Advances in cryopreservation of Syzygium maire (swamp maire, maire tawake) zygotic embryos, a critically endangered tree species endemic to New Zealand
- 1Otari Native Botanic Garden, Wellington City Council, Wellington, New Zealand
- 2New Cultivar Innovation, The New Zealand Institute for Plant and Food Research Limited, Palmerston North, New Zealand
- 3Independent Researcher, Videira, Brazil
Introduction: Syzygium maire is a threatened Myrtaceae tree species endemic to New Zealand. Due to its highly recalcitrant seed, cryopreservation is the only viable long-term ex situ conservation option for this species. Our previous attempts to cryopreserve the embryonic axis (EAs) of S. maire were unsuccessful but did provide a better understanding of desiccation behavior, biochemical composition, oxidative status, and ultrastructural changes associated with desiccation in EAs.
Methods: We incorporated this knowledge with biophysical information to investigate two advanced cryopreservation technologies: a droplet vacuum infiltration vitrification (DVIV) method and a novel metal-mesh vacuum infiltration vitrification (MVIV) method using Plant Vitrification Solution 2 (PVS2) for cryopreservation of the EAs.
Results: The PVS2 treatment at room temperature (~20°C) proved phytotoxic with extended PVS2 incubation significantly reducing EA survival. No EAs survived cryopreservation using DVIV, however MVIV resulted in post-cryopreservation survival of up to 19% following PVS2 incubation for 20 min. Biophysical thermal analysis using Differential Scanning Calorimetry revealed a 15-fold reduction in ice crystallization following incubation in PVS2 for 20 min or more, with all freezable water removed after 60 min incubation.
Discussion: These results present a significant advance in being able to successfully cryopreserve S. maire EAs. The findings from this study will aid the development of cryopreservation protocols for other extremely recalcitrant seeded species, many of which are threatened with extinction due to climate change, plant pathogens, and habitat destruction.
1 Introduction
Syzygium Gaertn. is the largest genus in the Myrtaceae family and with more than 6000 species, it is the tree genus with the most species globally (POWO, 2023). The Pacific region is considered the center of diversity for Syzygium which originated in Australia-New Guinea, diversifying eastwards to the Pacific and westwards to India and Africa (Low et al., 2022). S. aromaticum (glove), commonly used as a spice, is the best-known species in the genus while S. aqueum, S. cumini, S. jambos, S. malaccense, and S. samarangense are widely cultivated for their fruits (Nair, 2017). Medicinal properties have been formally studied for at least 24 Syzygium species and there is anecdotal evidence of medicinal or traditional use for many more species in Africa, Australia, and India (Goyal and Ayeleso, 2019). Moreover, natural compounds such as phenolics, oleanolic acid, betulinic acid and dimethyl cardamonin associated with Syzygium species have been found to inhibit the proliferation of cancer cells (Chua et al., 2019).
Although most Syzygium species are considered tropical or sub-tropical, one species, S. maire, is endemic to the temperate regions of New Zealand (Dawson et al., 2011) where it grows in coastal or lowland riparian forests in waterlogged ground or on the margins of streams (Dawson et al., 2011). S. maire is a glabrous tree up to 16 m high with smooth, grey-brown bark often flaking in irregular shards. Pneumatophores or the specialized aerial root systems adapted for oxygen uptake in plants growing in swamps, or muddy environments, are common on S. maire (Figures 1A, B). The white flowers, produced in clusters of up to 35 in number on terminal racemes, are approximately 90 to100 mm in diameter. The fruits are fleshy berries developing over 8 to 11 months turning bright red when mature (Figure 1C). Although polyembryony (multiple embryos arising within the embryo sac of the zygotic proembryo) is characteristic of many Syzygium species (Shü et al., 2011; Sivasubramaniam and Selvarani, 2012), S. maire generally bears a single embryo (Figure 1D).
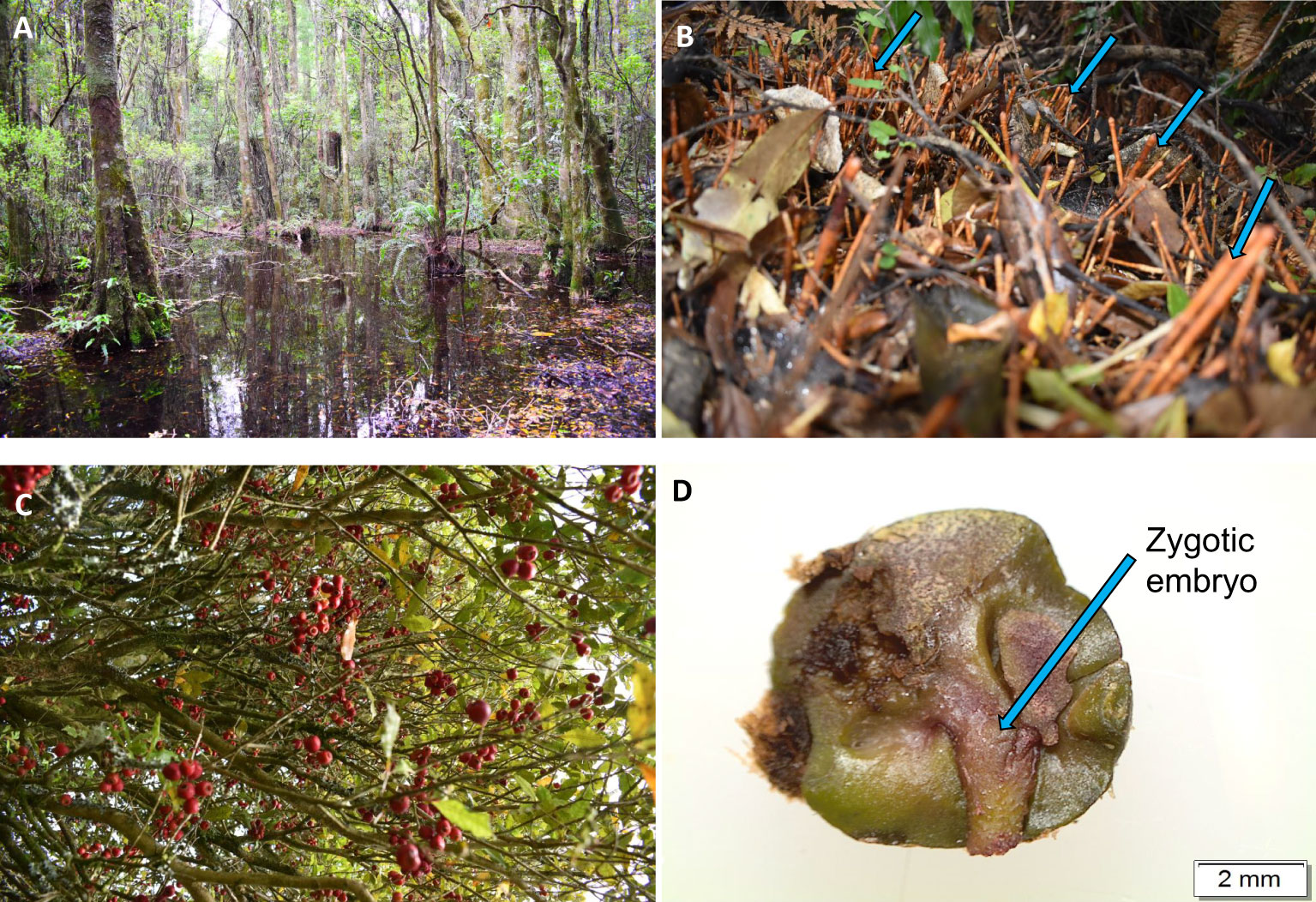
Figure 1 Syzygium maire grows in muddy, waterlogged habitat (A) and commonly produces pneumatophores (specialized aerial root system as indicated in blue arrows) (B) to aid in oxygen uptake. The red fleshy berries (C) mature in summer with usually a single embryo per seed (D).
Despite the ecological (van der Walt et al., 2021b), commercial (Nair, 2017) and pharmaceutical (Chua et al., 2019) values of Syzygium species, their conservation is largely limited to in situ conservation such as nature reserves and living collections. Living trees are vulnerable to climate change (Fernandez et al., 2023) and invasive plant pathogens such as Austropuccinia psidii (Myrtle Rust) (Beresford et al., 2020), making ex situ conservation using techniques such as seed banking essential. Seed banking is an effective ex situ method for the long-term preservation of plant genetic resources and an efficient form of conservation for plant genetic diversity (Nadarajan et al., 2023). However, all studies published to date report that Syzygium species shed their seeds with high moisture contents (> 44%), and the seeds are sensitive to desiccation (van der Walt et al., 2021b; Sharanya et al., 2022) with post-harvest seed lifespan limited to less than six months (Anandalakshmi et al., 2005; Coelho de Araújo et al., 2008) suggesting banking of S. maire seed is impossible.
The long-term storage of desiccation sensitive or recalcitrant seeds remains a challenge for many species (Wesley-Smith et al., 2001), and although embryo cryopreservation is an option, its application is complicated by their heterogeneous tissues, chemical composition, and high metabolic activity (Berjak and Pammenter, 2008; Nadarajan and Pritchard, 2014). Despite the low or lack of tolerance to desiccation in recalcitrant species, if material is to be cryopreserved, it is essential that excess water is removed to prevent the formation of lethal ice crystals during freezing (Fuller et al., 2004; Reed, 2008). This presents additional challenges for developing successful cryopreservation protocols for highly recalcitrant seeds.
In a previous study, we investigated the effectiveness of rapid desiccation for the cryopreservation of S. maire zygotic embryonic axis (EAs) (van der Walt et al., 2022). We found that desiccation to moisture contents (MC) below 0.2 g H2O per g dry matter (g/g) was required to remove all freezable water, but this proved lethal to the EAs. Another indirect method to remove freezable water from the cells through an osmotic process is the use of permeable/colligative cryoprotectants, such as Plant Vitrification Solution 2 (PVS2), to achieve vitrification or a glassy state in plant cells (Sakai, 2004). We previously investigated the use of PVS2 for the cryopreservation of S. maire EAs, comparing the novel droplet vacuum infiltration vitrification (DVIV) method with conventional droplet vitrification (DV) (van der Walt et al., 2021a). Our study showed that DVIV treated embryos had better survival and regeneration formation than DV for non-cryopreserved treatments. However, neither method resulted in embryo survival following cryopreservation, possibly attributable to insufficient removal of all freezable water using the PVS2 incubation times tested (3, 6, 9, and 12 min at room temperature (~20°C)). One way to evaluate the efficacy of cryoprotectants in removing all freezable water, and therefore achieving a glassy state, is through biophysical thermal analysis using differential scanning calorimetry (DSC) (Nadarajan and Pritchard, 2014). Water quantity can be calculated as the energy or enthalpy changes in the form of heat absorbance or release using DSC. In addition, water phase transitions i.e., ice crystallization and melting can be monitored in DSC analyzed samples as a function of time and temperature (Benson et al., 1996; Benson, 2008b). This enables optimization of the dehydration treatment time with cryoprotectants i.e., when all the freezable water has been removed (Benson, 2008a) and correlation of post-cryopreservation survival with water thermal transitions (Volk and Walters, 2006).
Given the lack of post-cryopreservation survival in our previous studies, this study aimed to further investigate fundamental principles of S. maire EA cryopreservation. We followed a two-phased approach. In the first year, we further optimized the DVIV method by increasing PVS2 incubation times and assessed its impact on EA survival and regeneration before and after exposure to liquid nitrogen (LN). In the second year, we evaluated modified cryopreservation solutions and a different growth media and designed a novel metal-mesh vacuum infiltration vitrification (MVIV) method to allow rapid permeation of the cryoprotectant followed by rapid cooling and warming of the EAs. To gain insight into the mechanism of cryoprotection for each PVS2 exposure time, water thermal properties were analyzed using a DSC. We measured the size and temperature of exothermic and endothermic events within the EAs treated with PVS2. We aimed to reveal the optimal timing window for PVS2 cryoprotectant incubation of S. maire EAs with input from DSC thermal analysis.
2 Materials and methods
2.1 Seed material, embryo excision, and sterilization
For the DVIV experiment in year 1, mature S. maire fruits were collected in March 2022 from Midhirst in central North Island of New Zealand (39°17′55.02″ S; 174°11′16.56″ E). For the MVIV experiment, in Year 2, the fruits were collected from the same location in March 2023. In both years, collected fruits were placed in sealed plastic bags, transported to the laboratory, stored at 5°C and used within 24 h of collection. Prior to embryo excision, fruits were surface sterilized by immersion in 50% commercial bleach (Janola®, 4% sodium hypochlorite as active ingredient) for 5 min and rinsed three times with tap water. Excision of EAs (c. 3–5 mm in size) followed the procedures described by van der Walt et al. (2022). Following excision, for DVIV experiments, EAs were sterilized by immersion in 5 g/L sodium dichloroisocyanurate (NaDCC) (Sigma Aldrich, USA) solution for 10 min then rinsed three times using sterile distilled water, while for MVIV, EAs were sterilized by immersion in 5 g/L NaDCC solution for 20 min followed by 1 min in 70% ethanol and then rinsed three times using sterile distilled water. Surface sterilized EAs were used for all the cryopreservation experiments.
2.2 Experiment 1: droplet vacuum infiltration vitrification cryopreservation
2.2.1 Cryopreservation solutions and growth media
Solutions used for the cryopreservation protocol included loading solution (LS), PVS2 and washing solution (WS). The LS consisted of Murashige and Skoog (1962) (MS) macronutrients and micronutrients supplemented with 2.0 M glycerol and 0.4 M sucrose. The PVS2 was made up of MS macronutrients and micronutrients supplemented with 0.4 M sucrose, 30% w/v glycerol, 15% w/v ethylene glycol and 15% w/v dimethyl sulfoxide (DMSO) (Sakai et al., 1990). The WS was made with MS macronutrients and micronutrients supplemented with 1.2 M sucrose. The growth medium (GM) comprised MS macronutrients and micronutrients supplemented with 30 g/L sucrose and 10 mL/L antibiotic antimycotic solution (AAS) (Sigma Aldrich, USA), solidified with 7.5 g/L agar. All solutions and media were adjusted to pH 5.8 prior to autoclaving.
2.2.2 PVS2 treatment and DVIV cryopreservation
EAs were placed in LS at room temperature (20 ± 2°C) for 20 min followed by incubation in PVS2 for 0 (control), 3, 6, 9, 12, 20, 40 or 60 min. PVS2 incubation was also done at room temperature under vacuum at 381 mm (15 in) Hg (50 kPa) applied using a single stage vacuum pump (Aitcool VP 130, China) and 5 L stainless steel vacuum chamber. These PVS2 treated EAs were transferred onto aluminium foil strips (length 60 x width 10 mm) (10 EAs per strip), each EA was covered with a drop of fresh PVS2 and then plunged into LN (+LN). After 60 min in LN, aluminium foil strips containing the EAs were transferred into WS pre-warmed to 40°C in a sterile glass beaker and held in water bath (set at 40 ± 2°C) for 2 min and then maintained for 18 min at room temperature. For –LN (without immersing in LN), the aluminum strips containing EAs were transferred into WS for 20 min at room temperature following PVS2 incubation immersing in LN. Following the WS step, EAs from +LN and –LN treatments were blotted dry on a filter paper, transferred to GM, and incubated in a growth chamber at 12:12h cycle of 15/25°C in the dark for 1 week before exposure to a 12-h photoperiod at a photosynthetic flux density of 40 μmols−1 m−2 provided by cool-white, fluorescent tubes at same temperature. The ability of EAs to survive and regenerate following various PVS2 incubation times and LN exposure (+LN and –LN) was determined. The survival and regeneration assessments were conducted for both non-cryopreserved (–LN) and cryopreserved (+LN) EAs. EAs were considered to have survived when they remained green for 2 weeks following freezing and considered regenerated when the EAs showed radicle emergence (>5 mm) and the first cotyledonary leaves development thus forming a full plantlet. The survival and regeneration assessment were made weekly post-culture on GM. The workflow of this experimental procedure is summarized in Figure 2.
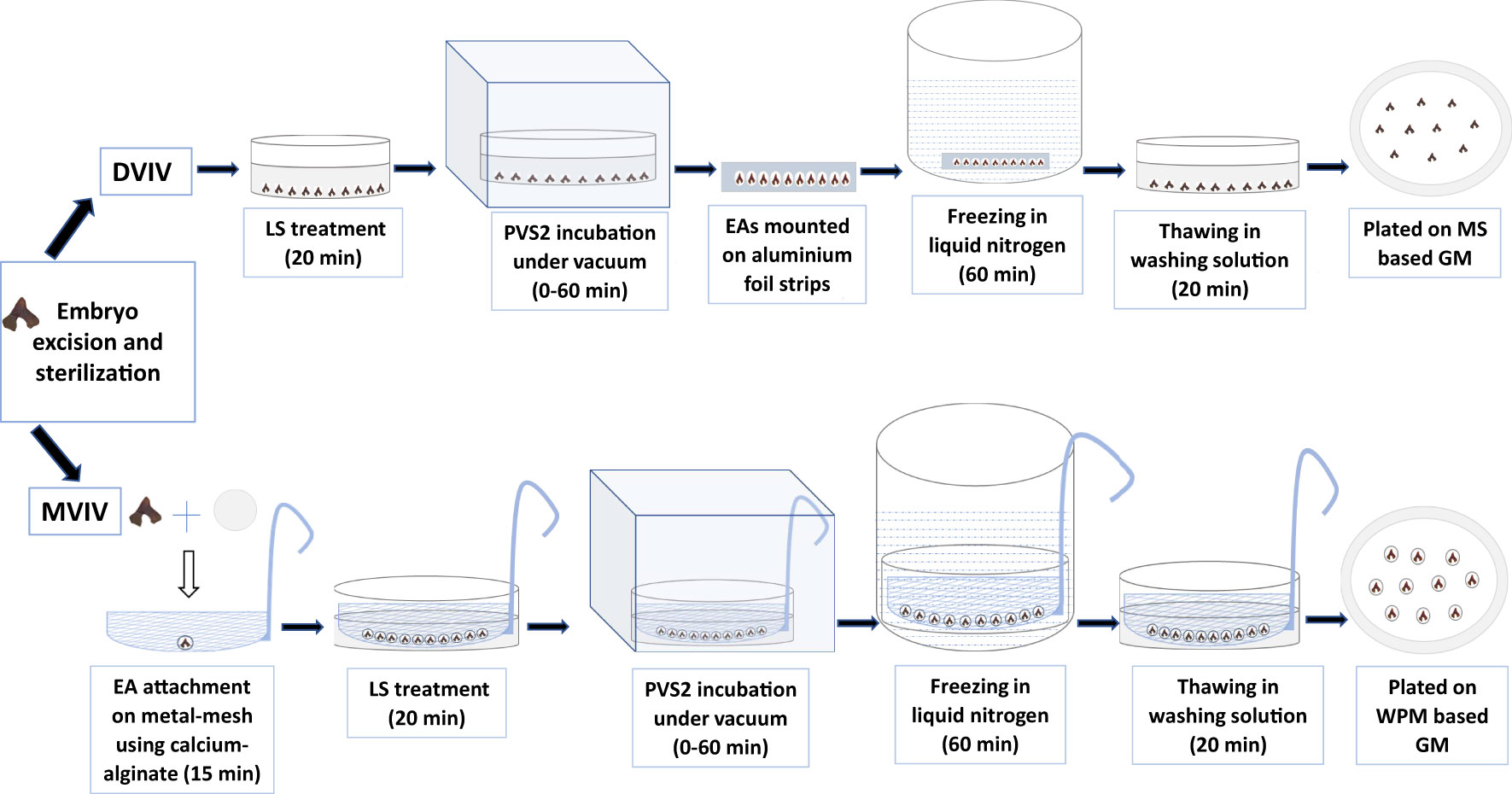
Figure 2 Droplet vacuum infiltration vitrification (DVIV) and metal-mesh vacuum infiltration vitrification (MVIV) methods for cryopreservation of Syzygium maire embryonic axis (EAs). LS, loading solution; PVS2, Plant Vitrification Solution 2; GM, growth medium; MS, Murashige & Skoog Medium; WPM, Woody Plant Medium.
2.3 Experiment 2: metal-mesh vacuum infiltration vitrification cryopreservation
2.3.1 Cryopreservation solutions and growth media
The LS consisted of half-strength Woody Plant Medium (McCown and Lloyd, 1981) (WPM) macronutrients and MS micronutrients supplemented with 1.6 M glycerol and 0.4 M sucrose. The PVS2 was made up of WPM macronutrients and MS micronutrients supplemented with 0.4 M sucrose, 30% w/v glycerol, 15% w/v ethylene glycol and 15% w/v DMSO. The WS composed of half-strength WPM macronutrients and MS micronutrients supplemented with 1.2 M sucrose. The sodium alginate (BDH Chemicals limited, England) (3% (w/v)) solution consisted of WPM macronutrients without CaCl2, MS micronutrients, 2 M glycerol and 0.4 M sucrose. The calcium chloride solution consisted of WPM macronutrients without CaCl2, MS micronutrients 10 mL/L AAS and 11 g/L CaCl2, 2 M glycerol and 0.4 M sucrose. The GM comprised of WPM macronutrients and MS micronutrients, supplemented with 2 mg/L zeatin, 1 mg/L copper sulphate, 20 g/L sucrose and solidified with 4 g/L Gelrite. The pH was adjusted to 5.8 prior to filter-sterilization of all solutions and autoclaving of GM, and 0.2 g/L AsA was supplemented after respective sterilization.
2.3.2 PVS2 treatment and MVIV cryopreservation
For MVIV, a 80 mm diameter sterile stainless-steel mesh with pore sizes of 1 mm2 (Supplementary Figures 1A, B) was placed over a 90 mm deep Petri dish. Ten drops (1 ml each) of sodium alginate solution, spaced 1-cm apart, were placed on the mesh and topped with a drop of calcium chloride solution. A single EA was placed on to the semi-formed bead and allowed to polymerize for 15 min under reduced light by covering the mesh with aluminium foil to avoid direct exposure to lights during this process. The mesh containing 10 EAs, each in its own bead, was submerged into LS in a deep Petri dish for 20 min at room temperature. The mesh was then transferred to PVS2 solution at room temperature in a deep Petri dish and incubated for 0 (control), 20, 40 or 60 min under vacuum as described above, thereafter the mesh containing the EAs was plunged into LN. After 60 min in LN, the mesh containing the EAs was transferred to warm (40 ± 2°C) WS as described in DVIV cryopreservation. For –LN, the mesh containing EAs were transferred into WS for 20 min at room temperature following PVS2 incubation without immersing in LN. The EAs from +LN and –LN were carefully detached from the mesh and were then blotted dry using sterile filter paper before transferring to liquid GM. They were incubated in a growth chamber at 12:12 h cycle of 15/25°C in the dark for 2 weeks before transfer to a 12-h photoperiod at a photosynthetic flux density of 40 μmols−1m−2 provided by cool-white fluorescent tubes. The survival and regeneration assessments were conducted as previously described for both non-cryopreserved (–LN) and cryopreserved (+LN) EAs. The workflow of this experimental procedure is summarized in Figure 2.
2.4 Differential scanning calorimetry
Thermal behavior in EAs incubated in PVS2 for both the DVIV and MVIV methods was determined using a Perkin-Elmer differential scanning calorimeter (DSC 8500) (Shelton, USA), calibrated for temperature and heat flow with zinc (melting point 419.5°C) and indium (melting point 156.6°C). One EA per treatment (control, LS and various PVS2 exposure times) was blotted dry and hermetically sealed into a (60 µL volume) stainless steel capsule (sample pan) using an O-ring with the aid of a Perkin-Elmer Universal Crimper (Shelton, USA). EA weight was measured using a micro-balance (Model: XPR6UD5; Mettler-Toledo, Switzerland), and the EA was subjected to calorimetric assessment within 5 min of sample preparation. EAs were cooled from 25°C to −100°C and held for 1 min before rewarming to 25°C at a rate of 10°C min−1. Exothermic- and endothermic-heat changes were derived from the crystallization and melt endotherm during the cooling and warming cycles. The onset temperature of the transition was determined as the temperature at which the tangent of the sharpest portion of the first peak intersected the baseline. The onset, peak and end temperatures were calculated using PYRIS software (Version 13.2). The areas of melt and crystallization peaks were calculated from the area above the baseline and expressed in millijoules (mJ). The enthalpies for these transitions are presented as joule per gram of sample weight (J/g). Calorimetric data were collected from three replicates per treatment.
2.5 Statistical analysis
All experiments involved 7–12 EAs for each treatment (average 9.9), repeated four times (a total of 35–41 EAs per treatment, average 39.7). Survival and regeneration assessment results were analyzed using logistic regression, followed by Fisher’s protected LSD (least significant difference) test for significantly different means (at p < 0.05). ANOVA followed by Tukey’s HSD (honestly significant difference) test was used to investigate differences in onset of melt temperature, freeze temperature and enthalpy of the melt. ANOVA assumptions were checked by visual inspection of residual plots. Enthalpy of melt data were log-transformed before analysis to equalize variances. All statistical analysis was performed at 0.05 level of significance, and results are expressed as mean ± SD. Statistical analysis was conducted using XLStat Software version 1.3 (2021) and SAS/STAT version 14.2.
3 Results
3.1 Experiment 1: EA survival and regeneration following droplet vacuum infiltration vitrification cryopreservation
EA survival and regeneration (–LN) were not affected by exposure to PVS2 for up to 12 min (Figure 3). However, exposure to PVS2 for longer than 20 min significantly reduced survival and regeneration following 60-min exposure (Figure 3). There was no survival in EAs exposed to cryopreservation (+LN).
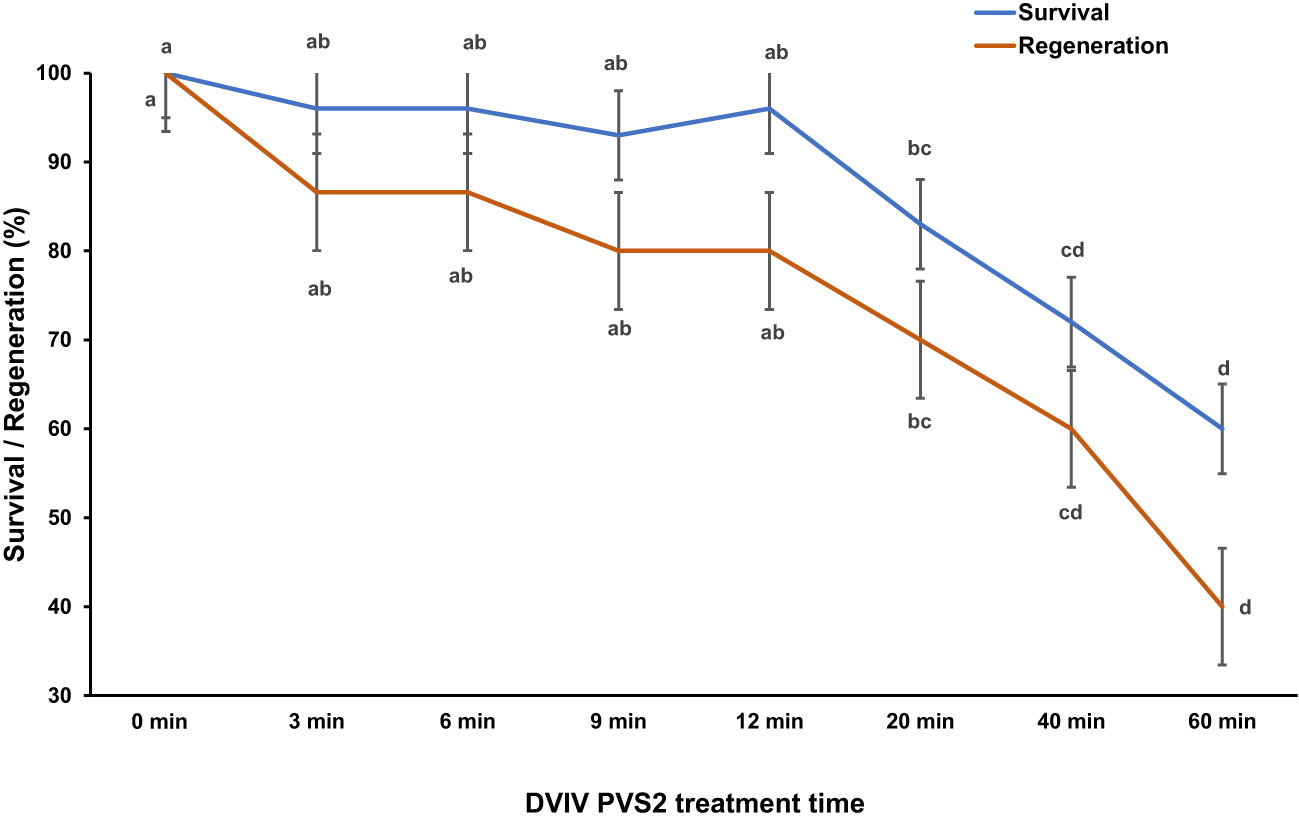
Figure 3 Mean percent survival and regeneration of Syzygium maire embryonic axes (EAs) following incubation in Plant Vitrification Solution 2 (PVS2) for 0 (control) –60 min using the droplet vacuum infiltration vitrification (DVIV) method and no liquid nitrogen exposure (–LN). Error bars are ± SD (all n=4). Means with the same letter do not differ significantly (5% LSD comparisons).
3.2 Experiment 2: EA survival and regeneration following metal-mesh vacuum infiltration vitrification cryopreservation
Survival percentages of non-cryopreserved EAs (–LN) decreased from c. 90% to 43%, 27% and 10% as the PVS2 exposure time was increase from 0 (control) min to 20, 40 and 60 min respectively (Figure 4). Freezing in LN (+LN) resulted in a further reduction in survival (Figure 4). Highest post-cryopreservation (+LN) survival (c. 19%) was noted for 20-min PVS2 treatment. Although EAs incubated in PVS2 for 20 and 40 min, remained green for up to 2 weeks after cryopreservation (+LN) (Figure 5), they subsequently succumbed to contamination, preventing the assessment of EA regeneration.
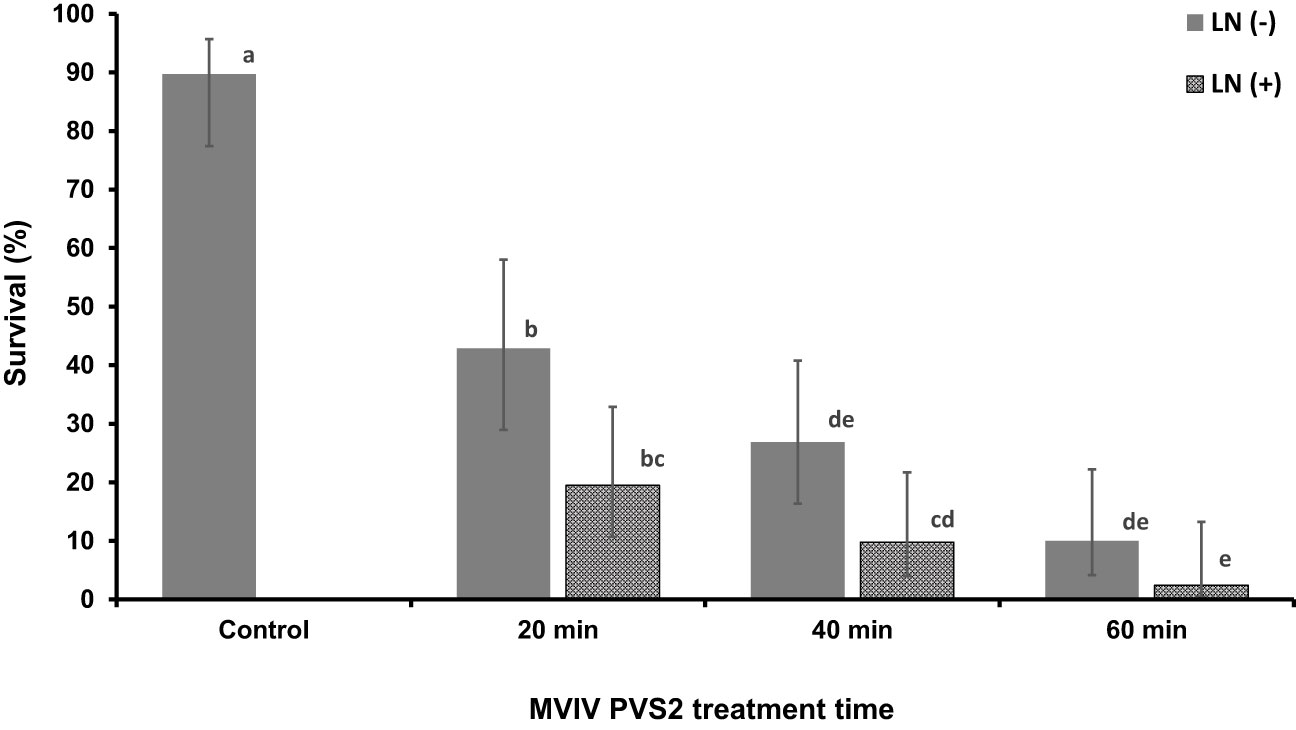
Figure 4 Survival of non-cryopreserved (–LN) and cryopreserved (+LN) Syzygium maire embryonic axis (EAs) using the metal-mesh vacuum infiltration vitrification (MVIV) following treatment with Plant Vitrification Solution 2 (PVS2) for 0 (control), 20, 40 or 60 mins (–LN) and after (+LN). Error bars are 95% confidence limits. Values followed by the same letter do not differ significantly (Fisher’s 5% LSD; n = 4).
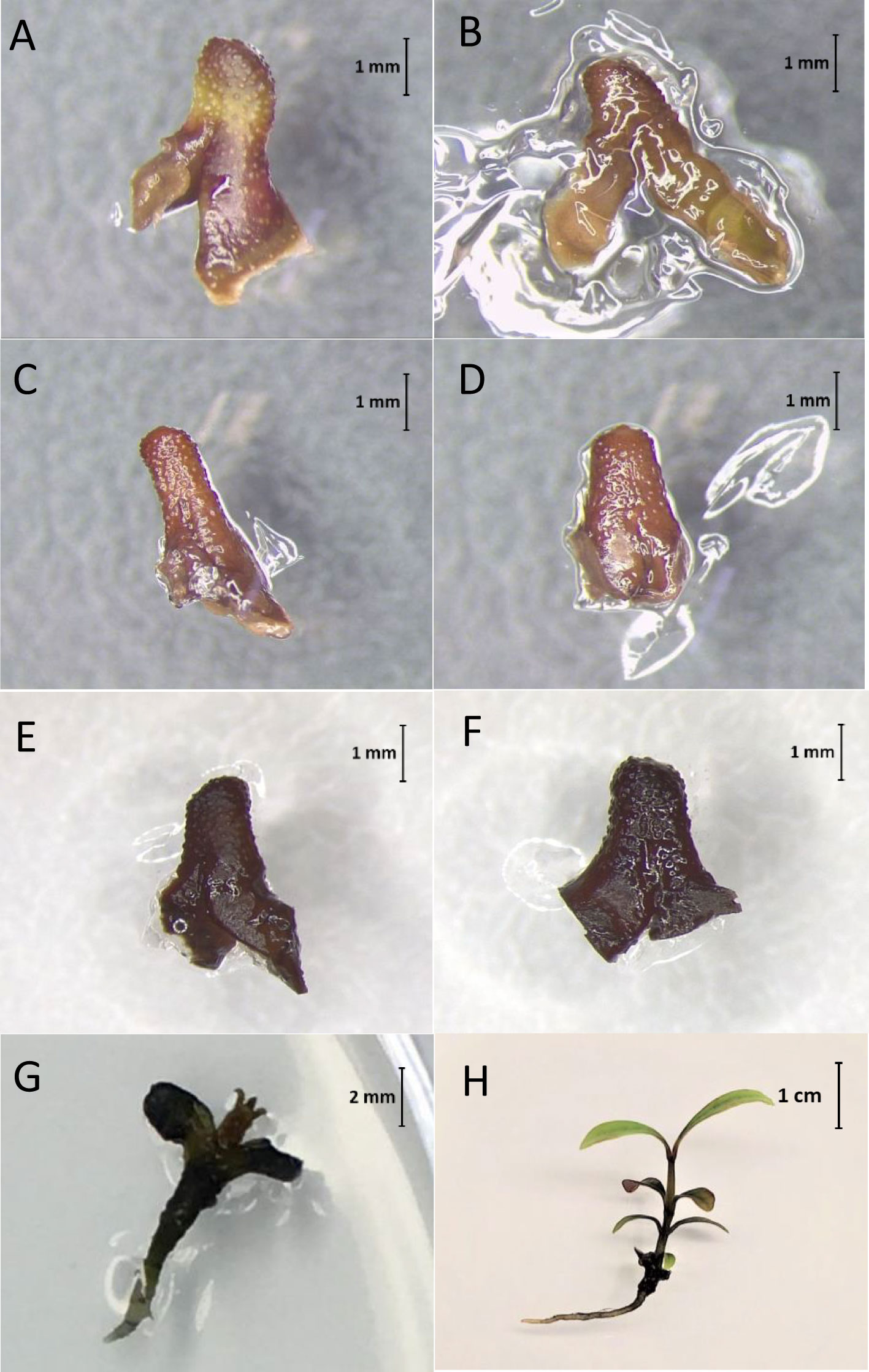
Figure 5 Syzygium maire embryonic axes (EAs) following cryopreservation in liquid nitrogen (+LN) using the metal-mesh vacuum infiltration vitrification (MVIV) method. EAs treated in Plant Vitrification Solution 2 for 0 (Control; A), 20 (B), 40 (C) or 60 min (D) remained green for 2 weeks post-cryopreservation (+LN). Whereas embryos treated with droplet vacuum infiltration vitrification (DVIV) for 20 min (E) and 60 min (F) in PVS2 turned brown within a few minutes following the cryoprotectant treatment and freezing in LN. Non-cryopreserved control EAs regenerating after 4 (G) and 8 (H) weeks of culture on growth medium respectively.
3.3 Differential scanning calorimetry
DSC thermograms were used to analyze critical cryopreservation parameters relating to onset/end temperatures for ice nucleation and melting events as well as enthalpies of ice crystallization and melting in the EAs subjected to DVIV and MVIV treatments. Substantial ice crystallization and melting transitions were observed on the DSC thermogram of untreated EAs (Figures 6A, B, 7A, B). For DVIV treatments, exothermic or ice crystallization events were detected during the cooling phase between −2°C and −23.9°C (Figure 6A; Table 1), while endothermic or ice melting events were observed in the warming phase between −1.7°C and 19.4°C (Figure 6B; Table 1). Similarly, in MVIV treatments, exothermic events were detected between −7°C and −30.5°C (Figure 7A; Table 1), while endothermic events were observed in the warming phase between −11.1°C and −18.3°C (Figure 7B; Table 1). The onset temperature for ice crystallization was lowered from −2°C in the control to −5°C in both DVIV–LS and MVIV–LS with the lowest ice crystallization onset temperature c. −15.5°C recorded for 40-min PVS2 exposure in both DVIV and MVIV (Table 1). The smallest ice melt enthalpies were recorded in EAs treated with DVIV (~1.8 mJ) and MVIV (~8.8 mJ), both for 40-min treatment, while no thermal activity was detected following 60 min for both DVIV and MVIV (Figures 6B, 7B; Table 1).
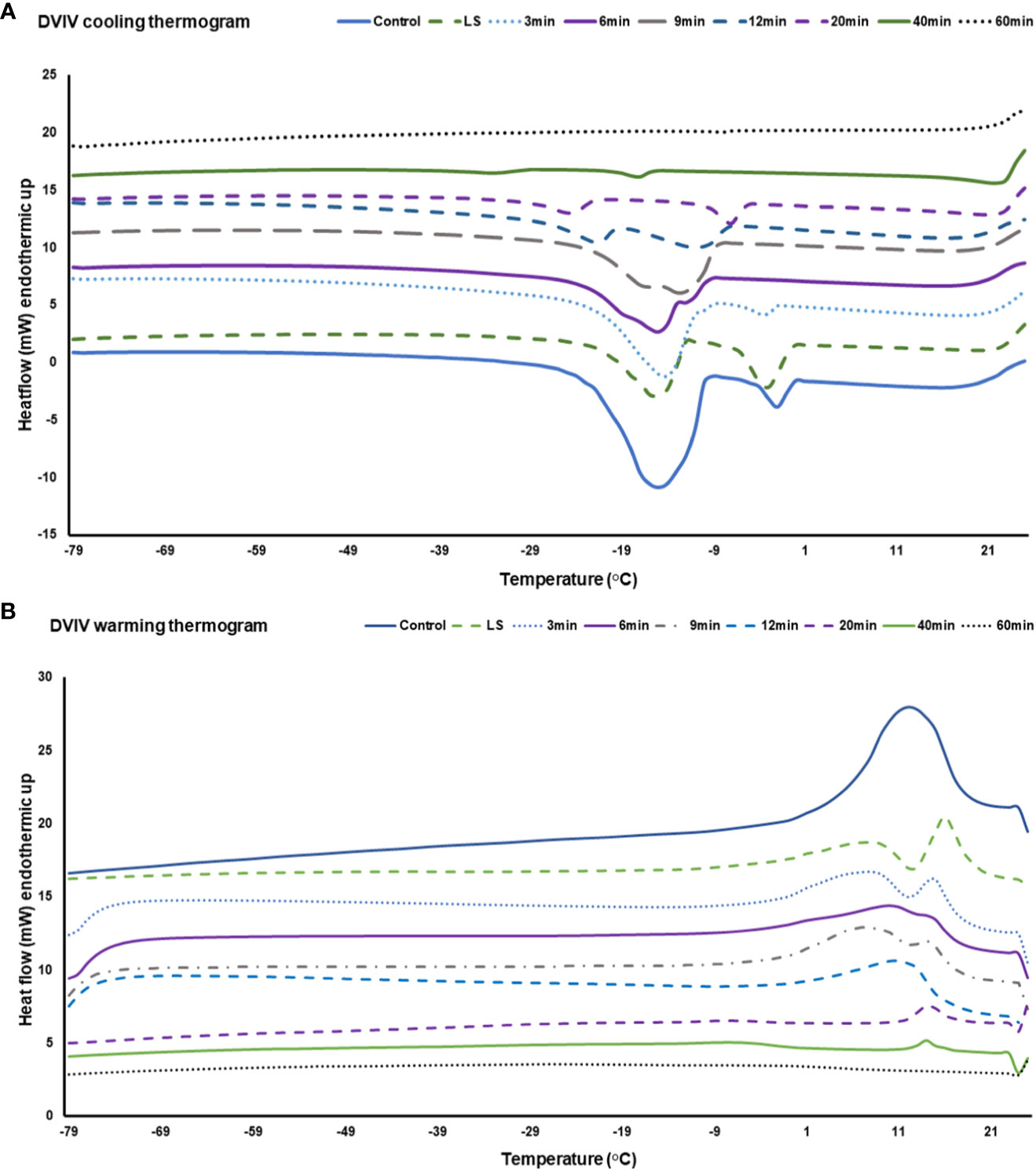
Figure 6 Representative Differential Scanning Calorimetry (DSC) cooling (A) and warming (B) thermograms for a Syzygium maire embryonic axis (EA) following droplet vacuum infiltration vitrification (DVIV). Treatments consisted of control (no treatment; solid dark blue line), 20 min incubation in loading solution (LS) (broken light green line) and 20 min LS solution followed by Plant Vitrification Solution 2 treatment for 3 min (blue dotted line), 6 min (solid purple line), 9 min (broken dark grey line), 12 min (broken blue line), 20 min (broken purple line), 40 min (solid green line) and 60 min (black dotted line). Samples were scanned at ±10°C min−1 from −100°C to 25°C. Thermogram is only showed for temperature range from −79°C to 25°C to highlight the significant thermal events.
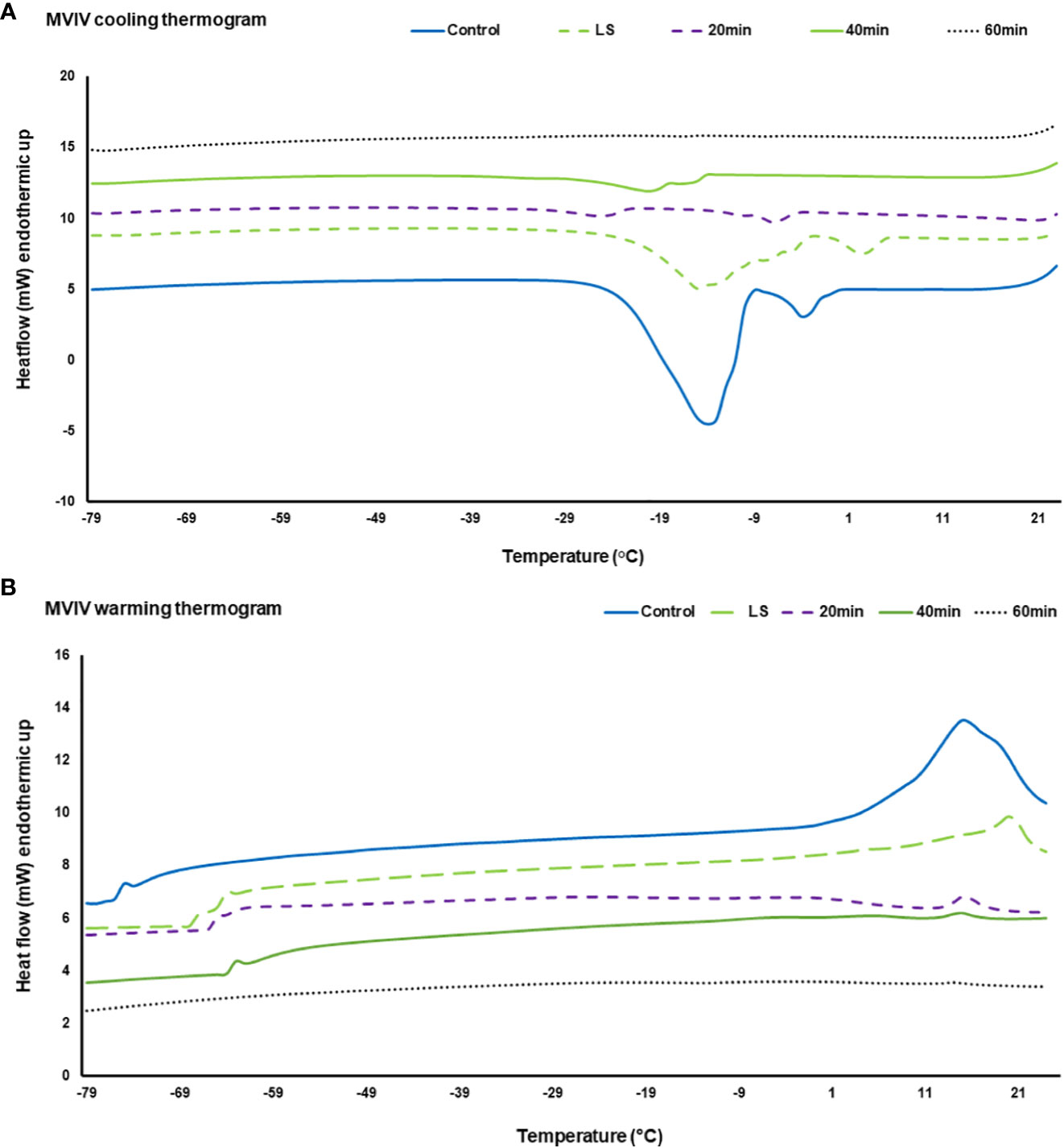
Figure 7 Representative Differential Scanning Calorimetry (DSC) cooling (A) and warming (B) thermograms for Syzygium maire embryonic axis (EA) following metal-mesh vacuum infiltration vitrification (MVIV). Treatments consisted of control (no treatment) (solid dark blue line), 20 min incubation in loading solution (LS) (broken light green line) and 20 min LS solution followed by Plant Vitrification Solution 2 treatment for 20 min (broken purple line), 40 min (solid green line) and 60 min (black dotted line). Samples were scanned at ±10°C min−1 from −100°C to 25°C. Thermogram is only showed for temperature range from −79°C to 25°C to highlight the significant thermal events.
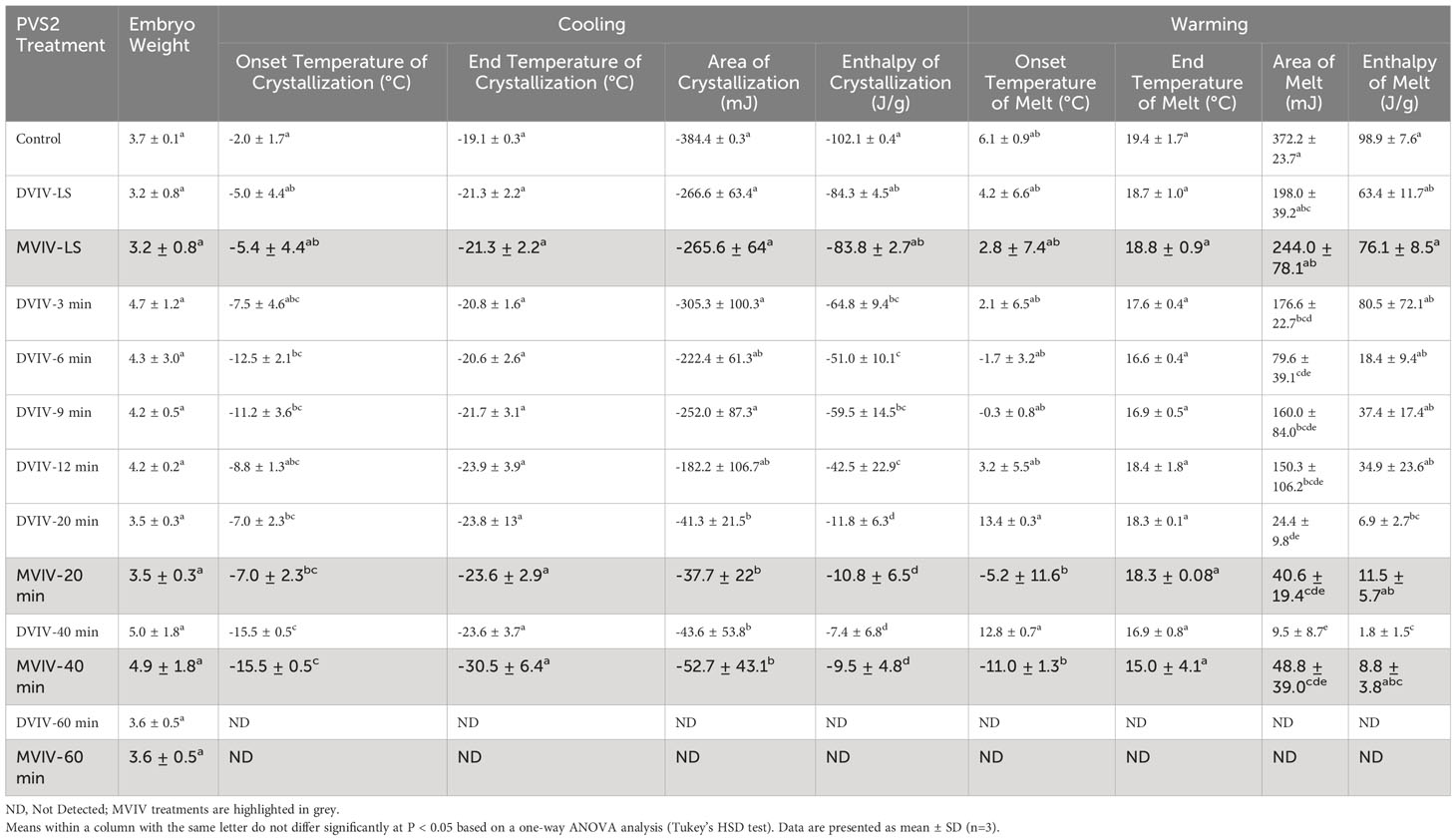
Table 1 Cooling and warming thermodynamic properties of Syzygium maire embryonic axes (EAs) following no treatment (control), submersion in loading solution (LS) for 20 min using the droplet vacuum infiltration vitrification (DVIV) or metal-mesh vacuum infiltration vitrification (MVIV) and EAs submersed in LS followed by Plant Vitrification Solution 2 (PVS2) treatment for 3, 6, 9, 12, 20, 40 or 60 min using DVIV and 20, 40 or 60 min using MVIV.
4 Discussion
There is no published protocol for the successful cryopreservation of zygotic embryos of any Syzygium species. This study compared the effectiveness of DVIV and a novel MVIV method for the cryopreservation of S. maire EAs by optimizing the PVS2 exposure time. Despite the risk of phytotoxicity, the application of cryoprotective agents such as PVS2 is vital for the development of a successful cryopreservation protocol for desiccation sensitive materials (Funnekotter et al., 2015). The survival and regeneration capacity of plant material exposed to concentrated vitrification solutions will depend not only on the type of plant tissue used but also on the composition of the vitrification solution, exposure time and temperature, and subsequent freezing (Xia et al., 2014; Bettoni et al., 2021). Droplet vitrification has been reported to increase explant post-cryopreservation survival through increased thermal conductivity resulting from the small amounts of cryoprotective agents used and the presence of aluminium foil (Le et al., 2019). Despite the advantage associated with droplet vitrification, effective vitrification is still dependent on extended incubation times in PVS2 that could lead to phytotoxicity (Volk et al., 2013; Nadarajan and Pritchard, 2014).
The application of vacuum infiltration vitrification (VIV) is postulated to eliminate the pressure differential of the air spaces found in live plant tissue, and facilitate a 10-fold reduction in PVS2 exposure time as reported for Arabidopsis thaliana, Carica papaya, Passiflora edulis and Laurus nobilis embryos (Nadarajan and Pritchard, 2014; Lukic et al., 2022). In our previous study, it was found that combining the droplet technique with VIV i.e., DVIV method, resulted in survival and regeneration of c. 95% of the EAs before cryopreservation for the PVS2 incubation times tested i.e., 3–12 min in S. maire EAs. This is in contrast to the EAs treated with a conventional vitrification technique, where survival and regeneration dropped to c. 50% and 40% after 60 and 90 min PVS2 treatments respectively (van der Walt et al., 2021a). In this study, extending the PVS2 incubation time for the DVIV cryopreservation, to 40 or 60 mins, showed a significant reduction on EA survival (~70% and 60% respectively) and regeneration before cryopreservation (~60% and 30% respectively) (Figure 3). However, none of the EAs survived exposure to LN following use of the DVIV method, irrespective of the PVS2 incubation time tested. Based on previously published records (Nadarajan and Pritchard, 2014; van der Walt et al., 2021a) and our observation, application of vacuum infiltration cryopreservation is recommended for highly recalcitrant and heterogeneous tissues such as S. maire embryos.
In comparison, EAs in DVIV have higher survival than EAs in MVIV for the same PVS2 treatment time without the LN exposure (–LN). This could be due to the stress during the surface sterilization procedure for the EAs in the MVIV method where the additional 70% ethanol treatment and the extended NaDCC treatment might have contributed to the low survival. In addition, for MVIV EAs, any residual PVS2 trapped inside the calcium-alginate encapsulation matrix covering part of the EAs after the washing treatment could be leading to low survival. In DVIV EAs (where the embryos do not have this alginate barrier), the PVS2 would have been washed off quickly during the unloading treatment. Hence, the EAs in MVIV may need a longer washing treatment to remove the cryoprotectants from the tissues, possibly washing for at least 30 min and by replacing with fresh washing solution in intervals as recommended by Ming-Hua and Sen-Rong (2010) and Kulus (2020). Furthermore, we noticed autoclaved PVS2 solution used in the DVIV had a lower pH (c. 3.2; (pH dropped during autoclaving) compared with filter sterilized PVS2 solution (pH 5.8) used in MVIV; this too could have resulted in low survival of EAs in MVIV prior to cryopreservation and needs further investigation, particularly if S. maire are acidophile in nature.
In contrast to DVIV, the EAs cryopreserved after PVS2 incubation for 20–60 min, using the novel MVIV method, survived (remained green and vigorous) for up to 2 weeks post-cryopreservation (+LN). This is a significant advancement as our previous trials did not result in green EAs after LN exposure. We postulate that regeneration of the embryos would have been possible if they had not succumbed to contamination. Overall, the MVIV technique is showing promising results compared with DVIV for the cryopreservation of S. maire EAs. There may be a range of reasons for this as discussed below.
The DVIV method requires direct handling of the individual EAs using forceps at each step of the protocol (i.e., during excision, transfer onto LS and PVS2 solutions, placement on aluminum foil strip, removal from WS and plating; Figure 2) as compared with the MVIV method where EAs are handled in batches and remain attached to the base of the metal wire mesh using the calcium-alginate matrix as adhesive from their excision until being plated in GM (Figure 2). It is likely that direct handling caused additional stress and mechanical damage to EAs, especially following freezing when the tissues are more likely to be fragile. In fact, the importance of sample handling in cryopreservation has led to the designing of specialized metal containers and cryo-plates (Marques et al., 2015; Salma and Engelmann, 2017). In addition, the thin film of calcium-alginate matrix that encapsulates the EAs in the MVIV method may protect explants that are sensitive to direct exposure to cryoprotectants (Tanaka et al., 2004) and from physical damage by forceps during manipulation of naked tissues (Benelli et al., 2013). We postulate that the incorporation of calcium-alginate encapsulation in the MVIV method not only minimized the damage of EAs but also facilitated the attachment of EAs to the metal-mesh to provide high thermal conductivity which is crucial during cryopreservation.
Due to the large size of zygotic embryos of recalcitrant seeds in general, their cryopreservation usually involves a reduction in explant size to facilitate cryoprotectant uptake (Nadarajan and Pritchard, 2014). Usually, this involves excision of the embryonic axis. However, such a procedure may initiate a burst of reactive oxygen species as reported previously in our research on S. maire embryos (van der Walt et al., 2021a), and in embryos of Castanea sativa (Roach et al., 2008), with further oxidative stress during subsequent desiccation and freezing. For this reason, the addition of free radical scavenging agents in the cryoprotectants is deemed crucial for survival of cryopreservation procedures (Mathew et al., 2019). In our study, the EAs exposed to the DVIV method turned brown (due to oxidation and phenolic compound production) during the PVS2 cryoprotection and subsequent LN freezing procedures. To mitigate this problem, we added 0.2 g/L AsA into the calcium-alginate matrix, loading solution, PVS2, unloading solution and regrowth media in MVIV. The EAs remained green and vigorous after the PVS2 and freezing steps and for a further 2 weeks. Hence, we postulate that the additional antioxidant protection provided by the AsA could have prevented EA oxidation and contributed to the survival of EAs following MVIV cryopreservation.
Establishing a suitable basal medium for supporting a full regeneration of the cryopreserved explants is an important aspect. In this study, we initially used MS basal medium (Greenway et al., 2012) for our experiment with DVIV. MS is widely used for regeneration in dicots and monocots however, there are instances in which MS is not considered optimum for growth as it contains high levels of nitrogen in nitrate and ammonium forms (Phillips and Garda, 2019). Compared with MS, WPM contains half of the ammonium and reduced nitrogen and is therefore often preferred for woody plant micropropagation. Interestingly, two Syzygium species were reported to have different preferences for their shoot proliferation and optimum growth in vitro e.g., S. cumini on WPM (Sirsat et al., 2019) compared with S. francissi on MS (Shatnawi et al., 2004). Using a modified MS in our previous studies resulted in 100% survival and regeneration in non-cryopreserved S. maire EAs (van der Walt et al., 2022). However, since no survival was recorded for the EAs after cryopreservation, we replaced MS macronutrients with WPM in MVIV cryopreservation solutions and growth medium in this study. Although WPM showed lower survival percentage compared with MS following incubation in PVS2 prior to cryopreservation (–LN), this medium showed EA survival following cryopreservation, which could be explained as follows. In vitrification-based methods, tolerance to freezing in LN is achieved through an osmotic process when tissues are placed in highly concentrated toxic vitrification solutions (Volk and Walters, 2006). In addition to the PVS2 treatment, LN exposure is known to destroy vacuolated and differentiated cells within the cryopreserved tissues resulting in fewer cells recovering after cryopreservation (Mathew et al., 2018; Bettoni et al., 2022; Wang et al., 2022). These recovering cells will be highly fragile and need to be handled with care and nurtured for further growth by optimizing both the growth media and the physical growth environment. We hypothesize that the WPM supplemented with zeatin, and copper sulphate, would have provided closer to optimum nutrition for growth of EAs after cryopreservation in this study compared with the MS medium with no added plant growth regulators.
The successful recovery of cryopreserved samples lies in finding the window of PVS2 treatment time that balances the toxicity of cryoprotectants against the need for dehydration (Panis et al., 2009; Kim et al., 2010). We used DSC thermal analysis to determine if the PVS2 incubation time, applied under vacuum, was effective in eliminating intracellular ice formation. It is evident that the chances of ice crystallization are higher when there is more capacity for the water molecules to come together and nucleate ice (Day et al., 2008) and this is highly influenced by the volume of the water content and solute concentration in the tissue. Dehydration of materials prior to cryopreservation will decrease the probability of the presence of water molecules sufficient quantities to aggregate and nucleate ice (Day et al., 2008). Additionally, the presence of micro-droplets of cryoprotective additive in cells and tissues will restrict the aggregation of water molecules and their capacity to form ice as the system becomes vitrified (Nadarajan and Pritchard, 2014).
In this study, substantial ice crystallization and melting transitions were observed in the thermograms of control EAs. The loading solution (LS) treatment alone decreased the ice crystallization enthalpy from c. 100 to 80 J/g in both DVIV and MVIV methods (Table 1). LS contains 0.4 M sucrose and 1.6 M glycerol. Sucrose acts as a non-penetrating/osmotic cryoprotectant whereas glycerol is a low penetrating/colligative cryoprotectant, although its permeability depends on tissue types (Nadarajan et al., 2008). Sucrose as a non-penetrating cryoprotectant has been reported to act as an osmotic dehydrant and reduce the amount of freezable water available therefore depressing the freezing point and impairing ice nucleation by restricting the mobility of water molecules (Fahy et al., 2004; Fuller, 2004; Volk and Walters, 2006; Benson, 2008b). However, it is worth noting that the LS treatment alone was not sufficient to eliminate lethal ice crystallization in these EAs. The area and enthalpies of ice crystallization and ice melt events were significantly reduced in EAs treated with PVS2 for 12 min (DVIV) and from 20–60 min irrespective of the method (DVIV vs MVIV) used (Table 1). PVS2 treatment of EAs for 60 min eliminated exothermic and endothermic peaks in both DVIV and MVIV indicating no presence of freezable water.
Interestingly, EAs treated with PVS2 for 20–40 min using MVIV method showed some post-cryopreservation survival despite the DSC thermal analysis indicating small ice melting peaks for these cryoprotected EAs. In these cases, water crystallization may have occurred in non-critical tissues, e.g., extracellularly, or ice formation might have been limited to those EAs that failed to tolerate cryopreservation, i.e., a population effect since the EAs were treated in batches in this method. A similar phenomenon of ice formation was observed in surviving shoot-tips of Parkia speciosa following encapsulation, 60-min PVS2 exposure and cryopreservation ({Nadarajan et al., 2008 #3}) and in Acer saccharinum embryonic axes (Wesley-Smith et al., 2014). The higher survival in DVIV prior to cryopreservation but nil survival after freezing in this study indicates that the balance has not been reached between optimum dehydration, cryoprotection and phytotoxicity of the PVS2 (Figure 8). As for the MVIV method, survival of EAs following cryopreservation peaked at 20-min PVS2 treatment (Figure 8), further investigation on narrowing down the PVS2 treatment to time around 20 ± 10 min would be valuable to optimize the window of PVS2 treatment time for cryopreservation of S. maire EAs. We also noted that for the same PVS2 treatment time, the DVIV treated EAs have smaller ice crystallization and melt enthalpies than MVIV treated EAs (Table 1). This is not surprising given the encapsulation matrix around EAs in the MVIV that might have an increased the tendency for minor localized ice nucleation events within a multi-component system (calcium-alginate matrix, embryo tissue and PVS2). Dumet et al. (2000) reported a glass destabilization event upon rewarming of 4 h desiccated alginate encapsulated Ribes ciliatum meristems, but the absence of glass transitions in the alginate bead and meristem when these were cooled or rewarmed separately. This was suggested to result from differences in the thermal properties between the alginate bead and the meristem. It was also postulated that a differential moisture gradient that exists between the tissue and the bead, could lead to glass destabilization upon rewarming (Nadarajan et al., 2008).
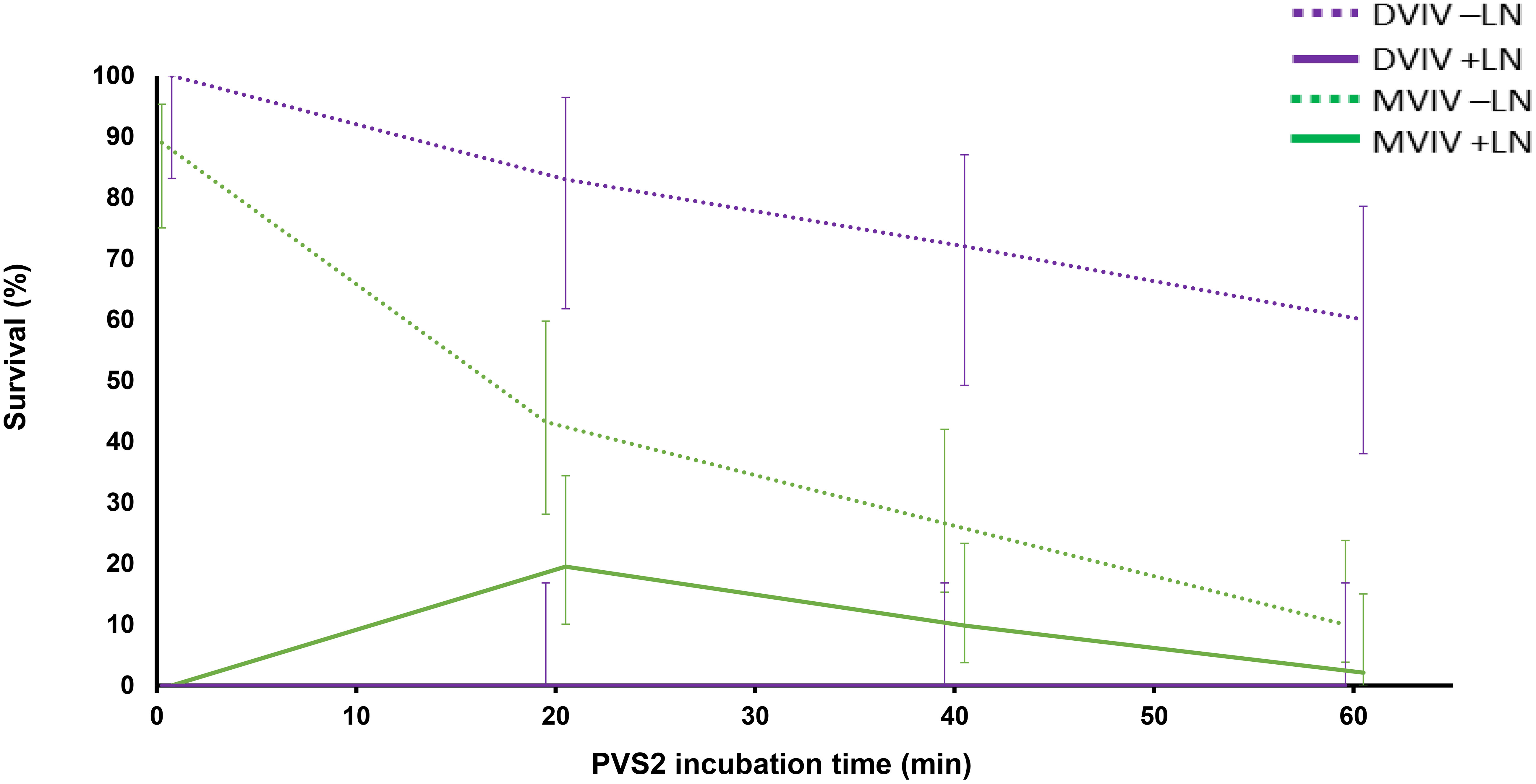
Figure 8 Mean percentage survival of non-cryopreserved (–LN) and cryopreserved (+LN) Syzygium maire embryonic axes following droplet vacuum infiltration vitrification (DVIV) and metal-mesh vacuum infiltration vitrification (MVIV) after treatment with PVS2 for 0 (control), 20, 40 or 60 mins. Error bars are 95% confidence intervals for a binomial distribution.
It should be noted that the design of this study meant seeds were collected in different seasons (albeit from the same population). Though results from previous studies using seed collected over multiple years from the same population did not reveal any noticeable difference in seed physiology of S. maire, seasonal variation cannot be ruled out as a factor contributing to lower EA survival (–LN) following incubation in PVS2 through the MVIV method. Intraspecific variation in seed physiology has been linked to other physiological attributes including seed development at the time of harvesting, parental plant characteristics, post-harvest seed handling, and climatic conditions between years (Hay and Probert, 1995).
5 Concluding remarks
This study investigated the use of PVS2 in the cryopreservation of S. maire EAs. We optimized PVS2 incubation times based on EA survival following PVS2 exposure. This was supported by thermal analysis using DSC. The novel MVIV technique resulted in EA survival following cryopreservation, although regeneration could not be verified due to contamination. These findings significantly advance our work in, and is to our knowledge the first published record of, embryo cryopreservation of a Syzygium species. Future activities to verify regeneration following cryopreservation using MVIV will be undertaken to increase embryo survival; faster cooling and warming rates will be investigated. The result from this study is expected to contribute to the knowledge base on cryopreservation of EAs of recalcitrant species, many of which are threatened with extinction due to climate change, plant pathogens, and habitat destruction.
Data availability statement
The raw data supporting the conclusions of this article will be made available by the authors, without undue reservation.
Author contributions
Kv: Conceptualization, Data curation, Formal Analysis, Funding acquisition, Investigation, Methodology, Resources, Software, Writing – original draft, Writing – review & editing. JN: Conceptualization, Data curation, Funding acquisition, Investigation, Methodology, Project administration, Resources, Software, Supervision, Writing – review & editing, Formal Analysis, Writing – original draft. LM: Data curation, Investigation, Methodology, Writing – review & editing. JB: Investigation, Writing – review & editing. JS: Investigation, Writing – review & editing.
Funding
The author(s) declare financial support was received for the research, authorship, and/or publication of this article. This research received funding from New Zealand’s Biological Heritage (NZBH) National Science Challenge Ngā Rākau Taketake (Challenge), authorized work under Manaaki Whenua Landcare Research Concession Number CA-31615-OTH. Financial support for the publication of the manuscript was provided by New Zealand’s Biological Heritage National Science Challenge.
Acknowledgments
Special thanks to the Te Rūnanga o Ngāti Ruanui, Te Kotahitanga o Te Atiawa and Te Korowai o Ngaruahine Iwi Authorities and Cliff Johns for allowing access to their takiwā from which the seed was collected. The authors would like to express their gratitude to Andrew Mullan and Belinda Diepenheim for laboratory management, tissue culture media preparation and recipe advice; Azadeh Esfandiari and Jasmine Divinagracia for internal review of the manuscript and Andrew McLachlan for statistical advice. The work was undertaken at the Palmerston North location of The New Zealand Institute for Plant and Food Research Limited and Ōtari Native Botanic Garden (Wellington City Council) with the findings of this work being communicated with representatives of Rangitāne o Manawatū and Port Nicholson Trust of Wellington.
Conflict of interest
Authors JN, LM, JB, and JS were employed by the company The New Zealand Institute for Plant and Food Research Limited.
The remaining author declares that the research was conducted in the absence of any commercial or financial relationships that could be construed as a potential conflict of interest.
Publisher’s note
All claims expressed in this article are solely those of the authors and do not necessarily represent those of their affiliated organizations, or those of the publisher, the editors and the reviewers. Any product that may be evaluated in this article, or claim that may be made by its manufacturer, is not guaranteed or endorsed by the publisher.
Supplementary material
The Supplementary Material for this article can be found online at: https://www.frontiersin.org/articles/10.3389/fcosc.2023.1269881/full#supplementary-material
Supplementary Figure 1 | A 80 mm diameter sterile stainless-steel mesh (A) with 1 mm2 pore size (B) used for metal-mesh vacuum infiltration vitrification (MVIV) cryopreservation experiments.
References
Anandalakshmi R., Sivakumar V., Warner R. R., Parimalam R., Vijayachandran S. N., Singh B. G. (2005). Seed storage studies on Syzygium cuminii. Kajian penyimpanan biji benih Syzygium cuminii. 17, 566–573.
Benelli C., De Carlo A., Engelmann F. (2013). Recent advances in the cryopreservation of shoot-derived germplasm of economically important fruit trees of Actinidia, Diospyros, Malus, Olea, Prunus, Pyrus and Vitis. Biotechnol. Adv. 31, 175–185. doi: 10.1016/j.biotechadv.2012.09.004
Benson E. (2008a). Cryopreservation of phytodiversity: A critical appraisal of theory & Practice. Crit. Rev. Plant Sci. 27, 141–219. doi: 10.1080/07352680802202034
Benson E. (2008b). “Cryopreservation theory,” in Plant Cryopreservation: A practical guide. Ed. REED B. M. (New York: Springer).
Benson E. E., Reed B. M., Brennan R. M., Clacher K. A., Ross D. R. (1996). Use of thermal analysis in the evaluation of cryopreservation protocols for Ribes nigrum L. germplasm. CryoLetters 17, 347–362.
Beresford R. M., Shuey L. S., Pegg G. S. (2020). Symptom development and latent period of Austropuccinia psidii (myrtle rust) in relation to host species, temperature, and ontogenic resistance. Plant Pathol. 69, 484–494. doi: 10.1111/ppa.13145
Berjak P., Pammenter N. W. (2008). From Avicennia to Zizania: Seed recalcitrance in perspective. Ann. Bot. 101, 213–228. doi: 10.1093/aob/mcm168
Bettoni J. C., Bonnart R., Volk G. M. (2021). Challenges in implementing plant shoot tip cryopreservation technologies. Plant Cell Tissue Organ Culture 144, 21–34. doi: 10.1007/s11240-020-01846-x
Bettoni J. C., Mathew L., Pathirana R., Wiedow C., Hunter D. A., Mclachlan A., et al. (2022). Eradication of Potato Virus S, Potato Virus A, and Potato Virus M From Infected in vitro-Grown Potato Shoots Using in vitro Therapies. Front. Plant Sci. 13, 878733. doi: 10.3389/fpls.2022.878733
Chua L. K., Lim C. L., Ling A. P. K., Chye S. M., Koh R. Y. (2019). Anticancer potential of Syzygium species: a review. Plant foods Hum. Nutr. (Dordrecht) 74, 18–27. doi: 10.1007/s11130-018-0704-z
Coelho de Araújo E., Vita Reis Mendonça A., Guerra Barroso D., De Alvarenga Ferreira D. (2008). Desiccation and storage effect over physiological quality of seeds of Syzygium jambolanum LAM. Rev. Ciencia Agronomica 39, 455–462.
Dawson J., Lucas R., Connor J., Brownsey P. J. (2011). New Zealand's native trees (Nelson, New Zealand: Craig Potton), 576.
Day J., Harding K., Nadarajan J., Benson E. E. (2008). “Cryopreservation: conservation of bioresources at ultra-low temperatures,” in Molecular biomethods handbook. Eds. Walker J. M., Rapley R. (United States, Totowa, New Jersey: Humana Press), 915–945.
Dumet D., Block W., Worland R., Reed B. M., Benson E. E. (2000). Profiling cryopreservation protocols for Ribes ciliatum using differential scanning calorimetry. CryoLett 21, 367–378.
Fahy G. M., Wowk B., Wu J., Paynter S. (2004). Improved vitrification solutions based on the predictability of vitrification solution toxicity. Cryobiology 48, 22–35. doi: 10.1016/j.cryobiol.2003.11.004
Fernandez A., Leon-Lobos P., Contreras S., Ovalle J. F., Naidoo S., van der Walt K., et al. (2023). The potential impacts of climate change on ex situ conservation options for recalcitrant-seeded species. Front. Forests Global Change 6. doi: 10.3389/ffgc.2023.1110431
Fuller B. J. (2004). Cryoprotectants: the essential antifreezes to protect life in the frozen state. CryoLett 25, 375–388. doi: 10.1201/9780203647073
Funnekotter B., Whiteley S. E., Turner S. R., Bunn E., Mancera R. L. (2015). Evaluation of the new vacuum infiltration vitrification (VIV) cryopreservation technique for native Australian plant shoot tips. CryoLetters 36, 104–113.
Goyal M. R., Ayeleso A. O. (2019). Bioactive compounds of medicinal plants : properties and potential for human health (Apple Academic Press).
Greenway M. B., Phillips I. C., Lloyd M. N., Hubstenberger J. F., Phillips G. C. (2012). A nutrient medium for diverse applications and tissue growth of plant species in vitro. In Vitro Cell. Dev. Biol. Plant 48, 403–410. doi: 10.1007/s11627-012-9452-1
Hay F. R., Probert R. J. (1995). Seed maturity and the effects of different drying conditions on desiccation tolerance and seed longevity in foxglove (Digitalis purpurea l.). Ann. Bot. 76, 639–647. doi: 10.1006/anbo.1995.1142
Kim H. H., Popova E. V., Yi J. Y., Cho G. T., Park S. U., Lee S. C., et al. (2010). Cryopreservation of hairy roots of Rubia akane (Nakai) using a droplet-vitrification procedure. Cryo Lett. 31, 473–484.
Kulus D. (2020). Shoot tip cryopreservation of Lamprocapnos spectabilis (L.) Fukuhara using different approaches and evaluation of stability on the molecular, biochemical, and plant architecture levels. Int. J. Mol. Med. Sci. 21, 3901. doi: 10.3390/ijms21113901
Le K.-C., Kim H.-H., Park S.-Y. (2019). Modification of the droplet-vitrification method of cryopreservation to enhance survival rates of adventitious roots of Panax ginseng. Horticult. Environment Biotechnol. 60, 501–510. doi: 10.1007/s13580-019-00150-8
Low Y. W., Rajaraman S., Tomlin C. M., Ahmad J. A., Ardi W. H., Armstrong K., et al. (2022). Genomic insights into rapid speciation within the world's largest tree genus Syzygium. Nat. Commun. 13, 5031–5031. doi: 10.1038/s41467-022-32637-x
Lukic M., Funnekotter B., Considine M. J., Bunn E., Mancera R. L. (2022). Evaluation of vacuum infiltration vitrification and cryo-mesh cryopreservation techniques with Arabidopsis thaliana shoot tips. Cryo Lett. 43, 328–333. doi: 10.54680/fr22610110712
Marques L. S., Bos-Mikich A., Godoy L. C., Silva L. A., Maschio D., Zhang T., et al. (2015). Viability of zebrafish (Danio rerio) ovarian follicles after vitrification in a metal container. Cryobiology 71, 367–373. doi: 10.1016/j.cryobiol.2015.09.004
Mathew L., Burritt D. J., McLachlan A., Pathirana R. (2019). Combined pre-treatments enhance antioxidant metabolism and improve survival of cryopreserved kiwifruit shoot tips. Plant Cell Tissue Organ Cult. 138, 193–205. doi: 10.1007/s11240-019-01617-3
Mathew L., Mclachlan A., Jibran R., Burritt D. J., Pathirana R. (2018). Cold, anitoxidant and osmotic pre-treatments maintain the structural integrity of meristematic cells and improve plant regeneration in cryopreserved kiwifruit shoot tips. Protoplasma 255, 1065–1077. doi: 10.1007/s00709-018-1215-3
McCown B., Lloyd G. (1981). Woody plant medium (WPM) - a mineral nutrient formulation for microculture of woody plant species. HortScience 16, 453.
Ming-Hua Y., Sen-Rong H. (2010). A simple cryopreservation protocol of Dioscorea bulbifera L. embryogenic calli by encapsulation-vitrification. Plant Cell Tissue Organ Cult. 101, 349–358. doi: 10.1007/s11240-010-9695-7
Murashige T., Skoog F. (1962). A revised medium for rapid growth and bioassays with tobacco tissue cultures. Physiol. Plantarum 15, 473–497. doi: 10.1111/j.1399-3054.1962.tb08052.x
Nadarajan J., Mansor M., Krishnapillay B., Staines H. J., Benson E. E., Harding K. (2008). Application of differential scanning calorimetry in developing cryopreservation strategies for Parkia speciosa, a tropical tree producing recalcitrant seeds. CryoLett 29, 95–110.
Nadarajan J., Pritchard H. W. (2014). Biophysical characteristics of successful oilseed embryo cryoprotection and cryopreservation using vacuum infiltration vitrification: An innovation in plant cell preservation. PloS One 9, e96169. doi: 10.1371/journal.pone.0096169
Nadarajan J., Walters C., Pritchard H. W., Ballesteros D., Colville L. (2023). Seed longevity—The evolution of knowledge and a conceptual framework. Plants 12, 471. doi: 10.3390/plants12030471
Nair N. K. (2017). The genus Syzygium : Syzygium cumini and other underutilized species (Boca Raton: CRC Press, Taylor & Francis Group).
Panis B., Piette B., Andre E., Van den Houwe I., Swennen R. (2009). Droplet vitrification: the first generic cryopreservation protocol for organized plant tissues? Acta Hortic. 908, 157–164. doi: 10.17660/ActaHortic.2011.908.17
Phillips G. C., Garda M. (2019). Plant tissue culture media and practices: an overview. In Vitro Cell. Dev. Biol. - Plant 55, 242–257. doi: 10.1007/s11627-019-09983-5
Powo (2023). Plants of the world online (Royal Botanic Gardens, Kew). Available at: http://plantsoftheworldonline.org/.
Reed B. M. (2008). Plant cryopreservation. [electronic resource] : a practical guide (Springer, New York: Springer).
Roach T., Ivanova M., Beckett R., Minibayeva F. V., Green I., Pritchard H. W., et al. (2008). An oxidative burst of superoxide in embryonic axes of recalcitrant sweet chestnut seeds as induced by excision and desiccation. Physiol. Plant 133, 131–139. doi: 10.1111/j.1399-3054.2007.00986.x
Sakai A. (2004). “Plant cryopreservation,” in Life in the frozen state. Eds. FULLER B., LANE N., BENSON E. E. (London, New York: CRC Press).
Sakai A., Kobayashi S., Oiyama I. (1990). Cryopreservation of nucellar cells of navel orange (Citrus sinensis Osb. var. brasiliensis Tanaka) by vitrification. Plant Cell Rep. 9, 30–33. doi: 10.1007/BF00232130
Salma M., Engelmann F. (2017). “Cryopreservation of date palm pro-embryonic masses using the D cryo-plate technique,” in Date Palm Biotechnology Protocols Volume II: Germplasm Conservation and Molecular Breeding. Eds. AL-KHAYRI J. M., JAIN S. M., JOHNSON D. V. (New York, NY: Springer New York).
Sharanya K. P., Kumar K. G. A., Nair P. S. (2022). Recalcitrant behaviour of the seeds of endangered Syzygium zeylanicum (L.) DC. J. Plant Growth Regulation 42, 2626–2636. doi: 10.1007/s00344-022-10732-z
Shatnawi M., Johnson K., Torpy F. (2004). In vitro propagation and cryostorage of Syzygium francissi (Myrtaceae) by the encapsulation-dehydration method. In Vitro Cell. Dev. Biol. - Plant 40, 403–407. doi: 10.1079/IVP2004551
Shü Z. H., Shiesh C. C., Lin H. L. (2011). Wax apple (Syzygium samarangense (Blume) Merr. and L.M. Perry) and related species. Postharvest Biol. Technol. Trop. Subtropical Fruits. 458–475. doi: 10.1533/9780857092618.458
Sirsat V., Rathod D., Gahukar S., Akhare A., Gawande V., Maske N., et al. (2019). In vitro Shoot Proliferation from Nodal Segments of Indian Blackberry (Syzygium cumini L.). Int. J. Curr. Microbiol. Appl. Sci. 8, 2464–2469. doi: 10.20546/ijcmas.2019.803.291
Sivasubramaniam K., Selvarani K. (2012). Viability and vigor of jamun (Syzygium cumini) seeds. Rev. Bras. Botanica 35, 397–400. doi: 10.1590/S0100-84042012000400012
Tanaka D., Niino T., Isuzugawa K., Hikage T., Uemura M. (2004). Cryopreservation of shoot apices of in-vitro grown gentian plants: Comparison of vitrifiction and encapsulation-vitrification protocols. CryoLetters 25, 167–176.
van der Walt K., Burritt D. J., Nadarajan J. (2022). Impacts of rapid desiccation on oxidative status, ultrastructure and physiological functions of Syzygium maire (Myrtaceae) zygotic embryos in preparation for cryopreservation. Plants (Basel) 11, 1056. doi: 10.3390/plants11081056
van der Walt K., Kemp P., Sofkova-Bobcheva S., Burritt D. J., Nadarajan J. (2021a). Evaluation of droplet-vitrification, vacuum infiltration vitrification and ecanpsulation-dehydration for the cryopreservation of Syzygium maire zygotic embryos. CryoLetters 42, 202–209.
van der Walt K., Kemp P., Sofkova-Bobcheva S., Burritt D. J., Nadarajan J. (2021b). Seed development, germination, and storage behaviour of Syzygium maire (Myrtaceae), a threatened endemic New Zealand tree. New Z. J. Bot. 59, 198–216. doi: 10.1080/0028825X.2020.1794911
Volk G. M., Henk B., Bonnart R., Shepherd A., Gross B. (2013). Plant shoot tip response to treatment with plant vitrification solution2. Int. Symposium Plant Cryopreservation 1039, 81–84. doi: 10.17660/ActaHortic.2014.1039.8
Volk G. M., Walters C. (2006). Plant vitrification solution 2 lowers water content and alters freezing behavior in shoot tips during cryoprotection. Cryobiology 52, 48–61. doi: 10.1016/j.cryobiol.2005.09.004
Wang M. R., Bi W. L., Bettoni J. C., Zhang D., Volk G. M., Wang Q. C. (2022). Shoot tip cryotherapy for plant pathogen eradication. Plant Pathol. 71, 1241–1254. doi: 10.1111/ppa.13565
Wesley-Smith J., Pammenter N., Berjak P., Walters C. (2001). The effects of two drying rates on the desiccation tolerance of embryonic axes of recalcitrant Jackfruit (Artocarpus heterophyllus Lamk.) seeds. Ann. Bot. 88, 653–664. doi: 10.1006/anbo.2001.1519
Wesley-Smith J., Berjak P., Pammenter N. W., Walters C. (2014). Intracellular ice and cell survival in cryo-exposed embryonic axes of recalcitrant seeds of Acer saccharinum: an ultrastructural study of factors affecting cell and ice structures. Ann. Bot. 113, 695–709. doi: 10.1093/aob/mct284
Keywords: differential scanning calorimetry, recalcitrant, Myrtaceae, myrtle rust, plant vitrification solution 2 (PVS2), vacuum infiltration vitrification (VIV)
Citation: van der Walt K, Nadarajan J, Mathew L, Bettoni JC and Souza JA (2023) Advances in cryopreservation of Syzygium maire (swamp maire, maire tawake) zygotic embryos, a critically endangered tree species endemic to New Zealand. Front. Conserv. Sci. 4:1269881. doi: 10.3389/fcosc.2023.1269881
Received: 31 July 2023; Accepted: 16 October 2023;
Published: 30 October 2023.
Edited by:
Anurag Dhyani, Jawaharlal Nehru Tropical Botanic Garden and Research Institute, IndiaReviewed by:
Lluvia Flores-Renteria, San Diego State University, United StatesR. Gowthami, Indian Council of Agricultural Research (ICAR), India
Copyright © 2023 van der Walt, Nadarajan, Mathew, Bettoni and Souza. This is an open-access article distributed under the terms of the Creative Commons Attribution License (CC BY). The use, distribution or reproduction in other forums is permitted, provided the original author(s) and the copyright owner(s) are credited and that the original publication in this journal is cited, in accordance with accepted academic practice. No use, distribution or reproduction is permitted which does not comply with these terms.
*Correspondence: Karin van der Walt, karin.vanderwalt@wcc.govt.nz; Jayanthi Nadarajan, Jayanthi.Nadarajan@plantandfood.co.nz
†These authors have contributed equally to this work and share first authorship