hydrology) conditions is often used to identify the pool of species to introduce at a restoration site (Cornell and Harrison, 2014; Durbecq et al., 2020). Depending on the goals of the restoration (e.g., to restore ecosystem function), it may not be necessary to have the exact complement of species or species combinations found in the reference community. Restoration practitioners often wish to include certain species based on their conservation value or ability to support ecosystem functions such as soil stabilization or pollination. However, even when germplasm for these target species is available, is introduced into a restoration site that appears to be suitable, and diligent efforts are made, some species may not germinate, establish, or persist (Barak et al., 2017). The inability of a target species to thrive at a restoration site is typically a symptom of one or more interacting factors, including genetics of the plant materials, biotic, and abiotic conditions at the site, and planning and management activities (Figure 1), all of which can be challenging to diagnose, let alone treat (Godefroid et al., 2011).
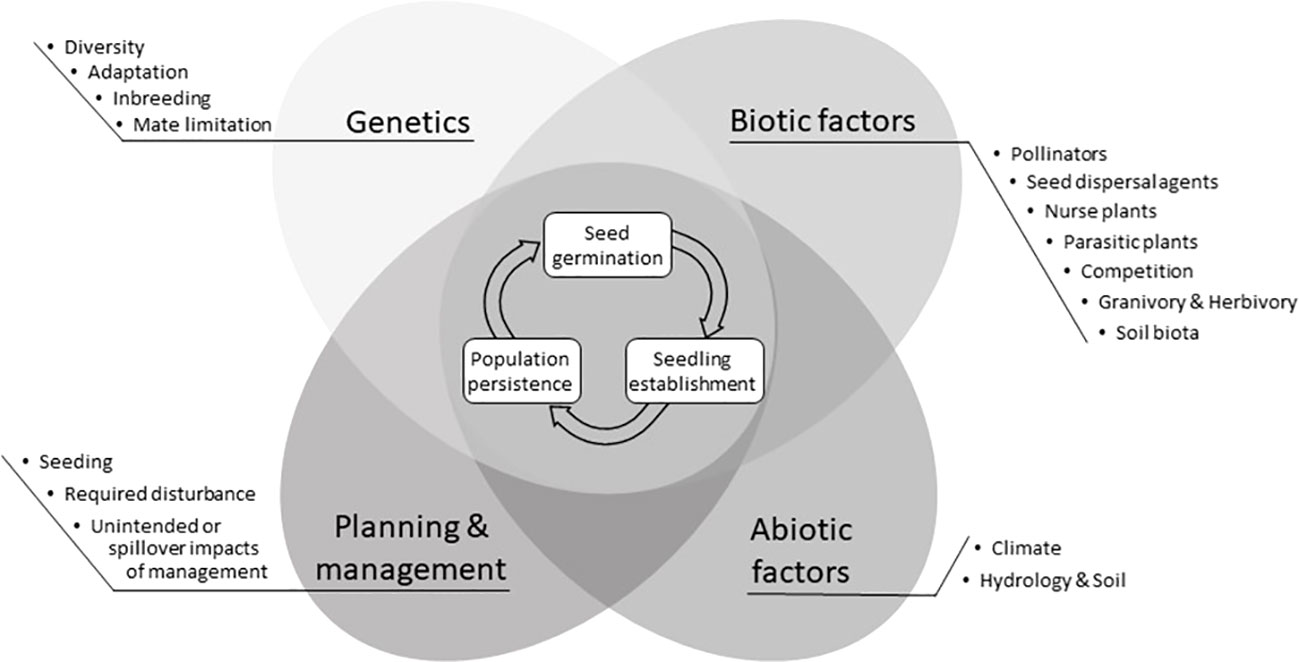
Figure 1 Major factors that may impede germination, establishment, or persistence of the target species at the restoration site. Each factor is grouped into four categories and has a corresponding explanation within the text on how to diagnose and treat the factor. Questions about restoration sites and target species intended to help readers focus their attention on one or more of these different sections are located in Table 1.
Many species seeded into grasslands do not establish: in studies of the establishment of restored prairie species, one quarter to half of planted species were never found in subsequent surveys (Foster et al., 2007; Hillhouse and Zedler, 2011; Barak et al., 2017). Establishment is often hindered by filters such as seed dispersal, seeding density, and environmental conditions at the restoration site, or a combination of factors (Grman et al., 2015). For example, native Viola species in tallgrass prairies of the USA are restoration-relevant because they are the larval host of the threatened Regal Fritillary butterfly (Speyeria idalia; Selby, 2007). Unfortunately, populations of Viola have rarely been successfully reintroduced (see Supplementary Information) despite using locally-sourced plant material installed in sites that harbor seemingly appropriate conditions and native plant communities. The plants fail to germinate, establish, or persist, hindering self-sustaining populations which in turn limits their ability to provide ecosystem services such as support for butterfly populations.
There are numerous factors acting on seed germination, seedling establishment, and population persistence which may influence the occurrence of target species at restoration sites (Figure 1). To diagnose why a species is not present at a site that appears appropriate, practitioners and researchers must work together to understand which factors act as bottlenecks, at what stage these bottlenecks occur, and to design and test measures to overcome or “treat” these bottlenecks. In this practical review, we use temperate grasslands and associated vascular plant species as a model to provide a framework for identifying and addressing bottlenecks.
To begin to diagnose the underlying factors limiting successful restoration outcomes, we suggest measures to fully identify the issues (Figure 2) beginning with a literature review of the target species. Next, it is critical to survey healthy native populations of the target species, as close to the restoration site as possible, and talk with experts who have worked with the target species. Collaboration between researchers and practitioners knowledgeable on the subject is crucial and will continue to shrink the science-practice divide (Ladouceur et al., 2022) critical to improving restoration outcomes. Practitioners should ensure baseline assumptions about the target species and the restoration site are met. We provide a tool to help determine which factors may contribute to the absence of the target species at a restoration site (Table 1), as well as diagnostic tests, and possible treatments after the discussion of each factor.
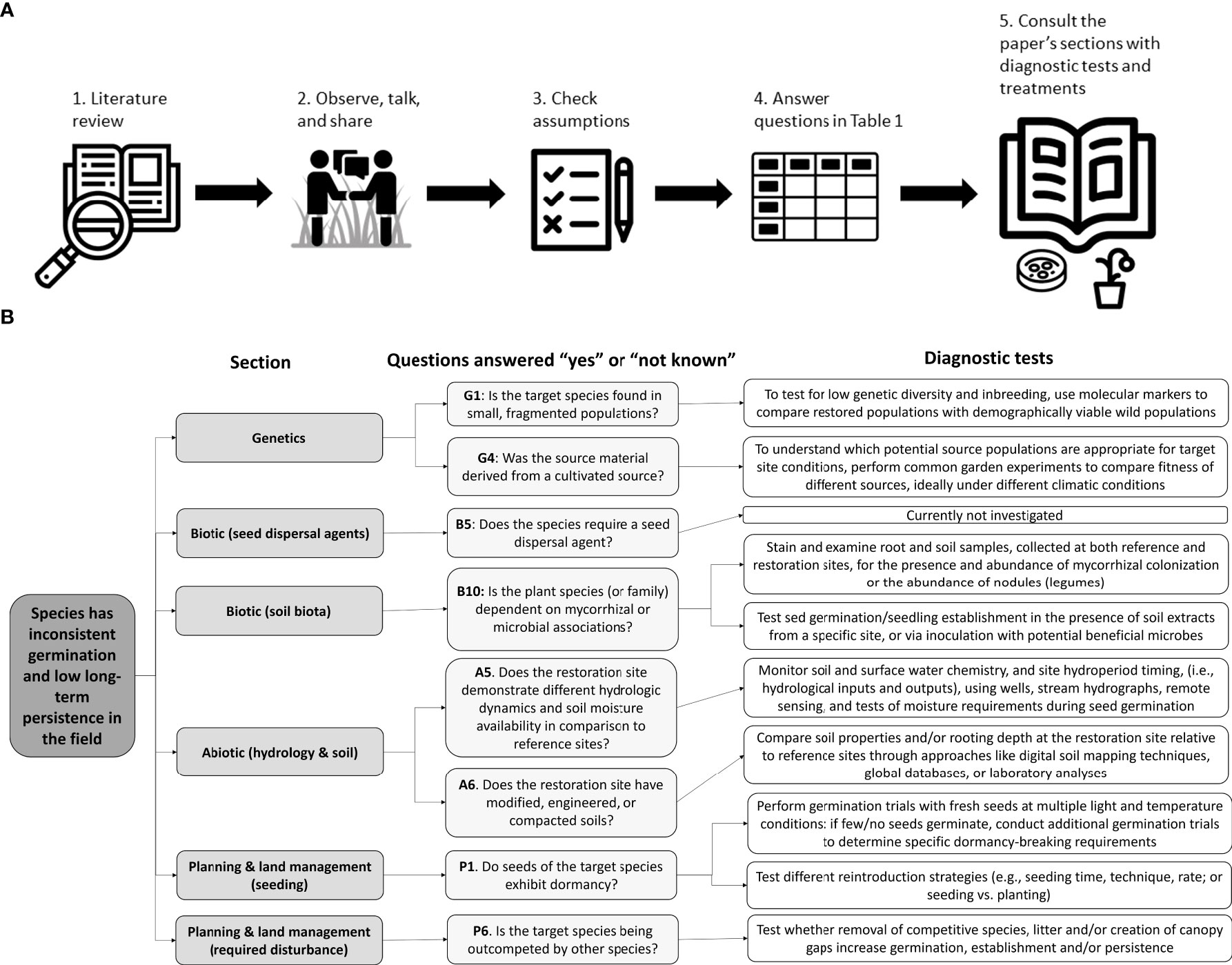
Figure 2 (A) Key steps of our diagnostic framework for identifying restoration bottlenecks starting with a literature review to a roadmap of potential solutions (Table 1), followed by (B) an example of how to use the diagnostic framework laid out in Table 1 for conservative violet species, identifying the section, specific questions answered “yes” or “don’t know”, and related diagnostic tests applied. See Supplementary Information for more details about this violet case study.
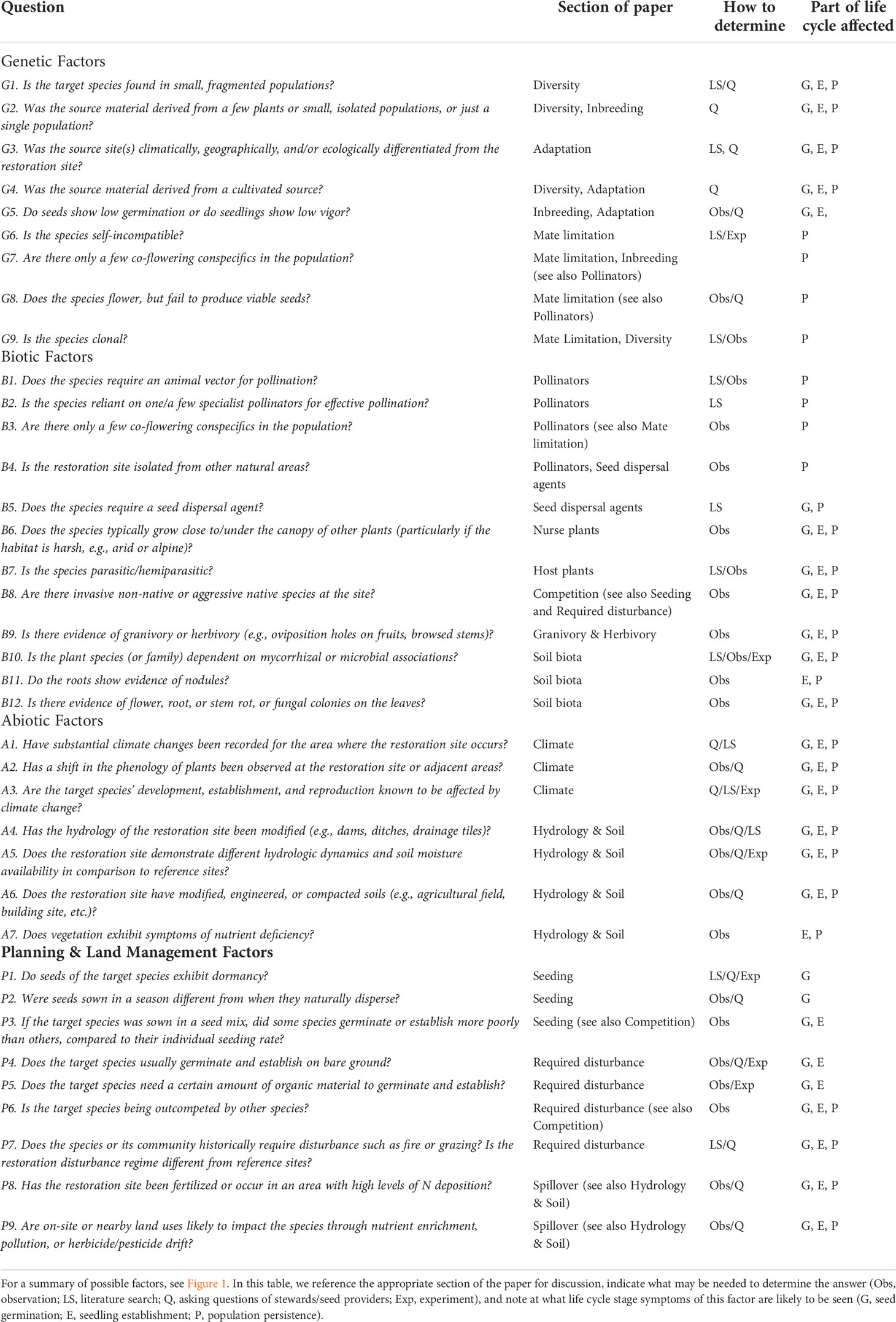
Table 1 List of questions to identify possible reasons a target species fails to persist as a viable population at a site. For each question, a “yes” answer means the factor may be affecting the presence of the target species.
We make several assumptions with these diagnostic tools and their recommendations, including that the target species is an appropriate member of the site’s reference community and species lists for restored and reference sites should be revisited and updated periodically to account for successional and climatic changes. Furthermore, if the target species has known taxonomic issues, the issues have been incorporated into selecting appropriate source material. Taxonomic issues include intraspecific ploidy variation (Kramer et al., 2018), cryptic diversity (Espíndola et al., 2016), or hybridization with other species (Mitchell et al., 2019). We also assume that if seeds were used for the target species restoration, they were viable. Additionally, sufficient time should have elapsed since propagules were introduced for the target species to be detected. This will vary by species and will depend on how long it takes for seeds to germinate and plants to grow large enough to be detected with established monitoring protocols. We assume that the target species can be detected by monitoring protocols in place. And lastly, we assume the restoration site is adequate in size to support a persistent population of the target species.
2 Factors that may influence the occurrence of target species at restoration sites
2.1 Genetic factors
Genetic factors are common precursors to population extinction (Frankham, 2005). If source material is genetically inappropriate, it may have low fitness at the restoration site and fail to persist after one or more generations. Early failure is often associated with inbred and/or poorly adapted source material (Leberg and Firmin, 2008). However, insufficient genetic diversity to respond and adapt to environmental pressures, or lack of appropriate mates (mate limitation), are not immediately evident and may take years to drive population extinction (Robinson et al., 2019). This can make it hard to pinpoint genetic factors as the cause of the target species not establishing at the restoration site. However, knowing the life history of the target species, as well as how source populations were selected and used in the restoration can provide important clues (Frankham et al., 2017).
2.1.1 Diversity
When collecting, producing, and using seeds or plugs for restoration, care must be taken to capture the genetic diversity present at a source site while avoiding steps that may narrow diversity reintroduced (Basey et al., 2015). Large populations, especially where plants are distributed over a wide range of microhabitats, should harbor higher genetic diversity, while small populations (especially those that are fragmented) are more likely to have lower diversity as well as greater risk of being inbred (McGlaughlin et al., 2002; Rosenberger et al., 2021; question G1, Table 1). For longer-term persistence, genetic diversity helps populations survive local disturbances and adapt to future climate changes (Lau et al., 2019). Collecting or using only a portion of diversity present in larger populations, relying on clonal propagation (Fant et al., 2008), or collecting only plants from small populations (Fant et al., 2013) may set restored populations up for future genetic issues, such as inbreeding depression and mate limitation.
Species that are clonal (question G9, Table 1), where restoration source material was derived from a few plants (question G2), and/or cultivated material (question G3) are most at-risk for genetic diversity-related failures to germinate, establish, or persist. For example, Ammophila breviligulata is a clonal beach grass widely used for habitat restoration. Fant et al. (2008) compared restorations in both Lake Michigan and Lake Superior (USA) to assess genetic diversity between native and restored populations after 15 years. They found that the restored populations had lower diversity than native populations, and in many cases the restored populations, which were recreated using plugs, were composed of a single commercially available cultivar. Although these monotypic populations are providing a service of sand stabilization, previous studies have shown that increasing genetic diversity of this dominant plant species may increase productivity of other co-occurring plant species (Crawford and Rudgers, 2012), and was more important than plant species diversity in increasing arthropod (Crawford and Rudgers, 2013) and fungal (Emery et al., 2010) community diversity.
2.1.2 Adaptation
Using appropriate source material with traits adapted to local conditions increases likelihood of germination, establishment, and persistence at the restoration site (McKay et al., 2005; Balazs et al., 2020). Mixing local sources to increase diversity can help overcome issues associated with lack of appropriate source material that closely matches the restoration site (Bucharova et al., 2019). While local wild populations are often assumed to be adapted to site conditions, this is not always the case (Bucharova et al., 2017a), especially for small populations with few individuals and low genetic diversity (Leimu and Fischer, 2008; Hereford, 2009) or given altered site conditions (Leger, 2008) and rapid climate change (Havens et al., 2015). Selecting adapted source material for a restoration could be a challenge when conditions at restoration sites differ from potential sources (Lawrence and Kaye, 2011), or if no remnant sites exist with similar habitat or climate analogues, which is exacerbated by habitat loss and degradation and climate change (Havens et al., 2015). Seed zones, where available, can guide selection of appropriately adapted germplasm (Bower et al., 2014), also considering local variation (e.g., wetlands and uplands ecotypes) and future climate conditions (Richardson and Chaney, 2018).
If the source site is climatically, geographically, and/or ecologically differentiated from the restoration site, if restoration germplasm is derived from a cultivated source, or if seedlings show low vigor, adaptation may be one of the reasons that a species is not able to germinate, establish, and/or persist at a site (questions G3, G4, and G5 on Table 1, respectively). An example is provided by the federally threatened golden paintbrush (Castilleja levisecta) where common garden experiments were conducted to test how each of six remaining wild populations from Washington State, USA performed at potential reintroduction sites in Oregon. Lawrence and Kaye (2011) grew these six sources at a field site targeted for reintroduction and monitored them during two growing seasons. Overall, plant community characteristics of the source site were better predictors of C. levisecta performance at the site than genetic diversity, population size, and geographic distance. These results were used to select source material for reintroductions, preferentially selecting populations from ecologically similar habitats, rather than just geographically close populations. Controlled crosses between populations in this common garden also revealed inbreeding depression. As a result, a regional admixture provenancing strategy (Bucharova et al., 2019) was used to develop germplasm for reintroductions, mixing four populations in a nursery setting to produce ample, genetically diverse germplasm for reintroductions. This strategy has been successful, establishing many genetically diverse and demographically viable populations (St. Clair et al., 2020).
2.1.3 Inbreeding
Inbreeding is common in nature (Keller & Waller, 2002). Small and isolated populations have higher likelihood of inbreeding than large, well-connected populations (Angeloni et al., 2011). Inbred plants can exhibit inbreeding depression, or a loss of fitness (e.g., low pollen viability, seed set, or competitive ability) relative to outbred plants, which is often more obvious under stressful field conditions (Fox and Reed, 2011). In a restoration setting, indications that inbreeding limit the ability of a target species to germinate, establish, or persist include seeds with low germination rates, seedlings with low vigor, or restored populations that have only a few co-flowering conspecifics (questions G5 and G7 on Table 1, respectively).
One example of inbreeding driving low germination rates comes from the eastern prairie fringed orchid (Platanthera leucophaea), where Bell et al. (2021) found significant differences in seed germination based on different hand-pollination treatments. Seedlings produced by crossing parents from different populations had the highest germination, while self-pollinating plants produced the lowest, with crosses between individuals within populations having intermediate germination. Demographic models showed that outcrossing among populations had a significant positive effect on population growth rate compared to the other two treatments, while self-pollination resulted in a negative growth rate and reduced population viability, driving populations to extinction in around 27 years.
In another example, Cirsium pitcheri, a federally threatened species, was reintroduced in Illinois, USA using source material from multiple small nearby populations in Wisconsin and Indiana. Both plugs and seeds were used to reintroduce the population over multiple years. While the population was initially deemed viable based on demographic monitoring, its population growth rate subsequently declined to less than 1, requiring additional inputs of new material (Halsey et al., 2017). Molecular genetics research showed significantly higher inbreeding in the source populations and the reintroduction relative to more distant but larger and more demographically-viable populations (Fant et al., 2013; Fant et al., 2014). This result may reflect the fact that few flowers are available for crosses each year. As a monocarpic perennial, C. pitcheri plants take several years to flower and then flower once and die, so the number of individual plants available for cross pollination in any given year is much smaller than the population size. This highlights the importance of considering not only potential diversity and inbreeding issues in the source material, but the effective population size of the reintroduction.
Sourcing exclusively from small populations can result in poor-performing plants at establishment, which could be mistaken for poor site match rather than inbreeding depression. Similarly, populations established from few maternal lines or clonal species will also lead to increased inbreeding over time, which can limit long-term persistence (Frankham, 2015).
2.1.4 Mate limitation
Low fruit and seed set can be due to a lack of suitable mates in the population (i.e., mate limitation: Young and Pickup, 2010). Initial signs of mate limitation such as scarce fruit and seed set (question Q8, Table 1) are easy to miss if seed set is not monitored (Kirchner et al., 2006). Mate limitation is more likely in in self-incompatible species (~39% of flowering plants; Igic et al., 2008; question Q6, Table 1) than self-compatible species (Levin et al., 2009; Thrall et al., 2014), as well as in species that produce different types of individual plants (e.g., dioecious species that produce female and male flowers on different plants; Molano-Flores, 2004). Additionally, clonal species, particularly those in populations with few co-flowering conspecifics, are particularly at risk of undetected mate limitation (questions Q9, Q7 respectively, Table 1) because they may look large but have very few genetically different individuals flowering at the same time (Gitzendanner et al., 2012; Van Rossum et al., 2021). Alternative causes of low seed production may include lack of pollinators (see Biotic Factors/Pollinators), outbreeding depression (decreased fitness because parents are too genetically different, see Frankham et al., 2011), and/or environmental stress.
An excellent example of mate-limited species comes from two self-incompatible, clonal species found in remnant habitats in Illinois, USA. In one species, seed production was only possible following genetic augmentation, i.e., the addition of plant material with different incompatibility genotypes (Eurybia furcata; Gavin-Smyth et al., 2021). In the other species (Asclepias lanuginosa, Kim et al., 2014), mate limitation was detected via molecular genetics and pollinator limitation was excluded as a possible reason for low seed set by comparing seed production in A. lanuginosa with a similar and co-occurring species (A. viridiflora). Both species are rare in Illinois but A. lanuginosa rarely produces pods, even in large populations. After comparing pollinators, genetic diversity, and seed set in both species at a number of sites, authors found that all populations of A. viridiflora produced pods, but not a single pod was found in A. lanuginosa populations, despite both species occurring at the same site and having similar numbers of individuals. Since the flowers were visited by the appropriate pollinators (Bombus griseocollis and Megachile brevis) they concluded the populations were not pollinator-limited. The lack of seed production was attributed to high clonality in A. lanuginosa populations (ranging from 1-11 unique individuals) resulting in a limited number of unrelated mates in the population. By contrast, in A. viridiflora populations, almost every plant was unique (13-43 unique individuals).
2.1.4.1 Diagnosing genetic factors
• To test for low genetic diversity and inbreeding, use molecular markers to compare restored populations with demographically viable wild populations (St. Clair et al., 2020).
• To investigate inbreeding and mate limitation, monitor seed production in restored populations and compare to large, demographically viable wild populations (Kim et al., 2014).
• To understand which potential source populations are best adapted to target site conditions, perform common garden experiments to compare fitness of different sources, ideally under different climatic conditions (Bucharova et al., 2017b) and over multiple years.
• To test for inbreeding, outbreeding, and mate limitation, conduct controlled crosses between increasingly different parents (self, within and between population) and compare seed set and offspring fitness (ideally growing in natural conditions) (Hufford et al., 2012).
2.1.4.2 Treating genetic factors
• Supplement existing populations by introducing genetically diverse germplasm, ideally from other source populations within the same ecoregion (Willi et al., 2007).
• Reintroduce populations by sourcing germplasm from large populations (>500 plants) whenever possible (Frankham et al., 2014); use seed zones or other regional sourcing tools where available (e.g., Bower et al., 2014); minimize loss or changes to genetic diversity when collecting, propagating and using germplasm (Basey et al., 2015); consider mixing source populations within a region to treat genetic issues (Bucharova et al., 2019) and minimize outbreeding depression risks (Frankham et al., 2011).
• In fire-dependent grasslands, conducting prescribed burns may increase flowering synchrony (Wagenius et al., 2020).
2.2 Biotic factors
Many biotic factors influence whether plants germinate, establish, and persist at a specific site (Ackerly, 2003). Here we consider how the presence of mutualists and antagonists may affect species restoration success.
2.2.1 Pollinators
Pollinator abundance, behavior, diversity, and identity can be strongly influenced by characteristics of the target species population, the plant community in which it grows, and the landscape matrix surrounding the site (Carrié et al., 2016). Most plant species depend on animal-mediated pollination (87%; Ollerton et al., 2011) and pollinator limitations can hinder reproductive success and population viability (Cariveau et al., 2020) (question B1, Table 1). Plant species most at risk from pollinator loss have tightly co-evolved adaptations to a single pollinator (Knight et al., 2005; question B2, Table 1). For example, Brighamia insignis, an endemic Hawaiian plant, is now extinct in the wild. One of the primary drivers of its decline was the extinction of its pollinator, believed to have been a hawkmoth (US Fish and Wildlife Service, 2007). Prior to the extinction of the last wild individuals, B. insignis plants never set seed on their own and relied on hand pollination by botanists who rappelled down the cliffs to reach the last extant population. Now found only in ex situ populations, reintroduction may not be possible without continued human management for pollination (Walsh et al., 2019).
Another example comes from Platanthera praeclara, an orchid from the Great Plains of the USA that seemed to be facing the same problem as Brighamia: pollen limitation due to lack of pollinators. It is pollinated by several species of hawkmoths, but visits were infrequent and seed set was quite low. However, recent pollinator surveys found a Eurasian hawkmoth, Hyles euphorbiae, introduced in the late 1990s to control the invasive plant leafy spurge, began visiting the orchid. It is now the most common visitor of P. praeclara by an order of magnitude, and has greatly increased pollinator service (Fox et al., 2013). This example demonstrates that new mutualisms can develop, giving hope for species where specialist pollinators have been lost, although we would not recommend introducing non-native organisms solely for this purpose.
Most flowering plant species, particularly in temperate regions, are pollinated by multiple species of varying efficiency (Koski et al., 2018). Any changes in the composition of the community, such as shifts in plant or pollinator phenology under climate change or asynchronous flowering of compatible mates (Luijten et al., 2000), can impact seed production (Byers, 2017). Decreases in population size can result in a smaller floral display making plants less attractive to pollinators (question B3, Table 1), with an increase in inbreeding risk (Menges, 1991) and decrease in seed set (Groom, 1998). Landscape fragmentation and site quality will also impact pollinator densities (question B4, Table 1), with greatest impact on less mobile specialist pollinator species (Dixon, 2009), leading to a higher proportion of generalist species (Xiao et al., 2016). Restoration sites that are larger, closer, and more connected with natural areas are likely to have greater pollinator abundance and diversity (Steffan-Dewenter and Tscharntke, 1999; Kremen et al., 2004; Townsend and Levey, 2005).
2.2.1.1 Diagnosing pollinator factors
● Assess floral resource levels of the site to ensure they are sufficient and span the growing season (Ebeling et al., 2008).
● Conduct pollinator observations and surveys and quantify seed set to determine abundance and diversity of pollinators, and pollination success after restoration activity (Breland et al., 2018).
● Use pollen limitation experiments to assess if pollen supplementation boosts seed set (Wagenius and Lyon, 2010).
2.2.1.2 Treating pollinator factors
● Increase population size of the target species (Bernhardt et al., 2008).
● Increase abundance and diversity of floral resources throughout the growing season (Delaney et al., 2015; Havens and Vitt, 2016).
● Provide proper resources and habitat for pollinators to nest (Winfree, 2010).
● Be aware of, and minimize, pesticide drift.
● Alternate burn areas and vary times of prescribed burns to protect fire-sensitive pollinator species (Carbone et al., 2019).
● Connect restorations to other natural areas via corridors to facilitate pollinator migration into the site (Townsend and Levey, 2005).
● Hand-pollinate to overcome pollen limitation (typically not sustainable for the long-term).
2.2.2 Seed dispersal agents
Most plants in temperate grasslands rely on wind, rather than animals, to disperse their seeds (Collins and Uno, 1985). For plant species that rely on both wind and animal dispersal, creating suitable conditions (e.g., corridors that connect fragmented habitat) to promote the movement of the target species could be a crucial step in promoting their establishment and persistence in restoration sites (Damschen et al., 2014; Prior et al., 2015). Seed dispersal and deposition, and species richness in restored sites have been shown to be negatively affected by fragmentation, with reduced recruitment of native species coinciding with increased fragmentation (Poschlod et al., 1998; Damschen et al., 2006; Vanden Broeck et al., 2015). Species that require animal dispersal agents or that are in fragmented populations where dispersal agents are not able to disperse seeds across fragment barriers (questions B5 and B4, respectively, Table 1) are most at-risk for seed dispersal as a limiting factor in their long-term establishment and persistence at a site. For example, some species that require ant dispersal benefit from nest mounds that create microsite conditions and patchiness favoring plant species that are generally less competitive with tall grasses in unmanaged meadows (Dean et al., 1997). Much larger dispersal agents, such as cattle and bison, may play a larger part in seed dispersal for some species than previously thought. As bison have been incorporated into North American grassland restorations, they have potential as dispersal agents through both epizoochory and endozoochory, with seed from over 70 species found in both hair and dung samples in one study (Rosas et al., 2008). Active seeding may still be needed for large-seeded species and for those for which the animal disperser is extinct, extirpated, or threatened (Pires et al., 2018).
For example, Bischoff (2002) investigated the dispersal and establishment of two species characteristic of wet grasslands, Serratula tinctoria and Silaum silaus, comparing them between a natural grassland, where both species were common, and a restored grassland, where both were still extremely rare after 10 years of extensive management. Results showed that in both species poor dispersal was the main limiting factor of their establishment in the restored grassland. Management activities did not increase dispersal distances, while grazers (cattle) did not appear to disperse seeds. While occasional water-based long-distance seed movement was not ruled out, the spatial distribution of seedlings around the parent plants suggested that wind was the main dispersal agent. These results suggest that suitable abiotic conditions at the restoration site alone cannot guarantee successful restoration of floodplain grasslands because dispersal may be the limiting factor, and therefore, an initial input of seeds may be necessary to establish new populations.
2.2.2.1 Diagnosing seed dispersal agent factors
● Identify possible seed dispersal agents of the target species and dispersal range through observations, field experiments, molecular markers, and fluorescent dyes (Levey and Sargent, 2000; Bischoff, 2002; Gelmi-Candusso et al., 2019).
● Determine whether seed handling by dispersers (e.g., ingestion, transport) affects germination, establishment and persistence by comparing germination of fresh and dispersed seeds (Steyaert et al., 2019) and/or by experimentally manipulating seed location and tracking success at the site (Calviño-Cancela, 2002).
● Use molecular markers to estimate recent patterns of seed dispersal and understand population connectivity (e.g., Vanden Broeck et al., 2015).
2.2.2.2 Treating seed dispersal agent factors
● Protect (Lindsell et al., 2015) and, when possible, reintroduce dispersers and eradicate non-native invasive species shown to displace native dispersers.
● For species requiring animal dispersal to germinate (e.g., passage through an animal gut), if the natural disperser is missing, a short-term solution is to treat seeds appropriately (e.g., acid scarification) to mimic natural dispersal (Kildisheva et al., 2020).
● Protect remnant grasslands and reduce fragmentation by increasing habitat connectivity to support species movement (Damschen et al., 2006; Damschen et al., 2014; Howe, 2016).
2.2.3 Nurse plants
Adult plants that facilitate seed germination and seedling establishment of other species are called nurse plants and play a particularly important role in environments with high abiotic stress (Padilla and Pugnaire, 2006; Gonzalez and Ghermandi, 2019) (question B6, Table 1). Aboveground, nurse plants can provide protection against herbivores (e.g., thorny plants), buffer against high irradiation and temperature, and attract pollinators; belowground, nurse plants can improve levels of soil moisture (e.g., hydraulic lift) and key nutrients (Padilla and Pugnaire, 2006; Gonzalez and Ghermandi, 2019), via their common mycorrhizal networks (Querejeta et al., 2009). For example, Dona and Galen (2007), studied the nurse effects of alpine willows (Salix spp.) on the over-winter survival of fireweed (Chamerion angustifolium), a circumboreally distributed herbaceous perennial, by planting established seedlings of fireweed under five different treatments (willow canopy, shade, wind block, shade plus wind block and control, i.e., open meadow vegetation with no manipulation), measuring also abiotic environmental conditions (soil moisture, light intensity, wind speed, and maximum temperature) within treatments, and by comparing results under these treatments. They found that willows promote over-winter survival of established seedling and adult fireweed.
Nurse plants may also increase germination rates in restorations, as seen in the facilitative shading effect of a leguminous shrub on the cactus Neobuxbumia tetetzo (Valiente-Banuet and Ezcurra, 1991). In this case, shaded microsites provided by the shrubs were found to increase germination and survival due to lower daytime temperatures and lower evaporative demand. Similarly, planting scattered N-fixing trees can accelerate forest regeneration, by ameliorating conditions beneath their canopies. The N-fixation restores soil nitrification to pre-clearing levels or those of reference forests, favoring establishment and growth of woody montane seedlings (Rhoades et al., 1998). Finally, Gonzalez and Ghermandi (2019) found that Acaena splendens shrubs act as nurse plants in grasslands of northwestern Patagonia, by facilitating the seedling recruitment of Festuca pallescens, a grass of high forage value present with a low cover in degraded grasslands. However, the authors found that the facilitation mechanism will fail in extremely stressful conditions, such as drought conditions, indicating that this restoration tool can be limited by the specific yearly climatic conditions.
2.2.3.1 Diagnosing nurse plant factors
● Assess whether the target species commonly co-occurs near particular species or plants with a certain structure at reference sites (Padilla and Pugnaire, 2006).
● Conduct field germination and seedling establishment trials with potential nurse plants to confirm if the target species requires, or benefits from, the presence of nurse plants (Padilla and Pugnaire, 2006).
2.2.3.2 Treating nurse plant factors
● Reintroduce nurse plant species before adding target species (Padilla and Pugnaire, 2006).
● Sow seeds and/or plant seedlings around mature nurse plants, near enough to allow root intermingling and provide benefits from hydraulic lift (Izumi et al., 2018) and mycorrhizal networks.
● Use artificial structures that provide same functions as nurse plants (Tuya et al., 2017).
2.2.4 Parasitic plants
Parasitic plants connect to the vascular tissues of the host, using specialized structures called haustoria, to obtain some or all (hemiparasitic and parasitic, respectively) of their carbon, water, and nutrient needs. Parasitic plants are often desired in restoration because they can increase soil nitrogen cycling (Bardgett et al., 2006), suppress dominant vegetation (which facilitates other species including forbs; Pennings and Callaway, 1996; Cole et al., 2019), and increase species and floral diversity (Bardgett et al., 2006; DiGiovanni et al., 2017; Těšitel et al., 2017). Yet these species are also most likely to be missing from restored grasslands (Barak et al., 2017) (question B7, Table 1). Many plant taxa of conservation concern are hemiparasites, including Castilleja levisecta in the Orobanchaceae, for which reintroduction is an important conservation tool; however, determining appropriate restoration strategies for parasitic or hemiparasitic species is challenging, as they may require specific host species to establish or persist (Molano-Flores et al., 2003; Lawrence and Kaye, 2011). Even generalist parasitic plants may be able to form haustoria or a connection from the roots with many different host plant species but may still show high levels of host preference (Press and Phoenix, 2005; Lawrence and Kaye, 2011). For example, Matthies (2017) grew Melampyrum arvense (Orobanchaceae), a hemiparasite from a German calcareous grassland with 27 potential grass, forb, and legume host species at two nutrient levels. Seeds of M. arvense and the host species were germinated in petri dishes in a refrigerator, then transplanted together into pots outdoors. Results showed variation in the quality of hosts, with legumes supporting the greatest parasite biomass, followed by forbs, then grasses (Matthies, 2017). Similar methods in controlled settings can help determine whether specific parasitic or hemiparasitic target species form effective parasitic relationships with different hosts.
2.2.4.1 Diagnosing parasitic plant factors
● If permitted, field-collect target species with nearby plants suspected of serving as host(s) and look for haustorial connections (Yoshida et al., 2016).
● Grow target species and suspected host species together in pots, then examine roots for haustorial connections (Ren et al., 2010).
● When more than one host plant is known for the target species, run greenhouse experiments measuring the target species’ fitness with different hosts (Lawrence and Kaye, 2008).
2.2.4.2 Treating parasitic plant factors
● Ensure appropriate host plants are present at the restoration site prior to seeding or planting the target species (Lawrence and Kaye, 2008).
2.2.5 Competition
Competition occurs when one plant negatively impacts another, either indirectly such as by exploiting common resources like water (Foxx and Fort, 2019) and light, specifically in grasslands where woody encroachment limits grass species diversity (Hare et al., 2021) or by direct interference such as through allelopathy (Amarasekare, 2002; Adomako et al., 2019). It occurs both within and between species and is a key ecological process that shapes and determines plant community compositions (Vellend, 2010; Czarniecka-Wiera et al., 2019), and can limit restoration success (Mangla et al., 2011). While it is well-recognized that competition from aggressive plant species often negatively impacts species diversity in plant communities (Bennett et al., 2011) (question B8, Table 1), the magnitude of impact is not always well established. As reduction in competition can require time and labor-intensive measures, it is helpful to consider testing the degree to which competition may be affecting target species. By coupling neighbor removal with long term monitoring, the effects of competition can be quantified. For example, Maron (1997) found that bush lupine (Lupinus arboreus) had a 32% increase in survival of seedlings when neighboring species were removed. Lupine plants grown with competitors also had decreased root biomass.
Another study by Seahra et al. (2019) identified competition as a bottleneck to species establishment by comparing species performance when they were sown alone versus in mixtures with other species. Most of the studied target species showed greater establishment when sown alone than in mixtures. This method, sometimes referred to as mosaic planting, allows more time for species sensitive to competition to establish in patches and can thus facilitate the coexistence of dominant and subordinate species.
Additionally, changes to resource availability (e.g., nitrogen deposition; Funk and Vitousek, 2007) and interannual weather variability (e.g., precipitation; Groves and Brudvig, 2019) can alter the competitive landscape, favoring some species over others (see Abiotic Factors). Management activities that begin with observing declines in co-occurring species can help ameliorate some of these changes (see Planning & Land Management Factors).
2.2.5.1 Diagnosing competition factors
● Use monitoring data to determine if co-occurrence of some species leads to decline of others (Rinella et al., 2016).
● At the restoration site, test if and how partial or complete removal of invasive or aggressive species affects the target species (Maron, 1997).
2.2.5.2 Treating competition factors
• Plant species in monospecific patches to minimize competition with co-seeded species (Seahra et al., 2019).
• Plant subordinate species earlier than dominant species, or use seed priming (Deering and Young, 2006) so they germinate more rapidly than dominant species and are able to establish in lower-competitive environments (Young et al., 2017).
• Manipulate seed densities in mixes, with target species overrepresented and dominant species underrepresented (Dickson and Busby, 2009).
• Minimize or eliminate competition from dominant or aggressive species by either mowing, targeted scything, or complete removal (manually or with herbicide) at appropriate times to reduce biomass, energy reserves, and/or prevent seed set (Maron, 1997; Abella et al., 2020).
• Introduce appropriate parasitic or hemiparasitic plants to weaken dominant competitors (Press and Phoenix, 2005).
• Remove or replace topsoil where too many propagules of invasive/aggressive species are present (Buisson et al., 2008).
• If already heavily impacted, manage the site in row crops for 2-3 years to deplete the weed seed bank in the soil before sowing native species (Rowe, 2010).
• Seed in difficult to establish species over multiple years to overcome interannual variation in establishment success due to weather or other factors (Groves and Brudvig, 2019).
2.2.6 Granivory and herbivory
Granivory, or seed predation by wildlife or insects before or after it is dispersed, can drive species to extinction (Kurkjian et al., 2017) or keep them from establishing entirely (Vaz Ferreira et al., 2011). This is particularly true at sites where granivore densities are high due to a lack of natural predators (Hulme and Benkman, 2002). Some species have strategies like masting to compensate for losses due to seed predation, but these may only occur when conditions are suitable (Kelly and Sullivan, 1997). Some management practices can help minimize exposure of seeds to predation (e.g., sowing seeds that don’t require cold stratification in the spring instead of fall to minimize predation during the winter; Linabury et al., 2019; see Planning & Land Management Factors). Annual or biennial plants with transient seed banks are particularly vulnerable to seed predation (Maron and Crone, 2006).
Herbivory by wildlife or insects can also significantly impact plant establishment and persistence (Bevill et al., 1999; Orrock et al., 2009). Many herbivores are generalists, although they may prefer some species over others, while specialists are tightly co-evolved with specific plant species or families (Davidson, 1993). Herbivory can produce plant communities largely composed of species (Howe et al., 2002; Barlow et al., 2013), or even ecotypes (Scherber et al., 2003), that are less palatable to herbivores present at the site. This is particularly true in systems with unnaturally high herbivore densities (Anderson et al., 2007). Evidence of granivory or herbivory, such as oviposition holes on seeds or fruits, or stems that are browsed, are clear signs that a population may be impacted by granivory or herbivory (question B9, Table 1). However, the impacts of granivory or herbivory are not always obvious.
In particular, pre-dispersal granivory from insects can be difficult to identify, come from unexpected places, and lead to unexpected outcomes. For example, several insect species have been deliberately introduced to North America (or actively promoted and distributed after arriving on their own) as biocontrol agents for non-native weedy thistle species (e.g., Cirsium arvense, C. vulgare). These insects include Rhinocyllus conicus and Larinus planus (now known as L. carolinae), both seed-feeding weevils, and Cleonus pigra, a root weevil. Unfortunately, none of these insects are host-specific and they have been found feeding on a wide array of native thistles, including the federally-listed threatened species, Cirsium pitcheri (Havens et al., 2012). Although no longer promoted for use by USDA, these insects are widespread in the USA, and are negatively impacting fecundity and/or survival to reproductive maturity of many native thistle populations (Louda and O’Brien, 2002; Louda et al., 2003; Rose et al., 2005). Symptoms of infestation of seed head weevils include the presence of larvae or frass in heads and few or no seeds. Small oviposition holes are visible upon close inspection. Cleonus pigra causes mature plants to wilt and die, typically before any seed set. At present, there are no successful treatments for the seed head weevils because they are active at the same time flowers open and require pollinator visitation for seed set (i.e., any pesticide use would also kill the pollinators).
The impacts of post-dispersal granivory can also be challenging to identify. Selective foraging by birds, rodents, and ants can influence plant population dynamics. Bird granivory of large and intermediate seed sizes in Midwest prairie experiments resulted in reduced plant densities and grass biomass, while plant species with small seeds, deemed below optimal foraging level, were unaffected (Howe and Brown, 1999). In an experiment using fluorescent dye coatings on seeds to track their fates, roughly 10 more seedlings/m2 emerged in closed exclosures compared to controls accessible to small vertebrate granivores in tallgrass prairie restorations (Pellish et al., 2018). Exclosures of different mesh sizes were used to study the impact of different sized consumers in restoration of a native grass in California. The study concluded that voles and mice were able to reduce seedling recruitment by 30% through granivory, but they did not affect subsequent seedling height or tiller length (Orrock et al., 2009). In California rangelands, the giant kangaroo rat (Dipodomys ingens) reduced seedling recruitment by 7% via granivory and by 20% via soil disturbance from burrowing (Gurney et al., 2015).
The full impacts of herbivory can also be challenging to see without a control that excludes the herbivore. For example, the use of deer exclosures in degraded riparian corridors led the density of saplings of woody riparian plant species to be ten times higher in exclosures than in control areas where dark tailed deer could freely browse (Opperman and Merenlender, 2000). Deer exclosures in restored wet meadows improved species richness and diversity significantly (Fraser and Madson, 2008). While the threshold at which herbivore densities will negatively impact a specific target species likely varies depending on the species, site, and year, Urbanek et al. (2012) found that deer densities greater than 21 deer/km2 negatively impacted species diversity in Midwestern USA savanna and forest restorations.
2.2.6.1 Diagnosing granivory/herbivory factors
• Assess extent of seed predation either experimentally (Calviño-Cancela, 2002) or visually (e.g., examine fruits for damage/oviposition holes).
• Conduct granivore/herbivore exclosure experiments (Orrock et al., 2009).
• Test different reintroduction strategies (seeding vs. planting; Godefroid et al., 2011).
• Estimate granivore/herbivore densities at the restoration site and compare with those found in sites where persistent populations of the target species occur (Pender et al., 2013).
• Track seeds with fluorescent dye to determine their fate (Pellish et al., 2018).
2.2.6.2 Treating granivory/herbivory factors
• For some species, fences, cages, or netting can be used to exclude herbivores to protect plants (Bevill et al., 1999; Orrock et al., 2009), but this can be expensive and time-consuming, and does not address the causes of high herbivore or granivore densities.
• If the issue is at the seed stage (granivory), reintroduce the species via plugs instead of seeding (Wallin et al., 2009), or overseed to compensate for predation (Orrock et al., 2009; Longland and Ostoja, 2013).
• Seed or plant the target species near thorny plants to protect plants from mammalian herbivory (see Biotic Factors/Nurse Plants).
• Coat seeds to deter granivory (Taylor et al., 2020).
• Investigate approaches to decrease mammalian densities range such as removing favorable herbivore habitat (e.g., cutting tall grass that mammals prefer) to hunting/trapping or increasing predator habitat (Wasson et al., 2021).
• Approaches to decrease insect densities range from pesticide application to biocontrol introduction, but these pose challenges and present risks that need to be carefully considered (see Planning & Land Management factors/Unintended or spillover impacts of management) (McLaughlin and Dearden, 2019).
2.2.7 Soil biota
Plant interactions with soil microbes (e.g., rhizobia, mycorrhizal fungi) comprise one of the major mechanisms contributing to plant diversity (Moeslund et al., 2017). Legumes form symbioses with nitrogen-fixing bacteria called rhizobia in their root nodules (Hirsch et al., 2001) (question B11, Table 1), while around 80% of all plant species, and 92% of all plant families, are believed to form associations with mycorrhizal fungi (Wang and Qiu, 2006) (question B10, Table 1). Mycorrhizal symbioses typically increase acquisition of nutrients like nitrogen and phosphorus, otherwise inaccessible to plant roots, and this promotes seedling establishment and enhances the competitive ability of subordinate plant species relative to dominant taxa (Van Der Heijden et al., 1998). Fungal and bacterial inoculations of the soil or even seeds can promote germination and plant establishment and can prove to be a useful management tool (Pedrini et al., 2020).
In tallgrass prairie species, numerous studies have shown that mycorrhizal inoculation improves plant establishment relative to non-inoculated plants. For example, inoculated plants showed greater plant height, root length, and biomass production (Ghimire et al., 2009) as well as increased survival rates in the first year of restoration vs. non-inoculated plants (Maltz and Treseder, 2015; Koziol et al., 2018). Such increases may be correlated with higher levels of mycorrhizal root colonization (e.g., Smith et al., 1998) and the development of mycorrhizal networks (Middleton et al., 2015). However, inoculation does not always enhance productivity in a target native grass species (Paluch et al., 2013) and root colonization may not correspond to improved plant establishment, especially in sites with existing mycorrhizal communities (White et al., 2008).
Similarly, other beneficial soil microbes may be introduced to enhance plant growth. In a study by Chaín et al. (2020), a superabsorbent polymer was used to deliver two strains of the bacteria Pseudomonas to greenhouse-grown Eucalyptus species. Plants inoculated with Pseudomonas showed greater growth (e.g., larger leaves, stems, water use efficiency) under drought than plants grown without the bacterial inoculants.
Conversely, accumulation of species-specific pathogens or microbial communities within the rhizosphere may lead to a species’ decline and/or replacement (Reynolds et al., 2003). Evidence that this may be contributing to the inability of a species to establish or persist at a site include flower, root, or stem rot, or fungal colonies on leaves (question B12, Table 1). Restoration actions also affect soil microbial communities (Dickens et al., 2015). For example, feedbacks between soil nutrients and early successional plant species (Kardol and Wardle, 2010) may create bacterial- or pathogen-enriched environments that are less favorable to seed germination and seedling establishment of target species (Kulmatiski et al., 2008).
2.2.7.1 Diagnosing soil biota factors
• Stain and examine root and soil samples, collected at both reference and restoration sites, for the presence and abundance of mycorrhizal colonization or the abundance of nodules (legumes) (Vierheilig et al., 1998).
• Test seed germination and seedling establishment in the presence of soil extracts from a specific site, or via inoculation with potential beneficial microbes (see Ghimire et al., 2009 for methodology).
• Analyze the abundance of bacteria relative to fungi in soil samples, comparing reference and restoration sites (Robertson et al., 1999).
• Assess mycorrhizal or microbial infectivity and efficacy using a bioassay (Djuuna et al., 2009).
2.2.7.2 Treating soil biota factors
• Inoculate soil restoration site with whole soil from a remnant site through the application of fresh topsoil, soil cores, or monoliths (Bulot et al., 2017).
• Introduce locally adapted mycorrhizal fungi or microbial-inoculated plants (Middleton et al., 2015), and include consortia of mycorrhizal or microbial species as inoculum rather than a single species (Koziol et al., 2018).
• Introduce native leguminous (Fabaceae; Rhoades et al., 1998) and actinorhizal plants (Betulaceae) to enhance communities of N2-fixing microbes (Paschke, 1997).
• Coat or pellet seeds with encapsulated microbes to facilitate the inoculation of seedlings (Rocha et al., 2019).
• Where appropriate, introduce biological crusts using cultivated or natural materials (Doherty et al., 2020).
2.3 Abiotic factors
In addition to biotic constraints, the establishment of target species is largely affected by resource requirements and physiological tolerances to abiotic conditions, including climate, soil, and hydrology (Fløjgaard et al., 2020). Here, we outline approaches available to diagnose and treat issues with these abiotic factors at the restoration site.
2.3.1 Climate
Climate is a significant factor controlling the distribution and abundance of species and altered temperature and precipitation patterns have a profound impact on species’ range expansion (e.g., Brusca et al., 2013; Guittar et al., 2020) and contraction (Leopold and Hess, 2019). As reported in the Intergovernmental Panel on Climate Change’s Sixth Assessment Report, including the Regional Synthesis Interactive Atlas (Gutiérrez et al., 2021), not all regions of the world are changing at the same pace for different factors like temperature and precipitation (question A1, Table 1). This intra- and inter-annual variation in temperature and precipitation can cause shifts in seasonality, hydrology, snow cover dynamics, soil temperature and moisture, and fire regimes that may affect when, where and whether different plant species and sources will be able to germinate, survive, establish, reproduce, and persist as adults as well as seeds and buds (Ooi, 2012; Ott et al., 2017). Rapidly changing climates and extreme climate conditions, expected to increase in frequency (Easterling et al., 2017), may shift current species (e.g., C3 vs. C4) geographic ranges and phenology (Knapp et al., 2020), and clonal growth (Sluis, 2020; Bam et al., 2022), making restoration efforts more important but also more challenging (Havens et al., 2015). This also means that reference plant communities may rapidly change, creating a need to periodically update reference communities for any given restoration site (Shackelford et al., 2022).
Other concerns related to climate change include impacts on plant germination as well as long-term survival, and mutualisms with species plants depend on for reproduction and dispersal. For example, climate impacts litter accumulation and decomposition rates (Fekete et al., 2016), potentially altering microclimate conditions at a site, which can affect seed germination and seedling emergence and establishment (Loydi et al., 2013). And over the long-term, remnant or restored plant populations may be threatened with extinction if climatic changes exceed their climatic niches, adaptive capabilities, and migration paces, especially in fragmented landscapes (Aitken et al., 2007). Finally, antagonistic (e.g., herbivores and their food plants) as well as mutualistic (e.g., pollination) relationships between plants and insects or vertebrates they interact with may be impacted if the species involved do not have similar phenological responses to climate change (Renner and Zohner, 2018; question A2, Table 1).
Understanding how extreme weather events or more variable climatic conditions impact the relative performance of native species, especially at early life history stages, could offer insight into their potential success under future climate change scenarios. The predicted increase in intra-annual precipitation variability in North American grasslands will produce larger individual precipitation events with longer intervening dry periods (Easterling et al., 2017). Such changes will generate more temporally dynamic soil moisture regimes creating more stressful conditions for native plants. While a recent experimental approach did not find any impacts of precipitation frequency on the clonal growth in Pascopyrum smithii, a common C3 grass species in North American grasslands (Bam et al., 2022), much more work is needed to understand how changing climates will impact germination, establishment, and persistence of different species (question A3, Table 1).
2.3.1.1 Diagnosing climate factors
• Examine historical long-term relative to current and projected climate data trends using the Intergovernmental Panel on Climate Change’s Interactive Atlas (https://interactive-atlas.ipcc.ch/).
• Track changes in plant phenology (e.g., using Budburst (www.budburst.org) or USA National Phenology Network (www.usanpn.org/usa-national-phenology-network).
• Conduct germination and establishment experiments to understand micro-climatic requirements, e.g., litter modification experiments (Loydi et al., 2013); light; bare soil, etc.
• Conduct field and/or laboratory experiments to simulate different climate scenarios (Knapp et al., 2020; Bam et al., 2022).
• Conduct common garden experiments to evaluate species-specific climatic tolerance limits (Leopold and Hess, 2019).
2.3.1.2 Treating climate factors
• Take into consideration climate predictions and extremes in restoration designs (e.g., using Climate–Smart with Seed Tool to select seed mixes to match with current and future projected key climatic variables in the USA.; e.g., Finch et al., 2019; https://climaterestorationtool.org/csrt/).
• Improve microclimatic conditions by managing the vegetation surrounding the reintroduction plots, e.g., manage litter cover and depth (Loydi et al., 2013).
• To manage climatic challenges at a site, ensure germplasm is genetically diverse and adapted to climate conditions at the site (see Genetic factors).
2.3.2 Hydrology and soil
Target species’ germination, establishment, and persistence can be impacted by site hydrology (e.g., erosion dynamics, currents, and flooding; Kettenring and Tarsa, 2020), with even small changes in hydrology influencing the plant community (Silvertown et al., 2015). Hydrology of restoration and remnant sites can be significantly impacted by engineering approaches like damming, ditch construction, and drain tile installation (Kelly et al., 2017) (questions A4 and A5, Table 1). This can make it challenging to understand variation in hydrologic dynamics and soil moisture availability between restoration and reference sites, but restoration of site hydrology may be critical prior to attempting to reintroduce target species or communities (Kettenring and Tarsa, 2020), but climate change is making this increasingly challenging (Cuthbert et al., 2019).
Similarly, shifts in soil nutrients, pH, conductivity, organic matter, contaminants, compaction, and structure can directly or indirectly (e.g., via microbial communities) impact seed germination and plant survival (Bach et al., 2010; Turley et al., 2020; question A6, Table 1). Nutrient-rich soils generally support fast-growing, early successional species, and inhibit slower-growing ones (de Vries et al., 2012). A loss of soil structural stability (e.g., via plowing of agricultural fields) may lead to compaction, especially in clay-rich soils (Rosenzweig et al., 2016), thereby decreasing soil porosity, water infiltration, and the ability of roots to forage for water and nutrients (Correa et al., 2019). In soils contaminated from metals, salt, or pollutants, reductions in root growth coupled with metal accumulation limits water and nutrient uptake, leading to dramatic declines in plant growth and gross macronutrient deficiencies (Rahman et al., 2018) (question A7, Table 1). Target species with low tolerances to these conditions may show negligible recruitment (Moeslund et al., 2017).
In natural sedge meadows, the highly organic soils act as a sponge, retaining water and drawing water upward from deeper sources by capillary action. These meadows also drain slowly owing to the low hydraulic conductivity of peat. Together, the capillarity and low hydraulic conductivity keep soils in sedge meadows largely saturated throughout the year. Conversely, restored wetlands contain soils with lower organic content and higher hydraulic conductivity. Many restored sites also lack the vegetative cover that would drive litter inputs. As a result, soils in created sedge meadows are exposed to direct sunlight (higher temperatures) and greater wind speeds and are often dry and cracked at the surface, even if saturated at depth. For example, the failure of Carex spp. to germinate in restored wetlands was attributed to low soil moisture levels (Van Der Valk et al., 1999). The authors tested the effect of five different soil moisture levels on germination in Carex stipata and C. stricta, and the effect of soil organic amendments on the growth of C. stricta seedlings. The results showed that amending the soil with organic matter improved seed germination and seedling establishment in Carex spp. by improving the water holding capacity of the soil and its fertility. Organic amendments may thus be useful in acidic soils or those sites where the topsoil has been removed.
In extreme situations, topsoil removal (or turf cutting) has been recognized as a measure to restore oligotrophic conditions by reducing organic matter and nutrient levels. This approach has been applied in heathlands where atmospheric N and S deposition has resulted in soil acidification and low base saturation. Van Den Berg et al. (2003) conducted field experiments that examined the impact of turf cutting to different depths on the germination of Arnica montana. In some of the experimental plots, lime was an additional treatment. The authors also conducted laboratory experiments to test the effect of Al and humic acids on the germination of the target species. They found that herbaceous plants of species-rich heathlands and grasslands, like A. montana, are vulnerable to the soil conditions created by turf cutting. The addition of lime and/or humic acids resulted in higher germination and improved plant establishment because the amendments increased soil base saturation and pH and reduced the levels of phytotoxic metals, such as Al.
2.3.2.1 Diagnosing hydrology and soil factors
• Compare soil properties and/or rooting depth at the restoration site relative to reference sites through approaches like digital soil mapping techniques (Goldman et al., 2020), global databases (e.g., Web Soil Survey), or laboratory analyses (e.g., bulk density, moisture, pH, aggregation, nutrients, organic matter, microbial abundance; Robertson et al., 1999).
• Monitor soil and surface water chemistry, and site hydroperiod timing, (i.e., hydrological inputs and outputs), using wells, stream hydrographs, remote sensing (drones, MODIS), and tests of moisture requirements during seed germination (Chasmer et al., 2020).
2.3.2.2 Treating hydrology and soil factors
• Restore site hydrology, e.g., remove drain tiles and plug drainage ditches (Kettenring and Tarsa, 2020); amend nutrients and organic matter (Van Der Valk et al., 1999).
• Create dams or micro-catchments to reduce run-off, erosion, and nutrient loss without inverting the upper soil profile, as tillage would do (Pueyo et al., 2009).
• Use high-quality carbon substrates to stimulate N (and P) immobilization in the microbial biomass and decrease soil N (or P) fertility (Sollenberger et al., 2016).
• Introduce natural disturbance regimes, e.g., fire, to induce functional shifts in plant community diversity and rhizosphere microbes by altering the quality and quantity of root-derived substrates entering the soil (Forest et al., 2005).
• Improve aeration and infiltration (e.g., alleviate soil compaction; reintroduce native burrowing invertebrate (Colloff et al., 2010) and vertebrates (Platt et al., 2016).
• Use soil amendments to improve plant growth including N or P fertilizer to ameliorate plant nutrient deficiencies in metal-contaminated soils and calcium amendment (liming) in acidic soils (Rahman et al., 2018).
• Remediate metal-contaminated soils using metal-tolerant plants (Wei et al., 2021) or nutrient beads with immobilized metal-tolerant mycorrhizal fungi (Egerton-Warburton, 2015) or bacteria (Sharma et al., 2018).
2.4 Planning and land management factors
From decisions about planting methods and seeding rates to ongoing management, adequate planning, preparation, and management are vital to support germination, establishment, and persistence of restored plant populations and communities.
2.4.1 Seeding
While the seeds of some species germinate immediately after exposure to ideal temperature and moisture conditions, others have specific seed dormancy requirements that dictate the conditions under which they will germinate (question P1, Table 1). A limited understanding of dormancy and germination behavior can hamper restoration efforts. For some species, seed dormancy and dormancy break are complex and can take months or even years to occur, however, there may be pre-treatments that can be applied prior to planting to encourage germination (Baskin and Baskin, 2014; Kildisheva et al., 2020). When determining timing of seeding, managers should consider information about seed dormancy and natural cycles of dispersal at restoration sites (question P2, Table 1). Optimizing dormancy break in restoration-relevant species can improve restoration outcomes by promoting recruitment and reducing post-emergence seedling mortality. For example, sowing seeds at an inappropriate time could cause seeds and germinants to be attacked by pathogens, or be damaged or killed by environmental conditions like wet-dry or freeze-thaw cycles (James et al., 2012).
For species introduced as part of a seed mix containing multiple species, seed mix composition can influence the probability that a given species will establish (question P3, Table 1). For example, in prairie seed mixes if the ratio of grasses to forbs is too high, it can impede the establishment of individual forb species (Dickson and Busby, 2009; Grman et al., 2015). Some species may benefit from repeated seeding (Sluis et al., 2018) and/or a higher seeding rate (Grman et al., 2015; Shackelford et al., 2021). For species that are difficult to establish from seed, planting plugs is often recommended (Gallagher and Wagenius, 2016), but some species are more likely to establish and persist at a site when planted from seeds (St. Clair et al., 2020; Anderson and Minor, 2021). Successful establishment of seeded or planted species is likely to be influenced by management and the level of disturbance at a site (see below).
2.4.1.1 Diagnosing seeding factors
• Track seed germination and early establishment (Kulpa and Leger, 2013).
• Perform germination trials with fresh seeds at multiple light and temperature conditions: if few/no seeds germinate, conduct additional germination trials to determine specific dormancy-breaking requirements (Kildisheva et al., 2020).
• Poor germination may be a sign that seeds are not viable, due to a range of possible factors, including environmental conditions at the collection site, genetic factors in the source population(s), and/or seed collection and storage conditions. Seed viability can be assessed using a range of methods (Riebkes et al., 2015).
• Test different reintroduction strategies (e.g., seeding timing, technique, rate; Shaw et al., 2020; or seeding vs. planting; Godefroid et al., 2011).
• Investigate seed longevity in the field and the ability to form a soil seed bank (Bakker et al., 1996).
2.4.1.2 Treating seeding factors
• Sow dormant seeds at the time of natural dispersal to increase chances of seed and seedling survival, or break the dormancy of seeds prior to sowing, adjusting timing of sowing to the ideal time for emergence (Shaw et al., 2020; Kildisheva et al., 2020).
• Adjust the seeding rate (or seed multiple times) if the target species does not establish well from the first seeding (Grman et al., 2015) or develop a persistent soil seed bank (Sluis et al., 2018).
• Mow, or apply similar management measures, to ensure seedlings have enough light for growth (see section ‘Required disturbance’).
• Identify topographic microsites (mounds, pits, flats) or use amendments (e.g., hay, mulch) to create microsites that enhance seed germination and establishment (Naeth et al., 2018).
• For species that have proven difficult to establish from seed, introduce as plugs (Gallagher and Wagenius, 2016).
• Coat seeds to help regulate dormancy and promote germination at the appropriate time (James et al., 2012).
2.4.2 Required disturbance
Landscape-level disturbance management, for example fire and grazing, is often necessary for the germination and persistence of many plant species in restored grasslands (Grman et al., 2015; Alstad et al., 2018; Török et al., 2021;questions P4, P5, and P6, Table 1). These disturbances may be natural and/or historically managed by indigenous people (Kimmerer and Lake, 2001). On a habitat level, disturbance may be required to maintain a particular grassland type (e.g., preventing woody encroachment and grassland conversion; Leach and Givnish, 1996) and be important in promoting establishment and persistence of individual target species. In general, habitats that are disturbance-managed, such as through fire, mowing, or grazing, often support species establishment and persistence because the applied management can decrease plant competition and litter; allow seeds to have better soil contact; reduce predation from pests; and increase heterogeneity in light, as well as nutrient and water availability (Vickery, 2002; Overbeck et al., 2003; Loydi et al., 2013; Alstad et al., 2018; Meissen et al., 2020).
Prescribed fire, mowing, and grazing have all been important in supporting establishment of target species in restored grasslands. Fire suppression has been a driver of the loss of many important prairie species, and prescribed fire can aid in their germination, establishment, and persistence (Leach and Givnish, 1996; Alstad et al., 2016). In grassland habitats where historical disturbance regimes include fire, but prescribed fire isn’t feasible, mowing or targeted weeding may be acceptable management substitutes (Macdougall and Turkington, 2007). Finally, some species may require multiple types of disturbance management, such as some native forb species in serpentine grasslands that emerged and persisted only following both burning and grazing (Hernández et al., 2021). It is important to note that management can only support the germination, emergence, and establishment of species that are on site (i.e., as resident plants, in the soil seedbank, or seeded into the site as described above).
In the tallgrass prairie, fire represents both a natural disturbance and a site preparation tool (Alstad et al., 2018). Alstad et al. (2018) tested the impacts of prescribed burning on germination on the seeds of eight prairie species in a field experiment. Despite very low emergence percentages overall, all species had higher germination and emergence when seeded into burned plots, as compared to unburned plots. Some species did not emerge at all in unburned plots, but emerged in burned plots, including Amorpha canescens, Dalea candida, and D. purpurea. Their hypothesis was that fire reduced the amount of litter in the system and allowed better soil contact for seeded species. In another tallgrass prairie study, frequent mowing facilitated establishment of forb species into a grass-dominated restoration: multiple species of forbs persisted through flowering stages in mowed plots, while they did not establish at all in non-mowed control plots (Williams et al., 2007).
2.4.2.1 Diagnosing required disturbance factors
• Test whether removal of competitive species, litter and/or creation of canopy gaps increase germination, establishment and/or persistence.
• If the target species or community has been historically managed through disturbance (e.g., fire, grazing), conduct an experiment testing the disturbance and compare germination, establishment, and persistence of the target species in both sites where the disturbance has been reintroduced and control (unmanaged) sites.
2.4.2.2 Treating required disturbance factors
• When indicated and possible, restore necessary disturbance regimes to support the target species, but be aware that co-occurring species may be negatively impacted (Palmer et al., 2017). Timing disturbances accordingly may help mitigate impacts on other species (Knapp et al., 2009).
• If a natural or managed disturbance is not possible at a landscape-level, simulate the effect of a disturbance at smaller scales (e.g., remove litter cover manually, clip vegetation) to increase heterogeneity in conditions (Overbeck et al., 2003; Loydi et al., 2013).
2.4.3 Unintended or spillover impacts of management
Management activities conducted at the restoration site to manage other species can have unintended or spillover impacts on the target species. For example, herbicides applied to manage aggressive or invasive species can damage other species or allow for secondary invasions (Bennion et al., 2020) (question P8, Table 1). In addition, while periodic management like prescribed fire is important for promoting germination of some species, decreasing the abundance of dominant species, and preventing woody encroachment, it can negatively impact other species, both plants and animals. For example, Lupinus oreganus is the larval host of the endangered butterfly, Icaricia icarioides fenderi in Willamette Valley prairie habitats (USA). Invasive grasses are taller than the lupine, and therefore interfere with the relationship between the plant and butterfly. Grass-specific herbicide has been tested to reduce the height of grasses so they are lower than the lupine. While the herbicide did reduce grass height, and improve access by pollinators, as well as increase seed production in the lupine, it also had unintended impacts on the lupine, including suppression of its growth, and introduction of several secondary invaders (particularly forbs) that arrived after the grass was reduced. The authors conclude that grass-specific herbicide is not the ideal tool to use in the restoration of L. oreganus/Icaricia icarioides fenderi habitat due to the secondary impacts (Bennion et al., 2020; Schultz and Ferguson, 2020). Herbicide has an important role in restoration, but it is important to consider the costs, benefits, and possible spillover impacts when deciding on an herbicide plan.
2.4.3.1 Diagnosing unintended or spillover impacts of management factors
• Carefully monitor germination, establishment, and persistence of the target species if herbicides or fertilizers are used on, or adjacent to, the restoration site (McManamen et al., 2018).
• Estimate the net benefit of restoration management practice: weigh the potential benefits of the management application (e.g., herbicides, pesticides) with the unintended consequences (Bennion et al., 2020).
2.4.3.2 Treating unintended or spillover impacts of management factors
• See Abiotic factors section.
• Avoid herbicide application in areas near developing seeds/seedlings (McManamen et al., 2018) or modify herbicide application interval (Crone et al., 2009).
• Isolate spatially or temporally fire-sensitive species from fire management (Knapp et al., 2009).
3 Discussion
Challenges in plant species germination, establishment, and persistence are critical problems preventing restorations from achieving the goals of the U.N. Decade on Ecosystem Restoration to re-establish species diversity. In this paper, we highlight key factors affecting why plant species may be missing and offer considerations to identify and treat these factors (ranging from genetic to biotic, abiotic, and planning & land management challenges). We hope this document will be useful to both practitioners and researchers as they work together to improve long-term restoration outcomes and support species diversity. We recognize that many of these diagnoses and treatments require access to equipment, funding, and staff that may not be readily available at many land management agencies and organizations. Partnering with universities and other scientific organizations can be a way to both accomplish the needed research and help build bridges between our communities. Additionally, while a wide range of resources are available to help identify and treat these factors, much more work is needed. Continued knowledge and data-sharing that supports synthesis to advance restoration science will be critical to continued progress in meeting these goals (Ladouceur et al., 2022).
In addition to the factors detailed above, it is important to note that a site’s history and time since restoration will also impact its capacity to meet species or diversity targets. Long-term studies from around the world illustrate that it can take decades for restored grassland communities to approach the diversity of a reference natural community and will depend on the intensity and kind of disturbance the site experienced prior to and following restoration (Kindscher and Tieszen, 1998; Fagan et al., 2008). Climate change, habitat loss and fragmentation, and pollution will increasingly influence plant population dynamics, with possible alteration, disruption, and shifts in species composition and range (Guittar et al., 2020), making it important to periodically revisit reference species lists to adjust expectations (Shackelford et al., 2022). And particularly in heavily-impacted, novel environments like urban and former industrial sites that do not have reference systems, restoration that supports biodiversity will require a new conceptual framework (Klaus and Kiehl, 2021).
Monitoring restoration sites is important for informing management actions, but this is resource-intensive and provide the most valuable data when they are conducted periodically over the long-term. This is because floristic surveys conducted at an early stage of restoration may be poor predictors of long-term restoration outcomes related to species composition, as some seeds may take several seasons to germinate and emerge, or only appear at a later successional stage. To limit the costs associated with long-term monitoring, project managers can leverage open-source datasets to update regional species lists. Such datasets include permanent monitoring programs (e.g., US National Ecological Observatory Network), community science approaches (e.g., Chicago Botanic Garden’s Plants of Concern), and their regional or global aggregation (e.g., Global Biodiversity Information Facility, iNaturalist). Novel approaches relying on open-source remote sensing datasets (e.g., Sentinel) or the custom acquisition of aerial images via low-cost drones (Anderson and Gaston, 2013), could help managers identify when field surveys are warranted to identify missing species.
Finally, building and sustaining respectful and reciprocal relationships among researchers and restoration practitioners will be critical to this work, supporting evidence-based practice (Suding, 2011). Additionally, while this tool was developed through the lens of western science, we acknowledge the importance of Indigenous knowledge and the leadership of Indigenous communities in stewarding natural areas (Dickson-Hoyle et al., 2022). While time and resource constraints might limit the feasibility of certain diagnostic tests and treatments highlighted here, we hope these relationships and the use of this tool will lead to improved restoration outcomes, one species at a time.
Author contributions
AK conceived the idea; MD, KaH, and AK developed and structured the idea; MD, AK, and KaH led the writing of the manuscript and produced figures and tables; All authors contributed to the article and approved the submitted version.
Acknowledgments
This was a project of The Synthesis Center for Conservation and Restoration (sCORE), funded by the Negaunee Institute for Plant Conservation Science and Action at the Chicago Botanic Garden. The authors thank Iza Redlinski, Stephanie Frischie, Jim Steffen, the Conservation/Restoration lab group and staff at the Chicago Botanic Garden, and two reviewers for providing helpful comments on prior versions of the manuscript, as well as the creators of icons used in the graphical abstract and figures of the paper: grass by Bakunetsu Kaito, study by Eucalyp; meeting by ProSymbols; test by Alzam; table by Douglas Machado; book by Gregor Cresnar; microbes by Mohamad Arif Prasetyo; dead Plant by Vectors Market from the Noun Project.
Conflict of interest
The authors declare that the research was conducted in the absence of any commercial or financial relationships that could be construed as a potential conflict of interest.
Publisher’s note
All claims expressed in this article are solely those of the authors and do not necessarily represent those of their affiliated organizations, or those of the publisher, the editors and the reviewers. Any product that may be evaluated in this article, or claim that may be made by its manufacturer, is not guaranteed or endorsed by the publisher.
Supplementary material
The Supplementary Material for this article can be found online at: https://www.frontiersin.org/articles/10.3389/fcosc.2022.1028295/full#supplementary-material
References
Abella S. R., Chiquoine L. P., Moss J. M., Lassance E. D., Schelz C. D. (2020). Developing minimal-input techniques for invasive plant management: perimeter treatments enlarge native grass patches. Invasive Plant Sci. Manage. 13 (2), 108–113. doi: 10.1017/inp.2020.9
Ackerly D. D. (2003). Community assembly, niche conservatism, and adaptive evolution in changing environments. Int. J. Plant Sci. 164 (S3), S165–S184. doi: 10.1086/368401
Adomako M. O., Ning L., Tang M., Du D.-L., Van Kleunen M., Yu F.-H. (2019). Diversity- and density-mediated allelopathic effects of resident plant communities on invasion by an exotic plant. Plant Soil 440 (1), 581–592. doi: 10.1007/s11104-019-04123-9
Aitken M., Roberts D. W., Shultz L. M. (2007). Modeling distributions of rare plants in the great basin, Western north America. Western North Am. Nat. 67 (1), 26–38. doi: 10.3398/1527-0904(2007)67[26:MDORPI]2.0.CO;2
Alstad A. O., Damschen E. I., Givnish T. J., Harrington J. A., Leach M. K., Rogers D. A., et al. (2016). The pace of plant community change is accelerating in remnant prairies. Sci. Adv. 2 (2), e1500975. doi: 10.1126/sciadv.1500975
Alstad A. O., Damschen E. I., Ladwig L. M. (2018). Fire as a site preparation tool in grassland restoration: Seed size effects on recruitment success. Ecol. Restor. 36 (3), 219–225. doi: 10.3368/er.36.3.219
Amarasekare P. (2002). Interference competition and species coexistence. Proc. R. Soc. B: Biol. Sci. 269 (1509), 2541–2550. doi: 10.1098/rspb.2002.2181
Anderson R. C., Dorick B., Crispino T. (2007). Impacts of initial species richness and deer browsing on the quality of restored prairie in central Illinois. Ecol. Restor. 25 (2), 99–102. doi: 10.3368/er.25.2.99
Anderson K., Gaston K. J. (2013). Lightweight unmanned aerial vehicles will revolutionize spatial ecology. Front. Ecol. Environ. 11 (3), 138–146. doi: 10.1890/120150
Anderson E. C., Minor E. S. (2021). Assessing four methods for establishing native plants on urban vacant land. Ambio 50 (3), 695–705. doi: 10.1007/s13280-020-01383-z
Angeloni F., Ouborg N. J., Leimu R. (2011). Meta-analysis on the association of population size and life history with inbreeding depression in plants. Biol. Conserv. 144 (1), 35–43. doi: 10.1016/j.biocon.2010.08.016
Bach E. M., Baer S. G., Meyer C. K., Six J. (2010). Soil texture affects soil microbial and structural recovery during grassland restoration. Soil Biol. Biochem. 42 (12), 2182–2191. doi: 10.1016/j.soilbio.2010.08.014
Bakker J. P., Poschlod P., Strykstra R. J., Bekker R. M., Thompson K. (1996). Seed banks and seed dispersal: Important topics in restoration ecology. Acta Botanica Neerlandica 45 (4), 461–490. doi: 10.1111/j.1438-8677.1996.tb00806.x
Balazs K. R., Kramer A. T., Munson S. M., Talkington N., Still S., Butterfield B. J. (2020). The right trait in the right place at the right time: Matching traits to environment improves restoration outcomes. Ecol. Appl. 30 (4), e02110. doi: 10.1002/eap.2110
Bam S., Ott J. P., Butler J. L., Xu L. (2022). Belowground mechanism reveals climate change impacts on invasive clonal plant establishment. Sci. Rep. 12 (1), 1–11. doi: 10.1038/s41598-022-06918-w
Barak R. S., Williams E. W., Hipp A. L., Bowles M. L., Carr G. M., Sherman R., et al. (2017). Restored tallgrass prairies have reduced phylogenetic diversity compared with remnants. J. Appl. Ecol. 54 (4), 1080–1090. doi: 10.1111/1365-2664.12881
Bardgett R. D., Smith R. S., Shiel R. S., Peacock S., Simkin J. M., Quirk H., et al. (2006). Parasitic plants indirectly regulate below-ground properties in grassland ecosystems. Nature 439 (7079), 969–972. doi: 10.1038/nature04197
Barlow S. E., Close A. J., Port G. R. (2013). The acceptability of meadow plants to the slug deroceras reticulatum and implications for grassland restoration. Ann. Bot. 112 (4), 721–730. doi: 10.1093/aob/mct086
Basey A. C., Fant J. B., Kramer A. T. (2015). Producing native plant materials for restoration: 10 rules to collect and maintain genetic diversity. Native Plants J. 16 (1), 37–53. doi: 10.3368/npj.16.1.37
Baskin C. C., Baskin J. M. (2014). Seeds: ecology, biogeography, and, evolution of dormancy and germination. 2nd ed. (San Diego: Academic Press).
Bell T. J., Bowles M. L., Zettler L. W., Pollack C. A., Ibberson J. E. (2021). Environmental and management effects on demographic processes in the US threatened Platanthera leucophaea (Nutt.) Lindl.(Orchidaceae). Plants 10 (7), 1308. doi: 10.3390/plants10071308
Bennett A. E., Thomsen M., Strauss S. Y. (2011). Multiple mechanisms enable invasive species to suppress native species. Am. J. Bot. 98 (7), 1086–1094. doi: 10.3732/ajb.1000177
Bennion L. D., Ferguson J. A., New L. F., Schultz C. B. (2020). Community-level effects of herbicide-based restoration treatments: structural benefits but at what cost? Restor. Ecol. 28 (3), 553–563. doi: 10.1111/rec.13118
Bernhardt C. E., Mitchell R. J., Michaelsy H. J. (2008). Effects of population size and density on pollinator visitation, pollinator behavior, and pollen tube abundance in lupinus perennis. Int. J. Plant Sci. 169 (7), 944–953. doi: 10.1086/589698
Bevill R. L., Louda S. M., Stanforth L. M. (1999). Protection from natural enemies in managing rare plant species. Conserv. Biol. 13 (6), 1323–1331. doi: 10.1046/j.1523-1739.1999.98450.x
Bischoff A. (2002). Dispersal and establishment of floodplain grassland species as limiting factors in restoration. Biol. Conserv. 104 (1), 25–33. doi: 10.1016/S0006-3207(01)00151-3
Bower A. D., Clair J. B. S., Erickson V. (2014). Generalized provisional seed zones for native plants. Ecol. Appl. 24 (5), 913–919. doi: 10.1890/13-0285.1
Breland S., Turley N. E., Gibbs J., Isaacs R., Brudvig L. A. (2018). Restoration increases bee abundance and richness but not pollination in remnant and post-agricultural woodlands. Ecosphere 9 (9), e02435. doi: 10.1002/ecs2.2435
Brusca R. C., Wiens J. F., Meyer W. M., Eble J., Franklin K., Overpeck J. T., et al. (2013). Dramatic response to climate change in the southwest: Robert whittaker's 1963 Arizona mountain plant transect revisited. Ecol. Evol. 3 (10), 3307–3319. doi: 10.1002/ece3.720
Bucharova A., Bossdorf O., Hölzel N., Kollmann J., Prasse R., Durka W. (2019). Mix and match: regional admixture provenancing strikes a balance among different seed-sourcing strategies for ecological restoration. Conserv. Genet. 20 (1), 7–17. doi: 10.1007/s10592-018-1067-6
Bucharova A., Durka W., Hölzel N., Kollmann J., Michalski S., Bossdorf O. (2017a). Are local plants the best for ecosystem restoration? it depends on how you analyze the data. Ecol. Evol. 7 (24), 10683–10689. doi: 10.1002/ece3.3585
Bucharova A., Michalski S., Hermann J. M., Heveling K., Durka W., Hölzel N., et al. (2017b). Genetic differentiation and regional adaptation among seed origins used for grassland restoration: lessons from a multispecies transplant experiment. J. Appl. Ecol. 54 (1), 127-136. doi: 10.1111/1365-2664.12645
Buisson E., Anderson S., Holl K. D., Corcket E., Hayes G. F., Peeters A., et al. (2008). Reintroduction of nassella pulchra to California coastal grasslands: Effects of topsoil removal, plant neighbour removal and grazing. Appl. Vegetation Sci. 11 (2), 195–204. doi: 10.3170/2008-7-18357
Bulot A., Potard K., Bureau F., Bérard A., Dutoit T. (2017). Ecological restoration by soil transfer: impacts on restored soil profiles and topsoil functions. Restor. Ecol. 25 (3), 354–366. doi: 10.1111/rec.12424
Byers D. L. (2017). Studying plant–pollinator interactions in a changing climate: A review of approaches. Appl. Plant Sci. 5 (6), 1700012. doi: 10.3732/apps.1700012
Calviño-Cancela M. (2002). Spatial patterns of seed dispersal and seedling recruitment in Corema album (Empetraceae): The importance of unspecialized dispersers for regeneration. J. Ecol. 90 (5), 775–784. doi: 10.1046/j.1365-2745.2002.00711.x
Carbone L. M., Tavella J., Pausas J. G., Aguilar R. (2019). A global synthesis of fire effects on pollinators. Global Ecol. Biogeogr 28 (10), 1487–1498. doi: 10.1111/geb.12939
Cariveau D. P., Bruninga-Socolar B., Pardee G. L. (2020). A review of the challenges and opportunities for restoring animal-mediated pollination of native plants. Emerging Topics Life Sci. 4 (1), 99–109. doi: 10.1042/ETLS20190073
Carrié R., Andrieu E., Cunningham S.A., Lentini P. E., Loreau M., Ouin A. (2016). Relationships among ecological traits of wild bee communities along gradients of habitat amount and fragmentation. Ecography 40 (1), 85–97. doi: 10.1111/ecog.02632
Chaín J. M., Tubert E., Graciano C., Castagno L. N., Recchi M., Pieckenstain F. L., et al. (2020). Growth promotion and protection from drought in eucalyptus grandis seedlings inoculated with beneficial bacteria embedded in a superabsorbent polymer. Sci. Rep. 10 (1), 1–17. doi: 10.1038/s41598-020-75212-4
Chasmer L., Mahoney C., Millard K., Nelson K., Peters D., Merchant M., et al. (2020). Remote sensing of boreal wetlands 2: methods for evaluating boreal wetland ecosystem state and drivers of change. Remote Sens. 12 (8), 1321. doi: 10.3390/rs12081321
Cole A. J., Griffiths R. I., Ward S. E., Whitaker J., Ostle N. J., Bardgett R. D. (2019). Grassland biodiversity restoration increases resistance of carbon fluxes to drought. J. Appl. Ecol. 56 (7), 1806–1816. doi: 10.1111/1365-2664.13402
Collins S. L., Uno G. E. (1985). Seed predation, seed dispersal, and disturbance in grasslands: A comment. Am. Nat. 125 (6), 866–872. doi: 10.1086/284384
Colloff M. J., Pullen K. R., Cunningham S. A. (2010). Restoration of an ecosystem function to revegetation communities: The role of invertebrate macropores in enhancing soil water infiltration. Restor. Ecol. 18 (S1), 65–72. doi: 10.1111/j.1526-100X.2010.00667.x
Cornell H. V., Harrison S. P. (2014). What are species pools and when are they important? Annu. Rev. Ecol Evolution Syst 45 (1), 45–67. doi: 10.1146/annurev-ecolsys-120213-091759
Correa J., Postma J. A., Watt M., Wojciechowski T. (2019). Soil compaction and the architectural plasticity of root systems. J. Exp. Bot. 70 (21), 6019–6034. doi: 10.1093/jxb/erz383
Crawford K. M., Rudgers J. A. (2012). Plant species diversity and genetic diversity within a dominant species interactively affect plant community biomass. J. Ecol. 100 (6), 1512–1521. doi: 10.1111/j.1365-2745.2012.02016.x
Crawford K. M., Rudgers J. A. (2013). Genetic diversity within a dominant plant outweighs plant species diversity in structuring an arthropod community. Ecology 94 (5), 1025–1035. doi: 10.1890/12-1468.1
Crone E. E., Marler M., Pearson D. E. (2009). Non-target effects of broadleaf herbicide on a native perennial forb: A demographic framework for assessing and minimizing impacts. J. Appl. Ecol. 46 (3), 673–682. doi: 10.1111/j.1365-2664.2009.01635.x
Cuthbert M. O., Gleeson T., Moosdorf N., Befus K. M., Schneider A., Hartmann J., et al. (2019). Global patterns and dynamics of climate–groundwater interactions. Nat. Climate Change 9 (2), 137–141. doi: 10.1038/s41558-018-0386-4
Czarniecka-Wiera M., Kącki Z., Chytrý M., Palpurina S. (2019). Diversity loss in grasslands due to the increasing dominance of alien and native competitive herbs. Biodiversity Conserv. 28 (11), 2781–2796. doi: 10.1007/s10531-019-01794-9
Damschen E. I., Baker D. V., Bohrer G., Nathan R., Orrock J. L., Turner J. R., et al. (2014). How fragmentation and corridors affect wind dynamics and seed dispersal in open habitats. Proc. Natl. Acad. Sci. 111 (9), 3484–3489. doi: 10.1073/pnas.1308968111
Damschen E. I., Haddad N. M., Orrock J. L., Tewksbury J. J., Levey D. J. (2006). Corridors increase plant species richness at large scales. Science 313 (5791), 1284–1286. doi: 10.1126/science.1130098
Davidson D. W. (1993). The effects of herbivory and granivory on terrestrial plant succession. Oikos 68 (1), 23–35. doi: 10.2307/3545305
Dean W. R. J., Milton S. J., Klotz S. (1997). The role of ant nest-mounds in maintaining small-scale patchiness in dry grasslands in central Germany. Biodiversity Conserv. 6 (9), 1293–1307. doi: 10.1023/A:1018313025896
Deering R., Young T. (2006). Germination speeds of exotic annual and native perennial grasses in California and the potential benefits of seed priming for grassland restoration. Grasslands 16, 14-15.
Delaney J. T., Jokela K. J., Debinski D. M. (2015). Seasonal succession of pollinator floral resources in four types of grasslands. Ecosphere 6 (11), 1–14. doi: 10.1890/ES15-00218.1
de Vries F. T., Manning P., Tallowin J. R. B., Mortimer S. R., Pilgrim E. S., Harrison K. A., et al. (2012). Abiotic drivers and plant traits explain landscape-scale patterns in soil microbial communities. Ecol. Lett. 15 (11), 1230–1239. doi: 10.1111/j.1461-0248.2012.01844.x
Dickens S. J. M., Allen E. B., Santiago L. S., Crowley D. (2015). Extractable nitrogen and microbial community structure respond to grassland restoration regardless of historical context and soil composition. AoB Plants 7 (1), 1-13. doi: 10.1093/aobpla/plu085
Dickson T. L., Busby W. H. (2009). Forb species establishment increases with decreased grass seeding density and with increased forb seeding density in a northeast Kansas, U.S.A., experimental prairie restoration. Restor. Ecol. 17 (5), 597–605. doi: 10.1111/j.1526-100X.2008.00427.x
Dickson-Hoyle S., Ignace R. E., Ignace M. B., Hagerman S. M., Daniels L. D., Copes-Gerbitz K. (2022). Walking on two legs: A pathway of indigenous restoration and reconciliation in fire-adapted landscapes. Restor. Ecol. 30 (4), e13566. doi: 10.1111/rec.13566
DiGiovanni J. P., Wysocki W. P., Burke S. V., Duvall M. R., Barber N. A. (2017). The role of hemiparasitic plants: influencing tallgrass prairie quality, diversity, and structure. Restor. Ecol. 25 (3), 405–413. doi: 10.1111/rec.12446
Dixon K. W. (2009). Pollination and restoration. Science 325 (5940), 571–573. doi: 10.1126/science.1176295
Djuuna I. A. F., Abbott L. K., Solaiman Z. M. (2009). Use of mycorrhiza bioassays in ecological studies. Berlin, Heidelberg: Springer. 41–50. doi: 10.1007/978-3-540-95894-9_3
Doherty K. D., Grover H. S., Bowker M. A., Durham R. A., Antoninka A. J., Ramsey P. W. (2020). Producing moss-colonized burlap fabric in a fog chamber for restoration of biocrust. Ecol. Eng. 158, 106019. doi: 10.1016/j.ecoleng.2020.106019
Dona A. J., Galen C. (2007). Nurse effects of alpine willows (Salix) enhance over-winter survival at the upper range limit of fireweed, chamerion angustifolium. Arctic Antarctic Alpine Res. 39 (1), 57–64. doi: 10.1657/1523-0430(2007)39[57:NEOAWS]2.0.CO;2
Durbecq A., Jaunatre R., Buisson E., Cluchier A., Bischoff A. (2020). Identifying reference communities in ecological restoration: The use of environmental conditions driving vegetation composition. Restor. Ecol. 28 (6), 1445–1453.
Easterling D. R., Kunkel K. E., Arnold J. R., Knutson T., LeGrande A. N., Leung L. R., et al. (2017). “Precipitation change in the united states,” in Climate science special report: Fourth national climate assessment, volume I. Eds. Wuebbles D. J., Fahey D. W., Hibbard K. A., Dokken D. J., Stewart B. C., Maycock T. K. (Washington, DC, USA: U.S. Global Change Research Program), 207–230. doi: 10.7930/J0H993CC
Ebeling A., Klein A. M., Schumacher J., Weisser W. W., Tscharntke T. (2008). How does plant richness affect pollinator richness and temporal stability of flower visits? Oikos 117 (12), 1808–1815. doi: 10.1111/j.1600-0706.2008.16819.x
Egerton-Warburton L. M. (2015). Aluminum-tolerant Pisolithus ectomycorrhizas confer increased growth, mineral nutrition, and metal tolerance to eucalyptus in acidic mine spoil. Appl. Environ. Soil Sci. 2015, 9. doi: 10.1155/2015/803821
Emery S. M., Thompson D., Rudgers J. A. (2010). Variation in endophyte symbiosis, herbivory and drought tolerance of Ammophila breviligulata populations in the great lakes region. Am. Midland Nat. 163 (1), 186–196. doi: 10.1674/0003-0031-163.1.186
Espíndola A., Ruffley M., Smith M. L., Carstens B. C., Tank D. C., Sullivan J. (2016). Identifying cryptic diversity with predictive phylogeography. Proc. R. Soc. B: Biol. Sci. 283 (1841), 20161529.
Fagan K. C., Pywell R. F., Bullock J. M., Marrs R. H. (2008). Do restored calcareous grasslands on former arable fields resemble ancient targets? the effect of time, methods and environment on outcomes. J. Appl. Ecol. 45 (4), 1293–1303. doi: 10.1111/j.1365-2664.2008.01492.x
Fant J. B., Havens K., Keller J. M., Radosavljevic A., Yates E. D. (2014). The influence of contemporary and historic landscape features on the genetic structure of the sand dune endemic, Cirsium pitcheri (Asteraceae). Heredity 112 (5), 519–530. doi: 10.1038/hdy.2013.134
Fant J. B., Holmstrom R. M., Sirkin E., Etterson J. R., Masi S. (2008). Genetic structure of threatened native populations and propagules used for restoration in a clonal species, American beachgrass (Ammophila breviligulata fern.). Restor. Ecol. 16 (4), 594–603. doi: 10.1111/j.1526-100X.2007.00348.x
Fant J. B., Kramer A., Sirkin E., Havens K. (2013). Genetics of reintroduced populations of the narrowly endemic thistle, Cirsium pitcheri (Asteraceae). Botany 91 (5), 301-308. doi: 10.1139/cjb-2012-0232
Fekete I., Varga C., Biró B., Tóth J. A., Várbíró G., Lajtha K., et al. (2016). The effects of litter production and litter depth on soil microclimate in a central european deciduous forest. Plant Soil 398 (1), 291–300. doi: 10.1007/s11104-015-2664-5
Finch J., Walck J. L., Hidayati S. N., Kramer A. T., Lason V., Havens K. (2019). Germination niche breadth varies inconsistently among three Asclepias congeners along a latitudinal gradient. Plant Biol. 21 (3), 425–438. doi: 10.1111/plb.12843
Fløjgaard C., Valdez J. W., Dalby L., Moeslund J. E., Clausen K. K., Ejrnæs R., et al. (2020). Dark diversity reveals importance of biotic resources and competition for plant diversity across habitats. Ecol. Evol. 10 (12), 6078–6088. doi: 10.1002/ece3.6351
Forest S. B., Nilsson M.-C., Wardle D. A. (2005). Understory vegetation as a forest ecosystem driver: Evidence from the northern Swedish Boreal forest. Front. Ecol. Environ. 3 (8), 421–428. doi: 10.1890/1540-9295(2005)003[0421:UVAAFE]2.0.CO;2
Foster B. L., Murphy C. A., Keller K. R., Aschenbach T. A., Questad E. J., Kindscher K. (2007). Restoration of prairie community structure and ecosystem function in an abandoned hayfield: A sowing experiment. Restor. Ecol. 15 (4), 652–661. doi: 10.1111/j.1526-100X.2007.00277.x
Fox C. W., Reed D. H. (2011). Inbreeding depression increases with environmental stress: An experimental study and meta-analysis. Evolution 65 (1), 246–258. doi: 10.1111/j.1558-5646.2010.01108.x
Fox K., Vitt P., Anderson K., Fauske G., Travers S., Vik D., et al. (2013). Pollination of a threatened orchid by an introduced hawk moth species in the tallgrass prairie of north America. Biol. Conserv. 167, 316–324. doi: 10.1016/j.biocon.2013.08.026
Foxx A. J., Fort F. (2019). Root and shoot competition lead to contrasting competitive outcomes under water stress: A systematic review and meta-analysis. PloS One 14 (12), 1–17. doi: 10.1371/journal.pone.0220674
Frankham R. (2005). Genetics and extinction. Biol. Conserv. 126 (2), 131–140. doi: 10.1016/j.biocon.2005.05.002
Frankham R. (2015). Genetic rescue of small inbred populations: meta-analysis reveals large and consistent benefits of gene flow. Mol. Ecol. 24 (11), 2610–2618. doi: 10.1111/mec.13139
Frankham R., Ballou J. D., Eldridge M. D. B. B., Lacy R. C., Ralls K., Dudash M. R., et al. (2011). Predicting the probability of outbreeding depression. Conserv. Biol. 25 (3), 465–475. doi: 10.1111/j.1523-1739.2011.01662.x
Frankham R., Ballou J. D., Ralls K., Eldridge M., Dudash M. R., Fenster C. B., et al. (2017). Genetic management of fragmented animal and plant populations (Oxford, UK: Oxford University Press). doi: 10.1093/oso/9780198783398.001.0001
Frankham R., Bradshaw C. J. A., Brook B. W. (2014). Genetics in conservation management: Revised recommendations for the 50/500 rules, red list criteria and population viability analyses. Biol. Conserv. 170, 56–63. doi: 10.1016/j.biocon.2013.12.036
Fraser L. H., Madson E. B. (2008). The interacting effects of herbivore exclosures and seed addition in a wet meadow. Oikos 117 (7), 1057–1063. doi: 10.1111/j.0030-1299.2008.16251.x
Funk J. L., Vitousek P. M. (2007). Resource-use efficiency and plant invasion in low-resource systems. Nature 446 (7139), 1079–1081. doi: 10.1038/nature05719
Gallagher M. K., Wagenius S. (2016). Seed source impacts germination and early establishment of dominant grasses in prairie restorations. J. Appl. Ecol. 53, 251–263. doi: 10.1111/1365-2664.12564
Gann G., Mcdonald T., Walder B., Aronson J., Nelson C., Jonson J., et al. (2019). International principles and standards for the practice of ecological restoration. 2nd ed. (Restoration Ecology), S1–S46.
Gavin-Smyth N., Kramer A. T., Urbina-Casanova R., Vitt P., Fant J. B. (2021). Genetic rescue reduces mate limitation in a threatened, clonal, and self-incompatible plant species. Restor. Ecol. 29 (8), e13458. doi: 10.1111/rec.13458
Gelmi-Candusso T. A., Bialozyt R., Slana D., Zárate Gómez R., Heymann E. W., Heer K. (2019). Estimating seed dispersal distance: A comparison of methods using animal movement and plant genetic data on two primate-dispersed Neotropical plant species. Ecol. Evol. 9 (16), 8965–8977. doi: 10.1002/ece3.5422
Ghimire S. R., Charlton N. D., Craven K. D. (2009). The mycorrhizal fungus, sebacina vermifera, enhances seed germination and biomass production in switchgrass (Panicum virgatum l). Bioenergy Res. 2 (1), 51–58. doi: 10.1007/s12155-009-9033-2
Gitzendanner M. A., Weekley C. W., Germain-Aubrey C. C., Soltis D. E., Soltis P. S. (2012). Microsatellite evidence for high clonality and limited genetic diversity in Ziziphus celata (Rhamnaceae), an endangered, self-incompatible shrub endemic to the lake Wales ridge, Florida, USA. Conserv. Genet. 13 (1), 223–234. doi: 10.1007/s10592-011-0287-9
Godefroid S., Piazza C., Rossi G., Buord S., Stevens A. D., Aguraiuja R., et al. (2011). How successful are plant species reintroductions? Biol. Conserv. 144 (2), 672–682. doi: 10.1016/j.biocon.2010.10.003
Goldman M. A., Needelman B. A., Rabenhorst M. C., Lang M. W., McCarty G. W., King P. (2020). Digital soil mapping in a low-relief landscape to support wetland restoration decisions. Geoderma 373, 114420. doi: 10.1016/j.geoderma.2020.114420
Gonzalez S. L., Ghermandi L. (2019). Dwarf shrub facilitates seedling recruitment and plant diversity in semiarid grasslands. PloS One 14 (2), e0212058. doi: 10.1371/journal.pone.0212058
Grman E., Bassett T., Zirbel C. R., Brudvig L. A. (2015). Dispersal and establishment filters influence the assembly of restored prairie plant communities. Restor. Ecol. 23 (6), 892–899. doi: 10.1111/rec.12271
Groom M. J. (1998). Allee effects limit population viability of an annual plant. Am. Nat. 151 (6), 487–496. doi: 10.1086/286135
Groves A. M., Brudvig L. A. (2019). Interannual variation in precipitation and other planting conditions impacts seedling establishment in sown plant communities. Restor. Ecol. 27 (1), 128–137. doi: 10.1111/rec.12708
Guittar J., Goldberg D., Klanderud K., Berge A., Ramírez Boixaderas M., Meineri E., et al. (2020). Quantifying the roles of seed dispersal, filtering, and climate on regional patterns of grassland biodiversity. Ecology 101 (10), e03061. doi: 10.1002/ecy.3061
Gurney C. M., Prugh L. R., Brashares J. S. (2015). Restoration of native plants is reduced by rodent-caused soil disturbance and seed removal. Rangeland Ecol. Manage. 68 (4), 359–366. doi: 10.1016/j.rama.2015.05.001
Gutiérrez J. M., Jones R. G., Narisma G. T., Alves L. M., Amjad M., Gorodetskaya I. V., et al. (2021). “Atlas,” in Climate change 2021: The physical science basis. contribution of working group I to the sixth assessment report of the intergovernmental panel on climate change. Eds. Masson-Delmotte V., Zhai P., Pirani A., Connors S. L., Péan C., Berger S., Caud N., Chen Y., Goldfarb L., Gomis M. I., Huang M., Leitzell K., Lonnoy E., Matthews J. B. R., Maycock T. K., Waterfield T., Yelekçi O., Yu R., Zhou B. (Cambridge, United Kingdom and New York, NY, USA: Cambridge University Press). Available at: http://interactive-atlas.ipcc.ch/.
Halsey S. J., Bell T. J., Bowles M. (2017). Initial transplant size and microsite influence transplant survivorship and growth of a threatened dune thistle. Ecol. Restor. 35 (1), 52–59. doi: 10.3368/er.35.1.52
Hare M. L., Xu X. W., Wang Y. D., Yuan Y., Gedda A. E. (2021). Do woody tree thinning and season have effect on grass species’ composition and biomass in a semi-arid savanna? the case of a semi-arid savanna, southern Ethiopia. Front. Environ. Sci. 9, 692239. doi: 10.3389/fenvs.2021.692239
Havens K., Jolls C. L., Marik J. E., Vitt P., McEachern A. K., Kind D. (2012). Effects of a non-native biocontrol weevil, larinus planus, and other emerging threats on populations of the federally threatened pitcher’s thistle, cirsium pitcheri. Biol. Conserv. 155, 202–211. doi: 10.1016/j.biocon.2012.06.010
Havens K., Vitt P. (2016). The importance of phenological diversity in seed mixes for pollinator restoration. Natural Areas J. 36, 500–506. doi: 10.3375/043.036.0418
Havens K., Vitt P., Still S., Kramer A. T., Fant J. B., Schatz K. (2015). Seed sourcing for restoration in an era of climate change. Natural Areas J. 35 (1), 122–133. doi: 10.3375/043.035.0116
Hereford J. (2009). A quantitative survey of local adaptation and fitness trade-offs. Am. Nat. 173 (5), 579–588. doi: 10.1086/597611
Hernández E., Shaw E. A., Aoyama L., Brambila A., Niederer C., Weiss S. B., et al. (2021). Fire versus grazing as tools to restore serpentine grasslands under global change. Restor. Ecol. 29 (S1), e13353. doi: 10.1111/rec.13353
Hillhouse H. L., Zedler P. H. (2011). Native species establishment in tallgrass prairie plantings. Am. Midland Nat. 166 (2), 292–308. doi: 10.1674/0003-0031-166.2.292
Hirsch A. M., Lum M. R., Downie J. A. (2001). What makes the rhizobia-legume symbiosis so special? Plant Physiol. 127 (4), 1484–1492.
Howe H. F. (2016). Making dispersal syndromes and networks useful in tropical conservation and restoration. Global Ecol. Conserv. 6, 152–178. doi: 10.1016/j.gecco.2016.03.002
Howe H. F., Brown J. S., Zorn-Arnold B. (2002). A rodent plague on prairie diversity. Ecol. Lett. 5 (1), 30–36. doi: 10.1046/j.1461-0248.2002.00276.x
Howe H. F., Brown J. S. (1999). Effects of birds and rodents on synthetic tallgrass communities. Ecology 80 (5), 1776–1781.
Hufford K. M., Krauss S. L., Veneklaas E. J. (2012). Inbreeding and outbreeding depression in Stylidium hispidum: Implications for mixing seed sources for ecological restoration. Ecol. Evol. 2 (9), 2262–2273. doi: 10.1002/ece3.302
Hulme P. E., Benkman C. W. (2002). “Granivory,” in Plant–animal interactions: an evolutionary approach (John Wiley & Sons), 132–154.
Igic B., Lande R., Kohn J. R. (2008). Loss of self-incompatibility and its evolutionary consequences. Int. J. Plant Sci. 169 (1), 93–104. doi: 10.1086/523362
Izumi Y., Okaichi S., Awala S. K., Kawato Y., Watanabe Y., Yamane K., et al. (2018). Water supply from pearl millet by hydraulic lift can mitigate drought stress and improve productivity of rice by the close mixed planting. Plant Production Sci. 21 (1), 8–15. doi: 10.1080/1343943X.2018.1428494
IPBES (2019). Summary for policymakers of the global assessment report on biodiversity and ecosystem services of the Intergovernmental Science-Policy Platform on Biodiversity and Ecosystem Services. Eds. Díaz S., Settele J., Brondízio E. S., Ngo H. T., Guèze M., Agard J. (Bonn, Germany: IPBES secretariat), 56.
James J. J., Rinella M. J., Svejcar T. (2012). Grass seedling demography and sagebrush steppe restoration. Rangeland Ecol. Manage. 65 (4), 409–417. doi: 10.2111/REM-D-11-00138.1
Jones H. P., Jones P. C., Barbier E. B., Blackburn R. C., Benayas J. M. R., Holl K. D., et al. (2018). Restoration and repair of earth's damaged ecosystems. Proc. R. Soc. B: Biol. Sci. 285 (1873), 20172577. doi: 10.1098/rspb.2017.2577
Kardol P., Wardle D. A. (2010). How understanding aboveground-belowground linkages can assist restoration ecology. Trends Ecol. Evol. 25 (11), 670–679. doi: 10.1016/j.tree.2010.09.001
Keller L. F., Waller D. M. (2002). Inbreeding effects in wild populations. Trends Ecol. Evol. 17 (5), 230–241. doi: 10.1016/S0169-5347(02)02489-8
Kelly D., Sullivan J. J. (1997). Quantifying the benefits of mast seeding on predator satiation and wind pollination in Chionochloa pallens (Poaceae). Oikos 78 (1), 143–150. doi: 10.2307/3545810
Kelly S. A., Takbiri Z., Belmont P., Foufoula-Georgiou E. (2017). Human amplified changes in precipitation–runoff patterns in large river basins of the Midwestern United States. Hydrology Earth System Sci. 21 (10), 5065–5088.
Kettenring K. M., Tarsa E. E. (2020). Need to seed? ecological, genetic, and evolutionary keys to seed-based wetland restoration. Front. Environ. Sci. 8. doi: 10.3389/fenvs.2020.00109
Kildisheva O. A., Dixon K. W., Silveira F. A. O., Chapman T., Di Sacco A., Mondoni A., et al. (2020). Dormancy and germination: making every seed count in restoration. Restor. Ecol. 28 (S3), S256–S265. doi: 10.1111/rec.13140
Kimmerer R. W., Lake F. K. (2001). Maintaining the mosaic: The role of indigenous burning in land management. J. Forestry 99 (11), 36–41. doi: 10.1093/jof/99.11.36
Kim E. S., Zaya D. N., Fant J. B., Ashley M. V. (2014). Genetic factors accelerate demographic decline in rare asclepias species. Conserv. Genet. 16 (2), 359–369. doi: 10.1007/s10592-014-0663-3
Kindscher K., Tieszen L. L. (1998). Floristic and soil organic matter changes after five and thirty-five years of native tallgrass prairie restoration. Restor. Ecol. 6 (2), 181–196. doi: 10.1111/j.1526-100X.1998.06210.x
Kirchner F., Robert A., Colas B. (2006). Modelling the dynamics of introduced populations in the narrow-endemic centaurea corymbosa: A demo-genetic integration. J. Appl. Ecol. 43 (5), 1011–1021. doi: 10.1111/j.1365-2664.2006.01179.x
Klaus V. H., Kiehl K. (2021). A conceptual framework for urban ecological restoration and rehabilitation. Basic Appl. Ecol. 52, 82–94. doi: 10.1016/j.baae.2021.02.010
Knapp A. K., Chen A., Griffin-Nolan R. J., Baur L. E., Carroll C. J. W., Gray J. E., et al. (2020). Resolving the dust bowl paradox of grassland responses to extreme drought. Proc. Natl. Acad. Sci. 117 (36), 22249–22255. doi: 10.1073/pnas.1922030117
Knapp E. E., Estes B. L., Skinner C. N. (2009). Ecological effects of prescribed fire season: a literature review and synthesis for managers (United States Department of Agriculture Forest Service, Pacific Southwest Research Station, General Technical Report PSW-GTR-224).
Knight T. M., Steets J. A., Vamosi J. C., Mazer S. J., Burd M., Campbell D. R., et al. (2005). Pollen limitation of plant reproduction: Pattern and process. Annu. Rev. Ecol Evol. Syst 36, 467–497. doi: 10.1146/annurev.ecolsys.36.102403.115320
Koski M. H., Ison J. L., Padilla A., Pham A. Q., Galloway L. F. (2018). Linking pollinator efficiency to patterns of pollen limitation: Small bees exploit the plant-pollinator mutualism. proceedings of the royal society b. Biol. Sci. 285 (1880), 20180635. doi: 10.1098/rspb.2018.0635
Koziol L., Schultz P. A., House G. L., Bauer J. T., Middleton E. L., Bever J. D. (2018). The plant microbiome and native plant restoration: The example of native mycorrhizal fungi. BioScience 68 (12), 996–1006. doi: 10.1093/biosci/biy125
Kramer A. T., Wood T. E., Frischie S., Havens K. (2018). Considering ploidy when producing and using mixed-source native plant materials for restoration. Restor. Ecol. 26 (1), 13–19. doi: 10.1111/rec.12636
Kremen C., Williams N. M., Bugg R. L., Fay J. P., Thorp R. W. (2004). The area requirements of an ecosystem service: Crop pollination by native bee communities in California. Ecol. Lett. 7 (11), 1109–1119. doi: 10.1111/j.1461-0248.2004.00662.x
Kulmatiski A., Beard K. H., Stevens J. R., Cobbold S. M. (2008). Plant-soil feedbacks: A meta-analytical review. Ecol. Lett. 11 (9), 980–992. doi: 10.1111/j.1461-0248.2008.01209.x
Kulpa S. M., Leger E. A. (2013). Strong natural selection during plant restoration favors an unexpected suite of plant traits. Evolutionary Appl. 6 (3), 510–523. doi: 10.1111/eva.12038
Kurkjian H. M., Carothers S. K., Jules E. S. (2017). Seed predation has the potential to drive a rare plant to extinction. J. Appl. Ecol. 54 (3), 862–871. doi: 10.1111/1365-2664.12808
Ladouceur E., Shackelford N., Bouazza K., Brudvig L., Bucharova A., Conradi T., et al. (2022). Knowledge sharing for shared success in the decade on ecosystem restoration. Ecol. Solutions Evidence 3, e12117. doi: 10.1002/2688-8319.12117
Lamb N., Havens K., Holloway J., Steffen J. F., Zeldin J., Kramer A. T. (2022). “Low passive restoration potential following invasive woody species removal in oak woodlands,” in Restoration ecology, vol. 30. doi: 10.1111/rec.13568
Lau J. A., Magnoli S. M., Zirbel C. R., Brudvig L. A. (2019). The limits to adaptation in restored ecosystems and how management can help overcome them. Ann. Missouri Botanical Garden 104 (3), 441–454. doi: 10.3417/2019430
Lawrence B. A., Kaye T. N. (2008). Direct and indirect effects of host plants: Implications for reintroduction of an endangered hemiparasitic plant (Castilleja levisecta). Madroño 55 (2), 151–158. doi: 10.3120/0024-9637(2008)55[151:daieoh]2.0.co;2
Lawrence B. A., Kaye T. N. (2011). Reintroduction of castilleja levisecta: effects of ecological similarity, source population genetics, and habitat quality. Restor. Ecol. 19 (2), 166–176. doi: 10.1111/j.1526-100X.2009.00549.x
Leach M. K., Givnish T. J. (1996). Ecological determinants of species loss in remnant prairies. Science 273, 1555–1558. doi: 10.1126/science.273.5281.1555
Leberg P. L., Firmin B. D. (2008). Role of inbreeding depression and purging in captive breeding and restoration programmes. Mol. Ecol. 17 (1), 334–343. doi: 10.1111/j.1365-294X.2007.03433.x
Leger E. A. (2008). The adaptive value of remnant native plants in invaded communities: an example from the great basin. Ecol. Appl. 18 (5), 1226–1235. doi: 10.1890/07-1598.1
Leimu R., Fischer M. (2008). A meta-analysis of local adaptation in plants. PloS One 3 (12), 1-8. doi: 10.1371/journal.pone.0004010
Leopold C. R., Hess S. C. (2019). Facilitating adaptation to climate change while restoring a montane plant community. PloS One 14 (6), e0218516. doi: 10.1371/journal.pone.0218516
Levey D. J., Sargent S. (2000). A simple method for tracking vertebrate-dispersed seeds. Ecology 81 (1), 267–274. doi: 10.1890/0012-9658(2000)081[0267:ASMFTV]2.0.CO;2
Levin D. A., Kelley C. D., Sarkar S. (2009). Enhancement of allee effects in plants due to self-incompatibility alleles. J. Ecol. 97 (3), 518–527. doi: 10.1111/j.1365-2745.2009.01499.x
Linabury M. C., Turley N. E., Brudvig L. A. (2019). Insects remove more seeds than mammals in first-year prairie restorations. Restor. Ecol. 27 (6), 1300–1306. doi: 10.1111/rec.13004
Lindsell J. A., Lee D. C., Powell V. J., Gemita E. (2015). Availability of large seed-dispersers for restoration of degraded tropical forest. Trop. Conserv. Sci. 8 (1), 17–27. doi: 10.1177/194008291500800104
Longland W. S., Ostoja S. M. (2013). Ecosystem services from keystone species: diversionary seeding and seed-caching desert rodents can enhance Indian ricegrass seedling establishment. Restor. Ecol. 21 (2), 285–291. doi: 10.1111/j.1526-100X.2012.00895.x
Louda S. M., O’Brien C. W. (2002). Unexpected ecological effects of distributing the exotic weevil, larinus planus (F.), for the biological control of Canada thistle. Conserv. Biol. 16 (3), 717–727. doi: 10.1046/j.1523-1739.2002.00541.x
Louda S. M., Pemberton R. W., Johnson M. T., Follett P. A. (2003). Nontarget effects - the achilles’ heel of biological control? Retrospective analyses to reduce risk associated with biocontrol introductions. Annu. Rev. Entomol 48, 365–396. doi: 10.1146/annurev.ento.48.060402.102800
Loydi A., Eckstein R. L., Otte A., Donath T. W. (2013). Effects of litter on seedling establishment in natural and semi-natural grasslands: A meta-analysis. J. Ecol. 101 (2), 454–464. doi: 10.1111/1365-2745.12033
Luijten S. H., Dierick A., Gerard J., Oostermeijer B., Raijmann L. E. L., Den Nijs H. C. M. (2000). Population size, genetic variation, and reproductive success in a rapid declining, self-incompatible perennial (Arnica montana) in the Netherlands. Conserv. Biol. 14 (6), 1776–1787. doi: 10.1046/j.1523-1739.2000.99345.x
Macdougall A. S., Turkington R. (2007). Does the type of disturbance matter when restoring disturbance-dependent grasslands? Restor. Ecol. 15 (2), 263–272. doi: 10.1111/j.1526-100X.2007.00209.x
Maltz M. R., Treseder K. K. (2015). Sources of inocula influence mycorrhizal colonization of plants in restoration projects: A meta-analysis. Restor. Ecol. 23 (5), 625–634. doi: 10.1111/rec.12231
Mangla S., Sheley R. L., James J. J., Radosevich S. R. (2011). Intra and interspecific competition among invasive and native species during early stages of plant growth. Plant Ecol. 212 (4), 531-542. doi: 10.1007/s11258-011-9909-z
Maron J. L. (1997). Interspecific competition and insect herbivory reduce bush lupine (Lupinus arboreus) seedling survival. Oecologia 110, 284–290. doi: 10.1007/s004420050161
Maron J. L., Crone E. (2006). Herbivory: Effects on plant abundance, distribution and population growth. Proc. R. Soc. B: Biol. Sci. 273 (1601), 2575–2584. doi: 10.1098/rspb.2006.3587
Matthies D. (2017). Interactions between a root hemiparasite and 27 different hosts: Growth, biomass allocation and plant architecture. Perspect. Plant Ecol Evol. Syst 24, 118–137. doi: 10.1016/j.ppees.2016.12.006
McDonald T., Gann G., Jonson J., Dixon K. (2016). International standards for the practice of ecological restoration – including principles and key concepts (Washington, D. C: Society for Ecological Restoration).
McGlaughlin M., Karoly K., Kaye T. (2002). Genetic variation and its relationship to population size in reintroduced populations of pink sand verbena, abronia umbellata subsp. breviflora (Nyctaginaceae). Conserv. Genet. 3 (4), 411–420. doi: 10.1023/A:1020507416654
McKay J. K., Christian C. E., Harrison S., Rice K. J. (2005). "How local is local?" a review of practical and conceptual issues in the genetics of restoration. Restor. Ecol. 13 (3), 432–440. doi: 10.1111/j.1526-100X.2005.00058.x
McLaughlin G. M., Dearden P. K. (2019). Invasive insects: Management methods explored. J. Insect Sci. 19 (5), 1-9. doi: 10.1093/jisesa/iez085
McManamen C., Nelson C. R., Wagner V. (2018). Timing of seeding after herbicide application influences rates of germination and seedling biomass of native plants used for grassland restoration. Restor. Ecol. 26 (6), 1137–1148. doi: 10.1111/rec.12679
Meissen J. C., Glidden A. J., Sherrard M. E., Elgersma K. J., Jackson L. L. (2020). Seed mix design and first year management influence multifunctionality and cost-effectiveness in prairie reconstruction. Restor. Ecol. 28 (4), 807–816. doi: 10.1111/rec.13013
Menges E. S. (1991). Seed germination percentage increases with population size in a fragmented prairie species. Conserv. Biol. 5 (2), 158–164. doi: 10.1111/j.1523-1739.1991.tb00120.x
Middleton E. L., Richardson S., Koziol L., Palmer C. E., Yermakov Z., Henning J. A., et al. (2015). Locally adapted arbuscular mycorrhizal fungi improve vigor and resistance to herbivory of native prairie plant species. Ecosphere 6 (12), 1–16. doi: 10.1890/ES15-00152.1
Mitchell N., Campbell L. G., Ahern J. R., Paine K. C., Giroldo A. B., Whitney K. D. (2019). Correlates of hybridization in plants. Evol. Lett. 3 (6), 570–585. doi: 10.1002/evl3.146
Moeslund J. E., Brunbjerg A. K., Clausen K. K., Dalby L., Fløjgaard C., Juel A., et al. (2017). Using dark diversity and plant characteristics to guide conservation and restoration. J. Appl. Ecol. 54 (6), 1730–1741. doi: 10.1111/1365-2664.12867
Molano-Flores B. (2004). Breeding systems of plants used for prairie restorations: a review. Trans. Illinois State Acad. Sci. 97 (2), 95–102.
Molano-Flores B., Feist M. A., Whelan C. J. (2003). Seed germination, seedling survivorship, and host preference of Agalinis auriculata (Michx.) Blake (Orobanchaceae), an Illinois, USA, threatened species. Natural Areas J. 23 (2), 152–157.
Naeth M. A., Fernández A. C. C., Mollard F. P., Yao L., Wilkinson S. R., Jiao Z. (2018). Enriched topographic microsites for improved native grass and forb establishment in reclamation. Rangeland Ecol. Manage. 71 (1), 12–18. doi: 10.1016/j.rama.2017.08.004
Ollerton J., Winfree R., Tarrant S. (2011). How many flowering plants are pollinated by animals? Oikos 120 (3), 321–326. doi: 10.1111/j.1600-0706.2010.18644.x
Ooi M. K. J. (2012). Seed bank persistence and climate change. Seed Sci. Res. 22 (S1), S53–S60. doi: 10.1017/S0960258511000407
Opperman J. J., Merenlender A. M. (2000). Deer herbivory as an ecological constraint to restoration of degraded riparian corridors. Restor. Ecol. 8 (1), 41–47. doi: 10.1046/j.1526-100x.2000.80006.x
Orrock J. L., Witter M. S., Reichman O. J. (2009). Native consumers and seed limitation constrain the restoration of a native perennial grass in exotic habitats. Restor. Ecol. 17 (1), 148–157. doi: 10.1111/j.1526-100X.2008.00384.x
Ott J. P., Butler J. L., Rong Y., Xu L. (2017). Greater bud outgrowth of bromus inermis than pascopyrum smithii under multiple environmental conditions. J. Plant Ecol. 10 (3), 518–527. doi: 10.1093/jpe/rtw045
Overbeck G., Kiehl K., Abs C. (2003). Seedling recruitment of succisella inflexa in fen meadows: Importance of seed and microsite availability. Appl. Vegetation Sci. 6 (1), 97–104. doi: 10.1111/j.1654-109X.2003.tb00568.x
Padilla F. M., Pugnaire F. I. (2006). The role of nurse plants in the restoration of degraded environments. Front. Ecol. Environ. 4 (4), 196–202. doi: 10.1890/1540-9295(2006)004[0196:TRONPI]2.0.CO;2
Palmer M. A., Zedler J. B., Falk D. A. (2017). Ecological theory and restoration ecology. Foundations Restor. Ecol: Second Edition 5 (4), 3–26. doi: 10.5822/978-1-61091-698-1_1
Paluch E. C., Thomsen M. A., Volk T. J. (2013). Effects of resident soil fungi and land use history outweigh those of commercial mycorrhizal inocula: Testing a restoration strategy in unsterilized soil. Restor. Ecol. 21 (3), 380–389. doi: 10.1111/j.1526-100X.2012.00894.x
Paschke M. W. (1997). Actinorhizal plants in rangelands of the Western united states. J. Range Manage. 50 (1), 62–72. doi: 10.2307/4002707
Pedrini S., Balestrazzi A., Madsen M. D., Bhalsing K., Hardegree S. P., Dixon K. W., et al. (2020). Seed enhancement: getting seeds restoration-ready. Restor. Ecol. 28, S266–S275. doi: 10.1111/rec.13184
Pellish C. A., Sherrard M. E., Leytem P. A., Jackson L. L. (2018). Small vertebrate granivores reduce seedling emergence in native tallgrass prairie restoration. Restor. Ecol. 26 (2), 323–330. doi: 10.1111/rec.12557
Pender R. J., Shiels A. B., Bialic-Murphy L., Mosher S. M. (2013). Large-Scale rodent control reduces pre-and post-dispersal seed predation of the endangered Hawaiian lobeliad, cyanea superba subsp. superba (Campanulaceae). Biol. Invasions 15 (1), 213–223. doi: 10.1007/s10530-012-0280-3
Pennings S. C., Callaway R. M. (1996). Impact of a parasitic plant on the structure and dynamics of salt marsh vegetation. Ecology 77 (5), 1410–1419. doi: 10.2307/2265538
Pires M. M., Guimarães P. R., Galetti M., Jordano P. (2018). Pleistocene megafaunal extinctions and the functional loss of long-distance seed-dispersal services. Ecography 41 (1), 153–163. doi: 10.1111/ecog.03163
Platt B. F., Kolb D. J., Kunhardt C. G., Milo S. P., New L. G. (2016). Burrowing through the literature: The impact of soil-disturbing vertebrates on physical and chemical properties of soil. Soil Sci. 181 (3/4), 175–191. doi: 10.1097/SS.0000000000000150
Poschlod P., Kiefer S., Tränkle U., Fischer S., Bonn S. (1998). Plant species richness in calcareous grasslands as affected by dispersability in space and time. Appl. Vegetation Sci. 1 (1), 75–91. doi: 10.2307/1479087
Prach K., Hobbs R. J. (2008). Spontaneous succession versus technical reclamation in the restoration of disturbed sites. Restor. Ecol. 16 (3), 363–366. doi: 10.1111/j.1526-100X.2008.00412.x
Press M. C., Phoenix G. K. (2005). Impacts of parasitic plants on natural communities. New Phytol. 166 (3), 737–751. doi: 10.1111/j.1469-8137.2005.01358.x
Prior K. M., Robinson J. M., Meadley Dunphy S. A., Frederickson M. E. (2015). Mutualism between co-introduced species facilitates invasion and alters plant community structure. proceedings of the royal society b. Biol. Sci. 282, 20142846. doi: 10.1098/rspb.2014.2846
Pueyo Y., Alados C. L., García-Ávila B., Kéfi S., Maestro M., Rietkerk M. (2009). Comparing direct abiotic amelioration and facilitation as tools for restoration of semiarid grasslands. Restor. Ecol. 17 (6), 908–916. doi: 10.1111/j.1526-100X.2008.00474.x
Querejeta J. I., Egerton-Warburton L. M., Allen M. F. (2009). Topographic position modulates the mycorrhizal response of oak trees to interannual rainfall variability. Ecology 90 (3), 649–662. doi: 10.1890/07-1696.1
Rahman M., Lee S. H., Ji H. C., Kabir A. H., Jones C. S., Lee K. W. (2018). Importance of mineral nutrition for mitigating aluminum toxicity in plants on acidic soils: current status and opportunities. Int. J. Mol. Sci. 19 (10), 3073. doi: 10.3390/ijms19103073
Ren Y. Q., Guan K. Y., Li A. R., Hu X. J., Zhang L. (2010). Host dependence and preference of the root hemiparasite, pedicularis cephalantha Franch.(Orobanchaceae). Folia Geobotanica 45 (4), 443–455. doi: 10.1007/s12224-010-9081-6
Renner S. S., Zohner C. M. (2018). Climate change and phenological mismatch in trophic interactions among plants, insects, and vertebrates. Annu. Rev. ecol evolution syst 49 (1), 165–182. doi: 10.1146/annurev-ecolsys-110617-062535
Reynolds H. L., Packer A., Bever J. D., Clay K. (2003). Grassroots ecology: plant–microbe–soil interactions as drivers of plant community structure and dynamics. Ecology 84 (9), 2281–2291. doi: 10.1890/02-0298
Rhoades C. C., Eckert G. E., Coleman D. C. (1998). Effect of pasture trees on soil nitrogen and organic matter: implications for tropical montane forest restoration. Restor. Ecol. 6 (3), 262–270. doi: 10.1046/j.1526-100X.1998.00639.x
Richardson B. A., Chaney L. (2018). Climate-based seed transfer of a widespread shrub: population shifts, restoration strategies, and the trailing edge. Ecol. Appl. 28 (8), 2165–2174. doi: 10.1002/eap.1804
Riebkes J. L., Barak R. S., Kramer A. T. (2015). Evaluating seed viability in prairie forbs: a test of three methods. Native Plants J. 16 (2), 96–106. doi: 10.3368/npj.16.2.96
Rinella M. J., Espeland E. K., Moffatt B. J. (2016). Studying long-term, large-scale grassland restoration outcomes to improve seeding methods and reveal knowledge gaps. J. Appl. Ecol. 53 (5), 1565–1574. doi: 10.1111/1365-2664.12722
Robertson G. P., Coleman D., Bledsoe C., Sollins P. (1999). Standard soil methods for long-term ecological research (Oxford, UK: Oxford University Press).
Robinson J. A., Räikkönen J., Vucetich L. M., Vucetich J. A., Peterson R. O., Lohmueller K. E., et al. (2019). Genomic signatures of extensive inbreeding in isle royale wolves, a population on the threshold of extinction. Sci. Adv. 5 (5), eaau0757. doi: 10.1126/sciadv.aau0757
Rocha I., Ma Y., Souza-Alonso P., Vosátka M., Freitas H., Oliveira R. S. (2019). Seed coating: A tool for delivering beneficial microbes to agricultural crops. Front. Plant Sci. 10. doi: 10.3389/fpls.2019.01357
Rosas C. A., Engle D. M., Shaw J. H., Palmer M. W. (2008). Seed dispersal by bison bison in a tallgrass prairie. J. Vegetation Sci. 19 (6), 769–778. doi: 10.3170/2008-8-18447
Rose K. E., Louda S. M., Rees M. (2005). Demographic and evolutionary impacts of native and invasive insect herbivores on cirsium canescens. Ecology 86 (2), 453–465. doi: 10.1890/03-0697
Rosenberger K., Schumacher E., Brown A., Hoban S. (2021). Proportional sampling strategy often captures more genetic diversity when population sizes vary. Biol. Conserv. 261, 109261. doi: 10.1016/j.biocon.2021.109261
Rosenzweig S. T., Carson M. A., Baer S. G., Blair J. M. (2016). Changes in soil properties, microbial biomass, and fluxes of c and n in soil following post-agricultural grassland restoration. Appl. Soil Ecol. 100, 186–194. doi: 10.1016/j.apsoil.2016.01.001
Rowe H. I. (2010). Tricks of the trade: techniques and opinions from 38 experts in tallgrass prairie restoration. Restor. Ecol. 18, 253–262. doi: 10.1111/j.1526-100X.2010.00663.x
Scherber C., Crawley M. J., Porembski S. (2003). The effects of herbivory and competition on the invasive alien plant senecio inaequidens (Asteraceae). Diversity Distributions 9 (6), 415–426. doi: 10.1046/j.1472-4642.2003.00049.x
Schultz C. B., Ferguson J. A. (2020). Demographic costs and benefits of herbicide-based restoration to enhance habitat for an endangered butterfly and a threatened plant. Restor. Ecol. 28 (3), 564–572. doi: 10.1111/rec.13102
Seahra S., Yurkonis K. A., Newman. J. A. (2019). Seeding tallgrass prairie in monospecific patches promotes native species establishment and cover. Restor. Ecol. 27 (1), 82–91. doi: 10.1111/rec.12715
Selby G. (2007). Regal fritillary (Speyeria idalia drury): a technical conservation assessment (Rocky Mountain Region: USDA Forest Service). Species Conservation Project.
Shackelford N., Dudney J., Stueber M. M., Temperton V. M., Suding K. L. (2022). Measuring at all scales: sourcing data for more flexible restoration references. Restor. Ecol., e13541. doi: 10.1111/rec.13541
Shackelford N., Paterno G. B., Winkler D. E., Erickson T. E., Leger E. A., Svejcar L. N., et al. (2021). Drivers of seedling establishment success in dryland restoration efforts. Nat. Ecol. Evol. 5 (9), 1283–1290. doi: 10.1038/s41559-021-01510-3
Sharma S., Tiwari S., Hasan A., Saxena V., Pandey L. M. (2018). Recent advances in conventional and contemporary methods for remediation of heavy metal-contaminated soils. 3 Biotech. 8 (4), 1–18. doi: 10.1007/s13205-018-1237-8
Shaw N., Barak R. S., Campbell R. E., Kirmer A., Pedrini S., Dixon K., et al. (2020). Seed use in the field: delivering seeds for restoration success. Restor. Ecol. 28 (S3), S276–S285. doi: 10.1111/rec.13210
Silvertown J., Araya Y., Gowing D. (2015). Hydrological niches in terrestrial plant communities: A review. J. Ecol. 103 (1), 93–108. doi: 10.1111/1365-2745.12332
Sluis W. (2020). Dark diversity in restorations: What’s missing? Ecol. Restor. 38 (3), 180–192. doi: 10.3368/ER.38.3.180
Sluis W. J., Bowles M., Jones M. (2018). Multiscale metrics differentiate among tallgrass prairie restorations and remnant ecosystems along a restorative continuum. Restor. Ecol. 26 (3), 466–475. doi: 10.1111/rec.12578
Smith M. R., Charvat I., Jacobson R. L. (1998). Arbuscular mycorrhizae promote establishment of prairie species in a tallgrass prairie restoration. Can. J. Bot. 76 (11), 1947–1954. doi: 10.1139/b98-205
Sollenberger D., Kadlec C., O'Shaughnessy J., Egerton-Warburton L. (2016). Environmental filtering mediates grassland community assembly following restoration with soil carbon additions. Restor. Ecol. 24 (5), 626–636. doi: 10.1111/rec.12362
St. Clair A. B., Dunwiddie P. W., Fant J. B., Kaye T. N., Kramer A. T. (2020). Mixing source populations increases genetic diversity of restored rare plant populations. Restor. Ecol. 28 (3), 583–593. doi: 10.1111/rec.13131
Steffan-Dewenter I., Tscharntke T. (1999). International association for ecology effects of habitat isolation on pollinator communities and seed set. Oecologia 121 (3), 432–440. doi: 10.1007/s004420050949
Steyaert S. M. J. G., Hertel A. G., Swenson J. E. (2019). Endozoochory by brown bears stimulates germination in bilberry. Wildlife Biol. 2019 (1), 1–5. doi: 10.2981/wlb.00573
Suding K. N. (2011). Toward an era of restoration in ecology: Successes, failures, and opportunities ahead. Annu. Rev. Ecol Evolution Syst 42 (1), 465–487. doi: 10.1146/annurev-ecolsys-102710-145115
Taylor J. B., Cass K. L., Armond D. N., Madsen M. D., Pearson D. E., St. Clair S. B. (2020). Deterring rodent seed-predation using seed-coating technologies. Restor. Ecol. 28 (4), 927–936. doi: 10.1111/rec.13158
Těšitel J., Mládek J., Horník J., Těšitelová T., Adamec V., Tichý L. (2017). Suppressing competitive dominants and community restoration with native parasitic plants using the hemiparasitic rhinanthus alectorolophus and the dominant grass calamagrostis epigejos. J. Appl. Ecol. 54 (5), 1487–1495. doi: 10.1111/1365-2664.12889
Thrall P. H., Encinas-Viso F., Hoebee S. E., Young A. G. (2014). Life history mediates mate limitation and population viability in self-incompatible plant species. Ecol. Evol. 4 (6), 673–687. doi: 10.1002/ece3.963
Török P., Brudvig L. A., Kollmann J., N Price J., Tóthmérész B. (2021). The present and future of grassland restoration. Restor. Ecol. 29, e13378. doi: 10.1111/rec.13378
Townsend P. A., Levey D. J. (2005). An experimental test of whether habitat corridors affect pollen transfer. Ecology 86 (2), 466–475. doi: 10.1890/03-0607
Turley N. E., Bell-Dereske L., Evans S. E., Brudvig L. A. (2020). Agricultural land-use history and restoration impact soil microbial biodiversity. J. Appl. Ecol. 57 (5), 852–863. doi: 10.1111/1365-2664.13591
Tuya F., Vila F., Bergasa O., Zarranz M., Espino F., Robaina R. R. (2017). Artificial seagrass leaves shield transplanted seagrass seedlings and increase their survivorship. Aquat. Bot. 136, 31–34. doi: 10.1016/j.aquabot.2016.09.001
United Nations (2019) Resolution adopted by the general assembly on 1 march 2019. Available at: https://undocs.org/A/RES/73/284 (Accessed 13 May 2022).
Urbanek R. E., Nielsen C. K., Glowacki G. A., Preuss T. S. (2012). White-tailed deer (Odocoileus virginianus zimm.) herbivory in herbaceous plant communities in northeastern Illinois. Natural Areas J. 32 (1), 6–14. doi: 10.3375/043.032.0103
US Fish and Wildlife Service (2007). “Brighamia insignis (Olulu) 5-year review,” in Summary and evaluation (Pacific Island Fish and Wildlife Office, Honolulu, Hawai‘i: US Fish and Wildlife Service).
Valiente-Banuet A., Ezcurra E. (1991). Shade as a cause of the association between the cactus neobuxbaumia tetetzo and the nurse plant mimosa luisana in the tehuacan valley, Mexico. J. Ecol. 79 (4), 961. doi: 10.2307/2261091
Van Den Berg L. J. L., Vergeer P., Roelofs J. G. M. (2003). Heathland restoration in the Netherlands: Effects of turf cutting depth on germination of arnica montana. Appl. Vegetation Sci. 6 (2), 117–124. doi: 10.1111/j.1654-109x.2003.tb00571.x
Vanden Broeck A., Ceulemans T., Kathagen G., Hoffmann M., Honnay O., Mergeay J. (2015). Dispersal constraints for the conservation of the grassland herb thymus pulegioides l. in a highly fragmented agricultural landscape. Conserv. Genet. 16 (4), 765–776. doi: 10.1007/s10592-015-0698-0
Van Der Heijden M. G., Klironomos J. N., Ursic M., Moutoglis P., Streitwolf-Engel R., Boller T., et al. (1998). Mycorrhizal fungal diversity determines plant biodiversity, ecosystem variability and productivity. Nature 396 (6706), 69–72. doi: 10.1038/23932
Van Der Valk A. G., Bremholm T. L., Gordon E. (1999). The restoration of sedge meadows: Seed viability, seed germination requirements, and seedling growth of carex species. Wetlands 19 (4), 756–764. doi: 10.1007/BF03161782
Van Rossum F., Destombes A., Raspé O. (2021). Are large census-sized populations always the best sources for plant translocations? Restor. Ecol. 29 (3), e13316. doi: 10.1111/rec.13316
Vaz Ferreira A., Bruna E. M., Vasconcelos H. L. (2011). Seed predators limit plant recruitment in Neotropical savannas. Oikos 120 (7), 1013–1022. doi: 10.1111/j.1600-0706.2010.19052.
Vellend M. (2010). Conceptual synthesis in community ecology. Q. Rev. Biol. 85 (2), 183–206. doi: 10.1086/652373
Vickery P. D. (2002). Effects of the size of prescribed fire on insect predation of northern blazing star, a rare grassland perennial. Conserv. Biol. 16 (2), 413–421. doi: 10.1046/j.1523-1739.2002.00494.x
Vierheilig H., Coughlan A. P., Wyss U. R. S., Piché Y. (1998). Ink and vinegar, a simple staining technique for arbuscular-mycorrhizal fungi. Appl. Environ. Microbiol. 64 (12), 5004–5007. doi: 10.1128/AEM.64.12.5004-5007.1998
Wagenius S., Beck J., Kiefer G. (2020). Fire synchronizes flowering and boosts reproduction in a widespread but declining prairie species. Proc. Natl. Acad. Sci. United States America 117 (6), 3000–3005. doi: 10.1073/pnas.1907320117
Wagenius S., Lyon S. P. (2010). Reproduction of echinacea angustifolia in fragmented prairie is pollen-limited but not pollinator-limited. Ecology 91 (3), 733–742. doi: 10.1890/08-1375.1
Wallin L., Svensson B. M., Lönn M. (2009). Artificial dispersal as a restoration tool in meadows: sowing or planting? Restor. Ecol. 17 (2), 270–279. doi: 10.1111/j.1526-100X.2007.00350.x
Walsh S. K., Pender R. J., Junker R. R., Daehler C. C., Morden C. W., Lorence D. H. (2019). “Pollination biology reveals challenges to restoring populations of brighamia insignis (Campanulaceae), a critically endangered plant species from hawai’i,” in Flora: Morphology, distribution, functional ecology of plants, vol. 259. , 151448. doi: 10.1016/j.flora.2019.151448
Wasson K., Tanner K. E., Woofolk A., Mccain S., Suraci J. P. (2021). Top-down and sideways: Herbivory and cross-ecosystem connectivity shape restoration success at the salt marsh-upland ecotone. PloS One 16 (2), e0247374. doi: 10.1371/journal.pone.0247374
Wang B., Qiu Y. L. (2006). Phylogenetic distribution and evolution of mycorrhizas in land plants. Mycorrhiza 16 (5), 299–363.
Wei Z., Van Le Q., Peng W., Yang Y., Yang H., Gu H., et al. (2021). A review on phytoremediation of contaminants in air, water and soil. J. hazardous mater 403, 123658. doi: 10.1016/j.jhazmat.2020.123658
White J. A., Tallaksen J., Charvat I. (2008). The effects of arbuscular mycorrhizal fungal inoculation at a roadside prairie restoration site. Mycologia 100 (1), 6–11. doi: 10.3852/mycologia.100.1.6
Williams D. W., Jackson L. L., Smith D. D. (2007). Effects of frequent mowing on survival and persistence of forbs seeded into a species-poor grassland. Restor. Ecol. 15 (1), 24–33. doi: 10.1111/j.1526-100X.2006.00186.x
Willi Y., van Kleunen M., Dietrich S., Fischer M. (2007). Genetic rescue persists beyond first-generation outbreeding in small populations of a rare plant. Proc. R. Soc. B: Biol. Sci. 274 (1623), 2357–2364. doi: 10.1098/rspb.2007.0768
Winfree R. (2010). The conservation and restoration of wild bees. Ann. New York Acad. Sci. 1195 (1), 169–197. doi: 10.1111/j.1749-6632.2010.05449.x
Xiao Y., Li X., Cao Y., Dong M. (2016). Towards an idea-centered, principle-base design to as creation approach support learning knowledge. Plant Ecol. 217 (7), 857–868. doi: 10.1007/sl
Yoshida S., Cui S., Ichihashi Y., Shirasu K. (2016). The haustorium, a specialized invasive organ in parasitic plants. Annu. Rev. Plant Biol. 67, 643–667. doi: 10.1146/annurev-arplant-043015-111702
Young A. G., Pickup M. (2010). Low s-allele numbers limit mate availability, reduce seed set and skew fitness in small populations of a self-incompatible plant. J. Appl. Ecol. 47 (3), 541–548. doi: 10.1111/j.1365-2664.2010.01798.x
Keywords: restoring plant diversity, germination bottleneck, establishment limitation, abiotic and biotic filters, restoration genetics, mutualism and antagonism, soil ecology and microbiome, restoration planning and land management
Citation: De Vitis M, Havens K, Barak RS, Egerton-Warburton L, Ernst AR, Evans M, Fant JB, Foxx AJ, Hadley K, Jabcon J, O’Shaughnessey J, Ramakrishna S, Sollenberger D, Taddeo S, Urbina-Casanova R, Woolridge C, Xu L, Zeldin J and Kramer AT (2022) Why are some plant species missing from restorations? A diagnostic tool for temperate grassland ecosystems. Front. Conserv. Sci. 3:1028295. doi: 10.3389/fcosc.2022.1028295
Received: 25 August 2022; Accepted: 06 October 2022;
Published: 24 October 2022.
Edited by:
Brenda Molano-Flores, Illinois Natural History Survey (INHS), United StatesReviewed by:
John Taft, Illinois Natural History Survey (INHS), United StatesThomas Edward Marler, University of Guam, Guam
Eric Ulaszek, Illinois Natural History Survey (INHS), United States
Copyright © 2022 De Vitis, Havens, Barak, Egerton-Warburton, Ernst, Evans, Fant, Foxx, Hadley, Jabcon, O’Shaughnessey, Ramakrishna, Sollenberger, Taddeo, Urbina-Casanova, Woolridge, Xu, Zeldin and Kramer. This is an open-access article distributed under the terms of the Creative Commons Attribution License (CC BY). The use, distribution or reproduction in other forums is permitted, provided the original author(s) and the copyright owner(s) are credited and that the original publication in this journal is cited, in accordance with accepted academic practice. No use, distribution or reproduction is permitted which does not comply with these terms.
*Correspondence: Andrea T. Kramer, YWtyYW1lckBjaGljYWdvYm90YW5pYy5vcmc=